- 1Post-graduate Program in Chemical Biology – Institute of Environmental Sciences, Chemical and Pharmaceutical, Federal University of São Paulo - UNIFESP, Diadema, Brazil
- 2Department of Biological Sciences, Institute of Environmental Sciences, Chemical and Pharmaceutical, Federal University of São Paulo - UNIFESP, Diadema, Brazil
We recently demonstrated that palmitoleic acid (C16:1n7), a monounsaturated fatty acid, increases the metabolic and oxidative capacity of 3T3-L1 adipocytes. Herein, the effect of 16:1n7 supplementation on metabolic parameters on white adipose tissue (WAT) and liver of obese mice induced by a high-fat diet (HFD) was addressed by analyzing metabolic (dys)function and altered genes expression in adipose tissue, as well as liver and serum biochemistry analysis. For this purpose, mice were induced to obesity for 8 weeks, and from the 5th week, they received 16:1n7 (300 mg/kg per day) or water for 30 days, by gavage. Subcutaneous inguinal (ING) and epididymal (EPI) WAT were removed for analysis of metabolic, (anti)inflammatory, adipogenic, and thermogenic genes expression by real-time reverse transcriptase–polymerase chain reaction. Additionally, metabolic activities of isolated adipocytes, such as glucose uptake, lipogenesis (triacylglycerol esterification), β-oxidation, and lipolysis in ING adipocytes, were also assessed. Despite the higher fat intake, the HFD group showed lower food intake but higher body weight, increased glucose, significant dyslipidemia, and increased liver and adipose depot mass, accompanied by liver steatosis. The 16:1n7 supplementation slowed down the body mass gain and prevented the increase of lipids in the liver. HFD+n7 animals presented increased fatty acid oxidation and lipogenesis compared to control, but no effect was observed on lipolysis and glucose uptake in ING isolated adipocytes. Besides, 16:1n7 increased the content of the mRNA encoding FABP4, but partially prevented the expression of genes encoding ATGL, HSL, perilipin, lipin, C/EBP-α, PPAR-γ, C/EBP-β, CPT1, NRF1, TFAM, PRDM16, and nitric oxide synthase 2 in ING depot from HFD group of animals. Finally, HFD increased Mcp1 and Tnfα expression, and 16:1n7 promoted a more marked increase in it. In summary, the data show that palmitoleic acid promotes metabolic changes and partially prevents the increase in gene expression on adipocytes triggered by obesity, suggesting that HFD+n7 animals do not require the same magnitude of metabolic adaptation to cope with energy demand from the HFD. In the long term, the effects of 16:1n7 may be more evident and beneficial for the function/dysfunction of WAT from an obese organism, with relevant repercussions in the systemic metabolic homeostasis.
Introduction
Obesity is the condition most often related to increased risk of developing metabolic disorders such as dyslipidemia, non-alcoholic fatty liver disease, insulin resistance and type 2 diabetes, hypertension, and cardiovascular disease, all of which contribute to higher death risk. Obesity results from the white adipose tissue (WAT) expansion due to an excessive triacylglycerol (TAG) storage triggered by a positive energy balance [e.g., during the high-fat diet (HFD)–induced obesity], which favors adipocyte hypertrophy, as well as metabolic tissue dysfunction that is closely related with a state of low-grade inflammation in WAT (1).
Increased lipogenesis and fatty acid uptake lead to TAG accumulation in adipose cells, whereas lipolysis and β-oxidation promote lipid decrease. Lipogenesis depends on lipogenic enzymes responsible for re-esterification of free fatty acids with glycerol and for de novo synthesis of TAG (2–4), which in turn increases the adipocyte size. On the other hand, sequential action of various lipolytic enzymes breaks down TAGs and mobilizes fatty acids (5), which may be released to the circulatory system and used as fuel by other tissues, re-esterified back to TAG, or oxidized directly inside the adipocyte (3, 4, 6, 7). Disturbances in these processes of WAT lipid metabolism, such as lipogenesis, lipolysis, and fatty acid β-oxidation, precipitates the metabolic diseases (4, 8).
Interestingly, metabolic diseases are also triggered by impaired differentiation of adipocytes in WAT (2, 9). This event, known as adipogenesis, is essential for WAT turnover and expansion in lean and obese subjects (10). It has been now accepted that a healthy WAT is characterized by the presence of smaller and more numerous adipocytes, suggestive of tissue expansion by increased adipogenesis (11).
Moreover, WAT is an endocrine organ that produces and releases numerous factors to the circulation to regulate food intake and energy expenditure, in addition to glucose and lipid metabolism, among other effects (12). Finally, WAT synthesizes and secretes palmitoleic acid (16:1n7), an omega-7 monounsaturated fatty acid that has shown beneficial metabolic effects in mice and humans (13–16). Considered a “lipokine” released by WAT, it promotes an increase in insulin sensitivity in muscle (14) and WAT (17, 18). Besides, 16:1n7 increased lipolytic and lipogenic activity, as well as oxygen consumption, fatty acid oxidation, and ATP content of 3T3-L1 white adipocytes (19). Furthermore, this fatty acid was associated with exercise-induced cardiac hypertrophy (20) and the anti-inflammatory action on macrophages (21, 22).
In an experimental model of spontaneous type 2 diabetes (KK-Ay mice), treatment for 4 weeks with 16:1n7 (300 mg/kg per day) increased insulin sensitivity and decreased the glycemic curve after glucose tolerance test (GTT) and plasma insulin concentration. Also, 16:1n7 decreased the concentration of triglycerides in plasma and reduced the gene expression of fatty acid synthase (FAS), sterol-regulatory element binding protein (SREBP-1c), stearoyl-CoA desaturase 1 (SCD1), tumor necrosis factor-α (TNF-α), and resistin in mesenteric WAT (16). Finally, in C57BL/6 mice with 12 weeks' HFD-induced obesity, the 2 weeks' treatment with 16:1n7 improved the GTT and also the insulin tolerance test (23). There is a great interest in therapies to treat obesity-associated diseases, or at least, agents that could improve some metabolic parameters triggered by this condition (such as non-alcoholic hepatic steatosis and adipose tissue dysfunction), making WAT somehow metabolically more active. Herein, we investigated the effects of palmitoleic acid on metabolic and genic parameters from WAT and liver of animals submitted to HFD-induced obesity.
Materials and Methods
Animals and Palmitoleic Acid Supplementation
Eight-week-old male C57BL/6 mice were maintained under controlled light–dark cycle of 12–12 h, temperature of 24 ± 1°C, and relative humidity 53 ± 2%. The mice were obtained from the Center for Development of Experimental Models (CEDEME), Federal University of São Paulo. The experimental protocol remained for 8 weeks. In the first 4 weeks (period I), mice were divided into two groups: (a) control (low fat) diet (control) and (b) HFD (obese). In the next 4 weeks (period II), the HFD group was subdivided into obese and (c) HFD supplemented with palmitoleic acid (obese+n7) groups. Control diet contains 76% carbohydrate, 15% protein, and 9% fat, and an HFD contains 26% carbohydrate, 15% protein, and 59% fat, in % kcal. Supplementation was performed by oral gavage at 300 mg/kg per day of pure palmitoleic acid (16:1n7) (Sigma, St. Louis, MO, USA) (17, 18). The control and obese groups received water by gavage at the same volume (~10 μL, according to the body weight). Gavages were carried daily between 16:00 and 17:00 h. All procedures were approved by the Ethics Committee on Animal Use of the Federal University of São Paulo (CEUA 8347020315).
Experimental Procedure
Body weight and food intake were measured weekly, and the food and energy efficiency were calculated by the ratio of body weight gain (g) to food ingestion (g), or by ratio of body weight gain (g) to caloric intake (kcal). After 8 weeks of the experimental protocol, 10- to 12-h fasted mice were anesthetized with isoflurane and killed by cervical dislocation, and after blood was collected through puncturing the orbital plexus. Blood samples were centrifuged at 1,500 rpm for 20 min at 4°C, and serum was stored at −80°C. Liver and adipose fat depots: ING (subcutaneous), EPI (epididymal), and RP (retroperitoneal), were harvested, weighed, and processed as described below.
Biochemical Analyses of Serum and Liver Samples
Plasma glucose, TAG, total cholesterol, low-density lipoprotein (LDL) cholesterol and high-density lipoprotein cholesterol, aspartate aminotransferase (AST), alanine aminotransferase (ALT), and gamma-glutamyltransferasase (γ-GT) levels were determined by colorimetric assays (Labtest Diagnostics, Lagoa Santa, MG, Brazil).
Liver TAG and total cholesterol content were measured in lipid extracts from previously frozen liver tissue using the same colorimetric assay kits. Fatty acids were extracted from the liver using previously described methods (24, 25). Data obtained were normalized by tissue protein concentration measured by BCA protein assay kit (Bio-Rad).
Adipocyte Isolation
For metabolic activities studies (glucose uptake, TAG esterification, β-oxidation and lipolysis), adipocytes were isolated from subcutaneous ING fat depot, as previously described (26). A small number of adipocytes were photographed under an optical microscope (100× magnification) using a microscope camera (Moticam 1000; Motic, Richmond, British Columbia, Canada), and mean adipocyte diameter was determined by measuring 50 cells using Motic-Images Plus 2.0 software.
Incorporation of [1-14C]-Palmitate Into TAG (Lipogenesis)
ING adipocytes (106 cells mL−1) were incubated in Krebs/Ringer/phosphate buffer (pH 7.4) containing bovine serum albumin (BSA) (1%), glucose (2 mM), and palmitate (200 μM), saturated with a gas mixture of 95% O2 and 5% CO2. [1-14C]-palmitate, was then added to the buffer (1,850 Bq/tube) and left for 2 h at 37°C. At the end of the incubation period, the mixture was transferred to a 1.5-mL tube containing 400 μL of silicone oil and centrifuged for 30 s. The cell pellet on the top of the oil layer was transferred to polypropylene tubes containing 2.5 mL of Dole's reagent for lipid extraction. After addition of n-heptane (1.5 mL) and distilled water (1.5 mL), tubes were vortexed, and the mixture decanted for 5 min. An aliquot of the upper phase was collected into a scintillation vial for determination of radioactivity trapped into TAG (1450 LSC, CouterMicro-Beta, Trilux; Perkin Elmer, Waltham, MA, USA). Results are expressed as nmol of palmitate incorporated into TAG per 1 × 106 cells h−1. A similar procedure was used in our previous study (19, 26).
Decarboxylation of [1-14C]-Palmitate (Fatty Acid Oxidation)
ING adipocytes (106 cells mL−1) were incubated in Krebs/Ringer/phosphate buffer (pH 7.4) containing BSA (1%), glucose (2 mM), and palmitate (200 μM), saturated with a gas mixture of 95% O2 and 5% CO2,. [1-14C]-palmitate was then added to the buffer (1,850 Bq/tube) and left for 2 h at 37°C. At the end of the incubation period, 0.2 mL of H2SO4 8 N was injected into the tubes in order to cause the rupture of the cells and release the CO2 resulting from the reaction. This CO2 was collected and retained by adsorption on piece of Whatman filter paper (2.0 × 4.0 cm) soaked in an ethanolamine solution (0.2 mL). After 45 min of CO2 trapping, the filter paper was removed and transferred to scintillation vials for radioactivity counting (19). Results are expressed as nmol of CO2 released per 1 × 106 cells h−1.
2-Deoxy-d-Glucose (2-DG) Uptake
ING adipocytes (1 × 106 cells mL−1) were incubated with or without insulin (10 nmol L−1) in buffer composed of (mM): 140 NaCl, 20 HEPES, 5 KCl, 2.5 MgSO4, 1 CaCl2, and BSA 1% (pH 7.4) for 20 min at 37°C. Subsequently, 2-deoxy-D-[3H]-glucose (0.4 mmol L−1, 1,850 Bq per tube or well) was added, and the reaction was allowed to occur for exactly 3 min. The reaction was interrupted by adding 250 μL of ice-cold phloretin (0.3 mmol L−1 in Earle's salts, HEPES 10 mm, BSA 1%, and dimethyl sulfoxide 0.05%). At the end of incubation, the glucose uptake was measured as described in our previous studies (27, 28). The results are expressed in relation to the surface area (pmol/cm2).
Lipolysis Measurement
The lipolysis rate was measured by quantifying the glycerol (Free Glycerol Determination Kit; Sigma) produced by the cells (1). For this, adipocytes (1 × 106 cells/mL) were incubated in Krebs/Ringer/phosphate buffer (pH 7.4) containing BSA (20 mM) and glucose (5 mM) for 30 min at 37°C in the absence (basal) and presence (stimulated) of isoproterenol (2 × 10−6 M). The reaction was stopped on ice, and media was carefully collected for measurement of glycerol release. Results were expressed as nanomoles of glycerol per 1 × 106 adipocytes.
RNA Extraction and Quantitative Real-Time Polymerase Chain Reaction
Total RNA was extracted from ING and EPI whole adipose depots using Trizol reagent (Invitrogen Life Technologies), analyzed for quality on ratios 260/280 and 260/230 nm on NANODROP (Thermo Scientific), and reverse transcribed to cDNA using the Superscript III cDNA kit (Thermo Scientific, EUA). Gene expression was evaluated by real-time quantitative polymerase chain reaction (PCR) using a Rotor Gene (Qiagen) and SYBR Green as fluorescent dye, as previously described (29). Analysis of real-time PCR data was performed using the 2−ΔΔCT method. Data are expressed as the ratio between the expression of the target gene and housekeeping gene (Gapdh). Primers used are presented in Table 1.
Statistical Analysis
Data are presented as mean ± SEM. One-way analysis of variance (ANOVA) followed by Tukey posttest was used for the comparison between groups. GraphPad Prism 8.4.4 version (GraphPad Software, Inc., San Diego, CA, USA) was used for analysis. The level of significance was set at p < 0.05.
Results
Palmitoleic Acid Partially Prevented Food and Energy Efficiency Increase and the Body Mass Gain of Animals Submitted to Obesity by HFD
To characterize the obesity model, we evaluated the animals' food intake and food and energy efficiency. The HFD promoted a significant decrease in food intake (by 50%; P < 0.05), as well as increased both food and energy efficiency (2,6- and 1,5-fold, respectively; P < 0.05), and 16:1n7 supplementation partially prevented the increase in food, as well as energy efficiency. Besides, palmitoleic acid did not affect food intake, because there was no difference between groups of obese animals. We also measured glucose, triglycerides, and total cholesterol and fractions plasma concentrations. HFD promoted an increase in these parameters in the plasma, but palmitoleic acid did not affect these parameters (Table 2).
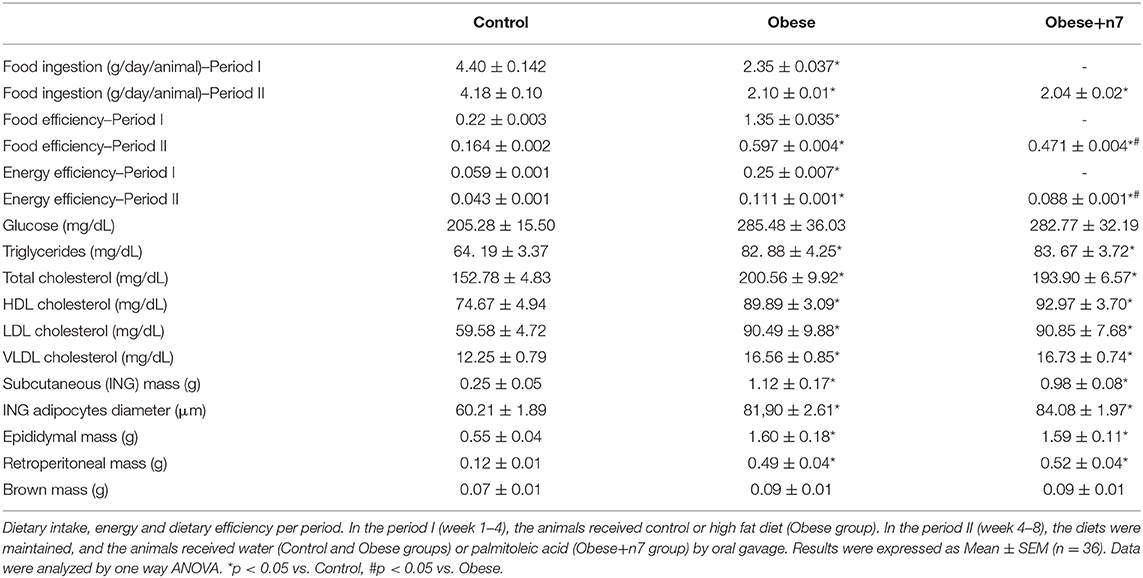
Table 2. Dietary intake, food and energy efficiency, fasting plasma glucose, triglycerides, total and fractions cholesterol, and adipose tissues mass.
The weight gain curve of the animals is shown in Figure 1A. We observed that the animals from the HFD group had an increase in body mass when compared to the control group. To analyze whether palmitoleic acid had any effect on the weight gain of obese animals, we analyzed the body mass gain during period II (weeks 4–8) of the experimental protocol, as well as the mass of the animals' fat pads. The HFD promoted an increase in body mass gain when compared to the control group in period I (by 70%; Figure 1B) and II (by 130%; Figure 1C), and palmitoleic acid was able to partially prevent this effect (decrease of 25% compared to HFD; P < 0.05; Figure 1C), even without exerting any effect on the mass of the subcutaneous (ING), epididymal, retroperitoneal, and brown fat pads or on the cell diameter of ING adipocytes (Table 2).
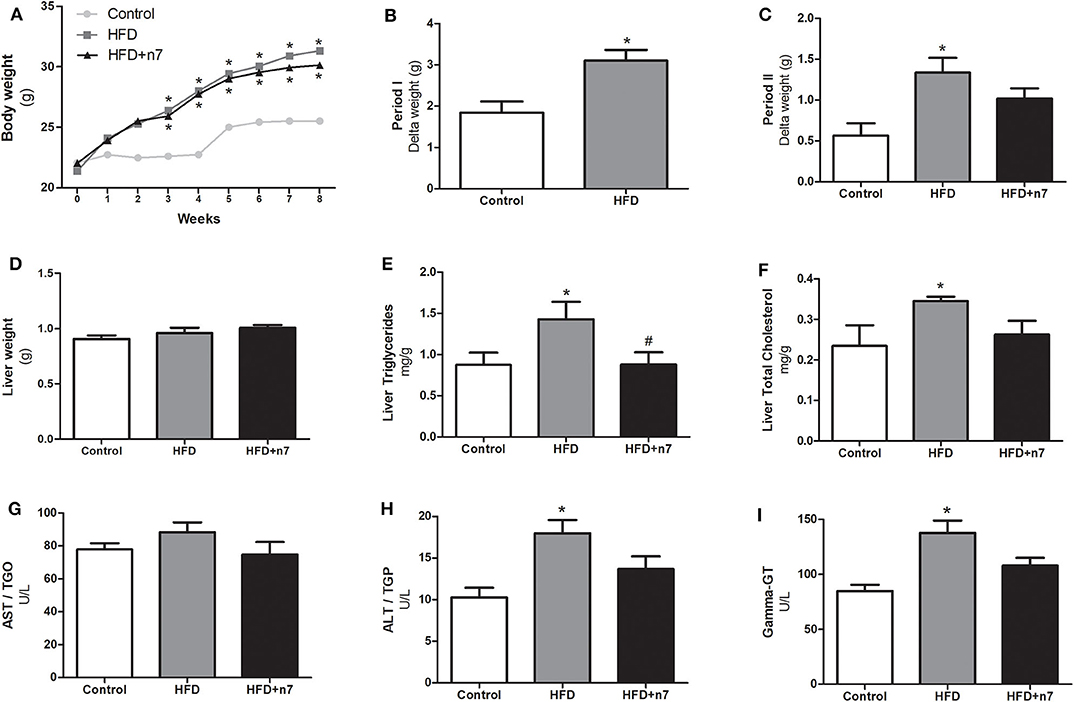
Figure 1. Weight gain curve (A), Body mass gain in period I (B) and II (C), liver mass (D), hepatic triglyceride in mg/g of liver weight (E), total hepatic cholesterol in mg/g of liver weight (F), AST (G), ALT (H), and γ-GT (I) levels in U/L in plasma. In period I (weeks 1–4), the animals received control or high-fat diet (HFD). In period II (weeks 4–8), the diets were maintained, and the animals received either water (control and HFD groups) or palmitoleic acid (HFD+n7 group) by oral gavage. Results were expressed as mean ± SEM (n = 12). Data were analyzed by one-way ANOVA. *p < 0.05 vs. control, #p < 0.05 vs. obese.
Palmitoleic Acid Prevented Hepatic Steatosis in Obese Mice
To assess the effect of the HFD as well as palmitoleic acid in liver, the following parameters were analyzed: liver mass, triglyceride, and total cholesterol. Also, the AST, ALT, and γ-GT enzyme plasma concentrations were determined. HFD had no effect on the mass of liver (Figure 1D), but significantly increased: liver triglycerides and total cholesterol concentration and ALT and γ-GT plasma concentrations (87, 57, 80, and 63%, respectively; P < 0.05; Figures 1E–G,I). Palmitoleic acid was able to bring liver triglycerides to the same concentrations as in control animals (Figure 1E) and promoted a partial decrease in the other parameters (Figures 1F,H,I).
Palmitoleic Acid Increased TAG Esterification and Oxidation of Fatty Acids in Subcutaneous (ING) WAT of Obese Mice
Taking in account that palmitoleic acid partially prevented the food and energy efficiency, as well as the body mass gain, we next evaluated the effects of palmitoleic acid on the adipose tissue metabolism in animals consuming a HFD. Palmitoleic acid promoted a significant increase in TAG esterification (by 80%; P < 0.05; Figure 2A) and fatty acid oxidation (by 70%; P < 0.05; Figure 2B).
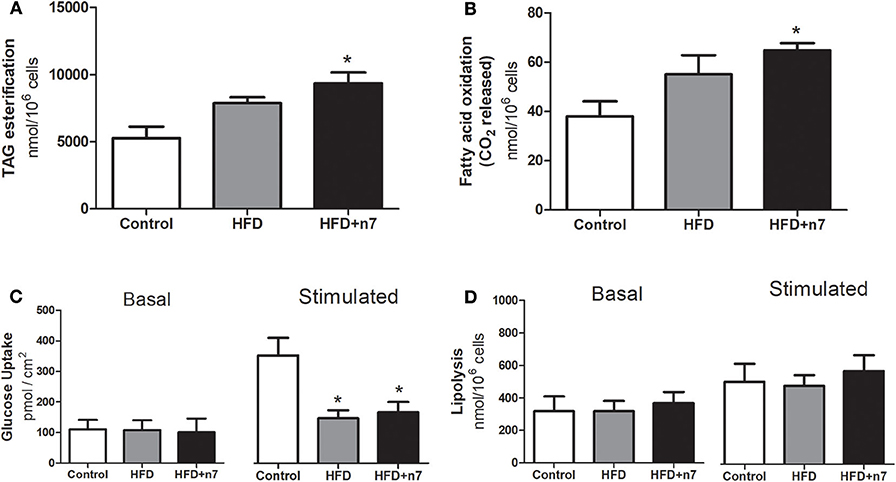
Figure 2. Metabolic activities in isolated adipocytes from ING fat depot. Lipogenesis/TAG esterification in nmol/106 cells (A), fatty acid oxidation in nmol/106 cells (B), basal and insulin-stimulated glucose uptake in pmol/cm2 (C), basal and stimulated (by isoproterenol) lipolysis in nmol/106 cells (D). Results were expressed as Mean ± SEM (n = 6). Data were analyzed by one-way ANOVA. *p < 0.05 vs. control.
HFD induced a significant reduction in maximally insulin-stimulated glucose uptake in obese animals compared with control animals (58%; Figure 2C), and this effect was not prevented by 16:1n7 supplementation.
Finally, ING adipocytes were evaluated for lipolysis activity (basal and stimulated by isoproterenol), but no statistical difference was observed between the groups (Figure 2D).
Palmitoleic Acid Partially Modulates the Expression of Genes Involved in Lipogenesis (Uptake and Fatty Acid Esterification), Lipolysis, and Fatty Acid Oxidation
The expression of genes encoding important proteins and enzymes involved in metabolic activities such as glucose and fatty acids uptake (Lpl, Fabp4, and Glut-4), de novo synthesis and esterification of fatty acids—lipogenesis (Fasn, Acc1, lipin, Dgat1, and Dgat2), lipolysis (Atgl, Hsl, and perilipin)—and fatty acid oxidation (Cpt1), as well as endocrine function (leptin and Adipoq), were analyzed in subcutaneous ING and visceral EPI fat depots and are shown in Figure 3.
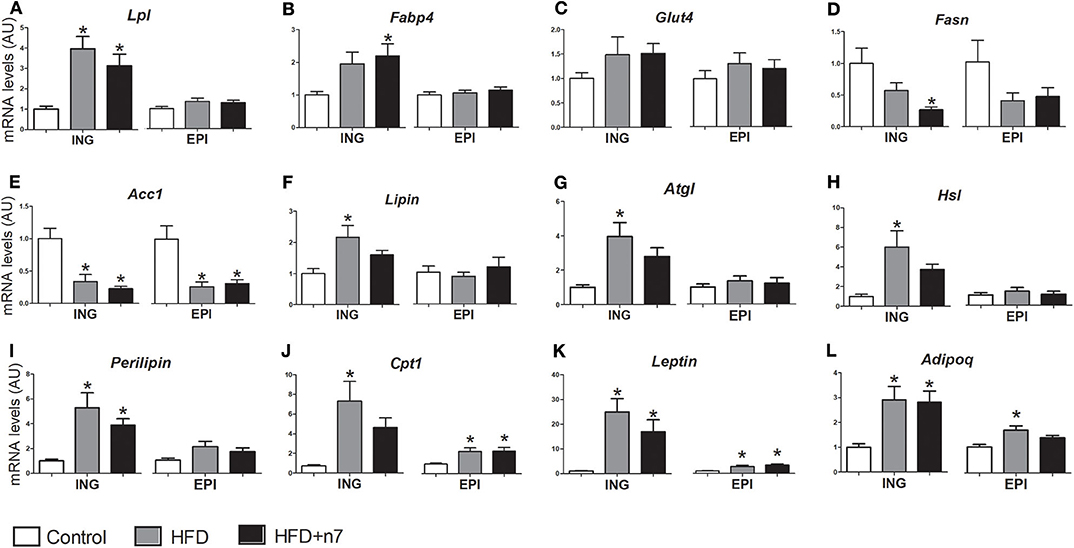
Figure 3. mRNA levels of Lpl (A), Fabp4 (B), Glut4 (C), Fasn (D), Acc1 (E), lipin (F), Atgl (G), Hsl (H), perilipin (I), Cpt1 (J), leptin (K), Adipoq (L) expressed by isolated adipocytes from ING and EPI fat depots in arbitrary units (AU), normalized by Gapdh. Results were expressed as mean ± SEM (n = 12). Data were analyzed by one-way ANOVA. *p < 0.05 vs. control.
HFD increased the expression of Lpl and perilipin (by 3- and 4.3-fold, respectively; P < 0.05; Figures 3A,K) in ING depot and decreased the expression of Acc1 (by 70%; Figure 3E) in ING and EPI depots. Palmitoleic acid positively modulated Fabp4 expression (by 120%; Figure 3B) and decreased Fasn expression (74%; Figure 3D) only in ING WAT. Glut4 gene expression was not modulated (Figure 3C).
The HFD also increased lipin, Dgat1, Dgat2, Atgl, Hsl, and Cpt1 expression in ING depot, but palmitoleic acid treatment partially prevented (Figures 3F,I,J,L) or completely abolished (Figures 3G,H) this effect. These genes expression were not modulated by HFD or palmitoleic acid in ING fat depot, except by Cpt1, whose expression increased (2-fold; Figure 3J) in both HFD and HFD+n7 groups.
Finally, HFD consumption caused an increase in leptin (Figure 3K) and Adipoq (Figure 3L) gene expression in both ING and EPI depots, which was not altered by treatment with palmitoleic acid.
Palmitoleic Acid Prevents Effects Promoted by the HFD on the Expression of Adipogenic and Mitochondrial Genes
Regarding the effects of the HFD and palmitoleic acid on the expression of genes involved in adipogenesis (Cebpa, Pparg, Pref1, and Cebpb), mitochondrial biogenesis (Nrf1, Tfam, Pgc1alfa, and Prdm16), and antioxidant function (Sod1, Sod2, Gpx, and Catalase), the data are illustrated in Figure 4.
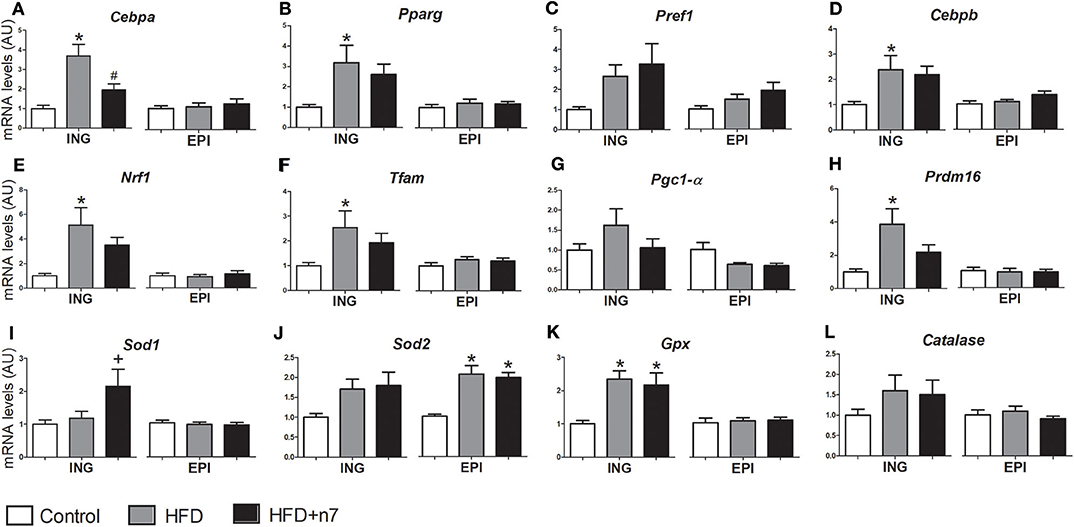
Figure 4. mRNA levels of Cebpa (A), Pparg (B), Pref1 (C), Cebpb (D), Nrf1 (E), Tfam (F), Pgc1-α (G), Prdm16 (H), Sod1 (I), Sod2 (J), Gpx (K), and Catalase (L) expressed by isolated adipocytes from ING and EPI fat depots in arbitrary units (AU), normalized by Gapdh. Results were expressed as mean ± SEM (n = 12). Data were analyzed by one-way ANOVA. *p < 0.05 vs. control, #p < 0.05 vs. obese, +p = 0.05 vs. all groups.
In ING fat depots, the expression of Cebpa (Figure 4A), Pparg (Figure 4B), Cebpb (Figure 4D), Nrf1 (Figure 4E), Tfam (Figure 4F), Prdm16 (Figure 4H), and Gpx (Figure 4K) was higher in the HFD group compared to control. Palmitoleic acid partially prevented all these increases, except for Cebpa expression that was reduced to control levels, and Gpx, which was not modulated by 16:1n7. Also, palmitoleic acid significantly increased Sod1 gene expression (by 80%; Figure 4I). There was no significant difference in Pref1 (Figure 4C), Pgc1-α (Figure 4G), Sod2 (Figure 4J), and catalase (Figure 4L) expression levels. On the other hand, in EPI fat depots, the expression of adipogenic and mitochondrial genes was not modulated by our treatment, except by an increase in Sod2 gene expression (2-fold; Figure 4J) in both HFD and HFD+n7 groups.
Palmitoleic Acid Modulated the Expression of Genes Related to Inflammation
We also investigated, by real-time quantitative PCR, the effect of HFD, associated or not with 16:1n7, on the expression of genes related to inflammation (Mcp1, Tnf-α, Nos2) in ING EPI fat depots.
HFD consumption caused an increase in Mcp1 (Figure 5A) in both ING and EPI fat depots and an increase in Tnf-α (Figure 5B) in EPI depot. Palmitoleic acid treatment did not reverse these effects. On the other hand, in ING fat depot 16:1n7 significantly increased the expression of Tnf-α (2-fold; Figure 5B) and partially prevented the increase in Nos2 expression (Figure 5C) triggered by the HFD.
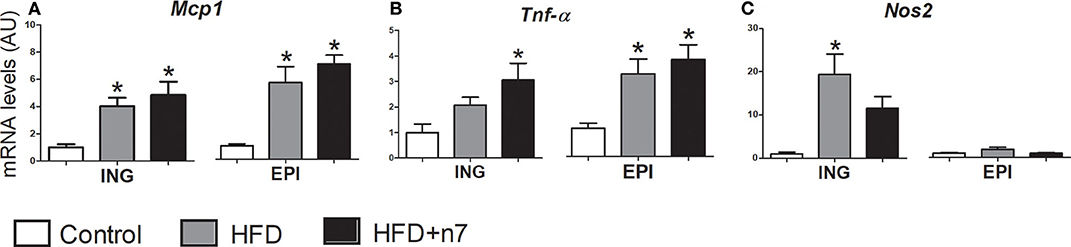
Figure 5. mRNA levels of Mcp1 (A), Tnf-α (B), and Nos2 (C) expressed by isolated adipocytes from ING and EPI fat depots in arbitrary units (AU), normalized by Gapdh. Results were expressed as mean ± SEM (n = 12). Data were analyzed by one-way ANOVA. *p < 0.05 vs. control.
Discussion
In the present work, we have tested the effects of palmitoleic acid in C57BL/6 mice fed a HFD for 8 weeks. We show that palmitoleic acid promotes metabolic and transcriptional changes in adipose tissue, improving mice hepatic parameters. Interestingly, palmitoleic acid prevented an increase in the expression of Cebpa and Pparg transcription factors, which were induced by HFD in subcutaneous ING adipocytes. The expression of several CEBPα and PPARγ target genes, such as Atgl, Hsl, and perilipin (lipolysis-related genes); Lpl, lipin, Dgat1, and Dgat2 (fatty acid uptake and lipogenesis-related genes); leptin and Nos2 (obesity inflammation-related genes); and Cpt1, Nrf1, Tfam, and Prdm16 (fatty acid oxidation and biogenesis-related genes) were also partially reversed in the ING fat depots of animals treated with palmitoleic acid. Therefore, palmitoleic acid seems to mitigate the impacts triggered by HFD. Our results suggest that WAT from animals subjected to HFD and palmitoleic acid gavage does not have the same adaptation challenge inflicted by HFD as that from animals subjected only to HFD and water gavage.
The challenge inflicted in animals fed an HFD led to a disruption of metabolic and energy homeostasis, with drastic repercussions in body mass and plasma lipid profile (increased triglycerides, total cholesterol, and LDL cholesterol). Treatment of HFD-induced obese animals for 4 weeks with palmitoleic acid could not reverse these features, although it prevented (even if very partially) the body mass gain. This finding is reinforced by the fact that palmitoleic acid partially prevented an increase in food and energy efficiency.
In agreement with previous findings in obese animals with hepatic steatosis (23), palmitoleic acid was not able to reduce the increment in WAT induced by HFD. We hypothesize that because palmitoleic acid is a nutrient it may take longer than 4 weeks to exert significant phenotypic alterations in WAT. Nevertheless, it exerts subtle effects that may bring important long-term repercussions.
The analysis of ING adipocytes' size (average cellular diameter) shows that they are hypertrophic. It is well-established in the literature that there is an important increase in cellular diameter in obesity, especially in pathological obesity (27, 30). We show that the treatment of obese animals with palmitoleic acid for 4 weeks could not attenuate adipocytes' hypertrophy.
We show that the 4 weeks' treatment with palmitoleic acid could prevent the increase in hepatic triglycerides and total cholesterol content and hepatic enzymes (ALT and γ-GT) promoted by HFD, even though the liver mass was not different among the groups. Previous work shows that the treatment with palmitoleic acid for only 15 days did not exert hepatic effects (23). Yang et al. (16) showed that palmitoleic acid supplementation promoted a reduction of hepatic triglycerides content in a model of diabetic genetically modified mice. It has been also shown that 16:1n7 improves hepatic steatosis in FABP knockout mice (aP2-mal1−/−) (14). Taken together, these findings strongly suggest that palmitoleic acid is a candidate for the prevention of liver steatosis caused by HFD.
Interestingly, a comparison of the results on fatty acid oxidation and TAG esterification in subcutaneous WAT of animals from both groups revealed that only HFD+n7 animals had an increase in both parameters. We have previously shown, in 3T3-L1 adipocytes, that palmitoleic acid increases not only lipogenesis and palmitate oxidation (19) but also lipolysis and glucose uptake and oxidation (17–19). Herein, our experimental model associates palmitoleic acid treatment to an HFD-induced obesity condition. Our results suggest that 16:1n7 promotes a persistent increase in lipogenesis (fatty incorporation into TAG) and fatty acid oxidation in WAT adipocytes. In agreement with these data, when we compared HFD group with HFD+n7, the former presented a slightly higher expression of Lpl gene in ING fat depots. Lpl encodes an enzyme (lipoprotein lipase) that hydrolyzes TAG molecules found in lipoproteins such as chylomicrons and very low-density lipoproteins. Palmitoleic acid also partially prevented the increase in expression of lipin but significantly hampered the increase in Dgat1, and Dgat2 gene expression, which encode enzymes related to fatty acid esterification for TAG synthesis. On the other hand, palmitoleic acid promoted a significant increase in Fabp4 expression, a protein that dictates the partitioning of lipids inside adipocytes. Therefore, one hypothesis is that while HFD leads to higher plasma and liver lipid concentration, palmitoleic acid promotes an increase in fatty acid uptake by ING adipocytes, incorporating that as neutral lipids or phospholipids, among other possibilities in the cell metabolism, such as β-oxidation.
It is well-known that hypercaloric (glucose) diet increases de novo lipogenesis (31–34), leading to an increase of circulating palmitoleic acid. Therefore, palmitoleic acid is considered a marker of de novo lipogenesis (14). It has also been described that de novo lipogenesis reduces the effects of HFD (27, 35, 36). Corroborating the literature, we found that Fasn and Acc1 gene expression was reduced in the HFD group, but these reductions were more significant in the HFD+n7 group for both ING and EPI fat depots. These data reinforce our previous findings showing that 16:1n7 reduces de novo lipogenesis (17). Even though there is a reduction in de novo synthesis of fatty acids, animals fed an HFD presented a significant increase in lipogenesis by an increase in TAG esterification.
Glucose uptake was also analyzed in subcutaneous ING adipocytes in basal and insulin-stimulated states. We did not detect any differences in basal glucose uptake among control, HFD, and HFD+n7 groups, although the HFD has significantly reduced the insulin-stimulated glucose uptake in both HFD and HFD+n7 groups. Although it was described by other researchers that 16:1n7 increases muscle glucose uptake (14), and we have previously demonstrated this increase in eutrophic mice epididymal WAT and 3T3-L1 adipocytes (17), we could not detect 16:1n7 effects in glucose uptake and GLUT4 mRNA levels in ING fat depot of HFD-fed mice. On the other side, mice fed an HFD for 12 weeks and treated for 2 weeks with palmitoleic acid after the 10th week presented lower glucose tolerance and higher insulin sensitivity (23).
Concerning the lipolysis, the results showed that HFD did not modulate this pathway in subcutaneous WAT. Also, palmitoleic acid has not affected lipolysis in obese animals, although we have previously demonstrated that 16:1n7 treatment increases lipolysis in epididymal white adipocytes from eutrophic mice and in 3T3-L1 cell line (18, 19). Even though palmitoleic acid did not (significantly) decrease the high expression of Atgl, Hsl, and perilipin (lipolysis related genes) observed in the HFD group, there was a clear tendency to reduce it, although no difference was detected in the lipolytic assay in the ING adipocytes. It is important to emphasize that we did not perform the measures in a visceral adipose depot but in a subcutaneous depot (ING), whose expansion is believed to protect against pathologic visceral adipose expansion in obesity. Different from visceral adipocytes, whose hypertrophy is positively correlated with metabolic diseases and undergo drastic changes in size due to diet (6–10 times more lipolytic), ING adipocytes are less affected by HFD (27).
In agreement with our findings, it was demonstrated that there is an increase in perilipin gene expression in obesity (37). It was described that depending on perilipin activation status, it can act protecting and delimitating the lipid droplet or facilitating TAG hydrolysis (38).
The HFD promoted a substantial increase in the expression of Cpt1, Nrf1, Tfam, and Prdm16 in ING (but not in EPI) fat depot, which are genes related to fatty acid oxidation, mitochondrial function, and biogenesis (29, 39, 40). The treatment with palmitoleic acid partially prevented these effects in the subcutaneous WAT depot. These findings lead us to hypothesize that mitochondria could undergo a metabolic adaptation, increasing adipocytes' mitochondrial activity, trying to deal in this early stage of obesity, with the high supply of fatty acids from HFD. Obese individuals have higher SOD2, PPARα, and PGC1β protein expression than eutrophic individuals (41). Considering the increase in fatty acid oxidation in HFD+n7 animals, it seems that even though these animals received the same diet as the HFD group, they do not need to adapt in the same proportion. Warfel et al. (42) have not detected any difference in Cpt1 gene expression in C57BL/6 subcutaneous WAT, although their obesity experimental model lasted 40 weeks. Confirming the failure in mitochondrial biogenesis with obesity progression, ob/ob mice showed reduced expression in Nrf1, Tfam, and Pgc-1α (43). PRDM16 induces Pgc-1α and Ucp1 expression and is a critical molecular unit that controls browning differentiation (44). Besides acting on the browning process, PRDM16 increases mitochondrial biogenesis, uncoupling, as well as oxygen consumption in WAT (45). Mitochondria have a crucial role in controlling the energetic balance, ATP synthesis, energy expenditure, and energetic support during adipogenesis, among several other functions. Excess of energy substrates leads to mitochondrial dysfunction, harming glucose, and lipid cell metabolism. Adipocytes help to maintain the proper balance between energy storage and expenditure. This balance requires a preserved mitochondrial function to provide proper support to WAT metabolic functions (45–47).
Concerning the expression of adipogenic transcription factors in ING and EPI fat depots, we observed that Cebpb, Cebpa, and Pparg gene expression were significantly increased in the HFD animals, but not in the HFD+n7 in the subcutaneous WAT. These findings bring us evidence that palmitoleic acid attenuates adipogenesis triggered by HFD.
In ING and EPI fat depots, the expression of inflammatory cytokines genes, Mcp1 and Tnf-α, was increased in both HFD and HFD+n7 groups when compared to control. Interestingly, the expression of these genes is more pronounced in HFD+n7 group. These findings suggest that palmitoleic acid treatment triggers changes in the immune system response to obesity in WAT. Further experiments and immunological assays must be performed. It was described that 15 days of palmitoleic acid treatment after 12 weeks of HFD promotes the reduction of TNF-α gene and protein expression in the liver of C57BL/6 mice (23), but we could not detect this alteration in our experimental protocol.
When macrophages derived from bone marrow were grown in a lipid-enriched medium mimicking the condition of animals subjected to HFD, treatment with palmitoleic acid successfully reversed TNF-α gene and protein expression of these cells (48). Besides, it was shown that palmitoleic acid promoted an increase in oxygen consumption in macrophages and in 3T3-L1 adipocyte (19). Palmitoleic acid prevented insulin resistance in the C2C12 muscle cell line, inhibiting the palmitic acid activation of macrophage J774 (22). Also, palmitoleic acid reduced inflammation of macrophage activated by lipopolysaccharide (LPS), inhibiting nuclear factor κB. It was previously shown that palmitoleic acid has a relevant anti-inflammatory effect reducing TNF-α, interleukin 6 (IL-6), and MCP1 expression by intraperitoneal activated macrophages (21).
In this work, we found increased WAT inflammation in HFD+n7 group. It is possible that 16:1n7 is exerting a counter-regulatory mechanism in WAT, because it was described that palmitoleic acid has important anti-inflammatory action in macrophages and hepatocytes (21, 23). Interestingly, genetically modified mice with reduced TNF-α signaling fed an HFD for 11 weeks showed an increase in glucose intolerance. Besides, mice with 10 days of life showed diminished WAT adipogenic capacity, as well as increased hepatic steatosis, indicating that TNF-α signaling pathway is essential for WAT expansion and remodeling during obesity (49). These data corroborate the increased Tnf-α expression found in our experimental model.
On the other hand, it was described that leptin increases TNF-α and IL-6 production by monocytes (50) and that the treatment with LPS and TNF-α induces the increase in leptin mRNA levels in hamster epididymal, omental, renal, and subcutaneous WAT (51). TNF-α also induces the increase in leptin gene expression in 3T3-L1 adipocytes (52). Therefore, the increased expression of Tnf-α supports the increased leptin levels in HFD and HFD+n7 groups in our experimental model.
Leptin is an important marker of body adipose mass and positively correlates with it (53). The subcutaneous WAT is the main leptin source (54). In agreement with that, we have detected an increase in leptin gene expression for both ING and EPI fat depots of HFD animals, although this increase is less pronounced in the HFD+n7 group.
Beyond these findings, the consumption of an HFD increased Nos2 gene expression in ING fat depot, but this increase was partially prevented by 16:1n7. This gene encodes for a nitric oxide–inducible enzyme [inducible nitric oxide synthase (iNOS) or NOS2] that catalyzes the production of nitric oxide. Other studies have shown that palmitoleic acid promotes a reduction of Nos2 in bone marrow macrophages of mice fed an HFD (48), besides leading to an increase in oxygen consumption and fatty acid oxidation in these macrophages, corroborating our previous work using 3T3-L1 adipocytes (19).
Finally, we have verified that HFD promoted an increase in Gpx gene expression in subcutaneous WAT. The increase in fatty acid oxidation, as well as other energy substrates in mitochondria, can lead to an increase in the production of reactive oxygen species such as superoxide anion and peroxynitrite, which originate from the nitric oxide production by the enzyme NOS. We hypothesize that this effect is compensation for the high lipid demand created by the HFD, suggesting a pathway to control the formation of free radicals, as the antioxidant enzymes (SOD, catalase, and GPX) are involved in the endogenous defense against oxidative stress (55, 56). Five weeks of HFD was sufficient to reduce the activity of the antioxidant enzymes catalase and glutathione peroxidase activity in rat liver (57). Besides, in our experimental protocol, 16:1n7 promoted an important increase of Sod1 expression in the subcutaneous WAT. This result is in agreement with our recently published work with 3T3-L1 cell line where we demonstrated that 16:1n7 increases Sod1 gene expression (19).
In that same work (19) we also showed that 16:1n7 increases fatty acid oxidation, lipolysis and lipogenesis, and intracellular ATP content, as well as oxygen consumption in 3T3-L1 cells, suggesting that palmitoleic acid increases the adipocytes' basal metabolic rate. Herein, using an HFD-induced obesity in animal model, the concomitant increase in fatty acid oxidation and lipogenesis in WAT suggests that the WAT from 16:1n7–treated animals is metabolically more active than that of non-treated animals, corroborating our previous published work in 3T3-L1 adipocytes.
In brief, palmitoleic acid treatment softened the body mass gain, prevented some aspects of hepatic steatosis, regulated lipogenesis and fatty acid oxidation in ING adipocytes, and modulated the expression of genes involved in the main WAT metabolic activity (lipolysis and lipogenesis), as well as genes related to mitochondrial function and biogenesis, fatty acid oxidation, adipogenesis (cell differentiation), and inflammation-related genes (Figure 6). The action of 16:1n7 is subtle but beneficial, and our data suggest that when the results caused by both HFD and palmitoleic acid are in the same direction, they add to each other, but when these effects are opposite, they are overlapped by the HFD-induced obesity (at least initially), except by gene expression changes, which we do not know if or how it will affect adipocyte metabolic activities, energy homeostasis and therefore in animal body mass in the long term.
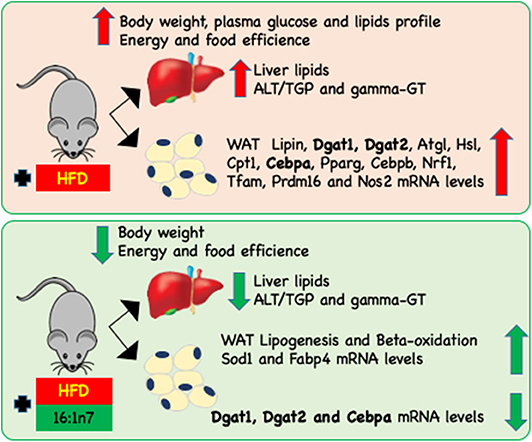
Figure 6. Schematic representation of changes induced by HFD on the body, liver, and inguinal (ING) adipose depot attenuated by 16:1n7 supplementation. HF diet led to increasing of body weight, food and energy efficiency, plasma glucose, triglyceride, total cholesterol and fractions, ALT/TGP and γ-GT, liver TAG, and total cholesterol levels, as well as lipin, Dgat1, Dgat2, Atgl, Hsl, Cpt1, Cebpa, Pparg, Cebpb, Nrf1, Tfam, Prdm16, and Nos2 mRNA levels in ING WAT. Treatment with 16:1n7 for 28 days reversed many of these effects. Additionally, 16:1n7 increased ING adipocytes lipogenesis and β-oxidation of fatty acids, and increased Sod1 and Fabp4 mRNA levels.
HFD is a challenge of metabolic regulation and energy homeostasis. If HFD-induced obesity progresses, it leads to changes in WAT metabolic activity to adapt/survive to this newly imposed condition. Interestingly, animals receiving palmitoleic acid treatment seem to require fewer adjustments, softening these changes, at least concerning gene expression and hepatic parameters. Further studies will be required to elucidate these 16:1n7 effects in obesity. Our data suggest that palmitoleic acid treatment reduces the need for adaptation imposed to the organism by the HFD, which is a challenge and, in the long term, prejudicial.
Data Availability Statement
The raw data supporting the conclusions of this article will be made available by the authors, without undue reservation, to any qualified researcher.
Ethics Statement
The animal study was reviewed and approved by Ethics Committee on Animal Use of the Federal University of São Paulo (CEUA 8347020315).
Author Contributions
MC, JS, RS, TF, VS, FA, VA, and LA-C performed the experiments, analyzed the results, and revised the manuscript. MA-V designed the study, analyzed the results, and supervised the study. All authors contributed to the article and approved the submitted version.
Funding
This work was supported by grants received from FAPESP (2018/05485-6) and CAPES.
Conflict of Interest
The authors declare that the research was conducted in the absence of any commercial or financial relationships that could be construed as a potential conflict of interest.
References
1. Hotamisligil GS. Inflammation and metabolic disorders. Nature. (2006) 444:860–7. doi: 10.1038/nature05485
2. Tchoukalova Y, Koutsari C, Jensen M. Committed subcutaneous preadipocytes are reduced in human obesity. Diabetologia. (2007) 50:151–7. doi: 10.1007/s00125-006-0496-9
3. Wang Y, Jones Voy B, Urs S, Kim S, Soltani-Bejnood M, Quigley N, et al. The human fatty acid synthase gene and de novo lipogenesis are coordinately regulated in human adipose tissue. J Nutr. (2004) 134:1032–8. doi: 10.1093/jn/134.5.1032
4. Proença ARG, Sertié RAL, Oliveira AC, Campaña AB, Caminhotto RO, Chimin P, et al. New concepts in white adipose tissue physiology. Braz J Med Biol Res. (2014) 47:192–205. doi: 10.1590/1414-431X20132911
5. Lafontan M, Langin D. Lipolysis and lipid mobilization in human adipose tissue. Prog Lipid Res. (2009) 48:275–97. doi: 10.1016/j.plipres.2009.05.001
6. Reshef L, Olswang Y, Cassuto H, Blum B, Croniger CM, Kalhan SC, et al. Glyceroneogenesis and the triglyceride/fatty acid cycle. J Biol Chem. (2003) 278:30413–6. doi: 10.1074/jbc.R300017200
7. Wang T, Zang Y, Ling W, Corkey BE, Guo W. Metabolic partitioning of endogenous fatty acid in adipocytes. Obes Res. (2003) 11:880–7. doi: 10.1038/oby.2003.121
8. Arner P, Bernard S, Salehpour M, Possnert G, Liebl J, Steier P, et al. Dynamics of human adipose lipid turnover in health and metabolic disease. Nature. (2011) 478:110–3. doi: 10.1038/nature10426
9. Virtue S, Vidal-Puig A. It's not how fat you are, it's what you do with it that counts. PLoS Biol. (2008) 6:1819–23. doi: 10.1371/journal.pbio.0060237
10. Spalding KL, Arner E, Westermark PO, Bernard S, Buchholz BA, Bergmann O, et al. Dynamics of fat cell turnover in humans. Nature. (2008) 453:783–7. doi: 10.1038/nature06902
11. Shao M, Vishvanath L, Busbuso NC, Hepler C, Shan B, Sharma AX, et al. De novo adipocyte differentiation from Pdgfrβ+ preadipocytes protects against pathologic visceral adipose expansion in obesity. Nat Commun. (2018) 9:890. doi: 10.1038/s41467-018-03196-x
12. Havel PJ. Control of energy homeostasis and insulin action by adipocyte hormones: leptin, acylation stimulating protein, and adiponectin. Curr Opin Lipidol. (2002) 13:51–9. doi: 10.1097/00041433-200202000-00008
13. Passos MEP, Alves HHO, Momesso CM, Faria FG, Murata G, Cury-Boaventura MF, et al. Differential effects of palmitoleic acid on human lymphocyte proliferation and function. Lipids Health Dis. (2016) 15:1–11. doi: 10.1186/s12944-016-0385-2
14. Cao H, Gerhold K, Mayers JR, Wiest MM, Watkins SM, Hotamisligil GS. Identification of a lipokine, a lipid hormone linking adipose tissue to systemic metabolism. Cell. (2008) 134:933–44. doi: 10.1016/j.cell.2008.07.048
15. Stefan N, Kantartzis K, Celebi N, Staiger H, Machann J, Schick F, et al. Circulating palmitoleate strongly and independently predicts insulin sensitivity in humans. Diabetes Care. (2010) 33:405–7. doi: 10.2337/dc09-0544
16. Yang Z-H, Miyahara H, Hatanaka A. Chronic administration of palmitoleic acid reduces insulin resistance and hepatic lipid accumulation in KK-Ay mice with genetic type 2 diabetes. Lipids Health Dis. (2011) 10:120. doi: 10.1186/1476-511X-10-120
17. Bolsoni-Lopes A, Festuccia WT, Chimin P, Farias TS, Torres-Leal FL, Cruz MM, et al. Palmitoleic acid (n-7) increases white adipocytes GLUT4 content and glucose uptake in association with AMPK activation. Lipids Health Dis. (2014) 13:199. doi: 10.1186/1476-511X-13-199
18. Bolsoni-Lopes A, Festuccia WT, Farias TSM, Chimin P, Torres-Leal FL, Derogis PBM, et al. Palmitoleic acid (n-7) increases white adipocyte lipolysis and lipase content in a PPARα-dependent manner. Am J Physiol Endocrinol Metab. (2013) 305:E1093–102. doi: 10.1152/ajpendo.00082.2013
19. Cruz MM, Lopes AB, Crisma AR, de Sá RCC, Kuwabara WMT, Curi R, et al. Palmitoleic acid (16:1n7) increases oxygen consumption, fatty acid oxidation and ATP content in white adipocytes. Lipids Health Dis. (2018) 17:55. doi: 10.1186/s12944-018-0710-z
20. Foryst-Ludwig A, Kreissl MC, Benz V, Brix S, Smeir E, Ban Z, et al. Adipose tissue lipolysis promotes exercise-induced cardiac hypertrophy involving the lipokine C16:1n7-palmitoleate. J Biol Chem. (2015) 290:23603–15. doi: 10.1074/jbc.M115.645341
21. Souza CO, Teixeira AAS, Biondo LA, Silveira LS, Calder PC, Rosa Neto JC. Palmitoleic acid reduces the inflammation in LPS-stimulated macrophages by inhibition of NFκB, independently of PPARs. Clin Exp Pharmacol Physiol. (2017) 44:566–75. doi: 10.1111/1440-1681.12736
22. Talbot NA, Wheeler-Jones CP, Cleasby ME. Palmitoleic acid prevents palmitic acid-induced macrophage activation and consequent p38 MAPK-mediated skeletal muscle insulin resistance. Mol Cell Endocrinol. (2014) 393:129–42. doi: 10.1016/j.mce.2014.06.010
23. Souza CO, Teixeira AAS, Lima EA, Batatinha HAP, Gomes LM, Carvalho-Silva M, et al. Palmitoleic acid (n-7) attenuates the immunometabolic disturbances caused by a high-fat diet independently of PPARα. Mediators Inflamm. (2014) 2014:582197. doi: 10.1155/2014/582197
24. Zhou YT, Grayburn P, Karim A, Shimabukuro M, Higa M, Baetens D, et al. Lipotoxic heart disease in obese rats: implications for human obesity. Proc Natl Acad Sci USA. (2000) 97:1784–9. doi: 10.1073/pnas.97.4.1784
25. Sun X, Pan H, Tan H, Yu Y. High free fatty acids level related with cardiac dysfunction in obese rats. Diabetes Res Clin Pract. (2012) 95:251–9. doi: 10.1016/j.diabres.2011.10.028
26. de Farias TDSM, Cruz MM, de Sa RCDC, Severi I, Perugini J, Senzacqua M, et al. Melatonin supplementation decreases hypertrophic obesity and inflammation induced by high-fat diet in mice. Front Endocrinol. (2019) 10:750. doi: 10.3389/fendo.2019.00750
27. de Sá RD, Crisma AR, Cruz MM, Martins AR, Masi LN, do Amaral CL, et al. Fish oil prevents changes induced by a high-fat diet on metabolism and adipokine secretion in mice subcutaneous and visceral adipocytes. J Physiol. (2016) 594:6301–17. doi: 10.1113/JP272541
28. da Cunha de Sá RDC, Cruz MM, de Farias TM, da Silva VS, de Jesus Simão J, Telles MM, et al. Fish oil reverses metabolic syndrome, adipocyte dysfunction, and altered adipokines secretion triggered by high-fat diet-induced obesity. Physiol Rep. (2020) 8:e14380. doi: 10.14814/phy2.14380
29. Chan DC. Mitochondria: dynamic organelles in disease, aging, and development. Cell. (2006) 125:1241–52. doi: 10.1016/j.cell.2006.06.010
30. Masi LN, Martins AR, Crisma AR, do Amaral CL, Davanso MR, Serdan TDA, et al. Combination of a high-fat diet with sweetened condensed milk exacerbates inflammation and insulin resistance induced by each separately in mice. Sci Rep. (2017) 7:3937. doi: 10.1038/s41598-017-04308-1
31. Stoeckman AK, Towle HC. The role of SREBP-1c in nutritional regulation of lipogenic enzyme gene expression. J Biol Chem. (2002) 277:27029–35. doi: 10.1074/jbc.M202638200
32. Uyeda K, Yamashita H, Kawaguchi T. Carbohydrate responsive element-binding protein (ChREBP): a key regulator of glucose metabolism and fat storage. Biochem Pharmacol. (2002) 63:2075–80. doi: 10.1016/S0006-2952(02)01012-2
33. Kersten S. Mechanisms of nutritional and hormonal regulation of lipogenesis. EMBO Rep. (2001) 2:282–6. doi: 10.1093/embo-reports/kve071
34. Delzenne N, Ferré P, Beylot M, Daubioul C, Declercq B, Diraison F, et al. Study of the regulation by nutrients of the expression of genes involved in lipogenesis and obesity in humans and animals. Nutr Metab Cardiovasc Dis. (2001) 11(Suppl. 4):118–21.
35. Delgado TC, Pinheiro D, Caldeira M, Castro MMCA, Geraldes CFGC, López-Larrubia P, et al. Sources of hepatic triglyceride accumulation during high-fat feeding in the healthy rat. NMR Biomed. (2009) 22:310–7. doi: 10.1002/nbm.1327
36. Duarte JAG, Carvalho F, Pearson M, Horton JD, Browning JD, Jones JG, et al. A high-fat diet suppresses de novo lipogenesis and desaturation but not elongation and triglyceride synthesis in mice. J Lipid Res. (2014) 55:2541–53. doi: 10.1194/jlr.M052308
37. Gallardo-Montejano VI, Saxena G, Kusminski CM, Yang C, McAfee JL, Hahner L, et al. Nuclear perilipin 5 integrates lipid droplet lipolysis with PGC-1α/SIRT1-dependent transcriptional regulation of mitochondrial function. Nat Commun. (2016) 7:12723. doi: 10.1038/ncomms12723
38. Brasaemle DL, Rubin B, Harten IA, Gruia-Gray J, Kimmel AR, Londos C. Perilipin A increases triacylglycerol storage by decreasing the rate of triacylglycerol hydrolysis. J Biol Chem. (2000) 275:38486–93. doi: 10.1074/jbc.M007322200
39. Ventura-Clapier R, Garnier A, Veksler V. Transcriptional control of mitochondrial biogenesis: the central role of PGC-1alpha. Cardiovasc Res. (2008) 79:208–17. doi: 10.1093/cvr/cvn098
40. Bi P, Shan T, Liu W, Yue F, Yang X, Liang XR, et al. Inhibition of notch signaling promotes browning of white adipose tissue and ameliorates obesity. Nat Med. (2014) 20:911–8. doi: 10.1038/nm.3615
41. Silveira MAD, Marcondes JPC, Lara JR, Scarano WR, Calderón IMP, Rudge MVC, et al. Mitochondrial-related gene associated to obesity can be modulated by in utero hyperglycemic environment. Reprod Toxicol. (2019) 85:59–64. doi: 10.1016/j.reprotox.2019.02.001
42. Warfel JD, Vandanmagsar B, Dubuisson OS, Hodgeson SM, Elks CM, Ravussin E, et al. Examination of carnitine palmitoyltransferase 1 abundance in white adipose tissue: implications in obesity research. Am J Physiol Regul Integr Comp Physiol. (2017) 312:R816–20. doi: 10.1152/ajpregu.00520.2016
43. Valerio A, Cardile A, Cozzi V, Bracale R, Tedesco L, Pisconti A, et al. TNF-alpha downregulates eNOS expression and mitochondrial biogenesis in fat and muscle of obese rodents. J Clin Invest. (2006) 116:2791–8. doi: 10.1172/JCI28570
44. Seale P, Conroe HM, Estall J, Kajimura S, Frontini A, Ishibashi J, et al. Prdm16 determines the thermogenic program of subcutaneous white adipose tissue in mice. J Clin Invest. (2011) 121:96–105. doi: 10.1172/JCI44271
45. Bournat JC, Brown CW. Mitochondrial dysfunction in obesity. Curr Opin Endocrinol Diabetes Obes. (2010) 17:446–52. doi: 10.1097/MED.0b013e32833c3026
46. Ducluzeau PH, Priou M, Weitheimer M, Flamment M, Duluc L, Iacobazi F, et al. Dynamic regulation of mitochondrial network and oxidative functions during 3T3-L1 fat cell differentiation. J Physiol Biochem. (2011) 67:285–96. doi: 10.1007/s13105-011-0074-6
47. Gao CL, Zhu C, Zhao YP, Chen XH, Ji CB, Zhang CM, et al. Mitochondrial dysfunction is induced by high levels of glucose and free fatty acids in 3T3-L1 adipocytes. Mol Cell Endocrinol. (2010) 320:25–33. doi: 10.1016/j.mce.2010.01.039
48. Chan KL, Pillon NJ, Sivaloganathan DM, Costford SR, Liu Z, Théret M, et al. Palmitoleate reverses high fat-induced proinflammatory macrophage polarization via AMP-activated protein kinase (AMPK). J Biol Chem. (2015) 290:16979–88. doi: 10.1074/jbc.M115.646992
49. Wernstedt Asterholm I, Tao C, Morley TS, Wang QA, Delgado-Lopez F, Wang ZV, et al. Adipocyte inflammation is essential for healthy adipose tissue expansion and remodeling. Cell Metab. (2014) 20:103–18. doi: 10.1016/j.cmet.2014.05.005
50. Santos-Alvarez J, Goberna R, Sánchez-Margalet V. Human leptin stimulates proliferation and activation of human circulating monocytes. Cell Immunol. (1999) 194:6–11. doi: 10.1006/cimm.1999.1490
51. Grunfeld C, Zhao C, Fuller J, Pollack A, Moser A, Friedman J, et al. Endotoxin and cytokines induce expression of leptin, the ob gene product, in hamsters. J Clin Invest. (1996) 97:2152–7. doi: 10.1172/JCI118653
52. Kirchgessner TG, Uysal KT, Wiesbrock SM, Marino MW, Hotamisligil GS. Tumor necrosis factor-α contributes to obesity-related hyperleptinemia by regulating leptin release from adipocytes. J Clin Invest. (1997) 100:2777–82. doi: 10.1172/JCI119824
53. Bastard JP, Maachi M, Lagathu C, Kim MJ, Caron M, Vidal H, et al. Recent advances in the relationship between obesity, inflammation, and insulin resistance. Eur Cytokine Netw. (2006) 17:4–12.
54. Wronska A, Kmiec Z. Structural and biochemical characteristics of various white adipose tissue depots. Acta Physiol. (2012) 205:194–208. doi: 10.1111/j.1748-1716.2012.02409.x
55. Farhangi MA, Mesgari-Abbasi M, Hajiluian G, Nameni G, Shahabi P. Adipose tissue inflammation and oxidative stress: the ameliorative effects of vitamin D. Inflammation. (2017) 40:1688–97. doi: 10.1007/s10753-017-0610-9
56. Liu W, Baker S, Baker R, Zhu L. Antioxidant mechanisms in nonalcoholic fatty liver disease. Curr Drug Targets. (2015) 16:1301–14. doi: 10.2174/1389450116666150427155342
Keywords: high-fat diet, n-7 fatty acid, adipocytes metabolism, hepatic triglycerides, WAT gene expression
Citation: Cruz MM, Simão JJ, Sá RDCCd, Farias TSM, Silva VSd, Abdala F, Antraco VJ, Armelin-Correa L and Alonso-Vale MIC (2020) Palmitoleic Acid Decreases Non-alcoholic Hepatic Steatosis and Increases Lipogenesis and Fatty Acid Oxidation in Adipose Tissue From Obese Mice. Front. Endocrinol. 11:537061. doi: 10.3389/fendo.2020.537061
Received: 22 February 2020; Accepted: 28 August 2020;
Published: 30 September 2020.
Edited by:
Nigel Turner, University of New South Wales, AustraliaReviewed by:
Alessandra Feraco, San Raffaele Pisana (IRCCS), ItalyMagdalene K. Montgomery, The University of Melbourne, Australia
Copyright © 2020 Cruz, Simão, Sá, Farias, Silva, Abdala, Antraco, Armelin-Correa and Alonso-Vale. This is an open-access article distributed under the terms of the Creative Commons Attribution License (CC BY). The use, distribution or reproduction in other forums is permitted, provided the original author(s) and the copyright owner(s) are credited and that the original publication in this journal is cited, in accordance with accepted academic practice. No use, distribution or reproduction is permitted which does not comply with these terms.
*Correspondence: Maria Isabel C. Alonso-Vale, YWxvbnNvdmFsZUBnbWFpbC5jb20=
†These authors have contributed equally to this work