- 1Key Laboratory of Birth Defects and Related Diseases of Women and Children of MOE, Department of Pediatrics, West China Second University Hospital, Sichuan University, Chengdu, China
- 2State Key Laboratory of Biocatalysis and Enzyme Engineering, School of Life Science, Hubei University, Wuhan, China
Sepsis is a syndrome with life-threatening organ dysfunction induced by a dysregulated host response to infection. The heart is one of the most commonly involved organs during sepsis, and cardiac dysfunction, which is usually indicative of an extremely poor clinical outcome, is a leading cause of death in septic cases. Despite substantial improvements in the understanding of the mechanisms that contribute to the origin and responses to sepsis, the prognosis of sepsis-induced cardiac dysfunction (SICD) remains poor and its molecular pathophysiological changes are not well-characterized. The recently discovered group of mediators known as long non-coding RNAs (lncRNAs) have presented novel insights and opportunities to explore the mechanisms and development of SICD and may provide new targets for diagnosis and therapeutic strategies. LncRNAs are RNA transcripts of more than 200 nucleotides with limited or no protein-coding potential. Evidence has rapidly accumulated from numerous studies on how lncRNAs function in associated regulatory circuits during SICD. This review outlines the direct evidence of the effect of lncRNAs on SICD based on clinical trials and animal studies. Furthermore, potential functional lncRNAs in SICD that have been identified in sepsis studies are summarized with a proven biological function in research on other cardiovascular diseases.
Introduction
Sepsis is a syndrome with life-threatening organ dysfunction induced by a dysregulated host response to infection (1, 2). In-hospital mortality among patients with septic shock is reported to reach up to 40% (1). Septic shock is a series of circulatory, metabolic, and cellular abnormalities and is defined by a requirement for vasopressor support and persistent hyperlactatemia in the absence of hypovolemia (3, 4). Epidemiological studies showed that ~28.3 to 41% of all hospitalized sepsis patients died due to multiple organ failure (5), and sepsis-induced cardiac dysfunction (SICD) was identified as being closely associated with higher mortality rates (6, 7). Cardiac dysfunction is one of the major complications to sepsis, hence is predictive of a poor clinical outcome. Due to the pathophysiological changes of sepsis, cardiac lesions might be induced by a series of factors including myocardial ischemia, myocardial depressant substance, inflammation, adrenergic pathways deregulation, calcium overload, mitochondrial disorder, coronary microvascular dysfunction, and myocardial damages (4). Animal and cell experiments with lipopolysaccharide (LPS)-induced sepsis models demonstrated a significantly higher rate of cardiomyocyte apoptosis, intracellular ROS accumulation, elevated cytoplasm cytochrome C levels, and activated inflammatory pathways (8).
The development of genome-wide association studies (GWAS) and RNA sequencing (RNA-Seq) facilitated the discovery that a large part of the nucleotide genome presents limited or no protein-coding capabilities, although these regions are still effectively transcribed. The RNAs related to these regions were named non-coding RNA (ncRNA) (9). Long non-coding RNA (lncRNA) is a type of ncRNA that is composed of more than 200 nucleotides and contributes to transcriptional and post-transcriptional regulation of RNA. According to their molecular function, lncRNAs can be classified as signal, decoy, guide, scaffold, enhancer, or sponge lncRNAs (especially circular RNAs) (10, 11) (Figure 1). Whether circular RNAs (circRNAs) belong to the lncRNAs is a matter of controversy. However, in consideration of their similarities in function and definition to lncRNAs, we regard circRNAs as a unique subtype of lncRNA, and consequently they are included in this review (11–13).
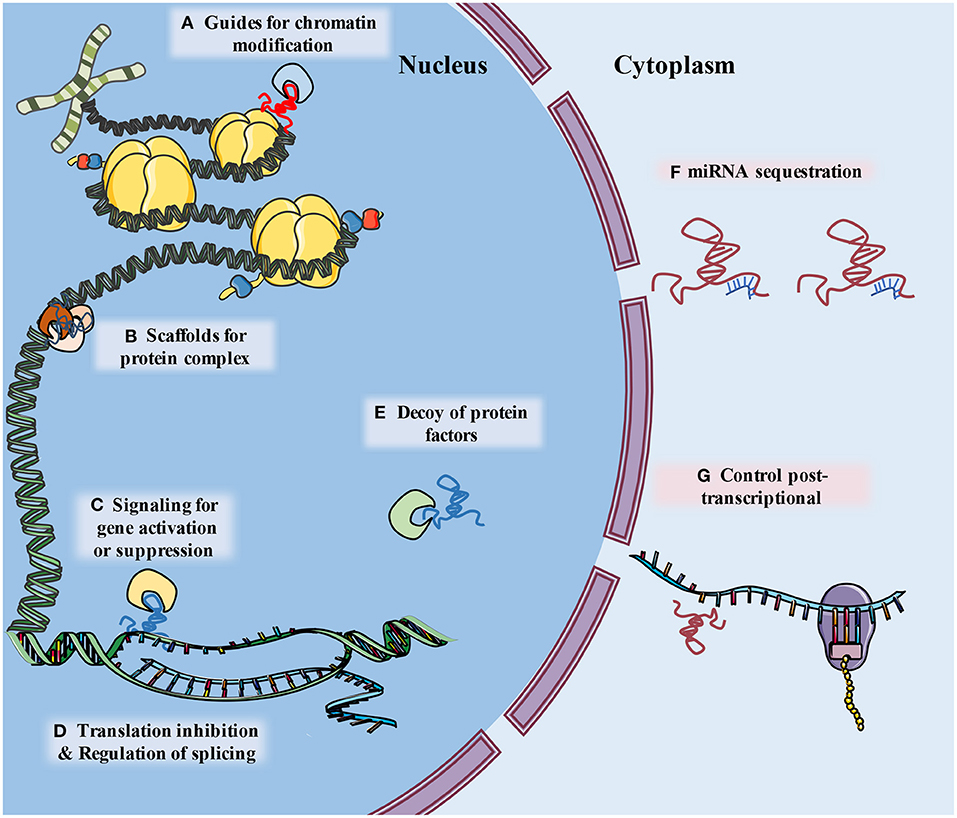
Figure 1. The schematic diagram describes classification of lncRNA functions. (A) LncRNAs guide ribonucleoprotein complexes to specific location of chromatin. (B) LncRNAs support assembly of protein complex. (C) lncRNAs serve as molecular signals for tissue and temporary specific activation of transcription. (D) LncRNAs can alter splicing patterns of mRNA and suppress transcription by sequestering transcription factors. (E) LncRNAs can bind to and take away protein factors, such as transcription factors and chromatin modifiers, to influence transcriptome. (F) LncRNA can “sponge” miRNA by base pairing with their complementary base sequence and reduce their effects (G) lncRNAs may interact with a variety of RNA binding proteins (RBPs), leading to alternations of mRNA stability, splicing, protein stability and subcellular localization.
Modulation of lncRNA plays important roles in various stages of sepsis development and pathophysiological processes, and this may offer potential novel diagnostic and therapeutic strategies to reduce the mortality and burden of SICD. Using sequencing analysis, more than 80% of the primary genetic elements were observed to change in patients with critical sepsis (14). In vitro, human umbilical vein endothelial cells (HUVECs) exposed to LPS showed a 28- to 70-fold increase in the expression of lncRNAs (15). Differential expression of lncRNAs has been observed in several other cell types after exposure to the plasma of septic patients or LPS, including human tubular epithelial cells, monocytes, and cardiomyocytes, indicating a tissue-specific biological function of lncRNA (16–18). Therefore, the lncRNAs involved in SICD regulate both cardiomyocytes and non-cardiomyocytes. Current evidence indicates a role for lncRNAs in regulation of cardiomyocyte functions, such as mitochondrial homeostasis, calcium handling, contraction, and apoptosis.
Activation of inflammatory pathways mediated by Toll-like receptor (TLR) signaling in response to pathogen-associated molecular patterns (PAMPs) and damage-associated molecular patterns (DAMPs) is an important mechanism of cardiomyocyte injuries caused by sepsis. These inflammatory pathways include those involving nuclear factor-κB (NF-κB) and mitogen-activated protein kinase (MAPK), as well as some other pathways (19). The lncRNAs involved in immune responses are also likely to contribute to the origins of SICD. However, since lncRNAs present multiple modalities of action with low conservation in vertebrates, exploring the individual functions of a particular lncRNA is challenging and more difficult than similar research on microRNAs (miRNAs). Hence, several lncRNAs involved in inflammatory responses in cardiomyocytes lack associated evidence in SICD (20).
This review summarizes the direct evidence for the involvement of lncRNAs in SICD based on clinical research studies of patients with SICD and basic biology explorations using animal or cell models of SICD. Furthermore, the lncRNAs involved in both sepsis and cardiovascular diseases (CVD) among individual studies are described and their potential associations in SICD are analyzed; these studies were treated as indirect evidence for the role of lncRNAs in SICD.
The Association Between lncRNAs and SICD
A recent study using microarray and whole genomic transcription sequencing with bioinformatics analyses on blood samples from patients with sepsis discovered 46 differentially expressed lncRNAs (DElncRNAs) (21). Additionally, 28 upregulated and 61 downregulated lncRNAs were identified in the public reported NCBI GEO dataset (22). Similar analyses based on cardiac tissue from mouse or rat sepsis models reported 74 (23) to 1,275 DElncRNAs, and revealed 14 lncRNAs that were highly correlated with 11 mitochondria-related differentially expressed mRNAs (24) and 11 differentially expressed circRNAs (25). Kyoto Encyclopedia of Genes and Genomes (KEGG) analysis indicated that upregulated lncRNAs were significantly enriched in the p53, NF-κB, and HIF-1 signaling pathways (26). Tissue-specific RNA-Seq in artificial induced inflammation revealed that some LPS-mediated lncRNAs were correlated to cardiometabolic traits (16). Thus, lncRNAs participate in regulating mitochondrial function, metabolic homeostasis, and inflammation signaling in cardiomyocytes during sepsis attacks.
Evidence in the literature linking lncRNAs and SICD can be divided into two distinct types. The first type of evidence (direct) presents clear confirmation of the involvement of lncRNAs in SICD, either from clinical samples or animal models, with definite molecular function demonstrated. The second type of evidence (indirect) describes studies where lncRNAs displayed differentiated expression in sepsis samples and were proven to have a critical role in maintaining cardiomyocyte function but lacked convincing evidence in SICD.
lncRNA Involved in SICD Among Various Cell Types
Here, direct evidence of the involvement of lncRNAs in SICD is summarized (Figure 2). This evidence is based on the findings from basic molecular biological research using animal models of hypodynamic septic shock induced by LPS and cecal ligation and puncture (CLP) (27); cardiac muscle cell lines (primary culture cardiomyocytes, H9C2, HL-1, and AC-16 cell lines) and microvascular cell lines exposed to serum from septic patients or administered with LPS (28); and clinical studies of sepsis patients subjected to cardiac dysfunction (Table 1).
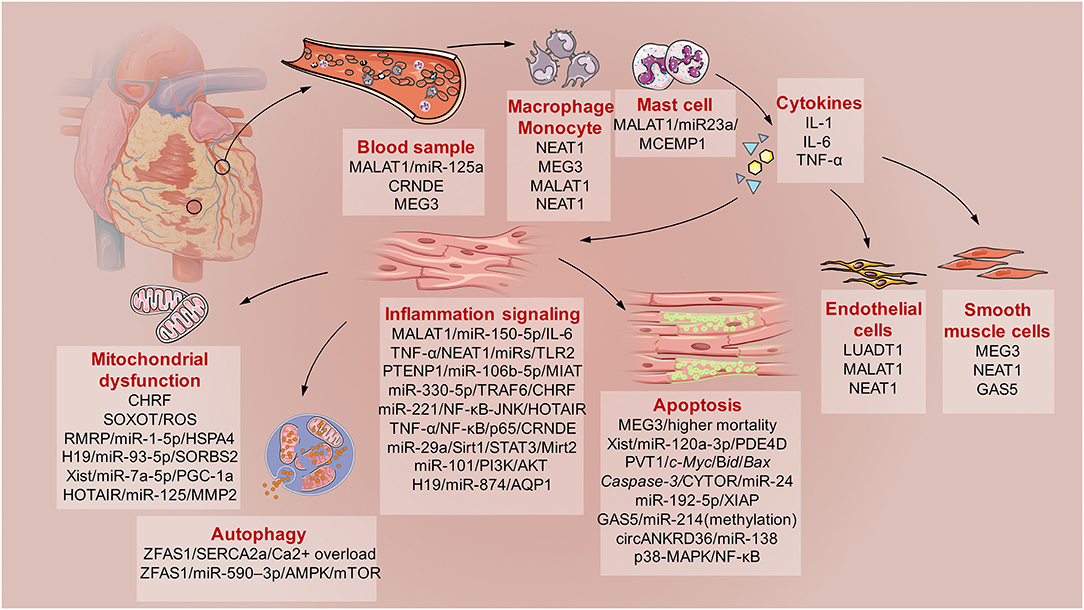
Figure 2. The schematic diagram describes the involved lncRNAs in sepsis induced cardiac dysfunction (SICD). Generally, current evidences demonstrated some lncRNAs served as biomarkers for SCID. Then, monocytes, macrophage, and mast cells would be activated with kinds of cytokines secretion. After that, immune responses of cardiomyocytes would lead to mitochondrial dysfunction, apoptosis and autophagy under the regulation of specific lncRNAs. Besides, lncRNAs also participates in the regulation of endothelial cells and smooth muscle cells during SICD.
Cardiomyocytes
LncRNAs participate in cardiomyocyte function through inflammatory signaling pathways, cytokine release, mitochondria homeostasis, apoptotic processes, and cell proliferation and migration during SICD.
Inflammation Signaling
LncRNAs are involved in the inflammatory process by regulating inflammation signaling, including the NF-κB, JAK/STAT, and MAPK pathways, and production of cytokines, such as IL-1, IL-6, IL-10, and TNF-α. The lncRNA MALAT1 is responsible for the septic inflammatory response under LPS administration in cardiomyocytes by downregulating miR-150-5p to increase expression of IL-6, TNF-α and the NF-κB signaling pathway (29), and TNF-α induction partly relied on serum amyloid antigen 3 (SAA3) (30). MALAT1 also interacts with p38 MAPK/NF-kB and miR-125b to aggravate cardiac inflammation and dysfunction in sepsis (31). The lncRNA NEAT1 was associated with disease severity, higher mortality risk, and unfavorable prognosis in sepsis patients (32). Furthermore, NEAT1 plays an important role in cardiomyocyte injury and apoptosis associated with miR-140-5p, miR-193a, miR-27b, miR-181b, miR-129-5p, miR-495-3p, miR-125a-5p, and their corresponding downstream regulated genes (33–40). NEAT1 knockdown can improve the outcome of LPS-induced myocardial injuries in mice by upregulating miR-144-3p (41) and downregulating expression of TLR2 and p65 and mRNA levels of inflammatory indicators to inhibit the TLR2/NF-κB signaling pathway (42). Expression of lncRNA PTENP1 was upregulated in sepsis models subjected to LPS administration, while miR-106b-5p expression was downregulated. Matrine administration could attenuate changes in expression of these two ncRNAs, and the cardioprotective effects of matrine were reversed by overexpression of PTENP1 or knockdown of miR-106b-5p (43). The lncRNA MIAT directly binds to miR-330-5p to activate TRAF6/NF-κB signaling axis and further promotes inflammatory response as well as oxidative stress in LPS-induced septic cardiomyopathy (44).
Silencing the lncRNA CHRF protected H9c2 cells against LPS-induced injury via upregulation of miR-221 and modulation of NF-κB and JNK pathways (45). In addition, silencing HOTAIR lncRNA reduced secretion of TNF-α into the circulation by inhibition of NF-κB signaling through dephosphorization of NF-κB p65 subunit, and helped preserve cardiac function in septic mice (18). Moreover, knockdown of circHIPK3 effectively alleviated LPS-induced myocarditis (46).
Beyond the lncRNAs that contribute to triggering inflammation, there is a series of lncRNAs that present a protective value of SICD. LncRNA CRNDE attenuates miRNA-29a to enhance expression of Sirt1, which contributes to inhibition of NF-κB and STAT3 inflammation signaling in myocardial tissue under septic attack (47). LncRNA Mirt2 silenced miR-101 and attenuated the myocardial inflammatory response in sepsis rats through the PI3K/AKT signaling pathway, and this improved cardiac remodeling and function (48). However, no human homologs of Mirt1 and Mirt2 have been described to date.
In an in vitro model established on cardiomyocytes subjected to LPS, there was a negative relationship between lncRNA H19 and miR-874, and a positive correlation between H19 and Aquaporin 1 (AQP1). H19 could act as AQP1 competing endogenous RNA (ceRNA) by regulating miR-874 and restoring LPS over-activated inflammatory responses and myocardial dysfunction (49, 50).
Mitochondria
Mitochondria are one of the most important organelles of cardiomyocytes, but they are quite sensitive to external and internal stimulations, resulting in mitochondrial dysfunction and leading to metabolic disorder with accumulation of reactive oxygen species (ROS). Mitochondrial dysfunction is associated with DNA damage and apoptosis. In experimental models of sepsis attacks, reduced mitochondrial membrane potential (MMP), elevated mitochondrial cytochrome C, and downregulated ROS scavenging were identified (8). LncRNAs make significant contributions to maintaining cardiac mitochondria homeostasis, hence these studies revealed a critical role of lncRNAs in response to sepsis attack.
Zhang et al. (45) demonstrated that silencing the lncRNA CHRF prevented LPS-triggered mitochondrial apoptosis and inflammation of cardiomyocytes. LncRNA SOX2 overlapping transcript (SOX2OT) is a proven mitochondrial damage factor in sepsis and contributes to mitochondrial dysfunction progression by inhibiting SOX2 expression in septic cardiomyopathy. Knockdown of SOX2OT could restore the MMP, along with reduction of ROS production induced by LPS, while overexpression of SOX2OT enhanced mitochondrial damage (51).
LncRNA RMRP acts as a sponge for miR-1-5p and provides a protective effect to mitochondria via the RMRP-miR-1-5p-HSPA4 network, which is known to play crucial roles in inflammation (8). LncRNA H19 and SORBS2 (Sorbin and SH3 domain-containing protein 2) were downregulated in H9C2 cells following administration of LPS, and miR-93-5p was simultaneously upregulated. LncRNA Xist is instrumental in X-chromosome inactivation and inhibits apoptosis in acute myocardial infarction (MI) (52). Peroxisome proliferator-activated receptor-γ coactivator-1α (PGC-1α) and adenosine triphosphate (ATP) expression was markedly reduced in sepsis leading to mitochondrial dysfunction, but mitochondrial function was restored after the inhibition of Xist and mir-7a-5p, which reduced apoptosis in response to LPS (53). Inhibition of lnc-HOTAIR aggravates oxidative stress-induced damage of H9c2 cells through the HOTAIR/miR-125/MMP2 axis (54).
LncRNA H19 is an important regulator of mammalian development and disease in that it inhibits cell proliferation (55). H19 is normally highly expressed during in utero development and then downregulated at birth (56), while re-expression occurs in some cardiovascular disease settings (57–59). In accordance with its inhibition function of cell proliferation, H19 was proved as precursor of miR-675, which inhibits cardiomyocyte hypertrophy and contributes to cardiac fibroblast proliferation and fibrosis through repression of DUSP5/ERK1/2 (60). Furthermore, H19 is involved in myocardial ischemic preconditioning via increasing the stability of nucleolin protein, which mitigates the damage caused by MI (61). Human GWASs demonstrated significant associations between the H19 locus and systolic or mean arterial blood pressure (9). LPS-induced cell growth inhibition and mitochondrial damage was significantly reversed by overexpression of H19, which sponged miR-93-5p to promote SORBS2 expression (62).
Apoptosis
Cardiomyocyte apoptosis, which is a key parameter for SICD and leads to long-term myocardial dysfunction, has been proposed to occur as a result of a sequence of cellular damages (63). Several signaling pathways are involved in apoptosis regulation via nuclear and mitochondrial approaches. However, crosstalk between lncRNAs and signaling pathways has been identified, and several lncRNAs regulate the process of apoptosis.
Overexpression of lncRNA MEG3 is associated with high mortality rates in patients with sepsis, thus is indicative of poor clinical outcomes and is believed to be associated with LPS-induced renal epithelial cell and cardiomyocyte apoptosis (64). LncRNA Xist promoted apoptosis of cardiomyocytes and inhibited proliferation of these cells by downregulating miR-130a-3p and upregulating PDE4D, which is a direct target of miR-130a-3p (52).
LncRNA PVT1 also showed significant upregulation and a vital functional role in maintaining the myocardial contractile function in rat models of hypodynamic septic shock induced by LPS. Knockdown of PVT1 induced cell apoptosis in LPS-induced cardiomyocytes through increasing the expression of c-Myc, Bid, Bax, and Caspase-3 and decreasing expression of Myd88 and Bcl-2 (23). LncRNA CYTOR was markedly downregulated during sepsis. This lncRNA negatively regulated expression of miR-24 and apoptosis-related proteins that were regulated by miR-24. MiR-24 directly targeted the 3′UTR of X-chromosome-linked inhibitor of apoptosis (XIAP) and suppressed its expression. Downregulation of CYTOR aggravated sepsis-induced cardiac injury via regulation of miR-24/XIAP (65). The lncRNA KCNQ1OT1 is similar in mechanism to CYTOR. It was considerably downregulated in myocardial tissues of septic rats, whereas miR-192-5p was increased in these tissues. CYTOR regulates XIAP through miR-192-5p, which pairs with the 3′UTR of XIAP, and represses its protein translation. These findings show that downregulation of KCNQ1OT1 aggravates cardiac injury through the miR-192-5p/XIAP axis during sepsis (66). LncRNA GAS5 may upregulate miR-214 through a methylation pathway to inhibit cardiomyocyte apoptosis in sepsis (67).
Fan et al. (46) demonstrated that circHIPK3 expression was significantly upregulated when exposed to LPS in vivo and in vitro, and that knockdown of circHIPK3 effectively alleviated LPS-induced myocarditis by attenuating inflammation-induced apoptosis of cardiomyocytes. Furthermore, silencing circANKRD36 exerted an anti-inflammatory and anti-apoptosis function in LPS-exposed H9c2 cells via the p38-MAPK/NF-κB pathway and upregulation of miR-138 (68). Another study confirmed an association between circANKRD36 and miR-15/MyD in regulating apoptosis due to inflammation damage (69).
Autophagy
Autophagy is an important biological process for regulating cellular homeostasis. However, there is currently limited data demonstrating the involvement of lncRNAs in regulating cardiomyocyte autophagy. One study revealed that lncRNA ZFAS1 was an endogenous SERCA2a inhibitor and induces mitochondria-mediated apoptosis via cytosolic Ca2+ overload (70). ZFAS1 is activated by the transcription factor SP1 and aggravates the progression of sepsis-induced cardiac dysfunction via miR-590–3p/AMPK/mTOR signaling-mediated autophagy and pyroptosis of cardiomyocytes (71, 72).
Immune Cells
During sepsis, the immune system is the frontier responding to harmful stimulations, and monocytes, macrophages, and neutrophils all make significant contributions to targeting organ damage. The innate immune response induces strong activation of the cytokine system, which has plethoric effects on various organs and the vasculature, leading to changes in vascular permeability, endothelial function, and activation of further mediators such as bradykinin, histamine, and the complement and coagulation systems.
The lnc-MALAT1/miR-125a axis presents excellent value in differentiating sepsis patients from healthy controls using peripheral blood samples (73). In another study using clinical blood samples, lnc-CRNDE was found to trigger inflammation through the TLR3-NF-κB-cytokine signaling pathway and the downstream release of inflammatory cytokines (74). As a major protein related to innate immune and inflammatory responses, TLR3 is known to cause cardiac dysfunction and other organ damage during sepsis (74, 75). Low expression of lnc-MEG3 might also serve as a potential biomarker for the development, progression, and prognosis prediction of sepsis (76–78). Furthermore, overexpression of MEG3 prevented LPS-induced macrophage apoptosis and secretion of inflammatory factors by inhibiting activation of the NF-κB signaling pathway (77).
Lnc-MALAT1 plays multiple roles in inflammatory stimulation in the macrophage cell line RAW264.7 (79). This lncRNA could inhibit the proliferation of LPS-stimulated RAW264.7 cells by inducing SMAD3 expression via downregulation of hsa-miR-346 (79). Lnc-MALAT1 also promotes inflammation in septic mice by binding to miR-23a to upregulate mast cell-expressed membrane protein 1 (MCEMP1) (80).
High expression of NEAT1 in peripheral blood mononuclear cells (PBMCs) can be considered as an additive marker for the diagnosis of sepsis (81), while another study confirmed that monocyte-enriched NEAT1 was suppressed in post-MI patients (82). Data from experiments with NEAT1-knockout (NEAT1-KO) mice identified NEAT1 as a novel lncRNA-type immunoregulator affecting monocyte-macrophage functions and T cell differentiation. NEAT1-KO marrow-derived macrophages (BMDMs) responded to LPS with increased ROS production and disturbed phagocytic activity (82).
Endothelial Cells
Cardiomyocytes are the dominant type of cells in the heart. However, various cell types comprise functional heart tissue. Endothelial cells contribute to form microvascular circulation in myocardia. Endothelial cell dysfunction impairs the micro-circulation function, inducing ischemic cardiac lesions. In sepsis attacks, endothelial cells are also major targeted sites. However, few studies have drawn correlations between lncRNAs and endothelial cell damage. The lncRNA LUADT1 was downregulated in patients with sepsis and in cultured human primary coronary artery endothelial cells (HCAECs) exposed to LPS. Overexpression of LUADT1 upregulated the expression of PIM1, a target of miR-195. These findings indicated that overexpression of either LUADT1 or PIM1 would reduce the damage effects of miR-195 on LPS-induced apoptosis of cardiac endothelial cells (83). Yu et al. (84) demonstrated that the drug Ulinastatin protected against LPS-induced cell hyperpermeability and apoptosis of cardiac microvascular endothelial cell (CMVECs) via downregulation of lncRNA MALAT1 and EZH2. Moreover, Liu et al. (85) reported that miR-150 could induce sepsis-induced endothelial injury by regulating endoplasmic reticulum (ER) stress and inflammation via the MALAT1-mediated NF-κB pathway. Lnc-NEAT1 also participates in the viability and survival of coronary endothelial cells (86, 87).
Smooth Muscle Cells
Smooth muscle cells also significantly contribute to maintenance of coronary vessel circulation. However, smooth muscle cells were the targets of inflammation damage due to sepsis attacks. Ahmed et al. (88) demonstrated a role of NEAT1 in regulating phenotypic switching by repressing smooth muscle-contractile gene expression through an epigenetic regulatory mechanism. Silencing lnc-NEAT1 in vascular smooth muscle cells (VSMCs) enhanced expression of smooth muscle-specific genes while attenuating proliferation and migration of the VSMCs. The lncRNA MEG3 could modulate the balance of proliferation/apoptosis in VSMCs by regulating the miR-26a/SMAD1 axis (89). In addition, the lncRNA GAS5 exacerbates hypertensive arterial remodeling by regulating VSMC phenotypic conversion, which leads to microvascular dysfunction (90). However, there is lacking convinced evidence of GAS5 on SICD.
Predicted lncRNAs Based on Available Evidence
In addition to the above-mentioned lncRNAs with direct evidence in SICD, some other lncRNAs were reported to be involved both in sepsis and some types of CVD by other mechanisms. In view of the molecular functions of lncRNAs in regulating cardiomyocyte homeostasis and their expression during sepsis but without convincing evidence presented in a single study focusing on SICD, the most reported lncRNAs and associated mechanisms are summarized in this review to demonstrate their comprehensive impacts. Table 2 lists the lncRNAs that we predicted might play a role in SICD although no direct evidence is available from biological experiments or clinical trials. These lncRNAs were found to express differentially or function in sepsis and participate in CVD or other cardiac psychopathological processes in individual studies.
LncRNAs That Present Similar Functions in Sepsis and CVD
The mechanisms of lncRNAs in regulating downstream signaling is complicated, although research on ncRNAs is growing. However, direct evidence of lncRNAs on SICD remain limited. Moreover, sepsis is considered as a type of syndrome that damages various organs. Hence, research on sepsis includes investigations on various damages beyond cardiac dysfunction, such as lung injuries, kidney disorders, and other damages. Based on this predicament, we selected to review the lncRNAs involved in sepsis without evidence based on SICD but which had been confirmed as having similar protective or adverse roles in other types of CVD. The lncRNAs outlined in this part of the review are highly likely to have their capabilities proven in future SICD studies.
ANRIL
Several studies demonstrated that the lnc-ANRIL/miR-125a axis could serve as a predictor for prognosis, severity, and inflammation among sepsis patients (91–93). LncRNA ANRIL is the prime candidate gene at Chr9p21 and widely recognized as a critical part of endothelial inflammation and cell proliferation (91, 94–97). Single nucleotide polymorphisms (SNPs) and splice variants of ANRIL were reported to regulate endothelial cell activities involved in coronary artery heart disease (CAD) and MI (98–103). Abnormal expression of ANRIL is associated with vascular endothelium injury and proliferation, migration, and apoptosis of VSMCs; which also contribute to mononuclear cell adhesion and proliferation (104, 105). ANRIL knockdown induced cardiomyocyte apoptosis in acute MI by regulating IL-33/ST2 or Akt (106, 107). Enhanced expression of ANRIL and suppressed expression of miR-181b, which was inhibited by ANRIL, were recorded in CAD populations and confirmed ANRIL as an independent risk factor (108).
DC
Lnc-DC, also known as whey acidic protein/four-disulfide core domain 21 (Wfdc21), was reported to be correlated with immune responses. Knockdown of lnc-DC downregulated expression of pro-inflammatory factors, such as IL-1β and TNF-α, in LPS-treated macrophages through the STAT3/TLR4 signaling pathway (109). Alikhah et al. (110) found significant correlations between expression of lnc-DC with SOCS1 and STAT3 in CAD patients.
THRIL
LncRNA THRIL is upregulated during sepsis and may serve as a sponge of miR-19a to upregulate TNF-α (111). This lncRNA is considered to play important roles in the innate immune response and inflammatory diseases in humans (112). THRIL mediates autophagy of endothelial progenitor cells via the AKT pathway and FUS (113). Knockdown of THRIL protected H9C2 cells against hypoxia-induced injuries by regulating miR-99a (114). This mechanism was further demonstrated by Sheng et al. (115) with the observation that Geniposide alleviated hypoxia-induced injury through downregulation of THRIL in H9c2 cells. In addition, THRIL was increased in CAD patients and proved as a biomarker to evaluate CAD risk (116).
SNHG16
The lncRNA SNHG16 can act as a ceRNA to downregulate the miR-15a/16 cluster, reducing LPS-induced inflammatory signaling (117). SNHG16 also helps regulate miR-218-5p and promotes the proliferation and migration of coronary artery VSMCs via the Wnt/β-catenin pathway, protecting against the injuries from MI (118). Furthermore, silencing of SNHG16 repressed Ang II-imposed cardiac hypertrophy by targeting the miR-182-5p/IGF1 axis (119).
lncRNAs That Present Opposite Roles in Sepsis and CVD
Selection of potential lncRNAs involved in SICD is difficult, even with meticulous attention. Some findings from different individual studies demonstrated opposing functions of lncRNAs between sepsis and CVD, either in a protective or adverse direction. However, it is possible that there may be a shared intermediate target. Here, such lncRNAs are briefly described, but further analysis is required in relation to these lncRNAs and SICD. Moreover, the long-term effects of lncRNAs on CVD also lacks convincing data and this is another area that requires further research.
UCA1
Upregulation of lncRNA UCA1 is necessary for the response of pro-inflammatory immune cells during LPS-induced sepsis (120, 121). However, UCA1 inhibits ischemia/reperfusion (I/R)-induced oxidative stress and mitochondria dysfunction via suppression of ER stress (122).
HULC
HULC could induce pro-inflammatory mediators in response to LPS exposure in endothelial cells (120). Overexpression of HULC in HUVECs promoted angiogenesis by increasing cell viability, proliferation, and tube-like structure formation through downregulation of miR-29b (123). HULC also participated in TNF-α- (124) and I/R- (125) induced cardiomyocyte apoptosis through regulation of miR-9 and miR-377-5p expression.
P21
LncRNA-p21 serves as a repressor in p53-dependent transcriptional responses (126). This lncRNA regulates neointima formation, VSMC apoptosis, and atherosclerosis by enhancing p53 activity (127). Expression of lncRNA-p21 was significantly increased in a septic model and it predominantly functioned in cis to activate expression of p21, its neighboring gene (128). P21 itself is involved in regulation of macrophage activation, septic shock susceptibility (129), autophagy in LPS-induced cardiac dysfunction (130), and cardiomyocyte adheres junctions in endotoxemia (131).
TUG1
The lncRNA TUG1 promoted proliferation and migration of VSMCs in the hypertensive state by activating the miR-145-5p/FGF10 axis and the Wnt/β-catenin pathway to aggregate vascular remodeling (132). Another study reported that knockdown of TUG1 ameliorated atherosclerosis via upregulation of miR-133a expression following its target gene FGF1 (133). TUG1 expression was also reported to help alleviate acute lung injuries by targeting miR-34b-5p/GAB1 (134).
Another Strategy in Searching for lncRNAs
It is theoretically possible to regulate typical molecules of signal pathways by interfering with their corresponding lncRNAs. However, in terms of the extensive functions of those pathways, this train of thought is a low priority. For example, lncRNA HIFa-AS is a natural antisense transcript of Hypoxia-inducible factor 1-α (HIF1α) and is overexpressed in the failing heart. HIFa-AS destabilizes the mRNA producing HIF1α, which regulates transcription of cellular responses to hypoxia, especially in post-ischemic angiogenesis (135). HIFa-AS was also discovered to play a role in MI (98). Huang et al. reported that lncRNAs upregulated in sepsis were significantly enriched in the HIF-1 signaling pathway via KEGG analyses (26), and two studies found that HIF-1α participated in acute lung injury after sepsis (136, 137). Nevertheless, there are no studies reporting the role of lncRNA HIFa-AS in sepsis.
lncRNAs as Biomarkers and Therapeutic Targets
SICD is more like a functional disorder than a biochemical phenomenon. There is uncertainty as to whether SICD itself is pathogenic or is simply a reflection of the severity of the underlying disease process of sepsis. Diagnosis of SICD largely relies on ultrasonography imaging and troponin measurement. Increasing ultrasonic measurement indicators of left ventricular systolic and diastolic performances, and right ventricular dysfunction are applied to clinical practice and scientific research (4). Similar to troponin, the elevation of hormones B-type natriuretic peptide (BNP) and N-terminal pro-BNP (NT-proBNP) are determined mainly by the severity of sepsis other than specific abnormalities in cardiac function (138). To date, no ultrasonic prognostication has been demonstrated in patients with septic cardiomyopathy (139–142). Apart from lacking reliable biomarkers, the degree to which cardiac dysfunction represents cardiac structure damage and heart failure instead of a protective hibernation-type mechanism remain difficult to resolve (143). Current therapy for sepsis is predominantly focused on restoring cardiac output by inotropic agents and fluid resuscitation. The Surviving Sepsis Campaign guidelines recommend inotropic therapy in patients with persistent hypoperfusion despite adequate fluid loading (144). Limited and underperforming inotropic agent options, including dobutamine, catecholamines, and levosimendan, also contribute to SICD-related deaths (145–147). Therefore, novel biomarkers and therapeutic targets are urgently needed to improve the diagnosis and treatment of SICD.
LncRNAs as Predictive Biomarkers
As previously discussed, numerous lncRNAs are aberrantly expressed in SICD compared with normal cardiac tissue or cell lines, and this is useful to distinguish SICD patients from healthy cohorts. Although nearly all lncRNAs with direct evidence of their involvement in SICD were declared as potential biomarkers of SICD, those lncRNAs also show aberrant expression patterns in sepsis without cardiac dysfunction, especially critical patients, and in other non-sepsis situations such as MI, I/R, and acute kidney injury (21, 22, 24, 26, 110, 114, 148). This reduces the reliability of using these lncRNAs as potential biomarkers of SICD. To date, there is no study reporting the sensitivity and specificity of the diagnostic efficiency of these lncRNAs.
Compared with myocardial biopsy, blood sampling is largely non-invasive and thus is an ideal diagnostic approach. Several SICD-related lncRNAs can be present in the blood, as are the aforementioned cases. However, the circulating lncRNA differential expression profile is heterogeneous among different studies, partly due to severity, genetic background, and the pathogenic microorganism involved (149). One challenge with the clinical application of these lncRNAs is how to develop a convenient and rapid technique to detect the target lncRNAs in sepsis and thus bring the advantage of being less time-consuming than microbial culture into full play.
LncRNAs as Therapeutic Targets
To date, many studies have confirmed that lncRNAs are essential contributors to SICD progression due to the diversity of actions and cellular processes implicated. However, few practical examples of therapeutic applications of lncRNAs have been reported. The prognosis of SICD in general is poor, and this is in part due to the lack of therapeutic targets. The critical roles of lncRNAs in SICD make them promising targets for novel therapeutic interventions, and the base-pairing principle is much more straightforward than designing a specific protein-binding inhibitor. Multiple different approaches can be used in perturbing specific lncRNAs, including RNA interference (RNAi), antisense oligonucleotides (ASOs), clustered regularly interspaced short palindromic repeats (CRISPR)/Cas, CRISPR-Display, and the λN –Gal4 system.
Some lncRNAs, which regulate transcriptional outputs in cis, do not function in exogenous overexpression studies. Therefore, the λN –Gal4 system has been used to overcome this constraint by enhancing the overexpression of lncRNA in cis (150, 151). As duplex RNAs that have to be loaded into AGO2 protein to form an RNA-induced silencing complex (RISC) and interact with target lncRNA, RNAi is a reliable approach for targeting lncRNAs in the cytoplasm and inhibiting gene expression (152). Compared with RNAi, ASOs, as single-stranded DNAs, are more reliable gene silencing agents than duplex RNAs for the RNAs that are localized to cell nuclei (153, 154). ASOs with appropriate modifications have become readily available (155) and newer generation ASOs allow spatial control of target delivery (156). However, unlike RNAi (42, 53), no study to date has reported the application of ASOs in SICD. With higher efficiency, specificity, and the ability to modulate gene expression (157), the CRISPR/Cas method has dominated in recent years (158, 159) and CRISPR Display, which allows the insertion of RNA domains into DNA loci, was specially developed to modulate the expression of lncRNAs (160). Except for cell and animal models, ASOs and RNAi have already been applied in clinical trials for treatment of HBV (161).
Conclusion
Sepsis-induced cardiac dysfunction is challenged by a lack of uniformity in its definition of incidence, prognosis, and clinical importance. Two other core problems are whether cardiac dysfunction definitely contributes to poor outcome or prognosis, or is simply a reflection of organ failure in general, and the degree to which sepsis-induced cardiac dysfunction is adaptive or pathological (4). Construction of an ideal SICD animal model is difficult and existing research on this condition has only utilized sepsis models to investigate cardiac dysfunction, which partly accounts for the ambiguous mechanisms of SICD. The development and widespread use of GWAS and RNA-Seq has facilitated more discoveries and deeper understanding of lncRNAs, which in turn has helped exploration of SICD regulatory circuits and molecular mechanisms to make a comprehensive and clear definition of this condition, rather than it simply being based on observation of clinical patients.
Several limitations and challenges need to be solved before lncRNAs can reach clinical application. A primary concern is how to specifically target certain tissues or cell populations. As previously mentioned, nearly all identified lncRNAs in SICD or sepsis exhibit functions in other organisms or display multiple mechanisms of action. Second, it is well-established that unlike protein-coding genes, the majority of human long non-coding RNAs (lncRNAs) are considered non-conserved, suggesting variable evolutionary pressure between mRNA and lncRNAs (162). LncRNA conservation includes four dimensions: the sequence, structure, function, and expression from syntenic loci (163). However, several lncRNAs, such as HOTAIR (164) and Xist (165), exhibit clear functional roles in various mammalian species with poor sequence conservation (166). This phenomenon may be due to conserved secondary structures that do not alter with mutations in the sequence outside of structural regions (167, 168). Lnc-H19 and MALAT1 has been proved to be promising targets for cancer therapy (169, 170). Most of published studies of homolog lncRNAs were related to cancers, and now, more than forty clinical trials associated with lncRNA, including a study of lnc-NBR2 in sepsis, are in process in clinicaltrials.gov. Moreover, owing to unique secondary structure, circRNAs resist degradation by exoribonucleases, resulting in more abundant expression. Long-read sequencing technologies promise to improve current annotations and provide a novel perspective to locate homologs in human (171). However, secondary structures are more difficult to intervene in by conventional means than sequence mutation based on existing knowledge and technology. This leads to difficulties in constructing lncRNA knockout animal models. In addition, Joung et al. (172) reported that ~50% of lncRNAs influence the expression of neighboring protein-coding genes and many lncRNAs overlap with protein-coding genes, making it difficult to specifically knockout a lncRNA without affecting neighboring genes. RNA modification, especially m6A modification, also influences lncRNA function, for example in the case of m6A of Xist (173). Furthermore, the finding that micropeptides are encoded by lncRNAs (174) means research on lncRNAs has become more complicated and confusing. Overall, lncRNA of SICD is a promising field and remains largely undiscovered.
Author Contributions
DZ and YL conceived the presented idea. JL, YZ, and YL summarized the reference and drafted the manuscript. JL drafted the table. DZ organized the figure with online free material. DZ and YL supervised the project and contributed equally to the final version of the manuscript. All authors contributed to the article and approved the submitted version.
Funding
All phase of this study was supported by a Hubei Province Science Fund for Distinguished Young Scholars (2019CFA092), Key R&D Program of Sichuan Province of China (2020YFS0102), and Natural Science Foundation of China (81700360, 31871496).
Conflict of Interest
The authors declare that the research was conducted in the absence of any commercial or financial relationships that could be construed as a potential conflict of interest.
Abbreviations
ASOs, antisense oligonucleotides; CAD, coronary artery heart disease; circRNAs, circular RNAs; CLP, cecal ligation and puncture; CMVECs, cardiac microvascular endothelial cell; CRISPR, clustered regularly interspaced short palindromic repeats; CVD, cardiovascular disease; DAMPs, damage-associated molecular patterns; GWAS, genome-wide analyses; HCAECs, human primary coronary artery endothelial cells; HCASMC, coronary artery smooth muscle cells; HIF1α, Hypoxia-inducible factors 1α; HUVECs, human umbilical vein endothelial cells; I/R, Ischemia/Reperfusion; lncRNAs, long non-coding RNAs; LPS, lipopolysaccharide; MI, myocardial infarction; NF-κB, nuclear factor κB; PAMPs, pathogen-associated molecular patterns; PBMCs, Peripheral blood mononuclear cells; RNAi, RNA interference; SICD, sepsis-induced cardiac dysfunction; XIAP, X-chromosome-linked inhibitor of apoptosis; VSMCs, vascular smooth muscle cells.
References
1. Singer M, Deutschman CS, Seymour CW, Shankar-Hari M, Annane D, Bauer M, et al. The third international consensus definitions for sepsis and septic shock (Sepsis-3). JAMA. (2016) 315:801–10. doi: 10.1001/jama.2016.0287
2. Cecconi M, Evans L, Levy M, Rhodes A. Sepsis and septic shock. Lancet. (2018) 392:75–87. doi: 10.1016/S0140-6736(18)30696-2
3. Gotts JE, Matthay MA. Sepsis: pathophysiology and clinical management. BMJ. (2016) 353:i1585. doi: 10.1136/bmj.i1585
4. Hollenberg SM, Singer M. Pathophysiology of sepsis-induced cardiomyopathy. Nat Rev Cardiol. (2021). doi: 10.1038/s41569-020-00492-2. [Epub ahead of print].
5. Levy MM, Artigas A, Phillips GS, Rhodes A, Beale R, Osborn T, et al. Outcomes of the surviving sepsis campaign in intensive care units in the USA and Europe: a prospective cohort study. Lancet Infect Dis. (2012) 12:919–24. doi: 10.1016/S1473-3099(12)70239-6
6. Hochstadt A, Meroz Y, Landesberg G. Myocardial dysfunction in severe sepsis and septic shock: more questions than answers? J Cardiothorac Vasc Anesth. (2011) 25:526–35. doi: 10.1053/j.jvca.2010.11.026
7. Romero-Bermejo FJ, Ruiz-Bailen M, Gil-Cebrian J, Huertos-Ranchal MJ. Sepsis-induced cardiomyopathy. Curr Cardiol Rev. (2011) 7:163–83. doi: 10.2174/157340311798220494
8. Han Y, Cai Y, Lai X, Wang Z, Wei S, Tan K, et al. lncRNA RMRP prevents mitochondrial dysfunction and cardiomyocyte apoptosis via the miR-1-5p/hsp70 axis in LPS-induced sepsis mice. Inflammation. (2020) 43:605–18. doi: 10.1007/s10753-019-01141-8
9. Gomes CPC, Spencer H, Ford KL, Michel LYM, Baker AH, Emanueli C, et al. The function and therapeutic potential of long non-coding RNAs in cardiovascular development and disease. Mol Ther Nucleic Acids. (2017) 8:494–507. doi: 10.1016/j.omtn.2017.07.014
10. Ørom UA, Derrien T, Beringer M, Gumireddy K, Gardini A, Bussotti G, et al. Long noncoding RNAs with enhancer-like function in human cells. Cell. (2010) 143:46–58. doi: 10.1016/j.cell.2010.09.001
11. Hansen TB, Jensen TI, Clausen BH, Bramsen JB, Finsen B, Damgaard CK, et al. Natural RNA circles function as efficient microRNA sponges. Nature. (2013) 495:384–8. doi: 10.1038/nature11993
12. Wang KC, Chang HY. Molecular mechanisms of long noncoding RNAs. Mol Cell. (2011) 43:904–14. doi: 10.1016/j.molcel.2011.08.018
13. Chen LL. The biogenesis and emerging roles of circular RNAs. Nat Rev Mol Cell Biol. (2016) 17:205–11. doi: 10.1038/nrm.2015.32
14. Xiao W, Mindrinos MN, Seok J, Cuschieri J, Cuenca AG, Gao H, et al. A genomic storm in critically injured humans. J Exp Med. (2011) 208:2581–90. doi: 10.1084/jem.20111354
15. Singh KK, Matkar PN, Muhammad S, Quan A, Gupta V, Teoh H, et al. Investigation of novel LPS-induced differentially expressed long non-coding RNAs in endothelial cells. Mol Cell Biochem. (2016) 421:157–68. doi: 10.1007/s11010-016-2797-8
16. Liu Y, Ferguson JF, Xue C, Ballantyne RL, Silverman IM, Gosai SJ, et al. Tissue-specific RNA-Seq in human evoked inflammation identifies blood and adipose LincRNA signatures of cardiometabolic diseases. Arterioscler Thromb Vasc Biol. (2014) 34:902–12. doi: 10.1161/ATVBAHA.113.303123
17. Lin J, Zhang X, Xue C, Zhang H, Shashaty MG, Gosai SJ, et al. The long noncoding RNA landscape in hypoxic and inflammatory renal epithelial injury. Am J Physiol Renal Physiol. (2015) 309:F901–13. doi: 10.1152/ajprenal.00290.2015
18. Wu H, Liu J, Li W, Liu G, Li Z. LncRNA-HOTAIR promotes TNF-α production in cardiomyocytes of LPS-induced sepsis mice by activating NF-κB pathway. Biochem Biophys Res Commun. (2016) 471:240–6. doi: 10.1016/j.bbrc.2016.01.117
19. Conway-Morris A, Wilson J, Shankar-Hari M. Immune activation in sepsis. Crit Care Clin. (2018) 34:29–42. doi: 10.1016/j.ccc.2017.08.002
20. Johnsson P, Lipovich L, Grandér D, Morris KV. Evolutionary conservation of long non-coding RNAs; sequence, structure, function. Biochim Biophys Acta. (2014) 1840:1063–71.
21. Guo X, Qin Y, Wang L, Dong S, Yan Y, Bian X, et al. A competing endogenous RNA network reveals key lncRNAs associated with sepsis. Mol Genet Genomic Med. (2020) 9:e1557. doi: 10.1002/mgg3.1557
22. Bu L, Wang ZW, Hu SQ, Zhao WJ, Geng XJ, Zhou T, et al. Identification of Key mRNAs and lncRNAs in neonatal sepsis by gene expression profiling. Comput Math Methods Med. (2020) 2020:8741739. doi: 10.1155/2020/8741739
23. Zhang TN, Goodwin JE, Liu B, Li D, Wen R, Yang N, et al. Characterization of long noncoding RNA and mRNA profiles in sepsis-induced myocardial depression. Mol Ther Nucleic Acids. (2019) 17:852–66. doi: 10.1016/j.omtn.2019.07.020
24. Shi Y, Zheng X, Zheng M, Wang L, Chen Y, Shen Y. Identification of mitochondrial function-associated lncRNAs in septic mice myocardium. J Cell Biochem. (2021) 122:53–68. doi: 10.1002/jcb.29831
25. Zhang TN, Yang N, Goodwin JE, Mahrer K, Li D, Xia J, et al. Characterization of circular RNA and microRNA profiles in septic myocardial depression: a lipopolysaccharide-induced rat septic shock model. Inflammation. (2019) 42:1990–2002. doi: 10.1007/s10753-019-01060-8
26. Huang J, Liu Y, Xie Q, Liang G, Kong H, Liu M, et al. Expression profiling of long noncoding RNA and messenger RNA in a cecal ligation and puncture-induced colon injury mouse model. Mediat Inflamm. (2020) 2020:8925973. doi: 10.1155/2020/8925973
27. Korneev KV. [Mouse models of sepsis and septic shock]. Mol Biol. (2019) 53:799–814. doi: 10.1134/S0026893319050108
28. Hobai IA, Morse JC, Siwik DA, Colucci WS. Lipopolysaccharide and cytokines inhibit rat cardiomyocyte contractility in vitro. J Surg Res. (2015) 193:888–901. doi: 10.1016/j.jss.2014.09.015
29. Wei S, Liu Q. Long noncoding RNA MALAT1 modulates sepsis-induced cardiac inflammation through the miR-150-5p/NF-κB axis. Int J Clin Exp Pathol. (2019) 12:3311–9.
30. Zhuang YT, Xu DY, Wang GY, Sun JL, Huang Y, Wang SZ. IL-6 induced lncRNA MALAT1 enhances TNF-α expression in LPS-induced septic cardiomyocytes via activation of SAA3. Eur Rev Med Pharmacol Sci. (2017) 21:302–9.
31. Chen H, Wang X, Yan X, Cheng X, He X, Zheng W. LncRNA MALAT1 regulates sepsis-induced cardiac inflammation and dysfunction via interaction with miR-125b and p38 MAPK/NFκB. Int Immunopharmacol. (2018) 55:69–76. doi: 10.1016/j.intimp.2017.11.038
32. Huang Q, Huang C, Luo Y, He F, Zhang R. Circulating lncRNA NEAT1 correlates with increased risk, elevated severity and unfavorable prognosis in sepsis patients. Am J Emerg Med. (2018) 36:1659–63. doi: 10.1016/j.ajem.2018.06.008
33. Ren L, Chen S, Liu W, Hou P, Sun W, Yan H. Downregulation of long non-coding RNA nuclear enriched abundant transcript 1 promotes cell proliferation and inhibits cell apoptosis by targeting miR-193a in myocardial ischemia/reperfusion injury. BMC Cardiovasc Disord. (2019) 19:192. doi: 10.1186/s12872-019-1122-3
34. Ruan Z, Wang S, Yu W, Deng F. LncRNA NEAT1 aggravates diabetic myocardial ischemia-reperfusion injury through regulating PINK1 by targeting miR-27b. Int J Cardiol. (2019) 286:136. doi: 10.1016/j.ijcard.2019.03.046
35. Yan H, Liang H, Liu L, Chen D, Zhang Q. Long noncoding RNA NEAT1 sponges miR-125a-5p to suppress cardiomyocyte apoptosis via BCL2L12. Mol Med Rep. (2019) 19:4468–74. doi: 10.3892/mmr.2019.10095
36. Zou G, Zhong W, Wu F, Wang X, Liu L. Catalpol attenuates cardiomyocyte apoptosis in diabetic cardiomyopathy via Neat1/miR-140-5p/HDAC4 axis. Biochimie. (2019) 165:90–9. doi: 10.1016/j.biochi.2019.05.005
37. Chen XX, Jiang YJ, Zeng T, Li JJ. Overexpression of the long noncoding RNA NEAT1 protects against As2O3-induced injury of cardiomyocyte by inhibiting the miR-124/NF-κB signaling pathway. Eur Rev Med Pharmacol Sci. (2020) 24:1378–90. doi: 10.26355/eurrev_202002_20195
38. Luo M, Sun Q, Zhao H, Tao J, Yan D. Long noncoding RNA NEAT1 sponges miR-495-3p to enhance myocardial ischemia-reperfusion injury via MAPK6 activation. J Cell Physiol. (2020) 235:105–13. doi: 10.1002/jcp.28791
39. Lv Y, Liu Z, Huang J, Yu J, Dong Y, Wang J. LncRNA nuclear-enriched abundant transcript 1 regulates hypoxia-evoked apoptosis and autophagy via mediation of microRNA-181b. Mol Cell Biochem. (2020) 464:193–203. doi: 10.1007/s11010-019-03660-2
40. Zhao J, Chen F, Ma W, Zhang P. Suppression of long noncoding RNA NEAT1 attenuates hypoxia-induced cardiomyocytes injury by targeting miR-378a-3p. Gene. (2020) 731:144324. doi: 10.1016/j.gene.2019.144324
41. Wei JL, Wu CJ, Chen JJ, Shang FT, Guo SG, Zhang XC, et al. LncRNA NEAT1 promotes the progression of sepsis-induced myocardial cell injury by sponging miR-144-3p. Eur Rev Med Pharmacol Sci. (2020) 24:851–61. doi: 10.26355/eurrev_202001_20069
42. Wang SM, Liu GQ, Xian HB, Si JL, Qi SX, Yu YP. LncRNA NEAT1 alleviates sepsis-induced myocardial injury by regulating the TLR2/NF-κB signaling pathway. Eur Rev Med Pharmacol Sci. (2019) 23:4898–907. doi: 10.26355/eurrev_201906_18078
43. Liu Y, Liu L, Zhang J. Protective role of matrine in sepsis-associated cardiac dysfunction through regulating the lncRNA PTENP1/miR-106b-5p axis. Biomed Pharmacother. (2021) 134:111112. doi: 10.1016/j.biopha.2020.111112
44. Xing PC, An P, Hu GY, Wang DL, Zhou MJ. LncRNA MIAT promotes inflammation and oxidative stress in sepsis-induced cardiac injury by targeting miR-330-5p/TRAF6/NF-κB axis. Biochem Genet. (2020) 58:783–800. doi: 10.1007/s10528-020-09976-9
45. Zhang L, Wang L, Guo E, Qi Y. Silence of lncRNA CHRF protects H9c2 cells against lipopolysaccharide-induced injury via up-regulating microRNA-221. Exp Mol Pathol. (2019) 107:43–50. doi: 10.1016/j.yexmp.2019.01.010
46. Fan S, Hu K, Zhang D, Liu F. Interference of circRNA HIPK3 alleviates cardiac dysfunction in lipopolysaccharide-induced mice models and apoptosis in H9C2 cardiomyocytes. Ann Transl Med. (2020) 8:1147. doi: 10.21037/atm-20-5306
47. Zhu Y, Sun A, Meng T, Li H. Protective role of long noncoding RNA CRNDE in myocardial tissues from injury caused by sepsis through the microRNA-29a/SIRT1 axis. Life Sci. (2020) 255:117849. doi: 10.1016/j.lfs.2020.117849
48. Zhang XM, Li LB, Sun CH. The effect of myocardial infarction-associated transcript 2 (Mirt2) and miR-101 on sepsis-induced myocardial injury in rats. Eur Rev Med Pharmacol Sci. (2020) 24:6299–310. doi: 10.26355/eurrev_202006_21528
49. Tao H, Cao W, Yang JJ, Shi KH, Zhou X, Liu LP, et al. Long noncoding RNA H19 controls DUSP5/ERK1/2 axis in cardiac fibroblast proliferation and fibrosis. Cardiovasc Pathol. (2016) 25:381–9. doi: 10.1016/j.carpath.2016.05.005
50. Fang Y, Hu J, Wang Z, Zong H, Zhang L, Zhang R, et al. LncRNA H19 functions as an Aquaporin 1 competitive endogenous RNA to regulate microRNA-874 expression in LPS sepsis. Biomed Pharmacother. (2018) 105:1183–91. doi: 10.1016/j.biopha.2018.06.007
51. Chen M, Guan Y, Li A, Zhao YZ, Zhang L, Zhang L, et al. LncRNA SOX2OT mediates mitochondrial dysfunction in septic cardiomyopathy. DNA Cell Biol. (2019) 38:1197–206. doi: 10.1089/dna.2019.4839
52. Zhou T, Qin G, Yang L, Xiang D, Li S. LncRNA XIST regulates myocardial infarction by targeting miR-130a-3p. J Cell Physiol. (2019) 234:8659–67. doi: 10.1002/jcp.26327
53. Liang D, Jin Y, Lin M, Xia X, Chen X, Huang A. Down-regulation of Xist and Mir-7a-5p improves LPS-induced myocardial injury. Int J Med Sci. (2020) 17:2570–7. doi: 10.7150/ijms.45408
54. Carone N, Lingiardi V, Chirumbolo A, Baiocco R. Italian gay father families formed by surrogacy: parenting, stigmatization, and children's psychological adjustment. Dev Psychol. (2018) 54:1904–16. doi: 10.1037/dev0000571
55. Ratajczak MZ. Igf2-H19, an imprinted tandem gene, is an important regulator of embryonic development, a guardian of proliferation of adult pluripotent stem cells, a regulator of longevity, and a 'passkey' to cancerogenesis. Folia Histochem Cytobiol. (2012) 50:171–9. doi: 10.5603/FHC.2012.0026
56. Devlin AM, Bottiglieri T, Domann FE, Lentz SR. Tissue-specific changes in H19 methylation and expression in mice with hyperhomocysteinemia. J Biol Chem. (2005) 280:25506–11. doi: 10.1074/jbc.M504815200
57. Kim DK, Zhang L, Dzau VJ, Pratt RE. H19, a developmentally regulated gene, is reexpressed in rat vascular smooth muscle cells after injury. J Clin Invest. (1994) 93:355–60. doi: 10.1172/JCI116967
58. Han DK, Khaing ZZ, Pollock RA, Haudenschild CC, Liau G. H19, a marker of developmental transition, is reexpressed in human atherosclerotic plaques and is regulated by the insulin family of growth factors in cultured rabbit smooth muscle cells. J Clin Invest. (1996) 97:1276–85. doi: 10.1172/JCI118543
59. Ding GL, Wang FF, Shu J, Tian S, Jiang Y, Zhang D, et al. Transgenerational glucose intolerance with Igf2/H19 epigenetic alterations in mouse islet induced by intrauterine hyperglycemia. Diabetes. (2012) 61:1133–42. doi: 10.2337/db11-1314
60. Liu L, An X, Li Z, Song Y, Li L, Zuo S, et al. The H19 long noncoding RNA is a novel negative regulator of cardiomyocyte hypertrophy. Cardiovasc Res. (2016) 111:56–65. doi: 10.1093/cvr/cvw078
61. Chen C, Liu M, Tang Y, Sun H, Lin X, Liang P, et al. LncRNA H19 is involved in myocardial ischemic preconditioning via increasing the stability of nucleolin protein. J Cell Physiol. (2020) 235:5985–94. doi: 10.1002/jcp.29524
62. Shan B, Li JY, Liu YJ, Tang XB, Zhou Z, Luo LX. LncRNA H19 Inhibits the Progression of Sepsis-Induced Myocardial Injury via Regulation of the miR-93-5p/SORBS2 Axis. Inflammation. (2021) 44:344–57. doi: 10.1007/s10753-020-01340-8
63. González A, Fortuño MA, Querejeta R, Ravassa S, López B, López N, et al. Cardiomyocyte apoptosis in hypertensive cardiomyopathy. Cardiovasc Res. (2003) 59:549–62. doi: 10.1016/S0008-6363(03)00498-X
64. Chen K, Shi X, Jin Y, Wang F, Shen Q, Xu W. High lncRNA MEG3 expression is associated with high mortality rates in patients with sepsis and increased lipopolysaccharide-induced renal epithelial cell and cardiomyocyte apoptosis. Exp Ther Med. (2019) 18:3943–7. doi: 10.3892/etm.2019.8049
65. Schisterman EF, Sjaarda LA, Clemons T, Carrell DT, Perkins NJ, Johnstone E, et al. Effect of folic acid and zinc supplementation in men on semen quality and live birth among couples undergoing infertility treatment: a randomized clinical trial. JAMA. (2020) 323:35–48. doi: 10.1001/jama.2019.18714
66. Sun F, Yuan W, Wu H, Chen G, Sun Y, Yuan L, et al. LncRNA KCNQ1OT1 attenuates sepsis-induced myocardial injury via regulating miR-192-5p/XIAP axis. Exp Biol Med. (2020) 245:620–30. doi: 10.1177/1535370220908041
67. Li M, Zhang Z, Liu B, Chen L, Wang M. LncRNA GAS5 upregulates miR-214 through methylation to participate in cell apoptosis of sepsis. Arch Physiol Biochem. (2020) 1–6. doi: 10.1080/13813455.2020.1764051. [Epub ahead of print].
68. Shi S, Zhang S, Zhang H, Jin Q, Wu D. Silencing circANKRD36 protects H9c2 cells against lipopolysaccharide-induced injury via up-regulating miR-138. Exp Mol Pathol. (2019) 111:104300. doi: 10.1016/j.yexmp.2019.104300
69. Wang Q, Tao S, Zhu N, Li T, Yu L. Silencing circular RNA circANKRD36 remits lipopolysaccharide-induced inflammatory damage by regulating microRNA-15/MyD88. J Cell Biochem. (2020) 121:2704–12. doi: 10.1002/jcb.29490
70. Jiao L, Li M, Shao Y, Zhang Y, Gong M, Yang X, et al. lncRNA-ZFAS1 induces mitochondria-mediated apoptosis by causing cytosolic Ca(2+) overload in myocardial infarction mice model. Cell Death Dis. (2019) 10:942. doi: 10.1038/s41419-019-2136-6
71. Liu JJ, Li Y, Yang MS, Chen R, Cen CQ. SP1-induced ZFAS1 aggravates sepsis-induced cardiac dysfunction via miR-590-3p/NLRP3-mediated autophagy and pyroptosis. Arch Biochem Biophys. (2020) 695:108611. doi: 10.1016/j.abb.2020.108611
72. Chen DD, Wang HW, Cai XJ. Transcription factor Sp1 ameliorates sepsis-induced myocardial injury via ZFAS1/Notch signaling in H9C2 cells. Cytokine. (2021) 140:155426. doi: 10.1016/j.cyto.2021.155426
73. Liu W, Geng F, Yu L. Long non-coding RNA MALAT1/microRNA 125a axis presents excellent value in discriminating sepsis patients and exhibits positive association with general disease severity, organ injury, inflammation level, and mortality in sepsis patients. J Clin Lab Anal. (2020) 34:e23222. doi: 10.1002/jcla.23222
74. Yang J, Liu W, Xu M, Yu L. Long non-coding RNA CRNDE and toll-like receptor 3 correlate with disease severity, inflammation, and mortality in sepsis. J Clin Lab Anal. (2020) 34:e23360. doi: 10.1002/jcla.23360
75. Fattahi F, Russell MW, Malan EA, Parlett M, Abe E, Zetoune FS, et al. Harmful roles of TLR3 and TLR9 in cardiac dysfunction developing during polymicrobial sepsis. Biomed Res Int. (2018) 2018:4302726. doi: 10.1155/2018/4302726
76. Na L, Ding H, Xing E, Gao J, Liu B, Wang H, et al. Lnc-MEG3 acts as a potential biomarker for predicting increased disease risk, systemic inflammation, disease severity, and poor prognosis of sepsis via interacting with miR-21. J Clin Lab Anal. (2020) 34:e23123. doi: 10.1002/jcla.23123
77. Pan X, He L. LncRNA MEG3 expression in sepsis and its effect on LPS-induced macrophage function. Cell Mol Biol. (2020) 66:131–6. doi: 10.14715/cmb/2020.66.5.23
78. Wu X, Chen D, Yu L. The value of circulating long non-coding RNA maternally expressed gene 3 as a predictor of higher acute respiratory distress syndrome risk and 28-day mortality in sepsis patients. J Clin Lab Anal. (2020) 34:e23488. doi: 10.1002/jcla.23488
79. Yang Q, Cao K, Jin G, Zhang J. Hsa-miR-346 plays a role in the development of sepsis by downregulating SMAD3 expression and is negatively regulated by lncRNA MALAT1. Mol Cell Probes. (2019) 47:101444. doi: 10.1016/j.mcp.2019.101444
80. Xie W, Chen L, Chen L, Kou Q. Silencing of long non-coding RNA MALAT1 suppresses inflammation in septic mice: role of microRNA-23a in the down-regulation of MCEMP1 expression. Inflamm Res. (2020) 69:179–90. doi: 10.1007/s00011-019-01306-z
81. Huang S, Qian K, Zhu Y, Huang Z, Luo Q, Qing C. Diagnostic value of the lncRNA NEAT1 in peripheral blood mononuclear cells of patients with sepsis. Dis Mark. (2017) 2017:7962836. doi: 10.1155/2017/7962836
82. Gast M, Rauch BH, Haghikia A, Nakagawa S, Haas J, Stroux A, et al. Long noncoding RNA NEAT1 modulates immune cell functions and is suppressed in early onset myocardial infarction patients. Cardiovasc Res. (2019) 115:1886–906. doi: 10.1093/cvr/cvz085
83. Zhang Z, Lv M, Wang X, Zhao Z, Jiang D, Wang L. LncRNA LUADT1 sponges miR-195 to prevent cardiac endothelial cell apoptosis in sepsis. Mol Med. (2020) 26:112. doi: 10.1186/s10020-020-00228-5
84. Yu Z, Rayile A, Zhang X, Li Y, Zhao Q. Ulinastatin protects against lipopolysaccharide-induced cardiac microvascular endothelial cell dysfunction via downregulation of lncRNA MALAT1 and EZH2 in sepsis. Int J Mol Med. (2017) 39:1269–76. doi: 10.3892/ijmm.2017.2920
85. Liu L, Yan LN, Sui Z. MicroRNA-150 affects endoplasmic reticulum stress via MALAT1-miR-150 axis-mediated NF-κB pathway in LPS-challenged HUVECs and septic mice. Life Sci. (2021) 265:118744. doi: 10.1016/j.lfs.2020.118744
86. Zhang M, Wang X, Yao J, Qiu Z. Long non-coding RNA NEAT1 inhibits oxidative stress-induced vascular endothelial cell injury by activating the miR-181d-5p/CDKN3 axis. Artif Cells Nanomed Biotechnol. (2019) 47:3129–37. doi: 10.1080/21691401.2019.1646264
87. Zhang H, Ji N, Gong X, Ni S, Wang Y. NEAT1/miR-140-3p/MAPK1 mediates the viability and survival of coronary endothelial cells and affects coronary atherosclerotic heart disease. Acta Biochim Biophys Sin. (2020) 52:967–74. doi: 10.1093/abbs/gmaa087
88. Ahmed ASI, Dong K, Liu J, Wen T, Yu L, Xu F, et al. Long noncoding RNA NEAT1 (nuclear paraspeckle assembly transcript 1) is critical for phenotypic switching of vascular smooth muscle cells. Proc Natl Acad Sci USA. (2018) 115:E8660–7. doi: 10.1073/pnas.1803725115
89. Bai Y, Zhang Q, Su Y, Pu Z, Li K. Modulation of the proliferation/apoptosis balance of vascular smooth muscle cells in atherosclerosis by lncRNA-MEG3 via regulation of miR-26a/Smad1 axis. Int Heart J. (2019) 60:444–50. doi: 10.1536/ihj.18-195
90. Wang YN, Shan K, Yao MD, Yao J, Wang JJ, Li X, et al. Long noncoding RNA-GAS5: a novel regulator of hypertension-induced vascular remodeling. Hypertension. (2016) 68:736–48. doi: 10.1161/HYPERTENSIONAHA.116.07259
91. Congrains A, Kamide K, Katsuya T, Yasuda O, Oguro R, Yamamoto K, et al. CVD-associated non-coding RNA, ANRIL, modulates expression of atherogenic pathways in VSMC. Biochem Biophys Res Commun. (2012) 419:612–6. doi: 10.1016/j.bbrc.2012.02.050
92. Gui F, Peng H, Liu Y. Elevated circulating lnc-ANRIL/miR-125a axis level predicts higher risk, more severe disease condition, and worse prognosis of sepsis. J Clin Lab Anal. (2019) 33:e22917. doi: 10.1002/jcla.22917
93. Zhao CH, Cao HT, Zhang J, Jia QW, An FH, Chen ZH, et al. DNA methylation of antisense noncoding RNA in the INK locus (ANRIL) is associated with coronary artery disease in a Chinese population. Sci Rep. (2019) 9:15340. doi: 10.1038/s41598-019-51921-3
94. Bochenek G, Häsler R, El Mokhtari NE, König IR, Loos BG, Jepsen S, et al. The large non-coding RNA ANRIL, which is associated with atherosclerosis, periodontitis and several forms of cancer, regulates ADIPOR1, VAMP3 and C11ORF10. Hum Mol Genet. (2013) 22:4516–27. doi: 10.1093/hmg/ddt299
95. Holdt LM, Hoffmann S, Sass K, Langenberger D, Scholz M, Krohn K, et al. Alu elements in ANRIL non-coding RNA at chromosome 9p21 modulate atherogenic cell functions through trans-regulation of gene networks. PLoS Genet. (2013) 9:e1003588. doi: 10.1371/journal.pgen.1003588
96. Zhou X, Han X, Wittfeldt A, Sun J, Liu C, Wang X, et al. Long non-coding RNA ANRIL regulates inflammatory responses as a novel component of NF-κB pathway. RNA Biol. (2016) 13:98–108. doi: 10.1080/15476286.2015.1122164
97. Fang J, Pan Z, Guo X. [Research advance of ANRIL on atherosclerosis by regulating cell proliferation and apoptosis]. Zhejiang Da Xue Xue Bao Yi Xue Ban. (2020) 49:113–7.
98. Vausort M, Wagner DR, Devaux Y. Long noncoding RNAs in patients with acute myocardial infarction. Circ Res. (2014) 115:668–77. doi: 10.1161/CIRCRESAHA.115.303836
99. Hu L, Su G, Wang X. The roles of ANRIL polymorphisms in coronary artery disease: a meta-analysis. Biosci Rep. (2019) 39:BSR20181559. doi: 10.1042/BSR20181559
100. Cho H, Li Y, Archacki S, Wang F, Yu G, Chakrabarti S, et al. Splice variants of lncRNA RNA ANRIL exert opposing effects on endothelial cell activities associated with coronary artery disease. RNA Biol. (2020) 17:1391–401. doi: 10.1080/15476286.2020.1771519
101. Fang J, Pan Z, Wang D, Lv J, Dong Y, Xu R, et al. Multiple non-coding ANRIL transcripts are associated with risk of coronary artery disease: a promising circulating biomarker. J Cardiovasc Transl Res. (2020). doi: 10.1007/s12265-020-10053-0
102. Zhang C, Ge S, Gong W, Xu J, Guo Z, Liu Z, et al. LncRNA ANRIL acts as a modular scaffold of WDR5 and HDAC3 complexes and promotes alteration of the vascular smooth muscle cell phenotype. Cell Death Dis. (2020) 11:435. doi: 10.1038/s41419-020-2645-3
103. Zhang YN, Qiang B, Fu LJ. Association of ANRIL polymorphisms with coronary artery disease: a systemic meta-analysis. Medicine. (2020) 99:e22569. doi: 10.1097/MD.0000000000022569
104. Chen L, Qu H, Guo M, Zhang Y, Cui Y, Yang Q, et al. ANRIL and atherosclerosis. J Clin Pharm Ther. (2020) 45:240–8. doi: 10.1111/jcpt.13060
105. Liu X, Li S, Yang Y, Sun Y, Yang Q, Gu N, et al. The lncRNA ANRIL regulates endothelial dysfunction by targeting the let-7b/TGF-βR1 signalling pathway. J Cell Physiol. (2021) 236:2058–69. doi: 10.1002/jcp.29993
106. Yang J, Huang X, Hu F, Fu X, Jiang Z, Chen K. LncRNA ANRIL knockdown relieves myocardial cell apoptosis in acute myocardial infarction by regulating IL-33/ST2. Cell Cycle. (2019) 18:3393–403. doi: 10.1080/15384101.2019.1678965
107. Huang Q, Pan M, Zhou JP, Yin F. Overexpression of long non-coding RNA ANRIL promotes post-ischaemic angiogenesis and improves cardiac functions by targeting Akt. J Cell Mol Med. (2020) 24:6860–8. doi: 10.1111/jcmm.15343
108. Guo F, Tang C, Li Y, Liu Y, Lv P, Wang W, et al. The interplay of LncRNA ANRIL and miR-181b on the inflammation-relevant coronary artery disease through mediating NF-κB signalling pathway. J Cell Mol Med. (2018) 22:5062–75. doi: 10.1111/jcmm.13790
109. Xie Z, Guo Z, Liu J. Whey acidic protein/four-disulfide core domain 21 regulate sepsis pathogenesis in a mouse model and a macrophage cell line via the Stat3/Toll-like receptor 4 (TLR4) signaling pathway. Med Sci Monit. (2018) 24:4054–63. doi: 10.12659/MSM.907176
110. Alikhah A, Pahlevan Kakhki M, Ahmadi A, Dehghanzad R, Boroumand MA, Behmanesh M. The role of lnc-DC long non-coding RNA and SOCS1 in the regulation of STAT3 in coronary artery disease and type 2 diabetes mellitus. J Diabetes Complications. (2018) 32:258–65. doi: 10.1016/j.jdiacomp.2017.12.001
111. Liu T, Liu J, Tian C, Wang H, Wen M, Yan M. LncRNA THRIL is upregulated in sepsis and sponges miR-19a to upregulate TNF-α in human bronchial epithelial cells. J Inflamm. (2020) 17:31. doi: 10.1186/s12950-020-00259-z
112. Li Z, Chao TC, Chang KY, Lin N, Patil VS, Shimizu C, et al. The long noncoding RNA THRIL regulates TNFα expression through its interaction with hnRNPL. Proc Natl Acad Sci USA. (2014) 111:1002–7. doi: 10.1073/pnas.1313768111
113. Xiao J, Lu Y, Yang X. THRIL mediates endothelial progenitor cells autophagy via AKT pathway and FUS. Mol Med. (2020) 26:86. doi: 10.1186/s10020-020-00201-2
114. Xia J, Jiang N, Li Y, Wei Y, Zhang X. The long noncoding RNA THRIL knockdown protects hypoxia-induced injuries of H9C2 cells through regulating miR-99a. Cardiol J. (2019) 26:564–74. doi: 10.5603/CJ.a2018.0054
115. Sheng C, Hu F, Wu L. Geniposide alleviates hypoxia-induced injury by down-regulation of lncRNA THRIL in rat cardiomyocytes derived H9c2 cells. Eur J Pharmacol. (2019) 854:28–38. doi: 10.1016/j.ejphar.2019.03.058
116. Qi H, Shen J, Zhou W. Up-regulation of long non-coding RNA THRIL in coronary heart disease: Prediction for disease risk, correlation with inflammation, coronary artery stenosis, and major adverse cardiovascular events. J Clin Lab Anal. (2020) 34:e23196. doi: 10.1002/jcla.23196
117. Wang W, Lou C, Gao J, Zhang X, Du Y. LncRNA SNHG16 reverses the effects of miR-15a/16 on LPS-induced inflammatory pathway. Biomed Pharmacother. (2018) 106:1661–7. doi: 10.1016/j.biopha.2018.07.105
118. Huang L, Ding Y, Yang L, Jiang X, Xia Z, You Z. The effect of LncRNA SNHG16 on vascular smooth muscle cells in CHD by targeting miRNA-218-5p. Exp Mol Pathol. (2021) 118:104595. doi: 10.1016/j.yexmp.2020.104595
119. Wang D, Lin B, Zhang W, Wang X. Up-regulation of SNHG16 induced by CTCF accelerates cardiac hypertrophy by targeting miR-182-5p/IGF1 axis. Cell Biol Int. (2020) 44:1426–35. doi: 10.1002/cbin.11333
120. Chen Y, Fu Y, Song YF, Li N. Increased expression of lncRNA UCA1 and HULC is required for pro-inflammatory response during LPS induced sepsis in endothelial cells. Front Physiol. (2019) 10:608. doi: 10.3389/fphys.2019.00608
121. Zhao YJ, Chen YE, Zhang HJ, Gu X. LncRNA UCA1 remits LPS-engendered inflammatory damage through deactivation of miR-499b-5p/TLR4 axis. IUBMB Life. (2021) 73:463–73. doi: 10.1002/iub.2443
122. Chen J, Hu Q, Zhang BF, Liu XP, Yang S, Jiang H. Long noncoding RNA UCA1 inhibits ischaemia/reperfusion injury induced cardiomyocytes apoptosis via suppression of endoplasmic reticulum stress. Genes Genomics. (2019) 41:803–10. doi: 10.1007/s13258-019-00806-w
123. Chen ZL, Chen YX, Zhou J, Li Y, Gong CY, Wang XB. LncRNA HULC alleviates HUVEC inflammation and improves angiogenesis after myocardial infarction through down-regulating miR-29b. Eur Rev Med Pharmacol Sci. (2020) 24:6288–98. doi: 10.26355/eurrev_202006_21527
124. Ma Y, Huang D, Yang F, Tian M, Wang Y, Shen D, et al. Long noncoding RNA highly upregulated in liver cancer regulates the tumor necrosis factor-α-induced apoptosis in human vascular endothelial cells. DNA Cell Biol. (2016) 35:296–300. doi: 10.1089/dna.2015.3203
125. Liang H, Li F, Li H, Wang R, Du M. Overexpression of lncRNA HULC attenuates myocardial ischemia/reperfusion injury in rat models and apoptosis of hypoxia/reoxygenation cardiomyocytes via targeting miR-377-5p through NLRP3/Caspase-1/IL-1β signaling pathway inhibition. Immunol Invest. (2020) 1–14. doi: 10.1080/08820139.2020.1791178. [Epub ahead of print].
126. Huarte M, Guttman M, Feldser D, Garber M, Koziol MJ, Kenzelmann-Broz D, et al. A large intergenic noncoding RNA induced by p53 mediates global gene repression in the p53 response. Cell. (2010) 142:409–19. doi: 10.1016/j.cell.2010.06.040
127. Wu G, Cai J, Han Y, Chen J, Huang ZP, Chen C, et al. LincRNA-p21 regulates neointima formation, vascular smooth muscle cell proliferation, apoptosis, and atherosclerosis by enhancing p53 activity. Circulation. (2014) 130:1452–65. doi: 10.1161/CIRCULATIONAHA.114.011675
128. Dimitrova N, Zamudio JR, Jong RM, Soukup D, Resnick R, Sarma K, et al. LincRNA-p21 activates p21 in cis to promote Polycomb target gene expression and to enforce the G1/S checkpoint. Mol Cell. (2014) 54:777–90. doi: 10.1016/j.molcel.2014.04.025
129. Trakala M, Arias CF, García MI, Moreno-Ortiz MC, Tsilingiri K, Fernández PJ, et al. Regulation of macrophage activation and septic shock susceptibility via p21(WAF1/CIP1). Eur J Immunol. (2009) 39:810–9. doi: 10.1002/eji.200838676
130. Huang S, Xu M, Liu L, Yang J, Wang H, Wan C, et al. Autophagy is involved in the protective effect of p21 on LPS-induced cardiac dysfunction. Cell Death Dis. (2020) 11:554. doi: 10.1038/s41419-020-02765-7
131. Gu C, Liu M, Zhao T, Zhai L, Wang Y. Recombinant human annexin A5 can repair the disrupted cardiomyocyte adherens junctions in endotoxemia. Shock. (2015) 44:83–9. doi: 10.1097/SHK.0000000000000370
132. Shi L, Tian C, Sun L, Cao F, Meng Z. The lncRNA TUG1/miR-145-5p/FGF10 regulates proliferation and migration in VSMCs of hypertension. Biochem Biophys Res Commun. (2018) 501:688–95. doi: 10.1016/j.bbrc.2018.05.049
133. Zhang L, Cheng H, Yue Y, Li S, Zhang D, He R. TUG1 knockdown ameliorates atherosclerosis via up-regulating the expression of miR-133a target gene FGF1. Cardiovasc Pathol. (2018) 33:6–15. doi: 10.1016/j.carpath.2017.11.004
134. Qiu N, Xu X, He Y. LncRNA TUG1 alleviates sepsis-induced acute lung injury by targeting miR-34b-5p/GAB1. BMC Pulm Med. (2020) 20:49. doi: 10.1186/s12890-020-1084-3
135. Zolk O, Solbach TF, Eschenhagen T, Weidemann A, Fromm MF. Activation of negative regulators of the hypoxia-inducible factor (HIF) pathway in human end-stage heart failure. Biochem Biophys Res Commun. (2008) 376:315–20. doi: 10.1016/j.bbrc.2008.08.152
136. Han F, Wu G, Han S, Li Z, Jia Y, Bai L, et al. Hypoxia-inducible factor prolyl-hydroxylase inhibitor roxadustat (FG-4592) alleviates sepsis-induced acute lung injury. Respir Physiol Neurobiol. (2020) 281:103506. doi: 10.1016/j.resp.2020.103506
137. Li X, Yu J, Gong L, Zhang Y, Dong S, Shi J, et al. Heme oxygenase-1(HO-1) regulates Golgi stress and attenuates endotoxin-induced acute lung injury through hypoxia inducible factor-1α (HIF-1α)/HO-1 signaling pathway. Free Radic Biol Med. (2021) 165:243–53. doi: 10.1016/j.freeradbiomed.2021.01.028
138. Papanikolaou J, Makris D, Mpaka M, Palli E, Zygoulis P, Zakynthinos E. New insights into the mechanisms involved in B-type natriuretic peptide elevation and its prognostic value in septic patients. Crit Care. (2014) 18:R94. doi: 10.1186/cc13864
139. Jefic D, Lee JW, Jefic D, Savoy-Moore RT, Rosman HS. Utility of B-type natriuretic peptide and N-terminal pro B-type natriuretic peptide in evaluation of respiratory failure in critically ill patients. Chest. (2005) 128:288–95. doi: 10.1378/chest.128.1.288
140. Røsjø H, Varpula M, Hagve TA, Karlsson S, Ruokonen E, Pettilä V, et al. Circulating high sensitivity troponin T in severe sepsis and septic shock: distribution, associated factors, and relation to outcome. Intensive Care Med. (2011) 37:77–85. doi: 10.1007/s00134-010-2051-x
141. Orde SR, Pulido JN, Masaki M, Gillespie S, Spoon JN, Kane GC, et al. Outcome prediction in sepsis: speckle tracking echocardiography based assessment of myocardial function. Crit Care. (2014) 18:R149. doi: 10.1186/cc13987
142. De Geer L, Engvall J, Oscarsson A. Strain echocardiography in septic shock - a comparison with systolic and diastolic function parameters, cardiac biomarkers and outcome. Crit Care. (2015) 19:122. doi: 10.1186/s13054-015-0857-1
143. Levy RJ, Piel DA, Acton PD, Zhou R, Ferrari VA, Karp JS, et al. Evidence of myocardial hibernation in the septic heart. Crit Care Med. (2005) 33:2752–6. doi: 10.1097/01.CCM.0000189943.60945.77
144. Coopersmith CM, De Backer D, Deutschman CS, Ferrer R, Lat I, Machado FR, et al. Surviving sepsis campaign: research priorities for sepsis and septic shock. Intensive Care Med. (2018) 44:1400–26. doi: 10.1007/s00134-018-5175-z
145. Annane D, Vignon P, Renault A, Bollaert PE, Charpentier C, Martin C, et al. Norepinephrine plus dobutamine versus epinephrine alone for management of septic shock: a randomised trial. Lancet. (2007) 370:676–84. doi: 10.1016/S0140-6736(07)61344-0
146. Yealy DM, Kellum JA, Huang DT, Barnato AE, Weissfeld LA, Pike F, et al. A randomized trial of protocol-based care for early septic shock. N Engl J Med. (2014) 370:1683–93. doi: 10.1056/NEJMoa1401602
147. Gordon AC, Perkins GD, Singer M, Mcauley DF, Orme RM, Santhakumaran S, et al. Levosimendan for the prevention of acute organ dysfunction in sepsis. N Engl J Med. (2016) 375:1638–48. doi: 10.1056/NEJMoa1609409
148. Zhang Y, Zhang YY, Xia F, Yang AX, Qian JX, Zhao H, et al. Effect of lncRNA-MIAT on kidney injury in sepsis rats via regulating miR-29a expression. Eur Rev Med Pharmacol Sci. (2019) 23:10942–9.
149. Schlosser K, Hanson J, Villeneuve PJ, Dimitroulakos J, Mcintyre L, Pilote L, et al. Assessment of circulating LncRNAs under physiologic and pathologic conditions in humans reveals potential limitations as biomarkers. Sci Rep. (2016) 6:36596. doi: 10.1038/srep36596
150. Wang KC, Yang YW, Liu B, Sanyal A, Corces-Zimmerman R, Chen Y, et al. A long noncoding RNA maintains active chromatin to coordinate homeotic gene expression. Nature. (2011) 472:120–4. doi: 10.1038/nature09819
151. Li W, Notani D, Ma Q, Tanasa B, Nunez E, Chen AY, et al. Functional roles of enhancer RNAs for oestrogen-dependent transcriptional activation. Nature. (2013) 498:516–20. doi: 10.1038/nature12210
152. Matsui M, Corey DR. Non-coding RNAs as drug targets. Nat Rev Drug Discov. (2017) 16:167–79. doi: 10.1038/nrd.2016.117
153. Lennox KA, Behlke MA. Cellular localization of long non-coding RNAs affects silencing by RNAi more than by antisense oligonucleotides. Nucleic Acids Res. (2016) 44:863–77. doi: 10.1093/nar/gkv1206
154. Huang Z, Zhou JK, Peng Y, He W, Huang C. The role of long noncoding RNAs in hepatocellular carcinoma. Mol Cancer. (2020) 19:77. doi: 10.1186/s12943-020-01188-4
155. Sarma K, Levasseur P, Aristarkhov A, Lee JT. Locked nucleic acids (LNAs) reveal sequence requirements and kinetics of Xist RNA localization to the X chromosome. Proc Natl Acad Sci USA. (2010) 107:22196–201. doi: 10.1073/pnas.1009785107
156. Prakash TP, Graham MJ, Yu J, Carty R, Low A, Chappell A, et al. Targeted delivery of antisense oligonucleotides to hepatocytes using triantennary N-acetyl galactosamine improves potency 10-fold in mice. Nucleic Acids Res. (2014) 42:8796–807. doi: 10.1093/nar/gku531
157. Goyal A, Myacheva K, Groß M, Klingenberg M, Duran Arqué B, Diederichs S. Challenges of CRISPR/Cas9 applications for long non-coding RNA genes. Nucleic Acids Res. (2017) 45:e12. doi: 10.1093/nar/gkw883
158. Zhu S, Li W, Liu J, Chen CH, Liao Q, Xu P, et al. Genome-scale deletion screening of human long non-coding RNAs using a paired-guide RNA CRISPR-Cas9 library. Nat Biotechnol. (2016) 34:1279–86. doi: 10.1038/nbt.3715
159. Liu SJ, Horlbeck MA, Cho SW, Birk HS, Malatesta M, He D, et al. CRISPRi-based genome-scale identification of functional long noncoding RNA loci in human cells. Science. (2017) 355:aah7111. doi: 10.1126/science.aah7111
160. Shechner DM, Hacisuleyman E, Younger ST, Rinn JL. Multiplexable, locus-specific targeting of long RNAs with CRISPR-Display. Nat Methods. (2015) 12:664–70. doi: 10.1038/nmeth.3433
161. Thi EP, Dhillon AP, Ardzinski A, Bidirici-Ertekin L, Cobarrubias KD, Cuconati A, et al. ARB-1740, a RNA interference therapeutic for chronic hepatitis B infection. ACS Infect Dis. (2019) 5:725–37. doi: 10.1021/acsinfecdis.8b00191
162. Ruan ZB, Wang F, Gongben BD, Chen GC, Zhu L. Identification of circulating lncRNA expression profiles in patients with atrial fibrillation. Dis Mark. (2020) 2020:8872142. doi: 10.1155/2020/8872142
163. Diederichs S. The four dimensions of noncoding RNA conservation. Trends Genet. (2014) 30:121–3. doi: 10.1016/j.tig.2014.01.004
164. Schorderet P, Duboule D. Structural and functional differences in the long non-coding RNA hotair in mouse and human. PLoS Genet. (2011) 7:e1002071. doi: 10.1371/journal.pgen.1002071
165. Okamoto I, Arnaud D, Le Baccon P, Otte AP, Disteche CM, Avner P, et al. Evidence for de novo imprinted X-chromosome inactivation independent of meiotic inactivation in mice. Nature. (2005) 438:369–73. doi: 10.1038/nature04155
166. Chodroff RA, Goodstadt L, Sirey TM, Oliver PL, Davies KE, Green ED, et al. Long noncoding RNA genes: conservation of sequence and brain expression among diverse amniotes. Genome Biol. (2010) 11:R72. doi: 10.1186/gb-2010-11-7-r72
167. Juan V, Crain C, Wilson C. Evidence for evolutionarily conserved secondary structure in the H19 tumor suppressor RNA. Nucleic Acids Res. (2000) 28:1221–7. doi: 10.1093/nar/28.5.1221
168. Pegueroles C, Gabaldón T. Secondary structure impacts patterns of selection in human lncRNAs. BMC Biol. (2016) 14:60. doi: 10.1186/s12915-016-0283-0
169. Amodio N, Raimondi L, Juli G, Stamato MA, Caracciolo D, Tagliaferri P, et al. MALAT1: a druggable long non-coding RNA for targeted anti-cancer approaches. J Hematol Oncol. (2018) 11:63. doi: 10.1186/s13045-018-0606-4
170. Wang M, Xu T, Feng W, Liu J, Wang Z. Advances in understanding the LncRNA-mediated regulation of the hippo pathway in cancer. Onco Targets Ther. (2021) 14:2397–415. doi: 10.2147/OTT.S283157
171. Uszczynska-Ratajczak B, Lagarde J, Frankish A, Guigó R, Johnson R. Towards a complete map of the human long non-coding RNA transcriptome. Nat Rev Genet. (2018) 19:535–48. doi: 10.1038/s41576-018-0017-y
172. Joung J, Engreitz JM, Konermann S, Abudayyeh OO, Verdine VK, Aguet F, et al. Erratum: genome-scale activation screen identifies a lncRNA locus regulating a gene neighbourhood. Nature. (2017) 549:418. doi: 10.1038/nature24009
173. Patil DP, Chen CK, Pickering BF, Chow A, Jackson C, Guttman M, et al. m(6)A RNA methylation promotes XIST-mediated transcriptional repression. Nature. (2016) 537:369–73. doi: 10.1038/nature19342
Keywords: long non-coding RNA, sepsis, cardiac dysfunction, biomarker, gene therapy
Citation: Li J, Zhang Y, Zhang D and Li Y (2021) The Role of Long Non-coding RNAs in Sepsis-Induced Cardiac Dysfunction. Front. Cardiovasc. Med. 8:684348. doi: 10.3389/fcvm.2021.684348
Received: 23 March 2021; Accepted: 16 April 2021;
Published: 10 May 2021.
Edited by:
Zhanpeng Huang, The First Affiliated Hospital of Sun Yat-sen University, ChinaReviewed by:
Jinghai Chen, Zhejiang University, ChinaPingzhu Zhou, Boston Children's Hospital and Harvard Medical School, United States
Copyright © 2021 Li, Zhang, Zhang and Li. This is an open-access article distributed under the terms of the Creative Commons Attribution License (CC BY). The use, distribution or reproduction in other forums is permitted, provided the original author(s) and the copyright owner(s) are credited and that the original publication in this journal is cited, in accordance with accepted academic practice. No use, distribution or reproduction is permitted which does not comply with these terms.
*Correspondence: Yifei Li, bGl5Zndjc2hAc2N1LmVkdS5jbg==; Donghui Zhang, ZG9uZ2guemhhbmdAaHVidS5lZHUuY24=
†These authors have contributed equally to this work