- Rappaport Family Faculty of Medicine and Research Institute, Technion – Israel Institute of Technology, Haifa, Israel
The ability of a given genotype to produce different phenotypes in response to different environments is termed “plasticity,” and is part of the organism’s “adaptability” to environmental cues. The expressions of suites of genes, particularly during development or life history transitions, probably underlie the fundamental plasticity of an organism. Plasticity in developmental programming has evolved in order to provide the best chances of survival and reproductive success to organisms under changing environments. Environmental conditions that are experienced in early life can profoundly influence human biology, child growth and maturation, and long-term health and longevity. Developmental origins of health and disease and life history transitions are purported to use placental, nutritional, and endocrine cues for setting long-term biological, mental, and behavioral strategies for child growth and maturation in response to local ecological and/or social conditions. The window of developmental plasticity extends from conception to early childhood, and even beyond to the transition from juvenility to adolescence, and could be transmitted transgenerationally. It involves epigenetic responses to environmental changes, which exert their effects during life history phase transitions.
Trait variability, irrespective of whether it is physiological, morphological, behavioral, molecular, or cellular, is the leading edge of evolution. When facing the challenge of developing an individual that best fits its environment, nature demonstrates an interesting combination of five different adaptive processes that influence human phenotype, and each process operates on a different time scale (Table 1; Muller, 2007; Hochberg et al., 2011a). The first adaptive process involves changes in gene sequence and frequency in a population or species; for this type of adaptation, time is an important constraint – adaptive a genotype occurs over several hundred thousand years. The second process is the modification of homozygosity in a population, and this process occurs over several hundred years and numerous generations. The third process refers to plasticity, and this process occurs over the total life span of the individual, and may be carried forward for three to four generations. The fourth process is short-term acclimatization that can last several months or years. The fifth adaptive mechanism involves cultural adaptation, which also tends to be of moderate pace in the hundreds of years.
The ability of a given genotype to produce different phenotypes in response to different environments is termed “plasticity,” and is part of the organism’s “adaptability” to environmental cues (Bateson et al., 2004). The expressions of suites of genes, particularly during development or life history transitions, probably underlie the fundamental plasticity of an organism (Crews et al., 2007).
It was recently appreciated that the life history evolutionary theory is a powerful tool for understanding child growth and development from an evolutionary perspective (Hochberg, 2009). By applying this theory to developmental data, adaptive growth- and metabolic-related strategies for transition from one life history phase to the next and the timing of such transitions (inherent adaptive plasticity) have evolved.
The environmental conditions that are experienced in early life can profoundly influence human biology and long-term health. Early life nutrition and stress are among the best documented examples of such conditions because they influence the adult risk of developing metabolic diseases, such as type 2 diabetes mellitus (T2D), and cardiovascular diseases (Barker, 1995). Individuals who are born small-for-gestational age (SGA) have an increased risk of cardiovascular morbidity and mortality when they are adults (Barker and Osmond, 1986; Barker et al., 1989; Barker, 1995, 2006). This epidemiological evidence is now supported by an extensive experimental literature in animals (Gluckman et al., 2008). Evidence on the importance of prenatal and early postnatal growth for later morbidity suggests the existence of a link between developmental responses to early environments and adult biology. These associations are grounded in functional relationships, and are broadly consistent with life history evolution theory.
Plasticity in Developmental Programming
Environments change continuously, and a species adapts its phenotype to the prevailing environment, even when the environmental change is disruptive. A species is considered to be well adapted and fit in evolutionary terms when it can survive to reproduce, and display relative phenotypic consistency across many generations. Phenotype stability is most likely to occur when the species has adapted to a normative range of environments which remains relatively stable on generational time-scales. Yet, organisms exist within an environment that can change rapidly, and those species with a relatively fixed phenotype may not be able to respond sufficiently quickly in order to survive an unexpected environmental change. Adaptive plasticity enables a species to respond to an environmental change in order to survive and reproduce, and may manifest itself as polyphenism (alternative phenotypes in different environments, such as in metamorphosis) or as a continuous variation in traits. However, not all developmental responses to environmental cues have an adaptive basis. When the cue is severe or novel, the outcome may be disruptive, and may result in teratogenesis, disease, or death (Gluckman and Hanson, 2007a).
The predictability of environmental changes is also an important determinant of the degree of adaptive flexibility of a species (Hochberg et al., 2011a). In some instances, the environmental change is highly predictable, and an adapted species exists as a limited range of subtle, but distinct and definable, phenotypes. Adaptive plasticity of an organism is associated with immediate adaptive responses (forecasting or predicting), which are concerned with its immediate survival with no consideration for the long-term consequences (Gluckman and Hanson, 2007a). These adaptive responses adjust the developmental phenotype, and comprise a set of processes that can be triggered by a wide range of environmental cues in order to promote lifetime fitness.
Recognition of an environmental cue often occurs during sensitive periods in the lifespan of a species, namely the prenatal period and/or during transitions between life history phases (Hochberg, 2009; Hochberg et al., 2011a). Recognition of an environmental cue also enables the organism to adapt or acclimatize to an environment change, and creates future trajectories in its development. The resultant adaptive advantage depends upon the fidelity of the cue about the future state of the environment. High fidelity cues enable the organism to optimize its adaptation or fit to the anticipated environment. Low fidelity cues carry a fitness disadvantage, although the impact will depend upon the extent of mismatch between the predicted and actual future environment.
Two types of adaptive responses or plasticity exist (Gluckman and Hanson, 2007a). The first types are the anticipatory or predictive adaptive responses where the developing organism forecasts the future environment, and then adjusts its phenotypic trajectory accordingly. The second types are the immediate adaptive responses which promote short-term maternal or fetal survival with some advantages in later life (developmental plasticity). Since these two adaptive responses come with a significant cost, individual members of a species make a cost–benefit analysis in order to determine the true value of an adaptive response. Within the adaptive responses, the organism may engage in a trade-off between phenotypic changes in order to ensure its short-term survival at the expense of a long-term advantage. Hence, trade-offs occur because energy needs to be allocated in order to meet the different metabolic and physiological demands of a developing organism. Therefore, trade-offs can often manifest themselves as longevity as an alternative to reduced survival of the juveniles. Such is the consequence of embryonic fetal development when it occurs in a deprived intrauterine environment as a result of a limited transplacental nutrient supply. In response, the fetus protects the development of its heart and brain at the expense of other organs, and somatic growth is retarded. Intrauterine growth restriction (IUGR) is an example of an immediate cryptically maladaptive response to the environment (Gluckman and Hanson, 2005).
Plasticity in Phase Transitions of Human Life History
The secular trends in child growth and puberty are dazzling examples of such adaptation (Arcaleni, 2006). European men are now 13 cm taller than they were 150 years ago. This range of plasticity in growth over approximately six generations is not long enough to result from changes in the DNA sequence. Over the same six generations, the age of menarche in Western countries has decreased by 4 years. This reduction has a theoretical fitness advantage on the fecundity span in an environment that is rich in energy resources, and demonstrates plasticity in the maturation of the hypothalamic–pituitary–gonadal (HPG) axis. As a consequence of constantly changing life conditions and environment, today’s children may be stunted in growth or be tall, adapt their body composition and energy metabolism, and modulate their longevity, fertility, and fecundity. The signals of energy balance that modulate this plasticity are both intrinsic (internal) and extrinsic (environmental; Hochberg et al., 2011a). The internal signals include leptin, the growth hormone – insulin-like growth factor 1 (GH–IGF1) axis, ghrelin, thyroid hormones, insulin, and the cortisone–cortisol shuttle (11β-hydroxysteroid dehydrogenases), whereas the environmental signals include pre-and postnatal nutrition, stressors, endocrine disrupting chemicals (EDCs), and light (Gluckman and Hanson, 2005).
Human growth and development is an orchestrated process of well-recognized and predictable events with five overlapping, yet distinct, pre-adult life history phases: the prenatal, infantile, childhood, juvenile, and pubertal growth phases (Figure 1). The transition periods between these phases are sensitive windows of developmental plasticity, and there is now some evidence that the features of transition from one phase to the next are transmitted transgenerationally (Stein et al., 2004). With decreasing sensitivity, the transitions between phases are periods of adaptive plasticity, and the multifactorial regulation of growth during each phase mirrors the interplay between genetic, hormonal, environmental, and psychosocial factors.
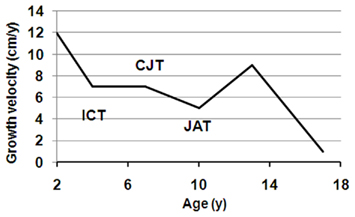
Figure 1. Growth during pre-adult life history stages. The 50th percentile of first derivatives for both boys (solid line) and girls (dashed line) were calculated from the US CDC (2000) data. The upper part indicates the four pre-adult life history stages: infancy (I), childhood (C), juvenility (J), and adolescence (A). The three transition points are marked by a circle, and designated as ICT, infancy–childhood transition; CJT, childhood–juvenility transition; JAT, juvenility–adolescence transition.
In response to environmental cues, especially those that relate to energy resources, a life history phase can be added or deleted (such as the added childhood phase in hominids), and can have its duration, intensity, and onset time altered (Hochberg and Albertsson-Wikland, 2008; Hochberg, 2009; Hochberg et al., 2011a). Thus, the timing of infancy–childhood transition (ICT) adaptively adjusts an individual’s size to the prevailing environment in response to environmental cues (Hochberg and Albertsson-Wikland, 2008; Gawlik et al., 2011). We have previously reported that the ICT is a major determinant of final adult height, and a delayed ICT is the most common cause of idiopathic short stature (Hochberg and Albertsson-Wikland, 2008). The transition from childhood to juvenility is entrusted with the programming of body composition (Hochberg, 2008, 2010). The transition from juvenility to adolescent-related puberty and the growth spurt is a function of maturation of the HPG axis. Poor quality of life during this transition delays fecundity and increases longevity (Pembrey et al., 2006). Hence, a series of control mechanisms must exist in order to enable (a) the GH–IGF1 axis to dominate as the child transits into childhood, (b) adrenarche at the onset of juvenility, and (c) an abrupt increase in sex hormones at initiation of puberty.
As already noted, an organism distributes its energy resources during its life by timed allocations toward growth, maintenance, avoiding death, reproduction, and raising offspring to independence in order to enhance its reproductive fitness (Bogin et al., 2007; Muller, 2007). Whereas the environment at any one geographical location may vary slowly, nutritional conditions may change rapidly. Evolution has provided organisms with the mechanisms to adapt to such extremes. Humans can also use socio-cultural adjustments to fill the gaps when the changes occur faster than the evolutionary time scale. This can be seen when one examines the evolution of hominid life history from Australopithecus afarensis to Homo sapiens. In humans, the duration of infancy has become shortened, and that of childhood has been prolonged, and these two phases are followed by a relative short juvenility and late adolescence in order to increase fitness (Pratt et al., 1994; Bogin et al., 2007; Hochberg, 2008). The overall result of this strategy is increased body size and longevity, and reproduction at a later age, as compared to other primates. This strategy has been very successful for humans, who can thrive and propagate in extremely diverse environments that encompass the entire range of geographic latitudes and altitudes.
An important environmental cue for infants and young children is the care giving behaviors of their parents, which can be used as a predictive indicator of the security of their environment. The resultant attachment patterns are transmitted transgenerationally (Belsky and Fearon, 2002; Del Giudice, 2009). The degree of security that is experienced during childhood sets development on alternative pathways, and adaptively shapes the individual’s future reproductive strategy. A secure attachment will result in a reproductive strategy that is based on late maturation, a commitment to a long-term relationship, and a large investment in parenting. In terms of evolutionary developmental biology (evo-devo), which studies the developmental mechanisms that control body shape and form and the alterations in gene expression and function that lead to changes in body shape and pattern (Goodman and Coughlin, 2000), the expected response to a secure environment will include investment in large body size (Liu et al., 1998, 2000). This example of transgenerational phenotypic plasticity contrasts that of an insecure attachment and a small parental investment that involves a large number of children: the response is a compromise in body size, early reproduction, and short-term mating.
Child growth and body composition display a vast range of adaptive plasticity. Short-term plasticity in the various child growth phases and transitions suggests that epigenetic mechanisms determine the extent of adaptive plasticity during growth in response to environmental cues. In the light of these new findings, this issue considers the utility of life history theory, and the links between epigenetics, developmental programming, and plasticity in early growth and nutrition. Current research in child health strives to identify mechanisms that underlie plasticity in developmental programming and life history transitions. Developmental programming and life history transitions are purported to use nutritional or endocrine cues for setting long-term biological strategies in response to local ecological and/or social conditions (Bateson et al., 2004; Gluckman and Hanson, 2007b; Kuzawa, 2007). Rapid changes in nutrition during one’s lifetime can then lead to “mismatch” and metabolic disease (Gluckman and Hanson, 2007b). It has been further proposed that intergenerational influences on nutrition and growth stabilize the nutritional signals that are received in utero in order to increase the reliability of an intrauterine cue as a predictive signal (Kuzawa, 2007). It is now also known that the effects of hormones, stress, and drugs during embryogenesis can not only influence the subsequent behavioral phenotype of the individual, but can also modify the individual’s response to adult experiences (Crews et al., 2007).
Plasticity in Human Growth
Postnatal growth in body weight and stature can be assessed by three measures: growth velocity, attained body size, and the timing or “tempo” of growth, which is a measure of how rapidly an individual achieves its growth potential. Human growth rates differ markedly between individuals, particularly during the most rapid phases of growth, which occur during infancy and adolescence (Parent et al., 2003; Ong et al., 2011).
Human growth demonstrates both “elasticity” and “plasticity” (or long-term programming) during the different growth periods. The concepts of growth elasticity and plasticity arose from the results of studies in experimental animals that date back to the sixties in which the influence of nutrition on growth was investigated. The results of these studies demonstrated that there are critical time windows in which the outcome of a programmed growth trajectory can be changed. McCance and Widdowson were the first to report this phenomenon when they showed that the exact timing of undernourishment in the growth phase can exert either a permanent or transient effect on final body size (McCance, 1962; McCance and Widdowson, 1974). When rats are transiently undernourished (food-restricted) in very early postnatal life, they remained smaller throughout later life than control rats which are not undernourished. In contrast, rats which are transiently undernourished during later growth phases show catch-up growth after the period of under-nutrition and attain the same adult weights as the control rats.
While human growth may be impacted by severe acute or chronic diseases, there is growing awareness that growth rates, and in particular the tempo of growth, may have marked influences on the subsequent risks for morbidity and mortality, and hence reproductive fitness. Birth weight is strongly correlated with perinatal mortality, and is the single strongest predictor of infant survival. Neonates who are born at term and weigh between 1500 and 2500 g (<10th percentile) have a 5- to 30-fold increase in perinatal morbidity and mortality when compared with neonates whose birth weights lie between 10th and 90th percentiles. The strength of the correlation between birth weight and perinatal mortality depends on gestational age [the lower the birth weight, the higher the rate of neonatal mortality for the estimated gestational age (Wilcox and Skjaerven, 1992)], and also on factors that are unrelated to gestational age. This low birth weight association with neonatal mortality is echoed in adult life with the development of later disease and mortality (Godfrey and Barker, 2001).
In postnatal life, there is growing evidence that the “natural variations” in body size and growth rate may have major relevance, not only on adult height, but also more importantly on infant and childhood survival and reproductive fitness. Pygmies are an “extreme” example of the interplay between postnatal growth and development, survival, and reproductive fitness. Their characteristic small adult size does not appear to have evolved through any positive selection for short stature, but rather as the result of a life history trade-off between the fertility benefits of large body size against the costs of late growth cessation in a setting of extremely high childhood and early adult mortality (Migliano et al., 2007).
In Western settings, rapid weight gain during early postnatal life is associated with increased risks for disease. For example, Ong et al. (2011) showed that children who showed catch-up growth between birth and 2 years were fatter, and have more central fat distribution at 5 years, when compared to children with normal early growth. Ekelund et al. (2007) examined the independent associations between weight gain during infancy (0–6 months) or early childhood (3–6 years) with components of the metabolic syndrome in young adults in a prospective cohort study in 128 individuals from birth to 17 years. They concluded that rapid weight gain during infancy (0–6 months), but not during early childhood (3–6 years), predicted the clustered metabolic risk at age 17 years. We have recently shown in a sample of 22 natural-fertility societies that the age at menarche correlated negatively with their average adult body mass, and the average adult body weight positively correlated with reproductive fitness (Hochberg et al., 2011b).
Infant feeding type and feeding patterns can also influence growth trajectories and disease risk. Compared to formula-feeding, breast feeding is associated with slower infant weight gain and lower later obesity risk. The results of several meta-analyses suggest that breast feeding has a protective effect, especially in SGA and preterm infants (Owen et al., 2005). Experimental evidence from several randomized control trials of nasogastric feeding of breast milk and various nutrient formulae for 4 weeks showed long-term differences on adiposity levels and the later propensity to cardiovascular disease (Singhal et al., 2002a,b). Precocious puberty that is associated with rapid weight gain and growth, particularly during infancy also has implications for future life events. Ong et al. (2007) have shown that an early age of menarche confers increased risk for disease, such as obesity, T2D, and hypertension, and death from cardiovascular disease and cancer in later life (Lakshman et al., 2009).
Finally, the mechanisms that signal and regulate early catch-up growth in the postnatal period may mediate or modify the associations between small size at birth and risks for disease in adulthood. The combination of low birth weight and a subsequent high body mass index (BMI) is related to the increased incidence of T2D in later life. Using longitudinal data that were collected from 8760 individuals who were born in Helsinki between 1934 and 1944, Eriksson et al. (2003) reported that the large differences in the incidence of T2D were associated with growth rates in utero, weight gain in infancy, and the age at adiposity rebound. These observations have implications for the early origins of both obesity and cardiovascular disease in that programmable windows of human obesity may exist during the periods of greatest weight velocity. However, current evidence has yet failed to agree on the specific programmable windows during postnatal growth and development for later disease risks (Singhal et al., 2002b; Eriksson et al., 2003; Owen et al., 2005).
Future Directions
The end-target of translational research is the patient with the goal to improve medical care. Traditionally, translational research – growth included – has followed medical reasoning, viewing organisms as machines whose design has been optimized by engineers to provide good health. This article takes the evolutionary reasoning: why those mechanisms are the way they are? Organisms are viewed here as packages of compromises (trade-offs) between traits shaped by natural selection to maximize reproduction.
Wide knowledge gaps still exist in our current understanding of the phenotypic plasticity and the putative epigenetic machinery, despite the increasing use of numerous experimental systems. As a result, we still do not know whether some of the epigenetic mechanisms that have been identified thus far using these experimental systems are operative in humans and other eutherians.
Hereditary, environmental, and stochastic factors determine the accumulation of epigenetic variation over time, but their relative contribution to the phenotypic outcome in terms of child growth and maturation is not known because few data are available.
That the environment can influence growth and developmental trajectories during pre-adult life history stages is well established, and later life outcomes have been much sought after. Yet, the mechanistic events that influence the transition from one life history stage to the next, growth and puberty are incompletely understood. Growth and puberty are regulated by insulin, growth hormone, the IGFs, and the sex hormones. These hormones drive the rate of growth and development, but it is unclear what determines the timing and degree of the different phases of developmental events and the quantity of growth. At the target tissues for these hormones, we need to first identify gene expression changes that occur in each tissue. Epigenetic events, including the cell type-specificity and tissue-specificity of chromatin regulation are great challenges for future human studies.
Since no other animal has a similar pre-adult life history to that of humans, an obvious question is whether the findings from any experimental animal can be extrapolated to humans. The mechanisms by which cues about nutrient availability in the uterus and postnatal environment are transmitted to the offspring and by which different stable phenotypes are induced are still unknown. The genetic control of the regulation of placental supply and fetal demand for maternal nutrients is not fully understood, and many of the detrimental events that occur in the fetus could be possibly due to epigenetic misprogramming.
Conflict of Interest Statement
The author declares that the research was conducted in the absence of any commercial or financial relationships that could be construed as a potential conflict of interest.
References
Arcaleni, E. (2006). Secular trend and regional differences in the stature of Italians. Econ. Hum. Biol. 4, 24–38.
Barker, D. J. (2006). Adult consequences of fetal growth restriction. Clin. Obstet. Gynecol. 49, 270–283.
Barker, D. J., and Osmond, C. (1986). Infant mortality, childhood nutrition, and ischaemic heart disease in England and Wales. Lancet 1, 1077–1081.
Barker, D. J., Osmond, C., and Law, C. M. (1989). The intrauterine and early postnatal origins of cardiovascular disease and chronic bronchitis. J. Epidemiol. Community Health 43, 237–240.
Bateson, P., Barker, D., Clutton-Brock, T., Deb, D., D’Udine, B., Foley, R. A., Gluckman, P., Godfrey, K., Kirkwood, T., Lahr, M. M., McNamara, J., Metcalfe, N. B., Monaghan, P., Spencer, H. G., and Sultan, S. E. (2004). Developmental plasticity and human health. Nature. 430, 419–421.
Belsky, J., and Fearon, R. M. (2002). Early attachment security, subsequent maternal sensitivity, and later child development: does continuity in development depend upon continuity of caregiving? Attach Hum Dev. 4, 361–387.
Bogin, B., Silva, M. I., and Rios, L. (2007). Life history trade-offs in human growth: adaptation or pathology? Am. J. Hum. Biol. 19, 631–642.
CDC. (2000). CDC growth charts. Available at: http://www.cdc.gov/growthcharts/
Crews, D., Gore, A. C., Hsu, T. S., Dangleben, N. L., Spinetta, M., Schallert, T., Anway, M. D., and Skinner, M. K. (2007). Transgenerational epigenetic imprints on mate preference. Proc. Natl. Acad. Sci. U.S.A. 104, 5942–5946.
Del Giudice, M. (2009). Sex, attachment, and the development of reproductive strategies. Behav. Brain. Sci. 32, 1–21; discussion 21–67.
Ekelund, U., Ong, K. K., Linne, Y., Neovius, M., Brage, S., Dunger, D. B., Wareham, N. J., and Rössner, S. (2007). Association of weight gain in infancy and early childhood with metabolic risk in young adults. J. Clin. Endocrinol. Metab. 92, 98–103.
Eriksson, J. G., Forsen, T., Tuomilehto, J., Osmond, C., and Barker, D. J. (2003). Early adiposity rebound in childhood and risk of type 2 diabetes in adult life. Diabetologia 46, 190–194.
Gawlik, A., Walker, R. S., and Hochberg, Z. (2011). Impact of infancy duration on adult size in 22 subsistence-based societies. Acta Paediatr. doi: 10.1111/j.1651-2227.2011.02395.x. [Epub ahead of print].
Gluckman, P. D., and Hanson, M. A. (2007a). Developmental plasticity and human disease: research directions. J. Intern. Med. 261, 461–471.
Gluckman, P. D., Hanson, M. A., Cooper, C., and Thornburg, K. L. (2008). Effect of in utero and early-life conditions on adult health and disease. N. Engl. J. Med. 359, 61–73.
Godfrey, K. M., and Barker, D. J. (2001). Fetal programming and adult health. Public Health Nutr. 4, 611–624.
Goodman, C. S., and Coughlin, B. C. (2000). Introduction. The evolution of evo-devo biology. Proc. Natl. Acad. Sci. U.S.A. 97, 4424–4425.
Hochberg, Z. (2008). Juvenility in the context of life history theory. Arch Dis. Child. 93, 534–539.
Hochberg, Z. (2009). Evo-devo of child growth II: human life history and transition between its phases. Eur. J. Endocrinol. 160, 135–141.
Hochberg, Z. (2010). Evo-devo of child growth III: premature juvenility as an evolutionary trade-off. Horm. Res. Paediatr. 73, 430–437.
Hochberg, Z., and Albertsson-Wikland, K. (2008). Evo-devo of infantile and childhood growth. Pediatr. Res. 64, 2–7.
Hochberg, Z., Feil, R., Constancia, M., Fraga, M., Junien, C., Carel, J. C., Boileau, P., Le Bouc, Y., Deal, C. L., Lillycrop, K., Scharfmann, R., Sheppard, A., Skinner, M., Szyf, M., Waterland, R. A., Waxman, D. J., Whitelaw, E., Ong, K., and Albertsson-Wikland, K. (2011a). Child health, developmental plasticity, and epigenetic programming. Endocr. Rev. 32 159–224.
Hochberg, Z., Gawlik, A., and Walker, R. S. (2011b). Evolutionary fitness as a function of pubertal age in 22 subsistence-based traditional societies. Int. J. Pediatr. Endocrinol. 2011, 1–7.
Kuzawa, C. W. (2007). Developmental origins of life history: growth, productivity, and reproduction. Am. J. Hum. Biol. 19, 654–661.
Lakshman, R., Forouhi, N. G., Sharp, S. J., Luben, R., Bingham, S. A., Khaw, K. T., Wareham, N. J., and Ong, K. K. (2009). Early age at menarche associated with cardiovascular disease and mortality. J. Clin. Endocrinol. Metab. 94, 4953–4960.
Liu, Y., Albertsson-Wikland, K., and Karlberg, J. (2000). Long-term consequences of early linear growth retardation (stunting) in Swedish children. Pediatr. Res. 47(Pt 1), 475–480.
Liu, Y. X., Jalil, F., and Karlberg, J. (1998). Growth stunting in early life in relation to the onset of the childhood component of growth. J. Pediatr. Endocrinol. Metab. 11, 247–260.
McCance, R. A., and Widdowson, E. M. (1974). The determinants of growth and form. Proc. R. Soc. Lond. B Biol. Sci. 185, 1–17.
Migliano, A. B., Vinicius, L., and Lahr, M. M. (2007). Life history trade-offs explain the evolution of human pygmies. Proc. Natl. Acad. Sci. U.S.A. 104, 20216–20219.
Ong, K. K., Elks, C. E., Wills, A. K., Wong, A., Wareham, N. J., Loos, R. J., Kuh, D., and Hardy, R. (2011). Associations between the pubertal timing-related variant in LIN28B and BMI vary across the life course. J. Clin. Endocrinol. Metab. 96, E125–E129.
Ong, K. K., Northstone, K., Wells, J. C., Rubin, C., Ness, A. R., Golding, J., and Dunger, D. B. (2007). Earlier mother’s age at menarche predicts rapid infancy growth and childhood obesity. PLoS Med. 4, e132. doi: 10.1371/journal.pmed.0040132
Owen, C. G., Martin, R. M., Whincup, P. H., Smith, G. D., and Cook, D. G. (2005). Effect of infant feeding on the risk of obesity across the life course: a quantitative review of published evidence. Pediatrics 115, 1367–1377.
Parent, A. S., Teilmann, G., Juul, A., Skakkebaek, N. E., Toppari, J., and Bourguignon, J. P. (2003). The timing of normal puberty and the age limits of sexual precocity: variations around the world, secular trends, and changes after migration. Endocr. Rev. 24, 668–693.
Pembrey, M. E., Bygren, L. O., Kaati, G., Edvinsson, S., Northstone, K., Sjostrom, M., Golding, J., and ALSPAC Study Team. (2006). Sex-specific, male-line transgenerational responses in humans. Eur. J. Hum. Genet. 14, 159–166.
Pratt, J. H., Manatunga, A. K., and Li, W. (1994). Familial influences on the adrenal androgen excretion rate during the adrenarche. Metabolism 43, 186–189.
Singhal, A., Farooqi, I. S., Cole, T. J., O’Rahilly, S., Fewtrell, M., Kattenhorn, M., Lucas, A., and Deanfield, J. (2002a). Influence of leptin on arterial distensibility: a novel link between obesity and cardiovascular disease? Circulation 106, 1919–1924.
Singhal, A., Farooqi, I. S., O’Rahilly, S., Cole, T. J., Fewtrell, M., and Lucas, A. (2002b). Early nutrition and leptin concentrations in later life. Am. J. Clin. Nutr. 75, 993–999.
Stein, A. D., Barnhart, H. X., Wang, M., Hoshen, M. B., Ologoudou, K., Ramakrishnan, U., Grajeda, R., Ramirez-Zea, M., and Martorell, R. (2004). Comparison of linear growth patterns in the first three years of life across two generations in Guatemala. Pediatrics 113(Pt 1), e270–e275.
Keywords: child growth, evolution, epigenetics, plasticity
Citation: Hochberg Z (2011) Developmental plasticity in child growth and maturation. Front. Endocrin. 2:41. doi: 10.3389/fendo.2011.00041
Received: 04 August 2011;
Paper pending published: 22 August 2011;
Accepted: 12 September 2011;
Published online: 29 September 2011.
Edited by:
Stefano Cianfarani, University of Rome, ItalyReviewed by:
Taneli Raivio, University of Helsinki, FinlandFrancesco Chiarelli, University of Chieti, Italy
Copyright: © 2011 Hochberg. This is an open-access article subject to a non-exclusive license between the authors and Frontiers Media SA, which permits use, distribution and reproduction in other forums, provided the original authors and source are credited and other Frontiers conditions are complied with.
*Correspondence: Ze’ev Hochberg, Rappaport Family Faculty of Medicine and Research Institute, Technion Israel Institute of Technology, Rambam Medical Center, POB 9602, Haifa 31096, Israel. e-mail:el9ob2NoYmVyZ0ByYW1iYW0uaGVhbHRoLmdvdi5pbA==