- Laboratorio de Neurobiología Molecular y Celular, Departamento de Genética del Desarrollo y Fisiología Molecular, Instituto de Biotecnología, Universidad Nacional Autónoma de México (UNAM), Cuernavaca, Mexico
Neonatal stress contributes to the development of obesity and has long-lasting effects on elements of the hypothalamus–pituitary–thyroid (HPT) axis. Given the importance of thyroid hormones in metabolic regulation, we studied the effects of maternal separation and a high-fat/high-carbohydrate diet (HFC), offered from puberty or adulthood, on HPT axis activity of adult male and female Wistar rats. Pups were non-handled (NH) or maternally separated (MS) 3 h/day at postnatal days (Pd) 2–21. In a first experiment, at Pd60, rats had access to chow or an HFC diet (cookies, peanuts, chow) for 1 month. Male and female NH and MS rats that consumed the HFC diet increased their caloric intake, body weight, and serum insulin levels; fat weight increased in all groups except in MS males, and serum leptin concentration increased only in females. Mediobasal hypothalamus (MBH) Pomc expression increased in NH-HFC females and Npy decreased in NH-HFC males. MS males showed insulinemia and hypercortisolemia that was attenuated by the HFC diet. The HPT axis activity response to an HFC diet was sex-specific; expression of MBH thyrotropin-releasing hormone-degrading ectoenzyme (Trhde) increased in NH and MS males; serum TSH concentration decreased in NH males, and T4 increased in NH females. In a second experiment, rats were fed chow or an HFC diet from Pd30 or 60 until Pd160 and exposed to 1 h restraint before sacrifice. Regardless of neonatal stress, age of diet exposition, or sex, the HFC diet increased body and fat weight and serum leptin concentration; it induced insulinemia in males, but in females only in Pd30 rats. The HFC diet's capacity to curtail the hypothalamus–pituitary–adrenal axis response to restraint was impaired in MS males. In restrained rats, expression of Trh in the paraventricular nucleus of the hypothalamus, Dio2 and Trhde in MBH, and serum thyroid hormone concentration were altered differently depending on sex, age of diet exposition, and neonatal stress. In conclusion, metabolic alterations associated to an HFC-diet-induced obesity are affected by sex or time of exposition, while various parameters of the HPT axis activity are additionally altered by MS, pointing to the complex interplay that these developmental influences exert on HPT axis activity in adult rats.
Introduction
Obesity is a devastating human health problem in modern societies (1). Sedentarism and stress, together with access to cheap palatable food high in fats and carbohydrates (HFC), are strong contributors whose interacting effects may synergize and provoke the metabolic syndrome (2, 3). Early-life stress due to deficient maternal care, mimicked in rodents by separation of pups from their mother, alters hypothalamus–pituitary–adrenal (HPA) axis reactivity to stress, food intake, and body weight, and impairs learning and induces anxiety and depressive behaviors (4–7). Outcomes vary among studies and may depend on time, length, and mode of pups' separation, age, or sex (4, 8–10). Daily maternal separation (MS) for 3 h during at least the first 10 postnatal days (Pd) induces long-term stress hyper-reactivity related to changes in DNA methylation of several rat or mice gene promoters involved in HPA axis activity, such as hippocampal glucocorticoid receptor (Gr), hypothalamic corticotrophin-releasing hormone (Crh) and vasopressin, and pituitary pro-opiomelanocortin (Pomc) (11–14). Consumption of a palatable diet rich in carbohydrates and/or fats attenuates stress response in both adult humans and rats (15–17); likewise, MS rats fed an HFC diet present a blunted HPA response to restraint stress compared to chow-fed rats (6).
The sympathoadrenal and neuroendocrine systems, including the hypothalamus–pituitary–thyroid (HPT) axis, are major regulators of energy homeostasis activating adrenergic, glucocorticoid and thyroid hormone (TH) receptors that modulate carbohydrate, lipid, and protein metabolism in multiple organs (18, 19). THs regulate basal metabolic rate accounting for 30% of energy expenditure (20) and, together with noradrenergic stimulation of brown adipose tissue, are responsible for thermogenesis (18). The important role of TH in energy homeostasis includes enhancement of lipolysis in adipose tissue followed by fatty acid uptake by active metabolic tissues, as well as increase of glucose metabolism (18, 21). The HPT axis is controlled by thyrotropin-releasing hormone (TRH) synthesized in neurons of the hypothalamic paraventricular nucleus (PVN) and released at the median eminence near portal vessels that communicate with the anterior pituitary where TRH activates TRH-R1 receptor in thyrotrophs increasing synthesis and release of thyrotropin (TSH). TSH controls the synthesis of thyroxine (T4) at the thyroid; T4 is converted to 3,3′,5-triiodo-L-thyronine (T3) by tissue deiodinases I or II (22–25). Released TRH at the median eminence may be degraded by the TRH-degrading ectoenzyme (TRH-DE), present in tanycytes, before it travels to the pituitary regulating the amount of TRH that reaches the thyrotrophs (Figure 5A) (26).
Chronic and some forms of acute stress inhibit various elements of the HPT axis in rats (24, 25, 27–29), and stress during critical periods of development may program its function. A history of physical/emotional abuse or neglect has been linked with reduced plasma levels of T3 in adolescents (30) and low plasma levels of TSH in adult women (31).
In a previous study, we showed that maternal separation causes long-term changes in the offspring in a sex-specific manner on some elements of the HPT axis of adult rats and blunts fasting-induced inhibition of the HPT axis activity of male rats but not females (32). Thus, we hypothesized that stress early in life may cause sex-specific responses of the HPT axis activity to an HFC diet in adult rats. Given the importance of THs in lipid and glucose metabolism as well as energy expenditure (18), the biological and physiological differences between males and females in energy metabolism (33), and especially in the neuroendocrine responses to stress (34, 35), we studied the effects of maternal separation and an HFC diet, starting at puberty or adulthood, on the HPT axis activity under basal conditions or after an acute stress insult in male and female adult rats. We also evaluated some metabolic (leptin, insulin, glucose) and neuroendocrine and endocrine (Pomc and Npy expression, HPA axis) parameters related to diet-induced obesity and stress, some of which modulate the activity of TRH neurons in the PVN.
Materials and Methods
Animals
Protocols followed the NIH Guide for the Care and Use of Laboratory Animals and the Official Mexican Norm for production, care, and use of laboratory animals NOM-062-ZOO-1999. All experiments were approved by the Bioethics Committee of the Instituto de Biotecnología, UNAM (authorization project number 311). Protocols for experiments 1 and 2 are described in Figure 1.
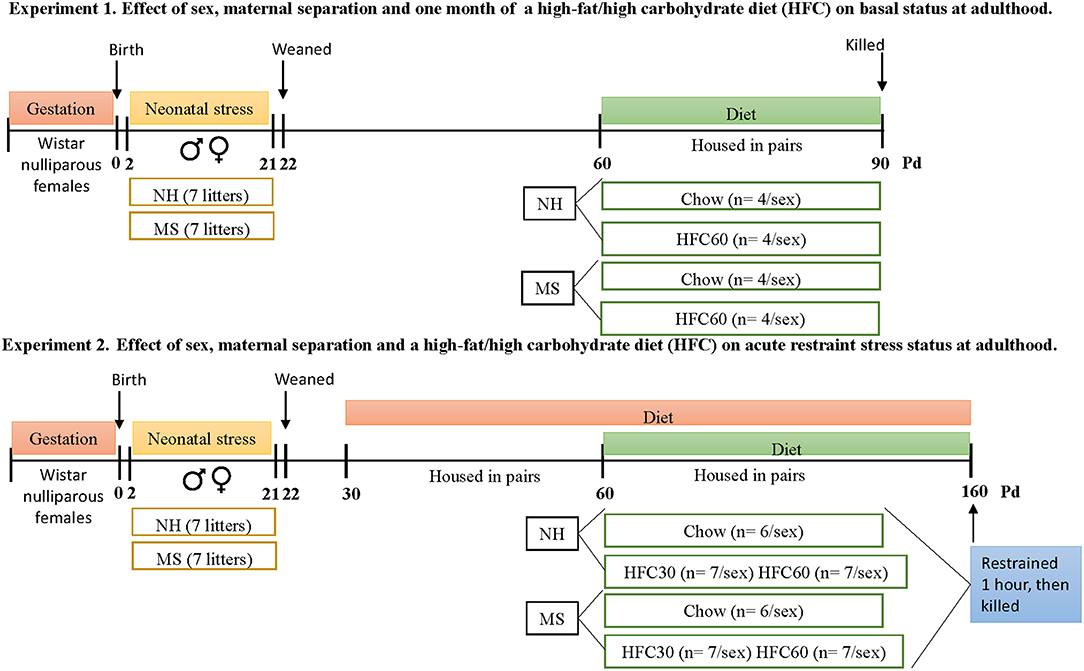
Figure 1. Timeline of protocols followed in experiments 1 and 2. Each experimental group from both experiments was composed by rats from a different litter, to avoid litter-mates within the same group. Abbreviations: HFC30, fed a high-fat/high-carbohydrate diet from postnatal day 30; HFC60, fed a high-fat/high-carbohydrate diet from postnatal day 60; MS, maternal separation; NH, non-handled; Pd, postnatal day.
Maternal Separation
Two independent experiments were performed, using a different cohort of nulliparous Wistar female rats (n = 14/experiment) from the Institute's outbred colony; pregnant rats were individually housed in a temperature (22 ± 1°C) and humidity (50–55%) controlled room on a 12-h dark–light cycle with chow (Teklad 2018SX, Envigo, USA) and water available ad libitum. As previously described (32), the day of birth was considered postnatal day 0 (Pd0); at Pd1, litters were culled to 8 pups/dam (4 males and 4 females) and were randomly assigned to the non-handled group (NH, n = 7 litters) for which pups were only manipulated once a week for cage cleaning or the maternal separation group (MS, n = 7 litters). Briefly, for the MS procedure, starting at Pd2 and until Pd21, pups were placed in a new cage with standard bedding material at 9:00 a.m. and moved to an adjacent room with controlled temperature (30–32 ± 0.5°C), 3 h/day. Pups were weaned at Pd22, and one rat from each litter was randomly selected to house four/cage according to sex and neonatal protocol (NH or MS); at Pd60, rats were housed two/cage, in separate rooms according to sex. In experiment 1, a total of 16 males and 16 females were used; in experiment 2, a total of 40 males and 40 females of a different cohort were used.
Diet
Maternal separation affects the metabolic response to a high-fat/high-sucrose diet later in life (4) and changes some elements of the HPT axis in adult rats (32); in addition, there is a clear relationship between palatable foods high in fats and carbohydrates and stress in humans and animals that suggests a therapeutic value of “comfort foods”; in turn, stress affects feeding behavior and food choice (2, 5, 36). Thus, in the first experiment (Figure 1), we evaluated the response of the HPT axis of MS adult rats to 1 month of a palatable diet high in fat and high in carbohydrates (HFC). From Pd60 to 90, rats were randomly exposed to chow (C; NH, n = 4/sex; MS, n = 4/sex) or an HFC diet (NH, n = 4/sex; MS, n = 4/sex); all animals within each experimental group came from different litters. Basal activity of the HPT axis is inhibited in response to an acute stress (24), and the type of diet can alter the stress response of adult rats (2, 5, 36). In addition, adolescence is a vulnerable developmental period where feeding behavior is susceptible to insults (37) and availability of palatable foods increases female susceptibility to develop obesity and metabolic syndrome later in life (37, 38) and it may be exacerbated by MS in adult female rats (9). Thus, in the second experiment (Figure 1), we studied the effect of restraint stress on the activity of the HPT axis of adult obese animals with or without neonatal stress. To ensure an obese state in adult animals, chow (NH, n = 6/sex; MS, n = 6/sex) or an HFC diet (NH, n = 7/sex; MS, n = 7/sex) was offered to juvenile (Pd30) or adult (Pd60) rats for a longer period (until Pd160) compared to experiment 1. As in the first experiment, all animals within each experimental group came from different litters. NH and MS groups had ad libitum access to either chow (Teklad 2018SX, Envigo, USA) or an HFC diet and water. Each cage of HFC-fed rats contained three choices of food: chow [Teklad 2018SX, Envigo, USA, diet composition: 3.1 kcal/g; 21.03 g of protein, 7.01 g of fat (84% of unsaturated fatty acids, 16% of saturated fats), and 50.8 g of complex carbohydrates per 100 g], organic-animal cookies [3.9 kcal/g; 7.14 g of protein, 10.71 g of fat (66.6% of unsaturated fatty acids, 33.3% of saturated fat), and 67.85 g of carbohydrate (73.6% of complex carbohydrates, 26.3% of sugar) per 100 g; vanilla flavored, Kirkland, USA], or raw unsalted-skinned peanuts produced in Mexico [5.68 kcal/g; 25.75 g of protein, 49.4 g of fat (93.6% of unsaturated fatty acids, 6.4% of saturated fats), and 16.23 g of carbohydrate per 100 g]. Body weight was registered weekly and food intake was registered every fourth day (considering spilled food). Intake of some essential amino acids was calculated, since wheat (from cookies) is deficient in lysine, and peanuts in threonine and methionine (39, 40); digestibility and bioavailability of protein was considered (39, 41).
Restraint Stress
To evaluate the HPT axis sensitivity of adult male and female obese rats to an acute stress with or without maternal separation, in the second experiment at Pd160, rats were restrained from 9:00 to 10:00 a.m. in a prone position, and immediately sacrificed. Since body size of rats varied, a metal wire mesh was folded according to their size, with their tail hanging out to avoid heating. A baseline blood sample was taken by tail clipping within 3 min (250–300 μl) and at 30 and 60 min following initiation of the restraint to quantify serum corticosterone levels (42). Blood glucose concentration was measured before and 60 min after introducing the rats to the restrainer using an Accutrend strip and analyzer (Roche Diagnostics, USA).
Tissue Collection
Non-fasted animals were killed (43) at Pd90 (experiment 1) or Pd160 (experiment 2) by decapitation (42), in an independent room, by an experienced technician; trunk blood was collected to obtain the serum and was stored in aliquots at −20°C for posterior hormone determinations. Brains were immediately removed and carefully placed on crushed dry ice; once frozen, they were stored at −70°C for mRNA purification and expression analyses of genes of interest. White adipose tissue (WAT) depots (gonadal, retroperitoneal, and interscapular) were dissected and weighed fresh.
mRNA Level Analyses
Coronal brains sections were dissected to obtain the hypothalamic PVN (Bregma −0.84 to −2.3 mm) or the mediobasal hypothalamus (MBH; Bregma −2.3 to −3.6 mm), which contains the arcuate nucleus, basal part of the third ventricle with tanycytes, and median eminence, using a 1- or a 0.5-mm-internal-diameter sample corer (Fine Science Tools, Foster City, CA), respectively. Total RNA was extracted from frozen PVN or MBH using the guanidine thiocyanate method (44); RNA concentration was quantified and its purity was verified, using a Nanodrop spectrophotometer (ThermoScientific 2000c). RNA integrity of every sample was verified by running an aliquot (0.5 μg) of intact RNA on a denaturing agarose gel; a 28S/18S rRNA ratio above 1.5 was considered adequate to use for RT-PCR. Relative mRNA levels were measured by RT-PCR; PVN Trh and Crh, and MBH Trhde and deiodinase type 2 (Dio2) mRNAs were measured as previously described (45–47). Amplification conditions for MBH Npy (5′-TATCCCTGCTCGTGTGTTTG-3′ and 5′-GTTCTGGGGGCATTTTCTG-3′) and Pomc (5′-TTGATGATGGCGTTCTTGAA-3′ and 5′-GAGATTCTGCTACAGTCGCTC-3′) cDNAs were as follows: Tm = 64°C, 26 and 27 cycles, respectively. The expression level of target genes was normalized against cyclophilin (experiment 1) or 18S ribosomal RNA (experiment 2; 5′-CGGACAGGATTGACAGATTG-3′, 5′-CAAATCGCTCCACCAACTAA-3′; Tm = 64°C and 17 cycles). Primers were synthesized in the synthesis and sequencing DNA unit of Instituto de Biotecnología, UNAM.
Serum Hormone Analyses
Serum concentration of TSH (NIDDK reagents, Bethesda, MD; sensitivity range = 2.5–80 ng/ml) and corticosterone (reagents from Merck-Millipore, Perkin Elmer and Sigma; limits of detection, 20–2,000 ng/ml) were quantified by radioimmunoassay. Total T3 (limits of detection, 0.75–10 ng/ml) and T4 (limits of detection, 1–30 μg/dl) were quantified by ELISA [kits from Diagnóstica Internacional (Zapopan, JAL., México)] following the manufacturer's instructions, except for one variation: standard curve was prepared by adding 25 μl of rat hypothyroid serum to the calibrators provided in the kit, as recommended (48). Leptin (assay range, 0.2–12.8 ng/ml) and insulin (sensitivity range, 0.1–12.8 ng/ml) were measured using rat ELISA kits from Crystal Chem Inc. (Downers Grove, IL), ACTH using an ELISA kit from USCNK Life Science (Wuhan P.R., China; detection range, 12.35–1,000 pg/ml), and 17β-estradiol using an ELISA kit from Arbor assays (Michigan, USA; limits of detection, 3.75–120 pg/ml). Experiments 1 and 2 were analyzed independently; for each experiment, samples from male and female rats were measured in duplicate within a single assay, and inter-assay and intra-assay coefficients of variation were below 10%.
Statistical Analyses
Results are reported as the mean ± S.E.M. Data were analyzed by a three-way ANOVA to determine effects of neonatal stress (NH vs. MS), diet (chow vs. HFC), sex (male vs. female rats), and the interaction of these variables. If a significant main effect or interaction was found, ANOVA was followed by the Holm–Sidak multiple comparisons test; the level of significance was set at p < 0.05. Pearson's correlation coefficient was used to examine the association between serum leptin or insulin levels and some predictive variables of obesity. Statistical analyses were performed using the GraphPad Prism8 software.
Results
Experiment 1. Effect of Sex, Maternal Separation, and 1 Month of an HFC Diet on Basal Status at Adulthood
Food Intake and Body Weight
Food intake and body weight were monitored from weaning until the end of the experiment (Pd90). There was no difference in the amount of chow consumed during Pd30–60 between MS and NH male rats (Supplementary Figure 1A), though MS males weighed slightly less than NH rats [stress effect F(1, 548) = 4.79, p = 0.04; Supplementary Figure 1B]. MS did not affect chow intake and body weight of females at this age period (Supplementary Figures 1A,B). During Pd60–90, there was an effect of MS [F(1, 80) = 6.21, p = 0.02], diet [F(2, 80) = 92.83, p < 0.0001], sex [F(1, 80) = 281.22, p < 0.0001], and an interaction of diet and sex [F(2, 80) = 4.5, p = 0.04] on food intake. Chow-fed MS male rats, but not females, reduced their food intake compared to the NH group (Supplementary Figure 2A), but MS rats gained weight similarly to the NH group in both sexes; no differences were observed in intake of kcal/kg of body weight (BW) between MS-chow and NH-chow (Table 1). The HFC diet offered starting at adulthood (Pd60) for 1 month reduced chow intake in both sexes, and both NH and MS groups increased caloric intake, mainly from cookies and peanuts (Supplementary Figures 2A,B), body weight [except MS-HFC60 females; diet effect F(1, 23) = 53.18, p < 0.0001; sex effect F(1, 23) = 17.63, p = 0.0003; MS and diet F(1, 23) = 5.87, p = 0.02; diet and sex F(1, 23) = 6.81, p = 0.015; MS, diet and sex F(1, 23) = 11.22, p = 0.002], and gonadal fat weight/kg [except in MS-HFC60 males; diet effect F(1, 23) = 15.4, p = 0.008; Table 1]. NH and MS females had a smaller intake of total kilocalories than males [sex effect F(1, 23) = 281.2, p < 0.0001], but given their lower body weight, the relative intake (kcal/kg BW) of NH-HFC60 in females was higher than in males [sex effect F(1, 23) = 6.78, p = 0.015; Table 1].
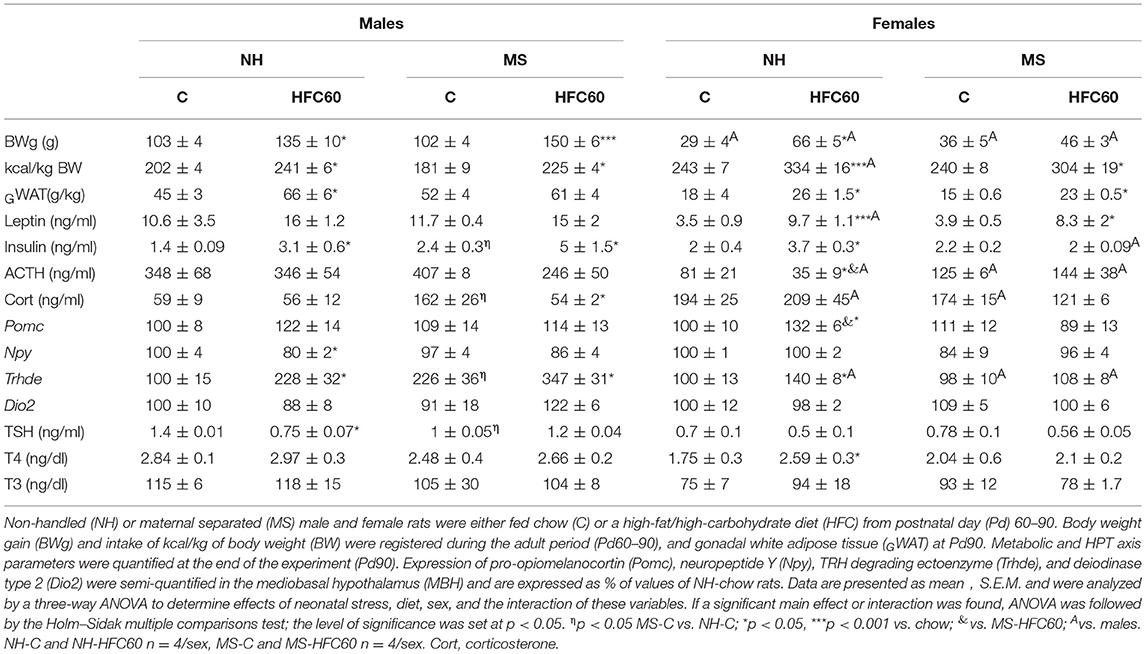
Table 1. Effect of maternal separation and 1 month of a high-fat/high-carbohydrate diet on metabolic and HPT axis parameters (experiment 1).
Neuroendocrine and Endocrine Parameters
Serum insulin levels slightly increased in MS-chow-fed males but not females [sex effect F(1, 23) = 5.34, p = 0.03; Table 1], as previously described (9). After 1 month of exposure to the HFC diet, although relative epididymal WAT weight increased in NH male rats, serum leptin concentration did not change, while insulinemia was induced in NH-HFC60- and MS-HFC60-fed male rats [diet effect F(1, 23) = 4.25, p = 0.05; Table 1]. Serum insulin concentration correlated positively with epididymal WAT weight of HFC-60 groups (r = 0.81; p = 0.01) but not of chow-fed males, whose epididymal WAT weight correlated instead with serum leptin concentration (r = 0.66; p = 0.03). In contrast, in NH and MS females fed an HFC diet, serum leptin concentration increased [diet effect F(1, 23) = 22.43, p = 0.0002; sex effect F(1, 23) = 5.96, p = 0.02; diet and sex effect F(1, 23) = 5.51, p = 0.03], while insulinemia was only present in NH-HFC60 rats (Table 1).
MS induced persistent stress in chow-fed male rats, evidenced by increased serum corticosterone concentration that was lowered by 1 month of feeding an HFC diet [MS and diet effect F(1, 23) = 6.48, p = 0.02; MS and sex effect F(1, 23) = 5.82, p = 0.02; Table 1], but no change in serum ACTH concentration was observed, compared to NH-chow-fed rats (Table 1). In contrast, neither an HFC diet nor MS affected stress markers in adult female rats, except for a lower serum ACTH concentration in NH-HFC60 compared to chow-fed female rats [MS and sex effect F(1, 23) = 12.11, p = 0.0025; MS, diet, and sex effect F(1, 23) = 5.41, p = 0.03; Table 1]. Serum 17β-estradiol concentration was not affected by MS or 1 month of feeding an HFC diet in female rats (Supplementary Figure 3A).
Expression of MBH peptides was modified by sex and/or the HFC diet, but not by MS; Pomc mRNA levels increased in NH-HFC60 females compared to NH-chow and MS-HFC60 groups [diet effect F(1, 23) = 5.04, p = 0.04; Table 1], and Npy mRNA levels decreased in NH-HFC60 males compared to chow-fed rats [diet effect F(1, 23) = 5.51, p = 0.04; diet and sex effect F(1, 23) = 13.5, p = 0.001; Table 1] as reported by Obici et al. (49) and Schwinkendorf et al. (50).
MS modified elements involved in the HPT axis activity, including an increase of Trhde mRNA levels in the MBH [MS effect F(1, 23) = 6.67, p = 0.019; sex effect F(1, 23) = 29.86, p < 0.0001; MS and sex effect F(1, 23) = 10.28, p = 0.005; Table 1] and a decrease of serum TSH concentration [MS effect F(1, 23) = 5.47, p = 0.04; Table 1] compared to NH males, but not in females, as published (32). Consumption of an HFC diet increased MBH-Trhde expression in NH and MS males [diet effect F(1, 23) = 13.02, p = 0.002; diet and sex effect F(1, 23) = 5.15, p = 0.03; Table 1], while only in NH-HFC60 females compared to NH-chow-fed rats (Table 1). MBH-Dio2 expression was not modified under any condition (Table 1). The HFC diet decreased serum TSH concentration in NH males compared to chow-fed rats [diet effect F(1, 23) = 4.31, p = 0.05; Table 1], whereas that of T4 was higher only in female NH-HFC60 than in NH-chow rats [diet effect F(1, 23) = 4.28, p = 0.05; Table 1].
Experiment 2. Effect of Sex, Maternal Separation, and an HFC Diet on Acute Restraint Stress Status at Adulthood
Food Intake and Diet Preference, Body Weight, and Composition
NH and MS rats were fed a chow or a high-fat/high-carbohydrate diet from Pd30 or 60 up to Pd160 and then exposed 1 h to restraint stress before sacrifice. As in experiment 1, MS males and females on a chow diet consumed similar kcal as their respective NH group during adolescence (Figures 2A,D). MS did not change food preference; however, MS-chow rats of both sexes had lower food intake than NH-chow animals during adulthood, with males consuming more kcal than females [MS effect F(1, 80) = 10.8, p < 0.0014; sex effect F(1, 80) = 65.4, p < 0.0001; MS and sex effect F(1, 80) = 5.66, p = 0.01; Figures 2B,E]; thus, the proportion of each macronutrient contributing to total kilocalorie intake remained the same (Supplementary Table 1). Availability of palatable food diminished chow intake [diet effect F(1, 80) = 4011.23, p < 0.0001; sex effect F(1, 80) = 20.45, p < 0.0001; sex and diet effect F(1, 80) = 15.45, p = 0.0002], with cookies being the largest contributor of kilocalorie intake in male and female rats followed by peanuts, at all ages (Figures 2A,B,D,E). NH and MS male and female rats that had access to the HFC from Pd30 (HFC30 group) consumed slightly more total kilocalories compared to chow-fed groups during the adolescence period [diet effect F(1, 80) = 157.24, p < 0.0001; MS and diet effect F(1, 80) = 11.2, p = 0.001; diet and sex effect F(1, 80) = 33.97, p < 0.0001; Figures 2A,B]. During the adult period (Pd61–160), all NH-HFC and MS-HFC fed male and female rats consumed higher total kilocalories than chow-fed rats, but kilocalorie intake was even higher in the NH-HFC60 group compared to MS-HFC60 males [MS effect F(1, 80) = 10.8, p = 0.0014; diet effect F(2, 80) = 77.45, p < 0.0001; diet and sex effect F(1, 80) = 33.97, p < 0.0001; Figures 2B,E].
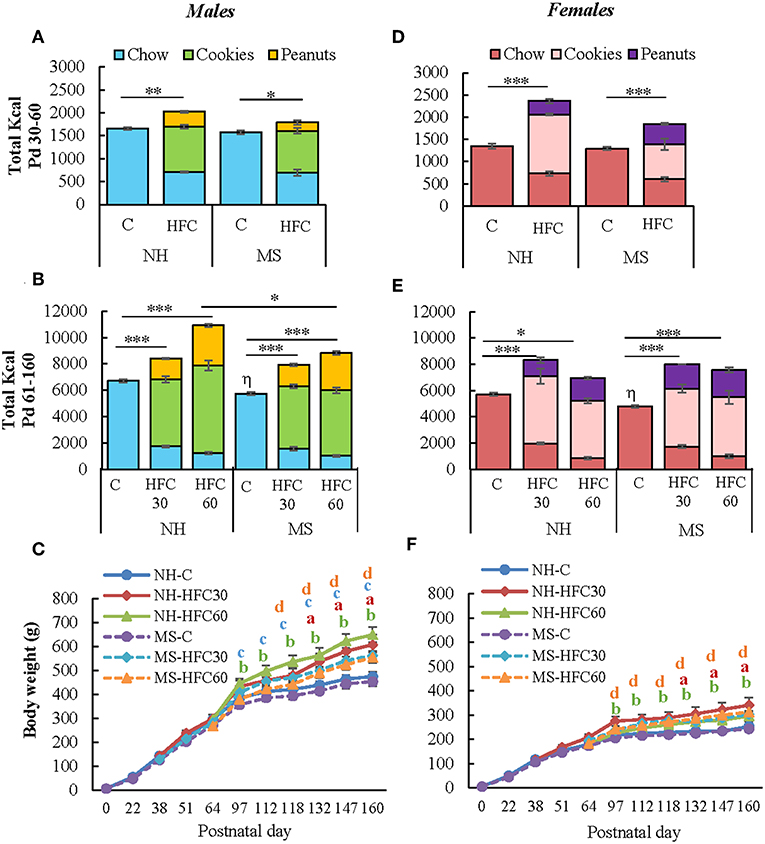
Figure 2. Effect of maternal separation and a high-fat/high-carbohydrate diet on food intake and body weight. Male and female pups were non-handled (NH) or separated from the dam (MS) daily from postnatal day 2–21 (Pd) for 3 h. NH and MS rats had free access to either chow (C) or a high-fat/high-carbohydrate diet (HFC: cookies, peanuts, and chow) from Pd30 (HFC30) or 60 (HFC60) until Pd160. Total kilocalorie intake during the juvenile period (A,D) and adulthood (B,E). (C,F) Body weight of male and female rats from birth until Pd160. Food intake was calculated by dividing the daily kilocalorie intake per cage by the number of rats in the cage (two rats/cage). Data are expressed as mean ± S.E.M. and were analyzed by a three-way ANOVA to determine effects of neonatal stress, diet, sex, and the interaction of these variables. If a significant main effect or interaction was found, ANOVA was followed by the Holm–Sidak multiple comparisons test; the level of significance was set at p < 0.05. *p < 0.05, **p < 0.01, ***p < 0.001, ηvs. NH-C, aNH-HFC30 vs. NH-C, bNH-HFC60 vs. NH-C, cMS-HFC30 vs. MS-C, dMS-HFC60 vs. MS-C. NH-C n = 6/sex, NH-HFC30 and NH-HFC60 n = 7/sex, MS-C n = 6/sex, MS-HFC30, and MS-HFC60 n = 7/sex.
Male and female rats fed an HFC diet, either from Pd30 or 60, ingested a similar proportion of macronutrients during Pd60–90 or Pd91–160, diminishing protein intake in favor of lipids (Supplementary Table 1). In HFC-fed animals, protein intake diminished, although to amounts and a proportion adequate for protein maintenance considering the quality of protein (51), and recommended intake of essential amino acids for growth maintenance was preserved (51) (Supplementary Table 1).
The slope of body weight gain was reduced after Pd97 in NH- and MS-chow-fed groups of both sexes while the reduction was less conspicuous in those receiving an HFC diet, especially in NH-HFC60 males compared to NH-chow and MS-HFC60 groups [diet effect F(2, 80) = 7.18, p = 0.007; sex effect F(1, 80) = 130.85, p < 0.0001; Figures 2C,F]. NH-chow and NH-HFC60 males consumed more kilocalories per day than females [diet effect F(1, 80) = 21.9, p < 0.0001; sex effect F(1, 80) = 65.4, p < 0.0001] but not relative to their body weight (Supplementary Table 1); MS-chow and MS-HFC males also consumed more kilocalories per day than females during Pd91–160 [diet and sex effect F(2, 80) = 4.8, p = 0.04; Supplementary Table 1]. Food efficiency was lower in female than in male groups [diet and sex effect F(2, 80) = 8.18, p = 0.003; Supplementary Table 1], as shown in adult rats (4).
Weights of gonadal fat [diet effect F(2, 80) = 55.52, p < 0.0001; diet and sex F(2, 80) = 10.98, p < 0.0001], retroperitoneal fat [diet effect F(2, 80) = 66.33, p < 0.0001; diet and sex effect F(2, 80) = 4.23, p = 0.018], and interscapular fat [diet effect F(2, 80) = 27.25, p < 0.0001] increased with consumption of an HFC diet, to a similar extent whether diet started at Pd30 or 60 in NH and MS males and females compared to chow-fed rats (Table 2); MS-HFC60 female rats showed a tendency of higher interscapular WAT weight than NH-HFC60 but did not achieve statistical significance (p = 0.06) (Table 2).
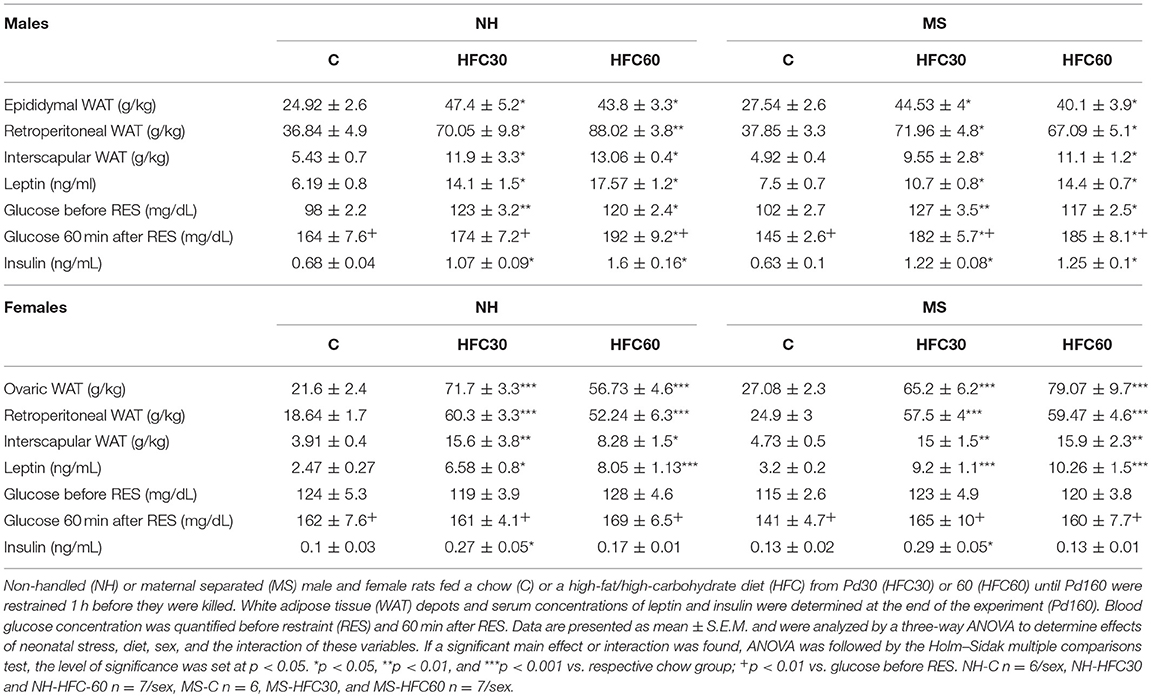
Table 2. Effect of maternal separation and 3–4 months of a high-fat/high-carbohydrate diet on metabolic parameters in acutely restrained rats (experiment 2).
Metabolic Parameters and Serum 17β-Estradiol
Serum leptin concentrations of HFC-fed NH and MS male and female rats were higher than those of NH-chow-fed rats [diet effect F(2, 80) = 42.82, p < 0.0001; Table 2]. Changes in serum insulin concentration followed a similar pattern to serum leptin in males; in contrast, in females, serum insulin concentration augmented only in NH-HFC30 and MS-HFC30 compared to chow and HFC60 groups [diet effect F(2, 80) = 10.22, p = 0.0001; diet and sex effect F(2, 80) = 5.33, p = 0.007; Table 2]. Basal glucose levels were not changed by MS in chow-fed animals, but levels were higher in HFC-fed NH and MS rats compared to chow-fed males, regardless of the age of access to the diet and were unchanged by an HFC diet in females [diet effect F(2, 80) = 12.03, p < 0.0001; sex effect F(1, 80) = 8.77, p = 0.004; Table 2]. In response to restraint, blood glucose levels increased in all chow and HFC groups in both sexes and were even higher in NH-HFC60, MS-HFC30, and MS-HFC60 males in comparison to chow-fed rats, with no differences between groups in females [diet effect F(2, 80) = 11.37, p < 0.0001; sex effect F(1, 80) = 11.46, p = 0.001; Table 2]. The concentration of 17β-estradiol was lower after 3–4 months of feeding an HFC diet to NH and MS female rats [diet effect F(2, 40) = 6.51, p = 0.003; Supplementary Figure 3B].
HPA Axis Parameters
After 1 h of restraint, serum corticosterone AUC was significantly higher in MS-chow than in NH-chow-fed male rats [MS effect F(1, 80) = 20.5, p < 0.0001; Figure 3C], but serum ACTH concentration and mRNA levels of Crh in the PVN were unchanged (Figures 3A,B). Ingestion of an HFC diet diminished the stress response only in NH males that consumed the diet from adulthood, but not of MS males, since PVN-Crh expression [MS effect F(1, 80) = 18.3, p < 0.0001; diet effect F(2, 80) = 4.38, p = 0.016; Figure 3A], serum concentration of ACTH [MS and sex effect F(1, 80) = 10.1, p < 0.002; Figure 3B], corticosterone at 30 min and corticosterone AUC were lower in NH-HFC60 than in chow-fed rats [MS effect F(1, 80) = 20.5, p < 0.0001; diet effect F(2, 80) = 17.59, p < 0.0001; MS and sex effect F(1, 80) = 15.4, p = 0.0002; Figures 3C,D]. Thus, MS impaired the inhibitory effect of an HFC diet on the restraint-induced activation of the HPA axis in male rats. Females responded differently; MS enhanced Crh expression in the PVN of chow-fed rats and was reduced in MS-HFC60 but not MS-HFC30 compared to chow-fed MS rats [sex effect F(1, 80) = 13.92, p = 0004; MS, diet and sex effect F(2, 80) = 17.45, p < 0.0001; Figure 3E]. No changes in serum ACTH concentration were found in females (Figure 3F), whereas serum corticosterone at 30 min and AUC was similar between NH- and MS-chow-fed groups and comparable lower in all groups fed an HFC diet regardless of neonatal stress or age of diet exposition [sex effect F(1, 80) = 6.03, p = 0.016; MS and sex effect F(1, 80) = 15.4, p = 0.0002; Figures 3G,H].
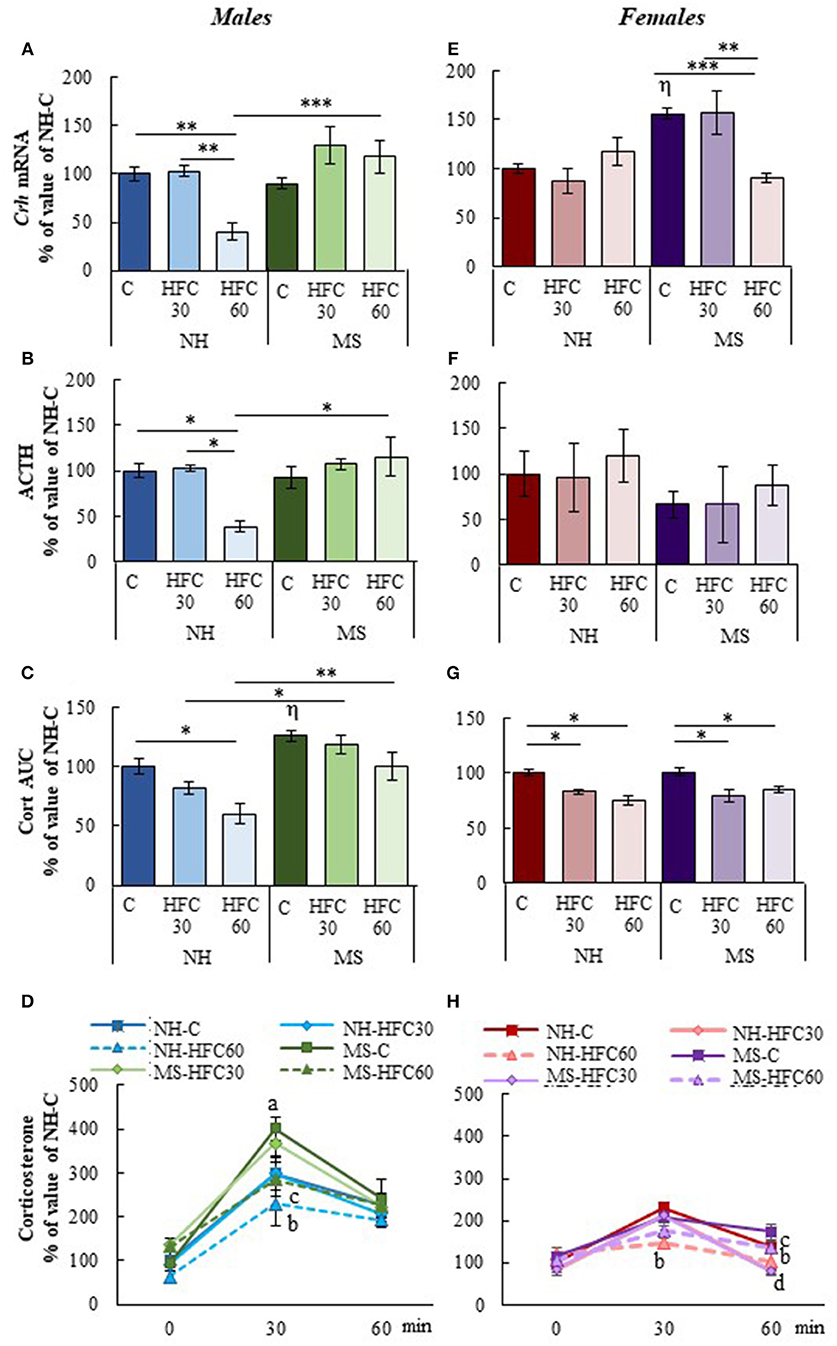
Figure 3. Effect of maternal separation and a high-fat/high-carbohydrate diet on HPA axis parameters in acutely restrained rats. Non-handled (NH) or maternal separated (MS) male and female rats fed a chow (C) or a high-fat/high-carbohydrate diet (HFC) from Pd30 (HFC30) or 60 (HFC60) until Pd160 were restrained 1 h before they were killed. (A,E) Expression levels of Crh in the paraventricular nucleus. (B,F) Serum concentration of ACTH. (C,G) Area under the curve (AUC) of serum corticosterone concentration response to restraint shown in (D,H); serum corticosterone values are presented in % of NH-chow groups to compare the extent of the stress response between males and females, as females present higher basal levels of serum corticosterone than male rats; absolute values are shown in Supplementary Figure 4. Data are mean ± S.E.M. expressed as % of values of NH-chow rats. Data were analyzed by a three-way ANOVA to determine effects of neonatal stress, diet, sex, and the interaction of these. If a significant main effect or interaction was found, ANOVA was followed by the Holm–Sidak multiple comparisons test, the level of significance was set at p < 0.05. *p < 0.05, **p < 0.01, ***p < 0.001, ηvs. NH-C, aMS vs. NH, bNH-HFC60 vs. NH-C, cMS-HFC60 vs. MS-C, dNH- and MS-HFC30 vs. NH-C and MS-C. NH-C n = 6/sex, NH-HFC30 and NH-HFC60 n = 7/sex, MS-C n = 6/sex, MS-HFC30 and MS-HFC60 n = 7/sex.
Mediobasal Hypothalamic Neuropeptides and HPT Axis Parameters
The PVN-Trh expression is regulated in part by peptides released from neurons located within the arcuate nucleus that project to TRH synthesizing neurons in the PVN of the hypothalamus, positively by POMC and negatively by NPY (22). MBH-Pomc expression in males was reduced by an HFC diet, with no effects of maternal separation or age of access to the HFC diet [diet effect F(2, 80) = 4.07, p = 0.022; sex effect F(1, 80) = 25.45, p < 0.0001; diet and sex effect F(2, 80) = 7.2, p = 0.001; Figure 4A], whereas no changes were found in female rats (Figure 4D). MBH-Npy expression was enhanced in both MS-chow male and female rats compared to NH-chow groups (Figures 4A,D) and reduced in MS-HFC60 males compared to MS-chow-fed rats [MS effect F(1, 80) = 22.2, p < 0.0001; sex effect F(1, 80) = 74.73, p < 0.0001; diet and sex effect F(2, 80) = 6.85, p = 0.002; Figure 4A].
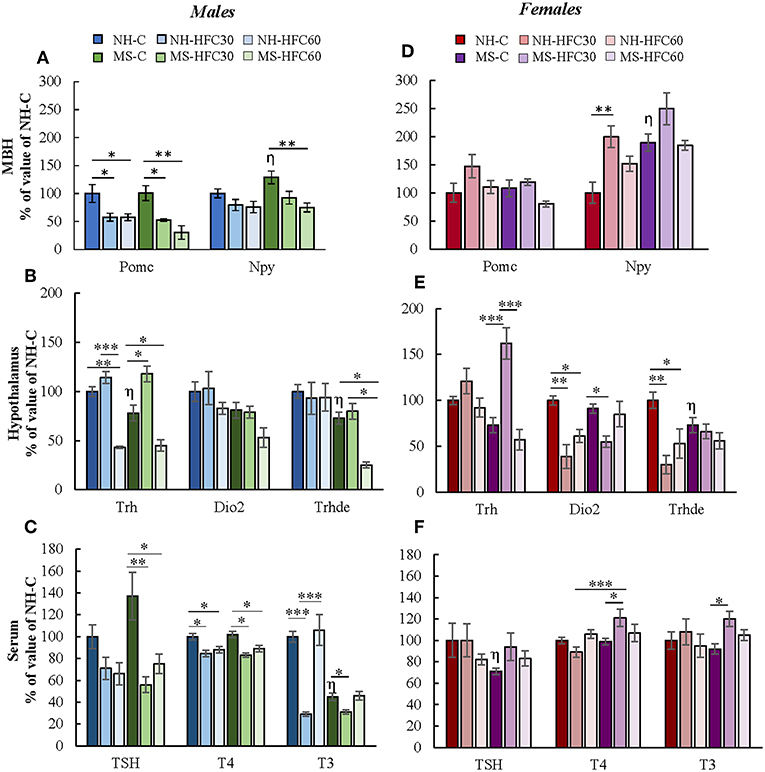
Figure 4. Effect of maternal separation, and a high-fat/high-carbohydrate diet on the expression of arcuate peptides and HPT axis parameters in acutely restrained rats. Non-handled (NH) or maternal separated (MS) male and female rats fed a chow (C) or a high-fat/high-carbohydrate diet (HFC) from Pd30 (HFC30) or 60 (HFC60) until Pd160 were restrained 1 h before they were killed. (A,D) Expression levels of pro-opiomelanocortin (Pomc) and neuropeptide Y (Npy) in the mediobasal hypothalamus (MBH). (B,E) Expression levels of Trh in the paraventricular nucleus and of Dio2 and Trhde in the MBH: (C,F) Serum concentrations of TSH, T4, and T3. Data are expressed as % of values of NH-chow illustrated as mean ± S.E.M. Data were analyzed by a three-way ANOVA to determine effects of neonatal stress, diet, sex, and the interaction of these variables. If a significant main effect or interaction was found, ANOVA was followed by the Holm–Sidak multiple comparisons test; the level of significance was set at p < 0.05. *p < 0.05, **p < 0.01, ***p < 0.001,ηvs. NH-C. NH-C n = 6/sex, NH-HFC30 and NH-HFC60 n = 7/sex, MS-C n = 6/sex, MS-HFC30 and MS-HFC60 n = 7/sex.
In adult male rats, acute restraint is reported to reduce PVN-Trh expression and serum TSH concentration (46), but serum T3 concentration only after a larger restraint span (52). Various parameters of the HPT axis in response to restraint stress were altered differently according to neonatal stress, sex, and age of access to the HFC diet. In male rats, MS-chow feeding regulated few HPT axis parameters, producing a small decrease in PVN-Trh mRNA levels [MS effect F(1, 80) = 4.75, p = 0.03; Figure 4B] and MBH-Trhde mRNA levels [MS effect F(1, 80) = 6.74, p = 0.01; Figure 4B] and lower serum T3 concentration than NH-chow males [MS effect F(1, 80) = 13.3, p = 0.0005; Figure 4C]. The HFC diet induced changes depending on age of diet initiation and neonatal stress; NH-HFC30 male rats had lower serum T4 [diet effect F(2, 80) = 4.83, p = 0.04; Figure 4C] and T3 concentration [diet effect F(2, 80) = 4.27, p = 0.01; Figure 4C] than the NH-chow fed group. Compared to MS-chow-fed animals, MS-HFC30 males had higher PVN-Trh expression [MS and diet effect F(2, 80) = 14.95, p < 0.0001; Figure 4B] and reduced serum concentration of TSH [diet effect F(2, 80) = 6.05, p = 0.004; MS, diet and sex effect F(2, 80) = 3.87, p = 0.026; Figure 4C], T4 [MS, diet and sex effect F(2, 80) = 3.73, p = 0.02; Figure 4C], and T3 [diet effect F(2, 80) = 4.27, p = 0.01; MS and diet effect F(2, 80) = 8.62, p = 0.0004; MS, diet and sex effect F(2, 80) = 3.87, p = 0.026; Figure 4C]. Different effects were observed in NH and MS male rats that had access to the HFC diet from Pd60; PVN-Trh expression and serum T4 concentration were lower in NH-HFC60 compared to NH-chow-fed animals (Figures 4B,C); in contrast, MS-HFC60 males had lower PVN-Trh and MBH-Trhde expression and serum TSH and T4 concentrations compared to MS-chow-fed rats (Figures 4B,C).
In female rats submitted to restraint, diet and neonatal stress effects were distinct from those observed in males. A decrease in mRNA levels of Trhde in MBH [sex effect F(1, 80) = 3.73, p = 0.05; MS and sex effect F(1, 80) = 10.57, p = 0.001; Figure 4E] and of serum TSH concentration [MS, diet and sex effect F(2, 80) = 3.87, p = 0.026; Figure 4F] was observed in MS compared to NH rats. In NH rats, access to an HFC diet, either starting at puberty or adulthood, reduced MBH-Trhde [sex effect F(1, 80) = 3.73, p = 0.05; MS and sex effect F(1, 80) = 10.57, p = 0.001; diet and sex effect F(2, 80) = 4.32, p = 0.04; Figure 4E] and Dio2 mRNA levels [diet effect F(2, 80) = 7.25, p = 0.001; sex effect F(1, 80) = 4.3, p = 0.01; diet and sex effect F(2, 80) = 6.36, p = 0.003; Figure 4E] compared to chow-fed females. In contrast, in MS rats, the HPT axis activity was altered in HFC30, but not in HFC60 rats compared to the chow-fed group: PVN-Trh expression was increased [sex effect F(1, 80) = 14.22, p = 0.0004; MS, diet and sex effect F(2, 80) = 5.46, p = 0.0065; Figure 4E], while MBH-Dio2 expression was reduced [MS and sex effect F(1, 80) = 6.17, p = 0.01; Figure 4E], and serum concentrations of T4 [MS, diet and sex effect F(2, 80) = 3.73, p = 0.02; Figure 4F] and T3 increased [MS and sex effect F(1, 8)0 = 26.51, p < 0.0001; MS, diet, and sex effect F(2, 80) = 3.24, p = 0.04; Figure 4F].
Discussion
Nutrition and stress play a major role in programming neuroendocrine systems that regulate energy homeostasis and body weight (53), but whether early-life stress contributes to metabolic alterations later in life is controversial with evidence for both improved or worsened metabolic disease risk (3, 10). Our results support published data indicating that compared to NH rats, MS reduces food intake in both sexes at adulthood (8) and enhances serum corticosterone and insulin concentrations, but only in males (54–56), suggesting a degree of insulin insensitivity as in Mela et al. (57). An HFC feeding increased serum insulin concentration in MS males but reduced that of corticosterone, consistent with previous data (5). MS-induced insulinemia is prevented by mechanical and tactical stimulation of the pups (56), supporting the role of neonatal stress shown to alter the pancreatic beta-cell function and insulin sensitivity in rats, with males being more sensitive than females (58, 59).
During adolescence, development of feeding behavior is vulnerable to insults, especially in females (37). Some reports describe that constant availability of palatable foods increases female susceptibility to develop obesity and metabolic syndrome later in life (37, 38), exacerbated by MS in adult female rats (9). Our results did not reproduce these findings; MS did not potentiate obesity in adult HFC-fed females, in agreement with other studies (5, 8). Differences could be due to the type and composition of the diet (10). It is interesting to notice the consistency in the proportion of macronutrient intake of NH and MS male and female rats with diet choice, even after 3–4 months of exposure to HFC foods; although their carbohydrate and fat intake was high, rats maintained the minimum protein intake required for growth maintenance (51). The high proportion of fats and carbohydrates consumed by the NH-HFC and MS-HFC groups most likely reinforced energy intake through enhanced hedonic feeding (60, 61).
MS and 1 Month of an HFC Diet Regulate the Basal Activity of the HPT Axis
Expression of MBH neuropeptides was evaluated due to their important role not only in food intake and energy balance but also in regulating the activity of PVN-TRH neurons (22). An HFC diet, but not MS, induced sex-specific changes in MBH-orexigenic and -anorexigenic peptides, with low Npy expression in NH-HFC males and elevated Pomc expression in NH-HFC females compared to chow-fed groups (49, 50). In contrast to previous reports of HPT axis activation with a high-fat saturated diet in male rodents (62–64), the HFC diet reduced serum TSH levels in NH males, coincident with increased expression of Trhde, supporting the lowering effect of a high-fat unsaturated diet on serum TSH concentration (65).
MS altered some parameters of the HPT axis activity only in males: expression of Trhde was higher in MS-HFC60 and MS-chow-fed rats than in NH animals, and serum TSH concentration decreased accordingly in MS-chow-fed rats as reported previously (32). NH females did show a slight activation of the HPT axis by an HFC diet, as serum T4 levels increased together with two activators of PVN-TRH neurons: serum leptin concentration and MBH-Pomc expression (22). Increased serum T4 could contribute to the enhanced expression of MBH-Trhde observed in NH-HFC females, as injection of this hormone increases the enzyme's expression in tanycytes (26). These changes, however, were not observed in MS females, in spite of having increased concentration of serum leptin.
MS and 3–4 Months of an HFC Diet Regulate Metabolic and Stress Parameters in Acutely Restrained Rats
Obesity and chronic hyperglycemia are risk factors in developing metabolic derangements (i.e., reduced insulin sensitivity) (66–68). Adolescent male rats appear to be more sensitive to a high-fat saturated diet-induced obesity than adults, producing more drastic metabolic effects when a high-fat diet is administered to young males compared to adult-fed rats (69, 70). Contrary to these reports, consumption of an HFC diet from either adolescence or adulthood induced a similar obese state and moderate basal hyperglycemia in MS and NH male rats, suggesting reduced insulin sensitivity (71) whether the HFC diet was offered starting at adolescence or adulthood, although this remains to be confirmed. In addition, the HFC diet heightened the hyperglycemic response to restraint stress in males, and consequently, serum insulin levels were higher in comparison to chow-fed groups, although serum insulin concentration was slightly higher in NH-HFC60 males. In contrast, the HFC diet enhanced serum insulin concentration in restrained females (independently of MS), if initiated at adolescence but not at adulthood, despite lack of differences in basal blood glucose concentration and similar stress-induced hyperglycemia, suggesting insulin resistance in NH-HFC30 and MS-HFC30 females (71). These results support the idea that puberty is a vulnerable period for females with unrestricted access to palatable foods and could increase their risk to develop metabolic alterations in adulthood (37). Nevertheless, our results show that an HFC diet, but not neonatal stress, is a major determinant of serum metabolic markers after acute stress in a sex-specific manner.
The restraint-induced serum corticosterone response was higher in MS than in NH males (6, 11, 72), and the HFC diet dampened it, but only in NH rats as reported (16, 36, 73, 74). A sex dimorphism was evidenced in the effects of early-life stress since MS males' response to stress was independent of diet in contrast to MS females, which had lower Crh expression in HFC60 and corticosterone concentration in HFC30 and HFC60 than chow-fed rats. Furthermore, although NH females have higher basal corticosterone concentration, the extent of their response to acute stress was lower than that in NH males, in contrast to some reports (34, 35). Serum concentration of 17β-estradiol could have contributed to the sex differences observed in the HPA axis activity in acutely restrained rats and to the effect of palatable foods to reduce restraint stress responses (34, 75), since serum 17β-estradiol levels were reduced by an HFC diet in NH and MS females, as reported previously (76, 77). These results suggest that an HFC diet alters the HPA axis activity differently in male than in female rats, with MS being a contributing factor in males but not in females, and highlights the complex interaction of diet, gonadal steroids, and stress.
MS and 3–4 Months of an HFC Diet Regulate HPT Axis Activity in Acutely Restrained Rats
Expression of arcuate peptides Pomc and Npy are regulated by the animal's energy state but also by stress (78–81). MBH-Npy expression was higher in MS-chow-fed restrained male and female rats than in NH-chow groups; neonatal stress per se does not affect Npy expression in male and female rats (5, 57, 82), but it increases hyperreactivity to restraint stress in adulthood (6, 11). As glucocorticoids (83) and restraint increase mRNA levels of Npy in the arcuate nucleus (albeit 24-h after initiated the stress) (78–80), the combination of MS and adult acute stress may have heightened Npy expression. Sex differences were observed in MBH neuropeptide expression in HFC-fed rats; Npy expression was higher in NH females that had access to the HFC from adolescence compared to NH-chow rats, but not in males, suggesting an altered diet-induced arcuate NPY activation and increased vulnerability of young females to chronic high-fat feeding (84). Restraint stress increases arcuate Pomc expression in adult male rats, although 4 h after stress (78, 79), its expression was inhibited in the MBH of NH-HFC30, MS-HFC30, and MSHFC-60 males compared to chow-fed rats, despite increased serum leptin levels, which is concordant with a hyperphagic behavior, a diet-induced obesity, and male rats' higher susceptibility to develop diet-induced leptin resistance compared to females (84–86). Thus, our results are in agreement with the reported impairment of the anorexigenic leptin–POMC system in males, whereas in females, there is an over-activation of the orexigenic NPY system in high-fat-fed rats (84).
Restraint and other forms of psychological stress rapidly inhibit HPT axis activity in adult male rats fed a standard diet and without a history of early-life stress (45, 46, 87). In contrast to the blunting effect of an HFC diet on restraint-induced Crh expression, compared to chow-fed rats, that of Trh was inhibited in NH-HFC60 and MS-HFC60 males as observed with Pomc expression. The restraint-induced decrease of serum TSH concentration in NH-chow males (46) was presumably blunted in MS-chow rats, most probably due to the lower expression of Trhde in MS in comparison to NH-chow males. These changes are not explained by altered serum TH or corticosterone concentration but could be due to lowered PVN-Trh expression since it has recently been reported that TRH may upregulate mRNA levels of Trhde and activity in tanycytes (88). Serum T4 concentration was lower in HFC- than in chow-fed males in both NH and MS groups, but did not coincide with changes in serum concentration of T3, which varied the most, as strong decreases were observed in MS-chow compared to NH-chow and in NH-HFC30 and MS-HFC30 groups compared to chow-fed animals.
Females responded differently to the acute stress situation dependent on diet initiation; NH-HFC30 and NH-HFC60 restrained females showed lower MBH expression of Trhde and Dio2 without changes in PVN-Trh, serum TSH, or T3 concentration compared to NH-chow rats; it is tempting to speculate that acute stress modulated by an HFC diet regulates the expression of these enzymes, although this remains to be elucidated. Parallel changes of Dio2 and Trhde expression are consistent with the proposal that T3 formed by D2 activates Trhde in MBH (26, 89). Even though Dio2 expression and activity are not modified under basal conditions by a hypercaloric diet, as observed in our first experiment (Table 1) and other studies (62, 63), the combination of obesity with acute stress may have altered MBH-Dio2 expression, since this enzyme is susceptible to various types of stimuli (47, 90–92). MS-HFC-fed females seemed to be less susceptible to stress than males, as reported previously (32). MS-HFC30 females had higher Trh expression and serum levels of T4 and T3 than MS-chow-fed rats, as well as decreased Dio2 expression; this decrease may explain why expression of Trh was increased in MS-HFC30 animals in spite of a small increment in serum T4, not being sufficient to exert negative feedback on TRH at the PVN level (22, 93, 94). These changes were not observed in MS females that were exposed to an HFC diet until adulthood (HFC60), supporting females' susceptibility during adolescence, particularly to a diet that makes them more resilient to restraint stress.
Conclusions
The HPT axis activity is regulated by energy-related cues (Figure 5A) as well as by stressors (Figure 5B). In this study, we show that MS did not exacerbate an obese phenotype or related metabolic alterations as hyperglycemia and insulinemia in either sex when rats have unrestricted access to an HFC diet composed mainly by unsaturated fatty acids. However, neonatal stress and/or long-term intake of an HFC diet altered the HPT axis activity in restraint-stressed rats in a sex-specific manner, and dependent on diet introduction at puberty or adulthood. Results are summarized in Figure 5B. Altogether, they emphasize the complex interaction of various environmental and physiological factors such as neonatal stress, nutrition, and sex in the regulation of the HPT axis activity, underlining the importance of studying sex differences, confirming that neuroendocrine responses to stress and nutrition are sexually dimorphic. Finally, our results are in line with clinical studies that report that different adult or adolescent psychopathologies due to childhood trauma are associated with alterations in thyroid function (29–31, 95, 96). The HPT axis is a dynamic and adaptive system; however, in this context, if adaptability is not met, psychological or metabolic pathologies may arise as observed in obese patients (97).
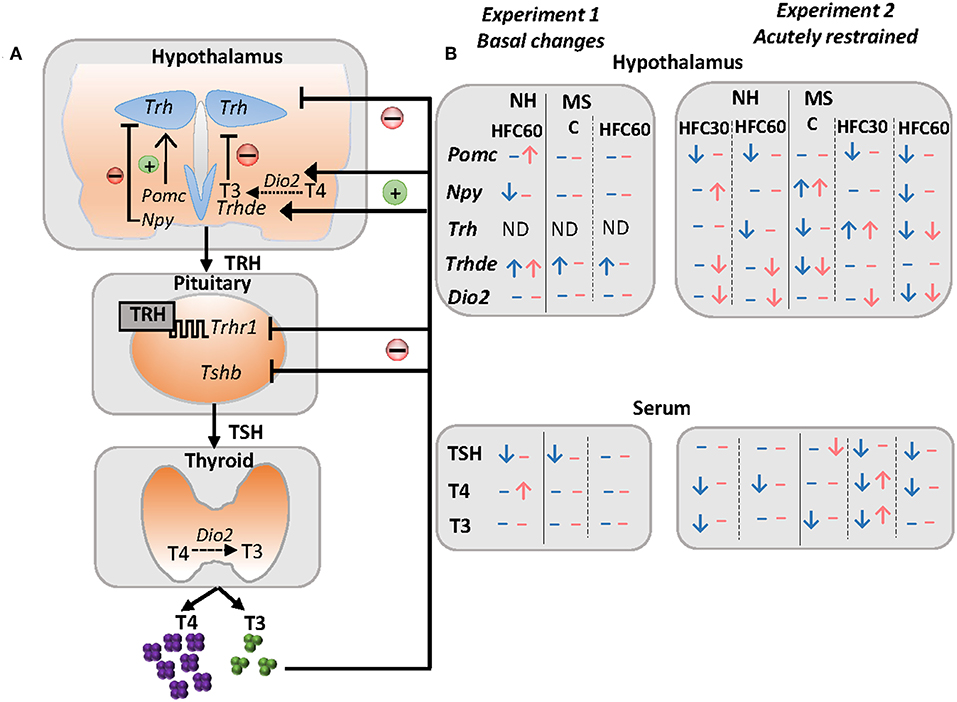
Figure 5. Schema of some peripheral inputs regulating the HPT axis and summary of changes of the HPT axis parameters and arcuate neuropeptides in response to maternal separation and 1–4 months of a high-fat/high-carbohydrate diet under basal conditions or after an acute stress in adult rats. (A) The HPT axis is controlled by thyrotropin-releasing hormone (TRH), synthesized in neurons of the hypothalamic paraventricular nucleus (PVN), and released at the median eminence near portal vessels that communicate with the anterior pituitary, where TRH activates its receptor (TRH-R1) increasing synthesis and release of thyrotropin (TSH) that controls the synthesis of thyroxine (T4) at the thyroid; T4 is converted to 3,3′,5-triiodo-L-thyronine (T3) by tissue deiodinases I or II (D1, D2). Released TRH at the median eminence may be degraded by the TRH-degrading ectoenzyme (TRH-DE) present in tanycytes before it travels to the pituitary, regulating the amount of TRH that reaches the thyrotrophs, and concomitantly, TSH and thyroid hormone synthesis and release, which in turn exert negative feedback at the pituitary and hypothalamus level. In addition, hypophysiotropic TRH neurons are negatively regulated by arcuate orexigenic neuropeptide Y (NPY), whereas anorexigenic neuropeptide pro-opiomelanocortin up-regulates Trh expression in the PVN. (B) Results summary of experiments 1 and 2. In experiment 1, we evaluated changes in maternal separated rats (MS) fed regular chow (C) vs. non-handled (NH), and the effect of 1 month of a high-fat/high-carbohydrate (HFC60) diet on NH and MS rats administered at Pd60. In experiment 2, we evaluated changes in NH and MS acutely restrained adult rats fed a C or an HFC diet starting at puberty (HFC30) or adulthood (HFC60) until postnatal day 160. NH and MS rats had free access to chow (C) or to an HFC diet from Pd30 or 60 until Pd160 and were restrained for 1 h before they were killed. Blue arrows and dashes represent males and pink denotes female rats; arrows indicate changes observed in NH-HFC30 and NH-HFC60 rats compared to the NH-C group; MS-C vs. NH-C; MS-HFC30 and MS-HFC60 compared to MS-C.
Data Availability
All datasets generated for this study are included in the manuscript and/or the Supplementary Files.
Ethics Statement
This study was carried out in accordance with the recommendations of the NIH guide for the care and use of laboratory animals and the Official Mexican Norm for production, care and use of laboratory animals NOM-062-ZOO-1999. The protocol and experiments were approved by the Bioethics Committee of Instituto de Biotecnología, UNAM, with authorization project number 311.
Author Contributions
LJ-H performed the maternal separation and HFC diet protocol of both experiments, ELISA analyses, data analyses, and writing and text editing of the manuscript. FR performed the RNA extraction of hypothalamic tissues and RT-PCRs. J-LC contributed to manuscript editing. PJ-B contributed in the experimental design, data analyses, manuscript writing, and editing.
Funding
This work was supported by DGAPA IA200417 (LJ-H) and CONACyT 180009 and 284883 (PJ-B).
Conflict of Interest Statement
The authors declare that the research was conducted in the absence of any commercial or financial relationships that could be construed as a potential conflict of interest.
Acknowledgments
We thank José Manuel Villa for his help in the care of animals during the experimental period, Q.F.B. Miguel Cisneros in radioimmunoassay analyses, and Mariana Gutiérrez-Mariscal, DSc, in assisting in the restraint stress protocol and sample collections. We also thank the synthesis and sequencing DNA unit of Instituto de Biotecnología, UNAM, for synthesizing primers used in these experiments and the computational unit for assisting in computer issues.
Supplementary Material
The Supplementary Material for this article can be found online at: https://www.frontiersin.org/articles/10.3389/fendo.2019.00445/full#supplementary-material
References
1. Hruby A, Hu FB. The epidemiology of obesity: a big picture. Pharmaco Economics. (2015) 33:673–89. doi: 10.1007/s40273-014-0243-x
2. Dallman MF. Stress-induced obesity and the emotional nervous system. Trends Endocrinol Metabol. (2010) 21:159–65. doi: 10.1016/j.tem.2009.10.004
3. Morris MJ, Beilharz JE, Maniam J, Reichelt AC, Westbrook RF. Why is obesity such a problem in the 21st century? The intersection of palatable food, cues and reward pathways, stress, and cognition. Neurosci Biobehav Rev. (2015) 58:36–45. doi: 10.1016/j.neubiorev.2014.12.002
4. Paternain L, Martisova E, Milagro FI, Ramírez MJ, Martínez JA, Campión J. Postnatal maternal separation modifies the response to an obesogenic diet in adulthood in rats. Dis Model Mech. (2012) 5:691–97. doi: 10.1242/dmm.009043
5. Maniam J, Morris MJ. Palatable cafeteria diet ameliorates anxiety and depression-like symptoms following an adverse early environment. Psychoneuroendocrinology. (2010) 35:717–28. doi: 10.1016/j.psyneuen.2009.10.013
6. Maniam J, Morris MJ. Voluntary exercise and palatable high-fat diet both improve behavioural profile and stress responses in male rats exposed to early life stress: role of hippocampus. Psychoneuroendocrinology. (2010) 35:1553–64. doi: 10.1016/j.psyneuen.2010.05.012
7. Miller AL, Lumeng JC. Pathways of association from stress to obesity in early childhood. Obesity. (2018) 26:1117–24. doi: 10.1002/oby.22155
8. Mela V, Llorente-Berzal Á, Díaz F, Argente J, Viveros M-P, Chowen JA. Maternal deprivation exacerbates the response to a high fat diet in a sexually dimorphic manner. PLoS ONE. (2012) 7:e48915. doi: 10.1371/journal.pone.0048915
9. Murphy MO, Herald JB, Wills CT, Unfried SG, Cohn DM, Loria AS. Postnatal treatment with metyrapone attenuates the effects of diet-induced obesity in female rats exposed to early-life stress. Am J Physiol Endocrinol Metabol. (2017) 312:E98–108. doi: 10.1152/ajpendo.00308.2016
10. Maniam J, Antoniadis C, Morris MJ. Early-life stress, HPA axis adaptation, and mechanisms contributing to later health outcomes. Front Endocrinol. (2014) 5:73. doi: 10.3389/fendo.2014.00073
11. Chen J, Evans AN, Liu Y, Honda M, Saavedra JM, Aguilera G. Maternal deprivation in rats is associated with corticotropin releasing hormone (CRH) promoter hypomethylation and enhances CRH transcriptional responses to stress in adulthood. J Neuroendocrinol. (2012) 24:1055–64. doi: 10.1016/j.biotechadv.2011.08.021.Secreted
12. Murgatroyd C, Patchev AV, Wu Y, Micale V, Bockmühl Y, Fischer D, et al. Dynamic DNA methylation programs persistent adverse effects of early-life stress. Nat. Neurosci. (2009) 12:1559–66. doi: 10.1038/nn.2436
13. Wu Y, Patchev AV, Daniel G, Almeida OFX, Spengler D. Early-life stress reduces DNA methylation of the Pomc gene in male mice. Endocrinology. (2014) 155:1751–62. doi: 10.1210/en.2013-1868
14. Todkar A, Granholm L, Aljumah M, Nilsson KW, Comasco E, Nylander I. HPA axis gene expression and DNA methylation profiles in rats exposed to early life stress, adult voluntary ethanol drinking and single housing. Front Mol Neurosci. (2016) 8:90. doi: 10.3389/fnmol.2015.00090
15. Foster MT, Warne JP, Ginsberg AB, Horneman HF, Pecoraro NC, Akana SF, et al. Palatable foods, stress, and energy stores sculpt corticotropin-releasing factor, adrenocorticotropin, and corticosterone concentrations after restraint. Endocrinology. (2009) 150:2325–33. doi: 10.1210/en.2008-1426
16. Tannenbaum BM, Brindley DN, Tannenbaum GS, Dallman MF, McArthur MD, Meaney MJ. High-fat feeding alters both basal and stress-induced hypothalamic–pituitary–adrenal activity in the rat. Am J Physiol. (1997) 273:E1168–77.
17. Macht M, Mueller J. Immediate effects of chocolate on experimentally induced mood states. Appetite. (2007) 49:667–74. doi: 10.1016/J.APPET.2007.05.004
18. Mullur R, Liu Y-Y, Brent GA. Thyroid hormone regulation of metabolism. Physiol Rev. (2014) 94:355–825. doi: 10.1152/physrev.00030.2013
19. Rabasa C, Dickson SL. Impact of stress on metabolism and energy balance. Curr Opinn Behav Sci. (2016) 9:71–7. doi: 10.1016/j.cobeha.2016.01.011
20. Silva JE. The thermogenic effect of thyroid hormone and its clinical implications. Ann Intern Med. (2003) 139:205–13. doi: 10.7326/0003-4819-139-3-200308050-00010
21. Klieverik LP, Coomans CP, Endert E, Sauerwein HP, Havekes LM, Voshol PJ, et al. Thyroid hormone effects on whole-body energy homeostasis and tissue-specific fatty acid uptake in vivo. Endocrinology. (2009) 150:5639–48. doi: 10.1210/en.2009-0297
22. Fekete C, Lechan RM. Central regulation of hypothalamic–pituitary–thyroid under physiological and pathophysiological conditions. Endocr Rev. (2014) 35:159–94. doi: 10.1210/er.2013-1087
23. Gereben B, McAninch EA, Ribeiro MO, Bianco AC. Scope and limitations of iodothyronine deiodinases in hypothyroidism. Nat Rev Endocrinol. (2015) 11:642–52. doi: 10.1038/nrendo.2015.155
24. Joseph-Bravo P, Jaimes-Hoy L, Charli JL. Regulation of TRH neurons and energy homeostasis-related signals under stress. J Endocrinol. (2015) 224:R139–59. doi: 10.1530/JOE-14-0593
25. Joseph-Bravo P, Jaimes-Hoy L, Uribe RM, Charli JL. TRH, the first hypophysiotropic releasing hormone isolated: control of the pituitary–thyroid axis. J. Endocrinology. (2015) 226:T85–100. doi: 10.1530/JOE-15-0124
26. Sánchez E, Vargas MA, Singru PS, Pascual I, Romero F, Fekete C, et al. Tanycyte pyroglutamyl peptidase II contributes to regulation of the hypothalamic–pituitary–thyroid axis through glial–axonal associations in the median eminence. Endocrinology. (2009) 150:2283–91. doi: 10.1210/en.2008-1643
27. Helmreich DL, Tylee D. Thyroid hormone regulation by stress and behavioral differences in adult male rats. Horm Behav. (2011) 60:284–91. doi: 10.1016/j.yhbeh.2011.06.003
28. Olivares EL, Silva-Almeida C, Pestana FM, Sonoda-Côrtes R, Araujo IG, Rodrigues NC, et al. Social stress-induced hypothyroidism is attenuated by antidepressant treatment in rats. Neuropharmacology. (2012) 62:446–56. doi: 10.1016/j.neuropharm.2011.08.035
29. Moog NK, Entringer S, Heim C, Wadhwa PD, Kathmann N, Buss C. Influence of maternal thyroid hormones during gestation on fetal brain development. Neuroscience. (2017) 342:68–100. doi: 10.1016/j.neuroscience.2015.09.070
30. Machado TD, Salum GA, Bosa VL, Goldani MZ, Meaney MJ, Agranonik M, et al. Early life trauma is associated with decreased peripheral levels of thyroid-hormone T3 in adolescents. Int J Dev Neurosci. (2015) 47:304–8. doi: 10.1016/J.IJDEVNEU.2015.10.005
31. Fischer S, Markert C, Strahler J, Doerr JM, Skoluda N, Kappert M, et al. Thyroid functioning and fatigue in women with functional somatic syndromes—Role of early life adversity. Front Physiol. (2018) 9:564. doi: 10.3389/fphys.2018.00564
32. Jaimes-Hoy L, Gutiérrez-Mariscal M, Vargas Y, Pérez-Maldonado A, Romero F, Sánchez-Jaramillo E, et al. Neonatal maternal separation alters, in a sex specific manner, the expression of TRH, of TRH-degrading ectoenzyme in the rat hypothalamus, and the response of the thyroid axis to starvation. Endocrinology. (2016) 157:3253–65. doi: 10.1210/en.2016-1239
33. Mauvais-Jarvis F. Sex differences in metabolic homeostasis, diabetes, and obesity. Biol Sex Differ. (2015) 6:14. doi: 10.1186/s13293-015-0033-y
34. Oyola MG, Handa RJ. Hypothalamic–pituitary–adrenal and hypothalamic–pituitary–gonadal axes: sex differences in regulation of stress responsivity. Stress. (2017) 20:476–94. doi: 10.1080/10253890.2017.1369523
35. Heck AL, Handa RJ. Sex differences in the hypothalamic–pituitary–adrenal axis' response to stress: an important role for gonadal hormones. Neuropsychopharmacology. (2019) 44:45–58. doi: 10.1038/s41386-018-0167-9
36. Pecoraro N, Reyes F, Gomez F, Bhargava A, Dallman MF. Chronic stress promotes palatable feeding, which reduces signs of stress: feedforward and feedback effects of chronic stress. Endocrinology. (2004) 145:3754–62. doi: 10.1210/en.2004-0305
37. Clawson RC, dela Cruz LN, Allen S, Wolgemuth T, Maner A, Dorsett A, et al. Continuous access to snacks from weaning onwards in female rats causes weight gain, insulin insensitivity, and sustained leptin resistance in adulthood. Physiol Behav. (2019) 201:165–74. doi: 10.1016/J.PHYSBEH.2018.11.026
38. Leibowitz SF, Akabayashi A, Alexander J, Karatayev O, Chang GQ. Puberty onset in female rats: relation to fat intake, ovarian steroids and the peptides, galanin and enkephalin, in the paraventricular and medial preoptic nuclei. J Neuroendocrinol. (2009) 21:538–49. doi: 10.1111/j.1365-2826.2009.01870.x.Puberty
39. Suarez Lopez M, Kizlansky A, Lopez L. Evaluación de la calidad de las proteínas en los alimentos calculando el escore de aminoácidos corrigedo por digestibilidad. Nutr Hosp. (2006) 21:47–51.
40. Venkatachalan M, Sathe SK. Chemical composition of selected edible nut seeds. J Agric Food Chem. (2006) 54:4705–14. doi: 10.1021/jf0606959
41. Schaafsma G. Criteria and Significance of Dietary Protein Sources in Humans. The protein digestibility-corrected amino acid score 1 (2000). pp. 1865–67.
42. Vahl TP, Ulrich-Lai YM, Ostrander MM, Dolgas CM, Elfers EE, Seeley RJ, et al. Comparative analysis of ACTH and corticosterone sampling methods in rats. Am J Physiol Endocrinol Metabol. (2005) 289:E823–8. doi: 10.1152/ajpendo.00122.2005
43. Sotelo-Rivera I, Jaimes-Hoy L, Cote-Velez A, Espinoza-Ayala C, Charli JL, Joseph-Bravo P. An acute injection of corticosterone increases thyrotrophin-releasing hormone expression in the paraventricular nucleus of the hypothalamus but interferes with the rapid hypothalamus pituitary thyroid axis response to cold in male rats. J Neuroendocrinol. (2014) 26:861–69. doi: 10.1111/jne.12224
44. Chomczynski P, Sacchi N. The single-step method of RNA isolation by acid guanidinium thiocyanate-phenol-chloroform extraction: twenty-something years on. Nat Protocols. (2006) 1:581–85. doi: 10.1038/nprot.2006.83
45. Aguilar-Valles A, Sánchez E, de Gortari P, García-Vazquez AI, Ramírez-Amaya V, Bermúdez-Rattoni F, et al. The expression of TRH, its receptors and degrading enzyme is differentially modulated in the rat limbic system during training in the Morris Water Maze. Neurochem Int. (2007) 50:404–17. doi: 10.1016/j.neuint.2006.09.009
46. Gutiérrez-Mariscal M, Sánchez E, García-Vázquez A, Rebolledo-Solleiro D, Charli JL, Joseph-Bravo P. Acute response of hypophysiotropic thyrotropin releasing hormone neurons and thyrotropin release to behavioral paradigms producing varying intensities of stress and physical activity. Regul Pept. (2012) 179:61–70. doi: 10.1016/j.regpep.2012.08.010
47. Uribe RM, Jaimes-Hoy L, Ramírez-Martínez C, García-Vázquez A, Romero F, Cisneros M, et al. Voluntary exercise adapts the hypothalamus–pituitary–thyroid axis in male rats. Endocrinology. (2014) 155:2020–30. doi: 10.1210/en.2013-1724
48. Bianco AC, Anderson G, Forrest D, Galton VA, Gereben B, Kim BW, et al. American Thyroid Association guide to investigating thyroid hormone economy and action in rodent and cell models. Thyroid. (2014) 24:88–168. doi: 10.1089/thy.2013.0109
49. Obici S, Feng Z, Morgan K, Stein D, Karkanias G, Rossetti L. Central administration of oleic acid inhibits glucose production and food intake. Diabetes. (2002) 51:271–75. doi: 10.2337/DIABETES.51.2.271
50. Schwinkendorf DR, Tsatsos NG, Gosnell BA, Mashek DG. Effects of central administration of distinct fatty acids on hypothalamic neuropeptide expression and energy metabolism. Int J Obes. (2011) 35:336–44. doi: 10.1038/ijo.2010.159
51. Subcommittee on Laboratory Animal Nutrition Committee on Animal Nutrition Board on Agriculture National Research Council. 1995. Nutrient Requirements of Laboratory Animals. Fourth Rev. Washington, DC: National Academies Press (1995). doi: 10.17226/4758
52. Bianco AC, Nunes MT, Hell NS, Maciel RMB. The role of glucocorticoids in the stress-induced reduction of extrathyroidal 3,5,3′-triiodothyronine generation in rats. Endocrinology. (1987) 120:1033–38. doi: 10.1210/endo-120-3-1033
53. Reynolds CM, Gray C, Li M, Segovia SA, Vickers MH. Early life nutrition and energy balance disorders in offspring in later life. Nutrients. (2015) 7:8090. doi: 10.3390/NU7095384
54. Vargas J, Junco M, Gomez C, Lajud N. Early life stress increases metabolic risk, HPA axis reactivity, and depressive-like behavior when combined with postweaning social isolation in rats. Edited by Cheryl M McCormick PLoS ONE. (2016) 11:e0162665. doi: 10.1371/journal.pone.0162665
55. Viveros MP, Llorente R, López-Gallardo M, Suarez J, Bermúdez-Silva F, De la Fuente M, et al. Sex-dependent alterations in response to maternal deprivation in rats. Psychoneuroendocrinology. (2009) 34(Suppl. 1):S217–26. doi: 10.1016/j.psyneuen.2009.05.015
56. Haley S, Neff K, Gulliver K, Gough G, Slater H, Lane RH, et al. Mechanical–tactile stimulation (MTS) intervention in a neonatal stress model alters adult adipose tissue deposition and prevents hyperinsulinemia in male rats. Early Human Dev. (2013) 89:387–92. doi: 10.1016/J.EARLHUMDEV.2012.12.005
57. Mela V, Díaz F, Vázquez MJ, Argente J, Tena-Sempere M, Viveros M-P, et al. Interaction between neonatal maternal deprivation and serum leptin levels on metabolism, pubertal development, and sexual behavior in male and female rats. Biol Sex Differ. (2016) 7:1–17. doi: 10.1186/s13293-015-0054-6
58. Gehrand AL, Hoeynck B, Jablonski M, Leonovicz C, Ye R, Scherer PE, et al. Sex differences in adult rat insulin and glucose responses to arginine: programming effects of neonatal separation, hypoxia, and hypothermia. Physiol Rep. (2016) 4:e12972. doi: 10.14814/phy2.12972
59. Raff H, Hoeynck B, Jablonski M, Leonovicz C, Phillips JM, Gehrand AL. Insulin sensitivity, leptin, adiponectin, resistin, and testosterone in adult male and female rats after maternal-neonatal separation and environmental stress. Am J Physiol Regul Integr Comp Physiol. (2018) 314:R12–21. doi: 10.1152/ajpregu.00271.2017
60. Leigh S-J, Morris MJ. The role of reward circuitry and food addiction in the obesity epidemic: an update. Biol Psychol. (2018) 131:31–42. doi: 10.1016/J.BIOPSYCHO.2016.12.013
61. Hoch T, Kreitz S, Gaffling S, Pischetsrieder M, Hess A. Fat/carbohydrate ratio but not energy density determines snack food intake and activates brain reward areas. Sci. Rep. (2015) 5:10041. doi: 10.1038/srep10041
62. Araujo RL, Andrade BM, Padrón AS, Gaidhu MP, Perry RLS, Carvalho DP, et al. High-fat diet increases thyrotropin and oxygen consumption without altering circulating 3,5,3′-triiodothyronine (T3) and thyroxine in rats: the role of iodothyronine deiodinases, reverse T3 production, and whole-body fat oxidation. Endocrinology. (2010) 151:3460–69. doi: 10.1210/en.2010-0026
63. Perello M, Çakir I, Cyr NE, Romero A, Stuart RC, Chiappini F, et al. Maintenance of the thyroid axis during diet-induced obesity in rodents is controlled at the central level. Am J Physiol Endocrinol Metabol. (2010) 299:E976–89. doi: 10.1152/ajpendo.00448.2010
64. Shao SS, Zhao YF, Song YF, Xu C, Yang JM, Xuan SM, et al. Dietary high-fat lard intake induces thyroid dysfunction and abnormal morphology in rats. Acta Pharmacologica Sinica. (2014) 35:1411–20. doi: 10.1038/aps.2014.82
65. Cano P, Jiménez-Ortega V, Larrad Á., Reyes Toso CF, Cardinali DP, Esquifino AI. Effect of a high-fat diet on 24-h pattern of circulating levels of prolactin, luteinizing hormone, testosterone, corticosterone, thyroid-stimulating hormone and glucose, and pineal melatonin content, in rats. Endocrine. (2008) 33:118–25. doi: 10.1007/s12020-008-9066-x
66. Chang J-S, You Y-H, Park S-Y, Kim J-W, Kim H-S, Yoon K-H, et al. Pattern of stress-induced hyperglycemia according to type of diabetes: a predator stress model. Diabetes Metab J. (2013) 37:475–83. doi: 10.4093/dmj.2013.37.6.475
67. Plummer MP, Finnis ME, Phillips LK, Kar P, Bihari S, Biradar V, et al. Stress induced hyperglycemia and the subsequent risk of type 2 diabetes in survivors of critical illness. PLoS ONE. (2016) 11:e0165923. doi: 10.1371/journal.pone.0165923
68. Beaudry JL, D'souza AM, Teich T, Tsushima R, Riddell MC. Exogenous glucocorticoids and a high-fat diet cause severe hyperglycemia and hyperinsulinemia and limit islet glucose responsiveness in young male Sprague–Dawley rats. Endocrinology. (2013) 154:3197–208. doi: 10.1210/en.2012-2114
69. Barella LF, De Oliveira JC, Branco RCS, Camargo RL, Gomes RM, Mendes FCV, et al. Early exposure to a high-fat diet has more drastic consequences on metabolism compared with exposure during adulthood in rats. Horm Metab Res. (2012) 44:458–64. doi: 10.1055/s-0032-1306300
70. Cheng HS, Ton SH, Phang SCW, Tan JBL, Abdul Kadir K. Increased susceptibility of post-weaning rats on high-fat diet to metabolic syndrome. J Adv Res. (2017) 8:743–52. doi: 10.1016/J.JARE.2017.10.002
71. Bowe JE, Franklin ZJ, Hauge-Evans AC, King AJ, Persaud SJ, Jones PM. Assessing glucose homeostasis in rodent models. J. Endocrinology. (2014) 222:13–25. doi: 10.1530/JOE-14-0182
72. Lippmann M, Bress A, Nemeroff CB, Plotsky PM, Monteggia LM. Long-term behavioural and molecular alterations associated with maternal separation in rats. Eur J Neurosci. (2007) 25:3091–98. doi: 10.1111/j.1460-9568.2007.05522.x
73. Dallman MF, Pecoraro N, Akana SF, La Fleur SE, Gomez F, Houshyar H, et al. Chronic stress and obesity: a new view of ‘comfort food’. Proc Natl Acad Sci USA. (2003) 100:11696–701. doi: 10.1073/pnas.1934666100
74. Kinzig KP, Hargrave SL, Honors MA. Binge-type eating attenuates corticosterone and hypophagic responses to restraint stress. Physiol Behav. (2008) 95:108–13. doi: 10.1016/J.PHYSBEH.2008.04.026
75. Egan AE, Thompson AMK, Buesing D, Fourman SM, Packard AEB, Terefe T, et al. Palatable food affects HPA axis responsivity and forebrain neurocircuitry in an estrous cycle-specific manner in female rats. Neuroscience. (2018) 384:224–40. doi: 10.1016/J.NEUROSCIENCE.2018.05.030
76. Balasubramanian P, Jagannathan L, Mahaley RE, Subramanian M, Gilbreath ET, MohanKumar PS, et al. High fat diet affects reproductive functions in female diet-induced obese and dietary resistant rats. J Neuroendocrinol. (2012) 24:748–55. doi: 10.1111/j.1365-2826.2011.02276.x
77. Lie MEK, Overgaard A, Mikkelsen JD. Effect of a postnatal high-fat diet exposure on puberty onset, estrous cycle regularity, and kisspeptin expression in female rats. Reprod Biol. (2013) 13:298–308. doi: 10.1016/J.REPBIO.2013.08.001
78. Larsen PJ, Mau SE. Effect of acute stress on the expression of hypothalamic messenger ribonucleic acids encoding the endogenous opioid precursors preproenkephalin A and proopiomelanocortin. Peptides. (1994) 15:783–90. doi: 10.1016/0196-9781(94)90030-2
79. Baubet V, Fèvre-Montange M, Gay N, Debilly G, Bobillier P, Cespuglio R. Effects of an acute immobilization stress upon proopiomelanocortin (POMC) MRNA levels in the mediobasal hypothalamus: a quantitative in situ hybridization study. Mol Brain Res. (1994) 26:163–68. doi: 10.1016/0169-328X(94)90087-6
80. Conrad CD, McEwen BS. Acute stress increases neuropeptide Y mRNA within the arcuate nucleus and hilus of the dentate gyrus. Brain Res Mol Brain Res. (2000) 79:102–9. doi: 10.1016/S0169-328X(00)00105-4
81. Makino S, Baker RA, Smith MA, Gold PW. Differential regulation of neuropeptide Y mRNA expression in the arcuate nucleus and locus coeruleus by stress and antidepressants. J Neuroendocrinol. (2000) 12:387–95. doi: 10.1046/j.1365-2826.2000.00451.x
82. Viveros MP, Llorente R, Díaz F, Romero-zerbo SY, Bermudez-silva FJ, Rodríguez F, et al. Maternal deprivation has sexually dimorphic long-term effects on hypothalamic cell-turnover, body weight and circulating hormone levels. Horm Behav. (2010) 58:808–19. doi: 10.1016/j.yhbeh.2010.08.003
83. Shimizu H, Arima H, Ozawa Y, Watanabe M, Banno R, Sugimura Y, et al. Glucocorticoids increase NPY gene expression in the arcuate nucleus by inhibiting MTOR signaling in rat hypothalamic organotypic cultures. Peptides. (2010) 31:145–49. doi: 10.1016/j.peptides.2009.09.036
84. Priego T, Sánchez J, Picó C, Palou A. Sex-associated differences in the leptin and ghrelin systems related with the induction of hyperphagia under high-fat diet exposure in rats. Horm Behav. (2009) 55:33–40. doi: 10.1016/j.yhbeh.2008.07.010
85. La Fleur SE, Van Rozen AJ, Luijendijk MCM, Groeneweg F, Adan RAH. A free-choice high-fat high-sugar diet induces changes in arcuate neuropeptide expression that support hyperphagia. Int J Obes. (2010) 34:537–46. doi: 10.1038/ijo.2009.257
86. Heuvel J. K. van den, Eggels L, Fliers E, Kalsbeek A, Adan RAH, la Fleur SE. Differential modulation of arcuate nucleus and mesolimbic gene expression levels by central leptin in rats on short-term high-fat high-sugar diet. PLoS ONE. (2014) 9:e87729. doi: 10.1371/JOURNAL.PONE.0087729
87. Cizza G, Brady LS, Esclapes M, Blackman MR. Age and gender influence basal and stress-modulated hypothalamic–pituitary–thyroidal function in Fischer 344/N rats. Neuroendocrinology. (1996) 64:440–48.
88. Müller-Fielitz H, Stahr M, Bernau M, Richter M, Abele S, Krajka V, et al. Tanycytes control the hormonal output of the hypothalamic–pituitary–thyroid axis. Nat Commun. (2017) 8:484. doi: 10.1038/s41467-017-00604-6
89. Marsili A, Sanchez E, Singru P, Harney JW, Zavacki AM, Lechan RM, et al. Thyroxine-induced expression of pyroglutamyl peptidase II and inhibition of TSH release precedes suppression of TRH MRNA and requires type 2 deiodinase. J. Endocrinology. (2011) 211:73–78. doi: 10.1530/JOE-11-0248
90. Diano S, Naftolin F, Goglia F, Horvath TL. Fasting-induced increase in type II iodothyronine deiodinase activity and messenger ribonucleic acid levels is not reversed by thyroxine in the rat hypothalamus. Endocrinology. (1998) 139:2879–84.
91. Fekete C, Sarkar S, Christoffolete MA, Emerson CH, Bianco AC, Lechan RM. Bacterial lipopolysaccharide (LPS)-induced type 2 iodothyronine deiodinase (D2) activation in the mediobasal hypothalamus (MBH) is independent of the LPS-induced fall in serum thyroid hormone levels. Brain Res. (2005) 1056:97–99. doi: 10.1016/j.brainres.2005.07.021
92. Lazcano I, Cabral A, Uribe RM, Jaimes-Hoy L, Perello M, Joseph-Bravo P, et al. Fasting enhances pyroglutamyl peptidase II activity in tanycytes of the mediobasal hypothalamus of male adult rats. Endocrinology. (2015) 156:2713–23. doi: 10.1210/en.2014-1885
93. Segerson T, Kauer J, Wolfe H, Mobtaker H, Wu P, Jackson I, et al. Thyroid hormone regulates TRH biosynthesis in the paraventricular nucleus of the rat hypothalamus. Science. (1987) 238:78–80. doi: 10.1126/science.3116669
94. Perello M, Friedman T, Paez-Espinosa V, Shen X, Stuart RC, Nillni EA. Thyroid hormones selectively regulate the posttranslational processing of prothyrotropin-releasing hormone in the paraventricular nucleus of the hypothalamus. Endocrinology. (2006) 147:2705–165. doi: 10.1210/en.2005-1609
95. Plaza A, Garcia-Esteve L, Ascaso C, Navarro P, Gelabert E, Halperin I, et al. Childhood sexual abuse and hypothalamus–pituitary–thyroid axis in postpartum major depression. J Affect Disord. (2010) 122:159–63. doi: 10.1016/J.JAD.2009.07.021
96. Sinai C, Hirvikoski T, Nordström A-L, Nordström P, Nilsonne A, Wilczek A, et al. Hypothalamic pituitary thyroid axis and exposure to interpersonal violence in childhood among women with borderline personality disorder. Eur J Psychotraumatol. (2014) 5:23911. doi: 10.3402/ejpt.v5.23911
Keywords: TRH, TRH-DE, thyroid hormones, maternal separation, stress, palatable diet, sex
Citation: Jaimes-Hoy L, Romero F, Charli J-L and Joseph-Bravo P (2019) Sex Dimorphic Responses of the Hypothalamus–Pituitary–Thyroid Axis to Maternal Separation and Palatable Diet. Front. Endocrinol. 10:445. doi: 10.3389/fendo.2019.00445
Received: 22 April 2019; Accepted: 20 June 2019;
Published: 11 July 2019.
Edited by:
Colin Brown, University of Otago, New ZealandReviewed by:
Julie A. Chowen, Niño Jesús University Children's Hospital, SpainPatricia Cristina Lisboa, Rio de Janeiro State University, Brazil
Copyright © 2019 Jaimes-Hoy, Romero, Charli and Joseph-Bravo. This is an open-access article distributed under the terms of the Creative Commons Attribution License (CC BY). The use, distribution or reproduction in other forums is permitted, provided the original author(s) and the copyright owner(s) are credited and that the original publication in this journal is cited, in accordance with accepted academic practice. No use, distribution or reproduction is permitted which does not comply with these terms.
*Correspondence: Lorraine Jaimes-Hoy, bGphaW1lc0BpYnQudW5hbS5teA==