- 1Department of Chemistry, Università Degli Studi di Milano, Milano, Italy
- 2CNR-SCITEC, Milano, Italy
We have recently shown that simple ammonium ferrates are competent catalyst for the cycloaddition reaction of CO2 to epoxides under moderate reaction conditions (T = 100°C, P(CO2) = 0.8 MPa). We report here that ammonium zincates of general formulae [TBA]2 [ZnX4] (TBA = tetrabutylammonium), simply obtained by treating an ethanolic solution of an appropriate zinc(II) salt with two equivalents of tetrabutylammonium halides, outperform ammonium ferrates in the synthesis of cyclic carbonates under milder reaction conditions (room temperature and atmospheric CO2 pressure). Using [TBA]2[ZnBr4] complex as homogeneous catalyst at 100°C and P(CO2) = 0.8 MPa a 52% conversion of styrene oxide with complete selectivity in styrene carbonate in just 15 min was observed, corresponding to a Turnover frequency (TOF) of 416 h−1. The same catalyst proved to be very active even at room temperature and atmospheric or very moderate CO2 pressures (0.2 MPa), with a quite broad range of substrates, especially in the case of terminal epoxides, with high selectivity towards cyclic carbonate products. The difference in reactivity of terminal and internal epoxides could be exploited using 4-vinylcyclohexene dioxide, where the endocyclic epoxide remained untouched when reacted at room temperature and the formation of the di-carbonate product was observed only at harsher conditions. A multigram scale conversion of propylene oxide was achieved (46 mmol) and the catalyst also proved to be recyclable (3 cycles) by distillation of the product and subsequent addition of fresh reagent, maintaining high conversion values and complete selectivity for propylene carbonate. This simple zinc-based catalytic system, which outperform the recently reported iron-based one by working at much milder conditions, could represent a valuable prospect in both laboratory and industrial scale, combining an inherent cheapness and synthetic easiness that should be deeply considered when the goal is to give value to a waste product as CO2.
Introduction
The growing interest in the use of greenhouse gas CO2 as C1 building block in organic synthesis is strongly correlated to the urgent need to find a solution towards the challenges that we are facing in terms of global carbon emission and the new paradigm in managing the carbon cycle (Martens et al., 2017; Das, 2020). Obviously, the replacement of fossil fuels-based chemistry cannot be the sole solution, but the new technologies based on the substitution of non-sustainable feedstock into renewable ones will help to the transition to a circular economy (Gabrielli et al., 2020; Modak et al., 2020). In this regard, ring strained small heterocycles, such as aziridines and epoxides play a prominent role in the field (Intrieri et al., 2019), since due to the high energy associated with these molecules, reaction with thermodynamically stable CO2 occurs smoothly (Dalpozzo et al., 2019). In particular, the selective formation of cyclic carbonates (Sit et al., 2005; Aomchad et al., 2021a) or polycarbonates (Inoue et al., 1969; Inoue, 1979) from the coupling reaction of epoxides with carbon dioxide represents a highly coveted target and it is amongst the few processes that employ CO2 as C1 feedstock that has been industrialized until now (Liu et al., 2015; Pescarmona, 2021). One the one side, the use of high energy substrates such as epoxides provides the necessary driving force to overcome the thermodynamical stability and kinetic inertness associated with the CO2 molecule, which is the most oxidized form of carbon (Bai et al., 2021). On the other side, in order to achieve a close carbon cycle, catalysis and catalyst design are critical aspect in order to lower the energetic requirements of the reaction and to limit the use of harsh reaction conditions (Keijer et al., 2019). For that reason, a continuous effort, especially in the last decade, has been made to develop new catalysts to promote this useful transformation under mild working conditions and with high efficiency (Shaikh et al., 2018). Generally, both a Lewis acid (LA) and a Lewis base are necessary respectively to activate the epoxide and for the nucleophilic attack that causes its ring opening (Pescarmona, 2021; Bhat and Darensbourg, 2022). Following that, the ring-opened epoxide can undergo either CO2 insertion to form a carbonate or repetitive insertion to lead to polyether formation (Kamphuis et al., 2019b). Especially in the case of less hindered terminal epoxides, once the carbonate is formed after CO2 insertion, a fast backbiting leads to the formation of industrially relevant cyclic carbonates (Schäffner et al., 2010; Besse et al., 2013; Aresta et al., 2016; Sathish et al., 2016). Several very efficient catalytic systems, either homogeneous (Castro-Osma et al., 2016; Rintjema and Kleij, 2017; Della Monica et al., 2018; Della Monica et al., 2019a; Della Monica et al., 2019b; Driscoll et al., 2019; Kamphuis et al., 2019a; Damiano et al., 2020; Della Monica et al., 2020; Maquilón et al., 2020; Aomchad et al., 2021b) or heterogeneous (Liang et al., 2019; Sodpiban et al., 2019, 2021; Wang et al., 2019; Singh Dhankhar et al., 2020; Liu F. et al., 2022; Liu M. et al., 2022), have recently been developed, where the former generally possess higher activities but lack in recyclability. Very often the actual catalyst act as the Lewis acid and in most cases a co-catalyst, typically organic halides, such as quaternary ammonium (Caló et al., 2002; Wang et al., 2012, 2021; Montoya et al., 2015) or bis(triphenylphosphine)iminium salts (Sit et al., 2005), was added to observe good reactivities. It is worth to note that in past literature, when a combination of a Lewis acid (catalyst) and a Lewis base (co-catalyst) have been used to promote the coupling reaction between CO2 and epoxides, TOF values have been calculated only considering the amount of added catalyst, neglecting the role played by the sole Lewis base (Campisciano et al., 2020). In the search of more efficient catalysts, many recent efforts have been done in the design of materials embedding both Lewis acidic and basic catalytic sites, for the CO2 cycloaddition reaction under milder reaction conditions, without the addition of any co-catalyst and both homogeneous (Tong et al., 2022) and heterogeneous catalysts (Nguyen et al., 2022; Su et al., 2022) have been reported especially in the last years. It should be noted that most of these catalysts work under solvent-free and ambient pressure CO2 reaction conditions, however the temperatures required to observe good conversion of the starting epoxide are in the range 80–120°C.
We have recently exploited the reactivity of tetrabutylammonium ferrates of the general formulae [TBA][FeX3Y] (TBA = nBu4N, X, Y = Cl, Br), that can be obtained from inexpensive chemicals such as tetrabutylammonium halides and ferric salts (Wyrzykowski et al., 2006, 2007), as stand-alone catalysts in the CO2 cycloaddition to epoxides (Panza et al., 2022). The effect of the experimental factors (reaction temperature, CO2 pressure, type of nucleophile and recycling of the catalyst), together with a full set of theoretical calculations, were studied in depth. Good yields of cyclic carbonates were obtained, especially for terminal epoxides with a broad reaction scope. Reaction conditions employed were quite mild, however, CO2 pressures between 0.4 and 0.8 MPa and temperatures between 100 and 150°C were needed in order to observe full conversion with high selectivity. We report here that analogous tetrabutylammonium zincates are competent catalysts for the same selective transformation even at room temperature.
Results and discussion
Synthesis of the ammonium zincates [TBA]2 [ZnX4] (X = cl, Br, I)
A series of ammonium zincates, [TBA]2[ZnX4], (X = Cl, Br, I) was synthesized by simply treating an ethanolic solution of 2 equivalents of the appropriate tetrabutylammonium halide with 1 equivalent of the zinc salt. As detailed in the Materials and methods section and in the Supplementary Material, ammonium zincates were obtained in good yields and purity by simple recrystallization from cold methanol (-20°C). The purity was confirmed by elemental analyses, whilst accurate high resolution mass spectra were carried out in CH3CN with the double aim to assess the proposed structure and to study the equilibria between the undissociated dianion [ZnX4]2- and the solvated forms ([ZnX3]- + X−) and (ZnX2 + 2X−). This kind of equilibria between “ate” and neutral salts and its relevance to the nucleophilic ring opening of epoxides has been already disclosed by us in the case of the tetrabutylammonium ferrates (Panza et al., 2022), and it was already predicted by Capacchione and co-workers in the case of [FeBr4]- anion (Della Monica et al., 2019b). Recently it has been also disclosed the existence of such an equilibrium by Baalbaki et al. in the case of indium bromide (Baalbaki et al., 2021). In the case of the tetrabutylammonium zincates, by ESI(-)-HRMS the expected dianion [ZnX4]2- was not found, but instead a more persistent monoanion [ZnX3]- was detected in the case of X = Cl, Br, while in the case of [ZnI4]2- only I− and I3- were detected most likely due to the higher lability of the compound. Table 1 collects the yields of the tetrabutylammonium zincates synthesized and used as catalyst in the CO2 cycloaddition to epoxides, whilst for their synthesis and characterization (elemental analyses and HRMS), the reader is referred to the Supplementary Material.
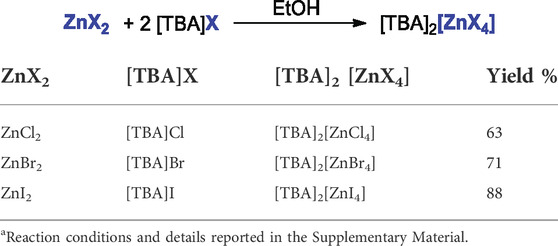
TABLE 1. Synthesis and characterization of the ammonium zincatesa.
Zincates catalyzed cyclic carbonate synthesis
We used styrene epoxide (SO), 1a, as the benchmark substrate to optimize the reaction conditions by employing the different tetrahalogenozincates salts in solvent-free conditions. Since the optimized conditions with the recently reported “ferrate” catalysts were found to be 0.5 mol% catalyst loading at 100°C and under 0.8 MPa of CO2, initially we set there the starting point for the optimization using tetrachloro-, tetrabromo- and tetraiodo-zincates. Under the previously described conditions, when using [TBA][FeCl3Br] as the catalyst, 83% of SO was converted into styrene carbonate (SC), 2a, in 4 h with 95% selectivity (Panza et al., 2022). To our delight, all the zincate catalysts tested gave instead quantitative conversions of the starting epoxide in 4 h reaction time, with excellent selectivity in the case of the bromide and iodide salts (94 and 95% respectively, entries 2 and 3, Table 2). We should emphasize, however, that in this case 0.5 mol% of the catalyst contains the double of equivalents of the ammonium cation with respect to the monoanionic ferrates analogues, but we already showed that its role is limited essentially to a rigid shift of all energies towards lower values (Panza et al., 2022). When we reduced the reaction time at 2h, we still observed a quantitative conversion, except for [TBA]2[ZnCl4] as catalyst, with selectivity in the range 91–98% (entries 4–6, Table 2). The most promising catalyst resulted to be the [TBA]2[ZnBr4] complex, that in just 1 h of reaction converted 98% of starting 1a with 96% selectivity in 2a (entry 8, Table 2). As we already noticed in the case of [Zn(II)pyclen] complexes reported recently as efficient catalysts for the chemical fixation of CO2 with epoxides (Cavalleri et al., 2021), the chloro-zincate salt was the less active one. Among the three zincates, the activity increased in the order X = Cl− < I− < Br− (compare entries 7, 8 and 9, Table 2) and this trend can be rationalized considering the following considerations regarding the overall catalytic activity: 1) lability of the halide from the zincate anion (vide supra, ESI(-)-HRMS analyses); 2) the nucleophilicity of the halide in the ring opening of the epoxide; and 3) the halide leaving group ability, in order to promote the cyclic carbonate formation in the ring closing step (Kamphuis et al., 2019a). Given the aprotic media in which the reactions are run, we must assume that the nucleophilicity increases in the order I− < Br− < Cl−, whereas the leaving ability decreases in the same order. Bromide seemed to provide the best balance between these three properties, as already observed by us also for related ferrate complexes (Panza et al., 2021), thus leading to the best observed catalytic activities. For instance, using [TBA]2[ZnBr4] salt as catalyst, a 52% conversion in just 15 min was observed, corresponding to the remarkable Turnover frequency (TOF) of 416 h−1 (Entry 14, Table 2).
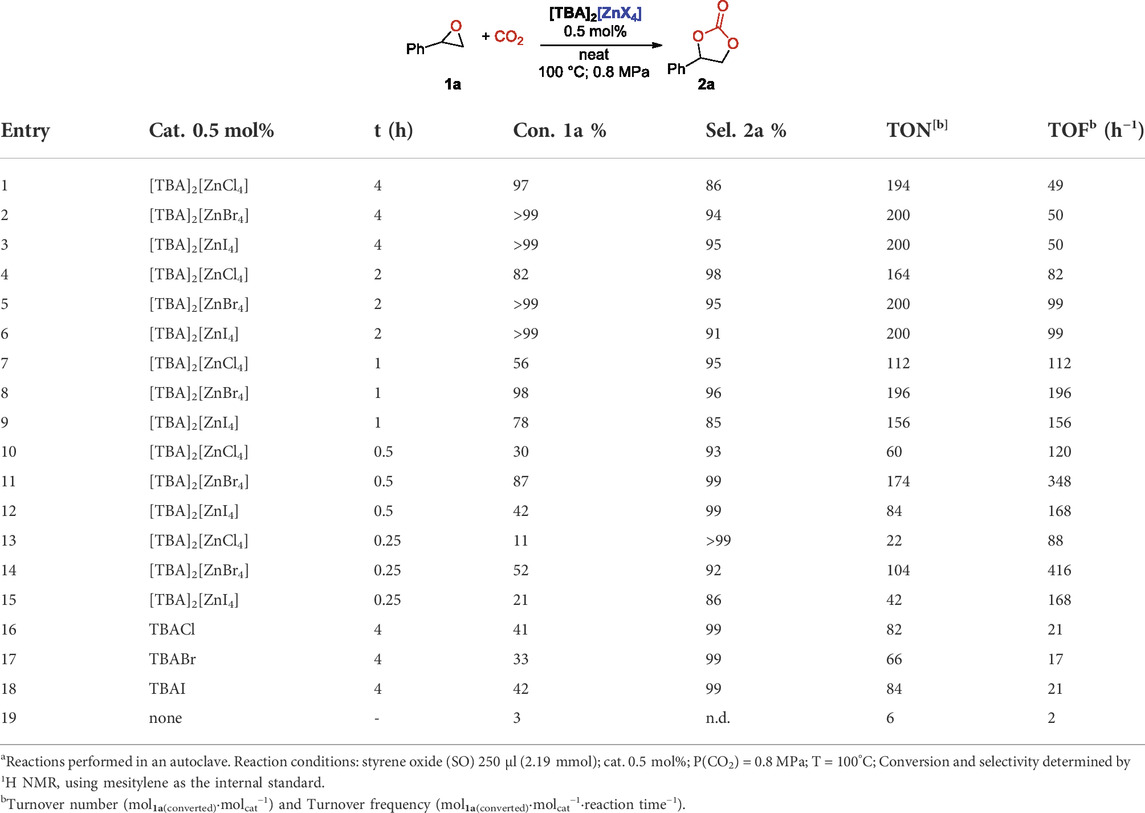
TABLE 2. Cycloaddition of CO2 to styrene oxide catalysed by the tetrahalogenozincate-saltsa.
It is worth to note that, as known from the literature, the activity of simple quaternary ammonium halides, i.e., TBAX, in this reaction is not negligible (Caló et al., 2002; Wang et al., 2012; Montoya et al., 2015). However, we have already shown that under the same reaction conditions, both TBACl and TBABr are underperforming, albeit maintaining very high selectivity (Panza et al., 2022). We repeated the reaction under the same catalytic conditions by using TBAI that performed comparably to the others ammonium halides and gave only a 42% conversion with 95% selectivity in 2a (See Supplementary Material). Finally, it should be pointed out that it has been reported that mixtures of metal halides and alkylammonium iodides can act as suitable catalyst in the cyclic carbonate synthesis (Kisch et al., 1986). However, ammonium zincates are less hygroscopic and easier to handle than the respective starting materials (zinc and tetrabutyl ammonium halides) and we have recently shown that in the case of tetrabutyl ammonium ferrates (Panza et al., 2022), a mixture of an iron (III) salt with tetrabutyl ammonium halides indeed act as a catalyst, but with lower conversion and TOF with respect to the pre-formed ammonium ferrate, so that there is no advantage in their in situ synthesis.
Effect of the temperature and the CO2 pressure
With the aim to find milder reaction conditions, we next studied the effect of lower temperatures and CO2 pressures on the reaction outcome. Initially we reduced the CO2 pressure to 0.1 MPa and reactions were performed in sealed vials with a CO2 balloon at 100°C. In all cases lower conversion but especially lower selectivity, due to competing rearrangement side reactions of the starting SO, were observed (entries 1–3, Table 3). When the same reactions were repeated at T = 50°C, given to the better solubility of CO2 under these conditions, selectivity was again improved, albeit at the cost of lower conversion (entries 4–6, Table 3). Finally, we tested the reactivity of the catalytic system at room temperature (we set an equilibrating bath at 30°C in order to have reproducible results) and under CO2 pressure (0.8 MPa) in autoclave. In this case we used a 1 mol% catalyst loading and we extended the reaction time to 16 h. Again, the less active catalyst resulted to be the chloro-zincate salt, that gave only a 11% conversion of the starting epoxide (entry 7, Table 3), whilst both [TBA]2[ZnBr4] and [TBA]2[ZnI4] gave almost quantitative conversions with full selectivity towards 2a (entries 8 and 9, Table 3).
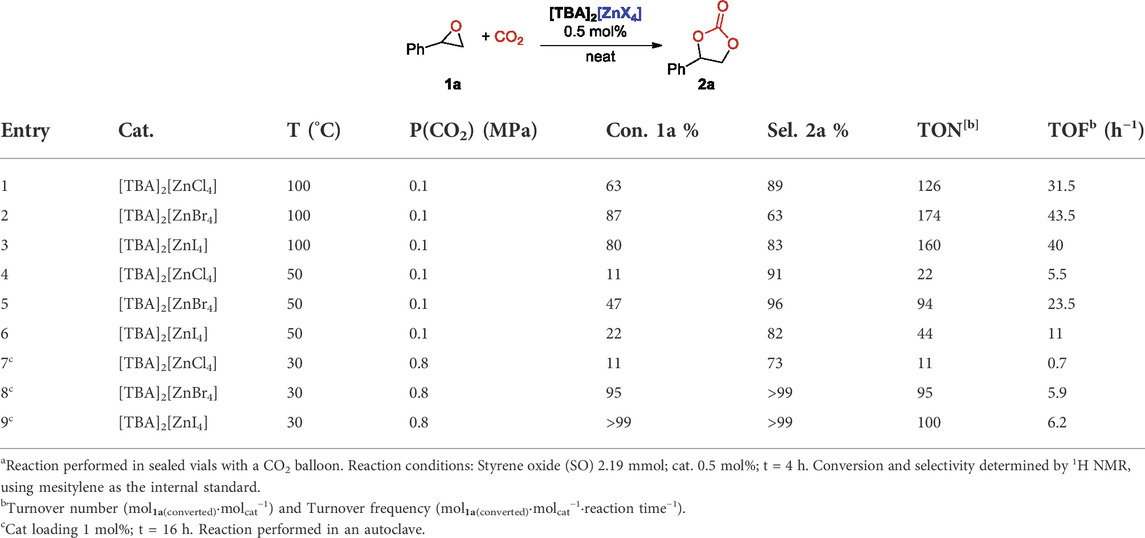
TABLE 3. Cycloaddition of CO2 to styrene oxide: Effect of the pressure and the temperaturea.
Effect of the catalyst loading
Since the best compromise between activity and selectivity was always found with [TBA]2[ZnBr4] as the catalyst, we decided to further optimize the reaction conditions and to study the scope of the reaction using this complex. Our aim was to find the mildest conditions to run the reaction, with the best compromise between catalyst loading, temperature and CO2 pressure. To do so, we initially set room temperature (25°C) and atmospheric CO2 pressure as the target and we changed the catalyst loading in order to maximize the yield of SC 2a. Reactions were performed by assuring CO2 atmosphere with a balloon. We noticed that in 24 h of reactions, the conversion observed of starting SO 1a was not linearly correlated with the catalyst loading. If 0.5 mol% of catalyst at 25°C converted 34% of the starting epoxide with good selectivity, with a reasonable TOF of 2.8 h−1, a double amount of the catalyst gave only the 47% of conversion, with a TOF of 2.0 h−1 (entries 1 and 2, Table 4). Surprisingly, a 5 mol% amount of catalyst gave the same conversion (entry 3, Table 4), corresponding to a TOF of only 0.4 h−1. This negative result was not justified by solubility limits of the zincate salt in neat epoxide 1a. We reasoned that this effect might be due to inhibition of the catalyst by the product formation or to the fact that despite the presence of the balloon, CO2 concentration after prolonged reaction times started to diminish. It is known that rubber balloons are not gas-tight and that carbon dioxide may leak through (Edwards and Pickering, 1920). To test this hypothesis, we repeated the same reaction, but working under CO2 flow at atmospheric pressure and we observed an increased conversion (69%) of starting 1a, with complete selectivity in favor of the SC, 2a, and with an increased TOF of 4.3 h−1 (entry 5, Table 4).
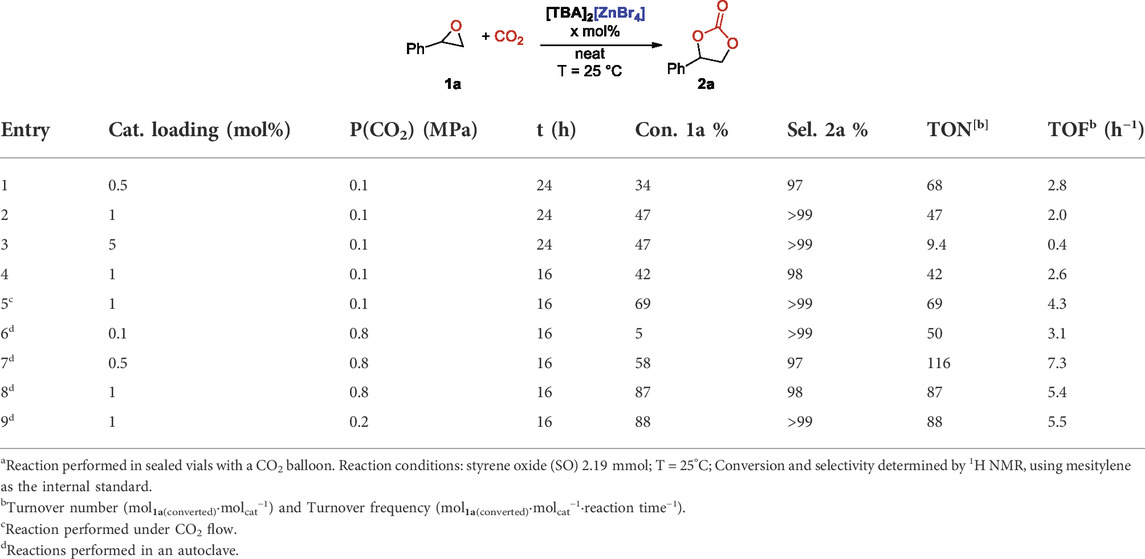
TABLE 4. Cycloaddition of CO2 to styrene oxide: Effect of the catalyst loadinga.
Since the CO2 wasted working under constant bubbling is however a limiting factor, we next monitored the effect of the catalyst loading working at room temperature but increasing CO2 pressure up to 0.8 MPa in autoclave (entries 6–8, Table 4). A loading of just 0.1 mol% of the catalyst was too low and only a 5% of conversion was observed, whilst even at room temperature a gratifying TOF of 7.3 h−1 was observed with a 0.5 mol% of [TBA]2[ZnBr4]. The best compromise between conversion and selectivity was obtained by using a 1 mol% loading of the catalyst. When we repeated the same reaction with just 0.2 MPa of CO2 pressure, we obtained almost identical results (entry 9, Table 4), proving that pressure is not a limiting factor while providing a sufficient quantity of CO2 and thus we set those as the optimal conditions to further study the reaction scope.
Reaction scope
Having in hand the best catalyst, [TBA]2[ZnBr4], and the optimized reaction conditions, room temperature (T = 30°C), solvent free and 0.2 MPa of CO2 pressure, we next studied the reaction scope by changing the steric and electronic factors of the starting epoxide. Results are summarized in Table 5. All terminal epoxides tested were well tolerated and cyclic carbonates were formed in good to excellent yields. Surprisingly, the activated epichlorohydrin 1b, in the optimized reaction conditions gave only a modest 64% conversion, albeit with full selectivity for the cyclic carbonate 2b (entry 1, Table 5). Linear alkyl substituted epoxides, 1c-e, were all transformed with high selectivities (>99%) into cyclic carbonates, 2c-e. The best results in terms of conversion, with a remarkable TOF of 6.2 h−1, were observed in the case of 1,2-epoxyhexane 2e (entry 5, Table 5). The observed reactivity trend is the opposite to that we have found for the related cycloaddition reaction of CO2 to epoxide catalyzed by [TBA][FeCl3Br], where we observed a decreased reactivity of the catalytic system in less polar media (Panza et al., 2022). We thus repeated the reaction of propylene epoxide, 1c, at the same temperature but with an increased CO2 pressure (1 MPa) and we observed a slightly improved conversion rate (entry 3, Table 5). Several glycidyl ethers, 1f-j, were also tested and very high conversion with excellent selectivity in favor of the cyclic carbonate was observed (entries 6–10, Table 5). The only exceptions were phenyl glycidyl ether, 1g, and 2-methylphenyl glycidyl ether, 1h, were an off-white tar, most probably due to polymeric material, was also formed in 24% selectivity. The presence of a coordinating heterocyclic group such as in the case of furfuryl glycidyl ether, 1j, was well tolerated and a TOF of 5.4 h−1 was observed (entry 10, Table 5). Internal or more sterically hindered epoxides, as expected (Kamphuis et al., 2019a), gave less satisfactory results and trans-Stilbene epoxide, 1k, almost failed to react (entry 12, Table 5). It should be noted, however, that in this case, since this product is solid at room temperature, CH3CN (0.5 ml) was added to the reaction mixture as the solvent. 1,2-Epoxy-methylpropane, 1l, and cyclohexene oxide, 1m, on the other hand could be converted to the corresponding cyclic carbonates only under harsher reaction conditions (T = 100°C, P(CO2) = 0.8 MPa, entries 13 and 15, Table 5). However, it should be emphasized that, especially in the case of cyclohexene oxide, generally considered a less reactive epoxide (Della Monica et al., 2019a), when increasing the pressure to 1.6 MPa and running the reaction for 16 h we observed a remarkable yield (80%; 89% selectivity) of cyclic carbonate 2i, and no formation of any polymeric material (entry 16, Table 5). A trisubstituted epoxide such as limonene oxide, 1n, failed to react also under those harsher reaction conditions (entry 17, Table 5).
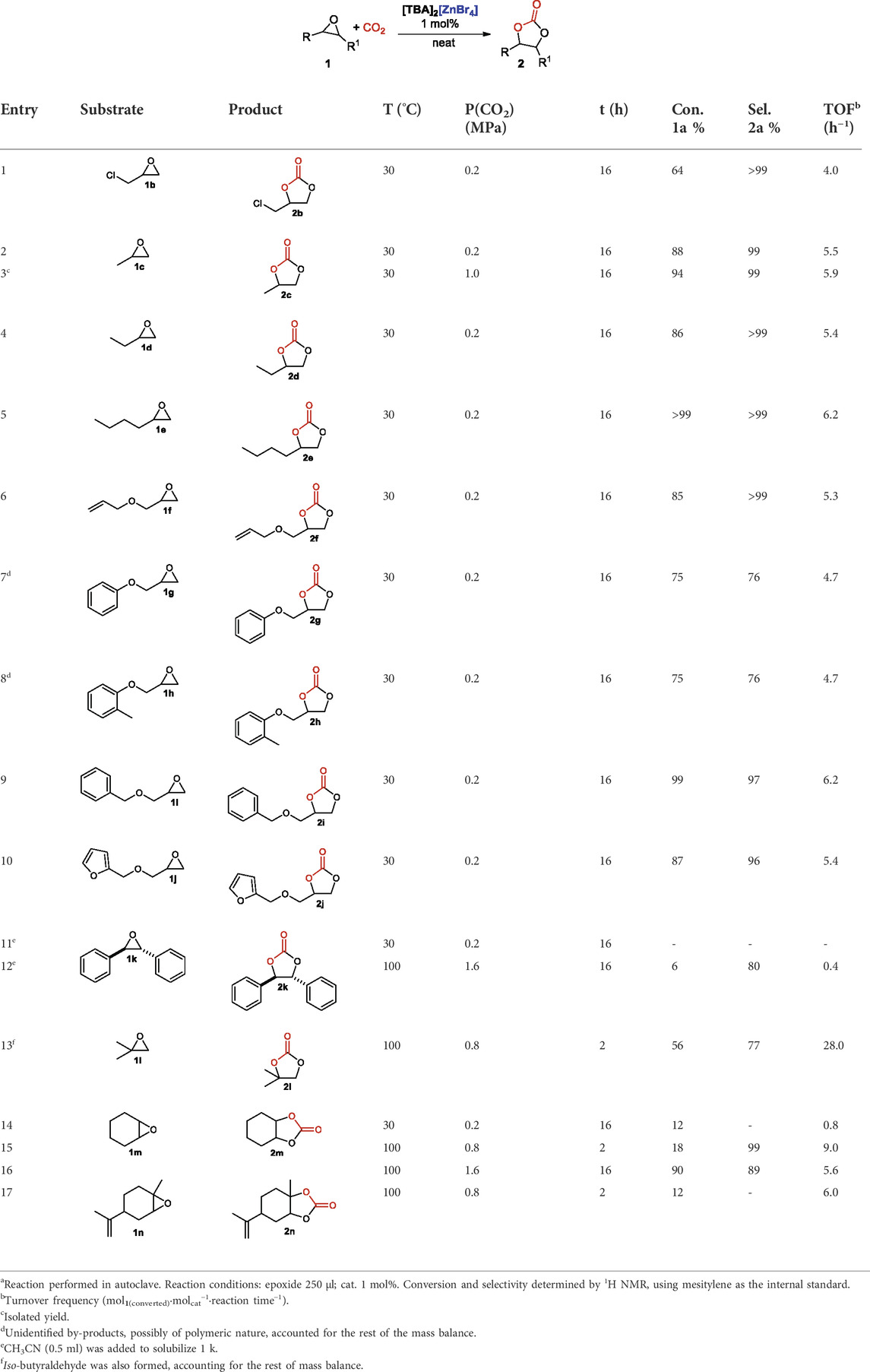
TABLE 5. Reaction scopea.
This difference in reactivity between terminal and more sterically hindered epoxides could be switched from a disadvantage to a selective useful tool. For example, when we reacted 4-vinylcyclohexene dioxide, 1o, containing both a terminal and an internal epoxide, at room temperature and low CO2 pressure, (0.2 MPa), we observed a 78% conversion of the starting material with the selective formation of the cyclic carbonate at the less substituted epoxide, 2o (Scheme 1A). When the reaction was repeated with a double amount of catalyst (1 mol% of [TBA]2[ZnBr4] for each epoxide present in the substrate) and under harsher reaction conditions, T = 100°C and 0.8 MPa of CO2, we observed a quantitative conversion of the starting epoxide, with a 72% selectivity in favor of the mono-cyclic carbonate, 2o, and a 28% selectivity for the di-carbonate product 2o’ (Scheme 1B), proving that modulating the reaction conditions can affect strongly the chemo-selectivity of such reaction.
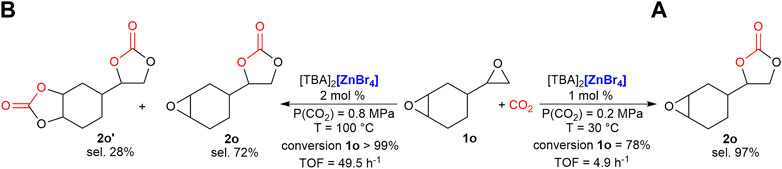
SCHEME 1. Selective synthesis of mono (A) and di-cyclic carbonates (B) of 4-vinylcyclohexene dioxide, 1°. Reaction conditions: epoxide 250 μl (1.94 mmol); cat. 1 or 2 mol%. Conversion and selectivity determined by 1H NMR using mesitylene as the internal standard. Turnover frequency (mol1o(converted)·molcat −1·reaction time−1).
Finally, other two terminal di-epoxides were tested as substrates for their possible application as non-isocyanate polyurethane monomers (Rix et al., 2016). In both cases, CH3CN was successfully employed as solvent and 2 mol% of catalyst loading was employed (1 mol% per mol of epoxide moiety). The bis-carbonate of (bisphenol-A)diglycidyl ether (BADGE), 2p, was obtained in 64% yield (85% selectivity, 75% conversion of starting 1p) in 16 h at 30°C. To the best of our knowledge, this is the highest yield obtained under such mild reaction conditions for this very interesting product (Scheme 2). Under the same reaction conditions, 1,4-bis(benzyloxy)diglycidyl ether was converted in the bis-carbonate product 2q with a 52% yield (91% selectivity, 57% conversion of starting 1o, Scheme 3), outperforming our previously reported synthesis employing ferrates as catalysts (Panza et al., 2022).
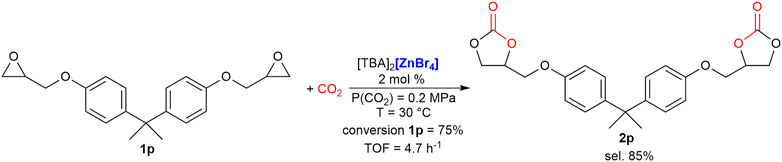
SCHEME 2. Selective synthesis of bis-carbonate of (bisphenol-A)diglycidyl ether (BADGE), 2p. Reaction conditions: epoxide, 1p, (340 mg, 1 mmol) dissolved in CH3CN (0.5 ml); cat. 2 mol%. Conversion and selectivity determined by 1H NMR using mesitylene as the internal standard. Turnover frequency (mol1p(converted)·molcat −1·reaction time−1).
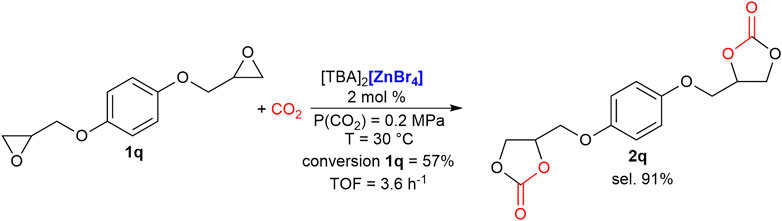
SCHEME 3. Selective synthesis of bis-carbonate of 1,4-bis(benzyloxy)diglycidyl ether, 2q. Reaction conditions: epoxide, 1q, (222 mg, 1 mmol) dissolved in CH3CN (0.5 ml); cat. 2 mol%. Conversion and selectivity determined by 1H NMR using mesitylene as the internal standard. Turnover frequency (mol1q(converted)·molcat −1·reaction time−1).
Scale-up and recycling
The ability of a catalytic system to undergo a scale-up reaction is an important feature that gives a preliminary idea about a possible industrial application. For this reason, we tested [TBA]2[ZnBr4] in the cycloaddition of propylene oxide 1c and CO2 in a multigram scale reaction, using 1 mol% catalyst loading and 3.24 ml (46 mmol) of PO at 1.0 MPa CO2 pressure, T = 30°C for 16 h. The product mixture was weighted and analyzed by 1H NMR analysis. At the end of the reaction only propylene carbonate 2c was recovered with a remarkable isolated yield of 94%, corresponding to 4.250 g of PC. Moreover, the possibility to recycle the catalyst for further reactions is indeed crucial, even if not always simple in the case of homogeneous systems. In our case, the product of the scale up reaction was distilled in vacuum to obtain pure propylene carbonate and pure [TBA]2[ZnBr4]. To the latter, 46 mmol of fresh propylene oxide were added and the mixture was subjected to the same procedure described before. The product was obtained and analyzed as previously detailed, obtaining again propylene carbonate as the sole product (selectivity for 2c >99%). The robustness of the catalyst was assured for a total of three cycles, after which a total of amount of 12.65 g of pure 2c was obtained (results are summarized in the Supplementary Material) [TBA]2[ZnBr4] as a catalyst proved to be recyclable without losing activity nor selectivity, making it an attractive tool for a possible further large-scale study.
Conclusion
In summary, we have shown that very simple inexpensive tetrabutylammonium zincates are efficient catalyst, without the need of any co-catalyst and in the absence of any solvent, for the cycloaddition of CO2 to epoxides under mild reaction conditions. The bifunctional nature of the catalyst, both as Lewis acid and nucleophilic Lewis base, is assured by the “ate” equilibrium between the dianoinic zincate salt and the monoanionic [ZnX3]- moiety and the halide anion. We have recently reported a theoretical calculation of the reaction mechanism and the role played by the combination of the Lewis acid (iron salt) and nucleophile (halide ion) in the case of the ammonium ferrate catalyzed cycloaddition of CO2 to epoxides (Panza et al., 2022). The formation of the cyclic carbonate can be schematized as occurring in three consecutive steps, in agreement with literature results (Pescarmona, 2021): 1) the halide anion act as the nucleophile attacking a carbon atom of the epoxide ring, which opens by breaking a C-O bond in a concerted mechanism; 2) the oxygen atom of the former epoxide attacks the C atom of carbon dioxide thus forming an open carbonate species; 3) the carbonate closes the ring and the formation of a C-O bond induces simultaneous breaking of the carbon - halide bond, releasing the halide ion. Although DFT calculations were not performed in the present case, we must assume that the also in this case zinc act as a Lewis acid lowering the barrier for the ring opening of the epoxide and stabilizing the first reaction intermediate (the open epoxide species). When no Lewis acid is present, the rate determining step of the whole process is the epoxide ring-opening. On the other hand, when the zinc atom interacts with the oxygen of the epoxide, this transition state is lowered in energy and the rate determining step becomes the ring closure to give the cyclic carbonate.
The best catalytic performances have been obtained by using the bromide-zincate [TBA]2[ZnBr4], which can be conveniently prepared in high yield and purity by mixing an ethanolic solution of ZnBr2 with TBABr, and a TOF of 416 h−1 in the styrene carbonate synthesis at T = 100°C has been observed. It should be emphasized, from a practical point of view, that the handling of the zincates salts, that are less hygroscopic than their staring materials, is easy and does not require any special caution. Moreover, all the zincate tested proved to be quite robust and at the end of the reaction they can be recovered by simply distilling off the organic products formed (cyclic carbonates). Remarkably, quantitative conversion of terminal epoxides with full selectivity towards the cyclic carbonate have been obtained at low temperature (T = 30°C) and under just 0.2 MPa of CO2 pressure. Reactions occur smoothly also at room temperature and under atmospheric CO2, at a big difference from most recently reported homogeneous (Tong et al., 2022) and heterogeneous (Nguyen et al., 2022; Su et al., 2022) systems operating without any co-catalyst added and in solvent free conditions, that normally are reported to be most performing at T 80–120°C.
Finally, the recyclability of the [TBA]2[ZnBr4] salt has been assessed by distilling off the pure propylene carbonate formed in the reaction with propylene oxide and restoring the catalytic cycle three times without any loss of catalytic activity observed. Reaction could also be scaled up and a total amount of 12.65 g of pure PC could be isolated with a global TON of 279. Based on these results, we think that amongst the several homogeneous catalysts reported in the last years for the synthesis of cyclic carbonates by cycloaddition of CO2 to epoxides, terabutylammonium zincates represents a considerable case of study for highly efficient greenhouse gas re-utilization.
Materials and methods
General considerations
All chemicals and solvents were commercially available and used as received except where specified. 1H NMR analyses were performed with 400 MHz spectrometers at room temperature. The coupling constants (J) are expressed in hertz (Hz), and the chemical shifts (δ) in ppm. Catalytic tests were analysed by 1H NMR spectroscopy. Low resolution MS spectra were acquired with instruments equipped with ESI/ion trap sources. High resolution MS spectra were acquired on a Q-ToF SYNAPT G2-Si HDMS 8K instrument (Waters) equipped with a ZsprayTM ESI source (Waters). The values are expressed as mass−charge ratio and the relative intensities of the most significant peaks are shown in brackets. Elemental analyses were recorded in the analytical laboratories of Università degli Studi di Milano. The collected data for all the cyclic carbonate reported are in accordance with those reported in literature (Yu et al., 2021; Panza et al., 2022).
Synthesis of the zincate salts
All the tetrahalogenozincate salts were prepared following a slightly modified procedure reported for the synthesis of the ferrate analogues (Panza et al., 2022), by mixing in an appropriate stoichiometric ratio an ethanolic solution of TBAX and ZnX2 with good yield, after recrystallization (Table 1). All the so-prepared materials were analysed by Elemental Analysis and HRMS. All the details about the synthesis and analyses can be found in the Supplementary Material.
General catalytic procedure in autoclave
A 250 ml stainless steel autoclave reactor was equipped with three 2.5 ml glass vials, containing the catalyst/epoxide mixture (250 µL of substrate). The vials were equipped with magnetic stirring bars and sealed with specific caps. The autoclave was then charged with a specific amount of CO2 and placed in a thermostated heating bath for a specific amount of time. The reactor was then cooled to room temperature (when needed) and the CO2 released. To each vial the appropriate amount of the internal standard (mesitylene) and 0.5 ml of CDCl3 were added to perform quantitative 1H NMR analysis.
General catalytic procedure in sealed vials
Reactions performed at ambient pressure of CO2 were placed in glass vials containing the epoxide, the catalyst and a magnetic stirring bar, sealed with a silicon septum and aluminium cap. A CO2 balloon, sealed to a plastic syringe, was inserted in the vial using a needle to ensure the pressure of CO2. At the end of the reaction, the appropriate amount of the internal standard (mesitylene) and 0.5 ml of CDCl3 were added to perform quantitative 1H NMR analysis.
Data availability statement
The original contributions presented in the study are included in the article/Supplementary Material, further inquiries can be directed to the corresponding author.
Author contributions
NP: Data curation and experimental procedures, Writing–original draft, Visualization. MA: Data curation and experimental procedures. CD: Data analysis and Writing—review and editing. AC: Conceptualization, Methodology, Writing—original draft, review and editing, Supervision, Project administration, Funding acquisition. All the authors critically revised and approved the final version of the manuscript.
Funding
This work was supported by the University of Milan (PSR 2020—financed project ‘‘Catalytic strategies for the synthesis of high added-value molecules from bio-based starting materials’’).
Acknowledgments
This research is part of the project “One Health Action Hub: University Task Force for the resilience of territorial ecosystems”, Supported by Università degli Studi di Milano—PSR 2021-GSA-Linea 6. We thank the MUR-Italy (Ph.D. fellowships to NP). Unitech—COSPECT (https://www.cospect.it/), Università degli Studi di Milano, is gratefully acknowledged for ESI (-) HRMS analyses. The authors acknowledge support from the University of Milan through the APC initiative.
Conflict of interest
The authors declare that the research was conducted in the absence of any commercial or financial relationships that could be construed as a potential conflict of interest.
Publisher’s note
All claims expressed in this article are solely those of the authors and do not necessarily represent those of their affiliated organizations, or those of the publisher, the editors and the reviewers. Any product that may be evaluated in this article, or claim that may be made by its manufacturer, is not guaranteed or endorsed by the publisher.
Supplementary material
The Supplementary Material for this article can be found online at: https://www.frontiersin.org/articles/10.3389/fctls.2022.991270/full#supplementary-material
References
Aomchad, V., Cristòfol, À., Della Monica, F., Limburg, B., D’Elia, V., and Kleij, A. W. (2021a). Recent progress in the catalytic transformation of carbon dioxide into biosourced organic carbonates. Green Chem. 23, 1077–1113. doi:10.1039/d0gc03824e
Aomchad, V., del Gobbo, S., Yingcharoen, P., Poater, A., and D’Elia, V. (2021b). Exploring the potential of group III salen complexes for the conversion of CO2 under ambient conditions. Catal. Today 375, 324–334. doi:10.1016/J.CATTOD.2020.01.021
Aresta, M., Dibenedetto, A., and Quaranta, E. (2016). State of the art and perspectives in catalytic processes for CO2 conversion into chemicals and fuels: The distinctive contribution of chemical catalysis and biotechnology. J. Catal. 343, 2–45. doi:10.1016/j.jcat.2016.04.003
Baalbaki, H. A., Roshandel, H., Hein, J. E., and Mehrkhodavandi, P. (2021). Conversion of dilute CO2 to cyclic carbonates at sub-atmospheric pressures by a simple indium catalyst. Catal. Sci. Technol. 11, 2119–2129. doi:10.1039/d0cy02028a
Bai, S. T., de Smet, G., Liao, Y., Sun, R., Zhou, C., Beller, M., et al. (2021). Homogeneous and heterogeneous catalysts for hydrogenation of CO2 to methanol under mild conditions. Chem. Soc. Rev. 50, 4259–4298. doi:10.1039/d0cs01331e
Besse, V., Camara, F., Voirin, C., Auvergne, R., Caillol, S., and Boutevin, B. (2013). Synthesis and applications of unsaturated cyclocarbonates. Polym. Chem. 4, 4545–4561. doi:10.1039/C3PY00343D
Bhat, G. A., and Darensbourg, D. J. (2022). Progress in the catalytic reactions of CO2 and epoxides to selectively provide cyclic or polymeric carbonates. Green Chem. 24, 5007–5034. doi:10.1039/d2gc01422j
Caló, V., Nacci, A., Monopoli, A., and Fanizzi, A. (2002). Cyclic carbonate formation from carbon dioxide and oxiranes in tetrabutylammonium halides as solvents and catalysts. Org. Lett. 4, 2561–2563. doi:10.1021/ol026189w
Campisciano, V., Calabrese, C., Giacalone, F., Aprile, C., lo Meo, P., and Gruttadauria, M. (2020). Reconsidering TOF calculation in the transformation of epoxides and CO2 into cyclic carbonates. J. CO2 Util. 38, 132–140. doi:10.1016/j.jcou.2020.01.015
Castro-Osma, J. A., Lamb, K. J., and North, M. (2016). Cr(salophen) complex catalyzed cyclic carbonate synthesis at ambient temperature and pressure. ACS Catal. 6, 5012–5025. doi:10.1021/acscatal.6b01386
Cavalleri, M., Panza, N., Biase, A., Tseberlidis, G., Rizzato, S., Abbiati, G., et al. (2021). [Zinc(II)(Pyridine-Containing ligand)] complexes as single-component efficient catalyst for chemical fixation of CO2 with epoxides. Eur. J. Org. Chem. 2021, 2764–2771. doi:10.1002/ejoc.202100409
Dalpozzo, R., della Ca, N., Gabriele, B., and Mancuso, R. (2019). Recent advances in the chemical fixation of carbon dioxide: A green route to carbonylated heterocycle synthesis. Catalysts 9, 511. doi:10.3390/catal9060511
Damiano, C., Sonzini, P., Intrieri, D., and Gallo, E. (2020). Synthesis of cyclic carbonates by ruthenium(VI) bis -imido porphyrin/TBACl-catalyzed reaction of epoxide with CO2. J. Porphyr. Phthalocyanines 24, 809–816. doi:10.1142/S1088424619501888
Das, S. (2020). CO2 as a building block in organic synthesis. (Weinheim, Germany: John Wiley & Sons). doi:10.1002/9783527821952
Della Monica, F., Maity, B., Pehl, T., Buonerba, A., de Nisi, A., Monari, M., et al. (2018). [OSSO]-Type iron(III) complexes for the low-pressure reaction of carbon dioxide with epoxides: Catalytic activity, reaction kinetics, and computational study. ACS Catal. 8, 6882–6893. doi:10.1021/acscatal.8b01695
Della Monica, F., Buonerba, A., and Capacchione, C. (2019a). Homogeneous iron catalysts in the reaction of epoxides with carbon dioxide. Adv. Synth. Catal. 361, 265–282. doi:10.1002/adsc.201801281
Della Monica, F., Buonerba, A., Paradiso, V., Milione, S., Grassi, A., Capacchione, C., et al. (2019b). [OSSO]-Type Fe(III) metallate as single-component catalyst for the CO2 cycloaddition to epoxides. Adv. Synth. Catal. 361, 283–288. doi:10.1002/adsc.201801240
Della Monica, F., Paradiso, V., Grassi, A., Milione, S., Cavallo, L., and Capacchione, C. (2020). A novel [OSSO]-Type chromium(III) complex as a versatile catalyst for copolymerization of carbon dioxide with epoxides. Chem. Eur. J. 26, 5347–5353. doi:10.1002/chem.201905455
Driscoll, O. J., Hafford-Tear, C. H., McKeown, P., Stewart, J. A., Kociok-Köhn, G., Mahon, M. F., et al. (2019). The synthesis, characterisation and application of iron(iii)-acetate complexes for cyclic carbonate formation and the polymerisation of lactide. Dalton Trans. 48, 15049–15058. doi:10.1039/c9dt03327k
Edwards, J. D., and Pickering, S. F. (1920). Permeability of rubber to gases. Sci. Pap. Bureau Stand. 16, 327–361. doi:10.6028/nbsscipaper.062
Gabrielli, P., Gazzani, M., and Mazzotti, M. (2020). The role of carbon capture and utilization, carbon capture and storage, and biomass to enable a net-zero-CO2 emissions chemical industry. Ind. Eng. Chem. Res. 59, 7033–7045. doi:10.1021/acs.iecr.9b06579
Inoue, S., Koinuma, H., and Tsuruta, T. (1969). Copolymerization of carbon dioxide and epoxide. J. Polym. Sci. B. Polym. Lett. 7, 287–292. doi:10.1002/pol.1969.110070408
Inoue, S. (1979). Copolymerization of carbon dioxide and epoxide. Am. Chem. Soc. Div. Polym. Chem. Prepr. 20, 331–337. doi:10.1007/978-94-009-3923-3_18
Intrieri, D., Damiano, C., Sonzini, P., and Gallo, E. (2019). Porphyrin-based homogeneous catalysts for the CO 2 cycloaddition to epoxides and aziridines. J. Porphyr. Phthalocyanines 23, 305–328. doi:10.1142/S1088424619300015
Kamphuis, A. J., Milocco, F., Koiter, L., Pescarmona, P. P., and Otten, E. (2019a). Highly selective single-component formazanate ferrate(II) catalysts for the conversion of CO2 into cyclic carbonates. ChemSusChem 12, 3635–3641. doi:10.1002/cssc.201900740
Kamphuis, A. J., Picchioni, F., and Pescarmona, P. P. (2019b). CO2-fixation into cyclic and polymeric carbonates: Principles and applications. Green Chem. 21, 406–448. doi:10.1039/c8gc03086c
Keijer, T., Bakker, V., and Slootweg, J. C. (2019). Circular chemistry to enable a circular economy. Nat. Chem. 11, 190–195. doi:10.1038/s41557-019-0226-9
Kisch, H., Millini, R., and Wang, I.-J. (1986). Bifunktionelle Katalysatoren zur Synthese cyclischer Carbonate aus Oxiranen und Kohlendioxid. Chem. Ber. 119, 1090–1094. doi:10.1002/cber.19861190329
Liang, J., Huang, Y. B., and Cao, R. (2019). Metal–organic frameworks and porous organic polymers for sustainable fixation of carbon dioxide into cyclic carbonates. Coord. Chem. Rev. 378, 32–65. doi:10.1016/J.CCR.2017.11.013
Liu, Q., Wu, L., Jackstell, R., and Beller, M. (2015). Using carbon dioxide as a building block in organic synthesis. Nat. Commun. 6, 5933. doi:10.1038/ncomms6933
Liu, F., Duan, X., Dai, X., Du, S., Ma, J., Liu, F., et al. (2022a). Metal-decorated porous organic frameworks with cross-linked pyridyl and triazinyl as efficient platforms for CO2 activation and conversion under mild conditions. Chem. Eng. J. 445, 136687. doi:10.1016/J.CEJ.2022.136687
Liu, M., Zhao, P., Zhang, W., Cheng, X., Fei, H., Ma, J., et al. (2022b). Rational self-assembly of triazine- and urea-functionalized periodic mesoporous organosilicas for efficient CO2 adsorption and conversion into cyclic carbonates. Fuel 315, 123230. doi:10.1016/J.FUEL.2022.123230
Maquilón, C., Limburg, B., Laserna, V., Garay-Ruiz, D., González-Fabra, J., Bo, C., et al. (2020). Effect of an Al(III) complex on the regio-and stereoisomeric formation of bicyclic organic carbonates. Organometallics 39, 1642–1651. doi:10.1021/acs.organomet.9b00773
Martens, J. A., Bogaerts, A., de Kimpe, N., Jacobs, P. A., Marin, G. B., Rabaey, K., et al. (2017). The chemical route to a carbon dioxide neutral world. ChemSusChem 10, 1039–1055. doi:10.1002/cssc.201601051
Modak, A., Bhanja, P., Dutta, S., Chowdhury, B., and Bhaumik, A. (2020). Catalytic reduction of CO2 into fuels and fine chemicals. Green Chem. 22, 4002–4033. doi:10.1039/D0GC01092H
Montoya, C. A., Paninho, A. B., Felix, P. M., Zakrzewska, M. E., Vital, J., Najdanovic-Visak, V., et al. (2015). Styrene carbonate synthesis from CO2 using tetrabutylammonium bromide as a non-supported heterogeneous catalyst phase. J. Supercrit. Fluids 100, 155–159. doi:10.1016/j.supflu.2015.01.027
Nguyen, Q. T., Do, X. H., Cho, K. Y., Lee, Y. R., and Baek, K. Y. (2022). Amine-functionalized bimetallic Co/Zn-zeolitic imidazolate frameworks as an efficient catalyst for the CO2 cycloaddition to epoxides under mild conditions. J. CO2 Util. 61, 102061. doi:10.1016/J.JCOU.2022.102061
Panza, N., Biase, A., Gallo, E., and Caselli, A. (2021). Unexpected “ ferrate ” species as single-component catalyst for the cycloaddition of CO2 to epoxides. J. CO2 Util. 51, 101635. doi:10.1016/j.jcou.2021.101635
Panza, N., Soave, R., Cargnoni, F., Trioni, M. I., and Caselli, A. (2022). Experimental and theoretical insight into the mechanism of CO2 cycloaddition to epoxides catalyzed by ammonium ferrates. J. CO2 Util. 62, 102062. doi:10.1016/J.JCOU.2022.102062
Pescarmona, P. P. (2021). Cyclic carbonates synthesised from CO2: applications, challenges and recent research trends. Curr. Opin. Green Sustain. Chem. 29, 100457. doi:10.1016/j.cogsc.2021.100457
Rintjema, J., and Kleij, A. W. (2017). Aluminum-mediated formation of cyclic carbonates: Benchmarking catalytic performance metrics. ChemSusChem 10, 1274–1282. doi:10.1002/cssc.201601712
Rix, E., Grau, E., Chollet, G., and Cramail, H. (2016). Synthesis of fatty acid-based non-isocyanate polyurethanes, NIPUs, in bulk and mini-emulsion. Eur. Polym. J. 84, 863–872. doi:10.1016/j.eurpolymj.2016.07.006
Sathish, M., Sreeram, K. J., Raghava Rao, J., and Unni Nair, B. (2016). Cyclic carbonate: A recyclable medium for zero discharge tanning. ACS Sustain. Chem. Eng. 4, 1032–1040. doi:10.1021/acssuschemeng.5b01121
Schäffner, B., Schäffner, F., Verevkin, S. P., and Börner, A. (2010). Organic carbonates as solvents in synthesis and catalysis. Chem. Rev. 110, 4554–4581. doi:10.1021/cr900393d
Shaikh, R. R., Pornpraprom, S., and D’Elia, V. (2018). Catalytic strategies for the cycloaddition of pure, diluted, and waste CO2 to epoxides under ambient conditions. ACS Catal. 8, 419–450. doi:10.1021/acscatal.7b03580
Singh Dhankhar, S., Ugale, B., and Nagaraja, C. M. (2020). Co-Catalyst-Free chemical fixation of CO 2 into cyclic carbonates by using metal-organic frameworks as efficient heterogeneous catalysts. Chem. Asian J. 2020, 2403–2427. doi:10.1002/asia.202000424
Sit, W. N., Ng, S. M., Kwong, K. Y., and Lau, C. P. (2005). Coupling reactions of CO2 with neat epoxides catalyzed by PPN salts to yield cyclic carbonates. J. Org. Chem. 70, 8583–8586. doi:10.1021/jo051077e
Sodpiban, O., del Gobbo, S., Barman, S., Aomchad, V., Kidkhunthod, P., Ould-Chikh, S., et al. (2019). Synthesis of well-defined yttrium-based Lewis acids by capturing a reaction intermediate and catalytic application for cycloaddition of CO2 to epoxides under atmospheric pressure. Catal. Sci. Technol. 9, 6152–6165. doi:10.1039/c9cy01642b
Sodpiban, O., Phungpanya, C., del Gobbo, S., Arayachukiat, S., Piromchart, T., and D’Elia, V. (2021). Rational engineering of single-component heterogeneous catalysts based on abundant metal centers for the mild conversion of pure and impure CO2 to cyclic carbonates. Chem. Eng. J. 422, 129930. doi:10.1016/J.CEJ.2021.129930
Su, Z., Ma, J., Wei, J., Bai, X., Wang, N., and Li, J. (2022). A zinc porphyrin polymer as efficient bifunctional catalyst for conversion of CO2 to cyclic carbonates. Appl. Organomet. Chem. 36, e6632. doi:10.1002/aoc.6632
Tong, H., Qu, Y., Li, Z., He, J., Zou, X., Zhou, Y., et al. (2022). Halide-free pyridinium saccharinate binary organocatalyst for the cycloaddition of CO2 into epoxides. Chem. Eng. J. 444, 135478. doi:10.1016/J.CEJ.2022.135478
Wang, J. Q., Dong, K., Cheng, W. G., Sun, J., and Zhang, S. J. (2012). Insights into quaternary ammonium salts-catalyzed fixation carbon dioxide with epoxides. Catal. Sci. Technol. 2, 1480–1484. doi:10.1039/c2cy20103h
Wang, S., Peng, J., Yang, H.-J., Ban, B., Wang, L., and Lei, B. (2019). et al.β-Cyclodextrin/Quaternary Ammonium Salt as an Efficient Catalyst System for Chemical Fixation of CO2. J. Nanosci. Nanotechnol. 19, 3263–3268. doi:10.1166/jnn.2019.16610
Wang, Z., Wang, Y., Xie, Q., Fan, Z., and Shen, Y. (2021). Aliphatic carboxylic acid as a hydrogen-bond donor for converting CO2 and epoxide into cyclic carbonate under mild conditions. New J. Chem. 45, 9403–9408. doi:10.1039/d1nj01285a
Wyrzykowski, D., Kruszyński, R., Kucharska, U., and Warnke, Z. (2006). Structural and physicochemical characteristics of tetrabutylammonium tetrahalogenoferrates(III), [(C4H9)4N] [FeBr4-nCln]. Z. Anorg. Allg. Chem. 632, 624–628. doi:10.1002/zaac.200500472
Wyrzykowski, D., Kruszyñski, R., Klak, J., Mrozinski, J., and Warnke, Z. (2007). Magnetic characteristics of tetrabutylammonium tetrahalogenoferrates(III): X-ray crystal structure of tetrabutylammonium tetrabromoferrate(III). Z. Anorg. Allg. Chem. 633, 2071–2076. doi:10.1002/zaac.200700261
Keywords: homogeneous catalysis, zinc, chemical utilization of CO2, cyclic carbonates, zincates
Citation: Panza N, Alberti M, Damiano C and Caselli A (2022) Ammonium zincates as suitable catalyst for the room temperature cycloaddition of CO2 to epoxides. Front. Catal. 2:991270. doi: 10.3389/fctls.2022.991270
Received: 11 July 2022; Accepted: 26 July 2022;
Published: 30 August 2022.
Edited by:
Alceo Macchioni, University of Perugia, ItalyReviewed by:
Giulio Bresciani, University of Pisa, ItalyAmit Kumar, University of St Andrews, United Kingdom
Copyright © 2022 Panza, Alberti, Damiano and Caselli. This is an open-access article distributed under the terms of the Creative Commons Attribution License (CC BY). The use, distribution or reproduction in other forums is permitted, provided the original author(s) and the copyright owner(s) are credited and that the original publication in this journal is cited, in accordance with accepted academic practice. No use, distribution or reproduction is permitted which does not comply with these terms.
*Correspondence: Alessandro Caselli, YWxlc3NhbmRyby5jYXNlbGxpQHVuaW1pLml0
†ORCID: Nicola Panza, orcid.org/0000-0002-9627-9741; Matteo Alberti, orcid.org/0000-0002-2121-9314; Caterina Damiano, orcid.org/0000-0001-5903-238X; Alessandro Caselli, orcid.org/0000-0002-5851-267X