- 1Skeletal Biology Laboratory, School of Biological and Population Health Sciences, Oregon State University, Corvallis, OR, United States
- 2Center for Healthy Aging Research, Oregon State University, Corvallis, OR, United States
- 3Biostatistics Program, School of Biological and Population Health Sciences, Oregon State University, Corvallis, OR, United States
Growing female mice housed at room temperature (22°C) weigh the same but differ in body composition compared to mice housed at thermoneutrality (32°C). Specifically, mice housed at room temperature have lower levels of white adipose tissue (WAT). Additionally, bone marrow adipose tissue (bMAT) and cancellous bone volume fraction in distal femur metaphysis are lower in room temperature-housed mice. The metabolic changes induced by sub-thermoneutral housing are associated with lower leptin levels in serum and higher levels of Ucp1 gene expression in brown adipose tissue. Although the precise mechanisms mediating adaptation to sub-thermoneutral temperature stress remain to be elucidated, there is evidence that increased sympathetic nervous system activity acting via β-adrenergic receptors plays an important role. We therefore evaluated the effect of the non-specific β-blocker propranolol (primarily β1 and β2 antagonist) on body composition, femur microarchitecture, and bMAT in growing female C57BL/6 mice housed at either room temperature or thermoneutral temperature. As anticipated, cancellous bone volume fraction, WAT and bMAT were lower in mice housed at room temperature. Propranolol had small but significant effects on bone microarchitecture (increased trabecular number and decreased trabecular spacing), but did not attenuate premature bone loss induced by room temperature housing. In contrast, propranolol treatment prevented housing temperature-associated differences in WAT and bMAT. To gain additional insight, we evaluated a panel of genes in tibia, using an adipogenesis PCR array. Housing temperature and treatment with propranolol had exclusive as well as shared effects on gene expression. Of particular interest was the finding that room temperature housing reduced, whereas propranolol increased, expression of the gene for acetyl-CoA carboxylase (Acacb), the rate-limiting step for fatty acid synthesis and a key regulator of β-oxidation. Taken together, these findings provide evidence that increased activation of β1 and/or β2 receptors contributes to reduced bMAT by regulating adipocyte metabolism, but that this pathway is unlikely to be responsible for premature cancellous bone loss in room temperature-housed mice.
Introduction
The thermoneutral zone, also called the zone of thermal comfort, is the temperature range where the resting rate of heat production is in equilibrium with the rate of heat loss to the environment. The thermoneutral range varies among species and is ~10°C higher for mice than for humans (1). Environmental temperatures outside the thermoneutral zone induce adaptive responses in homeothermic animals to preserve core body temperature (e.g., shivering to increase body temperature and sweating/panting to decrease body temperature). In contrast to humans and larger rodents such as rats, mice are not strict homeotherms, but instead function as daily facultative heterotherms. This fundamental difference in energy homeostasis contributes to important differences in physiology between mice and humans. Daily torpor, where decreases in body temperature result in energy savings, is a common strategy utilized by mice and other small mammals to cope with low environmental temperatures and fluctuations in food availability (2). Cold stress induced by housing mice at standard room temperature results in physiological changes that can influence experimental outcomes. Tumor growth, for example, is significantly greater in mice housed at room temperature than in mice housed at thermoneutrality, and anti-tumor immune response is suppressed at room temperature (3). Furthermore, chronic stress induced by housing mice at room temperature increases the resistance of hematopoietic stem and progenitor cells to total body radiation-induced apoptosis (4). The impact of cold stress on response to tumor growth and radiation in the aforementioned studies depends on β-adrenergic signaling and is antagonized by treatment with propranolol, a non-specific antagonist of β-adrenergic receptors (3, 4).
When housed at room temperature, C57BL/6 mice of both sexes exhibit rapid loss of cancellous bone prior to cessation of linear bone growth (5–7). Importantly, housing mice at thermoneutrality prevents the premature bone loss (6, 7). The lower cancellous bone volume fraction in distal femur of mice housed at room temperature compared to mice housed at thermoneutrality is due, in part, to decreased bone formation; osteoblast-lined bone perimeter, bone formation rate measured using fluorochrome labels, mRNA levels for bone matrix proteins, and serum osteocalcin are lower in mice housed at room temperature. In contrast, osteoclast-lined bone perimeter is higher, suggesting locally increased bone resorption (7). Bone formation and cancellous bone volume fraction are often inversely associated with bone marrow adiposity (8–15). However, an inverse relationship was not observed with housing temperature; female mice housed at room temperature had lower bone formation as well as lower bMAT compared to mice housed at thermoneutrality, whereas male mice had lower bone formation but did not exhibit differences in bMAT (6, 7).
Bone is innervated and bone growth and turnover balance is regulated, in part, via sensory and sympathetic signaling (16). β-adrenergic receptors are located in skeletal tissue (17) and treatment with propranolol is reported to influence bone mass in some animal models (17–21) and increase fat accrual in humans (22, 23). Thermogenesis induced by cold stress is mediated, at least in part, by increased sympathetic outflow from the hypothalamus to peripheral tissues, including brown adipose tissue (BAT) (24). The purpose of this study was to assess the role of β-adrenergic signaling (by blocking β-adrenergic receptors with propranolol) in mediating the differential effects of housing temperature on body composition, bone, and bMAT levels in female mice.
Materials and Methods
Experimental Design
The Institutional Animal Care and Use Committee approved the experimental protocol used in this study and animals were maintained in accordance with the NIH Guide for the Care and the Use of Laboratory Animals. A total of 40, 4-week-old, female C57BL/6 (B6) mice were obtained from Jackson Laboratory (Bar Harbor, ME, USA) and housed individually in a room on a 12 h light:12 h dark cycle. Food (Teklad 8604, Harlen Laboratories, Indianapolis, IN) and water were provided ad libitum to all animals. Body weight and food consumption were measured weekly for the 14-week duration of study.
Mice were randomized by weight into one of four groups, 22°C ± propranolol or 32°C ± propranolol (n = 10/group), and maintained at their respective temperatures and treatments until 18 weeks of age. Propranolol (Sigma, St. Louis) was administered in drinking water (0.5 g/l, pH 3.0) using aluminum foil-covered drinking tubes. Control mice received acidified water (vehicle). Water was changed twice/week. Water consumption was calculated as ml/d and the dose rate of propranolol calculated as mg/g/d. This method of delivery and dose of propranolol was chosen because it has been shown to be effective in blocking β1 and β2 but not β3 adrenergic receptors (25–27).
Mice were anesthetized with 2–3% isoflurane delivered in oxygen and body composition determined immediately prior to sacrifice. The mice were bled by cardiac puncture. Serum was collected and stored at −80°C for measurement of leptin and global markers of bone turnover. Abdominal white adipose tissue (WAT) and uteri were excised and weighed. Femora were removed, fixed overnight in 10% formalin, and stored in 70% ethanol for microcomputed tomography (μCT) and histomorphometric analyses. Tibiae and brown adipose tissue (BAT) were removed, frozen in liquid nitrogen, and stored at −80°C for mRNA analysis.
Serum Chemistry
Serum leptin was measured using Mouse Leptin Quantikine ELISA Kit (R&D Systems, Minneapolis, MN), serum osteocalcin was measured using Mouse Gla-Osteocalcin High Sensitive EIA Kit (Clontech, Mountain View, CA), and serum CTX-1 was measured using Mouse CTX-1 ELISA kit (Life Sciences Advanced Technologies, Petersburg, FL) according to the respective manufacturer's protocol.
Dual Energy X-Ray Absorptiometry
Percent body fat was determined using dual energy x-ray absorptiometry (DXA) (Piximus, Lunar Corp., Madison, WI, USA).
Microcomputed Tomography
Bone volume and architecture were assessed using μCT. We scanned right femora in 70% ethanol using a Scanco μCT40 scanner (Scanco Medical AG, Basserdorf, Switzerland) at a voxel size of 12 μm on a side (55 kVp x-ray voltage, 145 μA intensity, and 200 ms integration time). We set filtering parameters sigma and support to 0.8 and 1, respectively. Bone segmentation was conducted at a threshold of 245 (scale, 0–1,000) determined empirically. Total femur mineralized tissue volume (cancellous + cortical bone) was evaluated first. This was followed by evaluation of cancellous bone in the distal femur metaphysis. For the femoral metaphysis, 42 consecutive slices (504 μm) of cancellous bone, 45 slices (540 μm) proximal to the growth plate/metaphysis boundary, were evaluated. We used irregular manual contouring a few pixels interior to the endocortical surface to delineate cancellous from cortical bone. Direct cancellous bone measurements included cancellous bone volume fraction (bone volume/tissue volume, %), connectivity density (mm−3), trabecular thickness (μm), trabecular number (mm−1), and trabecular separation (μm).
Histomorphometry
Methods used for measuring bone histomorphometry have been described (28) with modifications for mice (29). Briefly, distal right femora were dehydrated in a graded series of ethanol and xylene, and embedded undecalcified in modified methyl methacrylate. A vertical bed microtome (Leica 2065) was used to cut coronal sections (4 μm thick), which were then affixed to slides precoated with 1% gelatin solution. One section/animal was stained for tartrate resistant acid phosphatase, counterstained with toluidine blue (Sigma, St. Louis), and used for cell-based measurements. All data were collected with a 20x objective using the OsteoMeasure System (OsteoMetrics, Inc., Atlanta, GA). The sampling site for the distal femoral metaphysis was located 0.25–1.25 mm proximal to the growth plate and 0.1 mm from cortical bone.
Cell-based measurements included osteoblast perimeter (osteoblast perimeter/bone perimeter, %), osteoclast perimeter (osteoclast perimeter/bone perimeter, %), marrow adipocyte area fraction (adipocyte area/tissue area, %), adipocyte density (number of adipocytes/tissue area, #/mm2) and adipocyte size (μm2). Osteoblasts were identified morphologically as plump cuboidal cells immediately adjacent to a thin layer of osteoid in direct contact with the bone perimeter. Osteoclasts were identified as multinucleated (two or more nuclei) cells with acid phosphatase positive (red-stained) cytoplasm in contact with the bone perimeter. Adipocytes were identified as large circular or oval-shaped cells bordered by a prominent cell membrane and lacking cytoplasmic staining due to alcohol extraction of intracellular lipids during processing. This method has been previously validated by fat extraction and analysis (30). All bone histomorphometric data are reported using standard 2-dimensional nomenclature (31).
Gene Expression
Tibiae (n = 8/group) were pulverized with a mortar and pestle in liquid nitrogen and homogenized in Trizol (Life Technologies, Grand Island, NY). Total RNA was isolated according to the manufacturer's protocol, and mRNA was reverse transcribed into cDNA using SuperScript III First-Strand Synthesis SuperMix for qRT-PCR (Life Technologies). Gene expression for a panel of genes related to adipocyte differentiation and function (Mouse Adipogenesis RT2 Profiler PCR Array, PAMM-049ZE-4) was determined according to the manufacturer's protocol (Qiagen, Valencia, CA). Gene expression was normalized to GAPDH. Relative quantification was determined (ΔΔCt method) using RT2 Profiler PCR Array Data Analysis software version 3.5 (Qiagen). Fold-change was calculated relative to mice housed at 32°C as the reference control. Specifically, we compared changes in tibia gene expression in (1) mice housed at 22°C relative to mice housed at 32°C and (2) mice housed at 22°C and treated with propranolol relative to mice housed at 32°C to identify overlap between genes differentially expressed in response to sub-thermoneutral housing or propranolol. Furthermore, the expression of additional genes (Adrb1, Adrb3, Alpl, and Bglap) was determined using gene-specific primers (Adrb1 - for: CTCATCGTGGTGGGTAACGTG, rev: ACACACAGCACATCTACCGAA; Adrb3 – for: GGCCCTCTCTAGTTCCCAG, rev: TAGCCATCAAACCTGTTGAGC; Alpl – Qiagen RT2 qPCR Primer Assay PPM03155A; Bglap– Qiagen RT2 qPCR Primer Assay PPM04465F), and changes in gene expression between treatment groups were analyzed using the ΔΔCt method as described above.
Statistical Analysis
A 2 × 2 factorial experimental design was used with mice randomized to a temperature group (22 or 32°C) and treatment group (vehicle control or propranolol). Mean comparisons were made using a two-factor linear model with an interaction between temperature and treatment. Residual analysis, Levene's test for homogeneity of variance, and Anderson-Darling tests of normality were used to assess the conditions for use of a linear model. A general linear model with unequal variances for the temperature groups, treatment groups, or both temperature and treatment groups (i.e., four distinct variance parameters) was used when the assumption of homogeneity of variance was violated. When interaction was present, inference focused on comparing temperature groups separately for vehicle and propranolol treated mice (i.e., focusing on how treatment modified the effect of temperature). The Benjamini and Hochberg (32) method for maintaining the false discovery rate at 5% was used to adjust for multiple comparisons. Differences were considered significant at p ≤ 0.05. All data are presented as mean ± SE. Data analysis was performed using R version 3.4.3.
Results
The daily dose of propranolol—administered in drinking water—in mice housed at room temperature (22°C) or thermoneutral temperature (32°C) is shown in Figure 1. When averaged over the 14 weeks of study, mice housed at 22 and 32°C consumed 0.13 ± 0.01 mg/g/d and 0.14 ± 0.01mg/g/d of propranolol, respectively.
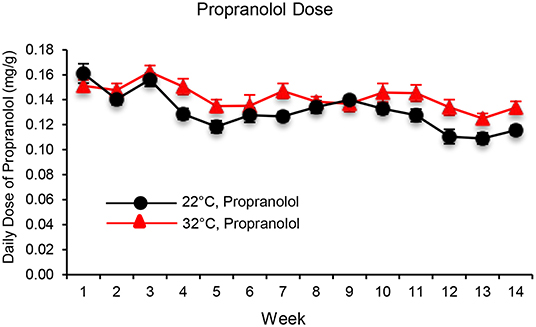
Figure 1. The daily dose of propranolol—administered in drinking water—in mice housed at room temperature (22°C) and thermoneutral temperature (32°C) over the 14-week duration of study. Data are mean ± SE. N = 10/group.
The respective effects of housing temperature and propranolol treatment on food and body weight, body composition, serum chemistry, and Ucp1 gene expression in BAT are shown in Figure 2. Mice housed at room temperature consumed more food per day than mice housed at thermoneutrality (panel A), resulting in 91% increase in cumulative food intake (panel B). However, housing temperature had no effect on body weight (panels C and D). Treatment with propranolol had no effect on daily or cumulative food intake or on body weight and no housing temperature x treatment interactions were noted for the aforementioned endpoints. Interactions between housing temperature and treatment were detected for percent body fat (panel E), abdominal WAT weight (panel F), and serum leptin levels (panel H); treatment with propranolol prevented the differential effects of housing temperature on these endpoints. Room temperature-housed mice had higher uterine weight (panel G). Finally, room temperature-housed mice had higher blood glucose (panel I) and higher BAT Ucp1 gene expression (panel J), endpoints not influenced by propranolol treatment.
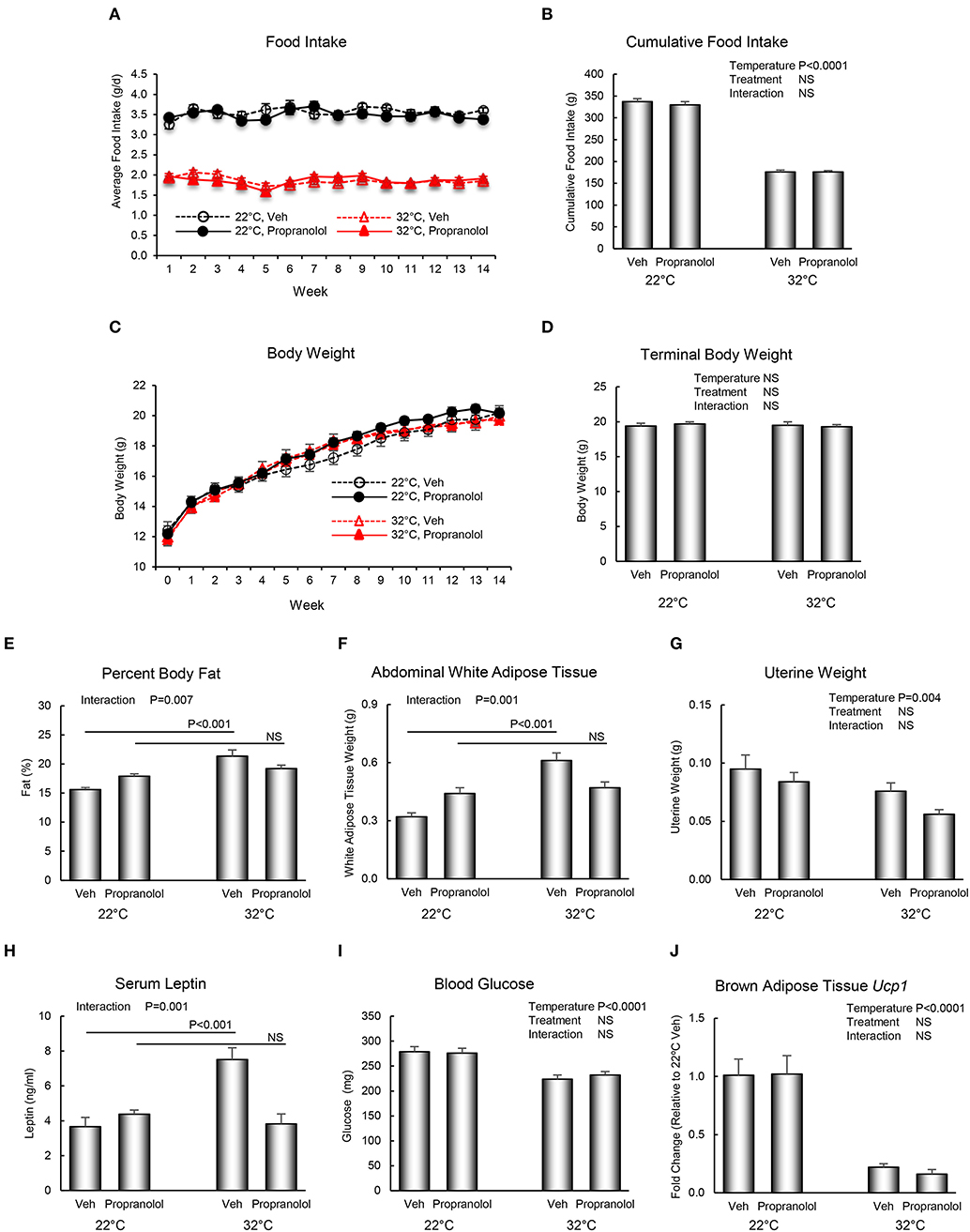
Figure 2. Effect of housing temperature (temperature) and treatment with propranolol (treatment) on (A,B) food intake, (C,D) body weight, (E) percent body fat, (F) abdominal white adipose tissue weight, (G) uterine weight, (H) serum leptin, (I) blood glucose, and (J) brown adipose tissue Ucp1 gene expression. Data are mean ± SE. N = 10/group for (A–I) and n = 8/group for (J). Post–hoc analysis was performed when there was a significant interaction term.
The respective effects of housing temperature and treatment with propranolol on bone microarchitecture in the distal femur metaphysis are shown in Figure 3. Mice housed at room temperature had lower bone volume fraction (panel A), connectivity density (panel B), trabecular thickness (panel C), and trabecular number (panel D), and higher trabecular separation (panel E). Treatment with propranolol resulted in higher trabecular number and lower trabecular separation. No housing temperature × treatment interactions were detected for any of the measured indices of bone microarchitecture.
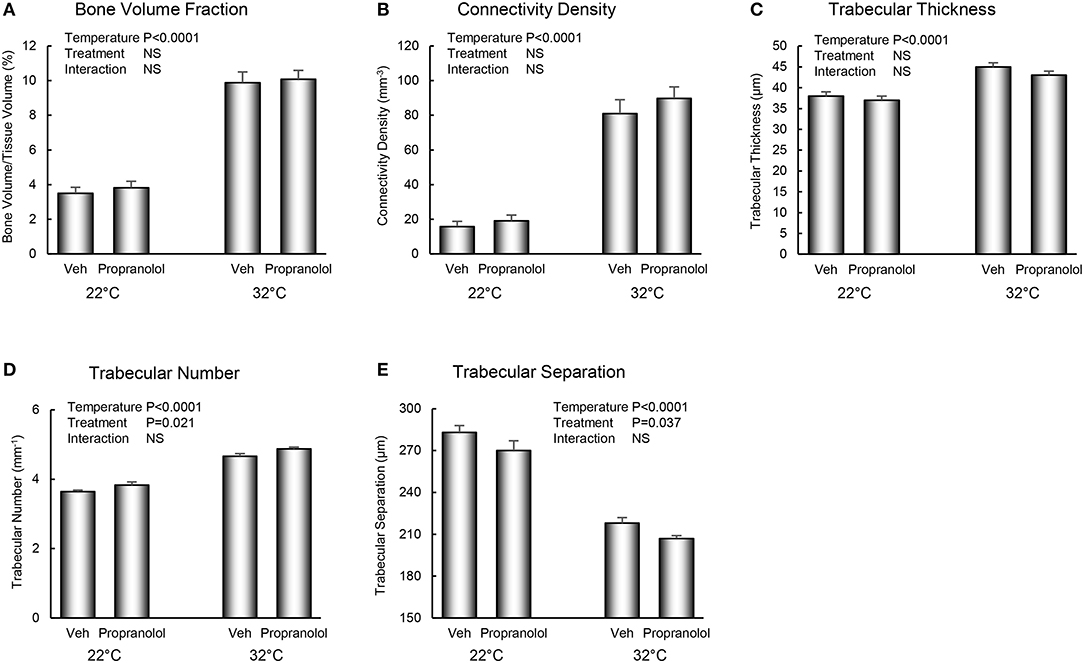
Figure 3. Effect of housing temperature (temperature) and treatment with propranolol (treatment) on cancellous bone architecture in distal femur metaphysic: (A) cancellous bone volume fraction, (B) connectivity density, (C) trabecular thickness, (D) trabecular number, and (E) trabecular separation. Data are mean ± SE. N = 10/group.
The respective effects of housing temperature and treatment with propranolol on osteoblast perimeter, osteoclast perimeter and indices of bone marrow adiposity in distal femur metaphysis are shown in Figure 4. Mice housed at room temperature had lower osteoblast perimeter (panel A) and higher osteoclast perimeter (panel B). Interactions between housing temperature and treatment were detected for adipocyte area fraction (panel C) and adipocyte density (panel D); specifically, treatment with propranolol prevented the differential effects of housing temperature on both indices of marrow adiposity. However, neither housing temperature nor treatment altered adipocyte size. The differences in histomorphometry can be appreciated in the representative micrographs shown in panels F–I.
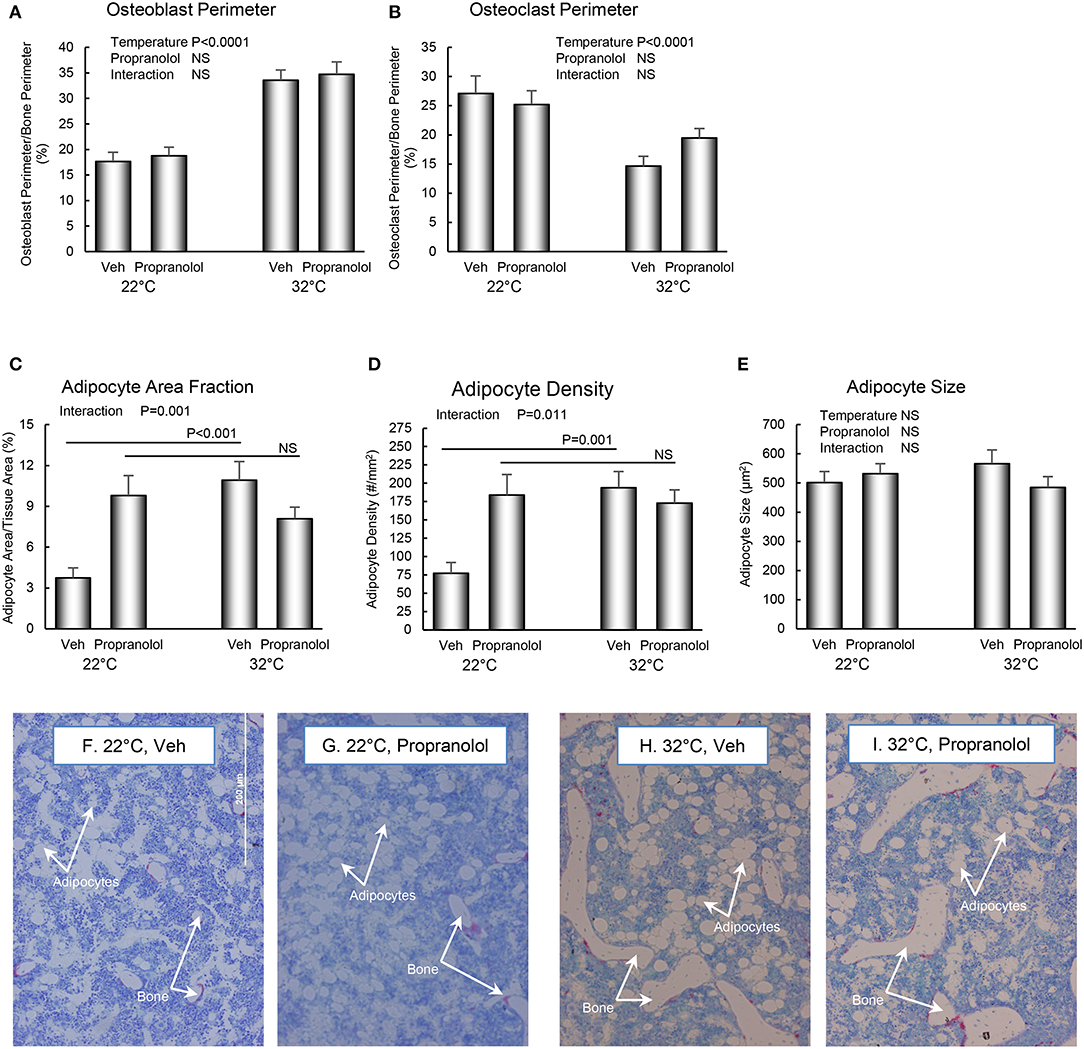
Figure 4. Effect of housing temperature (temperature) and treatment with propranolol (treatment) on indices of bone formation, bone resorption, and marrow adiposity in distal femur metaphysis: (A) osteoblast perimeter, (B) osteoclast perimeter, (C) adipocyte area fraction, (D) adipocyte density, and (E) adipocyte size. Representative images of the bone marrow compartment showing bMAT and cancellous bone in each treatment group in the distal femur metaphysis are shown in (F–I). Data are mean ± SE. N = 10/group. Post-hoc analysis was performed when there was a significant interaction term.
The respective effects of housing temperature and treatment with propranolol on serum osteocalcin and CTX-1 are shown in Figure 5. An interaction between housing temperature and treatment was noted for osteocalcin; the differential effects of housing temperature were attenuated by treatment with propranolol. Neither temperature nor treatment with propranolol had an effect on serum CTX-1.
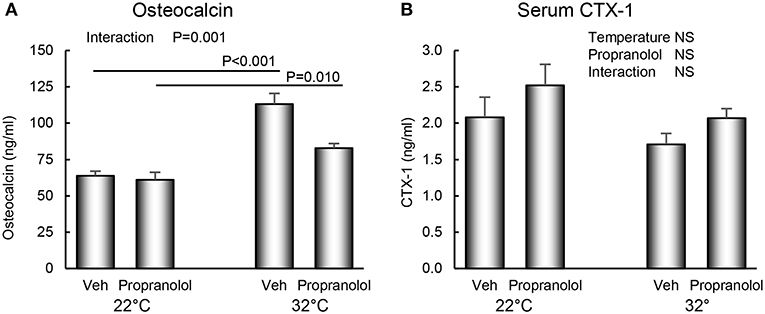
Figure 5. Effect of housing temperature (temperature) and treatment with propranolol (treatment) on (A) serum osteocalcin, a marker of global bone formation and (B) serum CTX-1, a marker of global bone resorption. Data are mean ± SE. N = 10/group. Post-hoc analysis was performed when there was a significant interaction term.
The respective effects of housing temperature and treatment on expression of a panel of 84 genes (adipogenesis PCR array), including Adrb2 (β-adrenergic receptor 2), in tibia of mice housed at 22°C ± propranolol relative to mice housed at 32°C are shown in Figure 6. Housing temperature resulted in differential expression of 27 genes (22°C Veh vs. 32°C Veh); 12 of these genes were not differentially expressed in mice housed at 22°C and treated with propranolol and included genes for hormones (Adipoq, Lep), transcription factors and enhancer proteins (Cebpb, Dkk1, Klf2, Klf15, Jun, Src), regulators of cell cycle progression (Cdk4, Cdkn1b, Foxc2), and extracellular signaling factors (Sfrp1). Compared to mice housed at 32°C, Acacb was higher in mice housed at 22°C but lower in mice housed at 22°C and treated with propranolol. Finally, 31 differentially expressed genes were unique to propranolol treatment (22°C propranolol vs. 32°C vehicle). Expression levels for Adrb2 did not differ between mice housed at 22 and 32°C. However, expression levels were higher in propranolol-treated mice housed at 22°C compared to vehicle-treated mice housed at 32°C.
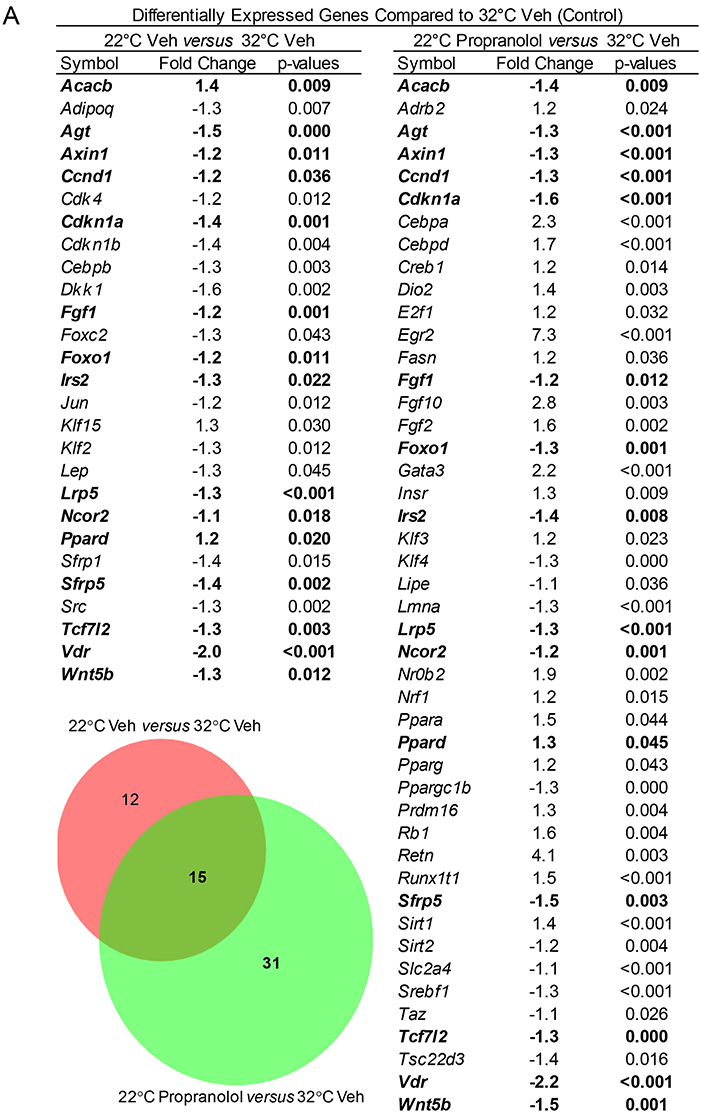
Figure 6. Effect of housing temperature and treatment with propranolol on expression of adipogenesis-associated genes (adipogenesis PCR array) in whole tibia. The Venn diagram shows the number of differentially expressed genes unique to temperature (22°C Veh vs. 32°C Veh, n = 12), number of differentially expressed genes unique to propranolol (22°C Propranolol vs. 32°C Veh, n = 31) and number shared by temperature and propranolol (n = 15). The gene list for differentially expressed genes is shown in the accompanying Table. Shared genes are bolded.
In addition to the panel of 84 genes described above, we determined the effect of housing temperature and propranolol treatment on expression of two additional β-adrenergic receptor subtypes (Adrb1 and Adrb3) and two genes coding for proteins related to bone formation, osteocalcin (Bglap) and alkaline phosphatase (Alpl). Mice housed at 22°C had lower levels of Adrb3 (−1.8; p < 0.002), Bglap (−1.3; p < 0.03) and Alpl (−1.4; p < 0.003) compared to mice housed at 32°C but no difference in expression of Adrb1. Propranolol treatment of mice housed at 22°C did not influence expression of Bglap, Alpl, or Adrb1. Expression levels of Adrb3 did not differ between propranolol-treated mice housed at 22°C and vehicle-treated mice housed at 32°C.
Discussion
Compared to thermoneutral temperature (32°C), mice housed at room temperature (22°C) had lower percent body fat, lower abdominal WAT, lower cancellous bone volume fraction, connectivity density, trabecular thickness, trabecular number, osteoblast-lined bone perimeter, and bMAT (adipocyte area fraction and adipocyte density) in distal femur metaphysis, lower leptin and osteocalcin in serum, and lower mRNA levels for osteocalcin and alkaline phosphatase in tibia. In contrast, osteoclast-lined bone perimeter and trabecular separation were higher in room temperature-housed mice. Treatment with propranolol prevented housing temperature-associated differences in percent body fat, abdominal WAT, serum leptin, and distal femur metaphysis bMAT, but had no effect on bone response to housing temperature. Finally, there were shared as well as unique genes differentially regulated in response to room temperature housing and treatment with propranolol.
Housing of growing female and male mice at room temperature results in dramatic cancellous bone loss prior to skeletal maturity (5–7). The bone loss is not uniform, with more loss occurring in long bones than in lumbar vertebrae (7, 33) and in the distal femur, more loss occurring in metaphysis than in epiphysis (34). Importantly, housing mice at thermoneutrality prevents premature bone loss (6, 7). In female mice, changes in bMAT levels in response to differences in housing temperature follow a similar pattern in that the bMAT levels are lower in distal femur metaphysis of mice housed at room temperature (7). We did not measure bone marrow adiposity in lumbar vertebra or distal femur epiphysis because adipocytes are very uncommon at these sites in mice housed at either room or thermoneutral temperature. The lower levels of bMAT and lower levels of cancellous bone observed in female mice housed at room temperature appear consistent with a shared mechanism. However, a different response occurred in male mice; whereas premature cancellous bone loss was evident in males housed at room temperature, differences in bMAT were not observed (6). The divergent response of bMAT to room temperature-induced cold stress in female and male mice does not support a shared mechanism.
The quantity of bMAT in distal femur metaphysis is context-dependent and, as recently reviewed (35), influenced by numerous factors, including skeletal site, age, food availability, and housing temperature. Increased bMAT occurs with extremes in energy availability; obesity and rapid weight loss each result in increased bMAT. Treatment of rats with β-adrenergic agonists, such as isoproterenol, increased lipolysis in bMAT but to a lesser extent than inguinal WAT (36). Baek et al. (37) found that β-blockade using propranolol had no effect on bMAT in mice fed a normal diet but partially reduced increases in bMAT induced by both calorie restriction and calorie excess. Blocking β-adrenergic signaling in growing female rats fed a calorie-restricted diet attenuated reductions in circulating leptin, cancellous bone mass and increases in marrow adiposity. However, as was the case for mice fed a normal diet, propranolol treatment had no effect on bMAT in ad libitum-fed rats (19). Propranolol was shown to reduce dietary fat absorption and fat mass in mice fed high fat diet by suppressing expression of pancreatic lipase (26). In the present study, propranolol treatment prevented the differences in bMAT in distal femur metaphysis between female mice housed at room temperature and thermoneutral temperature.
We housed mice individually in the present study to facilitate measurement of food intake and to prevent huddling. Additionally, the mice were not offered material for nest building. These measures were important because mice employ huddling and nesting as strategies to decrease reliance on non-shivering thermogenesis when housed at sub-thermoneutral temperature (38). The 10°C higher housing temperature resulted in an impressive ~280% difference in cancellous bone volume fraction in distal femur metaphysis, a commonly evaluated skeletal site. While a detailed temperature response curve has yet to be established, the magnitude of observed change suggest even small differences in housing temperature, within or among vivariums, could influence results. Thus, factors with potential for altering thermoregulation (e.g., housing conditions or treatment) should be evaluated for their effect on bone mass, architecture, growth, and turnover.
The mechanisms mediating the impact of adaptive thermogenesis on bone metabolism during room temperature housing have received little attention. As anticipated, housing temperature strongly influenced gene expression levels for Ucp1 in BAT but the adaptive response to changes in housing temperature was not altered by treatment with propranolol. This finding is consistent with evidence that activation of Ucp1 gene expression by increased sympathetic outflow induced by cold stress is primarily mediated through β3 receptor signaling (39). Commonly referred to as a non-specific β-blocker, propranolol inhibits β1, β2 and β3 receptors. However, it is reported to be only a weak inhibitor of β3 at the dose used in the present study (40). This conclusion is supported by the lack of an effect of propranolol in the present study on Ucp1 expression in BAT of mice housed at either room temperature or at thermoneutral. Notably, while propranolol treatment resulted in higher trabecular number in distal femur metaphysis, this effect was not influenced by temperature. Thus, it is unlikely that room temperature-induced bone loss requires β1 and β2 receptor signaling. It is interesting to note that room temperature housing resulted in lower mRNA levels for β3 receptor in tibia and that this response was blocked by treatment with propranolol. Further studies will be required to determine whether β3 receptor signaling contributes to bone loss in room temperature-housed mice.
Studies performed in Ucp1 knockout mice suggest that Ucp1 expression has a protective effect on bone during cold stress induced by sub-thermoneutral temperature housing (41). However, deletion of Ucp1 increases activation of alternative forms of thermogenesis to maintain energy homeostasis, including increased shivering, in mice housed at room temperature. Because of the increased energy cost to activate alternative forms of adaptive thermogenesis, Ucp1 knockout mice are resistant to diet-induced obesity when housed at room temperature. In contrast, when housed at thermoneutrality, Ucp1 knockout mice have increased sensitivity to diet-induced obesity (42, 43). The fundamental difference in thermoregulation between mice and humans and the growing number of examples that housing temperature influences experimental outcomes (3, 44–59) argue strongly that thermoneutral housing of mice more accurately reflects the thermal environment in humans and preclinical studies performed in mice should be conducted at housing temperatures that minimize cold stress.
Propranolol treatment is associated with increased body weight in some individuals (22, 23, 60) and deletion of β1, β2, and β3 receptors in mice increases sensitivity to diet-induced weight gain (61). Adaptive thermogenesis in response to cold stress is energy demanding; in the present study, mice housed at room temperature required ~90% more energy to match the growth rate of mice housed at thermoneutrality. Although body weight did not differ, there were differences in body composition and serum leptin levels. Specifically, mice housed at room temperature had lower percent body mass as adipose tissue, lower abdominal WAT, and not surprisingly, lower serum leptin levels. Treatment with propranolol had no effect on weight but prevented the temperature-associated changes in body composition, potentially by inhibiting expression of pancreatic lipase as well as suppressing β-adrenergic signaling in bone.
Some, but not all, studies report that propranolol influences bone growth, mass and turnover in mice and rats. Factors such as gonadal status, mechanical loading and energy balance appear to influence the skeletal response to treatment with propranolol (18, 19, 21). In the present study, propranolol-treated mice exhibited small but significant changes in bone microarchitecture; trabecular number was increased and trabecular separation decreased. In contrast to adipose tissues, propranolol did not blunt the dramatic differences in bone mass, osteoblast-lined and osteoclast-lined bone perimeters, or expression levels for osteocalcin and alkaline phosphatase in long bones between mice housed at room temperature and thermoneutral temperature. These findings provide additional strong evidence that the mechanisms responsible for adipose and skeletal adaptations to cold stress differ. Elevated glucocorticoid and thyroid hormone levels, and decreased leptin levels may contribute to the rapid cancellous bone loss observed in male and female mice housed at room temperature. Glucocorticoids, thyroid hormone and leptin are important regulators of bone metabolism and their levels are altered by housing temperature (62–69). Further research is required to establish the role of changes in these hormones in premature cancellous bone loss in mice housed at sub-thermoneutral temperatures.
As previously indicated, sensory and sympathetic nerve fibers innervate bone (70, 71) and adrenoceptors, and receptors for vasointestinal peptide, substance P and calcitonin gene-related peptide are expressed on cells located within bone marrow, including osteoblasts, osteoclasts, chondrocytes, and their precursors (72–74). Cells within skeletal tissue also synthesize neuropeptides. Thus, cells in bone marrow are well positioned to respond to circulating as well as locally-produced neuropeptides whose levels are influenced by temperature. However, no change was observed in Ucp1 gene expression in bone marrow (data not shown), a finding that contrasts with the increase in Ucp1 gene expression in brown fat in room temperature-housed mice. Additionally, the decreases in cancellous bone mass and bone formation rate in room temperature-housed mice were associated with lower leptin, an adipokine known to increase sympathetic tone in mice (75–77). These findings do not preclude a role for sympathetic outflow in mediating the osteopenic effects of cold stress induced by room temperature housing but rather suggest they are unlikely to be mediated through β1 and β2 receptor signaling (78).
We evaluated expression levels for genes associated with adipogenesis and adipocyte function (using a PCR microarray), β-adrenergic receptors, and bone formation. These analyses identified genes in tibia of mice housed at room temperature in the presence or absence of propranolol that were differentially expressed compared to mice housed at thermoneutrality. These data reflect the product of number of cells within the tissue expressing a gene and expression level/cell and provide a broad-based index of the magnitude of change resulting from differences in housing temperature and treatment. In addition to genes exclusive to housing temperature (n = 12) and propranolol (n = 31), there were genes differentially expressed and changed in the same direction by both interventions (n = 14). One shared gene changed in opposite direction. Taken together, these findings further support the conclusion that β1 and/or β2 signaling contribute(s) to the changes in bMAT associated with adaptation to cold temperature stress. Based on our initial screen, it is notable that propranolol altered the effect of room temperature housing on expression levels of Adipoq, Lep, Klf2, and Klf15, genes whose protein products play important roles in adipogenesis (79–81). Specifically, these genes were no longer differentially expressed in response to room temperature housing. The expression level of the gene for acetyl-CoA carboxylase (Acacb), the rate-limiting step for fatty acid synthesis and key regulator of β-oxidation (82), was higher in mice housed at room temperature and lower in propranolol-treated mice, findings consistent with propranolol attenuating the lower bMAT in room temperature-housed mice. It is also of interest that propranolol treatment resulted in differential expression of a large number of genes not influenced by housing temperature. Bone marrow is a complex organ system and β-adrenergic receptors are not limited to mesenchymal cell lineages (83). Thus, the antagonistic effects of propranolol on the adaptive response of bMAT to changes in housing temperature could be due a combination of direct effects on β-adrenergic signaling by adipocyte progenitors and/or adipocytes and indirect effects mediated through β-adrenergic signaling by cells not related to adipocytes (e.g., hematopoietic lineage cells). Additional research is required to decipher the precise contribution of target cells and individual signaling pathways.
In summary, the lower percent body fat and abdominal WAT weight and higher Ucp1 gene expression in BAT in room temperature-housed mice, compared to mice housed at thermoneutrality, is consistent with increased β-oxidation and increased non-shivering thermogenesis, respectively. Both mechanisms likely contribute to the temperature-associated differences in body composition. Our findings evaluating the effects of propranolol on mice housed at room temperature or thermoneutral suggest that β1/β2 receptor signaling contributes to lower WAT and bMAT levels in room temperature-housed mice. Osteoblasts and adipocytes share a common mesenchymal stem cell progenitor (84). However, failure of propranolol to attenuate the reduction in osteoblast-lined bone perimeter associated with room temperature cold stress does not support β1/β2 receptor signaling as a mechanism for premature bone loss associated with standard room temperature housing.
Data Availability Statement
All data for this study are included in the article. The datasets will also be deposited with NASA and made available to anyone upon request.
Author Contributions
RT, KP, AB, and UI: Conceptualization. KP, CW, AG, and RT: Data collection. AB: Data analysis. RT: Drafting manuscript. RT, KP, CW, AB, AG, and UI: Revising manuscript content. RT, KP, CW, AB, AG, and UI: Approving final version. UI: Takes responsibility for the integrity of the data.
Funding
This work was supported by NIH (AR 054609), USDA (38420-17804), and NASA (80NSSC19K0430).
Conflict of Interest
The authors declare that the research was conducted in the absence of any commercial or financial relationships that could be construed as a potential conflict of interest.
References
1. Gordon CJ. Thermal physiology of laboratory mice: defining thermoneutrality. J Therm Biol. (2012) 37:654–85. doi: 10.1016/j.jtherbio.2012.08.004
2. Schubert KA, Boerema AS, Vaanholt LM, de Boer SF, Strijkstra AM, Daan S. Daily torpor in mice: high foraging costs trigger energy saving hypothermia. Biol Lett. (2010) 6:132–5. doi: 10.1098/rsbl.2009.0569
3. Hylander BL, Eng JW, Repasky EA. The impact of housing temperature-induced chronic stress on preclinical mouse tumor models and therapeutic responses: an important role for the nervous system. Adv Exp Med Biol. (2017) 1036:173–89. doi: 10.1007/978-3-319-67577-0_12
4. Povinelli BJ, Kokolus KM, Eng JW, Dougher CW, Curtin L, Capitano ML, et al. Standard sub-thermoneutral caging temperature influences radiosensitivity of hematopoietic stem and progenitor cells. PLoS ONE. (2015) 10:e0120078. doi: 10.1371/journal.pone.0120078
5. Glatt V, Canalis E, Stadmeyer L, Bouxsein ML. Age-related changes in trabecular architecture differ in female and male C57BL/6J mice. J Bone Miner Res. (2007) 22:1197–207. doi: 10.1359/jbmr.070507
6. Martin SA, Philbrick KA, Wong CP, Olson DA, Branscum AJ, Jump DB, et al. Thermoneutral housing attenuates premature cancellous bone loss in male C57BL/6J mice. Endocr Connect. (2019) 8:1455–67. doi: 10.1530/EC-19-0359
7. Iwaniec UT, Philbrick KA, Wong CP, Gordon JL, Kahler-Quesada AM, Olson DA, et al. Room temperature housing results in premature cancellous bone loss in growing female mice: implications for the mouse as a preclinical model for age-related bone loss. Osteoporos Int. (2016) 27:3091–101. doi: 10.1007/s00198-016-3634-3
8. Burkhardt R, Kettner G, Bohm W, Schmidmeier M, Schlag R, Frisch B, et al. Changes in trabecular bone, hematopoiesis and bone marrow vessels in aplastic anemia, primary osteoporosis, and old age: a comparative histomorphometric study. Bone. (1987) 8:157–64. doi: 10.1016/8756-3282(87)90015-9
9. Gimble JM, Robinson CE, Wu X, Kelly KA, Rodriguez BR, Kliewer SA, et al. Peroxisome proliferator-activated receptor-gamma activation by thiazolidinediones induces adipogenesis in bone marrow stromal cells. Mol Pharmacol. (1996) 50:1087–94.
10. Justesen J, Stenderup K, Ebbesen EN, Mosekilde L, Steiniche T, Kassem M. Adipocyte tissue volume in bone marrow is increased with aging and in patients with osteoporosis. Biogerontology. (2001) 2:165–71. doi: 10.1023/A:1011513223894
11. Meunier P, Aaron J, Edouard C, Vignon G. Osteoporosis and the replacement of cell populations of the marrow by adipose tissue. A quantitative study of 84 iliac bone biopsies. Clin Orthop Relat Res. (1971) 80:147–54. doi: 10.1097/00003086-197110000-00021
12. Moerman EJ, Teng K, Lipschitz DA, Lecka-Czernik B. Aging activates adipogenic and suppresses osteogenic programs in mesenchymal marrow stroma/stem cells: the role of PPAR-gamma2 transcription factor and TGF-beta/BMP signaling pathways. Aging Cell. (2004) 3:379–89. doi: 10.1111/j.1474-9728.2004.00127.x
13. Nuttall ME, Patton AJ, Olivera DL, Nadeau DP, Gowen M. Human trabecular bone cells are able to express both osteoblastic and adipocytic phenotype: implications for osteopenic disorders. J Bone Miner Res. (1998) 13:371–82. doi: 10.1359/jbmr.1998.13.3.371
14. Rodriguez JP, Garat S, Gajardo H, Pino AM, Seitz G. Abnormal osteogenesis in osteoporotic patients is reflected by altered mesenchymal stem cells dynamics. J Cell Biochem. (1999) 75:414–23.
15. Rosen CJ, Ackert-Bicknell C, Rodriguez JP, Pino AM. Marrow fat and the bone microenvironment: developmental, functional, and pathological implications. Crit Rev Eukaryot Gene Expr. (2009) 19:109–24. doi: 10.1615/CritRevEukarGeneExpr.v19.i2.20
16. Hill EL, Turner R, Elde R. Effects of neonatal sympathectomy and capsaicin treatment on bone remodeling in rats. Neuroscience. (1991) 44:747–55. doi: 10.1016/0306-4522(91)90094-5
17. Bonnet N, Beaupied H, Vico L, Dolleans E, Laroche N, Courteix D, et al. Combined effects of exercise and propranolol on bone tissue in ovariectomized rats. J Bone Miner Res. (2007) 22:578–88. doi: 10.1359/jbmr.070117
18. Baek K, Bloomfield SA. Beta-adrenergic blockade and leptin replacement effectively mitigate disuse bone loss. J Bone Miner Res. (2009) 24:792–9. doi: 10.1359/jbmr.081241
19. Baek K, Bloomfield SA. Blocking beta-adrenergic signaling attenuates reductions in circulating leptin, cancellous bone mass, and marrow adiposity seen with dietary energy restriction. J Appl Physiol. (2012) 113:1792–801. doi: 10.1152/japplphysiol.00187.2012
20. Narkiewicz K, Kjeldsen SE, Oparil S, Hedner T. Beta-blockers as sub-optimal treatment for hypertension: time for first-line therapy revision? Blood Press. (2006) 15:323–4. doi: 10.1080/08037050601164986
21. Sliwinski L, Folwarczna J, Pytlik M, Cegiela U, Nowinska B, Trzeciak H, et al. Do effects of propranolol on the skeletal system depend on the estrogen status? Pharmacol Rep. (2013) 65:1345–56. doi: 10.1016/S1734-1140(13)71493-2
22. Martinez-Mir I, Navarro-Badenes J, Palop V, Morales-Olivas FJ, Rubio E. Weight gain induced by long-term propranolol treatment. Ann Pharmacother. (1993) 27:512. doi: 10.1177/106002809302700423
23. Sharma AM, Pischon T, Hardt S, Kunz I, Luft FC. Hypothesis: beta-adrenergic receptor blockers and weight gain: a systematic analysis. Hypertension. (2001) 37:250–4. doi: 10.1161/01.HYP.37.2.250
24. Morrison SF. Efferent neural pathways for the control of brown adipose tissue thermogenesis and shivering. Handb Clin Neurol. (2018) 156:281–303. doi: 10.1016/B978-0-444-63912-7.00017-5
25. Asai K, Yang GP, Geng YJ, Takagi G, Bishop S, Ishikawa Y, et al. Beta-adrenergic receptor blockade arrests myocyte damage and preserves cardiac function in the transgenic G(salpha) mouse. J Clin Invest. (1999) 104:551–8. doi: 10.1172/JCI7418
26. Baek K, Park D, Hwang HR, Kim SG, Lee H, Baek JH. Blocking beta(1)/beta(2)-adrenergic signaling reduces dietary fat absorption by suppressing expression of pancreatic lipase in high fat-fed mice. Int J Mol Sci. (2018) 19:E857. doi: 10.3390/ijms19030857
27. Kroeger RJ, Groszmann RJ. Effect of selective blockade of beta 2-adrenergic receptors on portal and systemic hemodynamics in a portal hypertensive rat model. Gastroenterology. (1985) 88:896–900. doi: 10.1016/S0016-5085(85)80005-6
28. Iwaniec UT, Wronski TJ, Turner RT. Histological analysis of bone. Methods Mol Biol. (2008) 447:325–41. doi: 10.1007/978-1-59745-242-7_21
29. Turner RT, Kalra SP, Wong CP, Philbrick KA, Lindenmaier LB, Boghossian S, et al. Peripheral leptin regulates bone formation. J Bone Miner Res. (2013) 28:22–34. doi: 10.1002/jbmr.1734
30. Menagh PJ, Turner RT, Jump DB, Wong CP, Lowry MB, Yakar S, et al. Growth hormone regulates the balance between bone formation and bone marrow adiposity. J Bone Miner Res. (2010) 25:757–68. doi: 10.1359/jbmr.091015
31. Dempster DW, Compston JE, Drezner MK, Glorieux FH, Kanis JA, Malluche H, et al. Standardized nomenclature, symbols, and units for bone histomorphometry: a 2012 update of the report of the ASBMR Histomorphometry Nomenclature Committee. J Bone Miner Res. (2013) 28:2–17. doi: 10.1002/jbmr.1805
32. Benjamini Y, Hochberg Y. Controlling the false discovery rate: a practical and powerful approach to multiple testing. J R Stat Soc Ser B. (1995) 57:289–300. doi: 10.1111/j.2517-6161.1995.tb02031.x
33. Rickard DJ, Iwaniec UT, Evans G, Hefferan TE, Hunter JC, Waters KM, et al. Bone growth and turnover in progesterone receptor knockout mice. Endocrinology. (2008) 149:2383–90. doi: 10.1210/en.2007-1247
34. Philbrick KA, Martin SA, Colagiovanni AR, Branscum AJ, Turner RT, Iwaniec UT. Effects of hypothalamic leptin gene therapy on osteopetrosis in leptin-deficient mice. J Endocrinol. (2018) 236:57–68. doi: 10.1530/JOE-17-0524
35. Turner RT, Martin SA, Iwaniec UT. Metabolic coupling between bone marrow adipose tissue and hematopoiesis. Curr Osteoporos Rep. (2018) 16:95–104. doi: 10.1007/s11914-018-0422-3
36. Scheller EL, Khandaker S, Learman BS, Cawthorn WP, Anderson LM, Pham HA, et al. Bone marrow adipocytes resist lipolysis and remodeling in response to beta-adrenergic stimulation. Bone. (2019) 118:32–41. doi: 10.1016/j.bone.2018.01.016
37. Baek K, Park HJ, Hwang HR, Baek JH. Propranolol attenuates calorie restriction- and high calorie diet-induced bone marrow adiposity. BMB Rep. (2014) 47:587–92. doi: 10.5483/BMBRep.2014.47.10.176
38. Gordon CJ, Aydin C, Repasky EA, Kokolus KM, Dheyongera G, Johnstone AF. Behaviorally mediated, warm adaptation: a physiological strategy when mice behaviorally thermoregulate. J Therm Biol. (2014) 44:41–6. doi: 10.1016/j.jtherbio.2014.06.006
39. Klaus S, Muzzin P, Revelli JP, Cawthorne MA, Giacobino JP, Ricquier D. Control of beta 3-adrenergic receptor gene expression in brown adipocytes in culture. Mol Cell Endocrinol. (1995) 109:189–95. doi: 10.1016/0303-7207(95)03502-X
40. Cannon B, Nedergaard J. Brown adipose tissue: function and physiological significance. Physiol Rev. (2004) 84:277–359. doi: 10.1152/physrev.00015.2003
41. Nguyen AD, Lee NJ, Wee NKY, Zhang L, Enriquez RF, Khor EC, et al. Uncoupling protein-1 is protective of bone mass under mild cold stress conditions. Bone. (2018) 106:167–78. doi: 10.1016/j.bone.2015.05.037
42. Enerback S, Jacobsson A, Simpson EM, Guerra C, Yamashita H, Harper ME, et al. Mice lacking mitochondrial uncoupling protein are cold-sensitive but not obese. Nature. (1997) 387:90–4. doi: 10.1038/387090a0
43. Liu X, Rossmeisl M, McClaine J, Riachi M, Harper ME, Kozak LP. Paradoxical resistance to diet-induced obesity in UCP1-deficient mice. J Clin Invest. (2003) 111:399–407. doi: 10.1172/JCI200315737
44. Clayton ZS, McCurdy CE. Short-term thermoneutral housing alters glucose metabolism and markers of adipose tissue browning in response to a high-fat diet in lean mice. Am J Physiol Regul Integr Comp Physiol. (2018) 315:R627–37. doi: 10.1152/ajpregu.00364.2017
45. Dickson I. NAFLD: thermoneutral housing of mice improves modelling of NAFLD. Nat Rev Gastroenterol Hepatol. (2017) 14:451. doi: 10.1038/nrgastro.2017.90
46. do Carmo JM, da Silva AA, Romero DG, Hall JE. Changes in ambient temperature elicit divergent control of metabolic and cardiovascular actions by leptin. FASEB J. (2017) 31:2418–28. doi: 10.1096/fj.201601224R
47. Fischer AW, Cannon B, Nedergaard J. Optimal housing temperatures for mice to mimic the thermal environment of humans: an experimental study. Mol Metab. (2018) 7:161–70. doi: 10.1016/j.molmet.2017.10.009
48. Ganeshan K, Chawla A. Warming the mouse to model human diseases. Nat Rev Endocrinol. (2017) 13:458–65. doi: 10.1038/nrendo.2017.48
49. Giles DA, Moreno-Fernandez ME, Stankiewicz TE, Graspeuntner S, Cappelletti M, Wu D, et al. Thermoneutral housing exacerbates nonalcoholic fatty liver disease in mice and allows for sex-independent disease modeling. Nat Med. (2017) 23:829–38. doi: 10.1038/nm.4346
50. Giles DA, Ramkhelawon B, Donelan EM, Stankiewicz TE, Hutchison SB, Mukherjee R, et al. Modulation of ambient temperature promotes inflammation and initiates atherosclerosis in wild type C57BL/6 mice. Mol Metab. (2016) 5:1121–30. doi: 10.1016/j.molmet.2016.09.008
51. Keijer J, Li M, Speakman JR. What is the best housing temperature to translate mouse experiments to humans? Mol Metab. (2019) 25:16–76. doi: 10.1016/j.molmet.2019.04.001
52. Lee B, Kim G, Jo Y, Lee B, Shin YI, Hong C. Aquatic exercise at thermoneutral water temperature enhances antitumor immune responses. Immune Netw. (2019) 19:e10. doi: 10.4110/in.2019.19.e10
53. Ndongson-Dongmo B, Lang GP, Mece O, Hechaichi N, Lajqi T, Hoyer D, et al. Reduced ambient temperature exacerbates SIRS-induced cardiac autonomic dysregulation and myocardial dysfunction in mice. Basic Res Cardiol. (2019) 114:26. doi: 10.1007/s00395-019-0734-1
55. Qi Y, Purtell L, Fu M, Sengmany K, Loh K, Zhang L, et al. Ambient temperature modulates the effects of the Prader-Willi syndrome candidate gene Snord116 on energy homeostasis. Neuropeptides. (2017) 61:87–93. doi: 10.1016/j.npep.2016.10.006
56. Rubin RL. Mice housed at elevated vivarium temperatures display enhanced T-cell response and survival to Francisella tularensis. Comp Med. (2017) 67:491–7.
57. Small L, Gong H, Yassmin C, Cooney GJ, Brandon AE. Thermoneutral housing does not influence fat mass or glucose homeostasis in C57BL/6 mice. J Endocrinol. (2018) 239:313–24. doi: 10.1530/JOE-18-0279
58. Stemmer K, Kotzbeck P, Zani F, Bauer M, Neff C, Muller TD, et al. Thermoneutral housing is a critical factor for immune function and diet-induced obesity in C57BL/6 nude mice. Int J Obes. (2015) 39:791–7. doi: 10.1038/ijo.2014.187
59. Uhlig S, Kuebler WM. Difficulties in modelling ARDS (2017 Grover Conference Series). Pulm Circ. (2018) 8:2045894018766737. doi: 10.1177/2045894018766737
60. Rossner S, Taylor CL, Byington RP, Furberg CD. Long term propranolol treatment and changes in body weight after myocardial infarction. BMJ. (1990) 300:902–3. doi: 10.1136/bmj.300.6729.902
61. Ueta CB, Fernandes GW, Capelo LP, Fonseca TL, Maculan FD, Gouveia CH, et al. beta(1) adrenergic receptor is key to cold- and diet-induced thermogenesis in mice. J Endocrinol. (2012) 214:359–65. doi: 10.1530/JOE-12-0155
62. Bassett JH, Williams GR. Role of thyroid hormones in skeletal development and bone maintenance. Endocr Rev. (2016) 37:135–87. doi: 10.1210/er.2015-1106
63. Canalis E, Delany AM. Mechanisms of glucocorticoid action in bone. Ann N Y Acad Sci. (2002) 966:73–81. doi: 10.1111/j.1749-6632.2002.tb04204.x
64. Coghlan MJ, Jacobson PB, Lane B, Nakane M, Lin CW, Elmore SW, et al. A novel antiinflammatory maintains glucocorticoid efficacy with reduced side effects. Mol Endocrinol. (2003) 17:860–9. doi: 10.1210/me.2002-0355
65. Gogakos AI, Duncan Bassett JH, Williams GR. Thyroid and bone. Arch Biochem Biophys. (2010) 503:129–36. doi: 10.1016/j.abb.2010.06.021
66. Iwen KA, Oelkrug R, Brabant G. Effects of thyroid hormones on thermogenesis and energy partitioning. J Mol Endocrinol. (2018) 60:R157–70. doi: 10.1530/JME-17-0319
67. Li L, Li B, Li M, Speakman JR. Switching on the furnace: regulation of heat production in brown adipose tissue. Mol Aspects Med. (2019) 68:60–73. doi: 10.1016/j.mam.2019.07.005
68. Philbrick KA, Wong CP, Branscum AJ, Turner RT, Iwaniec UT. Leptin stimulates bone formation in ob/ob mice at doses having minimal impact on energy metabolism. J Endocrinol. (2017) 232:461–74. doi: 10.1530/JOE-16-0484
69. Uchida K, Shiuchi T, Inada H, Minokoshi Y, Tominaga M. Metabolic adaptation of mice in a cool environment. Pflugers Arch. (2010) 459:765–74. doi: 10.1007/s00424-010-0795-3
70. Hill EL, Elde R. Distribution of CGRP-, VIP-, D beta H-, SP-, and NPY-immunoreactive nerves in the periosteum of the rat. Cell Tissue Res. (1991) 264:469–80. doi: 10.1007/BF00319037
71. Hohmann EL, Elde RP, Rysavy JA, Einzig S, Gebhard RL. Innervation of periosteum and bone by sympathetic vasoactive intestinal peptide-containing nerve fibers. Science. (1986) 232:868–71. doi: 10.1126/science.3518059
72. Aitken SJ, Landao-Bassonga E, Ralston SH, Idris AI. Beta2-adrenoreceptor ligands regulate osteoclast differentiation in vitro by direct and indirect mechanisms. Arch Biochem Biophys. (2009) 482:96–103. doi: 10.1016/j.abb.2008.11.012
73. Bliziotes M, Murtagh J, Wiren K. Beta-adrenergic receptor kinase-like activity and beta-arrestin are expressed in osteoblastic cells. J Bone Miner Res. (1996) 11:820–6. doi: 10.1002/jbmr.5650110613
74. Lai LP, Mitchell J. Beta2-adrenergic receptors expressed on murine chondrocytes stimulate cellular growth and inhibit the expression of Indian hedgehog and collagen type X. J Cell Biochem. (2008) 104:545–53. doi: 10.1002/jcb.21646
75. Belin de Chantemele EJ, Mintz JD, Rainey WE, Stepp DW. Impact of leptin-mediated sympatho-activation on cardiovascular function in obese mice. Hypertension. (2011) 58:271–9. doi: 10.1161/HYPERTENSIONAHA.110.168427
76. Enriori PJ, Sinnayah P, Simonds SE, Garcia Rudaz C, Cowley MA. Leptin action in the dorsomedial hypothalamus increases sympathetic tone to brown adipose tissue in spite of systemic leptin resistance. J Neurosci. (2011) 31:12189–97. doi: 10.1523/JNEUROSCI.2336-11.2011
77. Rahmouni K. Leptin-induced sympathetic nerve activation: signaling mechanisms and cardiovascular consequences in obesity. Curr Hypertens Rev. (2010) 6:104–209. doi: 10.2174/157340210791170994
78. Razzoli M, Emmett MJ, Lazar MA, Bartolomucci A. Beta-adrenergic receptors control brown adipose UCP-1 tone and cold response without affecting its circadian rhythmicity. FASEB J. (2018) 32:5640–6. doi: 10.1096/fj.201800452R
79. Lindenmaier LB, Philbrick KA, Branscum AJ, Kalra SP, Turner RT, Iwaniec UT. Hypothalamic leptin gene therapy reduces bone marrow adiposity in ob/ob mice fed regular and high-fat diets. Front Endocrinol. (2016) 7:110. doi: 10.3389/fendo.2016.00110
80. Pearson R, Fleetwood J, Eaton S, Crossley M, Bao S. Kruppel-like transcription factors: a functional family. Int J Biochem Cell Biol. (2008) 40:1996–2001. doi: 10.1016/j.biocel.2007.07.018
81. Yokota T, Meka CS, Medina KL, Igarashi H, Comp PC, Takahashi M, et al. Paracrine regulation of fat cell formation in bone marrow cultures via adiponectin and prostaglandins. J Clin Invest. (2002) 109:1303–10. doi: 10.1172/JCI0214506
82. Munday MR, Hemingway CJ. The regulation of acetyl-CoA carboxylase–a potential target for the action of hypolipidemic agents. Adv Enzyme Regul. (1999) 39:205–34. doi: 10.1016/S0065-2571(98)00016-8
83. Maestroni GJM. Adrenergic modulation of hematopoiesis. J Neuroimmune Pharmacol. (2019). doi: 10.1007/s11481-019-09840-7
Keywords: β-adrenergic, non-shivering thermogenesis, thermoneutral, cancellous bone, premature bone loss
Citation: Turner RT, Philbrick KA, Wong CP, Gamboa AR, Branscum AJ and Iwaniec UT (2020) Effects of Propranolol on Bone, White Adipose Tissue, and Bone Marrow Adipose Tissue in Mice Housed at Room Temperature or Thermoneutral Temperature. Front. Endocrinol. 11:117. doi: 10.3389/fendo.2020.00117
Received: 11 June 2019; Accepted: 21 February 2020;
Published: 17 March 2020.
Edited by:
Nathalie Bravenboer, VU University Medical Center, NetherlandsReviewed by:
Erica L. Scheller, Washington University in St. Louis, United StatesOrmond A. MacDougald, University of Michigan, United States
Copyright © 2020 Turner, Philbrick, Wong, Gamboa, Branscum and Iwaniec. This is an open-access article distributed under the terms of the Creative Commons Attribution License (CC BY). The use, distribution or reproduction in other forums is permitted, provided the original author(s) and the copyright owner(s) are credited and that the original publication in this journal is cited, in accordance with accepted academic practice. No use, distribution or reproduction is permitted which does not comply with these terms.
*Correspondence: Urszula T. Iwaniec, dXJzenVsYS5pd2FuaWVjQG9yZWdvbnN0YXRlLmVkdQ==