- 1Laboratory on Thymus Research, Oswaldo Cruz Institute, Oswaldo Cruz Foundation, Rio de Janeiro, Brazil
- 2Laboratory of Inflammation, Oswaldo Cruz Institute, Oswaldo Cruz Foundation, Rio de Janeiro, Brazil
- 3National Institute of Science and Technology on Neuroimmunomodulation (INCT-NIM), Rio de Janeiro, Brazil
Maintenance of thymus homeostasis is a delicate interplay involving hormones, neurotransmitters and local microenvironmental proteins, as well as saccharides, acting on both thymocytes and stromal cells. Disturbances in these interactions may lead to alterations on thymocyte development. We previously showed that galectin-3, a β-galactoside-binding protein, is constitutively expressed in the thymus, interacting with extracellular matrix glycoproteins and acting as a de-adhesion molecule, thus modulating thymocyte-stromal cell interactions. In this work, we aimed to investigate the participation of galectin-3 in the maintenance of thymus homeostasis, including hormonal-mediated circuits. For that, we used genetically engineered galectin-3-deficient mice. We observed that the thymus of galectin-3-deficient mice was reduced in mass and cellularity compared to wild-type controls; however, the proportions of different thymocyte subpopulations defined by CD4/CD8 expression were not changed. Considering the CD4−CD8− double-negative (DN) subpopulation, an accumulation of the most immature (DN1) stage was observed. Additionally, the proliferative capacity of thymocytes was decreased in all thymocyte subsets, whereas the percentage of apoptosis was increased, especially in the CD4+CD8+ double-positive thymocytes. As glucocorticoid hormones are known to be involved in thymus homeostasis, we evaluated serum and intrathymic corticosterone levels by radioimmunoassay, and the expression of steroidogenic machinery using real-time PCR. We detected a significant increase in corticosterone levels in both serum and thymus samples of galectin-3-deficient mice, as compared to age-matched controls. This was paralleled by an increase of gene transcription of the steroidogenic enzymes, steroidogenic acute regulatory protein (Star) and Cyp11b1 in thymus, 11β-Hydroxysteroid Dehydrogenase (Hsd11b1) in the adrenal, and Cyp11a1 in both glands. In conclusion, our findings show that the absence of galectin-3 subverts mouse thymus homeostasis by a mechanism likely associated to intrathymic and systemic stress-related endocrine circuitries, affecting thymocyte number, proliferation and apoptosis.
Introduction
Galectins are a family of 15 β-galactoside-binding lectins, containing at least one conserved carbohydrate-recognition domain, which can be found in the nucleus, cytosol, and bound to cell membrane glycoconjugates or to extracellular matrix glycocomponents (1). Depending to their location into the cell, galectins can influence cell proliferation, adhesion, migration, signaling, differentiation, and apoptosis (1, 2). They were also shown to modulate immune functions in health and disease (3, 4). We showed that galectin-3 is constitutively expressed by epithelial and phagocytic cells in both thymic cortex and medulla. Galectin-3 interacts with glycoconjugates on thymocyte surface and extracellular matrix glycoproteins acting as a de-adhesion molecule, thus modulating thymocyte-stromal cell interactions (5). Furthermore, we also noted that galectin-3 accumulates in the thymus of Trypanosoma cruzi infected mice, being related to increased thymocyte death and exit to the periphery, and consequent thymus atrophy (6).
Thymus involution is a physiological phenomenon of the organ, related to aging, leading to progressive alterations in the thymus microenvironment, with loss of thymus mass, thymic epithelial cell (TEC) number and function, resulting in a decrease in thymopoiesis. In consequence, a decrease in the immune function is observed in the elderly, with less resistance to infections, autoimmune diseases and cancer (7, 8). On the other hand, acute thymic involution is related to pathological conditions, such as metabolic and infectious diseases (9, 10).
In addition, activation of the hypothalamus–pituitary–adrenal axis induced by stress or some diseases, including diabetes and Chagas disease, was shown to cause severe atrophy of the thymus (11, 12). Glucocorticoids decrease proliferation and increase apoptosis of immature CD4+CD8+ double-positive (DP) thymocytes, inducing a strong atrophy in the thymus (13, 14). These actions of glucocorticoids on thymus are related to endocrine and paracrine actions of this hormone, since thymus presents the steroidogenic machinery, including StAR, 11β-HSD1, and 11β-HSD2, and is capable to produce glucocorticoids (15, 16).
Considering the multifunctional role of galectin-3 as a regulator of cell adhesion, migration, proliferation, signaling, differentiation and apoptosis, our hypothesis is that galectin-3 is a key player to maintain thymus homeostasis. Here, we undertook this study to evaluate the role of galectin-3 on thymus homeostasis in association with its effects on local and systemic production of glucocorticoids.
Materials and Methods
Mice
Male BALB/c wild type (WT) and galectin-3 deficient mice (4–6 week old) were obtained from the Oswaldo Cruz Foundation animal facilities, Rio de Janeiro, Brazil. Galectin-3 deficient mice (Gal-3−/−) were generated by backcrossing with their BALB/c littermates for 9 generations (17). Mice were housed in groups of three in a temperature-, humidity-, and light-controlled (12 h light: 12 h darkness cycle) colony room. Mice were given ad libitum access to food and water. All protocols for the use and care of animals were approved by the Ethics Committee for the Use of Experimental Animals of the Oswaldo Cruz Foundation, under licenses number L-024/09 and L-004/2014.
Analysis of T Cell Subpopulations
Individual thymuses were minced, resuspended in phosphate buffered saline solution (PBS) (Sigma Aldrich, St Louis, MO, USA) with 5% Fetal Calf Serum (FCS) (Cultilab, Campinas, SP, Brazil) and counted in Neubauer chamber in the presence of Trypan Blue (Sigma Aldrich) for evaluation of cell viability. Trypan Blue evaluation of cell viability in fresh thymocytes showed that about 95 and 85% of cells were alive in the thymus of WT and Gal-3−/− mice, respectively. The phenotype of the main thymocyte subpopulations was evaluated by Flow Cytometry with the use of monoclonal antibodies to mouse CD4, CD8, CD44, and CD25 conjugated to different fluorochromes (BD, San Diego, CA, USA). Control isotype immunoglobulins conjugated to correspondent fluorochromes were used for negative staining determination (BD). Briefly, 106 thymocytes were incubated for 15 min with 2 μL of normal mouse serum for blockage of unspecific binding, and subsequently with 10 μL of different antibody combinations for 30 min. Cells were then washed in PBS, fixed with 1% formaldehyde and analyzed by Flow Cytometry in a FACSCanto II equipped with the FACSDiva Software (BD). Data were analyzed with the Summit 4.3 Software (Dako Cytomation,Carpinteria, CA, USA).
Evaluation of Thymocyte Proliferation
For evaluation of spontaneous thymocyte proliferation, thymocytes were incubated for 3 h in RPMI medium (Sigma Aldrich) with 10% FCS containing 60 μM bromodeoxyuridine (BrdU) (Sigma Aldrich). Cells were then incubated with anti-CD4, anti-CD8, anti-CD44, anti-CD25 monoclonal antibodies (BD) conjugated to different fluorochromes. After washings, cells were permeabilized using the kit BD Cytofix/Cytoperm™ (BD). Subsequently, BrdU incorporated to cell DNA was exposed by treatment of cells with 100U DNAse I (Roche, Mannheim, BW, Germany) for 40 min at room temperature. Cells were then washed twice for 5 min at 450 × g, and subsequently incubated with FITC conjugated anti-BrdU (eBioscience, Inc., San Diego, CA, USA). Samples were acquired in a FACSCanto II device (BD) and analyzed with Summit 4.3 Software (Dako Cytomation).
Measurement of Thymocyte Apoptosis
For evaluation of cell death, thymocytes were first stained for surface molecules CD4 and CD8 conjugated to different fluorochromes (BD), washed, suspended in Annexin V buffer and treated for 10 min with 1 μL Annexin V conjugated to fluorescein isothiocyanate (FITC) (BD) according to the manufacturer's recommendations. Cells were immediately analyzed by Flow Cytometry using a FACSCanto II device (BD) equipped with the FACSDiva Software (BD).
Immunofluorescence
The evaluation of thymic epithelial compartment was performed by immunofluorescence. Thymuses (5 animals/group) were removed and frozen in Tissue Tek (Optimal Cutting Temperature Compound, Sakura Finetek, Torrance, CA, USA). Slices of 5 μm-thick thymic sections were obtained in cryostat (Leica CM 1850 - Leica Microsystems Inc.; Buffalo Grove, IL, USA) and fixed in cold acetone for 5 min. Tissue sections were then incubated with 2.5% BSA in PBS for 1 h and subsequently subjected to the indirect immunofluorescence technique for immunolabeling with pan-cytokeratin antibody (Dako), or unrelated control IgG (Molecular Probes, Carlsbad, CA, USA) for 1 h at room temperature. Sections were then washed three times in PBS and incubated for 45 min with the secondary anti-rabbit antibody conjugated to Alexa Fluor 546 (Molecular Probes). Sections were washed again three times in PBS and mounted with Fluoroshield containing 4′,6-diamidino-2-phenylindole - DAPI (Sigma Aldrich) for nuclear staining. Samples were analyzed using a Carl Zeiss Axio Imager Upright Microscope (Zeiss, Oberkochen, BW, Germany).
Histology
Thymus histological analysis was performed by Hematoxylin & Eosin technique. Thymuses (3 animals/group) were fixed in buffered 10% formalin (Millonig buffer) for 48 h. Paraffin-embedded 5-μm sections were mounted on glass slides. The sections were deparaffinized with xylene, and rehydrated by a graded series of ethanol washes. Sections were then left in running water for 1 min, stained with Hematoxylin for 10 min, washed in running water for 1 min, and incubated in Eosin solution for 3 min (Sigma, Aldrich). Photos were taken using the Leica DM 2500 microscope.
Evaluation of Corticosterone Levels
Serum and thymus samples were obtained simultaneously from WT and Gal-3−/− mice.
Animals were euthanized in a CO2 chamber, during the nadir (08:00 h) of the circadian rhythm as described previously (18), and the blood was immediately collected from the abdominal aorta. After blood coagulation, individual sera was collected and stored at −20°C until use. Thymus samples were obtained and kept at a −20°C until use. After thawing, the thymuses were suspended in 150 μl PBS and then triturated in tissue homogenizer. The homogenates were centrifuged at 10,000 × g for 15 min at 4°C. Serum and thymus corticosterone levels were detected by radioimmunoassay (RIA) following manufacturer's guidelines (MP Biomedicals, Solon, OH, USA). Final intrathymic corticosterone levels were represented by the ratio of hormone concentration in supernatants and thymus mass.
Gene Expression of Steroidogenic Enzymes in Adrenals and Thymuses
Thymus and adrenal total RNA from WT and Gal-3−/− animals were obtained using the RNeasy Micro Kit (Qiagen, Valencia, CA, USA). The quantification was performed in the spectrophotometer NanoDrop 1000 (Thermo Ficher Scientific, Waltham, MA, USA). For the synthesis of cDNA, equivalent samples were used in 1 μg of RNA, using SuperScript III First-Strand Synthesis System (Invitrogen, Carlsbad, CA, USA), in the presence of a random primer, according to the manufacturer's recommendation. For the analysis of gene expression by real-time PCR, 100 ng of cDNA samples were diluted in Power SYBR Green PCR Master Mix (Applied Biosystems, Carlsbad, CA, USA) in the Step One Plus system (Applied Biosystems). The PCR method was performed at 95°C for 10 min followed by 40 cycles at 95°C for 15 s, 60°C for 1 min. The specificity of reaction products was verified through the dissociation curve. The data were analyzed by ABI Prism SDS v1.3.1 software. All primers were designed using the Primer Express 3.0 specific program for 7500 FAST Real Time PCR System. cDNA was amplified using specific murine primer sequences described in Table 1. After 40 cycles of amplification, expression of cytochrome P450, family 11, subfamily a, polypeptide 1 (Cyp11a1), Cyp11b1, steroidogenic acute regulatory protein (Star), and hydroxysteroid 11-beta dehydrogenase 1 (Hsd11b1) was assessed by comparing the expression of each to the normalizer constitutive reference transcript Rpl-13a (ribosomal protein L13A), using the Ct method as previously described (2−dCt × 1,000) (19), subsequent to the following primer efficiency analysis. Each experiment was run in triplicate with different cDNA preparations from the same mice.
Galectin-3 Inhibition Experiments
In order to investigate the possibility that the lack of galectin-3 is related to thymocyte death observed in our Gal-3−/− mice, we performed in vitro experiments using GCS-100, a modified citrus pectin described to act as galectin-3 inhibitor (kindly donated by Dr. S. Patel, La Jolla Pharmaceutical Company, San Diego, CA). Briefly, 106 thymocytes of 3-5 WT mice were cultured in RPMI 1640 medium supplemented with 10% fetal calf serum, 2 mM L-glutamine, 1 mM sodium pyruvate, 55 μM 2-mercaptoethanol (Gibco, Grand Island, NY), 100 U/mL penicillin, 0.1 mg/mL streptomycin and 0.25 μg/mL amphotericin B (Sigma Aldrich) in the presence of GCS-100, in the concentrations of 50–800 μg/mL, for 24 h at 37°C in a humidified atmosphere containing 5% CO2. For comparison we included in the experiment thymocytes treated with dexamethasone (0.01 μM). Cells were subsequently incubated with antibodies to CD3, CD4 and CD8 conjugated to different fluorochromes (BD), washed, suspended in Annexin V buffer and treated for 10 min with Annexin-V and 7-AAD for viability evaluation. Cells were immediately analyzed by Flow Cytometry using a FACSCanto II device (BD) equipped with the FACSDiva Software (BD).
Statistical Analysis
Data were evaluated to ensure normal distribution and were statistically analyzed by unpaired t-test or ANOVA using the Tukey's multiple comparison test. Data are shown as individual values and median or mean ± standard error (used for real time PCR analysis). Tests were performed using GraphPad Prism 5.0 software (Graphpad Software, San Diego, CA, USA).
Results
Lack of Galectin-3 Induces Thymus Atrophy With Microenvironmental Alterations
We initially observed that galectin-3 deficient mice (Gal-3−/−) have a significant thymus atrophy with lower mass and cellularity compared to wild type (WT) BALB/c mice (Figures 1A–C). It is important to note that no significant differences were observed considering body mass of both strains of mice. Once the maintenance of adequate thymic architecture is fundamental to thymocyte differentiation, thymus atrophy is frequently accompanied by morphological tissue alterations. So, we evaluated thymus microscopic structure in Gal-3−/− mice using Hematoxylin & Eosin staining. We observed that thymus cortex and medulla are preserved in both WT and Gal-3−/− mice (Figures 1D,E). However we noticed the presence of concentric structures formed by TEC, similar to Hassall bodies, in the thymic medullary region of Gal-3−/− mice (Figure 1G), that are not seen in WT thymuses (Figure 1F). We also evaluated the status of the epithelial component of thymic microenvironment by staining thymus sections with anti-pan-cytokeratin antibody. Immunofluorescence data showed a deep disorganization of the thymic epithelial network, with visible TEC-free regions in the thymus of Gal-3−/− mice (Figures 1I,K) that were not observed in WT animals (Figures 1H,J).
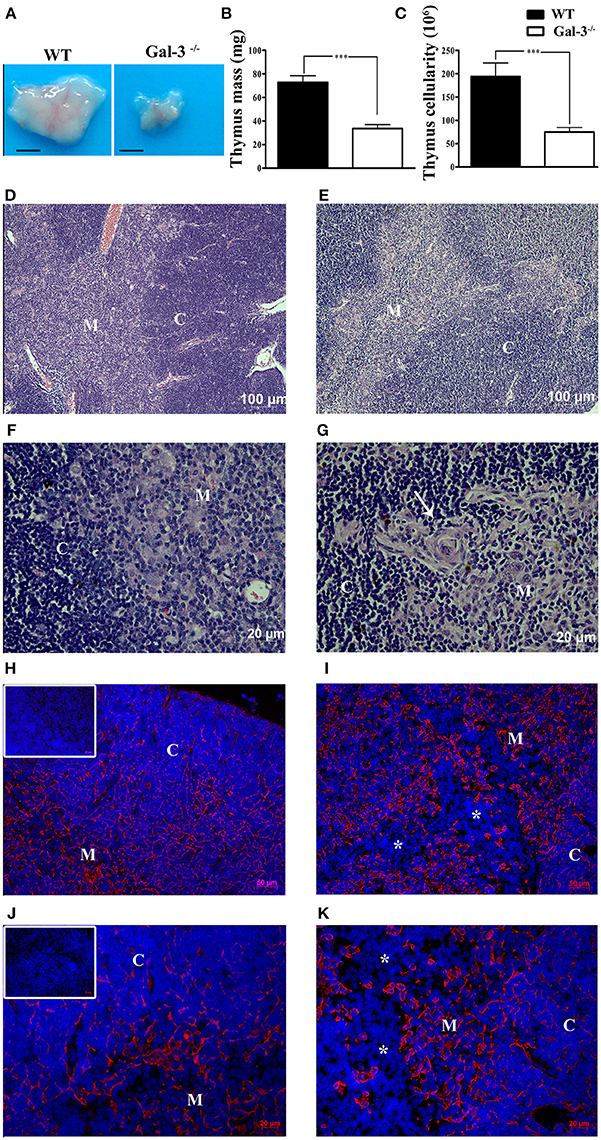
Figure 1. Thymus atrophy and microenvironmental alterations in the absence of galectin-3. (A) Shows comparative thymus pictures of WT and Gal-3−/− mice. Thymus mass (B) and cellularity (C) of Gal-3−/− mice are shown in comparison to WT control mice. Hematoxylin & Eosin stained sections of thymus of WT (D,F) and Gal-3−/− (E,G) mice. Presence of concentric Hassall body-like structures is shown in Gal-3−/− thymus (white arrow in G) but not in WT mice (F). Immunofluorescence staining with anti-pan-cytokeratin is shown in the thymus of WT (H,J) and Gal-3−/− (I,K) mice. Inserts in (H,J) show negative controls. Asterisks in (I,K) denote DAPI stained TEC-free regions. Blue staining in panels: DAPI, used to show cell nuclei. Images are representative of 5 animals/group. Bar in (A): 0.25 cm. C, Cortex; M, Medulla.
Lack of Galectin-3 Modulates Thymocyte Differentiation
In order to verify if galectin-3 interferes with thymocyte differentiation, we analyzed thymocyte phenotype by flow cytometry using the membrane markers CD4, CD8, CD25 and CD44. We did not find changes in the percentage of thymocyte subpopulations defined by CD4 and CD8 when we compared the two strains of mice (Figures 2A,B). However, considering absolute cell numbers, Gal-3−/− mice showed a significant decrease in all thymocyte subpopulations (Figure 2C).
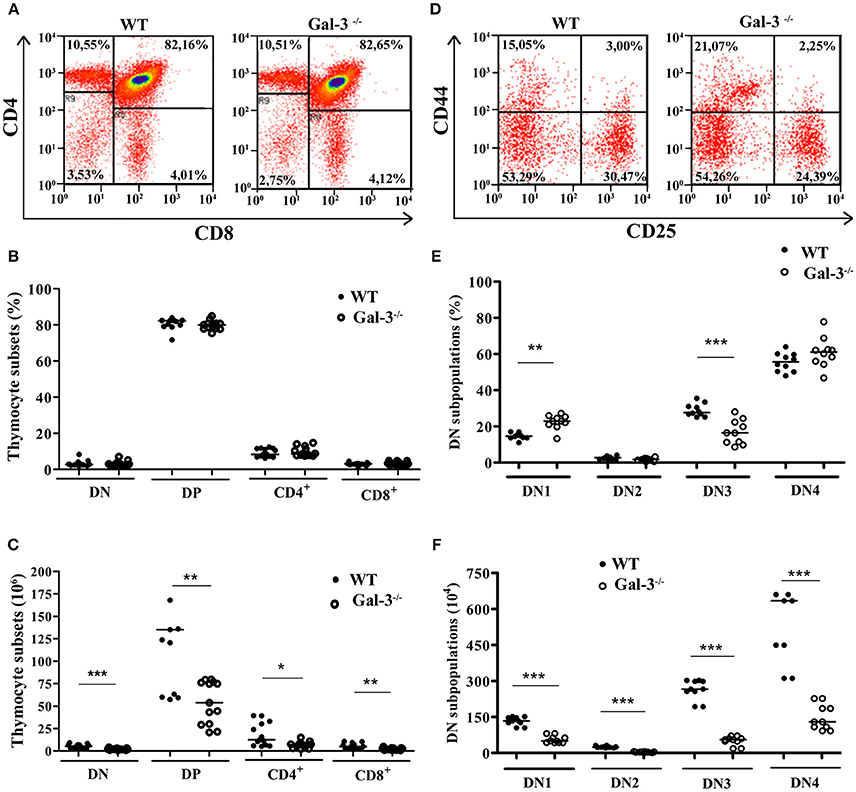
Figure 2. Modulation of thymocyte differentiation in the absence of galectin-3. (A) Shows representative dot plots obtained after CD4/CD8 staining of WT and Gal-3−/− thymocytes. (B,C) Respectively show percentage and absolute numbers of thymocyte subpopulations defined by CD4/CD8 staining of WT and Gal-3−/− mice. Data are representative of 14 animals/group. (D) Shows representative dot plots for CD44/CD25 staining of WT and Gal-3−/− thymocytes. (E,F) Respectively show percentage and absolute numbers of DN thymocyte subpopulations defined by CD44/CD25 staining of WT and Gal-3−/− mice. Data are representative of 10 animals/group. *p < 0.05; **p < 0.01; ***p < 0.001.
The most immature thymocytes are DN for CD4 and CD8, and these cells can be further subdivided in four subsets considering the expression of CD25 and CD44 on their cell membrane. The homeostasis of DN thymocytes is crucial for thymocyte development, as during this stage extensive proliferation, TCR rearrangement and commitment to the αβ or γδ T cell lineages take place. DN1 cells, the most immature DN subpopulation, express CD44 on their cell membrane, but not CD25. Thymocytes sequentially express both CD44 and CD25 (DN2 cells), then lose the expression of CD44 and express only CD25 (DN3 cells), and ultimately are negative for both CD44 and CD25 (DN4 cells; also known as pre-DP) (20). Analysis of DN thymocyte subpopulations in the Gal-3−/− mice showed a significant increase in the percentage of DN1 thymocytes and a decrease in cells in the DN3 stage compared to control WT animals, without alterations in the percentage of DN2 and DN4 cells (Figures 2D,E). In absolute numbers, Gal-3−/− mice showed a decrease in all DN subpopulations (Figure 2F).
Lack of Galectin 3 Interferes With Thymocyte Proliferation and Death
Proliferation and apoptosis are important events for thymocyte development and maintenance of thymus cellularity. We evaluated if the decrease in thymus mass and cellularity observed in Gal-3−/− mice was related to changes in the rates of both phenomena. Spontaneous thymocyte proliferation, evaluated after 3-h incubation with the thymidine analog BrdU, was decreased both in percentages and absolute numbers in total Gal-3−/− thymocytes when compared to WT (Figures 3A,B). Percentages of spontaneous proliferation were decreased in DN, DP and CD4+ subpopulations (Figure 3C). However, considering absolute numbers DN, DP and CD8+, but not CD4+ cells showed decreased proliferation rate (Figure 3D). We finally evaluated the levels of spontaneous proliferation in DN subsets of thymocytes and observed a decrease in all DN subpopulations, from DN1 to DN4, both in percentage and absolute numbers (Figures 3E,F).
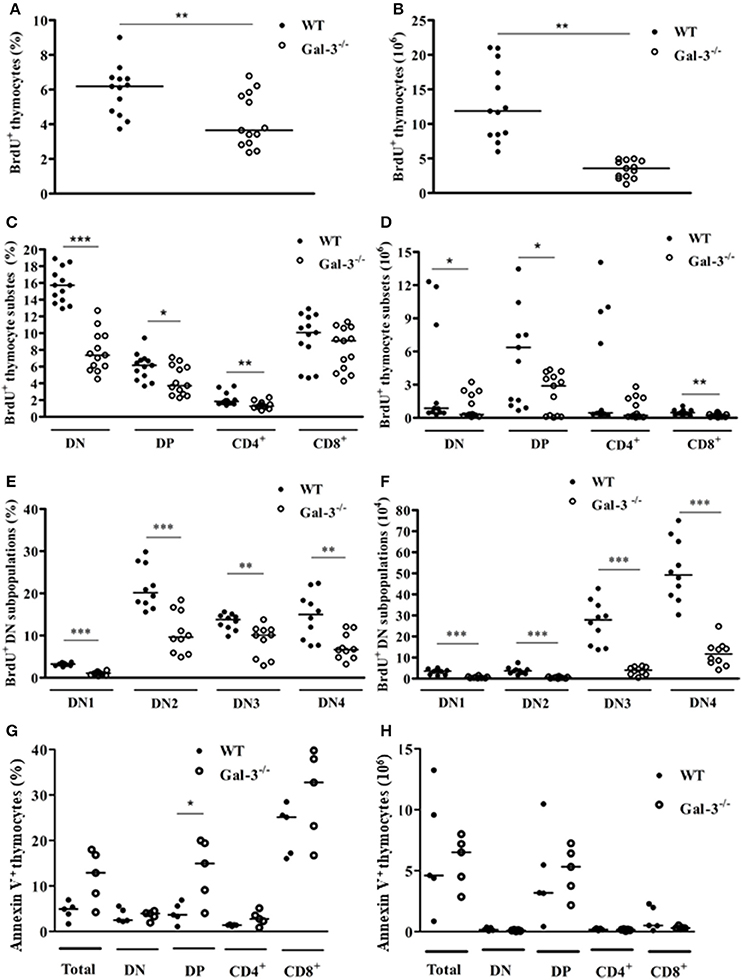
Figure 3. Changes in thymocyte proliferation and death in the absence of galectin-3. BrdU incorporation by total thymocytes of WT and Gal-3−/− mice is shown in percentage (A) and absolute cell numbers (B). (C,D) Respectively show BrdU incorporation by CD4/CD8-defined thymocyte subpopulations in percentage and absolute numbers. (E,F) Respectively show BrdU incorporation by DN thymocyte subpopulations in percentage and absolute numbers. Data are representative of 10 animals/group. (G,H) Respectively show percentage and absolute numbers of Annexin V+ cells in total and CD4/CD8-defined thymocyte subpopulations. Data are representative of 5 animals/group. *p < 0.05; **p < 0.01; ***p < 0.001.
The results of cell death analysis, evaluated after labeling cells with Annexin V, showed statistically significant increase in the percentage of Annexin V+ DP thymocytes of Gal-3−/− mice, compared to WT animals (Figure 3G). No differences were observed in absolute numbers (Figure 3H).
Lack of Galectin-3 Increases Serum and Intrathymic Corticosterone Levels
Considering the participation of glucocorticoids on thymus involution, we initially evaluated the levels of corticosterone in the serum and thymus of both strains of mice. We detected high corticosterone levels in both serum and thymus of Gal-3−/− mice compared to WT animals (Figure 4). In adrenal glands, Gal-3−/− mice presented an increase in the expression of steroidogenic enzyme genes Cyp11a1 (Figure 5B) and Hsd11b1 (Figure 5D), but did not alter the gene expression of Star (Figure 5A) and Cyp11b1 (Figure 5C), while in the thymus of Gal-3−/− mice we noticed higher expression of Star (Figure 5E), Cyp11a1 (Figure 5F), and Cyp11b1 (Figure 5G), and no difference in Hsd11b1 (Figure 5H) gene expression.
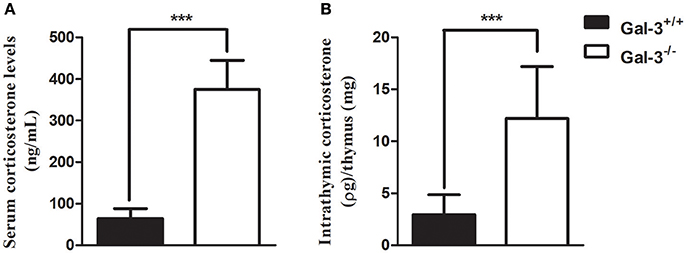
Figure 4. Increase in serum and intrathymic corticosterone levels in the absence of galectin-3. Radioimmune assay analysis of serum (A) and intrathymic (B) corticosterone levels of WT and Gal-3−/− mice. Final intrathymic corticosterone levels were represented by the ratio of hormone concentration in supernatants and thymus mass. Data are representative of 10 animals/group. ***p < 0.001.
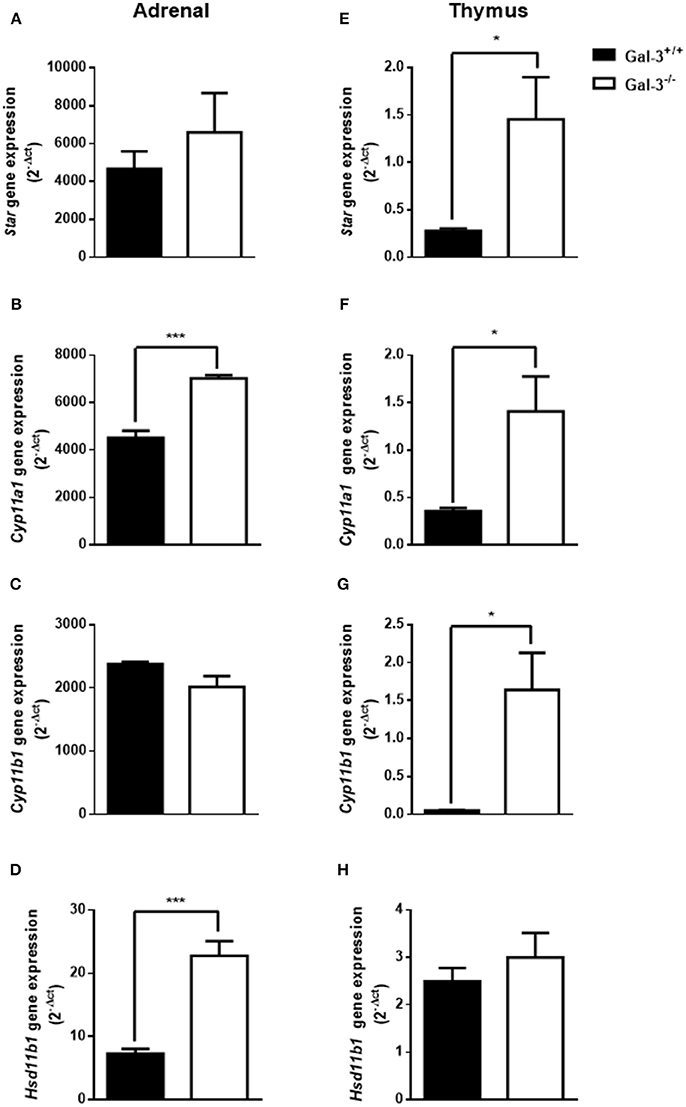
Figure 5. Changes of steroidogenic machinery in the absence of galectin-3. Gene expression of steroidogenic enzymes Star (A,E), Cyp11a1 (B,F), Cyp11b1(C,G), and Hsd11b1 (D,H) in adrenals and thymuses of WT and Gal-3−/− mice was measured by qPCR. The values were normalized to the constitutive reference transcript Rpl-13a. Values are represented as (2-dct) of gene expression are shown as mean ± standard error. Each experiment was run in triplicate with different cDNA preparations from the same mice. Data are representative of 4 animals/group. *p < 0.05; ***p < 0.001.
Inhibition of Galectin-3 in WT Mice Increases Thymocyte Apoptosis
To determine in which extent the increased thymocyte apoptosis observed in Gal-3−/− mice was due to the primary lack of galectin-3 or to the high corticosterone levels observed in these animals, we treated thymocytes obtained from WT mice with different concentrations of GCS-100, a modified citrus pectin described to function as a galectin-3 inhibitor (21, 22), or dexamethasone in vitro for 24 h. Our results showed that thymocytes treated with different concentrations of GCS-100 for 24 h were more susceptible to apoptosis than untreated cells, as shown by the staining with 7-AAD/Annexin V (Figure 6). Moreover, DP thymocytes were the most susceptible cells comparing different thymocyte subpopulations. As expected, dexamethasone-treated thymocytes were induced to apoptosis, mainly DP cells (Figure 6E).
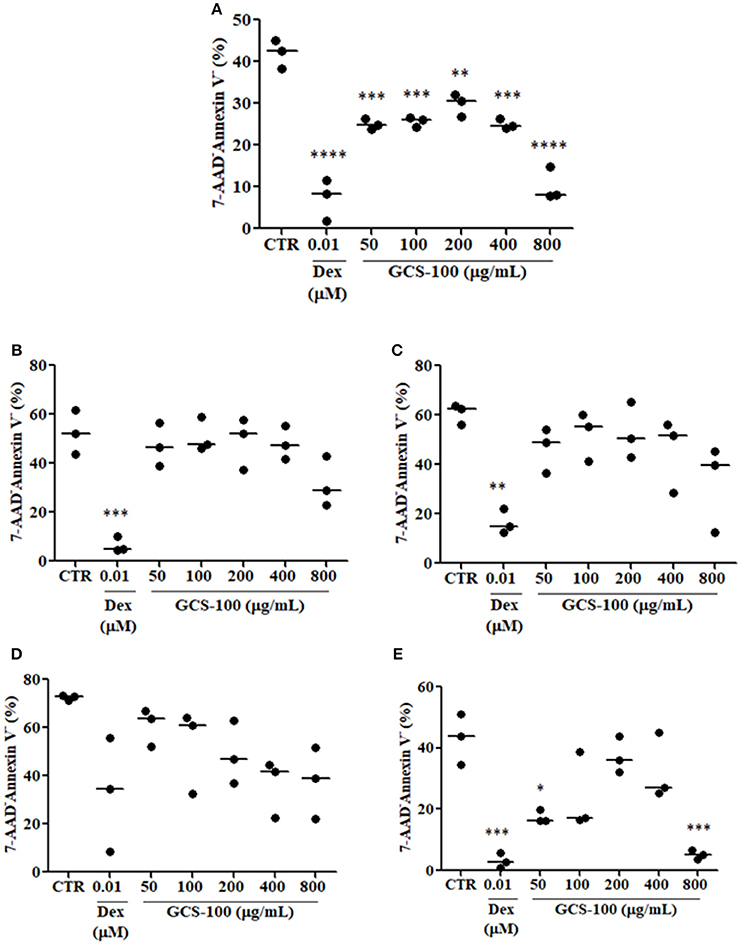
Figure 6. Inhibition of galectin-3 increases thymocyte apoptosis. (A) Shows % of living cells (7-AAD−/Annexin V−) in total thymocytes treated for 24 h with different concentrations of GCS-100 (50–800 μg/mL) or dexamethasone (0.01 μM). (B–E) Respectively show % of living cells (7-AAD−/Annexin V−) in CD4SP, CD8SP, DN/CD3− and DP thymocyte subpopulations submitted to the same treatments. Data show one representative of two independent experiments with 3 animals/group, with similar results. *p < 0.05; **p < 0.01; ***p < 0.001; ****p < 0.0001.
Discussion
Thymocyte differentiation from bone marrow-derived precursors is dependent on their interactions with the thymic microenvironment, composed of stromal cells, namely TEC, fibroblasts, macrophages, dendritic cells; extracellular matrix (ECM) molecules, represented by fibronectin, laminin, type IV collagen; and soluble proteins, such as cytokines, chemokines, galectins, and with the neuro and endocrine systems (12, 23). In the present study we showed that the lack of galectin-3 consistently affected thymus homeostasis in association to local and systemic glucocorticoid production. Initially, we noticed that thymus mass and cellularity were significantly decreased in Gal-3−/− mice compared to WT, presenting also alterations in the epithelial component of the thymic microenvironment, with regions without TEC in the thymus. These alterations in the structure of thymus epithelial network observed in Gal-3−/− mice could, at least partly, explain the reduction of cellularity in this organ, once we previously demonstrated that galectin-3 is expressed by epithelial cells of both thymus cortex and medulla, playing a de-adhesive role by modulating thymocyte interactions with stromal cells and ECM components (5). Furthermore, the present data suggest that galectin-3 is not only important for the interactions of thymocytes with the thymic stroma, but also to the maintenance of thymic architecture and thymocyte homeostasis.
Next, we showed that the absolute numbers of different thymocyte subsets, defined by CD4 and CD8 molecules, were affected in Gal-3−/− mice. The lack of galectin-3 seems to affect all thymocyte subpopulations, impacting the organ as a whole. Interestingly, considering DN thymocytes, the most immature subset in which important events of thymocyte differentiation occur, we observed an accumulation of DN1 and a decrease in DN3 cells, whereas in absolute numbers all DN subpopulations were decreased. In fact, we detected that thymocytes of Gal-3−/− mice proliferate significantly less than those of WT, and this reduction was importantly noted in DN thymocytes. These alterations may affect all thymocyte development, leading to decreased thymus mass and cellularity, as in the DN stage extensive proliferation, TCR rearrangement and commitment to the αβ or γδ T cell lineages were described to happen (20). Our data, pointing the DN subset as strongly affected in the absence of galectin-3, might be related to critical interactions of the lectin with its ligands within specific niches in the subcapsular or cortical zones of the thymus. Further mechanistic studies are warranted to elucidate such a possibility.
It is also important to mention that in Gal-3−/− mice, thymocyte death was increased in DP subset, the most numerous of the thymocyte subpopulations. Different data in the literature showed that galectin-3, a multifunctional molecule included in the class of matricellular proteins (1, 24, 25), acts both extracellularly, where it participates in cell adhesion and migration, and intracellularly, being able to regulate proliferation, apoptosis and cell signaling (3). In fact, galectin-3 was shown to protect cells from apoptosis, as it has the NWGR motif highly conserved in the BH1 domain of the Bcl-2 gene family, a well characterized suppressor of apoptosis, and was shown to interact with Bcl-2 (26, 27). Moreover, the expression of galectin-3 was shown to be upregulated in proliferating fibroblasts, suggesting a possible role for this lectin in the regulation of cell growth (28). Considering the important thymus atrophy noted in Gal-3−/− mice, and that immature thymocytes are extremely sensitive to glucocorticoids we evaluated the levels of serum and thymus GC in these mice. Our results showed that Gal-3−/− mice present extremely higher levels of serum and thymus corticosterone compared to WT. Previous works described the endocrine and paracrine actions of GC on thymocyte physiology, and that thymus presents the steroidogenic machinery, including StAR, 11β-HSD1 and 11β-HSD2 and is able to produce GC itself (15, 16), and express GC receptors (29, 30). Indeed, GC were shown to decrease proliferation and increase apoptosis of immature thymocytes (13, 14). Furthermore, blockage of GC receptors was shown to partially revert thymus atrophy observed in Trypanosoma cruzi infected mice (31). We showed here that the production of GC seems to be modulated by the lack of galectin-3, with concomitant increase in steroidogenic machinery both in the adrenals and thymus. We believe that high GC content in Gal-3−/− mice may be involved in thymus atrophy, namely increased immature thymocyte death and decreased proliferation. We must also keep in mind that the lack of galectin-3, an anti-apoptotic molecule, may also be involved in the increased thymocyte death observed in Gal-3−/− mice. To elucidate how much of the changes on thymocyte cellularity are due to hormonal changes and how much are due to the lack of galectin-3, we treated thymocytes from WT mice in vitro with a galectin-3 inhibitor (GCS-100) or with dexamethasone, and evaluated cell susceptibility to apoptosis. GCS-100 was previously shown to induce apoptosis in different cell lines and to regulate susceptibility to cell death (21, 22, 32). We showed here that thymocytes treated with different doses of GCS-100 were more susceptible to cell death in vitro. Moreover, double-positive thymocytes were the most affected cells, as observed also after dexamethasone treatment. From our new data, we suggest that both the increased GC contents and the lack of galectin-3 are likely to contribute to thymus atrophy in Gal-3−/− mice. Taking together our in vivo and in vitro results, it is important to point out the divergence regarding cell death susceptibility of double-positive subset in the absence of galectin-3. Further studies approaching in vivo treatment of thymocytes with GCS-100 inhibitor are needed to clarify this point. Another important issue to be considered is the production of other galectins by thymic epithelial cells described to induce apoptosis in thymocytes, namely galectins −1, −8 and −9 (33–36). It is possible that the lack of the anti-apoptotic galectin-3, together with the presence of the pro-apoptotic galectins −1, −8 and −9 would unbalance thymocyte homeostasis, favoring thymocytes to be more sensitive to pro-apoptotic effects of other galectins secreted by the thymus microenvironment and even to apoptotic factors such as GC.
We should also have in mind that the lack of galectin-3 during all lifespan of Gal-3−/− mice may have pleiotropic effects, influencing different organs that in sequence may affect the thymus. In fact, the lack of galectin-3 was shown to cause changes in the bone marrow, an important contributor to thymus cellularity with the generation of T cell precursors. This tissue was shown to be drastically modified in Gal-3−/− mice with reduced cell density (37). Alterations on B cell precursors were defined, but nothing was described for thymocyte precursors (38). A detailed study on T cell precursors of Gal-3−/− mice is missing.
Interesting changes in the thymus microenvironment were also observed in Gal-3−/− mice in relation to WT, such as the appearance of concentric structures similar to Hassall bodies, which are not commonly seen in young mouse thymuses. Hassall bodies are formed by TEC expressing high molecular weight cytokeratins, and represent advanced stages of TEC maturation (39). These alterations may be related to the high corticosterone contents observed in our mice, as GC hormones are able to induce senescence and changes in cytokeratin and ECM expression in TECs (40–42). The existence of a galectin-3/GC circuitry has not been well established up to now and demands further studies. It is not clear if the levels of GC hormones interfere with galectin-3 secretion and vice-versa. However, previous studies showed that mice submitted to stress or macrophages treated with GC have decreased galectin-3 expression (43–45).
Another intriguing alteration that called our attention in the thymus of Gal-3−/− mice was the presence of TEC-free regions in the thymic cortex, that were not observed in WT mice. Similar data were described previously in the thymus of aged mice and could be restored by oral zinc supplementation, as this chemical element is fundamental to thymus homeostasis, influencing the production of the thymic hormone thymulin, as well as TEC and thymocyte development (46). Cortical TEC-free regions have also been described in the thymus of different lupus strains of mice (NZB, MRL/MP-lpr/lpr, BXSB/MpJ Yaa, and C3H/HeJ-gld/gld), which undergo premature involution, but not in normal strains, including BALB/c, C57BL/6, and DBA (47). Changes in the thymic microenvironment, such as the occurrence of cortical TEC-free areas, may be harmful for T cell maturation, including positive selection that is dependent on cortical TEC/thymocyte interactions, reflecting in the accumulation of immature thymocytes and decreased thymus cellularity. Although no data relate these “TEC-free” regions to dysregulated selective events in the thymus, it is possible that the disturbed TEC-thymocyte interactions observed in Gal-3−/− mice contributes to the decrease in thymus cellularity.
Conclusion
Our data suggest that the lack of galectin-3 unbalances the steroidogenic machinery homeostasis in both thymus and adrenal, leading to an increase in local and systemic GC production, which in turn contributes to thymus atrophy by increasing thymocyte apoptosis and reducing thymocyte proliferation and TEC function. Besides, the direct effects of galectin-3 absence, such as defective intrathymic thymocyte migration, impaired proliferation and increased susceptibility to apoptosis, must be considered in the scenario of thymus atrophy observed in Gal-3−/−mice.
Author Contributions
EO performed all experiments and analyses, participated in study design and manuscript writing, DS participated in flow cytometry and immunofluorescence studies and analyses, AL participated in radioimmunoassay and performed qPCR analyses, MR participated in histological analyses, TR participated in immunofluorescence and proliferation studies, RF-R performed GCS-100 experiments, LV-F performed immunofluorescence analysis, RT participated in radioimmunoassay, VC-A participated in data interpretation and wrote the manuscript, VC performed radioimmunoassay studies, participated in data interpretation and wrote the manuscript, DV-V designed the study, participated in data interpretation and wrote the manuscript. All authors contributed to manuscript revision, read and approved the submitted version.
Conflict of Interest Statement
The authors declare that the research was conducted in the absence of any commercial or financial relationships that could be construed as a potential conflict of interest.
Acknowledgments
This work was supported by grants from CNPq (484235/2007-9 and 311242/2013-8), FAPERJ (E-26 111.373/2013), Oswaldo Cruz Institute, (Brazil) and Mercosur Fund for Structural Convergence (FOCEM) #03/11. The authors thank Dr. Sima Patel (La Jolla Pharmaceutical Company, San Diego, CA) for the kind donation of GCS-100.
References
1. Elola MT, Wolfenstein-Todel C, Troncoso MF, Vasta GR, Rabinovich GA. Galectins: matricellular glycan-binding proteins linking cell adhesion, migration, and survival. Cell Mol Life Sci. (2007) 64:1679–700. doi: 10.1007/s00018-007-7044-8
2. Liu FT, Patterson RJ, Wang JL. Intracellular functions of galectins. Biochim Biophys Acta (2002) 1572:263–73. doi: 10.1016/S0304-4165(02)00313-6
3. Dumic J, Dabelic S, Flögel M. Galectin-3: an open-ended story. Biochim Biophys Acta (2006) 1760:616–35. doi: 10.1016/j.bbagen.2005.12.020
4. Rabinovich GA, Toscano MA. Turning “sweet” on immunity: Galectin-glycan interactions in immune tolerance and inflammation. Nat Rev Immunol. (2009) 9:338–52. doi: 10.1038/nri2536
5. Villa-Verde DM, Silva-Monteiro E, Jasiulionis MG, Farias-De-Oliveira DA, Brentani RR, Savino W, et al. Galectin-3 modulates carbohydrate-dependent thymocyte interactions with the thymic microenvironment. Eur J Immunol. (2002) 32:1434–44. doi: 10.1002/1521-4141(200205)32:5<1434::AID-IMMU1434>3.0.CO;2-M
6. Silva-Monteiro E, Reis Lorenzato L, Kenji Nihei O, Junqueira M, Rabinovich GA, Hsu DK, et al. Altered expression of galectin-3 induces cortical thymocyte depletion and premature exit of immature thymocytes during Trypanosoma cruzi infection. Am J Pathol. (2007) 170:546–56. doi: 10.2353/ajpath.2007.060389
7. Taub DD, Longo DL. Insights into thymic aging and regeneration. Immunol Rev. (2005) 205:72–93. doi: 10.1111/j.0105-2896.2005.00275.x
8. Lynch HE, Goldberg GL, Chidgey A, Van den Brink MR, Boyd R, Sempowski GD. Thymic involution and immune reconstitution. Trends Immunol. (2009) 30:366–73. doi: 10.1016/j.it.2009.04.003
9. Gruver AL, Sempowski GD. Cytokines, leptin, and stress-induced thymic atrophy. J Leukoc Biol. (2008) 84:915–23. doi: 10.1189/jlb.0108025
10. De Meis J, Aurélio Farias-De-Oliveira D, Nunes Panzenhagen PH, Maran N, Villa-Verde DM, Morrot A, et al. Thymus atrophy and double-positive escape are common features in infectious diseases. J Parasitol Res. (2012) 2012:574020. doi: 10.1155/2012/574020
11. Savino W, Dardenne M. Immune-neuroendocrine interactions. Immunol Today (1995) 16:318–22. doi: 10.1016/0167-5699(95)80144-8
12. Savino W, Mendes-Da-Cruz DA, Lepletier A, Dardenne M. Hormonal control of T-cell development in health and disease. Nat Rev Endocrinol. (2016) 12:77–89. doi: 10.1038/nrendo.2015.168
14. Berki T, Pálinkás L, Boldizsár F NP. Glucocorticoid (GC) sensitivity and GC receptor expression differ in thymocyte subpopulations. Int Immunol. (2002) 14:463–9.
15. Vacchio MS, Lee JY, Ashwell JD. Thymus-derived glucocorticoids set the thresholds for thymocyte selection by inhibiting TCR-mediated thymocyte activation. J Immunol. (1999) 163:1327–33.
16. Lepletier A, de Carvalho VF, Rodrigues e Silva PM, Villar S, Pérez AR, Savino W, et al. Trypanosoma cruzi disrupts thymic homeostasis by altering intrathymic and systemic stress-related endocrine circuitries. PLoS Negl Trop Dis. (2013) 7:e2470. doi: 10.1371/journal.pntd.0002470.
17. Hsu DK, Yang RY, Pan Z, Yu L, Salomon DR, Fung-Leung WP, et al. Targeted disruption of the galectin-3 gene results in attenuated peritoneal inflammatory responses. Am J Pathol. (2000) 156:1073–83. doi: 10.1016/S0002-9440(10)64975-9
18. Torres RC, Magalhães NS, Rodrigues e Silva PM, Martins MA, Carvalho VF. Activation of PPAR-γ reduces HPA axis activity in diabetic rats by up-regulating PI3K expression. Exp Mol Pathol. (2016) 101:290–301. doi: 10.1016/j.yexmp.2016.10.002
19. Livak KJ, Schmittgen TD. Analysis of relative gene expression data using real-time quantitative PCR and the 2-ΔΔCT method. Methods (2001) 25:402–8. doi: 10.1006/meth.2001.1262
20. Ciofani M, Zúñiga-Pflücker JC. The thymus as an inductive site for T lymphopoiesis. Annu Rev Cell Dev Biol. (2007) 23:463–93. doi: 10.1146/annurev.cellbio.23.090506.123547
21. Chauhan D, Li G, Podar K, Hideshima T, Neri P, He D, et al. A novel carbohydrate-based therapeutic GCS-100 overcomes bortezomib resistance and enhances dexamethasone-induced apoptosis in multiple myeloma cells. Cancer Res. (2005) 65:8350–8. doi: 10.1158/0008-5472.CAN-05-0163
22. Streetly MJ, Maharaj L, Joel S, Schey SA, Gribben JG, Cotter FE. GCS-100, a novel galectin-3 antagonist, modulates MCL-1, NOXA, and cell cycle to induce myeloma cell death. Blood (2010) 115:3939–48. doi: 10.1182/blood-2009-10-251660
23. Savino W, Mendes-da-Cruz DA, Smaniotto S, Silva-Monteiro E, Villa-Verde DM. Molecular mechanisms governing thymocyte migration: combined role of chemokines and extracellular matrix. J Leukoc Biol. (2004) 75:951–61. doi: 10.1189/jlb.1003455
24. Sage EH, Bornstein P. Extracellular proteins that modulate cell-matrix interactions. SPARC, tenascin, and thrombospondin. J Biol Chem. (1991) 266:14831–4.
25. Levy Y, Arbel-Goren R, Hadari YR, Eshhar S, Ronen D, Elhanany E, et al. Galectin-8 functions as a matricellular modulator of cell adhesion. J Biol Chem. (2001) 276:31285–95. doi: 10.1074/jbc.M100340200
26. Yang RY, Hsu DK, Liu FT. Expression of galectin-3 modulates T-cell growth and apoptosis. Proc Natl Acad Sci U S A. (1996) 93:6737–42. doi: 10.1073/pnas.93.13.6737
27. Akahani S, Nangia-Makker P, Inohara Hi, Kim H-R Ci, Raz A. Galectin-3 : a novel antiapoptotic molecule with a functional BH1 ( NWGR ) A-J. Cancer Res. (1997) 57:5272–6.
28. Moutsatsos IK, Wadet M, Schindler M, Wang JL. Endogenous lectins from cultured cells : nuclear localization of carbohydrate-binding protein 35 in proliferating 3T3 fibroblasts. Proc Natl Acad Sci U S A. (1987) 84:6452–6456.
29. Tsawdaroglou NG, Govindan MV, Schmid W SC. Dexamethasone-binding proteins in cytosol and nucleus of rat thymocytes purification of three receptor proteins. Eur J Biochem. (1981) 114:305–13. doi: 10.1111/j.1432-1033.1981.tb05150.x
30. Ranelletti FO, Maggiano N, Aiello FB, Carbone A, Larocca LM, Musiani P, et al. Glucocorticoid receptors and corticosensitivity of human thymocytes at discrete stages of intrathymic differentiation. J Immunol. (1987) 138:440–5.
31. Farias-de-Oliveira DA, Villa-Verde DM, Panzenhagen PH, Silva dos Santos D, Berbert LR, Savino W, et al. Caspase-8 and caspase-9 mediate thymocyte apoptosis in trypanosoma cruzi acutely infected mice. J Leukoc Biol. (2013) 93:227–34. doi: 10.1189/jlb.1211589
32. Clark MC, Pang M, Hsu DK, Liu F, de Vos S, Gascoyne RD, et al. Galectin-3 binds to CD45 on diffuse large B-cell lymphoma cells to regulate susceptibility to cell death. Blood (2012) 120:4635–45. doi: 10.1182/blood-2012-06-438234
33. Baum LG, Pang M, Perillo NL, Wu T, Delegeane A, Uittenbogaart CH, et al. Human thymic epithelial cells express an endogenous lectin, galectin-1, which binds to core 2 O-glycans on thymocytes and T lymphoblastoid cells. J Exp Med. (1995) 181:877–87.
34. Tribulatti MV, Mucci J, Cattaneo V, Agücero F, Gilmartin T, Head SR, et al. Galectin-8 Induces apoptosis in the CD4highCD8high thymocyte subpopulation. Glycobiology (2007) 17:1404–12. doi: 10.1093/glycob/cwm104
35. Wada J, Ota K, Kumar A, Wallner EI, Kanwar YS. Developmental regulation, expression, and apoptotic potential of galectin-9, a beta-galactoside binding lectin. J Clin Invest. (1997) 99:2452–61. doi: 10.1172/JCI119429
36. Bi S, Earl LA, Jacobs L, Baum LG. Structural features of galectin-9 and galectin-1 that determine distinct T cell death pathways. J Biol Chem. (2008) 283:12248–58. doi: 10.1074/jbc.M800523200
37. Brand C, Oliveira FL, Ricon L, Fermino ML, Boldrini LC, Hsu DK, et al. The bone marrow compartment is modified in the absence of galectin-3. Cell Tissue Res. (2011) 346:427–37. doi: 10.1007/s00441-011-1276-5
38. De Oliveira FL, Dos Santos SN, Ricon L, Da Costa TP, Pereira JX, Brand C, et al. Lack of galectin-3 modifies differentially Notch ligands in bone marrow and spleen stromal cells interfering with B cell differentiation. Sci Rep. (2018) 8:1–14. doi: 10.1038/s41598-018-21409-7
39. Nicolas JF, Reano A, Kaiserlian D, Thivolet J. Epithelial cell heterogeneity in mammalian thymus: monoclonal antibody to high molecular weight keratins exclusively binds to Hassall's corpuscles. Histochem J. (1989) 21:357–64.
40. Talaber G, Kvell K, Varecza Z, Boldizsar F, Parnell SM, Jenkinson EJ, et al. Wnt-4 Protects Thymic Epithelial Cells Against Dexamethasone-Induced Senescence. Rejuvenation Res. (2011) 14:241–8. doi: 10.1089/rej.2010.1110
41. Cirne-Lima EO SW. Repeated in vivo hydrocortisone treatment promotes a dual modulation of cytokeratin expression by mouse thymic epithelial cells. Neuroimmunomodulation (1998) 5:328–31. doi: 10.1159/000026353
42. Lannes-Vieira J, Dardenne M, Savino W. Extracellular matrix components of the mouse thymus microenvironment: ontogenetic studies and modulation by glucocorticoid hormones. J Histochem Cytochem. (1991) 39:1539–46. doi: 10.1177/39.11.1918928
43. Dumić J1, Barisić K, Flögel M, Lauc G. Galectin-3 decreases in mice exposed to immobilization stress. Stress (2000) 3:241–6.
44. Maldonado CA, Sundblad V, Salatino M, Elia O, García LN, Leimgruber C, et al. Cell-type specific regulation of galectin-3 expression by glucocorticoids in lung Clara cells and macrophages. Histol Histopathol. (2011) 26:747–59. doi: 10.14670/HH-26.747
45. Dabelic S, Supraha S, Dumic J. Galectin-3 in macrophage-like cells exposed to immunomodulatory drugs. Biochim Biophys Acta (2006) 1760:701–9. doi: 10.1016/j.bbagen.2005.11.020
46. Dardenne M, Boukaiba N, Gagnerault MC, Homo-Delarche F, Chappuis P, Lemonnier D et al. Restoration of the thymus in aging mice by in vivo zinc supplementation. Clin Immunol Immunopathol. (1993) 66:127–35. doi: 10.1006/clin.1993.1016
Keywords: galectin-3, thymus, thymocytes, proliferation, cell death, glucocorticoid, steroidogenic machinery
Citation: Oliveira-de-Abreu E, Silva-dos-Santos D, Lepletier A, Ramos TDP, Ferreira-Reis R, Vasconcelos-Fontes L, Ramos MT, Torres RC, Cotta-de-Almeida V, Carvalho VF and Villa-Verde DMS (2018) Lack of Galectin-3 Disrupts Thymus Homeostasis in Association to Increase of Local and Systemic Glucocorticoid Levels and Steroidogenic Machinery. Front. Endocrinol. 9:365. doi: 10.3389/fendo.2018.00365
Received: 01 February 2018; Accepted: 18 June 2018;
Published: 10 July 2018.
Edited by:
Vincent Geenen, University of Liège, BelgiumReviewed by:
Gábor B. Makara, Hungarian Academy of Sciences (MTA), HungaryEva Tolosa, Universitätsklinikum Hamburg-Eppendorf, Germany
Copyright © 2018 Oliveira-de-Abreu, Silva-dos-Santos, Lepletier, Ramos, Ferreira-Reis, Vasconcelos-Fontes, Ramos, Torres, Cotta-de-Almeida, Carvalho and Villa-Verde. This is an open-access article distributed under the terms of the Creative Commons Attribution License (CC BY). The use, distribution or reproduction in other forums is permitted, provided the original author(s) and the copyright owner(s) are credited and that the original publication in this journal is cited, in accordance with accepted academic practice. No use, distribution or reproduction is permitted which does not comply with these terms.
*Correspondence: Déa M. S. Villa-Verde, ZHZ2QGlvYy5maW9jcnV6LmJy; ZGVhdmlsbGF2ZXJkZUBnbWFpbC5jb20=
†Present Address: Ailin Lepletier, Laboratory of Immunology in Cancer and Infection, Department of Immunology, Queensland Institute of Medical Research, Brisbane, Australia