- Brain Research Institute, Jeffrey Cheah School of Medicine and Health Sciences, Monash University Malaysia, Bandar Sunway, Malaysia
Substance P (SP) and neurokinin A (NKA), encoded by TAC1/Tac1 gene are members of the tachykinin family, which exert their neuromodulatory roles in vertebrate reproduction. In mammals, SP and NKA have been shown to regulate gonadotropin-releasing hormone (GnRH) and luteinizing hormone (LH) secretion via kisspeptin neurons. On the other hand, the role of SP/NKA in the regulation of reproduction in non-mammalian vertebrates is not well known. In the present study, we first localized expression of tac1 mRNA in the brain of male and female zebrafish, Danio rerio. Next, using an antibody against zebrafish tachykinin1 (Tac1), we examined the neural association of SP/NKA neural processes with GnRH3 neurons, and with kisspeptin (kiss2) neurons, in the brains of male and female zebrafish. In situ hybridization showed an apparent male-dominant tac1 expression in the ventral telencephalic area, the anterior and posterior parts of the parvocellular preoptic nucleus, and the suprachiasmatic nucleus. On the other hand, there was female-dominant tac1 expression in the ventral periventricular hypothalamus. Confocal images of double-labeled zebrafish Tac1 and GnRH3 showed associations between Tac1-immunoreactive processes and GnRH3 neurons in the ventral telencephalic area. In contrast, there was no apparent proximity of Tac1 processes to kiss2 mRNA-expressing neurons in the hypothalamus. Lastly, to elucidate possible direct action of SP/NKA on GnRH3 or Kiss2 neurons, expression of SP/NKA receptor, tacr1a mRNA was examined in regions containing GnRH3 or Kiss2 neurons by in situ hybridization. Expression of tacr1a mRNA was seen in several brain regions including the olfactory bulb, preoptic area and hypothalamus, where GnRH3 and Kiss2 cells are present. These results suggest that unlike in mammals, Tac1 may be involved in male reproductive functions via direct action on GnRH3 neurons but independent of kisspeptin in the zebrafish.
Introduction
Substance P (SP) and neurokinin A (NKA) are tachykinin peptides encoded by TAC1/Tac1 gene, which are involved in a variety of biological actions in the central nervous system such as pain transmission (1), emotional behavior (2, 3), learning and memory (4, 5). In mammals, the TAC1/Tac1 gene produces four different splicing variants (α-, β-, γ-, and δ-Tac1), which produce SP (encoded by α-, β-, γ-, and δ-Tac1), NKA (encoded by β- and γ-Tac1) and extended form of NKA peptide: neuropeptide K (NPK, encoded by β-Tac1) or neuropeptide gamma (NPγ, encoded by γ-Tac1) (6). Variant dependent expression of Tac1 has been observed in different tissues, whereby β-Tac1 and γ-Tac1 mRNA are most abundantly expressed in the central nervous system (7). In the guinea-pig and rats, SP and NKA are co-expressed in the spinal ganglion cells and primary sensory neurons (8–10). Further, their co-release from the spinal cord has been shown in the guinea-pig (11). These results suggest that SP and NKA are synthesized together and function as co-transmitters in the same presynaptic terminals. SP and NKA preferentially bind to tachykinin receptors, NK1 receptor and NK2 receptor, respectively. In the brain, NK1 receptor is widely distributed, while NK2 receptor is sparsely but widely distributed in the peripheral nervous system (12).
In some mammals, SP and NKA have also been demonstrated as regulators of reproductive functions. In rodents, SP has been shown to stimulate gonadotropin-releasing hormone (GnRH) secretion and GnRH-induced luteinizing hormone (LH) release via NK1 receptor (13–16). Similarly, high doses of an agonist for NKA receptor (NK2 receptor) has been shown to stimulate GnRH pulse generation and LH release (17). However, in mice, NK1 receptor is expressed in 20% of GnRH neurons, and NK2 receptor is absent in GnRH neurons (16, 18), suggesting that SP/NKA may modulate GnRH-LH release via multiple mechanisms. GnRH neurons are known to be regulated by kisspeptin (Kiss1) and its receptor GPR54 (Kiss1 receptor) signaling, particularly during the onset of puberty. Recently, SP and NKA have been shown to modulate Kiss1 neurons and kisspeptin release (16, 19–21). In rodents, SP activates Kiss1 neurons (19) and approximately half of the Kiss1 neurons express NK1 receptor (16). Furthermore, Tac1-gene knockout male mice exhibit delayed puberty onset (22). In mammals, kisspeptin co-regulates GnRH neurons, together with other neuropeptides such as neurokinin B and dynorphin (KNDy hypothesis) (23). Therefore, SP/NKA could act as a potential regulator of the KNDy system to control the onset of puberty, although this may not be the case in primates and ewes (24, 25). Interestingly, it is well documented that SP neurons exhibit male-dominant expression pattern in the posterior-medial amygdala and basal ganglia regions (26–31). In addition, male rats have significantly greater concentrations of SP than females in preoptic-hypothalamic regions and the anterior pituitary (28, 32, 33). Male-dominant sexually dimorphic expression of SP has also been demonstrated in the diencephalic nucleus mainly in gymnotiform electric fish, Apteronotus sp. In A. leptorhynchus, SP-immunoreactive cell bodies in the lateral hypothalamus and their fiber innervations of the preoptic-hypothalamic areas are more abundant in the male, while no sex difference in SP-binding sites has been observed (34, 35). However, so far, the sexually dimorphic expression of SP/NKA has not been reported in other fish species, except for one report in goldfish showing sexually dimorphic (female dominant) expression of tac1 mRNA in association with seasonal variations in the olfactory bulbar regions (36).
The role of SP/NKA in reproductive functions has also been demonstrated in non-mammalian vertebrates. In lizard, Podarcis sicula, SP has been shown to modulate brain aromatase activity, androgen and estrogen levels during reproductive behavior (37). In crested newt, Triturus carnifex, SP downregulates GnRH-induced LH secretion in cultured pituitary cells (38). In some teleosts, SP neurons directly innervate the pituitary (34, 39, 40). However, despite the evolutionarily conserved molecular structures of SP/NKA and their receptors, the role of SP/NKA signaling in reproductive functions has not been demonstrated in teleosts. In some teleosts, there are three GnRH (GnRH1, GnRH2, and GnRH3) (41, 42), while in others, there are two GnRH (GnRH2 and GnRH3) with multiple receptor types (43). In the zebrafish (Danio rerio), GnRH3 is the hypophysiotropic form since the ablation of GnRH3-expressing cells during early zebrafish development results in infertility (44). On the other hand, GnRH2 is involved in the regulation of food intake (45, 46). In addition, some teleosts possess multiple kisspeptin (Kiss1 and Kiss2) and their receptor types (43). In the brain of zebrafish, Kiss1 is predominantly expressed in the habenula which is involved in social functions, while Kiss2 present in the preoptic-hypothalamic regions regulates GnRH3-LH secretion (47, 48). In mammals, several morphological evidences show the central role of SP/NKA in the regulation of the gonadotropic axis. For example, SP immunoreactivity has been detected within Kiss1 neurons in the human infundibular nucleus (49). SP processes have been shown to make close apposition with kisspeptin (KNDy) neurons in the arcuate nucleus in rhesus monkey and goats (24, 50). In addition, Kiss1 and SP processes make occasional contacts with GnRH processes in the human postinfundibular eminence (51). However, neuronal and functional associations between SP/NKA and GnRH3 or Kiss2 remain unknown in non-mammalian vertebrates.
In the present study, we first examined the expression of tac1 mRNA in the male and female zebrafish brain to confirm if there is any sexual dimorphism in tac1 gene expression in the brain. Second, to visualize SP/NKA neural processes (dendrites, axons and axon terminals), we created an antibody against zebrafish prepro-tachykinin-1 (referred to here as Tac1). Using the zebrafish Tac1 antibody, we examined the association between SP/NKA neural processes and GnRH3 or Kiss2 neurons in the male and female brains of the zebrafish. Finally, we examined the expression of SP/NKA receptor (NK1 receptor, tacr1a) in the brain regions containing GnRH3 and Kiss2 neurons.
Material and Methods
Animals
Sexually mature (< 6 months) male and female zebrafish, Danio rerio, were maintained in freshwater aquaria at 27 ± 0.5°C under a controlled natural photo-regimen (14/10 h, light/dark). The fish were fed an Adult Zebrafish Diet (Zeigler, Gardners, PA, USA) twice daily. All experimental procedures were performed under the guidelines of the Animal Ethics Committee of Monash University (Approval number: MARP/2012/120).
In Situ Hybridization of tac1 mRNA
In situ hybridization was performed for the localization of tac1 mRNA expressing cell population as described previously (52). Sexually mature male and female zebrafish (n = 4) were anesthetized by immersion in 0.01% solution of 3-aminobenzoic acid ethyl ester methanesulfonate (MS-222; Sigma, St. Louis, MO) before decapitation. The brains were fixed in 4% paraformaldehyde buffered in 0.2 M phosphate buffer (pH7.5) for 6 h, cryoprotected in 20% sucrose and embedded in Tissue Tek OCT compound (Sakura Finetechnical, Tokyo, Japan). Coronal sections of male and female zebrafish brain (n = 4) (15 µm thickness) were cut using a cryostat and were thaw-mounted onto 3-aminopropylsilane-coated glass slides. The sections were permeabilized with 0.2 M HCl for 10 min and were treated with proteinase K (1 μg/ml) for 15 min at room temperature, pre-hybridized at 55°C for 2 h, and hybridized with digoxigenin (DIG)-labeled 342 nucleotides (nt) fragments of zebrafish tac1 riboprobe (50 ng/ml) covering 110-451 nt of zebrafish tac1 mRNA [GenBank accession number: NM_001256391.1, retrieved from the National Center for Biotechnology Information (NCBI)] at 55°C overnight in a humidified chamber. The sections were then washed and blocked with 2% normal sheep serum. The DIG-labeled probe was detected using an alkaline phosphatase-conjugated anti-DIG antibody (Roche Diagnostics, Mannheim, Germany; 1:500 dilution), and chromogenic reaction was developed using 4-nitroblue tetrazolium chloride/5-bromo-4-chloro-3-indolyl-phosphate (Roche Diagnostics).
Zebrafish Tac1 Antibody Creation
SP and NKA are encoded by the same gene, tac1, and they share a common carboxy-terminal amino acid sequence (Glu-Leu-Met) with other tachykinin family of peptides. Hence, some mammalian SP antibody may cross-react with other tachykinin peptides. Therefore, we created an antibody against preproTachyknin-1 (Tac1) to refer to SP and NKA peptides. An anti-zebrafish Tac1 polyclonal antiserum (Cat# PAS 15351/15352) was raised against a synthetic peptide (C-SYEWGTVQIYDKRRS) from the zebrafish tac1 by 1st BASE Sdn Bhd (Selangor, Malaysia). The peptide was conjugated with keyhole limpet hemocyanin (KLH) as a carrier molecule and immunized in rabbits. Following the final injection, blood was obtained, centrifuged and the affinity-purified serum was stored at -80°C. The specificity of the immunoreactivity of the anti-zebrafish Tac1 antiserum was confirmed by i) immunohistochemistry using the antibody pre-absorbed with 10 µg/ml antigen peptide (1st BASE) or KLH and ii) dot-blot assay. The patterns of zebrafish Tac1 immunoreactive cells were also compared to those of zebrafish tac1 mRNA expressing cells to validate the antibody specificity.
Dot Blot Analysis for Tac1 Antiserum
Cross reactivities of the Tac1 antiserum, produced in our laboratory, was analyzed by dot blot analysis. Antigens, zebrafish Tac1 peptide (C-SYEWGTVQIYDKRRS) and mammalian (human) SP peptide (RPKPQQFFGLM, CAS 33507-63-0, Calbiochem) (10 µg) were spotted on a polyvinylidene difluoride (PVDF) membrane (Hybond-P, Amersham Biosciences, Buckinghamshire, England) and dried by air. The PVDF membranes were blocked with Tris-buffered saline (pH 7.4) containing 1% Tween-20 (TBST) buffer containing 0.5% nonfat dry milk for 2 h at room temperature, and incubated with Tac1 antiserum (1:1000), rabbit anti-mammalian SP antibody (#20064, ImmunoStar, 1:1000) and Tac1 antiserum preabsorbed with Tac1 antigen peptide (10 µg/ml in a final concentration) for overnight at 4°C. The membranes were washed with TBST and incubated with horseradish peroxidase-conjugated donkey anti-rabbit IgG (Amersham Biosciences) for 1 h at room temperature. Immunoreactivity was detected with ECL Plus Western Blotting Detection Reagents (Amersham Biosciences) and visualized with Light-Capture System attached with a cool CCD camera (ATTO, Tokyo, Japan).
Immunohistochemistry for Tac1 in the Brain of Zebrafish
Immunohistochemistry was performed to examine the distribution of Tac1 in the brain of zebrafish. Coronal sections of male and female brains (n = 4) were pretreated with 0.3% hydrogen peroxide (H2O2) in methanol for 20 min followed by incubation in blocking solution containing 2% normal goat serum and 0.5% Triton X-100 in phosphate buffer saline (PBS) for 30 min at room temperature. The sections were then incubated with rabbit anti-zebrafish Tac1 antiserum (1:500 dilution) prepared in blocking solution overnight at 4°C in a moist chamber. The sections were incubated with biotinylated anti-rabbit IgG (1:200 dilution) (Vectastain ABC Elite kit, Vector Laboratories Cat# PK-6101) for 1 h followed by avidin-biotin peroxidase complex for 45 min at room temperature. Chromogenic development was achieved with 0.05% 3, 3’diaminobenzidine tetrahydrochloride with 0.03% H2O2 in 0.05 M Tris-HCl (pH 7.5). Upon development of staining, sections were dehydrated in a series of ethanol concentration followed by xylene and mounted with DPX mountant (Fisher Chemical, New Jersey, USA) and sealed with a coverslip.
Intracranial Administration of Colchicine
To enhance Tac1-immunoreactivity in female brains, female fish were intracranially administered with colchicine according to a previously reported procedure (53, 54). Fish were anesthetized as described above and a pinhole was made in the skull covering the telencephalic region by using a needle tip, and colchicine (0.5μg/g body weight) or water (n=3 for each treatment) in a volume of 1 µl was administered through a 35-G needle. The fish was individually placed in a recovery tank, and the brain was collected 24h after administration, and processed for immunohistochemistry for Tac1 as described above.
Double-Labeling for Tac1 Processes With GnRH3 and kiss2 Cells
The double–immunofluorescence for Tac1 processes with GnRH3 was conducted in male and female zebrafish. Sagittal brain sections of male and female zebrafish (n = 3; 15 µm thickness) were subjected to double-immunofluorescence of Tac1 and GnRH3. Sections were incubated with rabbit anti-zebrafish Tac1 antiserum (1:500 dilution) prepared in blocking solution overnight at 4°C in a moist chamber followed by development with Alexa Fluor 594-labeled anti-rabbit IgG (dilution of 1:500, Life Technologies Cat# A11037). Sections were then washed with 0.01 M PBS, incubated with mouse monoclonal anti-GnRH antibody [#LRH-13; dilution 1:2000; a gift from Prof Wakabayashi, Gunma University, Japan] and developed with Alexa Fluor 488-labeled anti-mouse IgG (Invitrogen, 1:500 dilution). The specificity of LRH-13 antibody has been characterized (55), and it has been widely used to detect GnRH3 type in various teleosts including zebrafish (56–60).
To examine the association between Tac1 processes and kiss2 cell soma, double-labeling combining immunofluorescence (Tac1) and fluorescence in situ hybridization (kiss2) was carried out in male and female brains (n=3). Briefly, coronal brain sections were subjected to DIG-in situ hybridization for kiss2 (47) as described above. Following hybridization, the sections were incubated with horseradish peroxidase-conjugated anti-DIG antibody (Roche Diagnostics, Cat# 11207733910) diluted 1:500 in Tris-NaCl-Tween (TNT) buffer consisting of 0.1M Tris-HCl (pH7.4), 0.15M NaCl and Triton X-100 with 1% normal goat serum for 2 h at room temperature. The DIG-labeled antibody was detected using Tyramide Signal Amplification (TSA) Plus Fluorescein kit (PerkinElmer/NEN Life Science Products, Wellesley, MA) by adding 1:50 dilution of reconstituted fluorescent tyramide to Plus amplification diluent (PerkinElmer/NEN Life Science Products). After the fluorescence in situ hybridization for kiss2 mRNA, immunofluorescence labeling for Tac1 was performed as described above. Sections were then mounted with VECTASHIELD mounting medium (Vector Laboratories, Burlingame, CA).
tacr1a mRNA Expression in the Brain Regions Containing GnRH3 and kiss2 Cells
To elucidate the possible expression of SP/NKA (NK1) receptor in GnRH3 neurons, expression of NK1 receptor (tacr1a-202) mRNA was examined in the brain regions containing GnRH3 (forebrain) or kiss2 neurons (hypothalamus) by DIG-in situ hybridization. In situ hybridization on sagittal (for GnRH3) or coronal (for kiss2) brain sections (n=3, males) were conducted using zebrafish tacr1a mRNA probes (520 nt, positioned 624-1144 nt, GenBank accession numbers: NM_001270478) as described above. Presence of GnRH3 and kiss2 cells were confirmed in the respective alternate consecutive serial sections by immunohistochemistry or in situ hybridization as described above.
Image Acquisition
For Tac1-immunostaining and tac1-in situ hybridization, the stained brain sections were scanned on a MIRAX MIDI Digital Slide scanner (Carl Zeiss, GmbH, Gottingen, Germany), and images were captured using the MIRAX Viewer Image Software (3DTech, Budapest, Hungary) at a 230 nm resolution with a 20× objective. Double-labeled fluorescence images were captured and analyzed using a confocal laser scanning microscope (C1si, Nikon Instruments) and two separate images were superimposed using computer software (NIS Elements BR, Nikon Instruments). The red channel was then converted to magenta, and brightness and contrast adjustments were made in Adobe Photoshop CC (Adobe, San Jose, CA, USA). Nomenclature for the zebrafish brain regions was adopted from (61–66).
Cell Counting and Statistical Analysis
For manual counting of DIG-labeled tac1 expressing cell numbers (n = 4 for males and females), an average of 4–5 consecutive sections/region for each sample were used. Numbers of tac1 cells were counted in regions showing apparent sexually dimorphic expression of tac1 including the anterior and posterior parts of the parvocellular preoptic nucleus, suprachiasmatic nucleus, ventral zone of the periventricular hypothalamus, and superior raphe. tac1 cells were also counted in regions showing no sexually dimorphic expression, which included the periventricular nucleus of the posterior tuberculum, dorsal zone of the periventricular hypothalamus, midbrain region, and the periventricular gray zone of the optic tectum.
For manual counting of GnRH3 cells numbers, associated with or without Tac1 processes (n = 3 for males and females), an average of 10 sections/region [the ventral nucleus of ventral telencephalic area (Vv) and olfactory-terminal nerve (OB-TN)] for each sample were used. The percentage of GnRH3-immunoreactive cells accompanied by Tac1 processes was calculated.
Close associations between Tac1 processes and GnRH3 cell soma were defined according to the procedure previously reported (67). Briefly, the confocal images were captured with a 60× water immersion objective (numerical aperture = 1.2) with an additional 9.9× optical zoom to give a final magnification of 594×, which yielded a voxel size of 0.08 μm. The close association was quantified by calculating the limit of the image by optical resolution using the wavelength formula λ/2 × (numerical aperture) and finally determining the pixel size of each cube that existed between the two labeled processes to confirm whether there was any blank pixel present between them (68).
A single-blind study was used to count the cell numbers. No sample calculation was performed to predetermine the sample size. All values are presented as means (± SEM). The mean difference of cell numbers or percentage of cells between males and females were analyzed by unpaired Student’s t-test and size effects are reported as Cohen’s d with the significance set at P < 0.05.
Results
Distribution of tac1 mRNA and Tac1 Immunoreactivity in the Male and Female Brain
In both males and females, the similar distribution pattern of tac1 mRNA expression was seen in the olfactory bulb, medial and posterior zones of the dorsal telencephalic area, dorsal habenula, optic tectum, ventral, dorsal and caudal zones of the periventricular hypothalamus, lateral hypothalamic nucleus, periventricular nucleus of the posterior tuberculum, nucleus of the medial longitudinal fascicle, oculomotor nerve nucleus, torus semicircularis, and interpeduncular nucleus (Figures 1–3). On the other hand, in some ventral diencephalic nuclei such as the anterior and posterior parts of the parvocellular preoptic nucleus (PPa/PPp), and suprachiasmatic nucleus (SC), abundant expression of tac1 mRNA was observed in only males but not in females (Figures 1–A, A’–C’, and 4A−D). This significant difference was also confirmed by quantitative analysis, which showed the significantly higher number of tac1 expressing cells in the PPa/PPp region (P=0.007, Cohen’s d = 4.201) and SC (P=0.002, Cohen’s d=5.665) in males as compared to females (Figures 4C, D, I). Similarly, male-dominant distribution of Tac1-immunoreactive cells was seen in the PPa (Figures 4A’, B’) but not in the PPp (Figures 4C’, D’). We also found male-dominant expression of tac1 mRNA and Tac1-immunoreactivities in the superior raphe (Figures 3J, J’ and 4G−H”). However, the difference in the superior raphe was not statistically significant (P=0.144, Cohen’s d=1.479; Figure 4I). We also noted that the number of tac1 expressing cells in the ventral zone of the periventricular hypothalamus was significantly higher in females as compared to males (P=0.001, Cohen’s d=6.368; Figures 1D, D’ and 4E, F, I). In addition, higher densities of Tac1-immunoreactive processes were also seen in the telencephalon, diencephalon, and in the hindbrain regions (Figures 5, 6). To enhance the immunoreactivity of Tac1 in female brains, Tac1-immunoreactivity were examined in the brain of female fish treated with colchicine. However, there was no difference in Tac1 immunoreactivities between colchicine- and water-treated brain (data not shown). Pre-absorption of the primary antibody with antigen peptides mostly eliminated immunoreactivity (Supplementary Figures 1A, B). Furthermore, the dot-blot assay for the Tac1 antibody showed immunoreactivity against Tac1 but not against SP (Supplementary Figure 1C), confirming the specificity of the Tac1 antibody to Tac1.
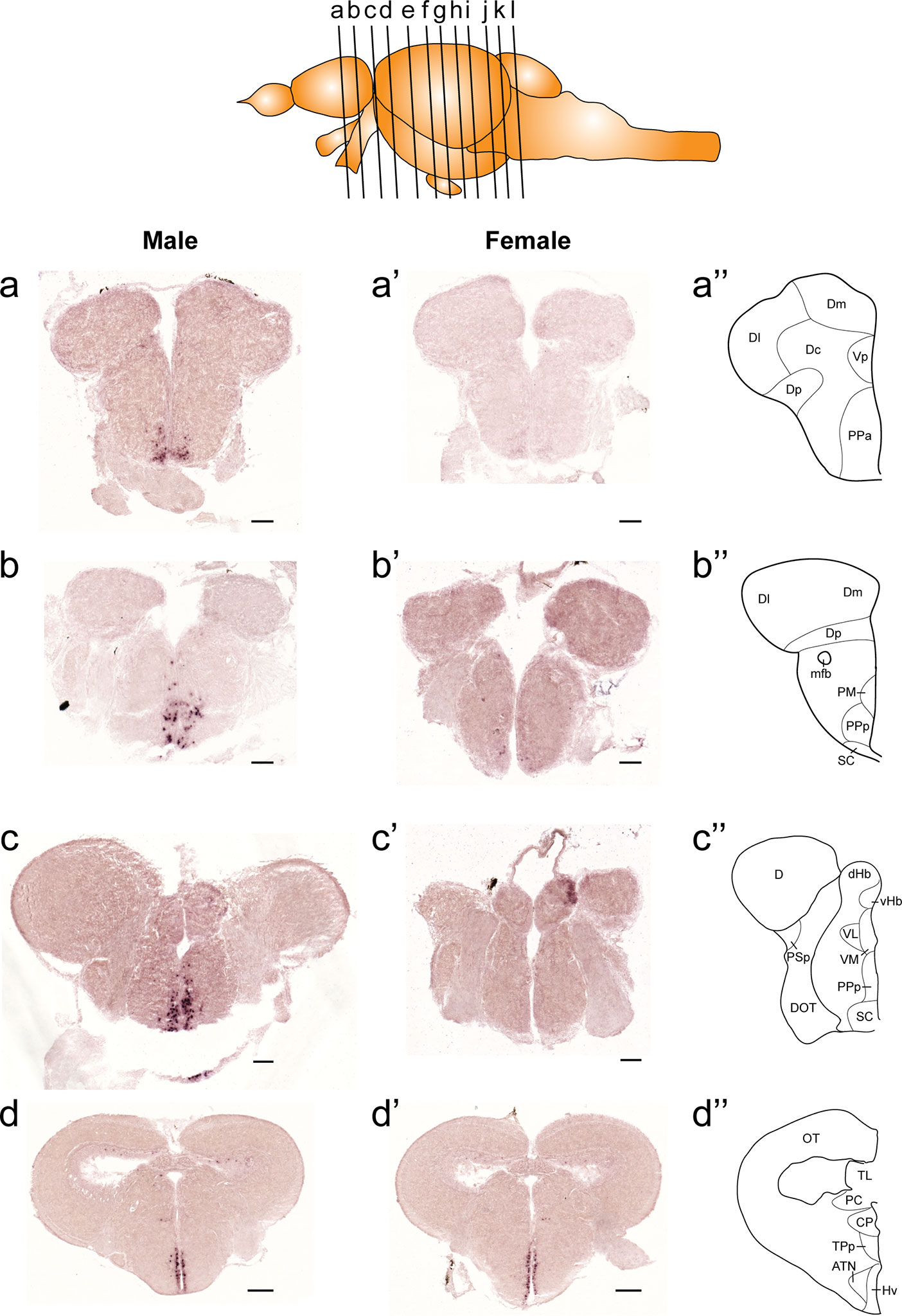
Figure 1 Comparison of expression patterns of tac1 mRNA in the forebrain regions of male (A−D) and female (A’−D’) zebrafish. In the top panel, a schematic drawing of an adult zebrafish brain (lateral view) showing levels of cross-sections presenting in Figures 1−3. Alphabets represent respective figure numbers (A−D: Figure 1, E−H: Figure 2, and I−L: Figure 3). In the most-right panels (A”−D”), a schematic drawing of half of the brain section (left side) processed for tac1 staining indicating major brain structures at this level. There was a sexually dimorphic expression of tac1 in the anterior part of the parvocellular preoptic nucleus (A, A’), posterior part of the parvocellular preoptic nucleus (B, B’), and suprachiasmatic nucleus (C, C’). In contrast, no sexually dimorphic expression of tac1 was observed in the dorsal habenula (C, C’) and the ventral region of the periventricular hypothalamus (D, D’). Scale bars: (A–C), (A’–C’), 100 µm; (D, D’), 200 µm. See Glossary for abbreviation.
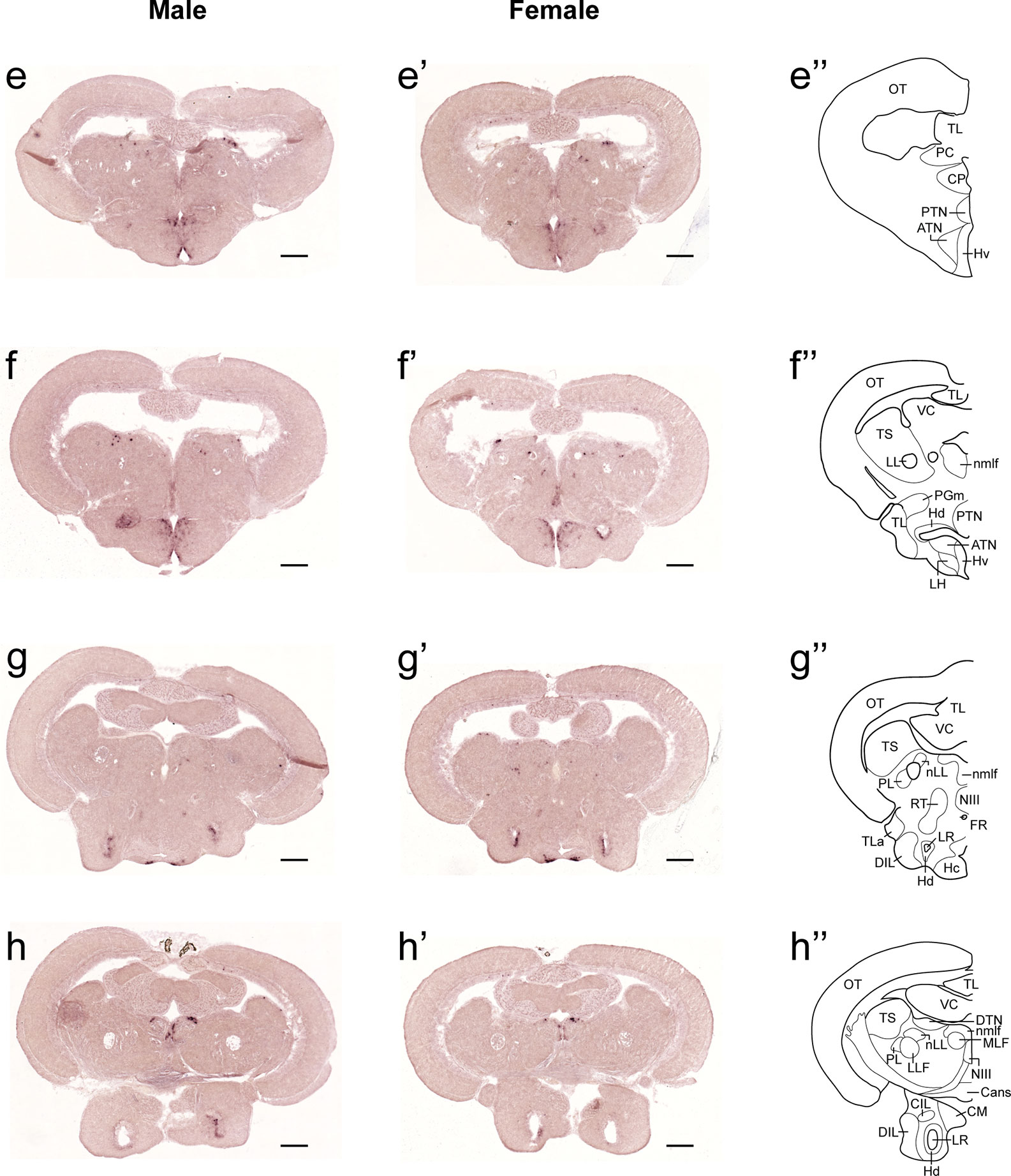
Figure 2 Comparison of expression patterns of tac1 mRNA in the midbrain regions of male (E−H) and female (E’−H’) zebrafish. In the most-right panels (E”−H”), a schematic drawing of half of the brain section (left side) processed for tac1 staining indicating major brain structures at this level. There was no sex difference in expression of tac1 in the diencephalic and mesencephalic regions including the torus semicircularis, dorsal tegmental nucleus, posterior tuberal nucleus, caudal and dorsal zones of the periventricular hypothalamus, nucleus of medial longitudinal fascicle, and oculomotor nucleus. Scale bars: 200 µm. See Glossary for abbreviation.
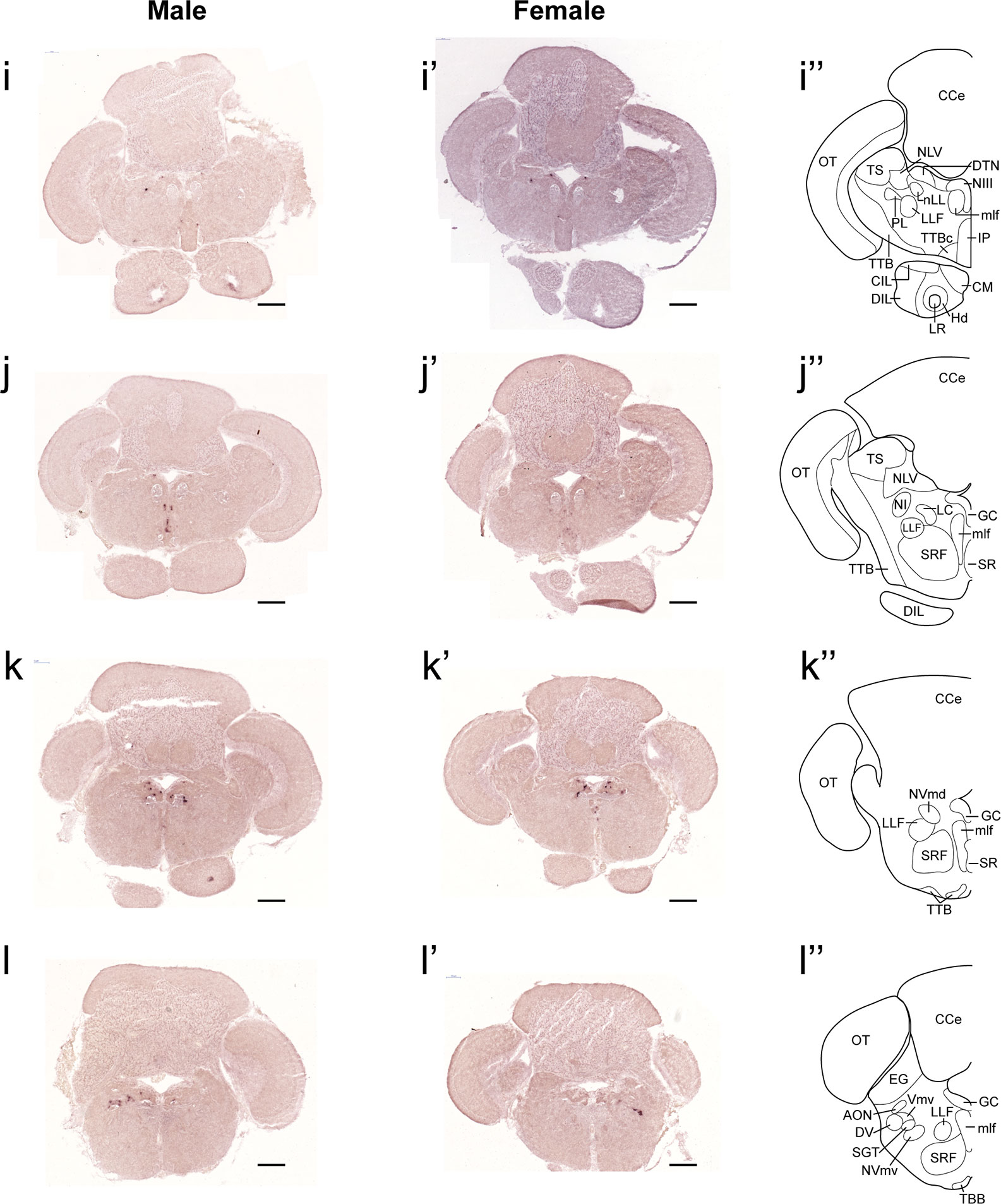
Figure 3 Comparison of expression patterns of tac1 mRNA in the hindbrain regions of male (I−L) and female (I’−L’) zebrafish. In the most-right panels (I”−L”), a schematic drawing of half of the brain section (left side) processed for tac1 staining indicating major brain structures at this level. There was no sex difference in expression of tac1 in the mesencephalic and rhombencephalic regions including the interpeduncular nucleus, dorsal tegmental nucleus, central gray, and trigeminal motor nucleus, while in the superior raphe, there was more tac1 expression in males as compared to females (J, J’). Scale bars: 200 µm. See glossary for abbreviation.
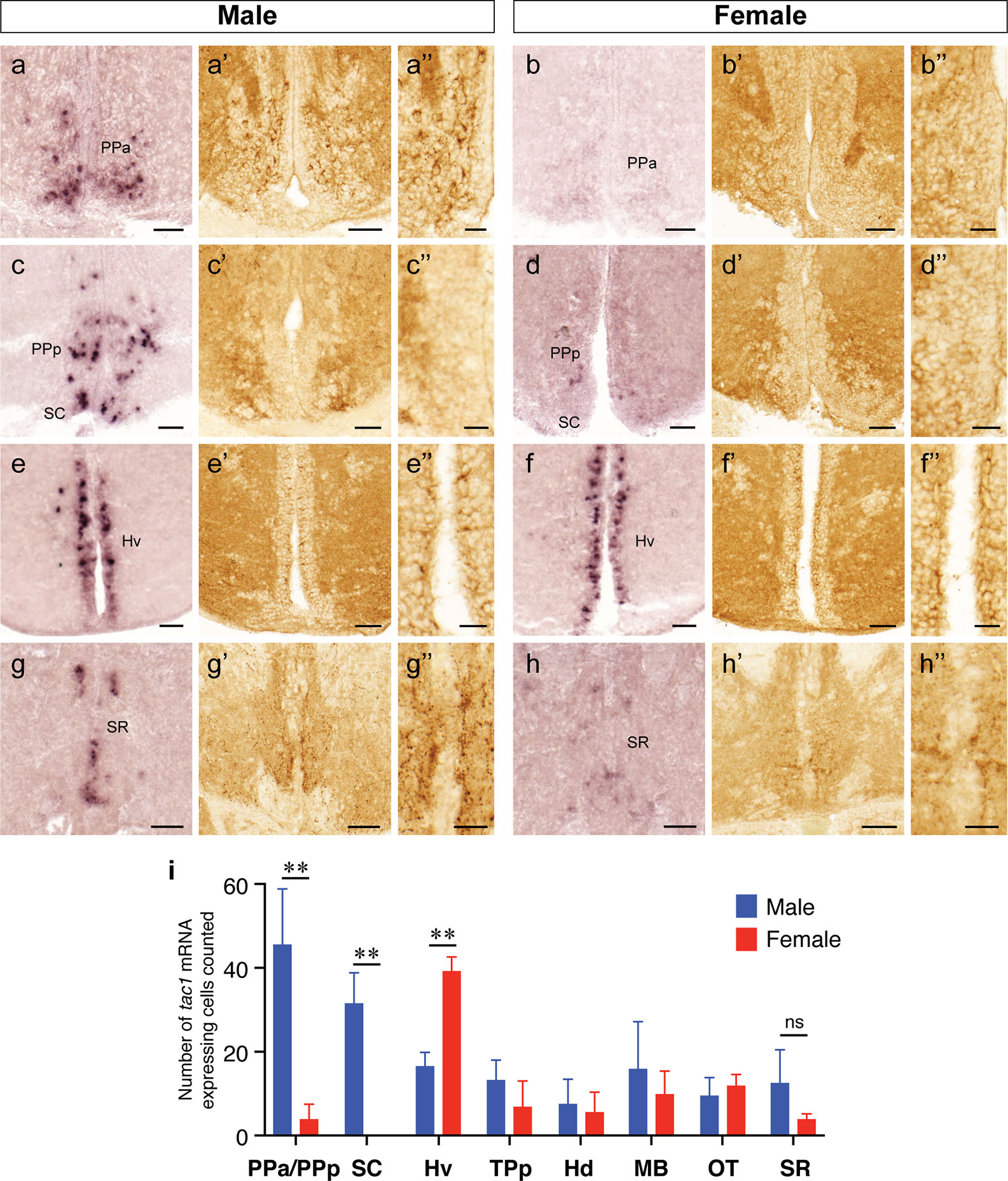
Figure 4 Sexually dimorphic expression of tac1 mRNA and Tac1 immunoreactivity in the brain. Photomicrographs in the left (A−H), middle (A’−H’) and the right (A”−H”) columns for each gender are tac1 mRNA, Tac1-immunoreactivity and a higher magnification of respective Tac1 immunoreactivities observed in the middle panels, respectively. Bar graphs in the bottom panel (I) represent the number of cells expressing tac1 counted in different brain regions of males (blue columns) and female (red columns) fish. In the anterior part of the parvocellular preoptic nucleus (PPa), there were male-dominant tac1 mRNA expression (A and B) and Tac1 immunoreactivity (A’ and B’). In the posterior part of the parvocellular preoptic nucleus (PPp) and suprachiasmatic nucleus (SC), tac1 mRNA expression was significantly higher in males (C and D), while no apparent sexual dimorphism was observed in Tac1 immunoreactivity (C’ and D’). In the ventral region of the periventricular hypothalamus (Hv), numbers of cells expressing tac1 mRNA were higher in females as compared to males (E, F, and I, P<0.01), while there was no apparent sexual morphism in Tac1 immunoreactivity (E’ and F’). In the superior raphe, more number of cells expressing tac1 mRNA were observed in males (G and G’) as compared to females (H and H’), but there was no significant difference (ns, I). Scale bars: A−H and A’−H’: 50 µm; A”−H”: 20 µm. **P<0.01. See Glossary for abbreviation.
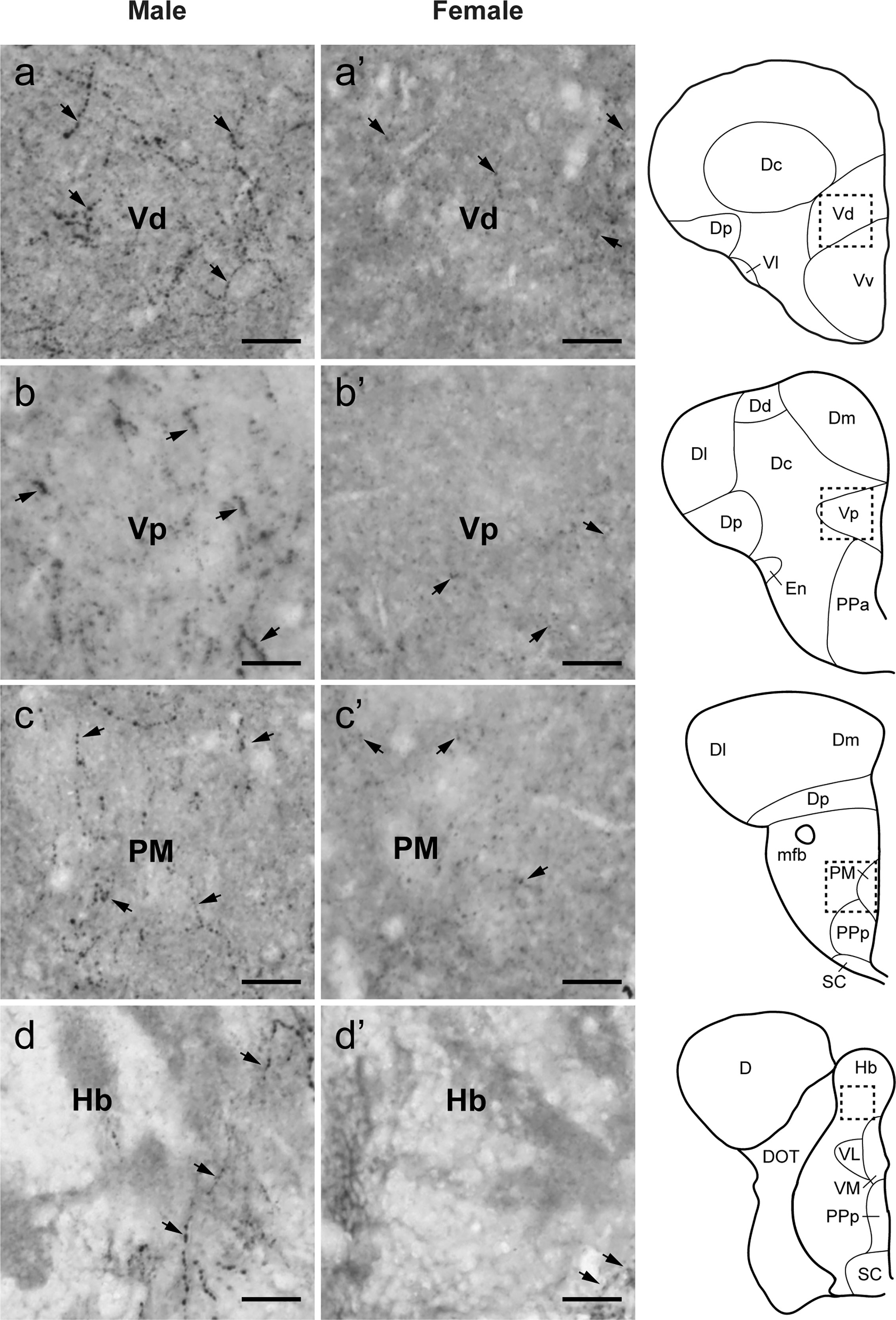
Figure 5 Sexual dimorphisms in Tac1-immunoreactive processes in the telencephalic and diencephalic regions. Left (A−D) and middle (A’−D’) columns represent photomicrographs of Tac1-immunoreactive processes (arrows) in the brain of males and females, respectively. Boxes with a dotted line in the schematic drawing of coronal view in the right column indicate the localization of the boundaries of the respective immunohistochemical illustrations. In the ventral part of the ventral telencephalon, more Tac1-immunoreactive processes are seen in male brains (A−C) as compared to females (A’−C’). Similarly, more Tac1-immunoreactive processes are observed in the habenula nuclei in males as compared to female (D, D’). Scale bars: 20 µm. See Glossary for abbreviation.
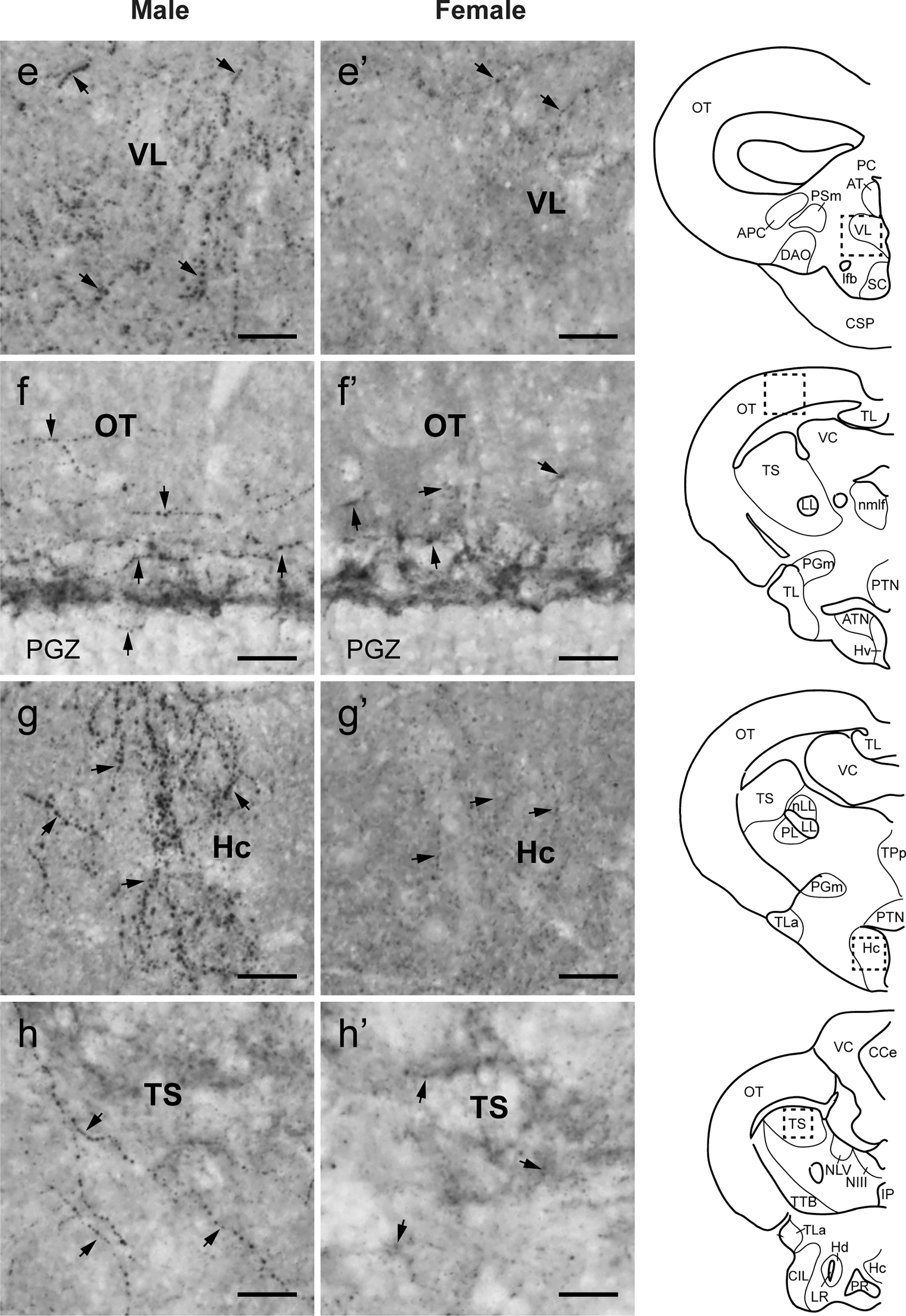
Figure 6 Comparison of Tac1-immunoreactive processes in the diencephalic and mesencephalic regions. Left (E−H) and middle (E’−H’) columns represent photomicrographs of Tac1-immunoreactive processes (arrows) in the brain of males and females, respectively. Boxes with a dotted line in the schematic drawing of coronal view in the right column indicate the localization of the boundaries of the respective immunohistochemical illustrations. In some diencephalic nuclei, more Tac1-immunoreactive processes are seen in male brains (E, G) as compared to females (E’, G’). In the mesencephalic nucleus, densities of Tac1-immunoreactive processes were slightly higher in males (F, H) as compared to females (F’, H’). Scale bars: 20 µm. See Glossary for abbreviation.
Association Between Tac1 Processes and GnRH3 or kiss2 Cells
To examine the potential association between Tac1-immunoreactive processes and GnRH3 or kiss2 neurons, double-labeling was performed in the brain of male and female zebrafish. Tac1-immunoreactive processes were closely located to GnRH3 cells in the ventral nucleus of ventral telencephalic area and the olfactory bulb in males (Figures 7A-A’”, B-B’”) and females (Figures 7C-C’”, D-D’”). In the ventral nucleus of ventral telencephalic area, the percentage of GnRH3 cells accompanied by Tac1 processes were higher in males (32/47 cells) as compared to females (12/26 cells), but it was statistically insignificant (P=0.203, Cohen’s d=1.243; Figure 7E). In the olfactory bulb, the percentage of GnRH3 cells accompanied by Tac1 processes were slightly higher in females (12/59 cells) as compared to males (12/113 cells), but it was statistically insignificant (P=0.093, Cohen’s d=1.792; Figure 7E). In the hypothalamic regions where kiss2 neurons are present, occasionally, there were Tac1-immunoreactive processes that were seen in proximity to kiss2 cells in males and females (Figure 8), but the densities of Tac1 processes were very subtle and they do not seem to form close associations based on the results of confocal scanning (data not shown).
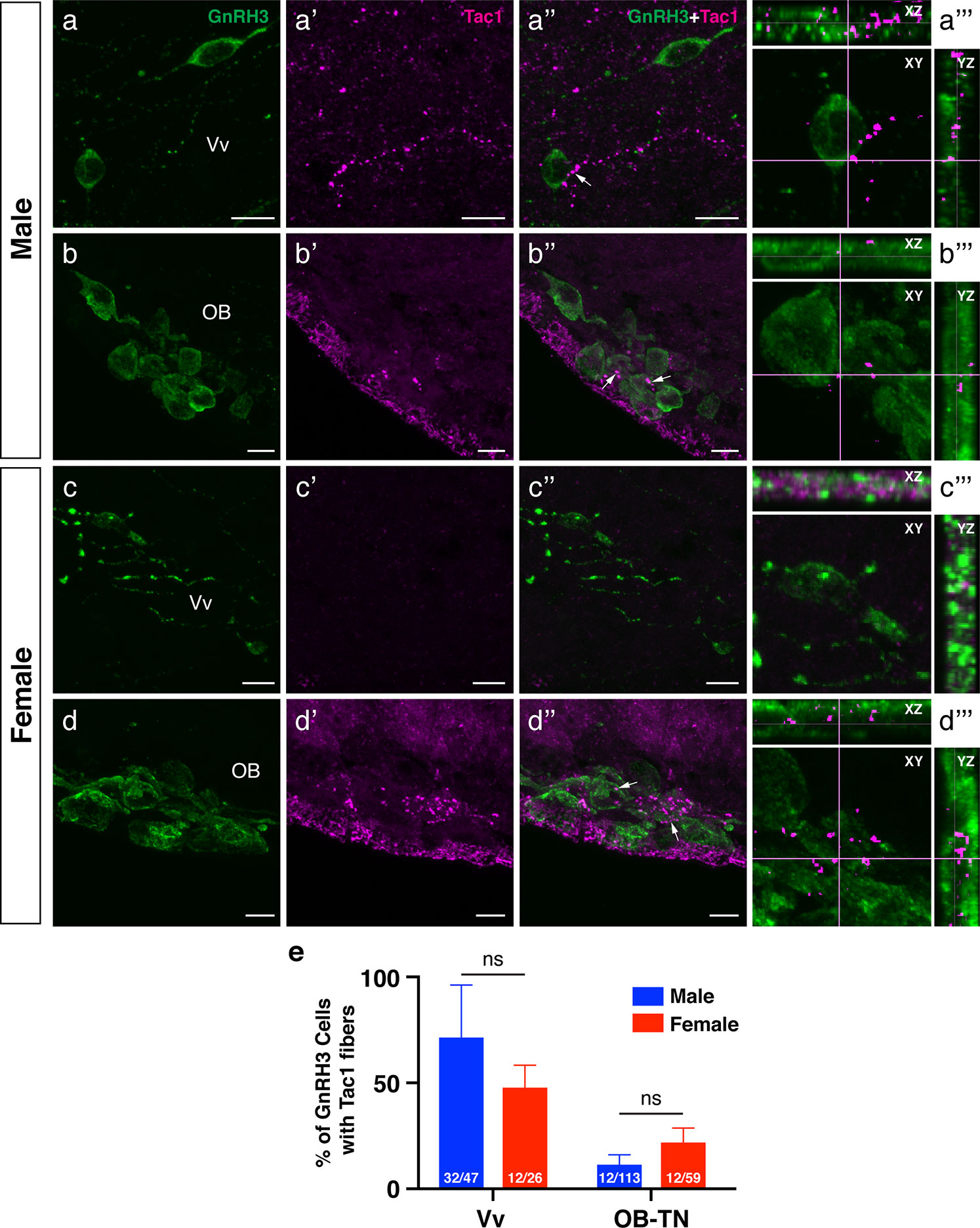
Figure 7 Neuronal association between Tac1-immunoreactive processes and GnRH3 cell soma in the male and female zebrafish. Photomicrographs for immunofluorescence of GnRH3 (1st column, A−D), and Tac1 (2nd column, A’−D’) were merged (3rd column, A”−D”) to elucidate the possible neuronal association (arrows) between Tac1 and GnRH3 neurons. A representative GnRH3 cell soma accompanied by Tac1 processes was further examined by two-dimensional confocal scanning in XY, XZ, and YZ plane (4th column, A’”−D’”). Bar graphs in the bottom panel (E) show the percentage of GnRH3 cells accompanied by Tac1 processes in males (blue columns) and females (red columns) in the ventral nucleus of ventral telencephalic area (Vv) and olfactory-terminal nerve (OB-TN) region. Numbers in each columns indicate the number of GnRH3 cells accompanied by Tac1 processes and total GnRH3 numbers counted. In the Vv, more Tac1-immunoreactive processes (magenta) are in proximities of GnRH3 cells (green) in males (A−A’”) as compared to females (C−C’”), but there was no significant difference in the percentage of GnRH3 cells between males and females (E). In the olfactory bulb (OB), OB-GnRH3 neurons are accompanied by Tac1 processes in both males (B−B’”) and females (D−D’”), and there was no significant sex difference in the percentage of GnRH3 cells (E). ns, non-significant. Scale bars: 10 µm.
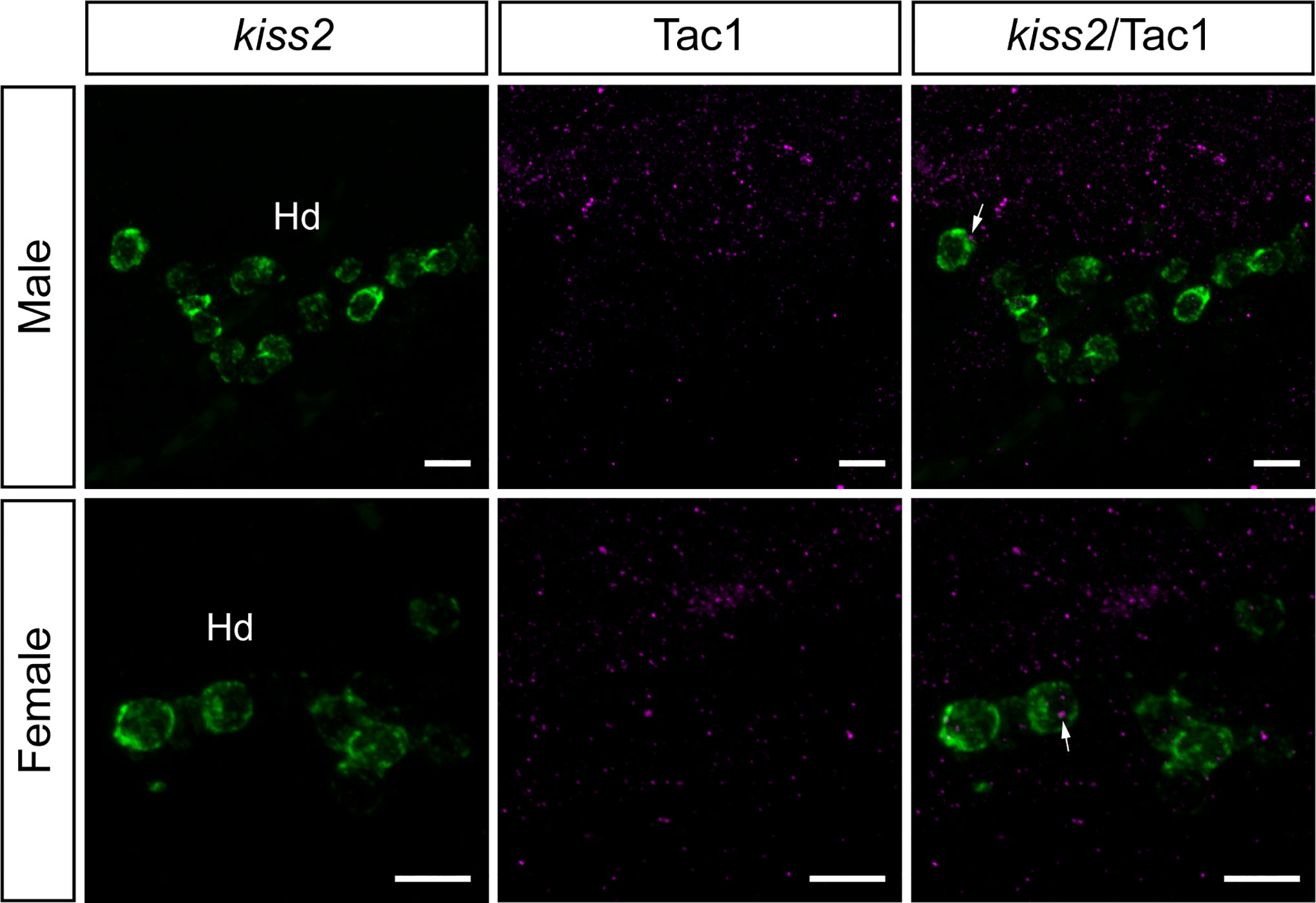
Figure 8 Neuronal association between Tac1-immunoreactive processes and kiss2 cell soma in the male and female zebrafish. Photomicrographs for mRNA expression of kiss2 (1st column), and Tac1-immunoreactivity (2nd column) were merged (3rd column) to elucidate the possible neuronal association between Tac1 and kiss2 neurons. Occasionally, some kiss2 mRNA containing cells (green) are accompanied by Tac1 processes (magenta, arrows) in the dorsal zones of periventricular hypothalamus (Hd). However, they do not seem to form close associations based on the results of confocal scanning (data not shown). Scale bars: 10µm.
Expression of NK1 Receptor mRNA in the Brain Regions Containing GnRH3 and kiss2 Neurons
To elucidate possible direct action of Tac1 on GnRH3 and Kiss2 neurons, expression of NK1 receptor (tacr1a) mRNA was examined in the brain regions where GnRH3 or kiss2 cells are present. In situ hybridization showed expression of tacr1a mRNA in the forebrain regions including the olfactory bulb, ventral telencephalic area and the parvocellular preoptic nucleus (Figure 9A). In the olfactory bulb, a few tacr1a expressing cells were seen along the border between the glomerular layer and the external cellular layer of the olfactory bulb (Figure 9B). tacr1a mRNA was also weakly expressed in the primary olfactory fiber layer, where olfactory-terminal nerve GnRH3 cells are present (Figures 9B, B’). In the ventral nucleus of the ventral telencephalic area, where preoptic-GnRH3 neurons are present, tacr1a mRNA was broadly expressed (Figures 9C, C’).
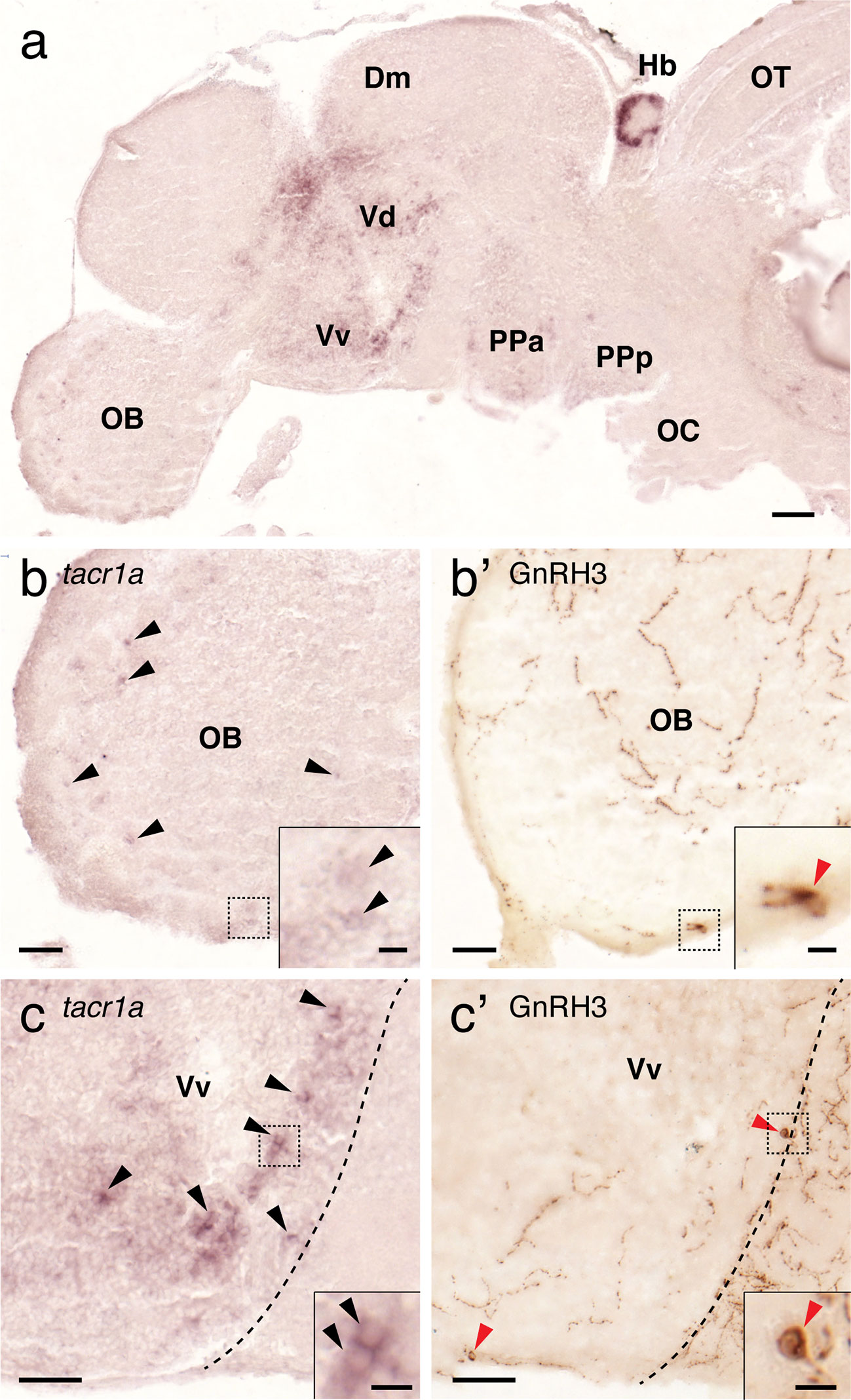
Figure 9 Expression of tacr1a mRNA in the forebrain regions containing GnRH3 cells. In the forebrain, tacr1a mRNA is expressed in the olfactory bulb (OB), dorsal (Vd) and ventral (Vd) regions of the ventral telencephalon, and anterior part of the parvocellular preoptic nucleus (PPa) (A). In the OB, weak expression of tacr1a mRNA was seen in the glomerular layer (b, black arrowheads), and in the primary olfactory fiber layer of the olfactory bulb where GnRH3 neurons are present (B’, red arrowheads). In the Vv, where another GnRH3 cell population is present (C’, red arrowheads), tacr1a mRNA is broadly expressed (C, black arrowheads). Doted box indicates the location of the inset. Scale bars: (A), 100 µm; (B, B’, C, C’), 50 µm; insets, 10 µm.
In the hypothalamus, tacr1a expression was seen along the diencephalic ventricle including the ventral nucleus of the ventral telencephalic area, posterior tuberal nucleus, central posterior thalamic nucleus (Figure 10A), and in the periventricular nucleus of the posterior tuberculum where kiss2 neurons are located (Figures 10C–E). In addition, tacr1a expression was also seen in the lateral hypothalamic nucleus towards the dorsal zone of the periventricular hypothalamus, where another group of kiss2 neurons is present (Figures 10B, F, G).
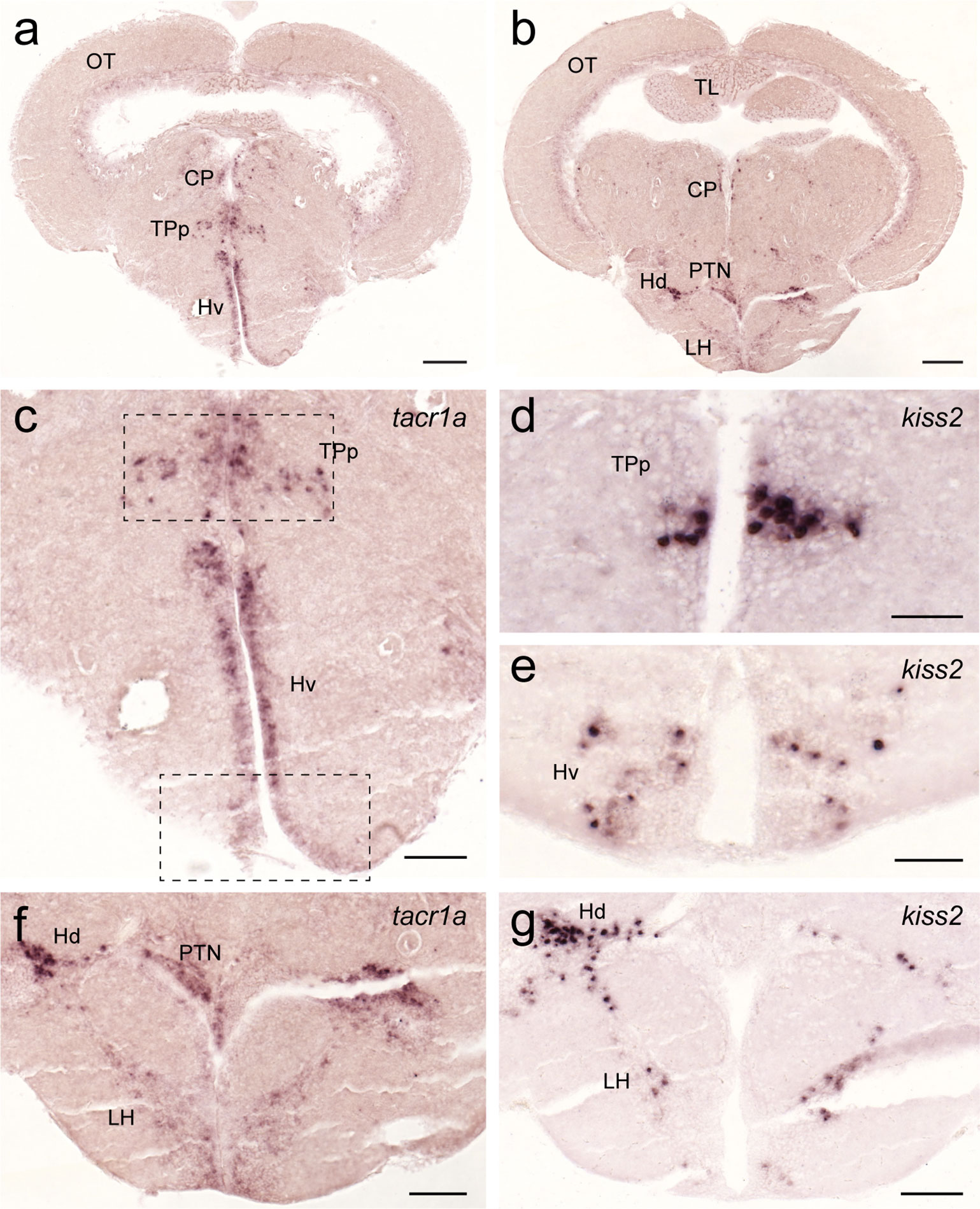
Figure 10 Expression of tacr1a mRNA in the hypothalamic regions containing kiss2 cells. In the hypothalamic regions, tacr1a mRNA is expressed in several regions (A, C) including the ventral nucleus of the ventral telencephalic area and the periventricular nucleus of the posterior tuberculum (dotted box), where kiss2 neurons are present (D, E). In addition, tacr1a expression was also seen in the lateral hypothalamic nucleus towards the dorsal zone of the periventricular hypothalamus (B, F), where another group of kiss2 neurons is present (G). Scale bars: (A, B), 200 µm; (C, F, G), 100 µm; (D, E), 50 µm. See Glossary for abbreviation.
Discussion
An apparent male-dominant sexually dimorphic expression of tac1 mRNA and Tac1-immunoreactivities was observed in the ventral telencephalic area, similar to mammals and other fish species (32, 34). In mammals, the male-dominant expression pattern of SP has also been reported in the medial amygdala and basal ganglia regions (30, 31). Although these brain structures are morphologically indistinguishable in the fish species, several hodological characterizations based on neuroanatomy, molecular and neurochemical markers suggest that the ventral subpallium, the ventral part of the ventral telencephalic area of the non-mammalian brain, may represent the basal ganglia (69–71). On the other hand, the teleostean medial zone of the dorsal telencephalon is considered to contain the homologue of the pallial amygdala because of their involvement in spatial cognition and fear expression in fish (72, 73). Recently, based on morphological and functional assays in zebrafish, the intermediate ventral telencephalic nucleus has been proposed as the teleostean medial amygdala (74). Since SP has been known as a common marker of the basal ganglia and amygdala (69, 75), male-dominant SP expression in the ventral part of the ventral telencephalic regions could be functional evidence supporting the ventral subpallium as the teleostean basal ganglia (and amygdala). In the zebrafish, we did not find any apparent sex differences in the diencephalon and mesencephalon, except for a female dominant tac1 expression in the ventral zone of periventricular hypothalamus. In humans, the number of SP cells in the hypothalamic infundibular (or arcuate) nucleus is higher in postmenopausal woman compared with age-matched men (49). This suggests that SP/NKA population in the basal forebrain is male dominant, while the hypothalamic SP/NKA population is female dominant. In the rhombencephalon, there was a stronger expression of tac1 mRNA in the superior raphe in males as compared to females, although the difference was insignificant. In human, SP is expressed in serotonergic neurons in the raphe nucleus (76, 77), and serotonin-immunoreactive signal intensity in the rat dorsal raphe is male-dominant (78). Therefore, sexually dimorphic tac1 expressing neurons in the raphe could be serotonergic.
Sexually dimorphic expression pattern of Tac1 cells and processes indicate that gonadal steroids may exert either organizational or activational effects on the Tac1 system. In an electric fish, Apteronotus leptorhynchus, treatment with androgens increased SP-immunoreactive cell numbers in the ventral telencephalon and hypothalamic regions (79, 80). Similarly, in rodents, administration of anabolic androgenic steroids increases SP immunoreactivity in several brain regions including the amygdala and hypothalamus (81–83). Alternatively, it is also possible that the lesser expression of tac1 in females could be due to the inhibitory action of estrogens on SP neurons. In primates and humans, there is a negative correlation between plasma levels of estradiol and SP during the follicular phase (84). Similarly, in female rats, the concentration of SP in the bed nucleus and ventromedial nucleus of the hypothalamus is decreased during the estrus stage as compared to diestrus and proestrus stages (28). On the other hand, in some mammalian species, estrogen upregulates hypothalamic SP (32, 85–89). In castrated rats, estrogen increases Tac1 mRNA levels in the hypothalamus, while androgen only upregulates Tac1 mRNA levels in the anterior pituitary but it does not affect hypothalamic Tac1 mRNA levels (32). In female medaka, higher aromatase expression and activity are seen in the periventricular hypothalamus as compared to males (90). This suggests that the higher expression of tac1 in the periventricular hypothalamus of female zebrafish could be under estrogenic regulation. In the brain of zebrafish, estrogen and androgen receptors are highly expressed in the ventral telencephalic regions where male dominant Tac1 neurons were found (91–93). In medaka, three types of estrogen receptors (esr1, esr2a, and esr2b) and two types of androgen receptors (ara and arb) genes exhibited brain region-specific sexually dimorphic expression patterns (94). In particular, in the ventral telencephalic-preoptic regions, esr2a exhibits male-dominant, while esr1, esr2b, and arb exhibit female-dominant expression (94). However, in zebrafish, there is no apparent sexual dimorphism in androgen receptor expression in the ventral telencephalic regions (93). Although the exact reason for this discrepancy remains unknown, male-dominant expression of Tac1 in the ventral telencephalic region could be a result of the organizational effect of sex steroids during brain development. These results suggest that Tac1 expression could be regulated by steroids in brain regions- and reproductive conditions-dependent manners.
In the present study, we generated an antibody against the carboxyl-terminal region of the zebrafish Tac1, which has low homology with other tachykinin family of peptides. Tac1-immunoreactivities in neurons and processes were widely observed in the brain, which was successfully eliminated by pre-absorption with the antigen peptide. Since our antibody is generated against the carboxyl-terminal region encoded by exon-5 of tac1 gene, which exists in all splicing variants, this antibody should recognize all precursors produced by different splice variant mRNAs of tac1 gene. Furthermore, Tac1-immunoreactive cells can only be found in brain regions where tac1 mRNA-expressing cells are present suggesting that cross-reactivity of the Tac1 antibody to other tachykinins is likely to be low. In some brain regions, Tac1-immunoreactivity appeared to be considerably lower than the densities of tac1 expression. We also observed Tac1-immunoreactivities in fish administered with colchicine (to enhance the staining), but we did not find any apparent difference in the intensity of Tac1-immunoreactivity (data not shown). Although the exact reason for this discrepancy remains unclear, it may suggest the presence of differential translational activity of tac1 mRNA among Tac1 cell populations. In fact, biosynthesis of Tac1 peptides has been suggested to be modulated by region specific translation or processing mechanism in the rat brain (95). Therefore, Tac1 peptide content levels could vary depending on the animal’s physiological condition or brain regions.
Using the zebrafish Tac1 antibody, we also found that GnRH3 neurons are closely accompanied by Tac1-immunoreactive processes in the forebrain. Further, we also localized NK1 receptor (tacr1a) mRNA in the forebrain regions containing GnRH3 cells, suggesting possible expression of NK1 receptor in GnRH3 cells. On the other hand, although tacr1a expression was seen in most of the hypothalamic regions containing kiss2 cells, Tac1-immunoreactive processes did not closely accompany kiss2 cells. These results imply that Tac1 peptides could act directly on GnRH3 cells but not on Kiss2 cells. However, whether tacr1a is expressed in GnRH3 and Kiss2 cells, and Tac1 processes form synaptic action on GnRH3/Kiss2 cells remain to be investigated.
In the ventral nucleus of ventral telencephalic area, more GnRH3 neurons are accompanied by Tac1 processes in males as compared to females. In some fish, sex-dependent expression pattern of GnRH has been reported. In wrasses, there are intra- and intersexual dimorphisms in the number of GnRH neurons in the preoptic area (96). In medaka, GnRH1 and GnRH3 genes exhibit brain region-specific sexually-dimorphic expression patterns (97). As preoptic-GnRH3 is considered to act as the hypophysiotropic form in the zebrafish (44, 98, 99), Tac1 may be responsible for a male-typical pattern of regulation of GnRH3 and GnRH3-dependent LH secretion in fish. In addition, GnRH3 cells in the olfactory bulbar-terminal nerve (OB-TN) region are also accompanied by Tac1 processes with a slight female dominance. In teleosts, OB-TN GnRH3 neural population has been shown to regulate social behaviors such as aggression, sexual behavior and partner preference (100–102). In mammals, NK1 receptor-mediated SP neurotransmission has been implicated in the control of social behaviors such as aggression, sexual behavior and anxiety (103–105). Hence, Tac1 signaling may be involved in OB-TN GnRH3 dependent social functions.
In summary, we found clear male-dominant sexually dimorphic expression pattern of tac1 in the ventral telencephalon and preoptic regions of zebrafish. Further, we also found that Tac1 processes are in proximities of GnRH3 cells but not with kiss2 cells. Therefore, in the zebrafish, sexually dimorphic Tac1 neuronal populations might directly act on GnRH3 neurons, independent of their influence on kisspeptin. However, it remains unknown whether there is any sex difference in the modulatory effect of SP/NKA on GnRH system. Although there was no apparent sex difference in number of GnRH3 cells accompanied by Tac1 processes, in males preoptic-GnRH3 neurons are in proximity with more Tac1 processes, while in females, more GnRH3 neurons are accompanied by Tac1 processes in the olfactory bulb. Hence, it can be hypothesized that SP/NKA may have a gender- and brain region-biased influence on GnRH3 neurons in the zebrafish.
Data Availability Statement
The raw data supporting the conclusions of this article will be made available by the authors, without undue reservation, to any qualified researcher.
Ethics Statement
The animal study was reviewed and approved by Animal Ethics Committee of Monash University (Approval number: MARP/2012/120).
Author Contributions
SO and ISP designed research. SO and ISP created antisera for zebrafish TAC1. PNR and RA performed the experiments. PNR and SO analyzed the data, and PNR, SO, and ISP wrote the paper. All authors contributed to the article and approved the submitted version.
Funding
This work is supported by Monash University Malaysia (M-NEU-RS-014); Malaysian Ministry of Higher Education, FRGS/2/2010/ST/MUSM/03/02 (to SO); and FRGS/1/2013/SKK01/MUSM/03/02 (to ISP) and Malaysian Ministry of Science and Technology and Innovation, 02-02-10-SF0161 (to ISP).
Conflict of Interest
The authors declare that the research was conducted in the absence of any commercial or financial relationships that could be construed as a potential conflict of interest.
Acknowledgments
We thank Monash University Malaysia for the Higher Degree Research Scholarship to PNR. We thank Dr. Mageswary Sivalingam for kind sharing of tacr1a probes for in situ hybridization. Immunohistochemistry and imaging works were conducted with technical support provided by the Bioimaging Infrastructure Platform, Monash University Malaysia.
Supplementary Material
The Supplementary Material for this article can be found online at: https://www.frontiersin.org/articles/10.3389/fendo.2020.534343/full#supplementary-material
Supplementary Figure 1 | Comparison of Tac1 immunoreactivity with and without pre-absorption with the antigen peptide. (A) Photomicrographs of Tac1-immunoreactive fibers (arrows) in the dorsal region of the ventral telencephalon (Vd), of which immunoreactivity was mostly diminished by the pre-absorption with the antigen peptide (B) Scale bars: 50 µm. (C) Dot-blot analysis showed that anti-SP antibody reacted to human SP, but not to the zebrafish Tac1 antigen peptide (first column), while the anti-zebrafish Tac1 antibody strongly reacted to the Tac1 antigen peptide, but not to human SP peptide (second column). Pre-absorption with the antigen peptide diminished the immunoreactivity to the Tac1 (third column).
References
1. Zieglgansberger W. Substance P and pain chronicity. Cell Tissue Res (2019) 375(1):227–41. doi: 10.1007/s00441-018-2922-y
2. Ebner K, Singewald N. The role of substance P in stress and anxiety responses. Amino Acids (2006) 31(3):251–72. doi: 10.1007/s00726-006-0335-9
3. De Felipe C, Herrero JF, O’Brien JA, Palmer JA, Doyle CA, Smith AJ, et al. Altered nociception, analgesia and aggression in mice lacking the receptor for substance P. Nature (1998) 392(6674):394–7. doi: 10.1038/32904
4. Lénárd L, László K, Kertes E, Ollmann T, Péczely L, Kovács A, et al. Substance P and neurotensin in the limbic system: Their roles in reinforcement and memory consolidation. Neurosci Biobehav Rev (2018) 85:1–20. doi: 10.1016/j.neubiorev.2017.09.003
5. Hasenohrl RU, Souza-Silva MA, Nikolaus S, Tomaz C, Brandao ML, Schwarting RK, et al. Substance P and its role in neural mechanisms governing learning, anxiety and functional recovery. Neuropeptides (2000) 34(5):272–80. doi: 10.1054/npep.2000.0824
6. Pennefather JN, Lecci A, Candenas ML, Patak E, Pinto FM, Maggi CA. Tachykinins and tachykinin receptors: a growing family. Life Sci (2004) 74(12):1445–63. doi: 10.1016/j.lfs.2003.09.039
7. Carter MS, Krause JE. Structure, expression, and some regulatory mechanisms of the rat preprotachykinin gene encoding substance P, neurokinin A, neuropeptide K, and neuropeptide gamma. J Neurosci (1990) 10(7):2203–14. doi: 10.1523/JNEUROSCI.10-07-02203.1990
8. Nevin K, Zhuo H, Helke CJ. Neurokinin A coexists with substance P and serotonin in ventral medullary spinally projecting neurons of the rat. Peptides (1994) 15(6):1003–11. doi: 10.1016/0196-9781(94)90063-9
9. Dalsgaard C-J, Haegerstrand A, Theodorsson-Norheim E, Brodin E, Hökfelt T. Neurokinin A-like immunoreactivity in rat primary sensory neurons; coexistence with substance P. Histochemistry (1985) 83(1):37–9. doi: 10.1007/BF00495297
10. Hua XY, Theodorsson-Norheim E, Brodin E, Lundberg JM, Hökfelt T. Multiple tachykinins (neurokinin A, neuropeptide K and substance P) in capsaicin-sensitive sensory neurons in the guinea-pig. Regul Peptides (1985) 13(1):1–19. doi: 10.1016/0167-0115(85)90082-5
11. Hua XY, Saria A, Gamse R, Theodorsson-Norheim E, Brodin E, Lundberg JM. Capsaicin induced release of multiple tachykinins (substance P, neurokinin A and eledoisin-like material) from guinea-pig spinal cord and ureter. Neuroscience (1986) 19(1):313–9. doi: 10.1016/0306-4522(86)90024-2
12. Saffroy M, Torrens Y, Glowinski J, Beaujouan JC. Autoradiographic distribution of tachykinin NK2 binding sites in the rat brain: comparison with NK1 and NK3 binding sites. Neuroscience (2003) 116(3):761–73. doi: 10.1016/s0306-4522(02)00748-0
13. Ohtsuka S, Miyake A, Nishizaki T, Tasaka K, Aono T, Tanizawa O. Substance P stimulates gonadotropin-releasing hormone release from rat hypothalamus in vitro with involvement of oestrogen. Acta Endocrinol (Copenh) (1987) 115(2):247–52. doi: 10.1530/acta.0.1150247
14. Duval P, Lenoir V, Garret C, Kerdelhue B. Reduction of the amplitude of preovulatory LH and FSH surges and of the amplitude of the in vitro GnRH-induced LH release by substance P. Reversal of the effect by RP 67580. Neuropharmacology (1996) 35(12):1805–10. doi: 10.1016/S0028-3908(96)00124-4
15. Tsuruo Y, Hisano S, Nakanishi J, Katoh S, Daikoku S. Immunohistochemical studies on the roles of substance P in the rat hypothalamus: possible implication in the hypothalamic-hypophysial-gonadal axis. Neuroendocrinology (1987) 45(5):389–401. doi: 10.1159/000124764
16. Navarro VM, Bosch MA, Leon S, Simavli S, True C, Pinilla L, et al. The integrated hypothalamic tachykinin-kisspeptin system as a central coordinator for reproduction. Endocrinology (2015) 156(2):627–37. doi: 10.1210/en.2014-1651
17. Yamamura T, Wakabayashi Y, Ohkura S, Navarro VM, Okamura H. Effects of intravenous administration of neurokinin receptor subtype-selective agonists on gonadotropin-releasing hormone pulse generator activity and luteinizing hormone secretion in goats. J Reprod Dev (2015) 61(1):20–9. doi: 10.1262/jrd.2014-109
18. Fergani C, Navarro VM. Expanding the role of tachykinins in the neuroendocrine control of reproduction. Reproduction (2016) 153(1):R1–R14. doi: 10.1530/REP-16-0378
19. de Croft S, Boehm U, Herbison AE. Neurokinin B activates arcuate kisspeptin neurons through multiple tachykinin receptors in the male mouse. Endocrinology (2013) 154(8):2750–60. doi: 10.1210/en.2013-1231
20. Simavli S, Thompson IR, Maguire CA, Gill JC, Carroll RS, Wolfe A, et al. Substance P regulates puberty onset and fertility in the female mouse. Endocrinology (2015) 156(6):2313–22. doi: 10.1210/en.2014-2012
21. León S, Fergani C, Talbi R, Simavli S, Maguire CA, Gerutshang A, et al. Characterization of the role of NKA in the control of puberty onset and gonadotropin release in the female mouse. Endocrinology (2019) 160(10):2453–63. doi: 10.1210/en.2019-00195
22. Maguire CA, Song YB, Wu M, León S, Carroll RS, Alreja M, et al. Tac1 Signaling is required for sexual maturation and responsiveness of GnRH neurons to kisspeptin in the male mouse. Endocrinology (2017) 158(7):2319–29. doi: 10.1210/en.2016-1807
23. Lehman MN, Coolen LM, Goodman RL. Importance of neuroanatomical data from domestic animals to the development and testing of the KNDy hypothesis for GnRH pulse generation. Domest Anim Endocrinol (2020) 73:106441. doi: 10.1016/j.domaniend.2020.106441
24. Kalil B, Ramaswamy S, Plant TM. The distribution of substance P and Kisspeptin in the mediobasal hypothalamus of the male rhesus monkey and a comparison of intravenous administration of these peptides to release GnRH as reflected by LH secretion. Neuroendocrinology (2016) 103(6):711–23. doi: 10.1159/000442420
25. Fergani C, Mazzella L, Coolen LM, McCosh RB, Hardy SL, Newcomb N, et al. Do substance P and neurokinin A play important roles in the control of LH secretion in ewes? Endocrinology (2016) 157(12):4829–41. doi: 10.1210/en.2016-1565
26. Tsuruo Y, Hisano S, Okamura Y, Tsukamoto N, Daikoku S. Hypothalamic substance P-containing neurons. Sex-dependent topographical differences and ultrastructural transformations associated with stages of the estrous cycle. Brain Res (1984) 305(2):331–41. doi: 10.1016/0006-8993(84)90439-6
27. Akesson TR. Androgen concentration by a sexually dimorphic population of tachykinin-immunoreactive neurons in the rat ventral premammillary nucleus. Brain Res (1993) 608(2):319–23. doi: 10.1016/0006-8993(93)91473-6
28. Micevych PE, Matt DW, Go VL. Concentrations of cholecystokinin, substance P, and bombesin in discrete regions of male and female rat brain: sex differences and estrogen effects. Exp Neurol (1988) 100(2):416–25. doi: 10.1016/0014-4886(88)90119-7
29. Frankfurt M, Siegel RA, Sim I, Wuttke W. Cholecystokinin and substance P concentrations in discrete areas of the rat brain: sex differences. Brain Res (1985) 358(1-2):53–8. doi: 10.1016/0006-8993(85)90947-3
30. Malsbury CW, McKay K. Sex difference in the substance P-immunoreactive innervation of the medial nucleus of the amygdala. Brain Res Bull (1989) 23(6):561–7. doi: 10.1016/0361-9230(89)90201-3
31. Hines M, Allen LS, Gorski RA. Sex differences in subregions of the medial nucleus of the amygdala and the bed nucleus of the stria terminalis of the rat. Brain Res (1992) 579(2):321–6. doi: 10.1016/0006-8993(92)90068-k
32. Brown ER, Harlan RE, Krause JE. Gonadal steroid regulation of substance P (SP) and SP-encoding messenger ribonucleic acids in the rat anterior pituitary and hypothalamus. Endocrinology (1990) 126(1):330–40. doi: 10.1210/endo-126-1-330
33. Brown ER, Roth KA, Krause JE. Sexually dimorphic distribution of substance P in specific anterior pituitary cell populations. Proc Natl Acad Sci (1991) 88(4):1222–6. doi: 10.1073/pnas.88.4.1222
34. Weld MM, Maler L. Substance P-like immunoreactivity in the brain of the gymnotiform fish Apteronotus leptorhynchus: presence of sex differences. J Chem Neuroanat (1992) 5(2):107–29. doi: 10.1016/0891-0618(92)90038-r
35. Weld MM, Kar S, Maler L, Quirion R. The distribution of tachykinin binding sites in the brain of an electric fish (Apteronotus leptorhynchus). J Chem Neuroanat (1994) 7(3):123–39. doi: 10.1016/0891-0618(94)90024-8
36. Peyon P, Saied H, Lin X, Peter RE. Preprotachykinin gene expression in goldfish brain: sexual, seasonal, and postprandial variations. Peptides (2000) 21(2):225–31. doi: 10.1016/S0196-9781(99)00190-4
37. Gobbetti A, Zerani M, Di Fiore MM, Botte V. Relationships among GnRH, substance P, prostaglandins, sex steroids and aromatase activity in the brain of the male lizard Podarcis sicula sicula during reproduction. J Reprod Fertil (1994) 101(3):523–9. doi: 10.1530/jrf.0.1010523
38. Gobbetti A, Petrelli C, Zerani M. Substance P downregulates basal and gonadotropin-releasing hormone-induced gonadotropin in vitro secretion by pituitary gland of crested newt, Triturus carnifex. J Neuroendocrinol (2000) 12(12):1160–6. doi: 10.1046/j.1365-2826.2000.00576.x
39. Moons L, Batten TF, Vandesande F. Comparative distribution of substance P (SP) and cholecystokinin (CCK) binding sites and immunoreactivity in the brain of the sea bass (Dicentrarchus labrax). Peptides (1992) 13(1):37–46. doi: 10.1016/0196-9781(92)90137-r
40. Lin XW, Peter RE. Goldfish gamma-preprotachykinin mRNA encodes the neuropeptides substance P, carassin, and neurokinin A. Peptides (1997) 18(6):817–24. doi: 10.1016/s0196-9781(97)00013-2
41. Okubo K, Nagahama Y. Structural and functional evolution of gonadotropin-releasing hormone in vertebrates. Acta Physiol (Oxf) (2008) 193(1):3–15. doi: 10.1111/j.1748-1716.2008.01832.x
42. Parhar IS. GnRH in tilapia: Three genes, three origins and their roles. In: Parhar IS, Sakuma Y, editors. GnRH neurons: Gene to Behavior. Tokyo: Brain Shuppan (1997). p. 99–122.
43. Gopurappilly R, Ogawa S, Parhar IS. Functional significance of GnRH and kisspeptin, and their cognate receptors in teleost reproduction. Front Endocrinol (2013) 4:24. doi: 10.3389/fendo.2013.00024
44. Abraham E, Palevitch O, Gothilf Y, Zohar Y. Targeted gonadotropin-releasing hormone-3 neuron ablation in zebrafish: effects on neurogenesis, neuronal migration, and reproduction. Endocrinology (2010) 151(1):332–40. doi: 10.1210/en.2009-0548
45. Marvel MM, Spicer OS, Wong TT, Zmora N, Zohar Y. Knockout of Gnrh2 in zebrafish (Danio rerio) reveals its roles in regulating feeding behavior and oocyte quality. Gen Comp Endocrinol (2019) 280:15–23. doi: 10.1016/j.ygcen.2019.04.002
46. Nishiguchi R, Azuma M, Yokobori E, Uchiyama M, Matsuda K. Gonadotropin-releasing hormone 2 suppresses food intake in the zebrafish, Danio rerio. Front Endocrinol (2012) 3:3389. doi: 10.3389/fendo.2012.00122
47. Kitahashi T, Ogawa S, Parhar IS. Cloning and expression of kiss2 in the zebrafish and medaka. Endocrinology (2009) 150(2):821–31. doi: 10.1210/en.2008-0940
48. Ogawa S, Nathan FM, Parhar IS. Habenular kisspeptin modulates fear in the zebrafish. Proc Natl Acad Sci USA (2014) 111(10):3841–6. doi: 10.1073/pnas.1314184111
49. Hrabovszky E, Borsay BÁ, Rácz K, Herczeg L, Ciofi P, Bloom SR, et al. Substance P immunoreactivity exhibits frequent colocalization with kisspeptin and neurokinin B in the human infundibular region. PloS One (2013) 8(8):e72369. doi: 10.1371/journal.pone.0072369
50. Okamura H, Yamamura T, Wakabayashi Y. Mapping of KNDy neurons and immunohistochemical analysis of the interaction between KNDy and substance P neural systems in goat. J Reprod Dev (2017) 63(6):571–80. doi: 10.1262/jrd.2017-103
51. Borsay BÁ, Skrapits K, Herczeg L, Ciofi P, Bloom SR, Ghatei MA, et al. Hypophysiotropic gonadotropin-releasing hormone projections are exposed to dense plexuses of kisspeptin, neurokinin B and substance P immunoreactive fibers in the human: a study on tissues from postmenopausal women. Neuroendocrinology (2014) 100(2-3):141–52. doi: 10.1159/000368362
52. Ogawa S, Ramadasan PN, Goschorska M, Anantharajah A, We Ng K, Parhar IS. Cloning and expression of tachykinins and their association with kisspeptins in the brains of zebrafish. J Comp Neurol (2012) 520(13):2991–3012. doi: 10.1002/cne.23103
53. Yokobori E, Kojima K, Azuma M, Kang KS, Maejima S, Uchiyama M, et al. Stimulatory effect of intracerebroventricular administration of orexin A on food intake in the zebrafish, Danio rerio. Peptides (2011) 32(7):1357–62. doi: 10.1016/j.peptides.2011.05.010
54. Nakamachi T, Kamata E, Tanigawa A, Konno N, Shioda S, Matsuda K. Distribution of pituitary adenylate cyclase-activating polypeptide 2 in zebrafish brain. Peptides (2018) 103:40–7. doi: 10.1016/j.peptides.2018.03.006
55. Park MK, Wakabayashi K. Preparation of a monoclonal antibody to common amino acid sequence of LHRH and its application. Endocrinol Jpn (1986) 33(2):257–72. doi: 10.1507/endocrj1954.33.257
56. Whitlock KE, Smith KM, Kim H, Harden MV. A role for foxd3 and sox10 in the differentiation of gonadotropin-releasing hormone (GnRH) cells in the zebrafish Danio rerio. Development (2005) 132(24):5491–502. doi: 10.1242/dev.02158
57. Gopinath A, Andrew TL, Whitlock KE. Temporal and spatial expression of gonadotropin releasing hormone (GnRH) in the brain of developing zebrafish (Danio rerio). Gene Expr Patterns (2004) 4(1):65–70. doi: 10.1016/s1567-133x(03)00149-2
58. Ramakrishnan S, Lee W, Navarre S, Kozlowski DJ, Wayne NL. Acquisition of spontaneous electrical activity during embryonic development of gonadotropin-releasing hormone-3 neurons located in the terminal nerve of transgenic zebrafish (Danio rerio). Gen Comp Endocrinol (2010) 168(3):401–7. doi: 10.1016/j.ygcen.2010.05.009
59. Whitlock KE, Wolf CD, Boyce ML. Gonadotropin-releasing hormone (GnRH) cells arise from cranial neural crest and adenohypophyseal regions of the neural plate in the zebrafish, Danio rerio. Dev Biol (2003) 257(1):140–52. doi: 10.1016/s0012-1606(03)00039-3
60. Miyasaka N, Morimoto K, Tsubokawa T, Higashijima S, Okamoto H, Yoshihara Y. From the olfactory bulb to higher brain centers: genetic visualization of secondary olfactory pathways in zebrafish. J Neurosci (2009) 29(15):4756–67. doi: 10.1523/jneurosci.0118-09.2009
61. Wullimann MF, Rupp B, Reichert H. Neuroanatomy of Zebrafish Brain: A Topological Atlas. Basel; Boston; Berlin: Birkhäuser (1996).
62. Mueller T, Guo S. The distribution of GAD67-mRNA in the adult zebrafish (teleost) forebrain reveals a prosomeric pattern and suggests previously unidentified homologies to tetrapods. J Comp Neurol (2009) 516(6):553–68. doi: 10.1002/cne.22122
63. Liu Q, Bhattarai S, Wang N, Sochacka-Marlowe A. Differential expression of protocadherin-19, protocadherin-17, and cadherin-6 in adult zebrafish brain. J Comp Neurol (2015) 523(9):1419–42. doi: 10.1002/cne.23746
64. Rink E, Wullimann MF. The teleostean (zebrafish) dopaminergic system ascending to the subpallium (striatum) is located in the basal diencephalon (posterior tuberculum). Brain Res (2001) 889(1–2):316–30. doi: 10.1016/S0006-8993(00)03174-7
65. Mueller T, Vernier P, Wullimann MF. The adult central nervous cholinergic system of a neurogenetic model animal, the zebrafish Danio rerio. Brain Res (2004) 1011(2):156–69. doi: 10.1016/j.brainres.2004.02.073
66. Meek J. Functional anatomy of the tectum mesencephali of the goldfish. An explorative analysis of the functional implications of the laminar structural organization of the tectum. Brain Res Rev (1983) 6(3):247–97. doi: 10.1016/0165-0173(83)90008-5
67. Prasad P, Ogawa S, Parhar IS. Serotonin reuptake iInhibitor citalopram inhibits GnRH synthesis and spermatogenesis in the male zebrafish1. Biol Reprod (2015) 93(4):102, 1-10. doi: 10.1095/biolreprod.115.129965
68. Corson JA, Erisir A. Monosynaptic convergence of chorda tympani and glossopharyngeal afferents onto ascending relay neurons in the nucleus of the solitary tract: A high-resolution confocal and correlative electron microscopy approach. J Comp Neurol (2013) 521(13):2907–26. doi: 10.1002/cne.23357
69. Moreno N, González A, Rétaux S. Development and evolution of the subpallium. Semin Cell Dev Biol (2009) 20(6):735–43. doi: 10.1016/j.semcdb.2009.04.007
70. Mueller T, Wullimann MF, Guo S. Early teleostean basal ganglia development visualized by Zebrafish Dlx2a, Lhx6, Lhx7, Tbr2 (eomesa), and GAD67 gene expression. J Comp Neurol (2008) 507(2):1245–57. doi: 10.1002/cne.21604
71. Reiner A, Medina L, Veenman CL. Structural and functional evolution of the basal ganglia in vertebrates. Brain Res Rev (1998) 28(3):235–85. doi: 10.1016/S0165-0173(98)00016-2
72. Rodrı́guez F, López JC, Vargas JP, Broglio C, Gómez Y, Salas C. Spatial memory and hippocampal pallium through vertebrate evolution: insights from reptiles and teleost fish. Brain Res Bull (2002) 57(3):499–503. doi: 10.1016/S0361-9230(01)00682-7
73. Lal P, Tanabe H, Suster ML, Ailani D, Kotani Y, Muto A, et al. Identification of a neuronal population in the telencephalon essential for fear conditioning in zebrafish. BMC Biol (2018) 16(1):45. doi: 10.1186/s12915-018-0502-y
74. Biechl D, Tietje K, Ryu S, Grothe B, Gerlach G, Wullimann MF. Identification of accessory olfactory system and medial amygdala in the zebrafish. Sci Rep (2017) 7(1):44295. doi: 10.1038/srep44295
75. Roberts GW, Woodhams PL, Polak JM, Crow TJ. Distribution of neuropeptides in the limbic system of the rat: the amygdaloid complex. Neuroscience (1982) 7(1):99–131. doi: 10.1016/0306-4522(82)90156-7
76. Sergeyev V, Hökfelt T, Hurd Y. Serotonin and substance P co-exist in dorsal raphe neurons of the human brain. Neuroreport (1999) 10(18):3967–70. doi: 10.1097/00001756-199912160-00044
77. Pelletier G, Steinbusch HWM, Verhofstad AAJ, Immunoreactive substance P and serotonin present in the same dense-core vesicles. Nature (1981) 293(5827):71–2. doi: 10.1038/293071a0
78. Kunimura Y, Iwata K, Iijima N, Kobayashi M, Ozawa H. Effect of sex steroid hormones on the number of serotonergic neurons in rat dorsal raphe nucleus. Neurosci Lett (2015) 594:127–32. doi: 10.1016/j.neulet.2015.03.060
79. Dulka JG, Ebling SL. Testosterone increases the number of substance P-like immunoreactive neurons in a specific sub-division of the lateral hypothalamus of the weakly electric, brown ghost knifefish, Apteronotus leptorhynchus. Brain Res (1999) 826(1):1–9. doi: 10.1016/S0006-8993(99)01183-X
80. Dulka JG, Maler L, Ellis W. Androgen-induced changes in electrocommunicatory behavior are correlated with changes in substance P-like immunoreactivity in the brain of the electric fish Apteronotus leptorhynchus. J Neurosci (1995) 15(3):1879–90. doi: 10.1523/JNEUROSCI.15-03-01879.1995
81. Hallberg M, Johansson P, Kindlundh AM, Nyberg F. Anabolic-androgenic steroids affect the content of substance P and substance P1–7 in the rat brain. Peptides (2000) 21(6):845–52. doi: 10.1016/s0196-9781(00)00218-7
82. Magnusson K, Hallberg M, Högberg AMSK, Nyberg F. Administration of the anabolic androgenic steroid nandrolone decanoate affects substance P endopeptidase-like activity in the rat brain. Peptides (2006) 27(1):114–21. doi: 10.1016/j.peptides.2005.06.016
83. Swann JM, Newman SW. Testosterone regulates substance P within neurons of the medial nucleus of the amygdala, the bed nucleus of the stria terminalis and the medial preoptic area of the male golden hamster. Brain Res (1992) 590(1-2):18–28. doi: 10.1016/0006-8993(92)91077-r
84. Lasaga M, Debeljuk L. Tachykinins and the hypothalamo–pituitary–gonadal axis: An update. Peptides (2011) 32(9):1972–8. doi: 10.1016/j.peptides.2011.07.009
85. Priest CA, Vink KL, Micevych PE. Temporal regulation by estrogen of beta-preprotachykinin mRNA expression in the rat ventromedial nucleus of the hypothalamus. Brain Res Mol Brain Res (1995) 28(1):61–71. doi: 10.1016/0169-328x(94)00184-g
86. Okamura H, Yokosuka M, Hayashi S. Induction of substance P-immunoreactivity by estrogen in neurons containing estrogen receptors in the anterovental periventricular nucleus of female but not male rats. J Neuroendocrinol (1994) 6(6):609–15. doi: 10.1111/j.1365-2826.1994.tb00626.x
87. Duval P, Lenoir V, Moussaoui S, Garret C, Kerdelhué B, Substance P. and neurokinin A variations throughout the rat estrous cycle; comparison with ovariectomized and male rats: II. Trigeminal nucleus and cervical spinal cord. J Neurosci Res (1996) 45(5):610–6. doi: 10.1002/(SICI)1097-4547(19960901)45:5<610::AID-JNR10>3.0.CO;2-2
88. Dufourny L, Warembourg M. Estrogen modulation of neuropeptides: somatostatin, neurotensin and substance P, in the ventrolateral and arcuate nuclei of the female guinea pig. Neurosci Res (1999) 33(3):223–8. doi: 10.1016/S0168-0102(99)00009-7
89. Akesson TR. Gonadal steroids regulate immunoreactive tachykinin in the ventromedial nucleus of the rat hypothalamus. J Comp Neurol (1994) 341(3):351–6. doi: 10.1002/cne.903410306
90. Melo AC, Ramsdell JS. Sexual dimorphism of brain aromatase activity in medaka: induction of a female phenotype by estradiol. Environ Health Perspect (2001) 109(3):257–64. doi: 10.1289/ehp.01109257
91. Menuet A, Pellegrini E, Anglade I, Blaise O, Laudet V, Kah O, et al. Molecular characterization of three estrogen receptor forms in zebrafish: binding characteristics, transactivation properties, and tissue distributions. Biol Reprod (2002) 66(6):1881–92. doi: 10.1095/biolreprod66.6.1881
92. Gorelick DA, Halpern ME. Visualization of estrogen receptor transcriptional activation in zebrafish. Endocrinology (2011) 152(7):2690–703. doi: 10.1210/en.2010-1257
93. Gorelick DA, Watson W, Halpern ME. Androgen receptor gene expression in the developing and adult zebrafish brain. Dev Dyn (2008) 237(10):2987–95. doi: 10.1002/dvdy.21700
94. Hiraki T, Takeuchi A, Tsumaki T, Zempo B, Kanda S, Oka Y, et al. Female-specific target sites for both oestrogen and androgen in the teleost brain. Proc R Soc B: Biol Sci (2012) 279(1749):5014–23. doi: 10.1098/rspb.2012.2011
95. Arai H, Emson PC. Regional distribution of neuropeptide K and other tachykinins (neurokinin A, neurokinin B and substance P) in rat central nervous system. Brain Res (1986) 399(2):240–9. doi: 10.1016/0006-8993(86)91514-3
96. Grober MS, Bass AH. Neuronal correlates of sex/role change in labrid fishes: LHRH-like immunoreactivity. Brain Behav Evol (1991) 38(6):302–12. doi: 10.1159/000114396
97. Kawabata Y, Hiraki T, Takeuchi A, Okubo K. Sex differences in the expression of vasotocin/isotocin, gonadotropin-releasing hormone, and tyrosine and tryptophan hydroxylase family genes in the medaka brain. Neuroscience (2012) 218:65–77. doi: 10.1016/j.neuroscience.2012.05.021
98. Marvel M, Spicer OS, Wong T-T, Zmora N, Zohar Y. Knockout of the Gnrh genes in zebrafish: effects on reproduction and potential compensation by reproductive and feeding-related neuropeptides. Biol Reprod (2018) 99(3):565–77. doi: 10.1093/biolre/ioy078
99. Palevitch O, Abraham E, Borodovsky N, Levkowitz G, Zohar Y, Gothilf Y. Nasal embryonic LHRH factor plays a role in the developmental migration and projection of gonadotropin-releasing hormone 3 neurons in zebrafish. Dev Dyn (2009) 238(1):66–75. doi: 10.1002/dvdy.21823
100. Yamamoto N, Oka Y, Kawashima S. Lesions of gonadotropin-releasing hormone-immunoreactive terminal nerve cells: effects on the reproductive behavior of male dwarf gouramis. Neuroendocrinology (1997) 65(6):403–12. doi: 10.1159/000127203
101. Ogawa S, Akiyama G, Kato S, Soga T, Sakuma Y, Parhar IS. Immunoneutralization of gonadotropin-releasing hormone type-III suppresses male reproductive behavior of cichlids. Neurosci Lett (2006) 403(3):201–5. doi: 10.1016/j.neulet.2006.02.041
102. Okuyama T, Yokoi S, Abe H, Isoe Y, Suehiro Y, Imada H, et al. A neural mechanism underlying mating preferences for familiar individuals in medaka fish. Science (2014) 343(6166):91–4. doi: 10.1126/science.1244724
103. Dornan WA, Malsbury CW. Peptidergic control of male rat sexual behavior: The effects of intracerebral injections of substance P and cholecystokinin. Physiol Behav (1989) 46(3):547–56. doi: 10.1016/0031-9384(89)90034-6
104. Katsouni E, Sakkas P, Zarros A, Skandali N, Liapi C. The involvement of substance P in the induction of aggressive behavior. Peptides (2009) 30(8):1586–91. doi: 10.1016/j.peptides.2009.05.001
105. Ebner K, Rupniak NM, Saria A, Singewald N. Substance P in the medial amygdala: emotional stress-sensitive release and modulation of anxiety-related behavior in rats. Proc Natl Acad Sci (2004) 101(12):4280–5. doi: 10.1073/pnas.0400794101
Glossary.
Keywords: Substance P, kisspeptin, gonadotropin-releasing hormone, preoptic area, reproduction
Citation: Ogawa S, Ramadasan PN, Anthonysamy R and Parhar IS (2021) Sexual Dimorphic Distribution of Hypothalamic Tachykinin1 Cells and Their Innervations to GnRH Neurons in the Zebrafish. Front. Endocrinol. 11:534343. doi: 10.3389/fendo.2020.534343
Received: 12 February 2020; Accepted: 28 December 2020;
Published: 03 March 2021.
Edited by:
Honoo Satake, Suntory Foundation for Life Sciences, JapanCopyright © 2021 Ogawa, Ramadasan, Anthonysamy and Parhar. This is an open-access article distributed under the terms of the Creative Commons Attribution License (CC BY). The use, distribution or reproduction in other forums is permitted, provided the original author(s) and the copyright owner(s) are credited and that the original publication in this journal is cited, in accordance with accepted academic practice. No use, distribution or reproduction is permitted which does not comply with these terms.
*Correspondence: Ishwar S. Parhar, aXNod2FyQG1vbmFzaC5lZHU=