- 1Children's Hospital of Soochow University, Suzhou, China
- 2Changzhou Maternity and Child Health Care Hospital affiliated to Nanjing Medical University, Changzhou, China
Fetal metabolic programming caused by the adverse intrauterine environment can induce metabolic syndrome in adult offspring. Adverse intrauterine environment introduces fetal long-term relatively irreversible changes in organs and metabolism, and thus causes fetal metabolic programming leading metabolic syndrome in adult offspring. Fetal metabolic programming of obesity and insulin resistance plays a key role in this process. The mechanism of fetal metabolic programming is still not very clear. It is suggested that epigenetic programming, also induced by the adverse intrauterine environment, is a critical underlying mechanism of fetal metabolic programming. Fetal epigenetic programming affects gene expression changes and cellular function through epigenetic modifications without DNA nucleotide sequence changes. Epigenetic modifications can be relatively stably retained and transmitted through mitosis and generations, and thereby induce the development of metabolic syndrome in adult offspring. This manuscript provides an overview of the critical role of epigenetic programming in fetal metabolic programming.
Metabolic syndrome is well-known as a syndrome involved in obesity, insulin resistance, impaired glucose tolerance/diabetes, disturbance of lipid metabolism, and cardiovascular complications. Metabolic syndrome includes at least three typical phenotypes as follow: (1) elevated abdominal obesity, (2) elevated fasting glucose, (3) elevated blood pressure, (4) elevated fasting triglycerides (TG), (5) reduced high-density lipoprotein cholesterol (HDL-C) (1). It is one of the most causes of morbidity and mortality in both the developed and developing countries.
Obesity and insulin resistance are key factors resulting metabolic syndrome (Figure 1). Prevalence of metabolic syndrome was thought to be mainly contributed by genetics under an unhealthy lifestyle in adult. However, genetics cannot explain all reasons causing metabolic syndrome. Nowadays, it is proposed that an adverse intrauterine environment can induce fetal metabolic programming of obesity and insulin resistance and further metabolic syndrome in adult offspring. Epigenetic programming, without DNA nucleotide sequence changes, is a critical underlying mechanism of fetal metabolic programming of metabolic syndrome in adult offspring (2–6). Intergenerational transmission of metabolic syndrome moves in cycles through fetal metabolic programming and fetal epigenetic programming as shown in Figure 2.
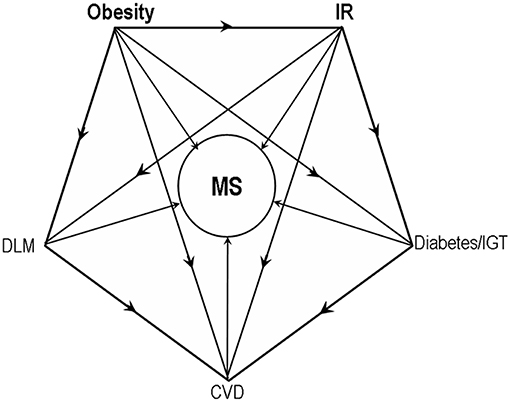
Figure 1. Obesity and insulin resistance as key factors resulting metabolic syndrome. IR, insulin resistance; IGT, Impaired glucose tolerance; DLM, disturbance of lipid metabolism; CVD, cardiovascular diseases.
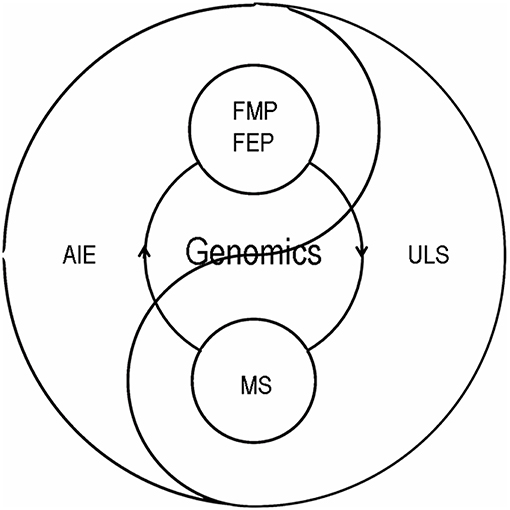
Figure 2. Intergenerational transmission of metabolic syndrome. Adverse intrauterine environment induced fetal epigenetic programming and fetal metabolic programming may introduce a circle of metabolic syndrome across generations without genetic changes. MS, metabolic syndrome; FMP, fetal metabolic programming; FEP, fetal epigenetic programming; AIE, adverse intrauterine environment; ULS, unhealthy lifestyle.
Fetal Metabolic Programming
The concept of fetal programming was firstly proposed by Barker and Hales in 1998, and later became the theory of developmental origins of health and disease (DOHaD) (7). This theory proposes that fetal metabolic programming caused by adverse intrauterine environment induces offspring metabolic diseases in adults. Fetal metabolic programming is involved in the relatively permanent changes of metabolic signaling pathways in growth and development, leading to relatively nonreversible effects on the structure and/or functional changes of fetal growth and development (8–10).
Although fetal metabolic programming is a relatively new concept, there have been various supporting evidence. It is proposed that the adverse intrauterine environment can cause intrauterine growth retardation (IGR), small for gestational age (SGA) and further metabolic syndrome risk in adult offspring. The intrauterine environment of malnutrition or nutrition deficiency can induce such fetal abnormal growth which is associated with metabolic syndrome in adult offspring (11). On the other hand, similar to intrauterine malnutrition, intrauterine over-nutrition exposure, such as in obesity or gestational diabetes, can also cause adverse fetal metabolic programming, leading to neonatal SGA or Large for gestational age (LGA) and further metabolic syndrome in adults (12–14).
Adverse intrauterine environment could introduce fetal long-term relatively irreversible changes in organs and metabolism, and thus may cause fetal metabolic programming of obesity and insulin resistance, leading metabolic syndrome in adult offspring.
Fetal Programming of Obesity
Human Studies
Offspring obesity in adults can be caused by intrauterine malnutrition exposure. This concept was first proposed in a study that the male offspring's obesity rate in adult was significantly associated with severe malnutrition in pregnant women in 40s Holland famine in the last century (15). Similar findings were observed in other countries (16). Also, adult obesity risk of low birth weight neonates was higher than normal weight neonates.
On the other hand, maternal over-nutrition or obesity is also highly correlated with offspring obesity (17–21). It is also proposed that pregnancy weight gain, independent of maternal obesity, is an independent factor causing neonatal adipose increase and subsequent obesity in children and adults (22). Maternal insulin resistance and glucose levels were reported to be important mediators of maternal obesity associated neonatal adipose increase (23). Otherwise, hyperglycemia in gestational diabetes could induce the risk of SGA or LGA.
Animal Model Studies
In the animal model studies, factors causing the prevalence of obesity in offspring can be elaborately studied by gestational interventions such as diet, drugs, surgery, and others. Animal model studies also offer an opportunity to disclose the detailed mechanism of fetal development programming.
In agreement with human studies, it was reported that offspring obesity could be induced by maternal malnutrition or over-nutrition during pregnancy, in studies in mice, rats, sheep, and non-human primates. Offspring obesity has been reported to be related to the significant expression changes of genes involved in abnormal metabolism, such as mitochondrial dysfunction (24) and significantly increased stress response (25, 26).
Rodent animal experiments have reported that fetal developmental programming caused by adverse intrauterine environment could induce hyperphagia leading offspring obesity in adults. Additional studies reported that the factors causing this offspring hyperphagia included hypothalamic programming of leptin resistance, increased neuropeptide Y and decreased proopiomelanocortin (27–30). It was reported that fetal development programming also introduced changes in offspring behavior patterns (sedentary and reduced physical activity), which contributed to the development of obesity in adults (31, 32).
Fetal Programming of Hyperinsulinemia and Insulin Resistance
Human Studies
The concept of fetal/neonatal hyperinsulinemia in pregnant women with gestational diabetes mellitus has been proposed a long time ago (33). Intrauterine hyperglycemia in gestational diabetes mellitus can induce fetal/neonatal hyperinsulinemia. Fetal/Neonatal hyperinsulinemia help to induce LGA/macrosomia. Also, fetal/neonatal hyperinsulinemia induced neonatal hypoglycemia in earlier hours after birth.
It is suggested that neonatal hyperinsulinemia may not only be a transient phenomenon caused by neonatal hyperglycemia but also reflect fetal or neonatal insulin resistance which would induce metabolic syndrome in adults. For one thing, fetal/neonatal hyperinsulinemia can be introduced by maternal obesity, higher weight gain, a high-fat diet or other factors (34–36). For another thing, it was reported that fetal/neonatal hyperinsulinemia could also be induced in glucose-well-controlled pregnant women with gestational diabetes (37, 38).
Fetal or neonatal insulin resistance was newly reported to be induced by gestational diabetes (37–41). Otherwise, fetal/neonatal insulin resistance can also be induced without intrauterine hyperglycemia exposure. For example, insulin resistance without hyperglycemia was reported in preterm neonates (42). Fetal insulin resistance was associated with maternal insulin resistance or insulin sensitivity in non-diabetic pregnancies (34, 43). Fetal/neonatal hyperinsulinemia could be induced in glucose-well-controlled pregnant women with gestational diabetes (37, 38). Fetal/neonatal insulin resistance was reported to be induced by maternal obesity (35, 44). Also, LGA was reported to be associated with decreased fetal Insulin sensitivity (39). Programming of fetal insulin resistance was reported to be induced by intrauterine abnormal activation of inflammation, adipokines and the endoplasmic reticulum (ER) stress (35).
Animal Model Studies
It has been reported by animal experiments that various maternal interventions could introduce hyperinsulinemia or insulin resistance in offspring.
Earlier studies proposed that fetal/neonatal hyperinsulinemia might be directly caused by fetal/neonatal simultaneous hyperglycemia. However, it was reported in some animal experiments that hyperinsulinemia can also be introduced without hyperglycemia in offspring. For example, fetal/neonatal hyperinsulinemia was reported to be induced by maternal hyperinsulinemia (45).
Further related animal experiments suggested that not only fetal/neonatal hyperinsulinemia but also fetal/neonatal insulin resistance can be induced by various maternal gestational interventions. It was reported that insulin resistance in offspring can be induced by gestational interventions such as high-fat diets (46–48), high-fructose diets (49), malnutrition or nutrition deficiency (50, 51), chronic intermittent hypoxia exposure (52, 53), abnormal levels of hormones (54–56), chemical or drug induction (57–59), and diabetic induction by genetic or non-genetic factors (60). Besides diabetes models, offspring hyperinsulinemia exists without maternal hyperglycemia in some other rodent animal experiments above (61, 62). Taken together, besides hyperglycemia, it is suggested that there is some other important potential mechanism causing fetal programming of insulin resistance.
Some adverse intrauterine environment also induces decreased number and/or function of pancreatic beta cells leading decreased glucose-stimulated insulin secretion and further abnormal glucose tolerance in adult offspring. For example, in animal experiments, the decreased number of pancreatic beta cells, introduced by maternal low-protein diet exposure, is caused by reduced activity of the mTOR signaling pathway (63). Besides, maternal low-protein diet exposure also introduces a decreased Pdx1 and Glut2 levels in offspring (64, 65). Both of them thereby may induce impaired glucose tolerance of offspring in adults.
It was recently reported that there was transgenerational programming of hyperinsulinemia, insulin resistance or glucose tolerance in various studies (4, 66, 67). For example, in several rodent studies, it has been reported that maternal high-fat diet induced obesity, insulin resistance, and glucose tolerance, not only in the first generation (F1) offspring but also in the second generation (F2) offspring (68, 69). In one of these studies above, it was observed that there were hyperinsulinemia and hyperleptinemia in the F2 generation, with normal body weight and glucose level (70). In another mouse model, it was reported that maternal obesogenic diet exposure induced hyperinsulinemia and expression alternation of some hepatic genes in the F2 offspring, interestingly without apparent phenotype in the F1 generation (71). Also, some fetal experiments in sheep reported similar results of transgenerational hyperinsulinemia, insulin resistance or glucose tolerance (72).
Fetal Epigenetic Programming
The critical mechanism underlying fetal metabolic programming induced by adverse intrauterine environment is still not very clear, while fetal metabolic programming has been reported to be contributed by changes in the developmental trajectories of tissues, stem cell numbers, neural circuits, and so on. Epigenetic programming, also induced by the adverse intrauterine environment, was reported to play an important role in the differentiation and development of embryonic, tissue, and stem cells (73–76). Could it be a critical underlying mechanism of fetal metabolic programming?
Epigenetics is the inheritance of variation in gene expression without changes in the DNA nucleotide sequence. Epigenetic modification can be relatively stably transmitted in the process of cell proliferation. The known mechanisms include DNA methylation, histone modification, genomic imprinting, chromatin remodeling, non-coding RNA, and so on. Such mechanism regulates gene expression at levels of pre-transcription (heterochromatinization), transcription (chromatin activation, binding of the cis-acting element, and the trans-acting factor) and post-transcription (protein translation).
DNA methylation and histone modification are known as key epigenetic regulation factors underlying the regulation of chromatin structure and gene transcription. DNA methylation adds methyl on 5-cytosine by DNA methyltransferase (Dnmt) at cis-acting element including promoter, enhancer, regulatory regions and sequence, response element, and so on. DNA methylation on such regions can cause DNA conformational changes, which may affect the binding of trans-acting factor and thereby regulate genes expression. Histone modification is known as the “histone code” including histone methylation, acetylation, phosphorylation, adenylation, ubiquitination, and so on. Histone modifications affect the structure and function of chromatin and thereby regulate the expression of the located genes.
Genomic imprinting, a parent-of-origin-specific silencing manner of certain gene alleles, is established (“imprinted”) in the germline (sperm or egg cells) mainly through marks of DNA methylation and histone modification. Non-coding RNA can be divided into long chain non-coding RNA (lncRNA) and short chain non-coding RNA (sncRNA), including miRNA, siRNA, piRNA, dsRNA, Xist RNA, Tsix RNA, telomere RNA, and so on. Micro RNA (miRNA) is the main executor of RNA interference effect on gene expression. LncRNA plays an important role in cell differentiation and individual development by multiple methods. It is involved in X chromosome silencing, genomic imprinting, chromatin modification, activation or inhibition of transcription, transportation in nuclear, and other unknown functions.
The concept of epigenetic programming was proposed as the potential key role of epigenetic modification in fetal metabolic programming (77, 78). Epigenetic modification without genetic changes is more easily induced by the adverse intrauterine environment during germ cells and fetal development, and relatively stably transmitted until adults (79). Epigenetic modification induced changes in cellular or tissue function can thereby be relatively stably transmitted earlier from fetuses until adults, and induce the development of metabolic syndrome in adult offspring. There is a sensitive “window” for epigenetic programming when germ cells, embryos, and fetuses are developing.
Epigenetic Programming of Obesity
Human Studies
Epigenetic programming of obesity was reported in human studies to be involved in abnormal fetal growth, adipocytokines, and other obesity-associated genes.
Global genome-wide epigenetic changes in offspring were reported to be associated with offspring obesity or an adverse intrauterine environment. Firstly, gestational diabetes induced genome-wide methylation changes in neonatal cord blood (80–82), adipose tissue or placenta (83). Genome-wide methylation changes were also reported existing in peripheral blood leukocytes of offspring at children (84, 85) or adult (86) exposed to the diabetic intrauterine environment. In addition, changes of global DNA methylation signatures were reported to exist in adult offspring under prenatal famine exposure in Dutch Hunger Winter and link to growth and metabolism (87). DNA methylation of metastable epialleles in neonatal lymphocytes and hair follicles was reported to be modulated by maternal nutrition at conception induced by Gambian Seasonal diet (88). Secondly, it was reported that maternal obesity was associated with genome-wide epigenetic changes in children or adult offspring (84, 89). Thirdly, DNA methylation changes in the placenta or the cord Blood were reported to be associated with abnormal birthweight or BMI in childhood (90, 91). Birth weight was also reported to be associated with methylation changes in children and adult offspring (92). What's more, circulating extracellular RNA ex- miR-122 was reported to be associated with regional adiposity in adults (93). Lastly, other adverse intrauterine environments, such as Arsenic or tobacco exposure, were also reported to be associated with genome-wide DNA methylation in cord blood (94, 95).
The insulin-like growth factor 2 (IGF2) and H19 genes are imprinted genes. Hypomethylation of the IGF2 gene and hypermethylation of H19 gene are associated with significantly higher expression of IGF2 (lower transcribed of H19), which induce neonatal adiposity through growth promoting effects. Such methylation changes of IGF2 and H19 in umbilical cord blood and placenta were reported to be associated with macrosomia exposed to GDM induced intrauterine hyperglycemia (96). Lower DNA methylation of the imprinted IGF2 gene was also reported in individuals who were prenatally exposed to famine during the Dutch Hunger Winter compared with their unexposed, same-sex siblings, supporting for the hypothesis that epigenetic changes induced by adverse intrauterine environment could persist throughout life (97). Changes in DNA methylation of the IGF2 gene was reported in cord blood from female offspring of pregnant women with an intervention of folic acid supplementation (98) or in blood from neonates of physically active pregnant women (99) than the control groups. In addition, in adult monozygotic twins with discordant birthweight, a significant positive association existed between birth weight and IGF1R DNA methylation differences in adult blood. It was suggested that intra-uterine growth differences were associated with methylation changes in the IGF1R gene in adulthood, independent of genetic effects (100). Higher methylation and lower expression of proopiomelanocortin (POMC) in cord blood was associated with lower birth weight and higher triglycerides in children blood, suggesting an early predictive marker of future metabolic syndrome (101).
Leptin could increase adipose tissue lipolysisl and hepatic β-oxidation of fatty acids, and inhibit hyperphagia. Hypermethylation and lower expression of the letpin gene in placenta and cord blood were reported to be associated with maternal impaired glucose tolerance (IGT) (102), GDM (103), or obesity (104). On the contrary, Lower DNA methylation and higher expression of the leptin gene were reported in the blood of obese children (105). Such inconsistency may be contributed by the differences between the intrauterine environment and the environment after birth. More interesting, the higher level of leptin cannot inhibit hyperphagia because of the leptin resistance. Adiponectin could increase GLUT4- mediated glucose uptake in adipocyte, resulting in adipogenesis and adipocyte lipid storage. Hypermethylation of adiponectin gene in newborn blood was reported in GDM group (106).
It was reported that abnormal birth weight was associated with cord blood or placental DNA methylation changes in energy homeostasis genes (107) and LINE-1 (91). It was reported that hypermethylations and lower expressions of the ATG2B, NKX6.1, and SLC13A5 genes (respectively, related to autophagy, beta-cell development and function, and lipid metabolism) and hypomethylation and higher expression of GPR120 gene (related to free fatty acid regulation) existed in the placenta and cord blood from SGA newborns (107). Abnormal birth weight was also reported to be related to placental methylation changes in WNT2 gene (108), Glucocorticoid receptor gene (109), fat mass- and obesity-associated genes (110), and Cardiometabolic Risk genes (111). In gestational diabetes, it was reported that hypermethylations and lower expressions of the lipoprotein lipase gene in the placenta are associated with offspring body composition at 5 years of age (112).
Studies in Animal Models or in vitro Models
Animal models and in vitro models disclosed more details of epigenetic programming of obesity in various tissues, which was involved in DNA or histone-modifying enzyme, abnormal fetal growth, hyperphagia, energy balance regulation, adipocyte differentiation/maturity, adipocytokines, hepatic metabolism, microRNA, and so on.
In liver, muscle and adipose tissues of offspring mice, global DNA methylation was reported to be induced by a maternal high-fat diet (113). A maternal high-fat diet induced epigenetically alters in fetal hepatic chromatin structure in primates by histone modifications and hence lends a molecular basis to the fetal origins of adult disease hypothesis (114). Non-human primate fetal hepatic multiple pathway dysregulation was reported to be associated with marked lipid accumulation, in response to maternal obesity induced by a high-fat, high-fructose diet prior to pregnancy, by unbiased gene and microRNA abundance analyses (115). DNA methylation levels in human in vitro maturation (IVM) oocytes were reported to be changed by High-glucose concentrations (116).
The fetal hepatic and placental expression of epigenetic machinery genes, particularly histone acetylation pathway genes, was reported to be sensitive to high-fat-diet-induced maternal obesity, leading to fetal growth restriction (FGR) in mice (117). More interesting, maternal weight loss appears beneficial to fetal growth, but those maternal obesity-induced effects were retained in offspring. Maternal high-fat diet increases fetal hepatic H3K14 acetylation with concomitant decreased SIRT1 expression and in vitro deacetylase activity in non-human primates (118). The protein deacetylase sirtuin-1 (SIRT1) was reported to be thought as a potential central co-ordinator of nutrient-led short and longer-term programming of tissue function (119).
Similar to human studies, the methylation changes in the imprinted gene IGF2/H19 was reported being an important factor involved in abnormal birth weight in various animal models (120–122). Decreased Histone modifications and higher expression of hepatic gene IGF1 were also reported in IUGR rat (123). In this study, the modulation of the rate of IUGR newborn catch-up growth may thus protect against IGF1 epigenetic modifications and, consequently, obesity and associated metabolic abnormalities.
Epigenetic programming of hyperphagia in offspring plays a role in the fetal programming of obesity. Leptin inhibits hyperphagia and stimulates energy expenditure through interactions with neuronal pathways in the hypothalamus. Leptin is higher in obese subjects. It was also reported that higher expression of the leptin gene, associated with higher histone or DNA methylation, was reported to be induced by maternal high-fat diet exposure (124) and to be involved in the transgenerational obesity (125). However, such obesogenic hyperphagia above is not suppressed because leptin resistance could be induced by epigenetic regulation of the leptin signaling circuit (126).
Epigenetic changes in the mouse adiponectin gene promoters were also reported to be associated with offspring obesity induced by paternal high-fat diet exposure (127). Lower adiponectin level and lower histone acetylation and higher histone methylation levels of the adiponectin gene promoter were reported in adipose tissues of mouse offspring with a high-fat-diet exposure during pregnancy (124).
Maternal periconceptional undernutrition induced decreased DNA methylation and increased histone acetylation was reported in pro-opiomelanocortin (POMC) (128) and the glucocorticoid receptor (GR) (129) in ovine fetal hypothalamus. Such epigenetic changes were associated with reduced POMC and increased GR levels, which potentially resulted in the altered energy balance regulation in the offspring. Another study reported that the hypermethylation and decreased expression of pro-opiomelanocortin gene caused hyperphagic obesity in mouse offspring under pregnant triclosan exposure (130). In the female hypothalamic paraventricular nucleus, it was reported that a perinatal high-fat diet environment induced decreased melanocortin 4 receptor (Mc4r) which was associated with food intake and energy balance (131). Such Mc4r downregulation may be contributed by histone acetylation of its promoter binding thyroid hormone receptor-β (TRβ), an inhibitor of Mc4r transcription, by using chromatin immunoprecipitation and bisulfite sequencing.
Epigenetic programming plays a role in adipocyte differentiation and maturity leading offspring obesity. DNA (cytosine-5) methyltransferase 3a (Dnmt3a) in 3T3-L1 preadipocytes was reported to be transcriptionally upregulated by Activator protein 2alpha (AP2alpha) through directly binding to its proximal promoter region, leading to increased promoter methylation of adipogenic genes which were required for granting preadipocyte the ability to differentiate (132). Also, it was reported that DNA methylation of obesity-related genes in adipose-derived stem cells in low birthweight may programme the mature adipocyte function which influences the risk of metabolic diseases (133). Decreased beige adipocyte number and mitochondrial respiration were reported to coincide with increased histone methyltransferase (G9a) and reduced FGF21 gene expression in subcutaneous adipose tissue of rats fed prenatal low protein and postnatal high-fat diets (134). Significantly enhanced hepatic acetylation of histone H3 surrounding fatty acid synthase (FAS) gene promoter was reported in adult male offspring under fetal and neonatal exposure to nicotine, associated with increased expression of fatty acid synthase induced augmented hepatic and circulating triglycerides (135). DNA and histone methylation modifications of Zfp423 gene were reported to be induced by maternal obesity and facilitate Zfp423 expression and enhance adipogenic differentiation in fetal mice adipose tissue (136).
A high-fat diet during pregnancy was reported to induce neonatal gender-specific hepatic fat accumulation by increased Phosphoenolpyruvate carboxykinase (PEPCK) expression and histone modification (137). These alterations were reported to persistent in adult offspring by further studies from the same team (135, 138). Developmental bisphenol A (BPA) exposure sex-specifically altered DNA methylation and histone marks (H3Ac, H4Ac, H3Me2K4, H3Me3K36), and decreased the binding of several transcription factors (Pol II, C/EBPβ, SREBP1) within the male Cpt1a gene, the key β-oxidation enzyme, which may exacerbate high-fat diet-induced hepatic steatosis (139). It was also reported that gestational high-fat diet programs hepatic phosphoenolpyruvate carboxykinase gene expression and histone modification in neonatal offspring rats (137) and female adult offspring rats (138). Another study reported that histone demethylase Plant Homeodomain Finger 2 (Phf2) was a new transcriptional co-activator of the transcription factor Carbohydrate Responsive Element (ChRE) Binding Protein, and acted as a molecular checkpoint to prevent NAFLD progression during obesity (140).
Changes of microRNA was reported to be induced by the adverse intrauterine environment and associated with offspring obesity (141). For example, maternal obesity-induced miRNA-let-7g downregulation in fetal skeletal muscle was reported to may enhance intramuscular adipogenesis during ovine fetal muscle development (142).
Epigenetic Programming of Insulin Resistance
Human Studies
Epigenetic programming of insulin resistance in human studies was reported to be involved in the epigenetic modifying enzyme, inflammatory/proinflammatory factors, insulin associated signaling, energy balance regulation, and microRNA.
Global genome-wide epigenetic changes were reported to be associated with insulin resistance. DNA methylation levels in neonatal blood were reported to be associated with insulin sensitivity in early childhood (90). In whole-blood of adults, potential DNA methylation biomarkers changes were reported to be strongly associated with obesity and insulin resistance (143). In visceral adipose tissue of obese subjects, it was reported that genome-wide DNA methylation pattern could differentiate insulin-resistant from insulin-sensitive (144). In the human liver of subjects with non-alcoholic steatohepatitis, methylation alterations are related to insulin resistance (145).
Changes of histone deacetylase 3 (HDAC3) in peripheral blood mononuclear cells is reported to be strongly related to insulin resistance and related proinflammatory mediators in patients with type 2 diabetes (143, 146).
Higher DNA methylation changes in neonatal adiponectin gene were reported in gestational diabetes group (103, 106). It was reported that IR was correlated negatively with DNA methylation of complement factor C3 gene, and correlated positively with the levels of complement factor C3, in adipose tissue from obese subjects (147). DNA methylation of the lymphocyte antigen 86 (LY86) gene was reported to be associated with obesity, and insulin resistance through a multi-stage cross-sectional study (148).
In white adipose tissue in insulin-resistant obese women, significantly differentially methylated sites were reported in IR-associated genes in pathways related to integrin cell surface interactions and insulin signaling pathway (149). In adult peripheral white blood cells, methylation changes of the endoplasmic reticulum (ER) genes were also reported to be associated with insulin resistance (150). DNA methylation of hypoxia-inducible factor 3-alpha (HIF3A) in both blood cells and subcutaneous adipose tissue (SAT) was reported to be associated with BMI and whole-body insulin sensitivity (151). Fatty acyl CoA reductase 2 (FAR2) gene methylation changes in peripheral blood cells were reported to be associated with atypical antipsychotic-induced insulin resistance and lipid metabolism (152). Higher pro-opiomelanocortin (POMC) methylation in cord blood was associated with hyperinsulinemia in children blood, suggesting an early predictive marker of future metabolic syndrome (101).
Expression of IR associated microRNA-15a and microRNA-15b was reported to be increased in skeletal muscle from adult offspring of women with diabetes in pregnancy (153). Circulating extracellular RNA ex-miR-122 was reported in another study to be associated with IR in children and adults (93).
Studies in Animal Models or in vitro Models
Epigenetic programming of insulin resistance was disclosed in more details involved in DNA or histone-modifying enzyme, adipocytokines, insulin associated signaling, lipid metabolism, microRNA, and so on.
Global changes of decreased hepatic DNA methylation and H4 acetylation or increased hepatic H3 acetylation was reported in offspring exposed to gestational germinated brown rice and its gamma-amino-butyric acid-rich, which could prevent high-fat-diet-induced insulin resistance in first generation rat offspring (154). Hepatic H3K14ac and H3K9me3 were reported to be significantly increased in WT and G4 (±) fetal and 5-week murine offspring with HFD exposure in utero (155).
Adipose DNA methyltransferase 3a (Dnmt3a) was reported to be a novel epigenetic mediator of insulin resistance in vitro and in vivo (156). In cultured mouse and human adipocytes, Dnmt3a was reported to be both necessary and sufficient to mediate diet-induced insulin resistance. Adipose-specific Dnmt3a knock-out mice were reported to be protected from diet-induced insulin resistance and glucose intolerance without accompanying changes in adiposity. Fgf21, a key negatively regulated Dnmt3a target gene, can rescue Dnmt3a-mediated insulin resistance. Consistent with this, DNA methylation at the FGF21 locus was elevated and negatively correlated with adipose FGF21 expression in human subjects with diabetes.
Epigenetic changes in the adiponectin gene play an important role in mediating insulin resistance. It was reported that insulin resistance was mediated by obesity-induced DNA hypermethylation of the adiponectin gene (157). It was reported in adipose tissue from HFD-induced obese mice that obesity-induced suppression of adiponectin expression was induced by the increased DNMT1 expression/enzymatic activity and related DNA methylation and chromatin remodeling in the adiponectin gene promoter, and could be stimulated by DNMT inhibitor with amelioration of obesity-induced glucose intolerance and insulin resistance in an adiponectin-dependent manner. However, little is known about how does DNMT1 selectively methylate adiponectin gene, which is a critical issue in fetal epigenetic programming. Another research reported that gestational sleep fragmentation induced insulin resistance and epigenetic changes in adiponectin gene in visceral white adipose tissue (VWAT) of male adult offspring mice (158). Reductions in 5-hydroxymethylcytosine and H3K4m3 and an increase in DNA 5-methylcytosine and H3K9m2 in the promoter and enhancer regions of adiponectin gene emerged in adipocytes from VWAT and correlated with adiponectin gene expression, accompanied with increased DNMT3a/b and reduced histone acetyltransferase activity and TET1/2/3 expression.
In liver and muscle tissues of rats, it was reported that offspring insulin resistance was programmed by IUGR with infantile overnutrition through higher methylation and lower expression of the peroxisome proliferator-activated receptor-gamma coactivator-1alpha (PGC-1α) gene (159). Increased methylation and decreased expression of glucose transporter 4 (Glut4) gene at the MYOD-binding site in gastrocnemius muscle of rat was reported to be induced by phthalate exposure in utero, associated with impaired insulin signaling (160). In a neonatal overfeeding mouse model, epigenetic programming of histone modifications at Monoacylglycerol O-acyltransferase 1 (Mogat1) locus was reported to link neonatal overnutrition with long-term hepatic insulin resistance and steatosis through increasing intracellular diacylglycerol content (161). In mice skeletal muscle, insulin sensitivity linked hypomethylation and increased expression of the Nr4a1 gene were reported to be programmed by the maternal high-fat diet and modulated by voluntary exercise in mice (162). Hepatic H3K14ac and H3K9me3 were reported to be significantly increased in WT and Glut4 (±) fetal and 5-week murine offspring with maternal high-fat-diet exposure in utero (155). Pathway analysis of ChIP-on-chip data revealed differential H3K14ac and H3K9me3 enrichment along pathways that regulate lipid metabolism, specifically in the promoter regions of Pparg, Ppara, Rxra, and Rora.
It was reported that elevated S-adenosylhomocysteine in adipocytes in vitro induced changes of DNA methylation, trimethylated histone H3-Lys27, and expression in genes involving glucose disposal and lipolysis pathway (163). In adipocytes differentiated from mesenchymal stem cells (MSCs) in Wharton's jelly of umbilical cord tissue of SGA neonates, it was reported that the increased acyl-coenzyme A synthetase 1 (ACSL1) (key roles in lipid metabolism) was highly associated with histone acetylation and could be a programmable mediator of insulin sensitivity and cellular lipid content (164).
Hepatic decreased miR-122 and increased miR-370 in mice offspring were reported to be induced by a maternal high-fat diet (165). Expression of insulin signaling associated hepatic miR-29b, miR-103, and miR-107 were reported to be increased in lambs with maternal obesity, and be ablated by maternal weight loss in the periconceptional period (166). Downregulation of IRS-1 in adipose tissue of offspring of obese mice was reported to be programmed cell-autonomously through increased miR-126 (167).
Epigenetic Programming of Pancreas Islet Beta Cell's Development
More and more studies revealed that DNA methylation, histone acetylation (168), non-coding RNA and other epigenetic modifications were involved in the development and differentiation of pancreatic beta cell (63, 169–171). When exposed to the adverse intrauterine environment, fetal development programming of pancreatic islet beta cells happens, leading decreased number and/or function of pancreatic beta cells.
Histone modifications and associated chromatin patterns were reported in regulatory elements of silent genes that are activated upon pancreas development fate choices (172). In this process, the histone acetyltransferase P300 and the histone methyltransferase Ezh2 had modulatory roles in the fate choice. This study revealed a functional “prepattern” of chromatin states within multipotent progenitors and potential targets to modulate cell fate induction in pancreas development. Another research reported Dynamics of genomic H3K27me3 domains during pancreatic endocrine specification and Ezh2 as a critical determinant of endocrine progenitor number (173).
During healthy neonatal life after birth in mice, DNA methylation, especially DNMT3A, was reported to play a role to direct the acquisition of pancreatic beta cell function of glucose-stimulated insulin secretion (GSIS) (174). In this study, the metabolic switch associated encoding hexokinase 1 (HK1) gene and lactate dehydrogenase A (LDHA) gene were reported to be bound and methylated by DNMT3A. Knockdown of these two genes restored the GSIS response in islets from animals with beta cell-specific Dnmt3a deletion. DNA methylation of Pdx-1(175, 176) was also reported playing an important role in the development and function of pancreatic beta cells (170, 177).
Increased miRNA-199a-3p and miRNA-342 were reported to cause the decrease of the mTOR signaling pathway, and thereby reduce the number of beta cells and insulin secretion (63, 178). Long noncoding RNA H19 was reported to play an important role in postnatal β-cell mass expansion in rats and contribute to the mechanisms compensating for insulin resistance in obesity (179). Low-moderate exercise in obese fathers was reported to induce partial restoration of pancreatic islet cell morphology and the expression of pancreatic microRNAs (let7d-5p, 194-5p) in male offspring (180).
Transgenerational Epigenetic Programming
Transgenerational epigenetic programming was considered a critical underlying mechanism of transgenerational inheritance caused by environmental interventions (2, 67, 181–183).
The concept of transgenerational epigenetic programming is proposed as epigenetic programming in germ cells. Twice genome-wide demethylation and further remethylation happen, respectively, during the development of germ cell and preimplantation embryo (6). The time of the development of germ cells and preimplantation embryos is considered to be important “windows” which is sensitive to epigenetic DNA methylation modification (184). Also, non-encoding RNA is involved in the transgenerational epigenetic programming (185, 185, 186). Such a concept means that the phenotype changes of F2 generation offspring after birth are caused by epigenetic programming in germ cells of F1 generation fetuses when exposed to the adverse intrauterine environment in the F0 generation.
Transgenerational epigenetic programming has been reported to be involved in a variety of phenotypes, including diabetes (4, 66), insulin resistance (3, 187), hypertension (188), reproduction (189), brain development (190, 191), and depression (185).
More and more researches supported the concept of transgenerational epigenetic programming of metabolic syndrome (4, 66, 183, 192). It was disclosed that transgenerational epigenetic programming could mediate the fetal programming of insulin resistance of insulin resistance, which was induced by high-fat diet, obesity or other interventions in F0 offspring (3, 68, 125). Transgenerational epigenetic programming was reported in adipose tissue development (5, 192). Also, transgenerational inheritance of obesity consistent with a leptin resistant was reported to be induced by transgenerational epigenetic programming of DNA methylome in adipose tissue (193–195).
Significant differential methylation changes in the promoter region of H19 were reported in a multigenerational model of intrauterine growth restriction (IUGR) (120).
Conclusion
Fetal epigenetic programming is a concept that the intrauterine environment induced fetal epigenetic modification and associated gene expression activity is relatively stably transmitted after birth until adults, and thereby decide the physiological phenotype in adult from fetal development. Strong evidence in this review indicates that fetal epigenetic programming could be a critical underlying mechanism of fetal metabolic programming, which induces the circle of metabolic syndrome across generations.
Epigenetic modification involved in fetal metabolic programming of metabolic syndrome has been discussed above and demonstrated in Table 1. Obesity and insulin resistance were the key factors resulting metabolic syndrome (Figure 1). In brief, the detailed mechanism includes genes involved in adipose tissue development (IGF2/H19, IGF1R, Zfp423, FGF21, FAS), hyperphagia and energy balance regulation (leptin, POMC, GR, WNT2, TRβ), lipid metabolism (leptin, adiponectin, lipoprotein lipase, Cpt1a, PEPCK, Cpt1a), insulin associated signaling (ER, HIF3A, FAR2, PGC-1α, Glut4, Nr4a1), adipocytokine and proinflammatory factors (adiponectin, C3, LY86), pancreas islet beta cell's development (HK1, LDHA, Pdx-1), and DNA or histone-modifying enzymes (Dnmt3a, HDAC3, SIRT1,Phf2, G9a, P300, Ezh2). These gene expression changes through fetal epigenetic modification, which was induced by the adverse intrauterine environment, and thereby contributes metabolic syndrome in adult.
There are still some unknowns and challenges in fetal epigenetic programming. Firstly, the molecular mechanism and regulation of epigenetic programming have not been fully disclosed. More key points and details are still required to be discovered. Secondly, results of related experiments sometimes could be disturbed by extra environmental disturbances not designed in experiments. Thirdly, epigenetic programming easily happens in susceptible “windows.” However, the threshold value of the adverse intrauterine environment is still not very clear in different types of diseases. Fourthly the size of epigenetic modifications is generally small in the whole epigenome. It may be caused by notable epigenetic modifications in fewer selectively targeted genes along with tiny epigenetic modifications in most non-targeted genes. The mechanism underlying the selective modifications is still needed to research. Beside of the selective epigenetic modifications around targeted gene region, the effects of more epigenetic modifications in other regions have not been well disclosed with their biological significance. Lastly, more importantly, there still are some limitations in the causality between epigenetic modifications and metabolic syndrome. There are a great deal of evidences of causality between epigenetic modifications and metabolic syndrome contributed by prospective clinical studies, animal and cell experiments in which the phenotypes of metabolic syndrome changed through the inhibition of DNA or histone modifying enzymes, and Chromatin immunoprecipitation assays identifying sequence-specific DNA binding protein which help to selectively modificate DNA or histone in a single gene's particular sequence. However, no molecular techniques have been invented to give direct logical evidence proving that metabolic syndrome could be induced or blocked by adding or erasing the epigenetic selectively modification in a particular sequence of a single gene. Here lies the most valuable breakthrough in epigenetic studies in future.
In spite of such unknowns, limitations, and challenges, the study of epigenetic programming in fetal metabolic programming has been developing rapidly, accompanied with more and more supporting evidence. It will not be surprising if future breakthroughs come soon in this field.
Author Contributions
ZZ and XL conceived and initiated this study. ZZ contributed to writing of the manuscript. FC participated in conception and writing of this manuscript.
Funding
This study was supported by grants from the Jiangsu Commission of Health (F201439,QNRC305) and the Changzhou Commission of Health (QN201724).
Conflict of Interest
The authors declare that the research was conducted in the absence of any commercial or financial relationships that could be construed as a potential conflict of interest.
Acknowledgments
Thanks to all those who have lent a hand in the course of writing this paper.
References
1. Grundy SM. Metabolic syndrome scientific statement by the American Heart Association and the National Heart, Lung, and Blood Institute. Arterioscler Thromb Vasc Biol. (2005) 25:2243–4. doi: 10.1161/01.ATV.0000189155.75833.c7
2. Miska EA, Ferguson-Smith AC. Transgenerational inheritance: models and mechanisms of non-DNA sequence-based inheritance. Science. (2016) 354:59–63. doi: 10.1126/science.aaf4945
3. Huypens P, Sass S, Wu M, Dyckhoff D, Tschop M, Theis F, et al. Epigenetic germline inheritance of diet-induced obesity and insulin resistance. Nat Genet. (2016) 48:497–9. doi: 10.1038/ng.3527
4. Barres R, Zierath JR. The role of diet and exercise in the transgenerational epigenetic landscape of T2DM. Nat Rev Endocrinol. (2016) 12:441–51. doi: 10.1038/nrendo.2016.87
5. Sun W, Dong H, Becker AS, Dapito DH, Modica S, Grandl G, et al. Cold-induced epigenetic programming of the sperm enhances brown adipose tissue activity in the offspring. Nat Med. (2018) 24:1776. doi: 10.1038/s41591-018-0162-z
6. Tang WW, Kobayashi T, Irie N, Dietmann S, Surani MA. Specification and epigenetic programming of the human germ line. Nat Rev Genet. (2016) 17:585–600. doi: 10.1038/nrg.2016.88
7. Barker DJ. In utero programming of chronic disease. Clin Sci. (1998) 95:115–28. doi: 10.1042/cs0950115
8. Bouchard L. Epigenetics and fetal metabolic programming: a call for integrated research on larger cohorts. Diabetes. (2013) 62:1026–8. doi: 10.2337/db12-1763
9. Gluckman PD, Hanson MA, Cooper C, Thornburg KL. Effect of in utero and early-life conditions on adult health and disease. N Engl J Med. (2008) 359:61–73. doi: 10.1056/NEJMra0708473
10. Houde AA, Ruchat SM, Allard C, Baillargeon JP, St Pierre J, Perron P, et al. LRP1B, BRD2 and CACNA1D: new candidate genes in fetal metabolic programming of newborns exposed to maternal hyperglycemia. Epigenomics-UK. (2015) 7:1111–22. doi: 10.2217/epi.15.72
11. Olmedo-Requena R, Amezcua-Prieto C, Luna-Del-Castillo JD, Lewis-Mikhael AM, Mozas-Moreno J, Bueno-Cavanillas A, et al. Association between low dairy intake during pregnancy and risk of small-for-gestational-age infants. Matern Child Health J. (2016) 20:1296–304. doi: 10.1007/s10995-016-1931-2
12. Santos MJ, Fernandes V, Marques O, Pereira ML. Effect of maternal body mass index and weight gain in women with gestational diabetes on the incidence of large-for-gestational-age infants. Diabetes Metab. (2016) 42:471–4. doi: 10.1016/j.diabet.2016.06.008
13. Wang D, Xu S, Chen H, Zhong L, Wang Z. The associations between triglyceride to high-density lipoprotein cholesterol ratios and the risks of gestational diabetes mellitus and large-for-gestational-age infant. Clin Endocrinol. (2015) 83:490–7. doi: 10.1111/cen.12742
14. Wang LF, Wang HJ, Ao D, Liu Z, Wang Y, Yang HX. Influence of pre-pregnancy obesity on the development of macrosomia and large for gestational age in women with or without gestational diabetes mellitus in Chinese population. J. Perinatol. (2015) 35:985–90. doi: 10.1038/jp.2015.119
15. Ravelli GP, Stein ZA, Susser MW. Obesity in young men after famine exposure in utero and early infancy. N Engl J Med. (1976) 295:349–53. doi: 10.1056/NEJM197608122950701
16. Wang J, Li Y, Han X, Liu B, Hu H, Wang F, et al. Exposure to the Chinese famine in childhood increases type 2 diabetes risk in adults. J. Nutr. (2016) 146:2289–95. doi: 10.3945/jn.116.234575
17. Hemond J, Robbins RB, Young PC. The effects of maternal obesity on neonates, infants, children, adolescents, and adults. Clin Obstet Gynecol. (2016) 59:216–27. doi: 10.1097/GRF.0000000000000179
18. Asmar J, Sibiude J. [Maternal obesity and pregnancy outcome]. Gynecol Obstet Fertil. (2015) 43:395–6. doi: 10.1016/j.gyobfe.2015.03.001
19. Santangeli L, Sattar N, Huda SS. Impact of maternal obesity on perinatal and childhood outcomes. Best Pract Res Clin Obstet Gynaecol. (2015) 29:438–48. doi: 10.1016/j.bpobgyn.2014.10.009
20. Gaudet L, Wen SW, Walker M. The combined effect of maternal obesity and fetal macrosomia on pregnancy outcomes. J Obstet Gynaecol Can. (2014) 36:776–84. doi: 10.1016/S1701-2163(15)30479-5
21. Dhana K, Haines J, Liu G, Zhang C, Wang X, Field AE, et al. Association between maternal adherence to healthy lifestyle practices and risk of obesity in offspring: results from two prospective cohort studies of mother-child pairs in the United States. BMJ. (2018) 362:k2486. doi: 10.1136/bmj.k2486
22. Lau EY, Liu J, Archer E, McDonald SM, Liu J. Maternal weight gain in pregnancy and risk of obesity among offspring: a systematic review. J Obes. (2014) 2014:524939. doi: 10.1155/2014/524939
23. Shapiro AL, Schmiege SJ, Brinton JT, Glueck D, Crume TL, Friedman JE, et al. Testing the fuel-mediated hypothesis: maternal insulin resistance and glucose mediate the association between maternal and neonatal adiposity, the Healthy Start study. Diabetologia. (2015) 58:937–41. doi: 10.1007/s00125-015-3505-z
24. Aiken CE, Tarry-Adkins JL, Penfold NC, Dearden L, Ozanne SE. Decreased ovarian reserve, dysregulation of mitochondrial biogenesis, and increased lipid peroxidation in female mouse offspring exposed to an obesogenic maternal diet. FASEB J. (2016) 30:1548–56. doi: 10.1096/fj.15-280800
25. Nguyen LT, Saad S, Tan Y, Pollock C, Chen H. Maternal high-fat diet induces metabolic stress response disorders in offspring hypothalamus. J Mol Endocrinol. (2017) 59:81–92. doi: 10.1530/JME-17-0056
26. Trzepizur W, Khalyfa A, Qiao Z, Popko B, Gozal D. Integrated stress response activation by sleep fragmentation during late gestation in mice leads to emergence of adverse metabolic phenotype in offspring. Metabolism. (2017) 69:188–98. doi: 10.1016/j.metabol.2017.01.026
27. Pedroso JA, Silveira MA, Lima LB, Furigo IC, Zampieri TT, Ramos-Lobo AM, et al. Changes in leptin signaling by SOCS3 modulate fasting-induced hyperphagia and weight regain in mice. Endocrinology. (2016) 157:3901–14. doi: 10.1210/en.2016-1038
28. Nakata M, Yamamoto S, Okada T, Gantulga D, Okano H, Ozawa K, et al. IL-10 gene transfer upregulates arcuate POMC and ameliorates hyperphagia, obesity and diabetes by substituting for leptin. Int J Obes. (2016) 40:425–33. doi: 10.1038/ijo.2015.201
29. Warne JP, Alemi F, Reed AS, Varonin JM, Chan H, Piper ML, et al. Retraction notice to: impairment of central leptin-mediated PI3K signaling manifested as hepatic steatosis independent of hyperphagia and obesity. Cell Metab. (2015) 21:648. doi: 10.1016/j.cmet.2015.03.008
30. de Lartigue G, Ronveaux CC, Raybould HE. Deletion of leptin signaling in vagal afferent neurons results in hyperphagia and obesity. Mol Metab. (2014) 3:595–607. doi: 10.1016/j.molmet.2014.06.003
31. Manti M, Fornes R, Qi X, Folmerz E, Linden HA, de Castro BT, et al. Maternal androgen excess and obesity induce sexually dimorphic anxiety-like behavior in the offspring. FASEB J. (2018) 32:4158–71. doi: 10.1096/fj.201701263RR
32. Kang SS, Kurti A, Fair DA, Fryer JD. Dietary intervention rescues maternal obesity induced behavior deficits and neuroinflammation in offspring. J Neuroinflammation. (2014) 11:156. doi: 10.1186/PREACCEPT-1047692381265837
33. Westgate JA, Lindsay RS, Beattie J, Pattison NS, Gamble G, Mildenhall LF, et al. Hyperinsulinemia in cord blood in mothers with type 2 diabetes and gestational diabetes mellitus in New Zealand. Diabetes Care. (2006) 29:1345–50. doi: 10.2337/dc05-1677
34. Walsh JM, Byrne J, Mahony RM, Foley ME, McAuliffe FM. Leptin, fetal growth and insulin resistance in non-diabetic pregnancies. Early Hum Dev. (2014) 90:271–4. doi: 10.1016/j.earlhumdev.2014.03.007
35. Westermeier F, Saez PJ, Villalobos-Labra R, Sobrevia L, Farias-Jofre M. Programming of fetal insulin resistance in pregnancies with maternal obesity by ER stress and inflammation. Biomed Res. Int. (2014) 2014:917672. doi: 10.1155/2014/917672
36. Pasquali R. Reproductive endocrinology: maternal and fetal insulin levels at birth in women with PCOS. Nat Rev Endocrinol. (2014) 10:382–4. doi: 10.1038/nrendo.2014.61
37. Wang Q, Huang R, Yu B, Cao F, Wang H, Zhang M, et al. Higher fetal insulin resistance in Chinese pregnant women with gestational diabetes mellitus and correlation with maternal insulin resistance. PLoS ONE. (2013) 8:e59845. doi: 10.1371/journal.pone.0059845
38. Luo ZC, Delvin E, Fraser WD, Audibert F, Deal CI, Julien P, et al. Maternal glucose tolerance in pregnancy affects fetal insulin sensitivity. Diabetes Care. (2010) 33:2055–61. doi: 10.2337/dc10-0819
39. Dong Y, Luo ZC, Nuyt AM, Audibert F, Wei SQ, Abenhaim HA, et al. Large-for-gestationalz-age may be associated with lower fetal insulin sensitivity and beta-cell function linked to leptin. J Clin Endocrinol Metab. (2018) 103:3837–44. doi: 10.1210/jc.2018-00917
40. Liu B, Xu Y, Liang JM, Voss C, Xiao HY, Sheng WY, et al. Intrauterine insulin resistance in fetuses of overweight mothers. J Obstet Gynaecol Res. (2013) 39:132–8. doi: 10.1111/j.1447-0756.2012.01919.x
41. Wang X, Yang T, Miao J, Liu H, Wu K, Guo J, et al. Correlation between maternal and fetal insulin resistance in pregnant women with gestational diabetes mellitus. Clin. Lab. (2018) 64:945–953. doi: 10.7754/Clin.Lab.2018.171214
42. Salis ER, Reith DM, Wheeler BJ, Broadbent RS, Medlicott NJ. Insulin resistance, glucagon-like peptide-1 and factors influencing glucose homeostasis in neonates. Arch Dis Child Fetal Neonatal Ed. (2017) 102:F162–6. doi: 10.1136/archdischild-2015-309174
43. Linder K, Schleger F, Ketterer C, Fritsche L, Kiefer-Schmidt I, Hennige A, et al. Maternal insulin sensitivity is associated with oral glucose-induced changes in fetal brain activity. Diabetologia. (2014) 57:1192–8. doi: 10.1007/s00125-014-3217-9
44. Sauder KA, Hockett CW, Ringham BM, Glueck DH, Dabelea D. Fetal overnutrition and offspring insulin resistance and beta-cell function: the Exploring Perinatal Outcomes among Children (EPOCH) study. Diabet Med. (2017) 34:1392–9. doi: 10.1111/dme.13417
45. Srinivasan M, Aalinkeel R, Song F, Mitrani P, Pandya JD, Strutt B, et al. Maternal hyperinsulinemia predisposes rat fetuses for hyperinsulinemia, and adult-onset obesity and maternal mild food restriction reverses this phenotype. Am J Physiol Endocrinol Metab. (2006) 290:E129–34. doi: 10.1152/ajpendo.00248.2005
46. Yokomizo H, Inoguchi T, Sonoda N, Sakaki Y, Maeda Y, Inoue T, et al. Maternal high-fat diet induces insulin resistance and deterioration of pancreatic beta-cell function in adult offspring with sex differences in mice. Am J Physiol Endocrinol Metab. (2014) 306:E1163–75. doi: 10.1152/ajpendo.00688.2013
47. Melo AM, Benatti RO, Ignacio-Souza LM, Okino C, Torsoni AS, Milanski M, et al. Hypothalamic endoplasmic reticulum stress and insulin resistance in offspring of mice dams fed high-fat diet during pregnancy and lactation. Metabolism. (2014) 63:682–92. doi: 10.1016/j.metabol.2014.02.002
48. Murabayashi N, Sugiyama T, Zhang L, Kamimoto Y, Umekawa T, Ma N, et al. Maternal high-fat diets cause insulin resistance through inflammatory changes in fetal adipose tissue. Eur J Obstet Gynecol Reprod Biol. (2013) 169:39–44. doi: 10.1016/j.ejogrb.2013.02.003
49. Saad AF, Dickerson J, Kechichian TB, Yin H, Gamble P, Salazar A, et al. High-fructose diet in pregnancy leads to fetal programming of hypertension, insulin resistance, and obesity in adult offspring. Am J Obstet Gynecol. (2016) 215:378.e1–6. doi: 10.1016/j.ajog.2016.03.038
50. Reynolds CM, Li M, Gray C, Vickers MH. Preweaning growth hormone treatment ameliorates adipose tissue insulin resistance and inflammation in adult male offspring following maternal undernutrition. Endocrinology. (2013) 154:2676–86. doi: 10.1210/en.2013-1146
51. Yates DT, Cadaret CN, Beede KA, Riley HE, Macko AR, Anderson MJ, et al. Intrauterine growth-restricted sheep fetuses exhibit smaller hindlimb muscle fibers and lower proportions of insulin-sensitive Type I fibers near term. Am J Physiol Regul Integr Comp Physiol. (2016) 310:R1020–9. doi: 10.1152/ajpregu.00528.2015
52. Iqbal W, Ciriello J. Effect of maternal chronic intermittent hypoxia during gestation on offspring growth in the rat. Am. J. Obstet. Gynecol. (2013) 209:564.e1–9. doi: 10.1016/j.ajog.2013.08.027
53. Raff H, Hoeynck B, Jablonski M, Leonovicz C, Phillips JM, Gehrand AL. Insulin sensitivity, leptin, adiponectin, resistin, and testosterone in adult male and female rats after maternal-neonatal separation and environmental stress. Am J Physiol Regul Integr Comp Physiol. (2018) 314:R12–21. doi: 10.1152/ajpregu.00271.2017
54. Pepe GJ, Maniu A, Aberdeen G, Lynch TJ, Kim SO, Nadler J, et al. Insulin resistance elicited in postpubertal primate offspring deprived of estrogen in utero. Endocrine. (2016) 54:788–97. doi: 10.1007/s12020-016-1145-9
55. Maniu A, Aberdeen GW, Lynch TJ, Nadler JL, Kim SO, Quon MJ, et al. Estrogen deprivation in primate pregnancy leads to insulin resistance in offspring. J. Endocrinol. (2016) 230:171–83. doi: 10.1530/JOE-15-0530
56. Xia T, Zhang X, Wang Y, Deng D. Effect of maternal hypothyroidism during pregnancy on insulin resistance, lipid accumulation, and mitochondrial dysfunction in skeletal muscle of fetal rats. Biosci Rep. (2018) 38:BSR20171731. doi: 10.1042/BSR20171731
57. Huang Y, Gao S, Chen J, Albrecht E, Zhao R, Yang X. Maternal butyrate supplementation induces insulin resistance associated with enhanced intramuscular fat deposition in the offspring. Oncotarget. (2017) 8:13073–84. doi: 10.18632/oncotarget.14375
58. Xie RH, Liu YJ, Retnakaran R, MacFarlane AJ, Hamilton J, Smith G, et al. Maternal folate status and obesity/insulin resistance in the offspring: a systematic review. Int J Obes. (2016) 40:1–9. doi: 10.1038/ijo.2015.189
59. Zhang H, Chu X, Huang Y, Li G, Wang Y, Li Y, et al. Maternal vitamin D deficiency during pregnancy results in insulin resistance in rat offspring, which is associated with inflammation and Ikappabalpha methylation. Diabetologia. (2014) 57:2165–72. doi: 10.1007/s00125-014-3316-7
60. Lorenzo C, Haffner SM, Stancakova A, Kuusisto J, Laakso M. Fasting and OGTT-derived measures of insulin resistance as compared with the euglycemic-hyperinsulinemic clamp in nondiabetic Finnish offspring of type 2 diabetic individuals. J Clin Endocrinol Metab. (2015) 100:544–50. doi: 10.1210/jc.2014-2299
61. Catalano PM, Presley L, Minium J, Hauguel-de MS. Fetuses of obese mothers develop insulin resistance in utero. Diabetes Care. (2009) 32:1076–80. doi: 10.2337/dc08-2077
62. Isganaitis E, Woo M, Ma H, Chen M, Kong W, Lytras A, et al. Developmental programming by maternal insulin resistance: hyperinsulinemia, glucose intolerance, and dysregulated lipid metabolism in male offspring of insulin-resistant mice. Diabetes. (2014) 63:688–700. doi: 10.2337/db13-0558
63. Alejandro EU, Gregg B, Wallen T, Kumusoglu D, Meister D, Chen A, et al. Maternal diet-induced microRNAs and mTOR underlie beta cell dysfunction in offspring. J Clin Invest. (2014) 124:4395–410. doi: 10.1172/JCI74237
64. Abuzgaia AM, Hardy DB, Arany E. Regulation of postnatal pancreatic Pdx1 and downstream target genes after gestational exposure to protein restriction in rats. Reproduction. (2015) 149:293–303. doi: 10.1530/REP-14-0245
65. Martin MA, Fernandez E, Pascual-Leone AM, Escriva F, Alvarez C. Protein calorie restriction has opposite effects on glucose metabolism and insulin gene expression in fetal and adult rat endocrine pancreas. Am J Physiol Endocrinol Metab. (2004) 286:E542–50. doi: 10.1152/ajpendo.00242.2003
66. Jimenez-Chillaron JC, Ramon-Krauel M, Ribo S, Diaz R. Transgenerational epigenetic inheritance of diabetes risk as a consequence of early nutritional imbalances. Proc Nutr Soc. (2016) 75:78–89. doi: 10.1017/S0029665115004231
67. Skinner MK. Endocrine disruptors in 2015: epigenetic transgenerational inheritance. Nat Rev Endocrinol. (2016) 12:68–70. doi: 10.1038/nrendo.2015.206
68. Hanafi MY, Saleh MM, Saad MI, Abdelkhalek TM, Kamel MA. Transgenerational effects of obesity and malnourishment on diabetes risk in F2 generation. Mol Cell Biochem. (2016) 412:269–80. doi: 10.1007/s11010-015-2633-6
69. Bays H, Scinta W. Adiposopathy and epigenetics: an introduction to obesity as a transgenerational disease. Curr Med Res Opin. (2015) 31:2059–69. doi: 10.1185/03007995.2015.1087983
70. Graus-Nunes F, Dalla CFE, Lannes WR, Da SMM, Mandarim-de-Lacerda CA, Souza-Mello V. Pregestational maternal obesity impairs endocrine pancreas in male F1 and F2 progeny. Nutrition. (2015) 31:380–7. doi: 10.1016/j.nut.2014.08.002
71. King V, Dakin RS, Liu L, Hadoke PW, Walker BR, Seckl JR, et al. Maternal obesity has little effect on the immediate offspring but impacts on the next generation. Endocrinology. (2013) 154:2514–24. doi: 10.1210/en.2013-1013
72. Shasa DR, Odhiambo JF, Long NM, Tuersunjiang N, Nathanielsz PW, Ford SP. Multigenerational impact of maternal overnutrition/obesity in the sheep on the neonatal leptin surge in granddaughters. Int J Obes. (2015) 39:695–701. doi: 10.1038/ijo.2014.190
73. Ziller MJ, Edri R, Yaffe Y, Donaghey J, Pop R, Mallard W, et al. Dissecting neural differentiation regulatory networks through epigenetic footprinting. Nature. (2015) 518:355–9. doi: 10.1038/nature13990
74. Atlasi Y, Stunnenberg HG. The interplay of epigenetic marks during stem cell differentiation and development. Nat Rev Genet. (2017) 18:643–58. doi: 10.1038/nrg.2017.57
75. Liang G, Zhang Y. Embryonic stem cell and induced pluripotent stem cell: an epigenetic perspective. Cell Res. (2013) 23:49–69. doi: 10.1038/cr.2012.175
76. Cheedipudi S, Genolet O, Dobreva G. Epigenetic inheritance of cell fates during embryonic development. Front Genet. (2014) 5:19. doi: 10.3389/fgene.2014.00019
77. Ruchat SM, Hivert MF, Bouchard L. Epigenetic programming of obesity and diabetes by in utero exposure to gestational diabetes mellitus. Nutr Rev. (2013) 71(Suppl 1):S88–94. doi: 10.1111/nure.12057
78. Pinney SE, Simmons RA. Metabolic programming, epigenetics, and gestational diabetes mellitus. Curr Diab Rep. (2012) 12:67–74. doi: 10.1007/s11892-011-0248-1
79. Jablonka E, Lamb MJ. The inheritance of acquired epigenetic variations. Int J Epidemiol. (2015) 44:1094–103. doi: 10.1093/ije/dyv020
80. Weng X, Liu F, Zhang H, Kan M, Wang T, Dong M, et al. Genome-wide DNA methylation profiling in infants born to gestational diabetes mellitus. Diabetes Res Clin Pract. (2018) 142:10–8. doi: 10.1016/j.diabres.2018.03.016
81. Haertle L, El Haji N, Dittrich M, Muller T, Nanda I, Lehnen H, et al. Epigenetic signatures of gestational diabetes mellitus on cord blood methylation. Clin Epigenetics. (2017) 9:28. doi: 10.1186/s13148-017-0329-3
82. Kang J, Lee CN, Li HY, Hsu KH, Lin SY. Genome-wide DNA methylation variation in maternal and cord blood of gestational diabetes population. Diabetes Res Clin Pract. (2017) 132:127–36. doi: 10.1016/j.diabres.2017.07.034
83. Reichetzeder C, Dwi PS, Pfab T, Slowinski T, Neuber C, Kleuser B, et al. Increased global placental DNA methylation levels are associated with gestational diabetes. Clin Epigenetics. (2016) 8:82. doi: 10.1186/s13148-016-0247-9
84. Hjort L, Martino D, Grunnet LG, Naeem H, Maksimovic J, Olsson AH, et al. Gestational diabetes and maternal obesity are associated with epigenome-wide methylation changes in children. JCI Insight. (2018) 3:e122572. doi: 10.1172/jci.insight.122572
85. Chen P, Piaggi P, Traurig M, Bogardus C, Knowler WC, Baier LJ, et al. Differential methylation of genes in individuals exposed to maternal diabetes in utero. Diabetologia. (2017) 60:645–55. doi: 10.1007/s00125-016-4203-1
86. Del RM, Ossowski V, Knowler WC, Bogardus C, Baier LJ, Hanson RL. Potential epigenetic dysregulation of genes associated with MODY and type 2 diabetes in humans exposed to a diabetic intrauterine environment: an analysis of genome-wide DNA methylation. Metabolism. (2014) 63:654–60. doi: 10.1016/j.metabol.2014.01.007
87. Tobi EW, Goeman JJ, Monajemi R, Gu H, Putter H, Zhang Y, et al. DNA methylation signatures link prenatal famine exposure to growth and metabolism. Nat. Commun. (2014) 5:5592. doi: 10.1038/ncomms6592
88. Dominguez-Salas P, Moore SE, Baker MS, Bergen AW, Cox SE, Dyer RA, et al. Maternal nutrition at conception modulates DNA methylation of human metastable epialleles. Nat Commun. (2014) 5:3746. doi: 10.1038/ncomms4746
89. Sharp GC, Salas LA, Monnereau C, Allard C, Yousefi P, Everson TM, et al. Maternal BMI at the start of pregnancy and offspring epigenome-wide DNA methylation: findings from the pregnancy and childhood epigenetics (PACE) consortium. Hum Mol Genet. (2017) 26:4067–85. doi: 10.1101/125492
90. van Dijk SJ, Peters TJ, Buckley M, Zhou J, Jones PA, Gibson RA, et al. DNA methylation in blood from neonatal screening cards and the association with BMI and insulin sensitivity in early childhood. Int J Obes. (2018) 42:28–35. doi: 10.1038/ijo.2017.228
91. Michels KB, Harris HR, Barault L. Birthweight, maternal weight trajectories and global DNA methylation of LINE-1 repetitive elements. PLoS ONE. (2011) 6:e25254. doi: 10.1371/journal.pone.0025254
92. Simpkin AJ, Suderman M, Gaunt TR, Lyttleton O, McArdle WL, Ring SM, et al. Longitudinal analysis of DNA methylation associated with birth weight and gestational age. Hum Mol Genet. (2015) 24:3752–63. doi: 10.1093/hmg/ddv119
93. Shah R, Murthy V, Pacold M, Danielson K, Tanriverdi K, Larson MG, et al. Extracellular RNAs are associated with insulin resistance and metabolic phenotypes. Diabetes Care. (2017) 40:546–53. doi: 10.2337/dc16-1354
94. Ivorra C, Fraga MF, Bayon GF, Fernandez AF, Garcia-Vicent C, Chaves FJ, et al. DNA methylation patterns in newborns exposed to tobacco in utero. J Transl Med. (2015) 13:25. doi: 10.1186/s12967-015-0384-5
95. Broberg K, Ahmed S, Engstrom K, Hossain MB, Jurkovic MS, Bottai M, et al. Arsenic exposure in early pregnancy alters genome-wide DNA methylation in cord blood, particularly in boys. J Dev Orig Health Dis. (2014) 5:288–98. doi: 10.1017/S2040174414000221
96. Su R, Wang C, Feng H, Lin L, Liu X, Wei Y, et al. Alteration in expression and methylation of IGF2/H19 in placenta and umbilical cord blood are associated with macrosomia exposed to intrauterine hyperglycemia. PLoS ONE. (2016) 11:e0148399. doi: 10.1371/journal.pone.0148399
97. Heijmans BT, Tobi EW, Stein AD, Putter H, Blauw GJ, Susser ES, et al. Persistent epigenetic differences associated with prenatal exposure to famine in humans. Proc Natl Acad Sci USA. (2008) 105:17046–9. doi: 10.1073/pnas.0806560105
98. Caffrey A, Irwin RE, McNulty H, Strain JJ, Lees-Murdock DJ, McNulty BA, et al. Gene-specific DNA methylation in newborns in response to folic acid supplementation during the second and third trimesters of pregnancy: epigenetic analysis from a randomized controlled trial. Am J Clin Nutr. (2018) 107:566–75. doi: 10.1093/ajcn/nqx069
99. Marshall MR, Paneth N, Gerlach JA, Mudd LM, Biery L, Ferguson DP, et al. Differential methylation of insulin-like growth factor 2 in offspring of physically active pregnant women. J Dev Orig Health Dis. (2018) 9:299–306. doi: 10.1017/S2040174417001106
100. Tsai PC, Van Dongen J, Tan Q, Willemsen G, Christiansen L, Boomsma DI, et al. DNA methylation changes in the IGF1R gene in birth weight discordant adult monozygotic twins. Twin Res Hum Genet. (2015) 18:635–46. doi: 10.1017/thg.2015.76
101. Yoo JY, Lee S, Lee HA, Park H, Park YJ, Ha EH, et al. Can proopiomelanocortin methylation be used as an early predictor of metabolic syndrome? Diabetes Care. (2014) 37:734–9. doi: 10.2337/dc13-1012
102. Bouchard L, Thibault S, Guay SP, Santure M, Monpetit A, St Pierre J, et al. Leptin gene epigenetic adaptation to impaired glucose metabolism during pregnancy. Diabetes Care. (2010) 33:2436–41. doi: 10.2337/dc10-1024
103. Lesseur C, Armstrong DA, Paquette AG, Li Z, Padbury JF, Marsit CJ. Maternal obesity and gestational diabetes are associated with placental leptin DNA methylation. Am J Obstet Gynecol. (2014) 211:654.e1–9. doi: 10.1016/j.ajog.2014.06.037
104. Nogues P, Dos SE, Jammes H, Berveiller P, Arnould L, Vialard F, et al. Maternal obesity influences expression and DNA methylation of the adiponectin and leptin systems in human third-trimester placenta. Clin Epigenetics. (2019) 11:20. doi: 10.1186/s13148-019-0612-6
105. Garcia-Cardona MC, Huang F, Garcia-Vivas JM, Lopez-Camarillo C, Del RNB, Navarro OE, et al. DNA methylation of leptin and adiponectin promoters in children is reduced by the combined presence of obesity and insulin resistance. Int J Obes. (2014) 38:1457–65. doi: 10.1038/ijo.2014.30
106. Ott R, Stupin JH, Melchior K, Schellong K, Ziska T, Dudenhausen JW, et al. Alterations of adiponectin gene expression and DNA methylation in adipose tissues and blood cells are associated with gestational diabetes and neonatal outcome. Clin Epigenetics. (2018) 10:131. doi: 10.1186/s13148-018-0567-z
107. Diaz M, Garcia C, Sebastiani G, de Zegher F, Lopez-Bermejo A, Ibanez L. Placental and cord blood methylation of genes involved in energy homeostasis: association with fetal growth and neonatal body composition. Diabetes. (2017) 66:779–84. doi: 10.2337/db16-0776
108. Ferreira JC, Choufani S, Grafodatskaya D, Butcher DT, Zhao C, Chitayat D, et al. WNT2 promoter methylation in human placenta is associated with low birthweight percentile in the neonate. Epigenetics-US. (2011) 6:440–9. doi: 10.4161/epi.6.4.14554
109. Filiberto AC, Maccani MA, Koestler D, Wilhelm-Benartzi C, Avissar-Whiting M, Banister CE, et al. Birthweight is associated with DNA promoter methylation of the glucocorticoid receptor in human placenta. Epigenetics-US. (2011) 6:566–72. doi: 10.4161/epi.6.5.15236
110. Liu ZW, Zhang JT, Cai QY, Zhang HX, Wang YH, Yan HT, et al. Birth weight is associated with placental fat mass- and obesity-associated gene expression and promoter methylation in a Chinese population. J Matern Fetal Neonatal Med. (2016) 29:106–11. doi: 10.3109/14767058.2014.987749
111. Chen PY, Chu A, Liao WW, Rubbi L, Janzen C, Hsu FM, et al. Prenatal growth patterns and birthweight are associated with differential DNA methylation and gene expression of cardiometabolic risk genes in human placentas: a discovery-based approach. Reprod Sci. (2018) 25:523–39. doi: 10.1177/1933719117716779
112. Gagne-Ouellet V, Houde AA, Guay SP, Perron P, Gaudet D, Guerin R, et al. Placental lipoprotein lipase DNA methylation alterations are associated with gestational diabetes and body composition at 5 years of age. Epigenetics-US. (2017) 12:616–25. doi: 10.1080/15592294.2017.1322254
113. Attig L, Vige A, Gabory A, Karimi M, Beauger A, Gross MS, et al. Dietary alleviation of maternal obesity and diabetes: increased resistance to diet-induced obesity transcriptional and epigenetic signatures. PLoS ONE. (2013) 8:e66816. doi: 10.1371/journal.pone.0066816
114. Aagaard-Tillery KM, Grove K, Bishop J, Ke X, Fu Q, McKnight R, et al. Developmental origins of disease and determinants of chromatin structure: maternal diet modifies the primate fetal epigenome. J Mol Endocrinol. (2008) 41:91–102. doi: 10.1677/JME-08-0025
115. Puppala S, Li C, Glenn JP, Saxena R, Gawrieh S, Quinn A, et al. Primate fetal hepatic responses to maternal obesity: epigenetic signalling pathways and lipid accumulation. J Physiol. (2018) 596:5823–37. doi: 10.1113/JP275422
116. Wang Q, Tang SB, Song XB, Deng TF, Zhang TT, Yin S, et al. High-glucose concentrations change DNA methylation levels in human IVM oocytes. Hum Reprod. (2018) 33:474–81. doi: 10.1093/humrep/dey006
117. Panchenko PE, Voisin S, Jouin M, Jouneau L, Prezelin A, Lecoutre S, et al. Expression of epigenetic machinery genes is sensitive to maternal obesity and weight loss in relation to fetal growth in mice. Clin Epigenetics. (2016) 8:22. doi: 10.1186/s13148-016-0188-3
118. Suter MA, Chen A, Burdine MS, Choudhury M, Harris RA, Lane RH, et al. A maternal high-fat diet modulates fetal SIRT1 histone and protein deacetylase activity in nonhuman primates. FASEB J. (2012) 26:5106–14. doi: 10.1096/fj.12-212878
119. Holness MJ, Caton PW, Sugden MC. Acute and long-term nutrient-led modifications of gene expression: potential role of SIRT1 as a central co-ordinator of short and longer-term programming of tissue function. Nutrition. (2010) 26:491–501. doi: 10.1016/j.nut.2009.09.012
120. Gonzalez-Rodriguez P, Cantu J, O'Neil D, Seferovic MD, Goodspeed DM, Suter MA, et al. Alterations in expression of imprinted genes from the H19/IGF2 loci in a multigenerational model of intrauterine growth restriction (IUGR). Am J Obstet Gynecol. (2016) 214:625.e1–11. doi: 10.1016/j.ajog.2016.01.194
121. Ding GL, Wang FF, Shu J, Tian S, Jiang Y, Zhang D, et al. Transgenerational glucose intolerance with Igf2/H19 epigenetic alterations in mouse islet induced by intrauterine hyperglycemia. Diabetes. (2012) 61:1133–42. doi: 10.2337/db11-1314
122. Shao WJ, Tao LY, Gao C, Xie JY, Zhao RQ. Alterations in methylation and expression levels of imprinted genes H19 and Igf2 in the fetuses of diabetic mice. Comp Med. (2008) 58:341–6.
123. Tosh DN, Fu Q, Callaway CW, McKnight RA, McMillen IC, Ross MG, et al. Epigenetics of programmed obesity: alteration in IUGR rat hepatic IGF1 mRNA expression and histone structure in rapid vs. delayed postnatal catch-up growth. Am J Physiol Gastrointest Liver Physiol. (2010) 299:G1023–9. doi: 10.1152/ajpgi.00052.2010
124. Masuyama H, Hiramatsu Y. Effects of a high-fat diet exposure in utero on the metabolic syndrome-like phenomenon in mouse offspring through epigenetic changes in adipocytokine gene expression. Endocrinology. (2012) 153:2823–30. doi: 10.1210/en.2011-2161
125. Masuyama H, Mitsui T, Nobumoto E, Hiramatsu Y. The effects of high-fat diet exposure in utero on the obesogenic and diabetogenic traits through epigenetic changes in adiponectin and leptin gene expression for multiple generations in female mice. Endocrinology. (2015) 156:2482–91. doi: 10.1210/en.2014-2020
126. Crujeiras AB, Carreira MC, Cabia B, Andrade S, Amil M, Casanueva FF. Leptin resistance in obesity: an epigenetic landscape. Life Sci. (2015) 140:57–63. doi: 10.1016/j.lfs.2015.05.003
127. Masuyama H, Mitsui T, Eguchi T, Tamada S, Hiramatsu Y. The effects of paternal high-fat diet exposure on offspring metabolism with epigenetic changes in the mouse adiponectin and leptin gene promoters. Am J Physiol Endocrinol Metab. (2016) 311:E236–45. doi: 10.1152/ajpendo.00095.2016
128. Begum G, Stevens A, Smith EB, Connor K, Challis JR, Bloomfield F, et al. Epigenetic changes in fetal hypothalamic energy regulating pathways are associated with maternal undernutrition and twinning. FASEB J. (2012) 26:1694–703. doi: 10.1096/fj.11-198762
129. Stevens A, Begum G, Cook A, Connor K, Rumball C, Oliver M, et al. Epigenetic changes in the hypothalamic proopiomelanocortin and glucocorticoid receptor genes in the ovine fetus after periconceptional undernutrition. Endocrinology. (2010) 151:3652–64. doi: 10.1210/en.2010-0094
130. Hua X, Xiong JW, Zhang YJ, Cao XY, Sun P, Wu J, et al. Exposure of pregnant mice to triclosan causes hyperphagic obesity of offspring via the hypermethylation of proopiomelanocortin promoter. Arch Toxicol. (2019) 93:547–58. doi: 10.1007/s00204-018-2338-1
131. Tabachnik T, Kisliouk T, Marco A, Meiri N, Weller A. Thyroid hormone-dependent epigenetic regulation of melanocortin 4 receptor levels in female offspring of obese rats. Endocrinology. (2017) 158:842–51. doi: 10.1210/en.2016-1854
132. Guo W, Chen J, Yang Y, Zhu J, Wu J. Epigenetic programming of Dnmt3a mediated by AP2alpha is required for granting preadipocyte the ability to differentiate. Cell Death Dis. (2016) 7:e2496. doi: 10.1038/cddis.2016.378
133. Broholm C, Olsson AH, Perfilyev A, Hansen NS, Schrolkamp M, Strasko KS, et al. Epigenetic programming of adipose-derived stem cells in low birthweight individuals. Diabetologia. (2016) 59:2664–73. doi: 10.1007/s00125-016-4099-9
134. Claycombe KJ, Vomhof-DeKrey EE, Garcia R, Johnson WT, Uthus E, Roemmich JN. Decreased beige adipocyte number and mitochondrial respiration coincide with increased histone methyl transferase (G9a) and reduced FGF21 gene expression in Sprague-Dawley rats fed prenatal low protein and postnatal high-fat diets. J Nutr Biochem. (2016) 31:113–21. doi: 10.1016/j.jnutbio.2016.01.008
135. Ma N, Nicholson CJ, Wong M, Holloway AC, Hardy DB. Fetal and neonatal exposure to nicotine leads to augmented hepatic and circulating triglycerides in adult male offspring due to increased expression of fatty acid synthase. Toxicol Appl Pharmacol. (2014) 275:1–11. doi: 10.1016/j.taap.2013.12.010
136. Yang QY, Liang JF, Rogers CJ, Zhao JX, Zhu MJ, Du M. Maternal obesity induces epigenetic modifications to facilitate Zfp423 expression and enhance adipogenic differentiation in fetal mice. Diabetes. (2013) 62:3727–35. doi: 10.2337/db13-0433
137. Strakovsky RS, Zhang X, Zhou D, Pan YX. Gestational high fat diet programs hepatic phosphoenolpyruvate carboxykinase gene expression and histone modification in neonatal offspring rats. J Physiol. (2011) 589(Pt 11):2707–17. doi: 10.1113/jphysiol.2010.203950
138. Zhou D, Wang H, Cui H, Chen H, Pan YX. Early-life exposure to high-fat diet may predispose rats to gender-specific hepatic fat accumulation by programming Pepck expression. J Nutr Biochem. (2015) 26:433–40. doi: 10.1016/j.jnutbio.2014.10.009
139. Strakovsky RS, Wang H, Engeseth NJ, Flaws JA, Helferich WG, Pan YX, et al. Developmental bisphenol A (BPA) exposure leads to sex-specific modification of hepatic gene expression and epigenome at birth that may exacerbate high-fat diet-induced hepatic steatosis. Toxicol Appl Pharmacol. (2015) 284:101–12. doi: 10.1016/j.taap.2015.02.021
140. Bricambert J, Alves-Guerra MC, Esteves P, Prip-Buus C, Bertrand-Michel J, Guillou H, et al. The histone demethylase Phf2 acts as a molecular checkpoint to prevent NAFLD progression during obesity. Nat Commun. (2018) 9:2092. doi: 10.1038/s41467-018-04361-y
141. Iljas JD, Guanzon D, Elfeky O, Rice GE, Salomon C. Review: bio-compartmentalization of microRNAs in exosomes during gestational diabetes mellitus. Placenta. (2017) 54:76–82. doi: 10.1016/j.placenta.2016.12.002
142. Yan X, Huang Y, Zhao JX, Rogers CJ, Zhu MJ, Ford SP, et al. Maternal obesity downregulates microRNA let-7g expression, a possible mechanism for enhanced adipogenesis during ovine fetal skeletal muscle development. Int J Obes. (2013) 37:568–75. doi: 10.1038/ijo.2012.69
143. Day SE, Coletta RL, Kim JY, Garcia LA, Campbell LE, Benjamin TR, et al. Potential epigenetic biomarkers of obesity-related insulin resistance in human whole-blood. Epigenetics-US. (2017) 12:254–63. doi: 10.1080/15592294.2017.1281501
144. Crujeiras AB, Diaz-Lagares A, Moreno-Navarrete JM, Sandoval J, Hervas D, Gomez A, et al. Genome-wide DNA methylation pattern in visceral adipose tissue differentiates insulin-resistant from insulin-sensitive obese subjects. Transl. Res. (2016) 178:13–24.e5. doi: 10.1016/j.trsl.2016.07.002
145. de Mello VD, Matte A, Perfilyev A, Mannisto V, Ronn T, Nilsson E, et al. Human liver epigenetic alterations in non-alcoholic steatohepatitis are related to insulin action. Epigenetics-US. (2017) 12:287–95. doi: 10.1080/15592294.2017.1294305
146. Sathishkumar C, Prabu P, Balakumar M, Lenin R, Prabhu D, Anjana RM, et al. Augmentation of histone deacetylase 3 (HDAC3) epigenetic signature at the interface of proinflammation and insulin resistance in patients with type 2 diabetes. Clin Epigenetics. (2016) 8:125. doi: 10.1186/s13148-016-0293-3
147. Castellano-Castillo D, Moreno-Indias I, Fernandez-Garcia JC, Clemente-Postigo M, Castro-Cabezas M, Tinahones FJ, et al. Complement factor C3 methylation and mRNA expression is associated to BMI and insulin resistance in obesity. Genes. (2018) 9:E410. doi: 10.3390/genes9080410
148. Su S, Zhu H, Xu X, Wang X, Dong Y, Kapuku G, et al. DNA methylation of the LY86 gene is associated with obesity, insulin resistance, and inflammation. Twin Res Hum Genet. (2014) 17:183–91. doi: 10.1017/thg.2014.22
149. Arner P, Sahlqvist AS, Sinha I, Xu H, Yao X, Waterworth D, et al. The epigenetic signature of systemic insulin resistance in obese women. Diabetologia. (2016) 59:2393–405. doi: 10.1007/s00125-016-4074-5
150. Ramos-Lopez O, Riezu-Boj JI, Milagro FI, Martinez JA. DNA methylation signatures at endoplasmic reticulum stress genes are associated with adiposity and insulin resistance. Mol Genet Metab. (2018) 123:50–8. doi: 10.1016/j.ymgme.2017.11.011
151. Main AM, Gillberg L, Jacobsen AL, Nilsson E, Gjesing AP, Hansen T, et al. DNA methylation and gene expression of HIF3A: cross-tissue validation and associations with BMI and insulin resistance. Clin Epigenetics. (2016) 8:89. doi: 10.1186/s13148-016-0258-6
152. Burghardt KJ, Goodrich JM, Dolinoy DC, Ellingrod VL. Gene-specific DNA methylation may mediate atypical antipsychotic-induced insulin resistance. Bipolar Disord. (2016) 18:423–32. doi: 10.1111/bdi.12422
153. Houshmand-Oeregaard A, Schrolkamp M, Kelstrup L, Hansen NS, Hjort L, Thuesen A, et al. Increased expression of microRNA-15a and microRNA-15b in skeletal muscle from adult offspring of women with diabetes in pregnancy. Hum Mol Genet. (2018) 27:1763–71. doi: 10.1093/hmg/ddy085
154. Adamu HA, Imam MU, Ooi DJ, Esa NM, Rosli R, Ismail M. In utero exposure to germinated brown rice and its oryzanol-rich extract attenuated high fat diet-induced insulin resistance in F1 generation of rats. BMC Complement Altern Med. (2017) 17:67. doi: 10.1186/s12906-017-1571-0
155. Suter MA, Ma J, Vuguin PM, Hartil K, Fiallo A, Harris RA, et al. In utero exposure to a maternal high-fat diet alters the epigenetic histone code in a murine model. Am J Obstet Gynecol. (2014) 210:463.e1–11. doi: 10.1016/j.ajog.2014.01.045
156. You D, Nilsson E, Tenen DE, Lyubetskaya A, Lo JC, Jiang R, et al. Dnmt3a is an epigenetic mediator of adipose insulin resistance. eLife. (2017) 6:e30766. doi: 10.7554/eLife.30766
157. Kim AY, Park YJ, Pan X, Shin KC, Kwak SH, Bassas AF, et al. Obesity-induced DNA hypermethylation of the adiponectin gene mediates insulin resistance. Nat. Commun. (2015) 6:7585. doi: 10.1038/ncomms8585
158. Khalyfa A, Mutskov V, Carreras A, Khalyfa AA, Hakim F, Gozal D. Sleep fragmentation during late gestation induces metabolic perturbations and epigenetic changes in adiponectin gene expression in male adult offspring mice. Diabetes. (2014) 63:3230–41. doi: 10.2337/db14-0202
159. Xie X, Lin T, Zhang M, Liao L, Yuan G, Gao H, et al. IUGR with infantile overnutrition programs an insulin-resistant phenotype through DNA methylation of peroxisome proliferator-activated receptor-gamma coactivator-1alpha in rats. Pediatr Res. (2015) 77:625–32. doi: 10.1038/pr.2015.32
160. Rajesh P, Balasubramanian K. Phthalate exposure in utero causes epigenetic changes and impairs insulin signalling. J Endocrinol. (2014) 223:47–66. doi: 10.1530/JOE-14-0111
161. Ramon-Krauel M, Pentinat T, Bloks VW, Cebria J, Ribo S, Perez-Wienese R, et al. Epigenetic programming at the Mogat1 locus may link neonatal overnutrition with long-term hepatic steatosis and insulin resistance. FASEB J. (2018) 32:6025–37. doi: 10.1096/fj.201700717RR
162. Kasch J, Kanzleiter I, Saussenthaler S, Schurmann A, Keijer J, van Schothorst E, et al. Insulin sensitivity linked skeletal muscle Nr4a1 DNA methylation is programmed by the maternal diet and modulated by voluntary exercise in mice. J Nutr Biochem. (2018) 57:86–92. doi: 10.1016/j.jnutbio.2018.03.015
163. Ngo S, Li X, O'Neill R, Bhoothpur C, Gluckman P, Sheppard A. Elevated S-adenosylhomocysteine alters adipocyte functionality with corresponding changes in gene expression and associated epigenetic marks. Diabetes. (2014) 63:2273–83. doi: 10.2337/db13-1640
164. Joseph R, Poschmann J, Sukarieh R, Too PG, Julien SG, Xu F, et al. ACSL1 is associated with fetal programming of insulin sensitivity and cellular lipid content. Mol Endocrinol. (2015) 29:909–20. doi: 10.1210/me.2015-1020
165. Benatti RO, Melo AM, Borges FO, Ignacio-Souza LM, Simino LA, Milanski M, et al. Maternal high-fat diet consumption modulates hepatic lipid metabolism and microRNA-122 (miR-122) and microRNA-370 (miR-370) expression in offspring. Br J Nutr. (2014) 111:2112–22. doi: 10.1017/S0007114514000579
166. Nicholas LM, Rattanatray L, MacLaughlin SM, Ozanne SE, Kleemann DO, Walker SK, et al. Differential effects of maternal obesity and weight loss in the periconceptional period on the epigenetic regulation of hepatic insulin-signaling pathways in the offspring. FASEB J. (2013) 27:3786–96. doi: 10.1096/fj.13-227918
167. Fernandez-Twinn DS, Alfaradhi MZ, Martin-Gronert MS, Duque-Guimaraes DE, Piekarz A, Ferland-McCollough D, et al. Downregulation of IRS-1 in adipose tissue of offspring of obese mice is programmed cell-autonomously through post-transcriptional mechanisms. Mol Metab. (2014) 3:325–33. doi: 10.1016/j.molmet.2014.01.007
168. Komariah K, Manalu W, Kiranadi B, Winarto A, Handharyani E, Roeslan MO. Valproic acid exposure of pregnant rats during organogenesis disturbs pancreas development in insulin synthesis and secretion of the offspring. Toxicol Res. (2018) 34:173–82. doi: 10.5487/TR.2018.34.2.173
169. Wang P, Fiaschi-Taesch NM, Vasavada RC, Scott DK, Garcia-Ocana A, Stewart AF. Diabetes mellitus–advances and challenges in human beta-cell proliferation. Nat Rev. Endocrinol. (2015) 11:201–12. doi: 10.1038/nrendo.2015.9
170. Bernstein D, Golson ML, Kaestner KH. Epigenetic control of beta-cell function and failure. Diabetes Res Clin Pract. (2017) 123:24–36. doi: 10.1016/j.diabres.2016.11.009
171. Xie R, Carrano AC, Sander M. A systems view of epigenetic networks regulating pancreas development and beta-cell function. Wiley Interdiscip Rev Syst Biol Med. (2015) 7:1–11. doi: 10.1002/wsbm.1287
172. Xu CR, Cole PA, Meyers DJ, Kormish J, Dent S, Zaret KS. Chromatin “prepattern” and histone modifiers in a fate choice for liver and pancreas. Science. (2011) 332:963–6. doi: 10.1126/science.1202845
173. Xu CR, Li LC, Donahue G, Ying L, Zhang YW, Gadue P, et al. Dynamics of genomic H3K27me3 domains and role of EZH2 during pancreatic endocrine specification. EMBO J. (2014) 33:2157–70. doi: 10.15252/embj.201488671
174. Dhawan S, Tschen SI, Zeng C, Guo T, Hebrok M, Matveyenko A, et al. DNA methylation directs functional maturation of pancreatic beta cells. J. Clin. Invest. (2015) 125:2851–60. doi: 10.1172/JCI79956
175. Yang YP, Magnuson MA, Stein R, Wright CV. The mammal-specific Pdx1 Area II enhancer has multiple essential functions in early endocrine cell specification and postnatal beta-cell maturation. Development. (2017) 144:248–57. doi: 10.1242/dev.143123
176. Salguero-Aranda C, Tapia-Limonchi R, Cahuana GM, Hitos AB, Diaz I, Hmadcha A, et al. Differentiation of mouse embryonic stem cells towards functional pancreatic beta-cell surrogates through epigenetic regulation of Pdx1 by nitric oxide. Cell Transplant. (2016) 25:1879–92. doi: 10.3727/096368916X691178
177. Rajesh P, Balasubramanian K. Gestational exposure to di(2-ethylhexyl) phthalate (DEHP) impairs pancreatic beta-cell function in F1 rat offspring. Toxicol Lett. (2015) 232:46–57. doi: 10.1016/j.toxlet.2014.09.025
178. Russ HA, Hebrok M. Taming the young and restless–epigenetic gene regulation in pancreas and beta-cell precursors. EMBO J. (2014) 33:2135–6. doi: 10.15252/embj.201489751
179. Sanchez-Parra C, Jacovetti C, Dumortier O, Lee K, Peyot ML, Guay C, et al. Contribution of the long noncoding RNA H19 to beta-cell mass expansion in neonatal and adult rodents. Diabetes. (2018) 67:2254–67. doi: 10.2337/db18-0201
180. McPherson NO, Lane M, Sandeman L, Owens JA, Fullston T. An exercise-only intervention in obese fathers restores glucose and insulin regulation in conjunction with the rescue of pancreatic islet cell morphology and microRNA expression in male offspring. Nutrients. (2017) 9:122. doi: 10.3390/nu9020122
181. Crevillen P, Yang H, Cui X, Greeff C, Trick M, Qiu Q, et al. Epigenetic reprogramming that prevents transgenerational inheritance of the vernalized state. Nature. (2014) 515:587–90. doi: 10.1038/nature13722
182. Yang J, Chatterjee N, Kim Y, Roh JY, Kwon JH, Park MS, et al. Histone methylation-associated transgenerational inheritance of reproductive defects in Caenorhabditis elegans exposed to crude oil under various exposure scenarios. Chemosphere. (2018) 200:358–65. doi: 10.1016/j.chemosphere.2018.02.080
183. An K, Du F, Meng H, Li G, Zhang M, Liu Z, et al. Transgenerational analysis of H3K4me3 and H3K27me3 by ChIP-Seq links epigenetic inheritance to metabolism. J Genet Genomics. (2018) 45:169–72. doi: 10.1016/j.jgg.2017.11.004
184. Han L, Ren C, Li L, Li X, Ge J, Wang H, et al. Embryonic defects induced by maternal obesity in mice derive from Stella insufficiency in oocytes. Nat Genet. (2018) 50:432–42. doi: 10.1038/s41588-018-0055-6
185. Rodgers AB, Morgan CP, Leu NA, Bale TL. Transgenerational epigenetic programming via sperm microRNA recapitulates effects of paternal stress. Proc Natl Acad Sci USA. (2015) 112:13699–704. doi: 10.1073/pnas.1508347112
186. Larriba E, Del MJ. Role of non-coding RNAs in the transgenerational epigenetic transmission of the effects of reprotoxicants. Int J Mol Sci. (2016) 17:452. doi: 10.3390/ijms17040452
187. Su R, Yan J, Yang H. Transgenerational glucose intolerance of tumor necrosis factor with epigenetic alteration in rat perirenal adipose tissue induced by intrauterine hyperglycemia. J Diabetes Res. (2016) 2016:4952801. doi: 10.1155/2016/4952801
188. Briffa JF, Wlodek ME, Moritz KM. Transgenerational programming of nephron deficits and hypertension. Semin Cell Dev Biol. (2018). doi: 10.1016/j.semcdb.2018.05.025
189. Chen J, Wu S, Wen S, Shen L, Peng J, Yan C, et al. The mechanism of environmental endocrine disruptors (DEHP) induces epigenetic transgenerational inheritance of cryptorchidism. PLoS ONE. (2015) 10:e0126403. doi: 10.1371/journal.pone.0126403
190. Bale TL. Epigenetic and transgenerational reprogramming of brain development. Nat Rev Neurosci. (2015) 16:332–44. doi: 10.1038/nrn3818
191. Babenko O, Kovalchuk I, Metz GA. Stress-induced perinatal and transgenerational epigenetic programming of brain development and mental health. Neurosci Biobehav Rev. (2015) 48:70–91. doi: 10.1016/j.neubiorev.2014.11.013
192. Lecoutre S, Petrus P, Ryden M, Breton C. Transgenerational epigenetic mechanisms in adipose tissue development. Trends Endocrinol Metab. (2018) 29:675–85. doi: 10.1016/j.tem.2018.07.004
193. Chamorro-Garcia R, Diaz-Castillo C, Shoucri BM, Kach H, Leavitt R, Shioda T, et al. Ancestral perinatal obesogen exposure results in a transgenerational thrifty phenotype in mice. Nat Commun. (2017) 8:2012. doi: 10.1038/s41467-017-01944-z
194. Ksinanova M, Cikos S, Babel'Ova J, Sefcikova Z, Spirkova A, Koppel J, et al. The responses of mouse preimplantation embryos to leptin in vitro in a transgenerational model for obesity. Front Endocrinol. (2017) 8:233. doi: 10.3389/fendo.2017.00233
195. Gonzalez-Bulnes A, Astiz S, Ovilo C, Lopez-Bote CJ, Sanchez-Sanchez R, Perez-Solana ML, et al. Early-postnatal changes in adiposity and lipids profile by transgenerational developmental programming in swine with obesity/leptin resistance. J Endocrinol. (2014) 223:M17–29. doi: 10.1530/JOE-14-0217
Keywords: epigenetic programming, fetal metabolic programming, metabolic syndrome, obesity, insulin resistance, adverse intrauterine environment
Citation: Zhu Z, Cao F and Li X (2019) Epigenetic Programming and Fetal Metabolic Programming. Front. Endocrinol. 10:764. doi: 10.3389/fendo.2019.00764
Received: 03 September 2018; Accepted: 21 October 2019;
Published: 03 December 2019.
Edited by:
Tim S. Nawrot, University of Hasselt, BelgiumReviewed by:
Karen Vrijens, University of Hasselt, BelgiumSean L. McGee, Metabolic, Deakin University, Australia
Copyright © 2019 Zhu, Cao and Li. This is an open-access article distributed under the terms of the Creative Commons Attribution License (CC BY). The use, distribution or reproduction in other forums is permitted, provided the original author(s) and the copyright owner(s) are credited and that the original publication in this journal is cited, in accordance with accepted academic practice. No use, distribution or reproduction is permitted which does not comply with these terms.
*Correspondence: Xiaozhong Li, YW5zd2VyY25AMTYzLmNvbQ==