- 1School of Earth and Atmospheric Sciences, QUT Resilience Centre, Queensland University of Technology, Brisbane, QLD, Australia
- 2School of Biology and Environmental Sciences, Centre for Agriculture and the Bioeconomy, Queensland University of Technology, Brisbane, QLD, Australia
Digital optical and scanning electron microscopy (SEM) was used to study advance of incipient weathering of basaltic rock particles for two enhanced rock weathering (ERW) sites in Eastern Australia and three natural basalt sites (New Zealand and Eastern Australia). At the ERW sites, weathering of amended rock particles (up to 8 mm in diameter) induced a significant increase (1–1.5 pH unit) in shallow soil pH. After 14 months of incubation at the more recent site, 6–8 mm basalt particles showed dissolution of glass and olivine while pyroxene and plagioclase remained largely fresh. No secondary minerals were identified by SEM and high-quality X-ray diffraction analysis. Compared to the fresh, quarried basalt, the measured specific surface area (SSA) increased by 33%, suggesting microporosity formation via dissolution. At the >20 years ERW site, results were complex because of inconsistent application of basalt and greenschist facies ‘metabasalt’. Metamorphic rock particles showed negligible weathering while basalt particles could only be identified in the coarse (>6 mm) fraction of the shallowest (0–5 cm) soil. Within the finer particles in the deeper (5–10 cm) zone of elevated pH, potential ‘ghost’ basalt particles were identified by distribution patterns of ilmenite, suggesting near-complete basalt breakdown of mm-sized particles on decadal timescale. In variably weathered natural samples, dissolution also dominated over precipitation of secondary phases. Weathering progress in basaltic tephra deposited 150–600 years ago strongly depended on emplacement context. Tephra in free-draining >10 cm thick lapilli beds was only weakly altered, even where covered by soil, likely due to lacking connectivity of fracture networks for water access. In all studied incipiently weathered particles, we found sequential breakdown of glass and olivine before attack of pyroxene and plagioclase. Recognisable secondary mineral formation was minimal, but SSA increased over least weathered particles. The presence of interconnected glass and fracture networks apparently aids the rate of incipient weathering, increases microporosity and promotes particle disaggregation. This may permit application of relatively coarse (>5 mm) basalt for ERW but only for potential amendments where microscopic investigation and SSA have established suitable weathering fluid access networks.
1 Introduction
Despite gradually slowing increase in emission of carbon dioxide (CO2) from human activity, the concentration of CO2 in the atmosphere continues to increase at unabated pace (Friedlingstein et al., 2024). It is already at the point where intervention is urgent to stem the repercussions of a warming global climate. Many intervention methods have gained traction as possible CO2 remediation strategies over the past decade. They include direct air capture and storage, bio-energy carbon capture and storage, afforestation, soil organic carbon sequestration, enhanced rock weathering (ERW) and burial of biochar (Azar et al., 2010; Beuttler et al., 2019; Wang and Wang, 2019; Doelman et al., 2020; Mayer et al., 2022). Because of scalability and sustainability considerations, land-use competition, and water demand challenges, ERW has emerged as one promising CO2 removal solution with the potential to sequester 2.5 Gt CO2 yr−1 by 2,100 (Fajardy and Mac Dowell, 2017; Beerling et al., 2020; Doelman et al., 2020). It combines this potential with significant contributions to global food security, ocean acidification remediation, as well as co-benefits in terms of soil pH control, fertiliser use and soil restoration (Taylor et al., 2016; Beerling et al., 2018).
The ERW concept involves supplementing agricultural land with ground-up (finely crushed) rock, whose breakdown concomitantly fertilises the soil, sequesters carbon and increases pH. While other rock types and minerals have been proposed (notably olivine-rich dunite and wollastonite), consensus is converging on alkali-basalt being the most promising ERW candidate. It is fast-weathering, holds relatively high CO2 sequestration potential, is rich in several nutrients, releases limited toxic metals, and is abundantly available at the surface in most places globally (Beerling et al., 2018; Strefler et al., 2018; Kantzas et al., 2022). In view of these promising properties, many laboratory and mesocosm experiments have recently been undertaken [see Lu et al. (2024)] to quantify weathering rates, nutrient release and pH control from basalt in ERW settings. However, there are only very few studies that have systematically studied different basalt types as ERW amendments [e.g., Lewis et al. (2021)], and there are no multi-decadal field trials that could inform longer-term CO2 drawdown rates and full scalability. Laboratory studies and computer models of basalt breakdown have come to conflicting conclusions regarding weathering rates, weathering products, and the effectiveness of ERW as a whole (Gillman et al., 2002; Gordon and Brady, 2002; Shikazono et al., 2005; Dietzen et al., 2018; Schlesinger and Amundson, 2019; Wild et al., 2019; Vienne et al., 2022; Lunstrum et al., 2023; Iff et al., 2024). These diverging findings complicate efforts to monitor, report and verify (MRV) CO2 removal with ERW and are expected to be further complicated when quantifying the breakdown of basalt in full-scale natural field settings due to the complexities of soils, for example regarding the effects of microbiology [e.g., Lunstrum et al. (2023)], climate variability, and soil CO2 partial pressure.
One potential misconception in the current literature is the expectation that for a given basalt, there should be one single weathering rate that only depends on temperature, moisture, particle size and the presence of certain organic acids. This notion is counter-indicated by the soil development around active basaltic volcanoes, where basalt of rather homogenous composition can be observed to weather at vastly different rates depending on how it was emplaced. For example, 15–25 km away from the vent of Mt. Etna (Italy), the 122 B.C. ash and lapilli deposit is preserved as a 10–15 cm thick barely weathered layer. It is buried under >1 m of soil that has since accumulated from near-complete weathering of younger and thinner volcanic ash of the same composition (Coltelli et al., 1998). The thick layer of vesicle-rich scoria (frothy textured basalt lava ejected as fragments from the volcano) likely survived at depth due to its very high macro-porosity and permeability. This simple example shows that the nature of the particles and their emplacement context exert substantial control on the weathering of basalt in and on soil.
Theoretical considerations of ERW have highlighted the importance of determining the ‘ideal’ particle size and surface area of basalt amendment. Basalt is quarried and crushed and then ground to increase the surface area available to weathering fluids, with some ERW models suggesting that ideal particle sizes range from 10 to 500 μm (Kantzas et al., 2022; Beerling et al., 2018; Strefler et al., 2018), much smaller than the ‘fines’ typically available as byproducts at quarries (mostly <6 or <8 mm). Just how finely ground the particles need to be is very important because the energy consumed (and associated CO2 emitted) by the comminution process (grinding) jeopardises the carbon sequestration potential of ERW. For example, grinding rock down from <5 to <0.01 mm to increase the surface area is the final and most energy-intensive process in the ERW operation—requiring approximately 15–100 kWh tonne−1 (Renforth, 2012) besides adding logistical complexity to basalt procurement. Despite modelling by Kantzas et al. (2022) showing that particle sizes between 10 and 500 μm have little impact on CO2 removal over 10–20 years, several field ERW trials (Dupla et al., 2024; Guo et al., 2023; Smet et al., 2021) used particles smaller than 100 μm diameter (with p80 = 38.2–52 μm, 80% of the particles have a diameter equal to or less than the prescribed number). Other ERW studies (e.g., Beerling et al., 2024; Holden et al., 2024; Larkin et al., 2022; Skov et al., 2024) have used larger particles in field trials (p80 = 267–1,767 μm); however, there are very few published trials (if any) with particles as large as the ‘fines’ material from basalt quarries.
Reactive transport modelling by Kelland et al. (2020) and Lewis et al. (2021) supported larger particle sizes (125–1,250 μm and 267–1,767 μm, respectively) for effective CO2 removal (CDR) potential. These are still smaller than typical quarry fine byproducts (typically <6 or <8 mm). However, Israeli and Emmanuel (2018) and Israeli et al. (2021) presented models in which weathering reactions did not exclusively occur at the periphery of the ‘rock particle’ but also advanced along internal mineral grain boundaries within the particle. Accordingly, small constituent mineral grain size accelerates the physical breakdown of the rock particle through coupled chemical and mechanical alteration. If true, these findings would open the prospect of reducing the need for the energy-intensive grinding step by using much larger basalt particles for ERW. The present study was motivated by the paucity of data about the relationships between the rock particle size, basalt petrology, porosity, and geometry of weathering reaction progress. Rather than relying on chemical proxies for progress of weathering, we opted for optical and scanning electron microscopy (SEM) to study the incipient breakdown of primary phases in basalt within soils [e.g., Berner and Holdren (1979)]. We complemented the study with high-quality X-ray diffraction (XRD) and Brunauer-Emmet-Teller (BET) gas adsorption measurements of variably weathered basalt in natural and ERW settings.
2 Materials and methods
2.1 Samples
The rock samples for this study are from five locations and represent weathering of both naturally emplaced and artificially amended basalt (see Table 1 for summary sample descriptions). The first three sample sites are in eastern Australia and the others in New Zealand. Samples LS1/LS2 are weathering rinds developed on Cenozoic columnar basalt from the periphery of the Mount Warning shield volcano remnants in northernmost New South Wales (east coast of Australia). They occur naturally, covered under shallow soil and are locally extracted from pasture fields. They are sold commercially as ornaments and retaining wall construction material. The sampled basalt contains abundant plagioclase megacrysts up to 2 cm in diameter. These stand out within the 1–2 cm thick orange-beige weathering rinds. Thin sections were cut perpendicular to the column surface to expose a gradient from fresh to weathered material. The two other Australian sites were sampled for artificially amended basalt. The first suite was collected from Wodonga (southeast Queensland), a biodynamic macadamia nut farm fertilised with rock dust for at least 20 years, with family records suggesting that rock amendment was likely put down as fertiliser for more than 30 years. The site was selected as a long-term example of ‘farming with rocks’. The applied rock fines were sourced from multiple local quarries, including several that produce Cenozoic basalt but also others that sell greenschist facies ‘metabasalt’. Soil cores (5 cm diameter) were mechanically obtained with a push core rig to a depth of 0.7–1 m from the macadamia orchard and three control cores from adjacent woodland. The natural soil is classified as a Red Ferrosol, developed on highly weathered lateritic basalt (Isbell, 1996). The soil cores were sub-sectioned according to depth for analysis of grain size distribution, pH and other soil properties. The pH was measured using a 1:5 soil to deionised water suspension. For this study, the top two 5 cm core sections were prioritised as only they contained macroscopically visible particles that could potentially represent weathered amended rock fragments. Visible rock chips and 3–15 mm soil aggregates from 0 to 5 cm and 5–10 cm were made into thin sections. The second suite of amended basalt was taken from a different macadamia farm in Farnsfield (Wide Bay Burnett, Queensland). On this farm, the basalt amendment has only recently been trialled with a first application in 2022. Rock particles were extracted from the top 5 cm of soil, 14 months after the first application of 20 or 40 t ha−1. Sample FF_RD_02 was collected from the quarried basalt before a second application in 2023 as a ‘fresh’ sample comparison and was sieved for >2 mm particles.
Basaltic tephra samples were studied from fallout zones of two volcanoes in New Zealand’s North Island, chosen based on their composition (basalt) and eruption age and style. The first suite of samples erupted in the ~600 cal. yr. B.P. event of the Rangitoto volcano and can be found on both Rangitoto Island itself as well as locally on neighbouring Motutapu Island. The Rangitoto samples are variably weathered but mostly fresh lapilli from the Rangitoto cone. On Motutapu Island, basaltic lapilli could only be found in overturned roots of recently fallen trees in a gully on the northern side of the island. The second suite represents tephra from the June 10th, 1886 CE Plinian-style eruption of Mt. Tarawera near Rotorua. The volcano has a ~17 km fissure that produced both magmatic and phreatomagmatic deposits, including surge deposits as a result of erupting through current-day Lake Rotomahana in the southwest portion of the fissure (Rowe et al., 2021). Tephra beds of varying thicknesses and soil types were sampled in three locations.
2.2 Petrographic analysis—optical microscopy
Thin sections were made by first mounting rock particles onto a ceramic plate with epoxy, followed by lapping with 600-grit silicon carbide to create a flat surface that was then mounted onto a frosted glass slide. This was then cut to ½ mm thickness before lapping down to 35 μm and sequential polishing to a high-quality finish using Tegramin-30 and 1 μm diamond suspension. Polished thin sections of basaltic tephra and lava flow particles were scanned with a 40× magnification objective lens with a modified Olympus VS200 slide scanner, repurposed and adapted for petrological polarised light microscopy by Acevedo Zamora and Kamber (2023), housed at QUT’s Central Research Analytical Facility (CARF). The sections were scanned in three modalities: reflected light (RL), plane polarised light (PPL) in two orientations and cross-polarised light (XPL) in two orientations. Each illumination modality was saved as a separate image and simultaneously viewed in the Olympus microscope viewer software (OlyVIA).
2.3 Scanning electron microscopy
Selected thin sections were carbon coated and examined using a TESCAN TIMA Field Emission Scanning Electron Microscopy (SEM) at CARF, dedicated to microscopy of geological samples. Backscatter electron (BSE) and secondary electron (SE) images were acquired for regions of interest that had previously been identified on the optical images. The SE images provided textural and surface context, and BSE images presented in situ compositional information, particularly when coupled with the energy-dispersive X-ray spectroscopy (EDX) analysis. The SEM EDX system was deployed for spot analysis of chemical composition in search of alteration phases but proved to be insufficiently diagnostic.
2.4 Surface area and porosity
The Brunauer-Emmet-Teller (BET) gas adsorption method was used at CARF to compare the specific surface areas (SSA) (m2 g−1) of pairs of fresher and more weathered samples from most field locations (Brunauer et al., 1938). Particles (except FF_RD_02 and FF_35b) were gently hand-crushed using an agate mortar and pestle to fit sufficient mass into the sample tubes. The samples were degassed at 150°C (one sample at 350°C) for 24 h, then the SSA was measured using a Tristar II 3020 instrument with 99 adsorption points (except FF_RD_02–102 points). Both N2 and Ar were evaluated as adsorbates with sample-specific information provided in Supplementary Tables 1–4. Micropore measurements were modelled using the t-plot method and the Broekhoff and de Boer (1968) equation. Photographs of the samples were taken post-BET, and ImageJ and spreadsheet calculations were used for particle size analyses; see Supplementary Figures 1, 2.
2.5 X-ray diffraction (XRD) analysis
2.5.1 Bulk samples
Ten samples were selected for X-ray Diffraction (XRD) analysis. The rock particles were ground with a Rocklabs Ring Mill using a tungsten carbide bowl for 1–2 min to reduce the particle size. An aliquot of 2.4 ± 0.002 g of sample was spiked with 0.6 ± 0.002 g of corundum (Al2O3) and micronised for 6 min with 10 mL of ethanol in a McCrone micronising mill, reducing the particle size to ~<5 μm and dried in a 40°C oven for at least 4 h. A Co source Bruker D8 Advance diffractometer was used for analysis. Quantitative XRD phase analysis and Rietveld Refinement were performed using DIFFRAC.TOPAS. See section 4 in Supplementary material for full methods.
2.5.2 ‘Clay’ fraction samples
Four samples with suspected clay mineral content were further analysed for clay XRD characterisation. See Section 4 in Supplementary material for the full method.
3 Results
3.1 Summary of field observations
In the basalt column samples, the obvious contact between LS1 (weathered) and LS2 (fresher) shows the beginning of a multi-centimetre weathering rind (Figure 1A). LS1 has a distinctive clay-like and powdery texture and feel, contrasting with its dark-coloured LS2 equivalent, with large, visibly fractured plagioclase megacrysts (up to 10 mm) and an interconnected matrix of evenly distributed glass.
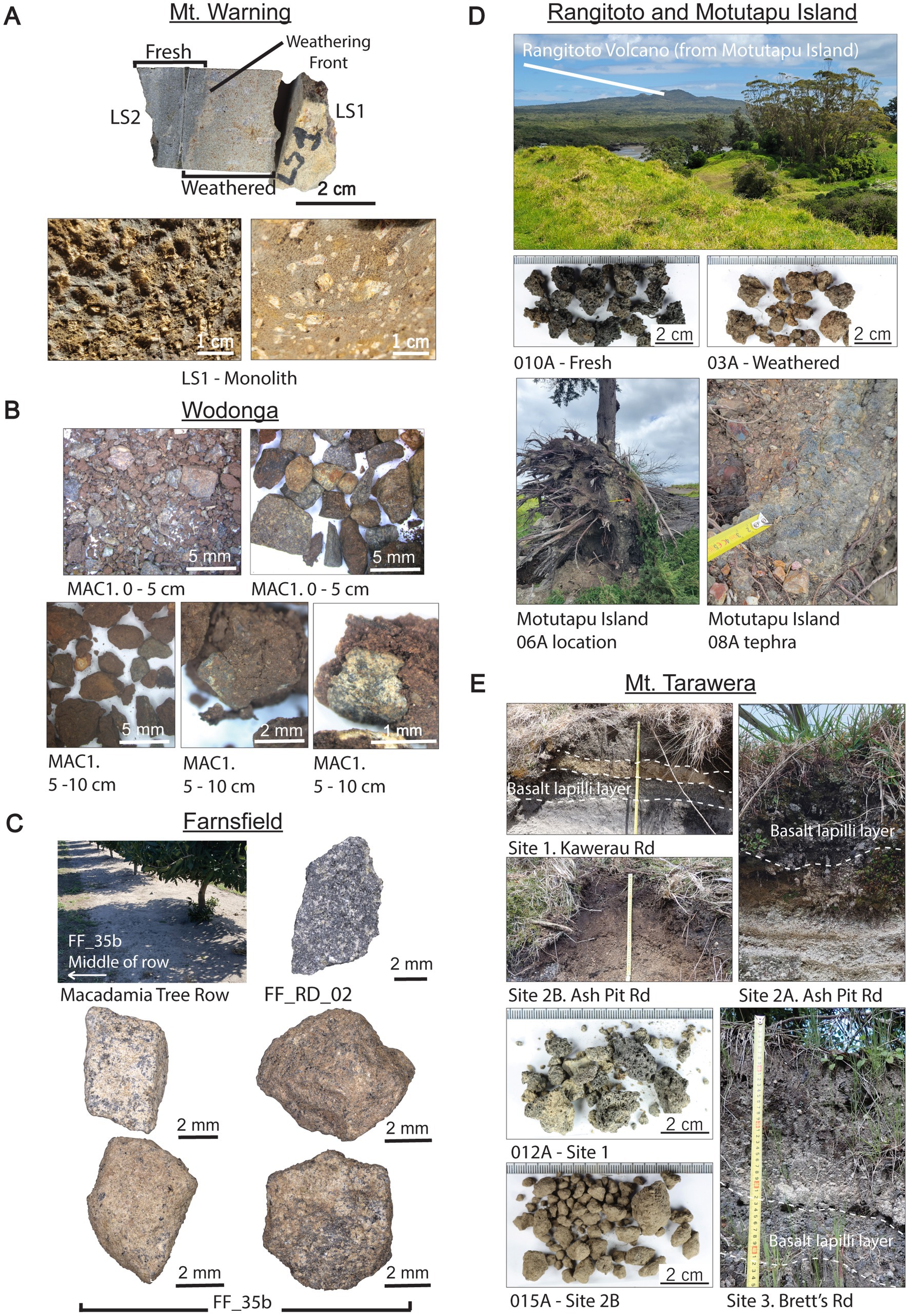
Figure 1. (A) LS1/LS2 hand sample from Mt. Warning. Plagioclase crystals protruding from weathered groundmass on LS1 weathering rind. Large plagioclase crystals distributed throughout LS1. (B) Rock particles of varied sizes, colours and morphologies extracted from MAC1 soil core. Small rock particles lodged in soil aggregates from deeper in MAC1 core. (C) Grass and mulch cover the row between tree lines, where FF_35b was extracted. Digital micrographs of unweathered basalt distributed at Farnsfield (FF_RD_02), and particles extracted from beneath mulch after 14 months (FF_35b). (D) Fresh and weathered tephra from Rangitoto Island. Overturned tree on Motutapu Island with tephra from Rangitoto Island embedded in the root system. (E) Photographs of the four tephra collection sites around Mt. Tarawera and a ‘fresh’ sample from a tephra bed at Site 1 (012A) and a ‘weathered’ tephra sample extracted from soil at Site 2B (015A).
The Wodonga site yielded almost no rock particles below 10 cm depth. The 5–10 cm core portion had disproportionally small particles remaining. Most of these were lodged in 1–10 mm diameter semi-coherent aggregates made of clay-sized soil, contrasting with the 0–5 cm depth interval, where larger (5–10 mm) unbound particles were prevalent (Figure 1B). Coherent particles from 10 cm and below were either a greenish rock or too friable and too few to be made into a thin section. Soil pH (Figure 2A) was found to increase towards the top of the core taken from the soil with ERW application, compared to the control by an average of 0.91 pH units, with the greatest distinction of 1.7 pH units at the 7.5 cm mark and a linear increase (r2 = 0.986) towards the surface from 25 to 7.5 cm average depth. The key increase was between 2.5 and 7.5 cm in MAC1, where the pH increased by 1 unit to 7.2 within a range of 5 cm. Despite ERW soil being more acidic at the shallowest depth (2.5 cm) than at 7.5 cm, its pH was still 0.85 units higher than the control.
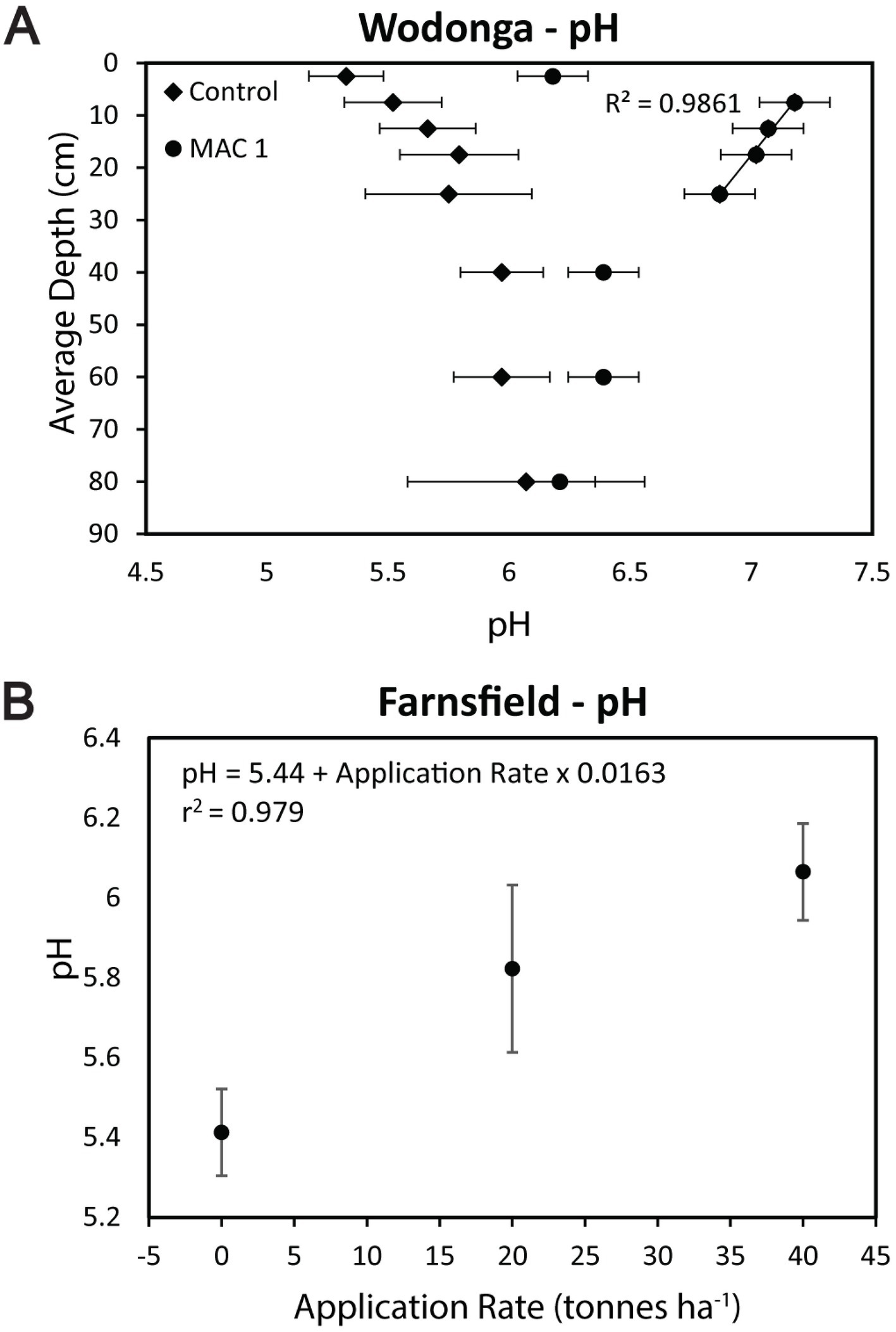
Figure 2. Changes in pH with basalt application. (A) Soil pH depth profiles. Wodonga MAC1 soil pH was higher than the control overall and increased linearly from ~25 to 7.5 cm. The biggest contrast to the control soil was from 2.5 cm to 7.5 cm depth, where the pH increased by one unit from 6.18 to 7.18. The control was more acidic towards the surface and 0.86 pH points lower than MAC1 at 2.5 cm. (B) pH measured from 0 to 5 cm depth in Farnsfield site soils increased linearly with basalt application rate by 0.65 pH points from 0 to 40 t ha−1 after 14 months from the initial application.
The Farnsfield site soils show a linear relationship (r2 = 0.979) between pH and basalt application rate (Figure 2B). The pH increased by an average of ~0.65 units from the control to the 40 tonnes per hectare basalt application. The particles collected directly under the mulch-free macadamia trees were still very fresh in appearance, dark grey coloured, and angular. Particles extracted from the inter-tree row mulch and in the top few centimetres of soil in the centre of a tree row were still quite angular, but some were encrusted with cream-coloured material (Figure 1C, FF_35b). See Supplementary Table 5 for pH data.
New Zealand’s field sites are natural volcanic systems where tephra formed beds of varying thicknesses. Rangitoto Island, a shield volcano, is comprised of a’a and pahoihoi lava fields and loose surface lapilli, variably covered with vegetation. Tephra also fell on the neighbouring non-volcanic Motutapu Island. Rangitoto-derived tephra does not form evident tephra beds on Motutapu, even on the proximal western shore, suggesting that it has been incorporated into the soil since the eruption 500–550 years ago. Fortuitously, large fallen trees recently exposed ~10–20 cm thick lapilli beds in a gully (Figure 1D).
Tarawera deposited thick (10–37 cm), clast-supported, permeable tephra beds, loosely held together by pervasive fine rootlets from the covering grass reaching through the tephra layer (Figure 1E). One site close to a vent section that erupted phreatomagmatically through Lake Rotomahana showed a different basalt tephra layer. While still distinct, the layer is bound by soil matrix and overlain by approximately 30 cm of tephra-bearing muddy soil, likely representing a vitric andisol from subsequent phreatomagmatic deposits (Rowe et al., 2021). Only larger particles (5–30 mm) were available for collection. Samples were collected from both the basaltic lapilli and muddy tephra layers (Figure 1E).
3.2 Optical microscopy
3.2.1 Summary observations by sample
3.2.1.1 Mt. Warning—LS1/LS2
The thin section photomicrograph of sample LS2/LS1 (Figure 3) shows a strong contrast between the darker, fresher side of the rock on the left and the brownish, weathered side on the right. The distinct contact between the two sides is a weathering front. It is best seen in PPL, where the colour contrast is most apparent (Figure 3A). At higher magnification in transmitted light, the busy arrangement of fine-grained minerals and glass makes it difficult to identify phases, such as olivine (Figure 3B, top and middle). By contrast, due to better visualisation of phase boundaries, olivine grains are clearly defined under RL (Figure 3B, bottom). RL also highlights a change in the reflectivity of the olivine on the weathered side (LS1) from the fresher side (LS2); a comparison is shown in Figure 4B. Similarly, while there appears to be no obvious textural change in the glass from LS2 to LS1, there is a change in reflectivity where the fresher glass appears in pronounced dark grey in RL, and the weathered glass is a light grey and more reflective (Figure 4A). The change in reflectivity in the olivine and glass from LS2 to LS1 suggests a possible compositional alteration. Since the less-weathered side of LS2 exhibits only minor incipient weathering, it will be referred to as ‘fresh’ hereafter.
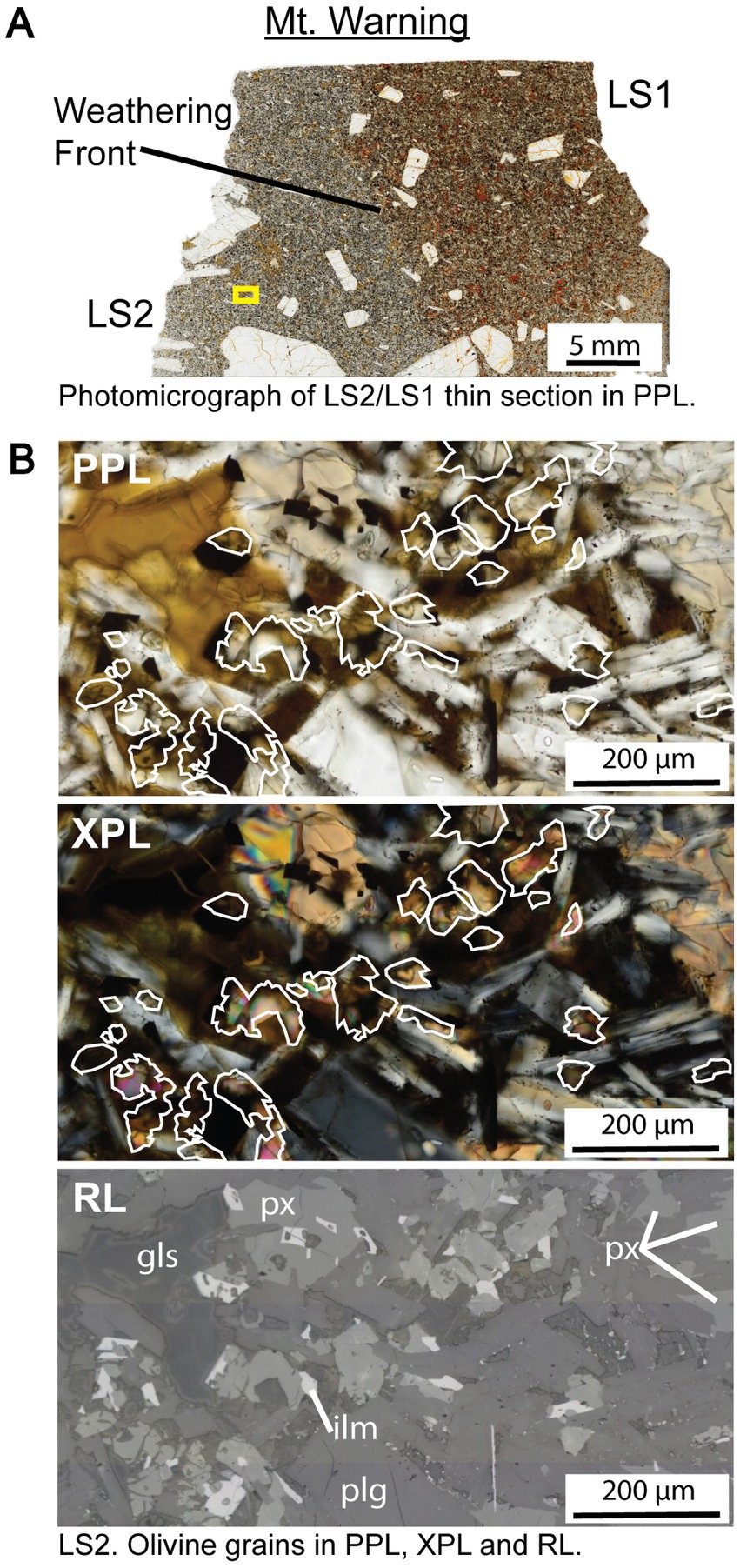
Figure 3. (A) Photomicrograph of LS2/LS1 in PPL. Porphyritic basalt with near vertical weathering front between the unweathered side on the left (LS2) and the weathered side on the right (LS1). (B) A comparison of three light illuminations demonstrates the difficulty of identifying olivine grains in transmitted light (both PPL and XPL). The detail region location is denoted with a yellow box on (A). The grain outlines (white) on the PPL (top) and XPL (middle) images highlight olivine locations on the surface of the thin section, identified in by light grey colour in RL (bottom). Px = pyroxene, ilm = ilmenite, plg = plagioclase, gls = glass.
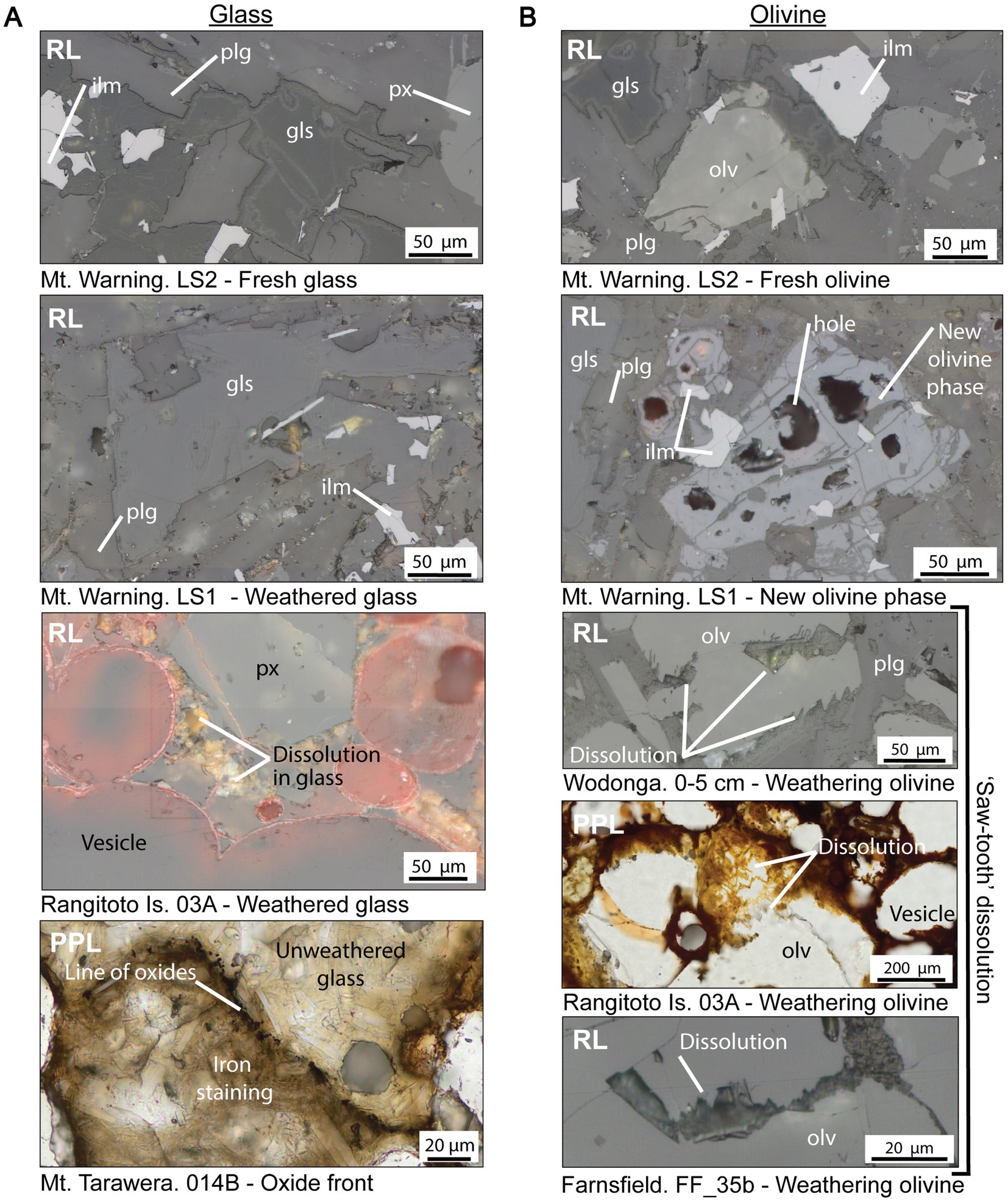
Figure 4. (A) LS2 ‘fresh’ glass (dark colour) rimmed with glass of a changed optical character (lighter-coloured edge). Weathered glass in LS1 appears lighter in reflected light, suggesting a compositional change. Deep dissolution cavities in sample 03A glass. Contrasted unweathered and stained glass in 014B from Mt. Tarawera, separated by a line of small oxides. (B) A comparison of unweathered olivine from LS2 with the new weathered phase of a different optical appearance in LS1. Three examples of weathered olivines from differing locations. Px = pyroxene, ilm = ilmenite, gls = glass, plg = plagioclase, olv = olivine, RL = reflected light, PPL = plane polarised light.
3.2.1.2 Wodonga
Most of the retrieved rock particles from 0 to 5 cm depth were not basalt, but metamorphic greenschist, of metabasalt and more quartzitic compositions (Figure 5A). A thin section scan of fresh ground rock from one quarry where recent ‘basalt’ was sourced also showed a dominance of greenschist (Figure 5A, bottom). Thus, despite the longevity of rock application at this site, the scientific value of the long-term experiment is compromised by the inconsistent application of rock types. Nevertheless, an interesting observation is that all scanned greenschist fragments in the soil aggregates are virtually unweathered between 0 and 5 cm, whereas the basalt has predominantly weathered to fragments consisting of surviving plagioclase, oxides and sometimes chlorite (Figure 5A). The few basalt particles that could be recovered as coherent fragments are holocrystalline dolerite without glass. They are porphyritic and overall coarser-grained (olivine = 0.2–1.2 mm, pyroxene = 0.1–0.4 mm, plagioclase = laths 0.05 mm wide to crystals up to 1.2 mm wide) than typical quarried basalt in the region. Preferential weathering of olivine is evident in all particles, initially along internal fractures or through pervasive alteration to ‘iddingsite’ throughout the grain.
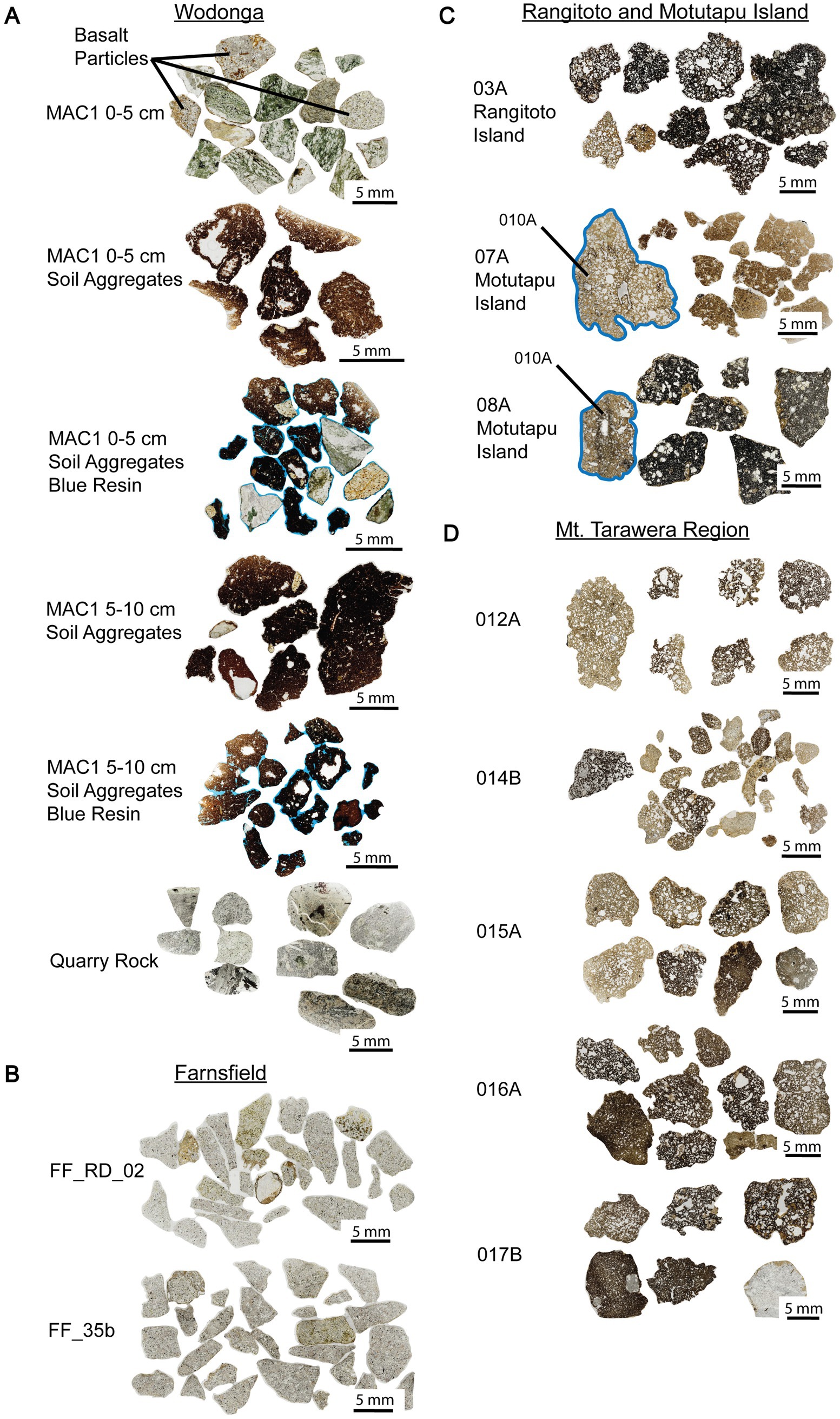
Figure 5. Thin section scans (40x magnification objective lens) of quarried basalt fine particles from Wodonga (A) and Farnsfield (B), and tephra particles from Rangitoto and Motutapu Islands (C) and the Mt. Tarawera region (D). Black lines connect the three basalt particles in the MAC1 0-5 cm sample from Wodonga (A); with remainder being greenschists. Alongside the 07A and 08A particles (C), one fresh tephra (blue outline) is shown from the Rangitoto volcano cone (sample 010A), for ease of comparison.
3.2.1.3 Farnsfield
Thin sections were made of >2 mm particles from a row of macadamia trees that received 40 t ha−1 of basalt 14 months prior (FF_35b) and the freshly quarried basalt (FF_RD_02) (Figure 5B). The sparseness of glass in FF_35b meant that incipient weathering of the most vulnerable phase was not obviously apparent when using optical microscopy. Under the 40× objective lens, the polished glass surface appeared predominantly smooth and homogenous and even under RL revealed no difference from fresh glass in FF_RD_02. However, in XPL, the glass in FF_35b is partially anisotropic, indicating possible internal stress or weathering (Figure 6A) (Rountree et al., 2009). This anisotropy was also seen in FF_RD_02 glass (Figure 6A). Given the limited evidence of weathering when using the optical microscope, FF_35b and FF_RD_02 were selected for SEM analysis.
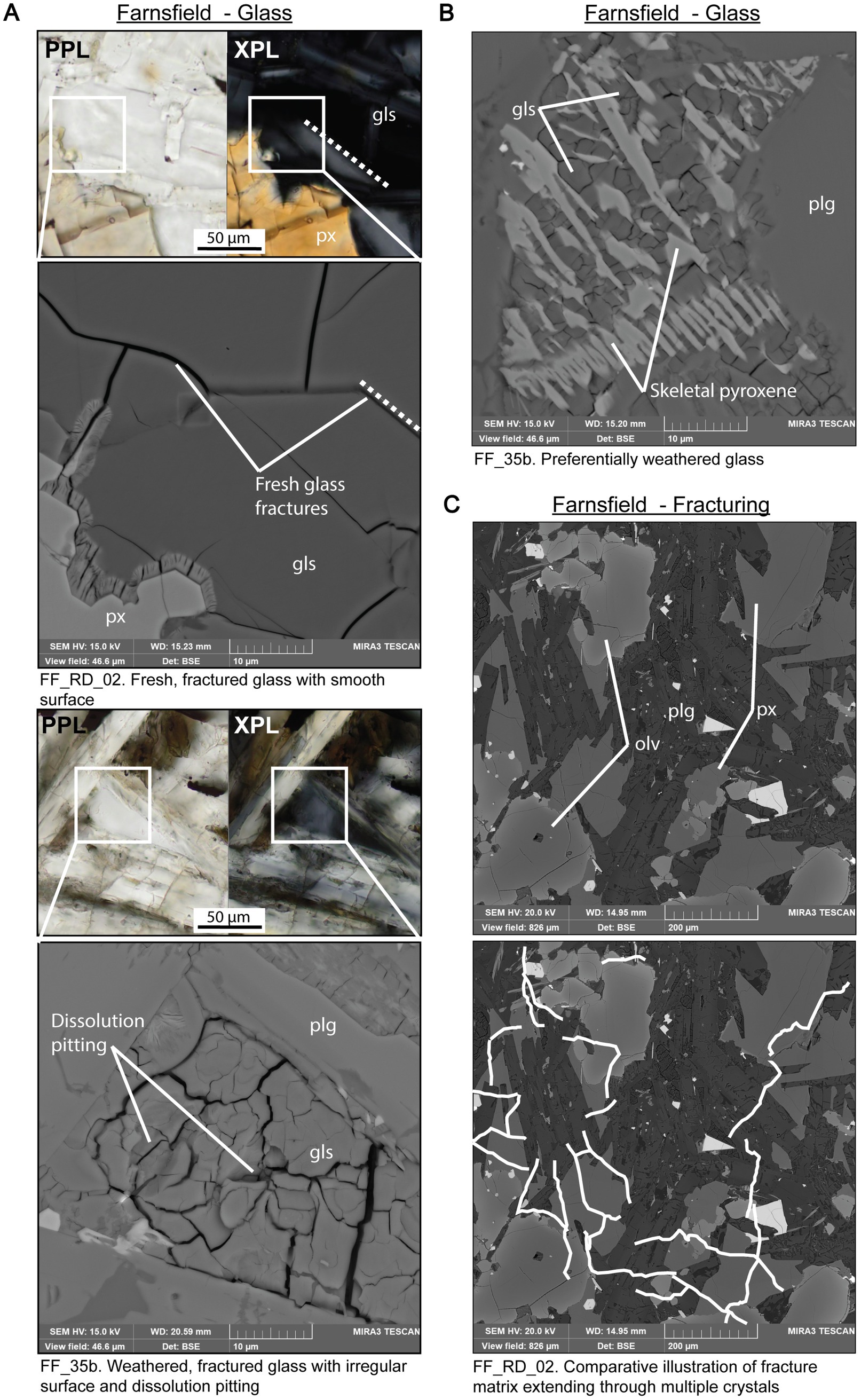
Figure 6. Optical and SEM BSE imagery of glass and glass fracturing in particles from Farnsfield. (A) A comparison of fresh glass in FF_RD_02 and weathered glass in FF_35b. The SEM BSE image area is shown as a white box on the PPL and XPL optical images. The XPL image (top right) shows some anisotropy (grey areas) within the otherwise isotropic black glass. This appears homogenous in PPL colour (light grey, top right). The BSE image shows that the XPL anisotropy is associated with a fracture in the glass, denoted with the white dotted line. Fresh glass surface is smooth. The XPL image of the weathered glass (middle right) shows mottled anisotropy, not caused by a single fracture. The weathered glass surface is highly cracked and shows dissolution (bottom panel). (B) SEM BSE image confirms that glass is preferentially weathered relative to skeletal pyroxene. (C) Two BSE images of a region on FF_RD_02. The bottom image is annotated for fractures that extend through multiple crystals. Px = pyroxene, gls = glass, plg = plagioclase, olv = olivine, PPL = plane polarised light, XPL = crossed polarised light.
3.2.1.4 Rangitoto and Motutapu Islands
Sample 010A is from the cone of the Rangitoto volcano (Figure 5C—particle in 07A and 08A). The sample is relatively fresh with some staining along fractures in the glass and around vesicle rims, likely caused by Fe oxidation. The primary minerals olivine, pyroxene and plagioclase appear unaffected by weathering processes in this fresh tephra sample. Sample 03A (Figure 5C) is from a shallow moss-covered vitric andisol on Rangitoto Island, approximately 330 m from the centre of the crater. Many of the particles are devitrified, making weathering observations of the glass difficult as it is black both in PPL and XPL. In RL, however, changes in the appearance of the devitrified glass are evident, but even under the 40× objective lens, the details are too fine to resolve. Olivine weathering is prominent throughout 03A (many have completely weathered), while the pyroxene and plagioclase crystals are fresher. Sample 08A (Figure 5C), collected from the tephra bed beneath the overturned trees on Motutapu Island, is also devitrified and shows no evidence to suggest weathering of the primary minerals.
3.2.1.5 Tarawera
Tarawera tephra particles in samples 012A, 014B, 015A, 016A, and 017B (Figure 5D) varied in vesicle shape and size, and the glass in the particles is often densely populated with microlites, predominantly pyroxene and plagioclase. The particles with large polylobate, irregular, and often connected vesicles resemble those in tephra from the Etna 122 B.C. Plinian eruption (Bamber et al., 2024). However, unlike the Etna 122 B.C. tephra, the Tarawera glass appears mostly unfractured. The glass shows some iron staining, particularly in 014B, similar to 010A from Rangitoto Island. These samples also include particles that have a high density of small, tightly packed vesicles with relatively low connectivity in 2D and occasional rhyolite tephra (e.g., sample 017B, Figure 5D). Olivine and pyroxene phenocrysts (macroscopically visible crystals) are small and rare as the particles are primarily comprised of a fine-grained microlite groundmass.
3.2.2 Summary observations by basalt constituent
3.2.2.1 Glass
Glass is present in all basalt samples in varying quantities. The tephra has a primarily glassy groundmass (Rangitoto and Tarawera), while the crystalline quarried basalt from lava flows, sills or dykes has interstitial glass (Wodonga, Farnsfield and LS1). Sample LS1 has a strongly interconnected matrix of glass. Aside from LS1, where RL highlighted a potential change in composition (Figure 4A), obvious weathering of the interstitial glass in other samples remained unresolvable using optical microscopy. The tephritic glass was most extensively weathered in 03A from Rangitoto Island, where sections of glass were dissolved and apparent secondary minerals precipitated (Figure 4A). All other tephra samples have no apparent secondary ‘clay mineral’ formation in glass, and optically, incipient weathering is only indicated by iron staining (Figure 4A, bottom). It is noted that devitrified basalt is not ideal for observing glass weathering because the glass is black in PPL, and because ubiquitous oxides conceal the glass texture. The development of fractures in volcanic glass was highly variable between sample locations. Tephra from Tarawera has virtually unfractured pristine glass, while glass in Rangitoto and Motutapu Island tephra is visibly fractured. Overall, despite the vulnerability of glass, particularly at low pH (Heřmanská et al., 2022), tephra that was collected from thick beds showed minor signs of incipient weathering in the glass, while particles extracted from soil showed the most alteration visible with the optical microscope.
3.2.2.2 Olivine
Olivine commonly alters to ‘iddingsite’, a secondary phase assemblage of uncertain mineralogical origin that forms either during low-temperature deuteric alteration or meteoric weathering (Delvigne et al., 1979). There is a possibility that some olivine in ERW amendment might already have decayed to iddingsite prior to application to the field. For this reason, our observations focused on olivines that had not undergone iddingitisation. In LS1, olivine visibly broke down to a new phase that is partly reflective. This has a bluish-grey colour in RL, distinct from white reflection of the oxide ilmenite (Figure 4B, middle). The secondary phase is co-located with deep brown staining in PPL, and contains cavities, representing obvious holes in the olivine pseudomorph. In all the other samples, olivine weathered differently. At one of the ERW sites (Wodonga), one quarry basalt particle out of the three (MAC1 0–5 cm) contained weathered olivine, while the other two had olivines dominated by iddingsite alteration. A common observation was ‘saw-tooth’ dissolution pitting in olivine, as described in Velbel (2009) and Delvigne et al. (1979). This was seen in particles from Wodonga, sample 03A from Rangitoto Island and sample FF_35b from Farnsfield (Figure 4B, bottom). Sample 03A was selected for SEM analysis as dissolution was most advanced. In the deeper sample from the Wodonga core (MAC1 5–10), olivine was no longer present in most of the particles.
3.2.2.3 Pyroxene
Pyroxene crystals in all the samples either appear strikingly ‘fresh’ compared to other phases or altered. Since most of the studied samples suffered only incipient weathering, the majority of pyroxenes are relatively unweathered with crisp grain boundaries and smooth polished surfaces. However, LS1 exhibits more advanced stages of weathering, and pyroxenes show signs of partial decay in transmitted light but no change in RL appearance. These pyroxene crystals, from deep within the sample, exposed irregularly shaped dissolution pits that reach into the grain centre with compromised and jagged grain boundaries (Figure 7A, top). However, the bulk of a surviving individual pyroxene grain still had a smooth polish and seemed structurally sound compared to the surrounding degraded structure of plagioclase remnants and the completely altered glass and olivine.
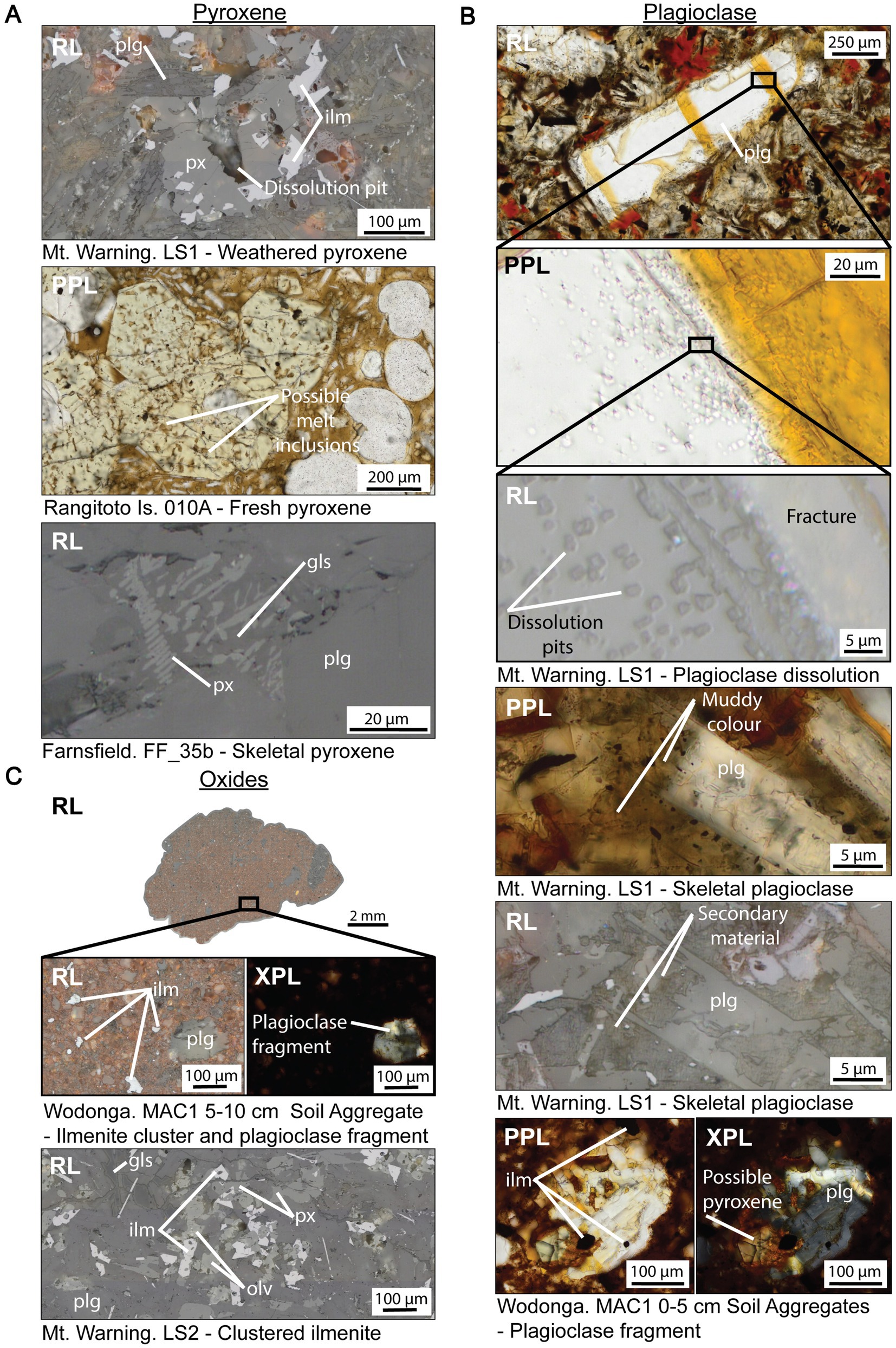
Figure 7. Optical microscope imagery. (A) Deep dissolution in LS1 pyroxene. Fresh, unweathered pyroxene in 010A with melt inclusions. Skeletal, unweathered pyroxene with more vulnerable, weathered glass in between pyroxene frame. (B) Plagioclase megacryst in LS1 with dissolution pitting concentrated along fractures. Magnified PPL and RL images confirm pitting, even in a large plagioclase phenocryst. PPL and RL images of skeletal plagioclase in LS1 where the secondary mineral seen in RL appears muddy-coloured and decayed in PPL. A surviving fragment of plagioclase and ilmenite with possible partially weathered pyroxene in soil aggregate from Wodonga. (C) RL image of a soil aggregate from Wodonga where clustered, liberated ilmenite are associated with a small plagioclase fragment, but no recognisable ‘basalt’ particles remain. A RL image of LS2 from Mt. Warning demonstrates ilmenite clustering around olivine and pyroxene phenocrysts. Px = pyroxene, ilm = ilmenite, gls = glass, plg = plagioclase, olv = olivine, RL = reflected light, PPL = plane polarised light, XPL = crossed polarised light.
In the other samples, pyroxene weathering was difficult to evaluate because it is unclear which of the optical features could be inherited from magmatic or post-magmatic processes. For example, the pyroxenes in the Rangitoto Island samples are rich in inclusions. These are likely melt inclusions and not dissolution or alteration features (Figure 7A, middle). Similarly, some pyroxene crystals in FF_35b and FF_RD_02 exist in skeletal morphology (Figure 7A, bottom). This is best expressed by a small pyroxene grain population and is also present in the control sample. The skeletal shape is thus unlikely a weathering feature but could have formed from pre-eruptive oversaturation of pyroxene in the magma.
3.2.2.4 Plagioclase
Out of the primary minerals in basalt, the plagioclase solid solution between albite (NaAlSi3O8) and anorthite (CaAl2Si2O8) shows the greatest variability in resistivity to weathering, as was already noted by Goldich (1938). This is reflected in the much higher thermodynamic dissolution rate for anorthite (9.8 × 104 mol m−2 s−1) than albite (0.7 mol m−2 s−1, Heřmanská et al., 2022). In most basalts, plagioclase shows a range of compositions, even within a rock fragment or single phenocryst, typically ranging from 30 to 80% anorthite. This places such feldspar at similar weatherability to clinopyroxene, depending on its exact composition (Manning, 2025). The plagioclase crystals in LS1 show evidence of decay in both the megacryst and groundmass grain size populations. One megacryst displays dissolution pits of prismatic shape that are clustered around fractures rather than just occurring at the sample surface (Figure 7B). These pits were initially observed using the 40× objective lens and were confirmed at 2.5× higher magnification (Figure 7B, middle). The imaged area was also selected for SEM analysis. Some of the groundmass plagioclase grains in LS1 have a skeletal morphology where partially formed crystals appeared infilled with secondary material with a muddy-coloured hue in PPL and poor reflectivity, making them unresolvable in RL (Figure 7B, middle). This degradation can be seen throughout the slide but at a much higher concentration on the more weathered sample side. At Wodonga, the plagioclase crystals are either fine laths or more equant crystals forming a poikilitic texture where the plagioclase encases pyroxene and olivine grains. Embedded in soil aggregates from MAC1 5–10 cm depth, are clasts of equant plagioclase crystals with small yet virtually completely weathered pyroxene leftovers that denote them as likely remains of original basalt particles (Figure 7B, bottom). There is also a noticeable lack of surviving particles that originally had lath-shaped plagioclase crystals.
3.2.2.5 Oxides
Ilmenite [(Fe,Ti)2O3] occurs in low abundance as an accessory mineral in basalt and is highly resistant to weathering. The control soil cores at Wodonga confirmed that the shallow soil contained no ilmenite prior to any ERW application, meaning that any ilmenite contained in the MAC1 core soil aggregates originated from the rock amendment. The highly reflective ilmenite grains seen dispersed throughout the MAC1 thin sections are best appreciated using RL and can be further emphasised by adjusting the contrast settings of the RL image. Ilmenite does not appear randomly in the soil aggregate particles but is sometimes clustered into areas of larger ilmenite grains compared to fine-grained, more evenly distributed ‘background’ ilmenite. These clusters of larger ilmenite could delineate ‘ghost’ basalt particles that had once existed but in which most silicate phases have since disintegrated, leaving behind unweathered, liberated ilmenite grains and occasionally surviving plagioclase fragments (Figure 7C, top). Clustering is also seen in LS1/LS2 where ilmenite grains are associated with pyroxene or olivine (Figure 7C, bottom). The new reflective phase of the altered olivine in LS1 reflects light like an oxide, though not as bright as ilmenite. This new phase was analysed with the SEM spectrum analyser for compositional changes, see Supplementary Figure 3.
Primary oxides, including ilmenite, are uncommon in the samples from Tarawera, and even more so from those of Rangitoto, but basalt from both volcanoes contains very small oxides associated with staining of the glass. Their detailed petrography is too fine to resolve with the 40× lens. To investigate the staining-oxide relationship more fully, sample 014B from Tarawera was selected for SEM analysis.
3.2.3 Scanning electron microscopy
3.2.3.1 LS1
The SEM was deployed to weathered target areas identified by microscope analysis, to further understand textural and compositional changes between the fresh and weathered sides. The glass is vastly different between LS1 and LS2. The BSE image in Figure 8A (top) shows that the fresh glass is rimmed with a material of higher average atomic number than the interior, with minor fractures but a smooth surface. Conversely, the weathered glass likely has a more mafic composition similar to the rim and also appears to have a smooth surface, though fracturing is more prevalent (Figure 8A, bottom). Normalised EDX spectral analyses of several glass patches show that the rims of the fresh glass yielded slightly increased Mg (~0.5 wt%) and decreased Si (~3 wt%), compared to interiors, while the weathered glass interiors had increased in Fe (~16 wt%) and Al (~1 wt%) and decreased in Mg (~3 wt%) and Si (~10 wt%), compared to the fresh glass interiors. This compositional change is not apparent from surface observations. Secondary electron (SE) images in Figure 8A (middle) show no discernible surface degradation from fresh to weathered glass, and the slight etching on the surface present in both glasses is likely a thin section polishing artefact.
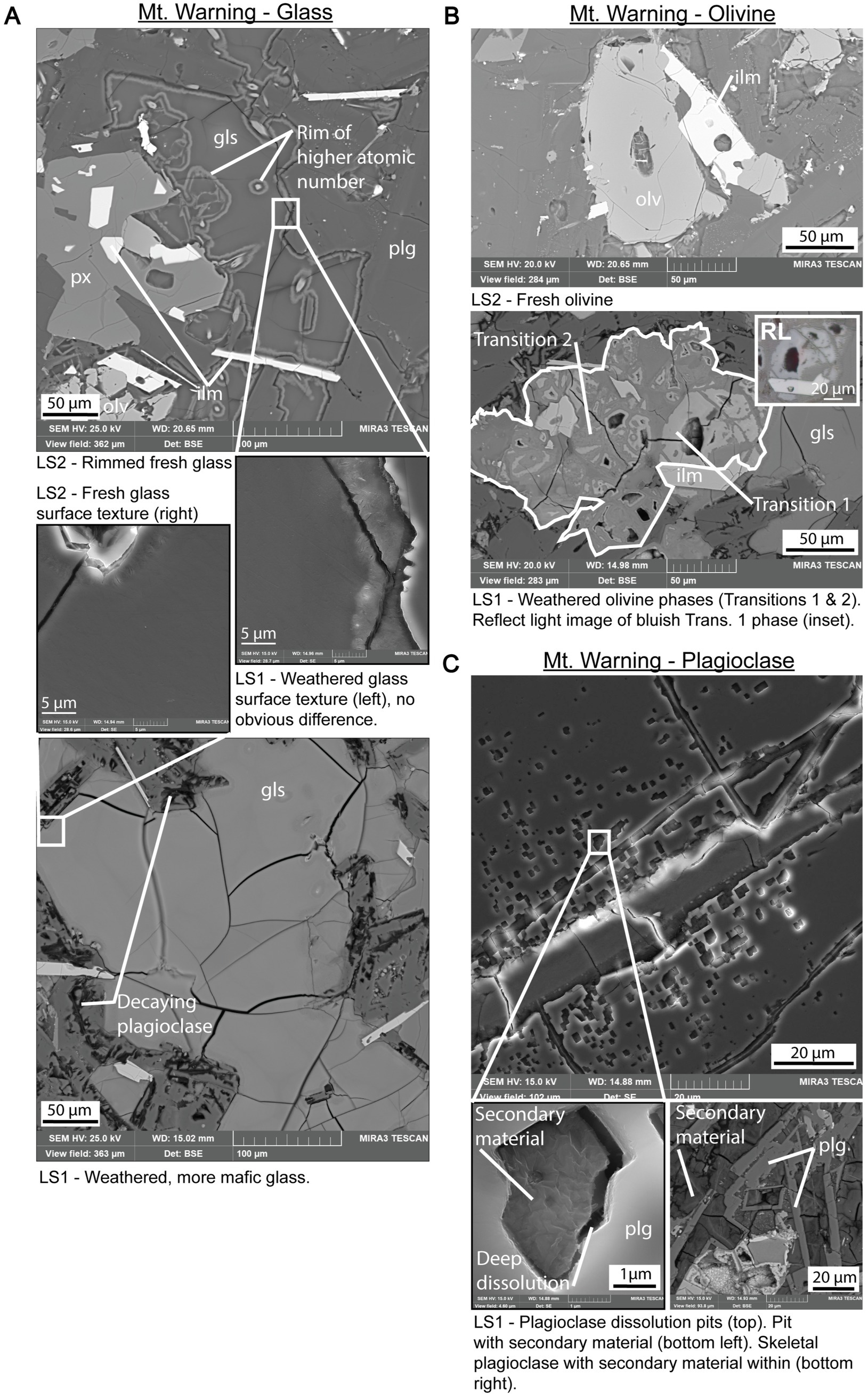
Figure 8. SEM imagery of glass, olivine and plagioclase from the Mt. Warning sample. (A) Comparison of fresh and weathered glass from LS2 and LS1, respectively. Note the difference in greyscale (and, therefore, composition) from fresh to weathered glass (top and bottom). High-magnification images of the surfaces of fresh and weathered glass (middle right and middle left) show no textural differences. (B) Comparison of fresh olivine from LS2 and weathered new phases after olivine in LS1. Inset provides a reference to the bluish reflective phase in RL. (C) SE image of plagioclase megacryst dissolution pitting in LS1. High-magnification imagery of a single pit shows a layer of secondary mineral precipitation and deep dissolution beyond the secondary mineral layer (bottom left). Skeletal groundmass plagioclase, also in LS1, with cracked clay material infilling the skeletal framework. Px = pyroxene, ilm = ilmenite, gls = glass, plg = plagioclase, olv = olivine, RL = reflected light.
In BSE, olivine grains show multiple shades of grey, apparently transitioning twice. Firstly, from the light grey, structurally intact fresh olivine crystal in LS2 (Figure 8B, top), to the bluish highly reflectively phase seen with the RL microscope (also light grey in BSE image, Figure 8B, bottom), to the rough-surfaced phase becoming a pseudomorph of the original olivine (slightly darker grey interstitial material within outline). The EDX spectra of all three phases show that the first transition into the second phase was associated with an increase in Fe and Al, a decrease in Si and a complete loss of Mg. The semi-quantitative SEM–EDX spectrum indicates that the second phase is a silicate despite its reflective appearance in RL. Compared to the second phase, the next transition involved Fe loss, increasing Si approximately back to the same levels before the first transition, and increasing the Al again, with a small addition of Mg (see Supplementary Figure 3 for spectral comparison).
The weathering of the plagioclase megacrysts and groundmass identified with the microscope was confirmed with the SEM. The predominantly prismatic dissolution pitting within the large plagioclase crystal was concentrated around a fracture in the grain. The pits are most abundant next to the fracture (Figure 8C) but, from there, appear to spread out, occasionally distally to the fracture. They could, however, be close to differently oriented fractures not exposed in the 2D thin section. A high-quality SE image of a singular pit (Figure 8C, bottom left) shows a layer of textured material and deep dissolution on the right side, where the pit is possibly merging with others in 3D space and causing the textured layer to collapse. This is suggestive of dissolution outpacing the formation of new materials, creating more porosity, as described in Kanakiya et al. (2017). Comparative spectra of the plagioclase host and the material in the pit reveal a loss of Ca and Si (~4 wt% and ~5.5 wt%, respectively) and a significant relative increase in Al (~9 wt%). This is consistent with the preferential weathering of Ca-rich plagioclase which has much higher dissolution rates than more Na-rich plagioclase (Heřmanská et al., 2022; Manning, 2025). The fractures appear partially infilled in SE. The groundmass skeletal plagioclase is illustrated in Figure 8C (bottom right). The straight plagioclase rims stand out against the cracked clay material (apparently composed only of Al, Si, and O), and are less weathered than the remnant olivine (bottom) that is now infilled with the zeolite mineral chabazite. The breakdown of the groundmass plagioclase in sample LS1 is also seen in Figure 8A (bottom), where the darker grey phase is clearly etched and dissolved, compared to the smooth dark grey plagioclase surfaces in Figure 8A (top) from the fresh side of the thin section, LS2. The plagioclase BSE grey scale contrast is again evidence for compositional variability of plagioclase of different resistivity to weathering.
3.2.3.2 Farnsfield
BSE images (Figure 6A) were obtained to compare a fresh glass patch from FF_RD_02 (Figure 6A, top) and a glass patch from the weathered FF_35b (Figure 6A, middle), both imaged with the optical microscope. The white dotted line denotes the fracture in the fresh glass that caused some anisotropy in XPL, implying that the glass is not homogenous and has experienced stress. In the control sample, the glass surface was found to be smooth and even, aside from cracks, which contrasts with the high density of cracks in the FF_35b glass. The cracks in the weathered sample were broader and more numerous, and the smaller cracks were mostly discontinuous (Figure 6A, bottom). Large topographical irregularities and dissolution pitting were found throughout the glass, differing from the smooth FF_RD_02 glass. The XPL image of the same area in sample FF_35b (Figure 6A, middle) shows the glass has a more mottled anisotropic appearance when compared with the anisotropic glass in XPL from FF_RD_02 (Figure 6A, top). The glass in FF_35b is the same throughout the sample. Figure 6B shows a skeletal pyroxene in FF_35b with the same type of cracked glass infilling the remainder of the crystal shape. The glass is weathered, but the pyroxene and the plagioclase (dark grey around border) appear unaffected by weathering reactions, showing a preferential attack of the glass by the weathering fluid. Semi-quantitative EDX spectra for the fresh (Figure 6A) and weathered glass in Figure 6B show that weathering is accompanied by loss of Ca, Si, Mg and Fe and a slight increase in Al. Both FF_RD_02 and FF_35b had a noticeably high extent of fracturing throughout the particles that cut through multiple crystals. This is demonstrated in Figure 6C, where the latter shows fractures in FF_RD_02 (annotated for clarity). Samples like LS1 have an interconnected matrix of glass, but the Farnsfield samples, while crystalline, have an interconnected matrix of fractures. Optical microscopy revealed olivine dissolution in FF_35b (see Figure 4B, bottom), however, this was not imaged with the SEM. Regardless, FF_35b shows multiple lines of evidence for incipient weathering after 14 months in amended soil.
3.2.3.3 Rangitoto Island
The SEM images acquired for 03A from Rangitoto were to study weathering features within the ~1–1.5 cm-sized particles. Weathering progressed into particle interiors of sample 03A via connected vesicles, grain boundaries and fractures. This is evidenced by minute oxide precipitation (white in BSE) along the vesicle edges (Figure 9A, top and top left) and fractures in Figure 9A. The glass in Figure 9 was severely degraded with deep dissolution voids and surface etching, particularly adjacent to the vesicle at the top of the particle and surrounding fractures. Plagioclase (darker grey, Figure 9A, top) also suffered dissolution, but the pyroxene endured with a largely featureless surface and intact grain boundaries, regardless of the mineral-water interaction of the immediately adjacent weathered glass, an example of which is denoted with the white box in Figure 9A (top). Olivine in 03A is commonly completely weathered to a new material, however, Figure 9A (bottom) captures the progressive dissolution of an olivine grain and the arrangement of the secondary mineral casts that remain. The saw-tooth dissolution edging, also seen in Wodonga and Farnsfield, progressively retreated towards the grain centre and was typically seen along grain boundaries or fractures. The secondary mineral casts appeared to be discontinuous, leaving unfilled cavities behind, destroying the integrity of the grain, and collectively weakening the tephra particle structure. Overall, the order in which basalt constituents were dissolved follows thermodynamic predictions, with plagioclase cone microphenocrysts quite Ca-rich (labradorite with 60–70% anorthite; Needham et al., 2011) and thus more prone to weathering than clinopyroxene.
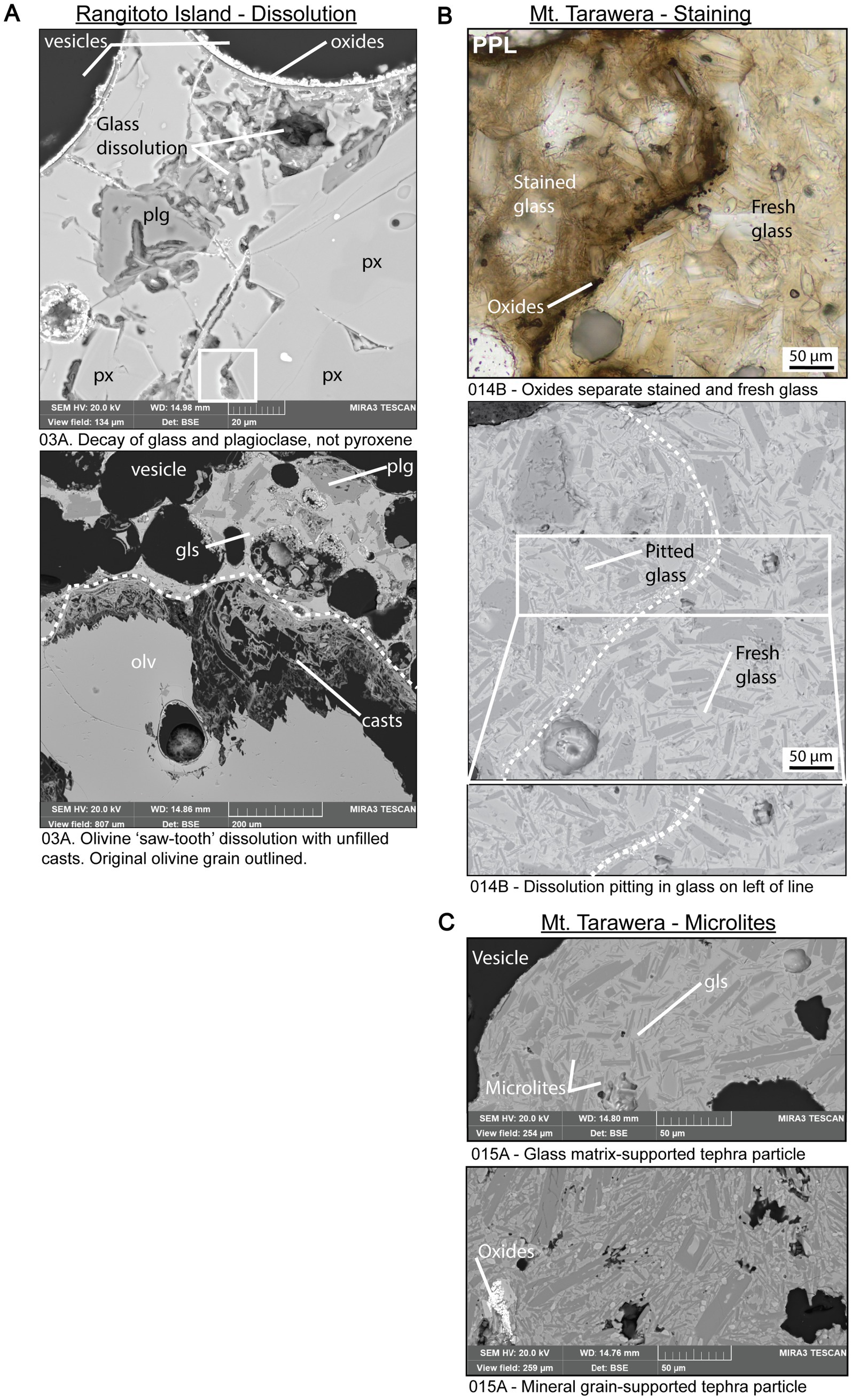
Figure 9. SEM imagery of dissolution from Rangitoto and Mt. Tarawera. (A) BSE image of glass and plagioclase from 03A, Rangitoto, preferentially decaying (top) before the unweathered pyroxene (right of top), an example highlighted with white box where the glass is decaying but the pyroxene is not. Olivine dissolution, also from 03A (bottom), where dissolution occurs towards the grain centre, leaving unfilled casts of secondary material. (B) A PPL image of oxide front and associated glass staining (top). The same region imaged with SEM shows pitting, only in stained glass. (C) BSE images of two tephra particles from 015A. Both show minimal fracturing and the bottom is heavily dominated with microlites, while the top is glass dominant. Px = pyroxene, gls = glass, olv = olivine.
3.2.3.4 Tarawera
With optical microscopy, we identified an area where the glass in a particle from 014B was stained, compared to adjacent glass. This was examined with the SEM, and a comparison is shown in Figure 9B. The oxides are only just visible in the SEM image, the white particles to the left of the white dashed line, where the surface of the glass is clearly pitted and etched. This coincides with the staining on the left side of the optical PPL image. The close-up image of the rectangle in Figure 9B (bottom) further demonstrates the textural difference in the polished surfaces of variably weathered glass, from the smoother, more uniform glass on the right (light grey interstitial material) to the rough, highly textured, partially dissolved glass on the left. In this case, the staining of the glass visible in PPL corresponds to breakdown and dissolution in the glass.
From the optical analysis, Tarawera basalt generated at least some particles with a very high population of microlites. Two particles from the same sample (015A) were imaged with the SEM. Sample 015A was extracted from a vitric andisol, rather than the thick tephra beds. Some of these particles appeared more weathered on the outside than particles from tephra beds (see Figure 1E). However, many particles in 015A remained quite fresh in the interior, despite being buried for ca. 138 years. One of the particles imaged with the SEM was fresh-looking and glassy; the other was also glassy but densely populated with microlites (Figure 9C (top) and (bottom), respectively). What they have in common is that they are relatively fracture-less, and save for the cluster of oxides concentrated near a hole in Figure 9C (bottom), there is essentially no evidence of weathering fluid penetrating the amorphous glass that appears to have protected the encased mineral grains.
3.2.4 BET and surface area analysis
BET analysis was completed on selected samples from Farnsfield, Rangitoto and Tarawera to compare specific surface area (SSA) of ‘fresh’ and more ‘weathered’ samples (from 010A to 03A, 012A to 015A, and FF_RD_02 to FF_35b). The particles selected for the BET surface area analysis had a p80 between 2,000 and 8,250 μm, see Table 2. The p80 approximations were determined from cumulative frequency plots using sieve fraction and ImageJ particle analysis data (see Supplementary Figure 2). The main findings from the BET analysis are that SSA varies greatly between basalts from different contexts, and, significantly, that in all cases, SSA is measurably higher in the weathered equivalent than in the fresh material.
Beginning with the quarried basalt ERW amendment from Farnsfield, which is from a lava flow field, the analysed particle sizes between weathered and fresh were highly comparable (FF_RD_02 and FF_35b with p80 = 6,500 and 6,250 μm, respectively). Surprisingly, despite the quite crystalline groundmass, the measured SSA of this basalt was very high. The SSA of the weathered FF_35b sample was 3.16 m2 g−1, 33% greater than the fresh FF_RD_02 sample at 2.38 m2 g−1. The micropore surface area was 0.1433 m2 g−1 larger in the weathered than the fresh sample, and micropore volume grew by ~30%. We note that these samples required long analysis times, and FF_RD_02 required four analysis attempts, a higher degassing temperature and the use of argon as the adsorbate to gain a usable SSA measurement, see Supplementary Tables 1, 2 for details.
The Rangitoto tephra (03A, p80 = 6,350 μm) had the highest SSA overall at 4.52 m2 g−1 and gained 70% from 010A (p80 = 7,750 μm), the highly angular, fresh tephra from the volcano cone. Similarly, the micropore SSA increased from 1.73 to 2.95 m2 g−1 from 010A to 03A, and the micropore volume expanded by 75% with weathering, from 0.00074 to 0.0013 cm3 g−1, respectively. Despite three attempts for 012A and four attempts for 015A, the samples from Tarawera consistently yielded SSA measurements that were well below the ~2 m2 per sample mass detection limit of the instrument, see Supplementary Tables 3, 4. Crushing 012A and 015A finer with each attempt (p80 approximations in Table 2) to allow for more sample mass in the analysis tube did not alleviate this issue. Instead, the SSA per gram counterintuitively decreased on three occasions, 012A—attempt 2 and 015A—attempts 2 and 4. Nonetheless, Tarawera analysis attempts showed a certain increase in SSA (23%), micropore SSA (127%) and micropore volume (285%) from the fresh to the weathered samples. Even though the SSA of the Tarawera samples increased from 0.34 m2 g−1 (fresh) to 0.41 m2 g−1 (weathered), the SSA of the weathered tephra is much less than the Rangitoto and Farnsfield equivalents, 4.52 and 3.16 m2 g−1, respectively. In all analyses, the SSA was comprised of more than 50% microporous surface area (51–78%), except for the final attempts for 012A and 015A where Ar was used, and the proportion of SSA that was microporous was 11 and 20%, respectively. Interestingly, for the two Farnsfield samples, the SSA consisted of 63% (FF_RD_02) and 52% (FF_35b) micropore SSA, even though these are most crystalline samples without vesicles.
For comparison to SSA, the theoretical outside surface area (m2 g−1) of the exact particles (except 012A_Attempt 3, 015A and 015_Attempt 4) used in the BET analyses was calculated assuming spheres and using the Feretmax diameter (Fmax) acquired with image analysis software (Table 3). Images of the samples post BET analysis are given in Supplementary Figure 1. Different densities were used to calculate the sample masses, depending on the different modes of deposition: 010A = 1.0, 03A, 012A and 015A = 1.5, FF_RD_02 and FF_35b = 2.8. The Fmax was justified by comparing the actual sample mass to the calculated sample mass, using the Fmax and the expected density for each sample. Using the Fmax as a base for the calculations meant that the calculated mass of the theoretical spheres associated with each particle (Table 3) was overestimated by a factor of 4 ± 1.7 on average, particularly for Farnsfield, where the particles are mostly elongated and tabular (Supplementary Figure 1) and thus worst approximated by a sphere. Using Fmax rather than a ratio between Fmax and the Feret minimum diameter somewhat compensates for the 2D representation and surface irregularity of each particle. Therefore, it is a more realistic parameter to use for an approximate theoretical particle surface area calculation. Table 3 shows surface area comparisons between the BET-measured SSA and the calculated surface area per gram. The surface area for the two sieved samples (012A_Attempt 3 and 015A_Attempt 4) was calculated using equations 11 and 13 from Tester et al. (1994). The difference between the calculated and measured surface area differs by several orders of magnitude; the actual SSA is between ~98 and 8,500 times larger than the calculated surface area. The smallest difference was found for Tarawera tephra, where optical and SEM observations revealed the least fractured glass.
3.2.5 XRD
Mineralogy was determined for four suites of samples with results reported in Table 4. Beginning with the visibly most weathered sample set, we milled LS1 and LS2 into separate powders for XRD analysis. This comparison confirmed notable losses of olivine and plagioclase (5.6 and 6.2 wt%, respectively) in LS1 over LS2. These are consistent with the breakdown of the two primary phases already inferred from optical and SEM microscopy. The new reflective phase that replaced olivine was not detected by the XRD results. Ilmenite is present (proportion relatively unchanged between LS1 and LS2 as expected), but no new secondary oxide was found. The diffraction lines attributable to plagioclase for LS1 and LS2, confirmed the optical microscopy observation of chemical heterogeneity. Plagioclase was modelled as a mixture of mostly labradorite (65% anorthite), with some andesine (50% anorthite) and subordinate oligoclase (25% anorthite). The most calcic plagioclase, labradorite, saw the largest decrease of ~5 wt%. This conforms with the empirical observations of the Goldich series of dissolution (Goldich, 1938), thermodynamic dissolution rate data (Heřmanská et al., 2022) and the predicted weathering sequence of basalt ERW (Manning, 2025). Pyroxene (augite) increased in weight per cent (~4%). However, this could be relative to the loss of the other phases (olivine and plagioclase) for LS1. Some of the increase in non-diffracting material (glass and non-diffracting proto-clay minerals) could also be partially attributed to the relative decrease in the other phases. Despite the apparent textural evidence of clay in LS1, the bulk XRD was unable to detect it. A clay-fraction specific analysis was then undertaken, but it also confirmed the absence of crystalline clays (e.g., smectite). However, there is a broad background feature between 5 and 7 degrees 2θ, indicating the possible presence of short-range-order clays (e.g., allophane and imogolite).
For the Farnsfield samples, the non-diffracting material in FF_35b was ~4 wt% lower than in the fresh sample (FF_RD_02), consistent with more glass visible in thin section. Alternatively, the reduction could be explained by the dissolution of glass without precipitation of new crystalline material. However, both Farnsfield samples had a very low proportion of non-diffracting material compared to other samples, due to the higher crystallinity of the groundmass in the lava flow sample. The increase in olivine, pyroxene and plagioclase from the fresh sample suggests no direct evidence of primary phase weathering in FF_35b aside from slight increases in kaolinite and illite, though the clay-specific analysis did identify smectite in the sample retrieved from the soil (FF_35b).
Sample 03A from Rangitoto predictably had a decrease in olivine (~4 wt%) compared to the fresh sample 010A, consistent with the dissolution of olivine seen in thin section. XRD found no decrease in the pyroxene or plagioclase fractions. Sample 03A also displayed a lower fraction of non-diffracting material, likely due to glass dissolution like FF_35b, but no diffracting or non-diffracting clays were evident from the XRD results.
Four samples from Tarawera were analysed. There was effectively no difference between the two fresh samples and the two incipiently ‘weathered’ samples. The SEM observations suggested that weathering could have caused a decrease in non-diffracting material, as the dissolution of glass without secondary mineral precipitation was evident. However, this did not transpire in the XRD patterns, possibly because Tarawera samples had only experienced minor initial dissolution or because glass weathered to X-ray amorphous clays. The XRD method is not suitable for differentiating between glass and secondary nanoclays like allophane and imogolite, as they both contribute to ‘non-diffracting’ background in the spectra. However, XRD highlights that the dissolution of glass, olivine and possibly plagioclase is faster than the precipitation of secondary clays, which has implications for increased porosity and particle stability.
4 Discussion
4.1 Evidence of sequential breakdown of phases with incipient weathering
Basalt is the most promising candidate for ERW material for several reasons, including its ability to break down relatively quickly under some conditions. Large basalt particles (>1 mm) are often assumed to break down slowly compared to finely ground basalt due to the exponential increase in particle surface area with diminishing diameter (Vandeginste et al., 2024). However, this is only true if the particle perimeter or equivalent geometric surface is the only portion of the particle accessible to weathering fluids. By contrast, natural basalt particles contain fractures, grain boundaries and vesicles that can act as an interconnected matrix allowing water to enter the interior of a particle and weather it from within. Israeli et al. (2021) modelled the dissolution of rocks with different types and proportions of grain boundaries and cracks and found that in all cases, weathering rates increased over idealised grains. The basalt particles microscopically analysed in this study contain evidence of weathering of the glass and mineral phases not only on the interior of large particles (diametermax > 10 mm) but also on the interior of individual constituent mineral grains. We propose that the access provided by the interconnected matrix of fractures, grain boundaries and vesicles allow water to permeate a particle. From there, the weathering fluid apparently preferentially attacked the most weatherable phases distributed throughout the entire particle, leaving more robust phases mostly unweathered.
This sequential breakdown of phases begins with glass. The glass on the ‘fresh’ side of the Mt. Warning thin section (LS2) shows early signs of incipient weathering but the olivine is yet to undergo transformation. Similarly, the irregularities in the glass of FF_35b from Farnsfield indicate dissolution, contrasting with the directly adjacent pyroxene and plagioclase that remain unetched and unweathered (Figures 6A,B). The Rangitoto volcano and Tarawera tephra samples 03A and 014B both show pyroxene crystals standing out as unweathered among the dissolution pitting visible in the surrounding glass (Figures 9A,B, respectively). It is noted that in laboratory studies, there is a strong pH dependence on glass dissolution rates and greater disagreement between individual studies than for minerals (Heřmanská et al., 2022). However, the dissolution and alteration observations from this study suggest glass to be the most weatherable phase in the studied basalts, including at the Farnsfield ERW trial site with shallow soil pH between 5.4 and 6.0 (Figure 2B). Olivine, the next most vulnerable phase to breakdown, sequentially and completely transitioned to new phases on the weathered side of the Mt. Warning thin section (LS1). This appears to have occurred only after all the glass had weathered to a different phase. Olivine weathering progressed while adjacent pyroxene experienced only minor to intermediate dissolution. Likewise, the breakdown of the pyroxene in 03A from Rangitoto Island was outpaced by the preferential partial to complete dissolution of the olivine. In both samples (LS1 and 03A), the XRD results reflect the preferential breakdown of olivine and show a loss of 72 and 19%, respectively. Unlike pyroxene, where silica tetrahedra linked by strong Si–O–Si bonds slow dissolution comparatively, the olivine structure consists of isolated silica tetrahedra separated by ionically bonded divalent metal cations (e.g., Mg2+). As such, breaking only the ionic bonds between the tetrahedra and metal cations (Mg–O) is sufficient to release the silica tetrahedra into the weathering fluid and cause the olivine to break down and dissolve (Oelkers et al., 2018). Finally, the last phases to break down in the weathering sequence in all the samples were pyroxene or plagioclase; the order of preferential dissolution seemingly depended either on their respective compositions and/or soil pH, where plagioclase shows greater solubility than pyroxene at low pH (Heřmanská et al., 2022). In short, the phases within the studied basalt (glass, olivine, pyroxene and plagioclase) weather at vastly different rates, with preferential breakdown of easily weathered phases and limited to no evidence for concomitant weathering.
The observed progressive breakdown of the most to the least weatherable phases aided by a matrix of fluid pathways may have important implications for ERW. In basalt, where the easily weatherable phases and fractures and cracks form a connective network, their weathering will structurally disintegrate even large (>10 mm) particles. This will release the more weathering-resistant constituent minerals into the soil. Modelling by Israeli and Emmanuel (2018) found mechanical detachment of grains could accelerate overall weathering progress by 37–50% in rocks with varying proportions of high and low reactive minerals. Interspersed, highly weatherable phases (glass and olivine) combined with water-accessible grain boundaries, fractures and vesicles make basalt particles prone to rapid chemical weathering and mechanical disintegration, ideal for ERW.
More advanced stages of the sequential breakdown of basalt are apparent in the Wodonga macadamia farm samples. The prevalence of weathering-resistant greenschist and quartz-rich lithologies with minor chlorite and epidote, and the scarcity of identifiable coherent basalt particles in the MAC1 5–10 cm thin section suggests that basalt buried to this depth is mostly broken down. Due to the dominance of greenschist rather than basalt in the rock dust amendment used at Wodonga, the lack of surviving basalt particles in the MAC1 soil core could be attributed to a potential lack of basalt historically applied. However, the overall pH increases in MAC1 compared to the control, would not have been possible if the rock dust amendment did not contain sufficient basalt to dissolve into the soil profile (Figure 2A). Additionally, MAC1 soil aggregates include small fragments of plagioclase containing oxides and scarce pyroxenes, suggesting advanced chemical and mechanical weathering of some basalt particles, while liberated ilmenite grains distributed throughout the aggregates indicate complete dissolution of others. If the historically applied basalt particles were sized similarly to basalt from MAC1 0–5 cm (~5–7 mm), the lack of large particles and the presence of only small fragments remaining in the soil aggregates (Figure 5A) suggests the basalt has undergone significant dissolution, over a decadal timescale. The dissolution and physical breakdown of the basalt particles from the Wodonga site samples is likely the result of water percolating through a particle, sequentially weathering the most vulnerable phases along an interconnected matrix of grain boundaries and fractures to such a degree that mechanical grain detachment caused the particles to disintegrate.
4.2 Evidence of increased surface area from incipient weathering
Particle surface area is a critical parameter for calculating carbon sequestration potential. The higher the reactive surface area (i.e., the smaller the particles), the faster the weathering rate (Hartmann et al., 2013; Vandeginste et al., 2024). The current study shows that large basaltic particles (p80 = 2–8.25 mm) not only have surface areas on par with fine particles (3.5–150 μm) in other studies (Oelkers and Gislason, 2001; Gislason and Oelkers, 2003; Wolff-Boenisch et al., 2004; Gudbrandsson et al., 2011; Xiong et al., 2017; Alraddadi and Assaedi, 2021; Delerce et al., 2023) (see Supplementary Table 6), but that the specific surface area (SSA) increased with incipient weathering. SEM imaging of the dissolution pits in a plagioclase grain in LS1 from Mt. Warning, the unfilled casts produced by the dissolving olivine in 03A from Rangitoto, and the glass dissolution pitting associated with iron staining in the Tarawera 014B tephra, suggest that the SSA increase is a result of increased porosity from weathering. BET analyses were instrumental in demonstrating that large ‘fresh’ particles (p80 = 6.5–7.75 mm post crushing) already had high specific surface areas in the case of the Farnsfield and Rangitoto samples (2.4 and 2.7 m2 g−1, respectively), and that the weathered particles had increased SSA due to the formation of additional microporosity caused by dissolution. The SSA was surprisingly high for the Farnsfield samples, given their crystallinity. It is unclear if the extent of fracturing is natural or whether additional microporosity was an accidental beneficial side effect of processing in the quarry (i.e., blasting, crushing, further grain reduction, sieving). In other words, the comminution process may create supplementary pathways for weathering fluid to saturate a particle (Figure 6C).
Alteration studies have reported secondary mineral precipitation that can cause diminished porosity and alteration rate-limiting blockages (Ruiz-Agudo et al., 2016; Vieira et al., 2021). We found no evidence to support blockages of internal pathways because of secondary mineral precipitation during incipient weathering. XRD results showed long-range-order clays (smectite, kaolinite and illite) in FF_35b from Farnsfield, but the latter two were also present in the control, and increased microporosity suggests that clays did not block fluid pathways and were not weathering rate-limiting. The glassier samples from Rangitoto and Mt. Tarawera had no X-ray-detectable clays, nor were there any broad, low-angle features in the bulk X-ray diffractograms that might have indicated the presence of short-range-order clays, like allophane and imogolite. In all samples, the absence of short-range-order clays and increased microporosity (except LS1, which possibly had SRO clays but was not analysed with BET) shows that rate-limiting layers or phases are not forming with incipient weathering in the studied basalts. Expansion of microporosity and absence of clay apparently resulted in increased reactable surface area, suggesting that dissolution is occurring at a faster rate than new mineral precipitation.
Kanakiya et al. (2017) found a similar rate disparity that caused structural damage and compromised particle rigidity after 140 days of basalt/carbonic acid reactions. An example of compromised rigidity is shown in the dissolution pits of the plagioclase grain in LS1 (Figure 8C), where pitting that outpaced precipitation appears to have connected with other pits in 3D space, reducing the overall integrity of the plagioclase grain. Kanakiya et al. (2017) correspondingly found that four out of five basalts from the Auckland Volcanic Field (where Rangitoto Island is located) experienced a change in porosity proportionate to the glass content in each sample. The authors noted that the fifth sample had a disproportionate increase in porosity, possibly due to fracturing during the experiment. The current study found fracturing that promotes pathways to a particle interior to be a controlling factor for the dissolution of glassy particles. Particles from the Tarawera samples were optically observed to have low fracture density in the glass that protected the abundant microlites within. BET analyses similarly showed samples 012A and 015A to have only 10–20% microporosity and a small increase in specific surface areas (0.3–0.4 m2 g−1, respectively), interpreted to be a result of restricted access to particle interiors due to limited fracturing in the glass, as opposed to blockages of fluid pathways.
Our study shows that relatively large basaltic particles (diametermax 10+ mm) can experience primary phase breakdown, including in grain interiors, and measurable increases in microporosity and surface area as a result of internal dissolution from incipient weathering within soil. This result is conditional upon particles initially having a network of fractures that increases the internal surface area available for attack by weathering fluids, whether the cause be natural (e.g., shrinkage) or mechanical comminution. However, the degree of internal weathering in particles observed in this study shows that the reactive surface area of a particle is much greater than the geometric or calculated surface area and that calculating weathering rates based on a geometric surface area that is 98–8,500 times less than the SSA can substantially underestimate the true weathering potential of a sample.
4.3 Recommendations for enhanced rock weathering
Our study investigated the potential role of surface area to mass ratio for weathering rates of basalt in natural settings. We used microscopy to observe phase breakdown in naturally weathered basaltic particles as an inexpensive method to infer potential physical proxies for weathering.
The particles used in the present study were intentionally large and of greater diameter than the finely ground basalt powder classically used in ERW modelling and pot and field plot experiments. The choice was informed by two considerations: firstly, smaller particles are notoriously difficult to extract from natural soils due to their friability. Secondly, we hypothesised that if there was a better understanding of how large basalt particles decay in natural soils, the CO2 emissions caused by the final, most energy-intensive step in the comminution process might be minimised. The final grinding step mills the basalt particles from <5,000 to <10 μm, using approximately 15–100 kWh of electricity per tonne (Renforth, 2012). The same amount of energy could theoretically be saved by using basalt with an average particle size of 5,000 μm. On two occasions in the current study, crushing particles smaller actually decreased, rather than increased the SSA by 23 and 11%, despite more particles added for crushing (see 012A_Attempt 2 and 015A_Attempt 2, Table 2). If basalt particles sequentially decay, as observed in this study, the most weatherable phases will break down first, as schematically illustrated in Figure 10. This will cause the particle to become structurally unstable through increased porosity and disintegrate, regardless of whether the original particle was >5,000 μm, as is likely the case with the MAC1 basalt particles from Wodonga and sample 03A from Rangitoto. The caveat is that a sufficient network of grain boundaries, fractures and vesicles must be initially distributed throughout the particle, contributing to a large foundational surface area available for weathering.
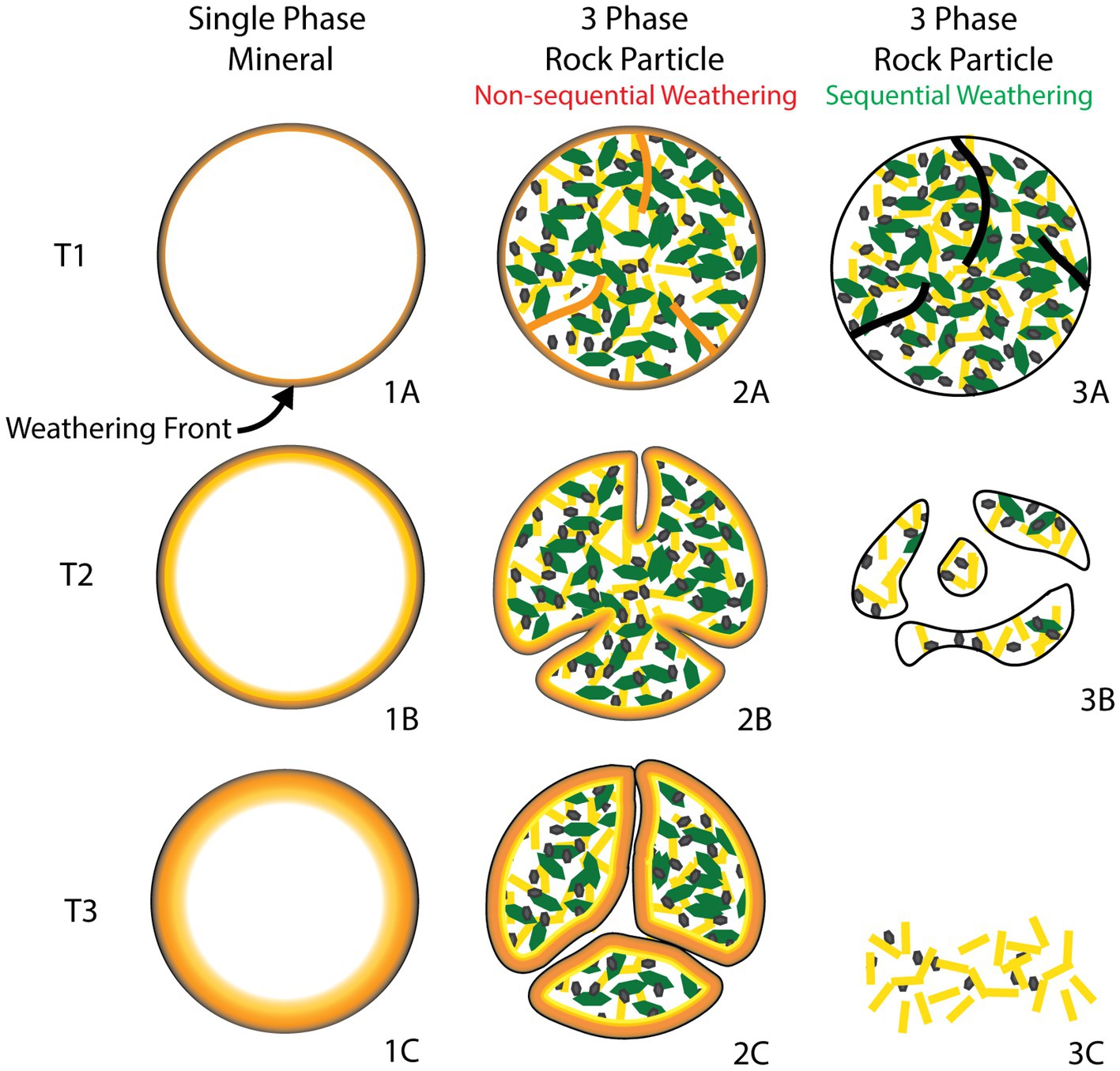
Figure 10. Schematic diagram illustrating the theoretical breakdown of particles that weather only around the outside versus particles that break down at the same rate (non-sequentially), or at different rates (sequentially). The single-phase mineral only weathers from the outside inwards through time (T1 to T3). The fractured, polycrystalline particle in 2A breaks down faster than the particle in 1A, but all phases weather at the same rate. The interior of particle 3A is accessed by fracturing that allows water to dissolve the most vulnerable phase first (green polygons), creating porosity and compromising the particle integrity (3B). The next most weatherable phase is mostly dissolved, but the particle has already disintegrated, and only liberated phases remain (3C).
Microscopy is an inexpensive and informative method of assessing weathering in specific basalt samples and their suitability as an ERW amendment. The following insights have been obtained:
• Rock dust composition—A thin section of the proposed rock dust can immediately inform on the particles’ composition and if the rock is indeed basalt and metabasalt. In the case of our field site at Wodonga, ‘basalt’ had been supplied from quarries that actually produce greenschist facies metabasalt (Figure 5A). This is an important distinction, as greenschist (i.e., metabasalt) has a completely different mineralogy and will weather substantially more slowly than igneous basalt. Additionally, the modal abundance of highly weatherable phases, such as olivine and glass, can be identified and quantified with the microscope.
• Material freshness—Optical mineralogy alone is a very suitable method for identifying the breakdown of highly weatherable phases. In transmitted light, weathering is expressed as iron staining, and in RL, weathering can be seen in reflectivity, surface polish quality and, under very high magnification, as pitting. In ERW, such microscopic analysis is essential for the feedstock to assess the extent of alteration prior to amendment. By contrast, XRD analyses will likely not detect X-ray amorphous clays present in a sample, or such clays will be attributed to the ‘non-diffraction’ portion of the rock, essentially allocating it to glass. This has important ramifications for carbon sequestration calculations as the potential to draw down CO2 will be overestimated if a proportion of the amount attributed to glass has already decayed to X-ray amorphous clay. Therefore, using XRD alone as a method to identify and quantify clays as a measure for weathering is not recommended.
• Pathways for fluid access—The optical and scanning electron microscopes are useful for an assessment of how grain boundaries, fractures and vesicles are distributed in the interior of particles. This is critical as they act as access pathways for water. Ubiquitous oxides in devitrified glass conceal fractures (all in PPL, most in RL) and polished surface texture, rendering weathering assessments inconclusive. Imagery of non-devitrified particles showed prominent fracturing and grain boundaries in sample FF_RD_02, revealing the main pathways for water to access and dissolve internal interstitial glass. In the 03A tephra sample, microscopy showed interconnected vesicles in 2D and notable fracturing, which collectively provided the pathways that accelerated weathering. Conversely, most of the Tarawera tephra samples were dominated by microlites and, therefore, grain boundaries, but it appears that microlites did not grow to the point where they could fracture the encasing protective glass, consequently restricting water access to the grains. The low degree of weathering in the samples from Tarawera (and 08A from Motutapu) could additionally have been caused by the very high permeability of the thick tephra beds they were emplaced in. In free-draining beds, water cannot saturate tephra particles. While this is likely the case for 08A, sample 015A was extracted from soil near the Mt. Tarawera vent, not from a tephra bed, and had minimal fracturing and a low SSA (0.41 m2 g−1). Consequently, the lack of weathering is probably due to the degree of impenetrability of the weakly fractured glass. By contrast, we observed that fractured basalt particles that were incorporated into the soil, rather than in beds, experienced the most efficient weathering, presumably due to full water saturation.
• Specific Surface Area—Microscopic assessment of potential fluid pathways and incipient weathering provides a useful context for surface areas available for attack by weathering, as measured with a BET analysis. Our results show a high BET SSA (>2 m2 g−1) of a fresh sample is a good indicator of internal weathering potential and that a low initial BET SSA (<0.5 m2 g−1) suggests less weatherable material. Samples like those from Farnsfield, a coarse-grained, largely crystalline material with a comparatively low glass content (XRD non-diffracting phases = 2 and 6 wt%), should not be discounted as a suitable product for ERW. The high fracture and grain boundary densities are likely responsible for the increase in total SSA and micropore SSA, 33 and 10%, respectively, after just 14 months in soil. More research is required to understand if the fracturing in quarried samples is original or caused by blasting and comminution. In summary, current methods for assessing ERW potential of basalts are mostly focussed on chemistry and mineralogy but could easily be complemented with inexpensive microscopy for analysis of the internal particle fabric.
• Potential physical proxies for weathering—Observations from analysing different particles with the optical microscope and SEM could be used to infer incipient weathering in basalts and could aid MRV efforts for CO2 removal by ERW. Staining in glass and the formation of very fine-grained oxides were found to coincide with dissolution pitting in 014B (Figure 9B). Changes in the reflectivity of olivine and glass did not correlate to changes in surface texture but did denote differences in composition. This was observed in the Mt. Warning LS1 sample, where the olivine became more reflective and lost all Mg and gained Fe and Al initially, then lost Fe and gained Al and Si in the secondary transition. The weathered glass in LS1 had significantly more Fe than the fresh glass (~17 wt%) but lost Mg and Si in the process. Pitting in mineral and glass phases indicates dissolution without pathway-blocking secondary mineral precipitation. This was observed in plagioclase in LS1 (Figure 8C), glass in 014B from Tarawera (Figure 9B) and FF_35b from Farnsfield (Figure 6A), and in the olivine in 03A from Rangitoto (Figure 9A). Dissolution corresponded to increased microporosity that resulted in an increased SSA available for weathering, confirmed with BET analysis. Finally, glass breakdown is indicated by changes from isotropic to mottled anisotropic appearance in XPL.
• The microscopic study of basalt particle breakdown becomes more difficult at intermediate and advanced stages of weathering because sequential dissolution and internal weathering tend to compromise a particle’s mechanical integrity, making it difficult or impossible to prepare particles as microscope slides.
Data availability statement
The original contributions presented in the study are included in the article/Supplementary material, further inquiries can be directed to the corresponding author.
Author contributions
TB: Formal analysis, Investigation, Methodology, Visualization, Writing – original draft. BK: Conceptualization, Formal analysis, Investigation, Methodology, Supervision, Writing – review & editing. DR: Conceptualization, Methodology, Writing – review & editing.
Funding
The author(s) declare that financial support was received for the research and/or publication of this article. This research was supported by an Australian Government Research Training Program Scholarship.
Acknowledgments
This research was enabled by the use of the Central Analytical Research Facility (CARF) at the Queensland University of Technology (QUT). We thank Donald McAuley and Gus Luthje for making the thin sections, and other CARF staff—Jun Zhang (Senior Technologist) and Liz Graham (Laboratory Coordinator) of the Physical Properties Laboratory, Henry Spratt (Senior X-ray Technologist), Ming Li (Senior Technologist [Material EM]) and Marco Acevedo Zamora (Microscopist) for their expertise and training. We also thank Emma Conway for operating the SEM. Special thanks to Marco Bobbert and the Russo family, the owners of the Wodonga and Farnsfield farms, for access to their orchards. Two journal reviewers are thanked for their constructive suggestions and Dr. Kolosz for editorial handling.
Conflict of interest
The authors declare that the research was conducted in the absence of any commercial or financial relationships that could be construed as a potential conflict of interest.
Generative AI statement
The authors declare that no Gen AI was used in the creation of this manuscript.
Publisher’s note
All claims expressed in this article are solely those of the authors and do not necessarily represent those of their affiliated organizations, or those of the publisher, the editors and the reviewers. Any product that may be evaluated in this article, or claim that may be made by its manufacturer, is not guaranteed or endorsed by the publisher.
Supplementary material
The Supplementary material for this article can be found online at: https://www.frontiersin.org/articles/10.3389/fclim.2025.1572341/full#supplementary-material
References
Acevedo Zamora, M. A., and Kamber, B. S. (2023). Petrographic microscopy with ray tracing and segmentation from multi-angle polarisation whole-slide images. Fortschr. Mineral. 13:156. doi: 10.3390/min13020156
Alraddadi, S., and Assaedi, H. (2021). Physical properties of mesoporous scoria and pumice volcanic rocks. J. Phys. Commun. 5:115018. doi: 10.1088/2399-6528/ac3a95
Azar, C., Lindgren, K., Obersteiner, M., Riahi, K., van Vuuren, D. P., den Elzen, K. M. G. J., et al. (2010). The feasibility of low CO2 concentration targets and the role of bio-energy with carbon capture and storage (BECCS). Clim. Chang. 100, 195–202. doi: 10.1007/s10584-010-9832-7
Bamber, E. C., La Spina, G., Arzilli, F., Polacci, M., Mancini, L., De’ Michieli Vitturi, M., et al. (2024). Outgassing behaviour during highly explosive basaltic eruptions. Commun. Earth Environ. 5:3. doi: 10.1038/s43247-023-01182-w
Beerling, D. J., Epihov, D. Z., Kantola, I. B., Masters, M. D., Reershemius, T., Planavsky, N. J., et al. (2024). Enhanced weathering in the US Corn Belt delivers carbon removal with agronomic benefits. Proc. Natl. Acad. Sci. USA 121:e2319436121. doi: 10.1073/pnas.2319436121
Beerling, D. J., Kantzas, E. P., Lomas, M. R., Wade, P., Eufrasio, R. M., Renforth, P., et al. (2020). Potential for large-scale CO2 removal via enhanced rock weathering with croplands. Nature 583, 242–248. doi: 10.1038/s41586-020-2448-9
Beerling, D. J., Leake, J. R., Long, S. P., Scholes, J. D., Ton, J., Nelson, P. N., et al. (2018). Farming with crops and rocks to address global climate, food and soil security. Nat. Plants 4, 138–147. doi: 10.1038/s41477-018-0108-y
Berner, R. A., and Holdren, G. R. (1979). Mechanism of feldspar weathering—II. Observations of feldspars from soils. Geochim. Cosmochim. Acta 43, 1173–1186. doi: 10.1016/0016-7037(79)90110-8
Beuttler, C., Charles, L., and Wurzbacher, J. (2019). The role of direct air capture in mitigation of anthropogenic greenhouse gas emissions. Front. Clim. 1:10. doi: 10.3389/fclim.2019.00010
Broekhoff, J. C. P., and de Boer, J. H. (1968). Studies on pore systems in catalysts: XIII. Pore distributions from the desorption branch of a nitrogen sorption isotherm in the case of cylindrical pores B. Applications. J. Catal. 10, 377–390. doi: 10.1016/0021-9517(68)90153-X
Brunauer, S., Emmett, P. H., and Teller, E. (1938). Adsorption of gases in multimolecular layers. J. Am. Chem. Soc. 60, 309–319. doi: 10.1021/ja01269a023
Coltelli, M., Del Carlo, P., and Vezzoli, L. (1998). Discovery of a Plinian basaltic eruption of Roman age at Etna volcano, Italy. Geology 26, 1095–1098. doi: 10.1130/0091-7613(1998)026<1095:Doapbe>2.3.Co;2
Delerce, S., Bénézeth, P., Schott, J., and Oelkers, E. H. (2023). The dissolution rates of naturally altered basalts at pH 3 and 120 °C: implications for the in-situ mineralization of CO2 injected into the subsurface. Chem. Geol. 621:121353. doi: 10.1016/j.chemgeo.2023.121353
Delvigne, J., Bisdom, E., Sleeman, J., and Stoops, G. (1979). Olivines, their pseudomorphs and secondary products. STIBOKA: Wageningen.
Dietzen, C., Harrison, R., and Michelsen-Correa, S. (2018). Effectiveness of enhanced mineral weathering as a carbon sequestration tool and alternative to agricultural lime: an incubation experiment. Int. J. Greenhouse Gas Control 74, 251–258. doi: 10.1016/j.ijggc.2018.05.007
Doelman, J. C., Stehfest, E., van Vuuren, D. P., Tabeau, A., Hof, A. F., Braakhekke, M. C., et al. (2020). Afforestation for climate change mitigation: potentials, risks and trade-offs. Glob. Chang. Biol. 26, 1576–1591. doi: 10.1111/gcb.14887
Dupla, X., Claustre, R., Bonvin, E., Graf, I., Le Bayon, R.-C., and Grand, S. (2024). Let the dust settle: impact of enhanced rock weathering on soil biological, physical, and geochemical fertility. Sci. Total Environ. 954:176297. doi: 10.1016/j.scitotenv.2024.176297
Fajardy, M., and Mac Dowell, N. (2017). Can BECCS deliver sustainable and resource efficient negative emissions? Energy Environ. Sci. 10, 1389–1426. doi: 10.1039/C7EE00465F
Friedlingstein, P., O'Sullivan, M., Jones, M. W., Andrew, R. M., Hauck, J., Landschützer, P., et al. (2024). Global carbon budget 2024. Earth Syst. Sci. Data Discuss. 17, 965–1039. doi: 10.5194/essd-17-965-2025
Gillman, G. P., Burkett, D. C., and Coventry, R. J. (2002). Amending highly weathered soils with finely ground basalt rock. Appl. Geochem. 17, 987–1001. doi: 10.1016/S0883-2927(02)00078-1
Gislason, S. R., and Oelkers, E. H. (2003). Mechanism, rates, and consequences of basaltic glass dissolution: II. An experimental study of the dissolution rates of basaltic glass as a function of pH and temperature. Geochim. Cosmochim. Acta 67, 3817–3832. doi: 10.1016/S0016-7037(03)00176-5
Gordon, S. J., and Brady, P. V. (2002). In situ determination of long-term basaltic glass dissolution in the unsaturated zone. Chem. Geol. 190, 113–122. doi: 10.1016/S0009-2541(02)00113-4
Gudbrandsson, S., Wolff-Boenisch, D., Gislason, S. R., and Oelkers, E. H. (2011). An experimental study of crystalline basalt dissolution from 2⩽pH⩽11 and temperatures from 5 to 75°C. Geochim. Cosmochim. Acta 75, 5496–5509. doi: 10.1016/j.gca.2011.06.035
Guo, F., Wang, Y., Zhu, H., Zhang, C., Sun, H., Fang, Z., et al. (2023). Crop productivity and soil inorganic carbon change mediated by enhanced rock weathering in farmland: a comparative field analysis of multi-agroclimatic regions in Central China. Agric. Syst. 210:103691. doi: 10.1016/j.agsy.2023.103691
Hartmann, J., West, A. J., Renforth, P., Köhler, P., De La Rocha, C. L., Wolf-Gladrow, D. A., et al. (2013). Enhanced chemical weathering as a geoengineering strategy to reduce atmospheric carbon dioxide, supply nutrients, and mitigate ocean acidification. Rev. Geophys. 51, 113–149. doi: 10.1002/rog.20004
Heřmanská, M., Voigt, M. J., Marieni, C., Declercq, J., and Oelkers, E. H. (2022). A comprehensive and internally consistent mineral dissolution rate database: part I: primary silicate minerals and glasses. Chem. Geol. 597:120807. doi: 10.1016/j.chemgeo.2022.120807
Holden, F. J., Davies, K., Bird, M. I., Hume, R., Green, H., Beerling, D. J., et al. (2024). In-field carbon dioxide removal via weathering of crushed basalt applied to acidic tropical agricultural soil. Sci. Total Environ. 955:176568. doi: 10.1016/j.scitotenv.2024.176568
Iff, N., Renforth, P., and Pogge von Strandmann, P. A. E. (2024). The dissolution of olivine added to soil at 32°C: the fate of weathering products and its implications for enhanced weathering at different temperatures. Front. Clim. 6:1252210. doi: 10.3389/fclim.2024.1252210
Isbell, R. F. (1996). The Australian soil classification. Collingwood, Victoria, Australia: CSIRO Publishing.
Israeli, Y., and Emmanuel, S. (2018). Impact of grain size and rock composition on simulated rock weathering. Earth Surf. Dyn. 6, 319–327. doi: 10.5194/esurf-6-319-2018
Israeli, Y., Salhov, E., and Emmanuel, S. (2021). Impact of textural patterns on modeled rock weathering rates and size distribution of weathered grains. Earth Surf. Process. Landf. 46, 1177–1187. doi: 10.1002/esp.5093
Kanakiya, S., Adam, L., Esteban, L., Rowe, M. C., and Shane, P. (2017). Dissolution and secondary mineral precipitation in basalts due to reactions with carbonic acid. J. Geophys. Res. Solid Earth 122, 4312–4327. doi: 10.1002/2017JB014019
Kantzas, E. P., Val Martin, M., Lomas, M. R., Eufrasio, R. M., Renforth, P., Lewis, A. L., et al. (2022). Substantial carbon drawdown potential from enhanced rock weathering in the United Kingdom. Nat. Geosci. 15, 382–389. doi: 10.1038/s41561-022-00925-2
Kelland, M. E., Wade, P. W., Lewis, A. L., Taylor, L. L., Sarkar, B., Andrews, M. G., et al. (2020). Increased yield and CO2 sequestration potential with the C4 cereal Sorghum bicolor cultivated in basaltic rock dust-amended agricultural soil. Glob. Chang. Biol. 26, 3658–3676. doi: 10.1111/gcb.15089
Larkin, C. S., Andrews, M. G., Pearce, C. R., Yeong, K. L., Beerling, D. J., Bellamy, J., et al. (2022). Quantification of CO2 removal in a large-scale enhanced weathering field trial on an oil palm plantation in Sabah, Malaysia. Front. Clim. 4:959229. doi: 10.3389/fclim.2022.959229
Lewis, A. L., Sarkar, B., Wade, P., Kemp, S. J., Hodson, M. E., Taylor, L. L., et al. (2021). Effects of mineralogy, chemistry and physical properties of basalts on carbon capture potential and plant-nutrient element release via enhanced weathering. Appl. Geochem. 132:105023. doi: 10.1016/j.apgeochem.2021.105023
Lu, P., Apps, J., Zhang, G., Gysi, A., and Zhu, C. (2024). Knowledge gaps and research needs for modeling CO2 mineralization in the basalt-CO2-water system: a review of laboratory experiments. Earth Sci. Rev. 254:104813. doi: 10.1016/j.earscirev.2024.104813
Lunstrum, A., Berghe, M. V. D., Bian, X., John, S., Nealson, K., and West, A. J. (2023). Bacterial use of siderophores increases olivine dissolution rates by nearly an order of magnitude. Geochem. Perspect. Lett. 25, 51–55. doi: 10.7185/geochemlet.2315
Mayer, S., Wiesmeier, M., Sakamoto, E., Hübner, R., Cardinael, R., Kühnel, A., et al. (2022). Soil organic carbon sequestration in temperate agroforestry systems – a meta-analysis. Agric. Ecosyst. Environ. 323:107689. doi: 10.1016/j.agee.2021.107689
Manning, D. A. C. (2025). Enhanced rock weathering - a nature-based solution for climate mitigation. Green Energy Sustain. 5:0003. doi: 10.47248/ges2505020003
Needham, A. J., Lindsay, J. M., Smith, I. E. M., Augustinus, P., and Shane, P. A. (2011). Sequential eruption of alkaline and sub-alkaline magmas from a small monogenetic volcano in the Auckland volcanic field, New Zealand. J. Volcanol. Geotherm. Res. 201, 126–142. doi: 10.1016/j.jvolgeores.2010.07.017
Oelkers, E. H., Declercq, J., Saldi, G. D., Gislason, S. R., and Schott, J. (2018). Olivine dissolution rates: a critical review. Chem. Geol. 500, 1–19. doi: 10.1016/j.chemgeo.2018.10.008
Oelkers, E. H., and Gislason, S. R. (2001). The mechanism, rates and consequences of basaltic glass dissolution: I. An experimental study of the dissolution rates of basaltic glass as a function of aqueous Al, Si and oxalic acid concentration at 25°C and pH = 3 and 11. Geochim. Cosmochim. Acta 65, 3671–3681. doi: 10.1016/S0016-7037(01)00664-0
Renforth, P. (2012). The potential of enhanced weathering in the UK. Int. J. Greenhouse Gas Control 10, 229–243. doi: 10.1016/j.ijggc.2012.06.011
Rountree, C. L., Vandembroucq, D., Talamali, M., Bouchaud, E., and Roux, S. (2009). Plasticity-induced structural anisotropy of silica glass. Phys. Rev. Lett. 102:195501. doi: 10.1103/PhysRevLett.102.195501
Rowe, M. C., Carey, R. J., White, J. D. L., Kilgour, G., Hughes, E., Ellis, B., et al. (2021). Tarawera 1886: an integrated review of volcanological and geochemical characteristics of a complex basaltic eruption. N. Z. J. Geol. Geophys. 64, 296–319. doi: 10.1080/00288306.2021.1914118
Ruiz-Agudo, E., King, H. E., Patiño-López, L. D., Putnis, C. V., Geisler, T., Rodriguez-Navarro, C., et al. (2016). Control of silicate weathering by interface-coupled dissolution-precipitation processes at the mineral-solution interface. Geology 44, 567–570. doi: 10.1130/g37856.1
Schlesinger, W. H., and Amundson, R. (2019). Managing for soil carbon sequestration: let’s get realistic. Glob. Chang. Biol. 25, 386–389. doi: 10.1111/gcb.14478
Shikazono, N., Takino, A., and Ohtani, H. (2005). An estimate of dissolution rate constant of volcanic glass in volcanic ash soil from the Mt. Fuji area, Central Japan. Geochem. J. 39, 185–196. doi: 10.2343/geochemj.39.185
Skov, K., Wardman, J., Healey, M., McBride, A., Bierowiec, T., Cooper, J., et al. (2024). Initial agronomic benefits of enhanced weathering using basalt: a study of spring oat in a temperate climate. PLoS One 19:e0295031. doi: 10.1371/journal.pone.0295031
Smet, I., Evangelou, E., Tsadilas, C., Hagens, M., Bijma, J., Hartmann, J., et al. (2021). Field trials of enhanced weathering combined with cotton farming in Thessaly, Greece. Conference abstract, Goldschmidt, Lyon, France. doi: 10.7185/gold2021.5033
Strefler, J., Amann, T., Bauer, N., Kriegler, E., and Hartmann, J. (2018). Potential and costs of carbon dioxide removal by enhanced weathering of rocks. Environ. Res. Lett. 13:034010. doi: 10.1088/1748-9326/aaa9c4
Taylor, L. L., Quirk, J., Thorley, R. M. S., Kharecha, P. A., Hansen, J., Ridgwell, A., et al. (2016). Enhanced weathering strategies for stabilizing climate and averting ocean acidification. Nat. Clim. Chang. 6, 402–406. doi: 10.1038/nclimate2882
Tester, J. W., Worley, W. G., Robinson, B. A., Grigsby, C. O., and Feerer, J. L. (1994). Correlating quartz dissolution kinetics in pure water from 25 to 625°C. Geochimica et Cosmochimica Acta, 58, 2407–2420. doi: 10.1016/0016-7037(94)90020-5
Vandeginste, V., Lim, C., and Ji, Y. (2024). Exploratory review on environmental aspects of enhanced weathering as a carbon dioxide removal method. Fortschr. Mineral. 14:75. doi: 10.3390/min14010075
Velbel, M. A. (2009). Dissolution of olivine during natural weathering. Geochim. Cosmochim. Acta 73, 6098–6113. doi: 10.1016/j.gca.2009.07.024
Vieira, L. D., Moreira, A. C., Mantovani, I. F., Honorato, A. R., Prado, O. F., Becker, M., et al. (2021). The influence of secondary processes on the porosity of volcanic rocks: a multiscale analysis using 3D X-ray microtomography. Appl. Radiat. Isot. 172:109657. doi: 10.1016/j.apradiso.2021.109657
Vienne, A., Poblador, S., Portillo-Estrada, M., Hartmann, J., Ijiehon, S., Wade, P., et al. (2022). Enhanced weathering using basalt rock powder: carbon sequestration, co-benefits and risks in a mesocosm study with solanum tuberosum. Front. Clim. 4:869456. doi: 10.3389/fclim.2022.869456
Wang, J., and Wang, S. (2019). Preparation, modification and environmental application of biochar: a review. J. Clean. Prod. 227, 1002–1022. doi: 10.1016/j.jclepro.2019.04.282
Wild, B., Daval, D., Micha, J.-S., Bourg, I., White, C., and Fernandez-Martinez, A. (2019). Physical properties of interfacial layers developed on weathered silicates: a case study based on labradorite feldspar. J. Phys. Chem. C 123, 24520–24532. doi: 10.1021/acs.jpcc.9b05491
Wolff-Boenisch, D., Gislason, S. R., Oelkers, E. H., and Putnis, C. V. (2004). The dissolution rates of natural glasses as a function of their composition at pH 4 and 10.6, and temperatures from 25 to 74°C. Geochim. Cosmochim. Acta 68, 4843–4858. doi: 10.1016/j.gca.2004.05.027
Keywords: enhanced rock weathering, surface area, basalt, carbon sequestration, microscopy, particle size
Citation: Burke TM, Kamber BS and Rowlings D (2025) Microscopic investigation of incipient basalt breakdown in soils: implications for selecting products for enhanced rock weathering. Front. Clim. 7:1572341. doi: 10.3389/fclim.2025.1572341
Edited by:
Ben W. Kolosz, University of Hull, United KingdomReviewed by:
David Manning, Newcastle University, United KingdomFatima Haque, University of Guelph, Canada
Copyright © 2025 Burke, Kamber and Rowlings. This is an open-access article distributed under the terms of the Creative Commons Attribution License (CC BY). The use, distribution or reproduction in other forums is permitted, provided the original author(s) and the copyright owner(s) are credited and that the original publication in this journal is cited, in accordance with accepted academic practice. No use, distribution or reproduction is permitted which does not comply with these terms.
*Correspondence: Balz S. Kamber, YmFsei5rYW1iZXJAcXV0LmVkdS5hdQ==