- 1Shenzhen Key Laboratory for Systemic Aging and Intervention, National Engineering Research Center for Biotechnology (Shenzhen), Medical Research Center, Shenzhen University Health Science Center, Shenzhen, China
- 2Key Laboratory of Optoelectronic Devices and Systems of Ministry of Education and Guangdong Province, College of Optoelectronic Engineering, Shenzhen University, Shenzhen, China
- 3Guangdong Key Laboratory of Genome Stability and Human Disease Prevention, Department of Biochemistry & Molecular Biology, School of Basic Medical Sciences, Shenzhen University, Shenzhen, China
Diabetic mellitus (DM) is a significant public health concern worldwide with an increased incidence of morbidity and mortality, which is particularly due to the diabetic vascular complications. Several pivotal underlying mechanisms are associated with vascular complications, including hyperglycemia, mitochondrial dysfunction, inflammation, and most importantly, oxidative stress. Oxidative stress triggers defective angiogenesis, activates pro-inflammatory pathways and causes long-lasting epigenetic changes to facilitate the development of vascular complications. Therefore, therapeutic interventions targeting oxidative stress are promising to manage diabetic vascular complications. Sirtuin1 (SIRT1), a class III histone deacetylase belonging to the sirtuin family, plays critical roles in regulating metabolism and ageing-related pathological conditions, such as vascular diseases. Growing evidence has indicated that SIRT1 acts as a sensing regulator in response to oxidative stress and attenuates vascular dysfunction via cooperating with adenosine-monophosphate-activated protein kinase (AMPK) to activate antioxidant signals through various downstream effectors, including peroxisome proliferator-activated receptor-gamma co-activator 1 (PGC-1α), forkhead transcription factors (FOXOs), and peroxisome proliferative-activated receptor α (PPARα). In addition, SIRT1 interacts with hydrogen sulfide (H2S), regulates NADPH oxidase, endothelial NO synthase, and mechanistic target of rapamycin (mTOR) to suppress oxidative stress. Furthermore, mRNA expression of sirt1 is affected by microRNAs in DM. In the current review, we summarize recent advances illustrating the importance of SIRT1 in antagonizing oxidative stress. We also discuss whether modulation of SIRT1 can serve as a therapeutic strategy to treat diabetic vascular complications.
Introduction
Diabetes mellitus (DM) is a complicated age-related diseases worldwide (1) and the main characteristics of DM are hyperglycemia and insulin resistance caused by β-cell dysfunction, resulting in increased risk for vascular disease (2–4). Notably, DM patients always accompany with various diseases associated with diabetic vascular complications, such as acquired blindness, atherosclerosis, end-stage renal failure, and neuropathies. Generally, diabetic vascular complications are classified into microvascular and macrovascular complications, the latter of which occur more frequently in patients with diabetes. The central pathological symptoms in macrovascular disease include hypertension, atherosclerosis, diabetic heart diseases, peripheral arterial disease, and stroke. Actually, diabetic patients have two times higher risk of developing stroke and hypertension than those who are healthy adults (5). On the contrary, microvascular complications involve diabetic nephropathy, neurophathy, and retinopathy (6–9). Considering the increased prevalence and the emergent requirement of therapeutic interventions to prevent these complications, it is important to explore the underlying connections between vascular disease and diabetes (10).
Advances in understanding of diabetes have made it clear that abnormal angiogenesis and endothelial dysfunction are two well-documented pathological characteristics of diabetic vascular complications (11–13). In particular, endothelial dysfunction plays central roles in the development of vascular complications, which is characterized by diminished bioavailability of nitric oxide, increased endothelium-derived contracting factors, and the impaired vasodilation. In addition, endothelial dysfunction is accompanied by the accumulation of cytokines and chemokines within the vascular microenvironment (14, 15). Therefore, elucidating the etiology and mechanisms of endothelial dysfunction will facilitate the development of diagnosis, prevention, and treatment even during suboptimal metabolic control of DM.
Oxidative Stress in Diabetic Vascular Complications
Oxidative stress is manifested as an overload of free radical accumulations, such as reactive oxygen species (ROS), and decreased antioxidants in the microvasculature, which always result in endothelial dysfunction. Importantly, amount of works have demonstrated that oxidative stress is one of the greatest contributors to the pathogenesis of vascular disorders and DM (16–18). For one hand, oxidative stress alters the endothelial signaling transduction and regulates the activation of transcriptional factors in response to redox, and thereby enhances vascular endothelial permeability and leukocyte adhesion. For another hand, oxidative stress contributes to insulin resistance, β-cell dysfunction, and hyperglycemia-mediated cellular injury, leading to the development of DM (16–18).
Specifically, metabolic abnormalities of DM, especially hyperglycemia and hyperlipidemia, can activate NADPH oxidases (NOXs) and endothelial Nitric Oxide Synthase (eNOS), increase advanced glycation end-products (AGE), and interrupt the polyol pathway as well as the mitochondrial respiratory chain, to impinge on oxidative stress (19). Consequently, oxidative stress may impair β-cell function via reducing insulin synthesis, hindering proinsulin inclusion, and inducing the apoptotic cell death of pancreatic cells (20). Mitochondrial collapse induced by oxidative stress is another important contributor to insulin resistance. Many high glucose levels associated biochemical pathways, such as glucose autoxidation, prostanoid synthesis, and protein glycation, crumble mitochondrial and promote mitochondria-mediated overproduction of ROS (predominantly superoxide anion) (21). As a result, excess ROS triggers several cellular mechanisms, including polyol pathway, mitogen-activated protein kinase (MAPK), adenosine-monophosphate-activated protein kinase (AMPK), NF-κB signaling pathways, and transcription factors such as forkhead transcription factors (FOXO), Nrf2 and AP-1, to initiate inflammation and deregulate insulin signaling pathways (22, 23). Taken together, therapeutic interventions aiming to reduce oxidative stress, such as anti-oxidative agents, are promising strategies reversing insulin resistance, ameliorating endothelial function and preventing cardiovascular morbidity in patients with diabetes.
Structure and Regulation of Sirt1
The mammalian sirtuins are nicotinamide adenine dinucleotide (NAD+)-dependent histone deacetylases, which regulate numerous cellular processes involved in DNA repair, cell survival and senescence, stress-stimulated metabolism alternations (24, 25). They require NAD+ as a cofactor for deacetylation of histone or non-histone substrates and are generally not inhibited by compounds that inhibit zinc-dependent deacetylases. Importantly, increasing reports have demonstrated that the sirtuin family participates in the development of vascular physiology and pathology (26, 27), suggesting the sirtuins as therapeutic targets in DM.
In mammals, the sirtuin family is comprised of seven members (SIRT1-SIRT7). Among these mammalian Sirts, SIRT1 has been investigated most extensively (28). SIRT1 has 747 amino acids and three independent domains, the central deacetylase domain conservative in species, the N-terminal region containing nuclear localization/export signals, and the C-terminal region containing the essential for SIRT1 activity (ESA) domain (Figure 1). Structurally, a substrate and a NAD+ binding pocket exists in the catalytic domain, whereas the regulatory element and binding domain of SIRT1 co-activator/co-repressor distribute in the N- and C-terminus. SIRT1 removes the acetyl groups from multiple cytoplasmic substrates rather than histones, such as peroxisome proliferator-activated receptor-gamma co-activator 1 (PGC-1α), farnesoid X receptor (FXR), liver X receptor (LXR), sterol-regulatory element binding protein 1 (SREBP1), peroxisome proliferative-activated receptor (PPAR) to exert various functions in cellular metabolism (gluconeogenesis, insulin sensitivity, fat mobilization, and lipid metabolism) (29, 30). In addition, SIRT1 also regulate mechanistic target of rapamycin (mTOR), p53, KU70, E2F, and FOXO to affect cell survival and senescence (Figure 1).
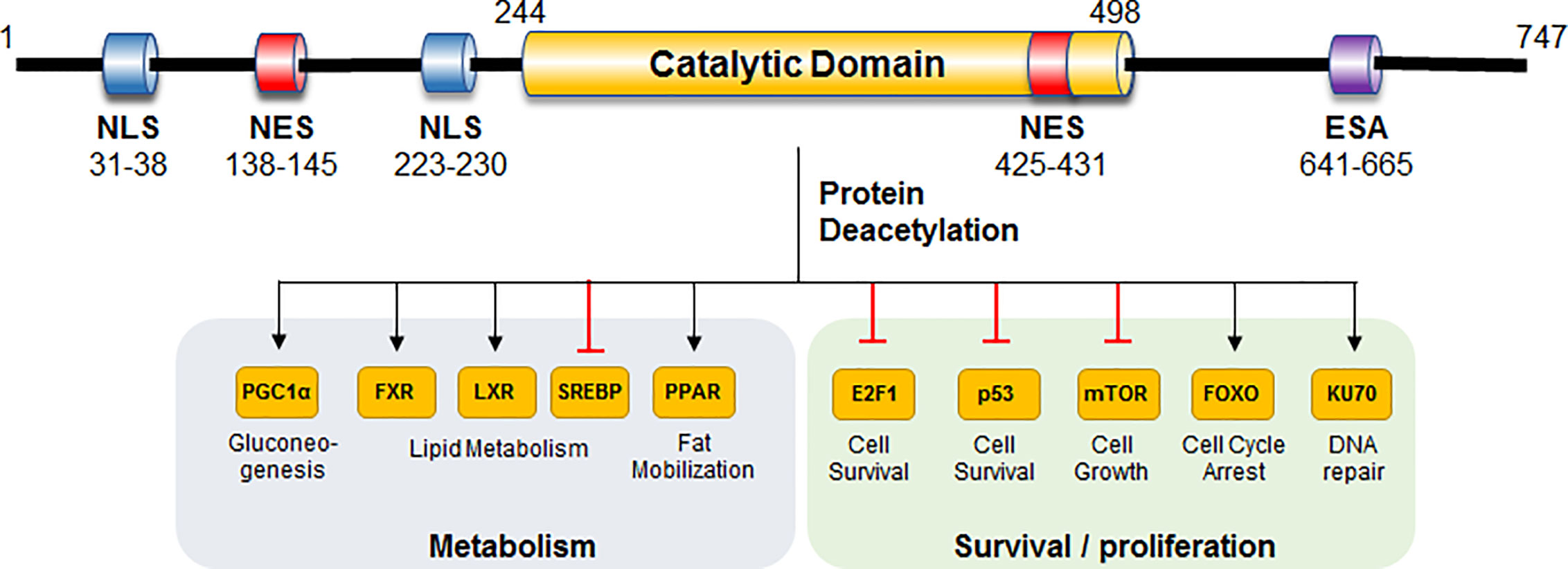
Figure 1 Structure and function of human SIRT1. Important domains and substrates of human SIRT1 are shown, and their corresponding functions are presented. NLS, nuclear localization signal; NES, nuclear export signal; ESA, essential for Sirt1 activity; FXR, farnesoid X receptor; LXR, liver X receptor; SREBP1, sterol-regulatory element binding protein 1.
Multiple studies on the regulation of SIRT1 activity have shown that post-translational modifications (PTMs), such as phosphorylation, S-nitrosylation, and SUMOylation, modulate SIRT1 protein level and its deacetylase activity (31). For instance, SIRT1 contains several sites for phosphorylation (e.g., N-terminus with seven residues and C-terminus with eight residues). In most cases, phosphorylation of SIRT1 mediated by different kinases, such as C-Jun N-terminal kinase 1, casein kinase 2, and cyclin-dependent kinase 1, enhances SIRT1 deacetylase activity and regulates its function in a substrate-dependent manner (31). In addition, SUMO is an ubiquitin like-modifier that exerts opposite effect of ubiquitin and covalently addition of SUMO protein to lysine residues (the so-called SUMOylation) can stabilize proteins. SUMOylation of SIRT1 at Lys 734 also increase its deacetylase activity and protein stability. Conversely, deSUMOylation of SIRT1 by specific deSUMOylating enzyme, sentrin-specific protease 1 (SENP1), reduces its deacetylase activity (32). Unlike SUMOylation, the covalently incorporating a nitric oxide moiety into Cys 387 and 390 of SIRT1 (S-nitrosylation) significantly reduces the deacetylase activity of SIRT1 toward to a widely reported substrate PGC-1α (33). Furthermore, SIRT1 activity is also regulated by protein-protein interaction. For instance, and deleted in breast cancer 1 (DBC1) and active regulator of SIRT1 (AROS) are negative and positive regulator of SIRT1, respectively. AROS binds to the N-terminus of SIRT1 to increase the SIRT1 activity (34), whereas DBC1 binds to the “essential for SIRT1 activity” (ESA) domain or the SIRT1 deacetylase core domain to inactivate SIRT1 (35). Finally, there are several small chemicals able to modify the activity of SIRT1, such as polyphenol resveratrol, sirtinol, and splitomicin (36). Resveratrol is a stilbenoid and is well-known for its ability to activating SIRT1 to exert its anti-aging, anti-diabetic, and anti-cardiovascular functions. Further works are inspired to discover more SIRT1 PTMs and protein regulators and to explore the possible crosstalk among different SIRT1 PTMs.
SIRT1 Antagonizes Oxidative Stress in Diabetic Mellitus
Recently published studies have clearly revealed that SIRT1 antagonizes oxidative stress in the pathogenesis of diabetic vasculopathy (37–39). For instance, the downregulation of SIRT1 by hyperglycemia caused vascular dysfunction in DM (40). On the contrary, upregulation of SIRT1 attenuated oxidative stress-induced endothelial senescence in diabetic mice (41). Notably, SIRT1 attenuates oxidative stress to regulate diabetic vascular complications through several important signal mediators, such as AMPK, NADPH oxidase, endothelial NO synthase, mTOR, and miRNAs (Figure 2). It seems that SIRT1 relies on the availability of different substrates to regulate cellular oxidative stress.
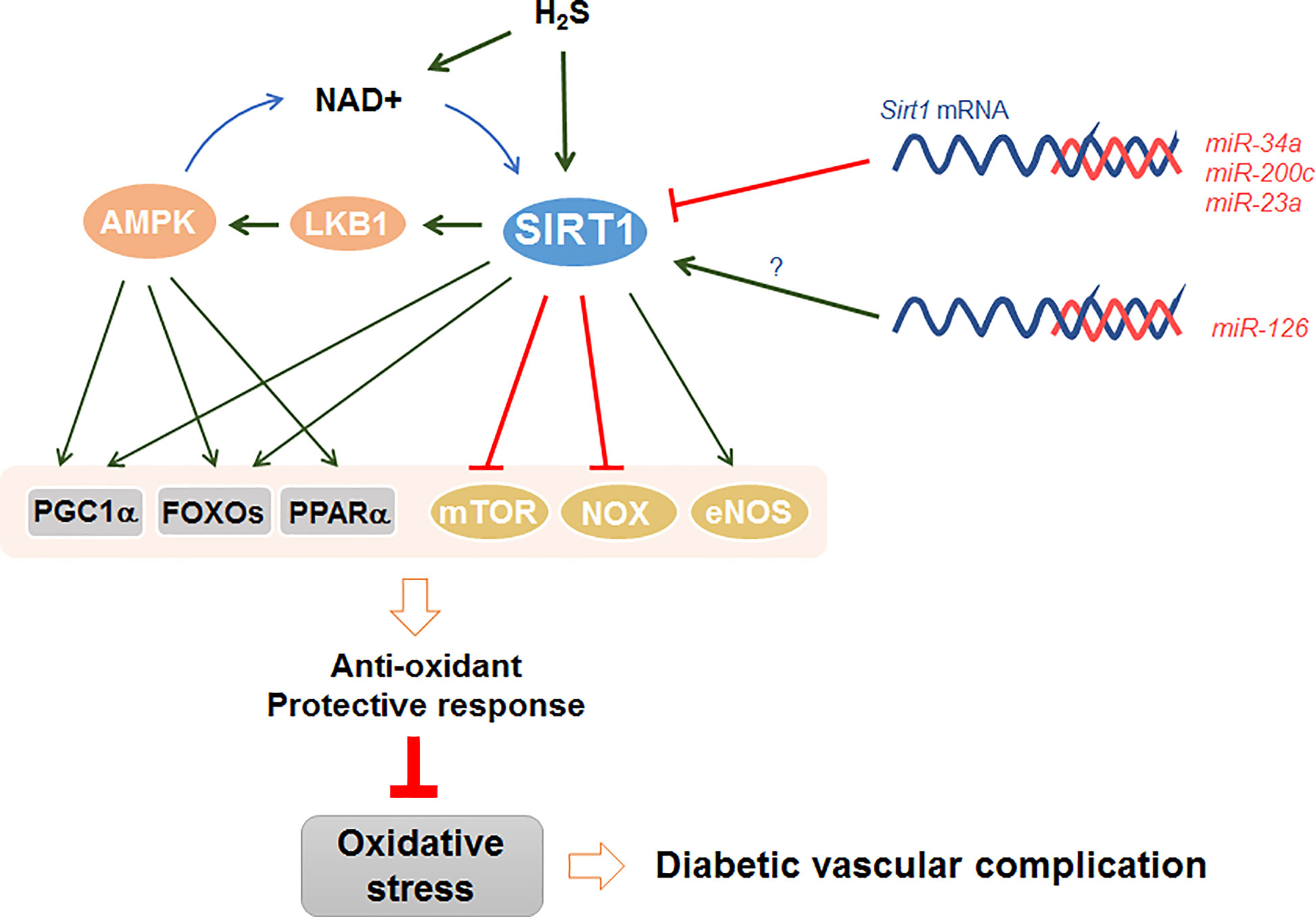
Figure 2 SIRT1 antagonizes oxidative stress in diabetic vascular complications. SIRT1 directly or cooperatively with AMPK to activate various downstream effectors, including PGC1α, FOXOs, and PPARα. SIRT1 also stimulate eNOS and inhibit NOX and mTOR to trigger anti-oxidant protective response. In addition, H2S can either activate SIRT1 directly via sulfydration, or indirectly via increasing NAD+ level. Furthermore, several miRNAs, such as miR-34a, miR-200c, and miR-23a, directly inhibit the mRNA expression of sirt1 in DM, while other miRNAs including miR-126 promote sirt1 expression indirectly with unclear mechanism.
SIRT1 Cooperates With AMPK to Antagonize Oxidative Stress in Diabetic Mellitus
AMPK is the main cellular mediator of metabolic stress and is activated by glucose deprivation (42). Importantly, AMPK and SIRT1 both regulate each other and have similar effects on various cellular processes such as cell metabolism, DNA repair, mitochondrial function, and cell growth (43). For instance, AMPK manipulates energy expenditure by increasing the intercellular NAD+ concentration to enhance SIRT1 activity (44). Alternatively, SIRT1 deacetylates and activates LKB1, the upstream kinase of AMPK, to activate AMPK and to suppress ROS production (45, 46). Furthermore, SIRT1 and AMPK share many common targets, such as forkhead transcription factors (FOXOs), peroxisome proliferative-activated receptor α (PPARα), and PGC-1α (43). The joint activation of AMPK and SIRT1 can individually or combinatory activates these downstream effectors to exert antioxidant protective response and to ameliorate oxidative stress in DM (47, 48).
Inhibition of Oxidative Stress by SIRT1-AMPK-PGC-1α
PGC-1α is recognized as a key metabolic sensor and regulator in response to malnutrition and hypoxia (49, 50). Upon activation, PGC-1α regulates mitochondrial biogenesis and thus ROS production by controlling many transcription factors to initiate the expression of mitochondrial genes, resulting in reduced oxidative stress (51). PGC-1α is activated either by AMPK-mediated phosphorylation or SIRT1-mediated deacetylation in an NAD+-dependent manner (43, 52). Notably, amount of evidence has proved that SIRT1 cooperates with AMPK to enhance the ability of PGC-1α to attenuate endothelial dysfunction via stimulating mitochondrial biogenesis (53) and activating gluconeogenic fatty acid oxidation genes (54, 55). In addition, SIRT1-AMPK-PGC-1α pathway exerts its anti-oxidative activity in other diabetic vascular complications including brain complications (56), diabetic cardiomyopathy, and diabetic nephropathy (57, 58). Furthermore, PGC-1α can be directly activated by SIRT1 without AMPK to ease metabolic disorders in high glucose-induced endothelial oxidative damage (59) and Drp-mediated mitochondrial fission in diabetic hearts (60). Therefore, whether SIRT1 regulates the PGC-1α-mediated mitochondrial respiration and ROS production, with or without the help of AMPK activation, rests on a context-dependent way.
Inhibition of Oxidative Stress by SIRT1-AMPK-FOXOs
The significance of FOXOs in regulating the metabolic activity of vascular endothelium has been gradually addressed (61). There are four FOXO members in mammals, FOXO1, FOXO3, FOXO4, and FOXO6. SIRT1 deacetylates FOXO1, FOXO3 and FOXO4, and stimulates FOXO-dependent expression of antioxidants to scavenge ROS, such as catalase, manganese superoxide dismutase (MnSOD), and thioredoxin, either positively or negatively (62, 63). For instance, SIRT1 deacetylated FOXO1 to combat oxidative stress in diabetic vascular complications, diabetic nephropathy, and particularly in diabetic cardiomyopathy under the treatment of resveratrol (64–66). Besides, SIRT1 directly deacetylated FOXO3a and promoted the expression of MnSOD and catalase that are involved in stress resistance, which in turn attenuated oxidative stress in diabetic cardiomyopathy and nephropathy (67, 68). Moreover, SIRT1 bound to FOXO4 and increased its transactivation capacity to inhibit NF-κB signaling and inflammation (63). Interestingly, AMPK has also been shown to phosphorylate and activate FOXOs, yet the precise mechanism of AMPK in SIRT1-FOXO pathway remains unclear (44, 64). It seems that AMPK can regulate the function of FOXOs either by direct phosphorylation or indirectly via the SIRT1-induced activation.
Inhibition of Oxidative Stress by SIRT1-AMPK-PPARα
As a transcriptional factor, PPARα is mainly expressed in many oxidative tissues that require high capacity of fatty acid oxidation and is essential for the regulation of glucose metabolism (69). Additionally, PPARα is able to attenuate oxidative stress and inflammation through repressing NF-κB-mediated gene transcription, as well as increasing NO expression and release (70–72). It has been proved that expression of PPARα was reduced by hyperglycemia, and SIRT1 activated PPARα pathway to reduce ROS in diabetic vascular diseases (73, 74). In addition, AMPK-SIRT1-PPARα was activated by resveratrol to alleviate oxidative stress and endothelial dysfunction in type 2 diabetic nephropathy (58). However, unlike FOXOs, PPARα is not a directly target deacetylated by SIRT1, but its activity can be enhanced by SIRT1 indirectly through the coactivators, such as AMPK and PGC-1α (75, 76). Moreover, various PPAR agonists have been proved to prevent diabetes in the non-obese diabetic mouse model, suggesting the therapeutic function of SIRT1-PPARα axis in DM.
SIRT1 Regulates eNOS and NOX to Suppress Oxidative Stress
Nicotinamide adenine dinucleotide phosphate (NADPH) oxidase (NOX) family protein are primary sources of regulated production of ROS and play critical functions in the pathogenesis of the macro and micro-vascular complications of diabetes (77). Several important mediators of DM, such as hyperglycemia, oxidized or glycated low-density lipoproteins (LDL) and AGEs, can activate NOX to generate ROS, resulting in cell and tissue injury characteristic of diabetic complications (19). Importantly, the closely connection between NOX and SIRT1 has been gradually recognized, which shows that SIRT1 inhibits NOX to diminish ROS. For instance, resveratrol-mediated activation of SIRT1 inhibited the expression of NOX4 and the NADPH oxidative activity to reduce vascular superoxide production and to ameliorate endothelial dysfunction (78). Besides, dulaglutide, a glucagon-like peptide-1 receptor agonist used to treat type 2 DM, activated SIRT1 to suppress high glucose-induced activation of NLRP3 inflammasome and to downregulate NOX4 expression and the generation of ROS in human umbilical vein endothelial cells (79). In addition, SIRT1 was activated by quercetin to antagonize oxidized LDL-induced endothelial oxidative damage through activating AMPK and inhibiting NOX2 and NOX4 activity (80). Although the detailed mechanisms about how SIRT1 inhibits NOX directly or indirectly though AMPK have not been eliminated, SIRT1 has an antergic action on NOX-mediated ROS production.
Endothelial NO synthase (eNOS) is the primary enzyme producing NO, which participate in mitochondrial biogenesis and has various anti-atherosclerotic functions. Patients with DM demonstrate uncoupled eNOS in blood vessels, resulting in excessive superoxide anion (O2−) production and diminished NO availability (81). Up to now, there are extensive connections between SIRT1 and eNOS in counteracting oxidative stress. For instance, in response to calorie restriction, SIRT1 promoted eNOS activity and NO bioavailability via the deacetylation of eNOS at lysine 496 and 506 (82, 83). In addition to deacetylation, SIRT1 also positively regulated the phosphorylation of eNOS to affect its ability in reducing oxidative stress and premature senescence (84). Except for SIRT1, AMPK has also been demonstrated to promote NO secretion by increasing eNOS phosphorylation as serine 633, which was another effective way for SIRT1 to overcome caloric restriction and facilitate vascular reconditioning after ischemia via activating the AMPK/eNOS axis (85, 86). In addition, SIRT1 enhances eNOS expression as evidenced by that resveratrol induced SIRT1-dependent upregulation of eNOS in endothelial cells, and endothelial-specific overexpression of Sirt1 led to elevation of eNOS expression (87–89). Furthermore, under resveratrol-stimulated conditions, knockdown of FOXO1 or FOXO3a by siRNAs led to a downregulation of eNOS transcription, implying that SIRT1 activates FOXOs to elevate eNOS (90). Taken together, SIRT1 either enhances eNOS expression or eNOS enzymatic activity to protect against oxidative stress and endothelial dysfunction.
H2S and SIRT1 Interaction Suppress Oxidative Stress
Hydrogen sulfide (H2S) is a gasotransmitter playing an important role in physiological conditions, dysregulation of which always occurs in vascular-related diseases. Amount of evidence has clearly demonstrated that interaction between H2S and SIRT1 is critical modulator of oxidative stress (91, 92). For one hand, H2S limits ROS production via directly enhancing SIRT1 activity or expression (93–96). H2S promotes the S-sulfydration of two CXXC motifs in the catalytic domain of SIRT1, resulting in elevated protein stability and deacetylase activity (96). H2S is also able to activate SIRT1 indirectly by increasing intracellular NAD+ levels (97). Furthermore, H2S exerts its anti-inflammatory and anti-oxidant effects against diabetic complications via stimulating AMPK (92). One possible mechanism is that H2S mediates the S-sulfydration of calcium/calmodulin-dependent protein kinase kinase β (CaMKKβ), one important upstream regulator of AMPK, to phosphorylate AMPK and to activate its downstream effector, the SIRT1/PGC-1α axis (98, 99). Taken together, H2S and SIRT1 interaction represents a protective mechanism against oxidative stress in DM.
SIRT1 Regulates mTOR Pathway to Repress Oxidative Stress
The mechanistic target of rapamycin (mTOR) is a nutrient sensor and initiator of cell growth, forming structurally and functionally distinct mTOR complex 1 (mTORC1) and complex 2 (mTORC2) (100). Importantly, mTOR is an important regulator of oxidative stress through regulating mitochondrial biogenesis and enhancing oxidative metabolism via PGC-1α pathway (101). Besides, insulin-mediated PI3K/Akt/mTOR signaling axis is one of the most well-known mechanisms in regulating cell cycle, glucose metabolism and endothelial dysfunction in DM (102, 103). Interestingly, under calorie restriction, mTOR was inhibited whereas SIRT1 activity was upregulated (104, 105), suggesting the feedback action between mTOR and SIRT1. Indeed, numerous studies have revealed their possible reciprocal connections. For instance, hepatic SIRT1 deficiency disrupted mTORC2/Akt signaling to cause hyperglycemia and oxidative stress in mice model (106). One possible mechanism is that SIRT1 directly regulated mTOR via erythropoietin to protect vascular cells in DM (107, 108). Another example is that SIRT1 activated the mTORC2 signaling via activating a cascade of Akt and FOXO phosphorylation, which in turn ameliorated myocardial ischemia or reperfusion injury in DM (103). Together, all the observations imply that SIRT1-mTOR pathway could be potentially therapeutic targets for diabetic vascular complications.
Regulation of Sirt1 by MicroRNAs in Diabetic Mellitus
MicroRNAs (miRNAs) are cluster of non-coding RNAs that trigger mRNA degradation and repress translation function mainly by targeting messenger RNAs (mRNAs) (109). Recently, the importance of miRNAs-mediated sirt1 regulation in diabetic vascular complications has gradually emerged (110). One extensively investigated miRNA was miR-34a (111, 112). Hyperglycemia-mediated oxidative stress recruited p66SHc, which then upregulated miR-34a to impair angiogenesis in endothelial cells via directly suppressing the stability of sirt1 mRNA, leading to the reduced protein level of SIRT1 (112–114). Besides, regulation of miR-34a, SIRT1, and eNOS synthesis promoted kallistatin to inhibit antioxidant gene expression in endothelial progenitor cells (115). In addition to miR-34a, miR-200c and miR-23a were also related to oxidative stress-induced diabetic vascular complications. Hyperglycemia triggered ROS production and upregulated miR-200c expression, which subsequently inhibited the mRNA expression of sirt1 to facilitate endothelial dysfunction in DM (116, 117). However, unlike miR-34a and miR-200c, increased level of miR-126 could facilitate the expressions of sirt1 and sod-2 to enhance oxidative stress and aggravate diabetic vascular complications, with unclear mechanism (118). Aside from miRNAs discussed above, many other miRNAs, such as miR-195, miR-9, and miR-132, have also been reported to target the mRNA of sirt1 (119–121). Whether they play a role in DM remains to be determined.
SIRT1 as a Therapeutic Target for Diabetic Mellitus
As discussed above, SIRT1 antagonises oxidative stress via different substrates in DM, implying activating SIRT1 as potent therapeutic strategy. Importantly, SIRT1 can be activated directly or indirectly by some naturally polyphenols in vitro and in vivo, such as resveratrol, quercetin, and catechins (122, 123). For instance, resveratrol is one of the well-studied SIRT1 activators that binds to SIRT1 to promote its substrate binding activity. Resveratrol exhibits various biological functions via activating SIRT1 and numerous clinical trials have been performed to test its protective effect against several diseases, such as neurodegeneration, type 2 diabetes, and insulin resistance (123). In addition, some completed phase 1/2 clinical trials have clearly showed that resveratrol is able to improve metabolic and vascular health of diabetes subjects (123). Notably, those natural SIRT1-activating compounds are mostly used as nutraceuticals in management of diabetic vascular complications. Whether their therapeutic effects rely on SIRT1 activation in DM remains to be examined. Considering that most natural compounds possess pleiotropic effects, more selective and specific SIRT1 activators are required, which may facilitate to establish a direct link between SIRT1 activation and human diseases. There are several other SIRT1 activators, such as SRT1720 (an analogue of resveratrol), that have been shown to enhance SIRT1 activity in mammals (36, 124). Whether these activators can exhibit higher specificity and activity than naturally polyphenols, as well as their potentially clinical application, remain to be determined.
Conclusion
SIRT1 plays a key role in many signaling pathways and regulates various important biological phenomena, especially in metabolism, inflammation, aging, and stress resistance through deacetylating transcription factors and histones. The involvement of SIRT1 in diabetic vascular complications has increased, and the diverse mechanisms of SIRT1 against oxidative stress gradually emerges, suggesting that modulation of SIRT1 activity may be an available therapeutic intervention against diabetes-induced vascular diseases (125). However, many detailed molecular interactions remain unsolved. For instance, a comprehensive understanding of how SIRT1 and AMPK are intertwined in mediating vascular diseases is still needed. It is difficult to parse how specific interactions between SIRT1 and AMPK contribute to cross-regulation in each vascular complication. Besides, except for SIRT1, there are still other members of the sirtuin family (e.g., SIRT3, SIRT6, and SIRT7) that are active in modulating vascular function (126, 127), which inspires further works to investigate their associations with oxidative stress and diabetic vascular complications. Finally, substantial efforts aiming to develop novel specifically pharmacological SIRT1 activators under active clinical investigation is needed.
Author Contributions
TM and WQ synthesized and generally organized the manuscript, Figures 1 and 2. BL supervised and edited the manuscript. All authors contributed to the article and approved the submitted version.
Funding
This study was supported by grants from the National Natural Science Foundation of China (91849208, 81972602, 81702909, and 81871114), the National Key R&D Program of China (2017YFA0503900), the Science and Technology Program of Guangdong Province (2017B030301016 and 2019B030301009), and the Shenzhen Municipal Commission of Science and Technology Innovation (ZDSYS20190902093401689, KQJSCX20180328093403969, and JCYJ20180507182044945).
Conflict of Interest
The authors declare that the research was conducted in the absence of any commercial or financial relationships that could be construed as a potential conflict of interest.
References
1. Disease GBD, Injury I, Prevalence C. Global, regional, and national incidence, prevalence, and years lived with disability for 328 diseases and injuries for 195 countries, 1990-2016: a systematic analysis for the Global Burden of Disease Study 2016. Lancet (2017) 390:1211–59. doi: 10.1016/S0140-6736(17)32154-2
2. van den Born JC, Hammes HP, Greffrath W, van Goor H, Hillebrands JL. Complications DGIRTGDM. Gasotransmitters in Vascular Complications of Diabetes. Diabetes (2016) 65:331–45. doi: 10.2337/db15-1003
3. Koulis C, Watson AM, Gray SP, Jandeleit-Dahm KA. Linking RAGE and Nox in diabetic micro- and macrovascular complications. Diabetes Metab (2015) 41:272–81. doi: 10.1016/j.diabet.2015.01.006
4. Gregg EW, Williams DE, Geiss L. Changes in diabetes-related complications in the United States. N Engl J Med (2014) 371:286–7. doi: 10.1056/NEJMc1406009
5. Shaikh A. A Practical Approach to Hypertension Management in Diabetes. Diabetes Ther (2017) 8:981–9. doi: 10.1007/s13300-017-0310-3
6. Leung A, Amaram V, Natarajan R. Linking diabetic vascular complications with LncRNAs. Vascul Pharmacol (2019) 114:139–44. doi: 10.1016/j.vph.2018.01.007
7. Yamazaki D, Hitomi H, Nishiyama A. Hypertension with diabetes mellitus complications. Hypertens Res (2018) 41:147–56. doi: 10.1038/s41440-017-0008-y
8. Choby B. Diabetes Update: Prevention and Management of Diabetes Complications. FP Essent (2017) 456:36–40.
9. Maric-Bilkan C. Sex differences in micro- and macro-vascular complications of diabetes mellitus. Clin Sci (Lond) (2017) 131:833–46. doi: 10.1042/CS20160998
10. Paneni F, Beckman JA, Creager MA, Cosentino F. Diabetes and vascular disease: pathophysiology, clinical consequences, and medical therapy: part I. Eur Heart J (2013) 34:2436–43. doi: 10.1093/eurheartj/eht149
11. Sorrentino FS, Matteini S, Bonifazzi C, Sebastiani A, Parmeggiani F. Diabetic retinopathy and endothelin system: microangiopathy versus endothelial dysfunction. Eye (Lond) (2018) 32:1157–63. doi: 10.1038/s41433-018-0032-4
12. Sena CM, Pereira AM, Seica R. Endothelial dysfunction - a major mediator of diabetic vascular disease. Biochim Biophys Acta (2013) 1832:2216–31. doi: 10.1016/j.bbadis.2013.08.006
13. Shi Y, Vanhoutte PM. Macro- and microvascular endothelial dysfunction in diabetes. J Diabetes (2017) 9:434–49. doi: 10.1111/1753-0407.12521
14. Capitão M, Soares R. Angiogenesis and Inflammation Crosstalk in Diabetic Retinopathy. J Cell Biochem (2016) 117:2443–53. doi: 10.1002/jcb.25575
15. Dinh QN, Drummond GR, Sobey CG, Chrissobolis S. Roles of inflammation, oxidative stress, and vascular dysfunction in hypertension. BioMed Res Int (2014) 2014:406960. doi: 10.1155/2014/406960
16. Odegaard AO, Jacobs DR Jr, Sanchez OA, Goff DC Jr, Reiner AP, Gross MD. Oxidative stress, inflammation, endothelial dysfunction and incidence of type 2 diabetes. Cardiovasc Diabetol (2016) 15:51. doi: 10.1186/s12933-016-0369-6
17. Pitocco D, Tesauro M, Alessandro R, Ghirlanda G, Cardillo C. Oxidative stress in diabetes: implications for vascular and other complications. Int J Mol Sci (2013) 14:21525–50. doi: 10.3390/ijms141121525
18. Ceriello A, Testa R, Genovese S. Clinical implications of oxidative stress and potential role of natural antioxidants in diabetic vascular complications. Nutr Metab Cardiovasc Dis (2016) 26:285–92. doi: 10.1016/j.numecd.2016.01.006
19. Rochette L, Zeller M, Cottin Y, Vergely C. Diabetes, oxidative stress and therapeutic strategies. Biochim Biophys Acta (2014) 1840(9):2709–29. doi: 10.1016/j.bbagen.2014.05.017
20. Yaribeygi H, Sathyapalan T, Atkin SL, Sahebkar A. Molecular Mechanisms Linking Oxidative Stress and Diabetes Mellitus. Oxid Med Cell Longev (2020) 2020:8609213. doi: 10.1155/2020/8609213
21. Kaludercic N, Di Lisa F. Mitochondrial ROS Formation in the Pathogenesis of Diabetic Cardiomyopathy. Front Cardiovasc Med (2020) 7:12. doi: 10.3389/fcvm.2020.00012
22. Keane KN, Cruzat VF, Carlessi R, de Bittencourt PI Jr, Newsholme P. Molecular Events Linking Oxidative Stress and Inflammation to Insulin Resistance and β-Cell Dysfunction. Oxid Med Cell Longev (2015) 2015:181643. doi: 10.1155/2015/181643
23. Volpe CMO, Villar-Delfino PH, Dos Anjos PMF, Nogueira-Machado JA. Cellular death, reactive oxygen species (ROS) and diabetic complications. Cell Death Dis (2018) 9(2):119. doi: 10.1038/s41419-017-0135-z
24. Chalkiadaki A, Guarente L. Sirtuins mediate mammalian metabolic responses to nutrient availability. Nat Rev Endocrinol (2012) 8:287–96. doi: 10.1038/nrendo.2011.225
25. Haigis MC, Sinclair DA. Mammalian sirtuins: biological insights and disease relevance. Annu Rev Pathol (2010) 5:253–95. doi: 10.1146/annurev.pathol.4.110807.092250
26. D’Onofrio N, Servillo L, Balestrieri ML. SIRT1 and SIRT6 Signaling Pathways in Cardiovascular Disease Protection. Antioxid Redox Signal (2018) 28:711–32. doi: 10.1089/ars.2017.7178
27. Sosnowska B, Mazidi M, Penson P, Gluba-Brzozka A, Rysz J, Banach M. The sirtuin family members SIRT1, SIRT3 and SIRT6: Their role in vascular biology and atherogenesis. Atherosclerosis (2017) 265:275–82. doi: 10.1016/j.atherosclerosis.2017.08.027
28. Zhang HN, Dai Y, Zhang CH, Omondi AM, Ghosh A, Khanra I, et al. Sirtuins family as a target in endothelial cell dysfunction: implications for vascular ageing. Biogerontology (2020) 21(5):495–516. doi: 10.1007/s10522-020-09873-z
29. Brooks CL, Gu W. How does SIRT1 affect metabolism, senescence and cancer? Nat Rev Cancer (2009) 9:123–8. doi: 10.1038/nrc2562
30. Hwang JW, Yao H, Caito S, Sundar IK, Rahman I. Redox regulation of SIRT1 in inflammation and cellular senescence. Free Radic Biol Med (2013) 61:95–110. doi: 10.1016/j.freeradbiomed.2013.03.015
31. Revollo JR, Li X. The ways and means that fine tune Sirt1 activity. Trends Biochem Sci (2013) 38:160–7. doi: 10.1016/j.tibs.2012.12.004
32. Yang Y, Fu W, Chen J, Olashaw N, Zhang X, Nicosia SV, et al. SIRT1 sumoylation regulates its deacetylase activity and cellular response to genotoxic stress. Nat Cell Biol (2007) 9:1253–62. doi: 10.1038/ncb1645
33. Kornberg MD, Sen N, Hara MR, Juluri KR, Nguyen JV, Snowman AM, et al. GAPDH mediates nitrosylation of nuclear proteins. Nat Cell Biol (2010) 12:1094–100. doi: 10.1038/ncb2114
34. Kim EJ, Kho JH, Kang MR, Um SJ. Active regulator of SIRT1 cooperates with SIRT1 and facilitates suppression of p53 activity. Mol Cell (2007) 28:277–90. doi: 10.1016/j.molcel.2007.08.030
35. Kim JE, Chen J, Lou Z. DBC1 is a negative regulator of SIRT1. Nature (2008) 451:583–6. doi: 10.1038/nature06500
36. Dai H, Sinclair DA, Ellis JL, Steegborn C. Sirtuin activators and inhibitors: Promises, achievements, and challenges. Pharmacol Ther (2018) 188:140–54. doi: 10.1016/j.pharmthera.2018.03.004
37. Mishra M, Duraisamy AJ, Kowluru RA. Sirt1: A Guardian of the Development of Diabetic Retinopathy. Diabetes (2018) 67:745–54. doi: 10.2337/db17-0996
38. Karbasforooshan H, Karimi G. The role of SIRT1 in diabetic retinopathy. BioMed Pharmacother (2018) 97:190–4. doi: 10.1016/j.biopha.2017.10.075
39. Kume S, Uzu T, Kashiwagi A, Koya D. SIRT1, a calorie restriction mimetic, in a new therapeutic approach for type 2 diabetes mellitus and diabetic vascular complications. Endocr Metab Immune Disord Drug Targets (2010) 10(1):16–24. doi: 10.2174/187153010790827957
40. Orimo M, Minamino T, Miyauchi H, Tateno K, Okada S, Moriya J, et al. Protective role of SIRT1 in diabetic vascular dysfunction. Arterioscler Thromb Vasc Biol (2009) 29:889–94. doi: 10.1161/ATVBAHA.109.185694
41. Ota H, Eto M, Kano MR, Kahyo T, Setou M, Ogawa S, et al. Induction of Endothelial Nitric Oxide Synthase, SIRT1, and Catalase by Statins Inhibits Endothelial Senescence Through the Akt Pathway. Arterioscl Throm Vas (2010) 30:2205–U2411. doi: 10.1161/ATVBAHA.110.210500
42. Herzig S, Shaw RJ. AMPK: guardian of metabolism and mitochondrial homeostasis. Nat Rev Mol Cell Biol (2018) 19:121–35. doi: 10.1038/nrm.2017.95
43. Ruderman NB, Xu XJ, Nelson L, Cacicedo JM, Saha AK, Lan F, et al. AMPK and SIRT1: a long-standing partnership? Am J Physiol Endocrinol Metab (2010) 298:E751–60. doi: 10.1152/ajpendo.00745.2009
44. Canto C, Gerhart-Hines Z, Feige JN, Lagouge M, Noriega L, Milne JC, et al. AMPK regulates energy expenditure by modulating NAD+ metabolism and SIRT1 activity. Nature (2009) 458:1056–60. doi: 10.1038/nature07813
45. Zheng Z, Chen H, Li J, Li T, Zheng B, Zheng Y, et al. Sirtuin 1-mediated cellular metabolic memory of high glucose viathe LKB1/AMPK/ROS pathway and therapeutic effects of metformin. Diabetes (2012) 61:217–28. doi: 10.2337/db11-0416
46. Chen S, Xiao X, Feng X, Li W, Zhou N, Zheng L, et al. Resveratrol induces Sirt1-dependent apoptosis in 3T3-L1 preadipocytes by activating AMPK and suppressing AKT activity and survivin expression. J Nutr Biochem (2012) 23:1100–12. doi: 10.1016/j.jnutbio.2011.06.003
47. Manna P, Achari AE, Jain SK. Vitamin D supplementation inhibits oxidative stress and upregulate SIRT1/AMPK/GLUT4 cascade in high glucose-treated 3T3L1 adipocytes and in adipose tissue of high fat diet-fed diabetic mice. Arch Biochem Biophys (2017) 615:22–34. doi: 10.1016/j.abb.2017.01.002
48. Tikoo K, Lodea S, Karpe PA, Kumar S. Calorie restriction mimicking effects of roflumilast prevents diabetic nephropathy. Biochem Biophys Res Commun (2014) 450:1581–6. doi: 10.1016/j.bbrc.2014.07.039
49. Cheng CF, Ku HC, Lin H. PGC-1α as a Pivotal Factor in Lipid and Metabolic Regulation. Int J Mol Sci (2018) 19(11):3447. doi: 10.3390/ijms19113447
50. Arany Z, Foo SY, Ma Y, Ruas JL, Bommi-Reddy A, Girnun G, et al. HIF-independent regulation of VEGF and angiogenesis by the transcriptional coactivator PGC-1alpha. Nature (2008) 451:1008–12. doi: 10.1038/nature06613
51. Rius-Pérez S, Torres-Cuevas I, Millán I, Ortega ÁL, Pérez S. PGC-1α, Inflammation, and Oxidative Stress: An Integrative View in Metabolism. Oxid Med Cell Longev (2020) 2020:1452696. doi: 10.1155/2020/1452696
52. Iwabu M, Yamauchi T, Okada-Iwabu M, Sato K, Nakagawa T, Funata M, et al. Adiponectin and AdipoR1 regulate PGC-1alpha and mitochondria by Ca(2+) and AMPK/SIRT1. Nature (2010) 464:1313–9. doi: 10.1038/nature08991
53. Yuan Y, Shi M, Li L, Liu J, Chen B, Chen Y, et al. Mesenchymal stem cell-conditioned media ameliorate diabetic endothelial dysfunction by improving mitochondrial bioenergetics viathe Sirt1/AMPK/PGC-1alpha pathway. Clin Sci (Lond) (2016) 130:2181–98. doi: 10.1042/CS20160235
54. Rodgers JT, Lerin C, Haas W, Gygi SP, Spiegelman BM, Puigserver P. Nutrient control of glucose homeostasis through a complex of PGC-1alpha and SIRT1. Nature (2005) 434:113–8. doi: 10.1038/nature03354
55. Rodgers JT, Lerin C, Gerhart-Hines Z, Puigserver P. Metabolic adaptations through the PGC-1 alpha and SIRT1 pathways. FEBS Lett (2008) 582:46–53. doi: 10.1016/j.febslet.2007.11.034
56. Zheng A, Li H, Xu J, Cao K, Li H, Pu W, et al. Hydroxytyrosol improves mitochondrial function and reduces oxidative stress in the brain of db/db mice: role of AMP-activated protein kinase activation. Br J Nutr (2015) 113:1667–76. doi: 10.1017/S0007114515000884
57. Gallardo-Montejano VI, Saxena G, Kusminski CM, Yang C, McAfee JL, Hahner L, et al. Nuclear Perilipin 5 integrates lipid droplet lipolysis with PGC-1alpha/SIRT1-dependent transcriptional regulation of mitochondrial function. Nat Commun (2016) 7:12723. doi: 10.1038/ncomms12723
58. Park HS, Lim JH, Kim MY, Kim Y, Hong YA, Choi SR, et al. Resveratrol increases AdipoR1 and AdipoR2 expression in type 2 diabetic nephropathy. J Transl Med (2016) 14:176. doi: 10.1186/s12967-016-0967-9
59. Cheng L, Li B, Chen X, Su J, Wang H, Yu S, et al. CTRP9 induces mitochondrial biogenesis and protects high glucose-induced endothelial oxidative damage via AdipoR1 -SIRT1- PGC-1alpha activation. Biochem Biophys Res Commun (2016) 477:685–91. doi: 10.1016/j.bbrc.2016.06.120
60. Ding M, Feng N, Tang D, Feng J, Li Z, Jia M, et al. Melatonin prevents Drp1-mediated mitochondrial fission in diabetic hearts through SIRT1-PGC1alpha pathway. J Pineal Res (2018) 65(2):e12491. doi: 10.1111/jpi.12491
61. Wilhelm K, Happel K, Eelen G, Schoors S, Oellerich MF, Lim R, et al. FOXO1 couples metabolic activity and growth state in the vascular endothelium. Nature (2016) 529:216–20. doi: 10.1038/nature16498
62. Giannakou ME, Partridge L. The interaction between FOXO and SIRT1: tipping the balance towards survival. Trends Cell Biol (2004) 14(8):408–12. doi: 10.1016/j.tcb.2004.07.006
63. Kobayashi Y, Furukawa-Hibi Y, Chen C, Horio Y, Isobe K, Ikeda K, et al. SIRT1 is critical regulator of FOXO-mediated transcription in response to oxidative stress. Int J Mol Med (2005) 16(2):237–43. doi: 10.3892/ijmm.16.2.237
64. Brunet A, Sweeney LB, Sturgill JF, Chua KF, Greer PL, Lin Y, et al. Stress-dependent regulation of FOXO transcription factors by the SIRT1 deacetylase. Science (2004) 303:2011–5. doi: 10.1126/science.1094637
65. Wang B, Yang Q, Sun YY, Xing YF, Wang YB, Lu XT, et al. Resveratrol-enhanced autophagic flux ameliorates myocardial oxidative stress injury in diabetic mice. J Cell Mol Med (2014) 18:1599–611. doi: 10.1111/jcmm.12312
66. Mori J, Patel VB, Ramprasath T, Alrob OA, DesAulniers J, Scholey JW, et al. Angiotensin 1-7 mediates renoprotection against diabetic nephropathy by reducing oxidative stress, inflammation, and lipotoxicity. Am J Physiol Renal Physiol (2014) 306:F812–821. doi: 10.1152/ajprenal.00655.2013
67. Wang X, Meng L, Zhao L, Wang Z, Liu H, Liu G, et al. Resveratrol ameliorates hyperglycemia-induced renal tubular oxidative stress damage via modulating the SIRT1/FOXO3a pathway. Diabetes Res Clin Pract (2017) 126:172–81. doi: 10.1016/j.diabres.2016.12.005
68. Yerra VG, Kalvala AK, Kumar A. Isoliquiritigenin reduces oxidative damage and alleviates mitochondrial impairment by SIRT1 activation in experimental diabetic neuropathy. J Nutr Biochem (2017) 47:41–52. doi: 10.1016/j.jnutbio.2017.05.001
69. Kersten S P, Desvergne B, Wahli W. Roles of PPARs in health and disease. Nature (2000) 405:421–4. doi: 10.1038/35013000
70. Poynter ME, Daynes RA. Peroxisome proliferator-activated receptor alpha activation modulates cellular redox status, represses nuclear factor-kappaB signaling, and reduces inflammatory cytokine production in aging. J Biol Chem (1998) 273:32833–41. doi: 10.1074/jbc.273.49.32833
71. Guellich A, Damy T, Lecarpentier Y, Conti M, Claes V, Samuel JL, et al. Role of oxidative stress in cardiac dysfunction of PPARalpha-/- mice. Am J Physiol Heart Circ Physiol (2007) 293(1):H93–H102. doi: 10.1152/ajpheart.00037.2007
72. Goya K, Sumitani S, Xu X, Kitamura T, Yamamoto H, Kurebayashi S, et al. Peroxisome proliferator-activated receptor alpha agonists increase nitric oxide synthase expression in vascular endothelial cells. Arterioscler Thromb Vasc Biol (2004) 24:658–63. doi: 10.1161/01.ATV.0000118682.58708.78
73. Kim MY, Kang ES, Ham SA, Hwang JS, Yoo TS, Lee H, et al. The PPARdelta-mediated inhibition of angiotensin II-induced premature senescence in human endothelial cells is SIRT1-dependent. Biochem Pharmacol (2012) 84:1627–34. doi: 10.1016/j.bcp.2012.09.008
74. Zhao S, Li J, Wang N, Zheng B, Li T, Gu Q, et al. Fenofibrate suppresses cellular metabolic memory of high glucose in diabetic retinopathy viaa sirtuin 1-dependent signalling pathway. Mol Med Rep (2015) 12:6112–8. doi: 10.3892/mmr.2015.4164
75. Purushotham A, Schug TT, Xu Q, Surapureddi S, Guo X, Li X. Hepatocyte-specific deletion of SIRT1 alters fatty acid metabolism and results in hepatic steatosis and inflammation. Cell Metab (2009) 9(4):327–38. doi: 10.1016/j.cmet.2009.02.006
76. Hayashida S, Arimoto A, Kuramoto Y, Kozako T, Honda S, Shimeno H, et al. Fasting promotes the expression of SIRT1, an NAD+ -dependent protein deacetylase, via activation of PPARalpha in mice. Mol Cell Biochem (2010) 339(1-2):285–92. doi: 10.1007/s11010-010-0391-z
77. Zarzuelo MJ, López-Sepúlveda R, Sánchez M, Romero M, Gómez-Guzmán M, Ungvary Z, et al. SIRT1 inhibits NADPH oxidase activation and protects endothelial function in the rat aorta: implications for vascular aging. Biochem Pharmacol (2013) 85(9):1288–96. doi: 10.1016/j.bcp.2013.02.015
78. Gano LB, Donato AJ, Pasha HM, Hearon CM Jr, Sindler AL, Seals DR. The SIRT1 activator SRT1720 reverses vascular endothelial dysfunction, excessive superoxide production, and inflammation with aging in mice. Am J Physiol Heart Circ Physiol (2014) 307(12):H1754–63. doi: 10.1152/ajpheart.00377.2014
79. Luo X, Hu Y, He S, Ye Q, Lv Z, Liu J, et al. Dulaglutide inhibits high glucose- induced endothelial dysfunction and NLRP3 inflammasome activation. Arch Biochem Biophys (2019) 671:203–9. doi: 10.1016/j.abb.2019.07.008
80. Hung CH, Chan SH, Chu PM, Tsai KL. Quercetin is a potent anti-atherosclerotic compound by activation of SIRT1 signaling under oxLDL stimulation. Mol Nutr Food Res (2015) 59(10):1905–17. doi: 10.1002/mnfr.201500144
81. Triggle CR, Ding H. A review of endothelial dysfunction in diabetes: a focus on the contribution of a dysfunctional eNOS. J Am Soc Hypertens (2010) 4(3):102–15. doi: 10.1016/j.jash.2010.02.004
82. Nisoli E, Tonello C, Cardile A, Cozzi V, Bracale R, Tedesco L, et al. Calorie restriction promotes mitochondrial biogenesis by inducing the expression of eNOS. Science (2005) 310(5746):314–7. doi: 10.1126/science.1117728
83. Mattagajasingh I, Kim CS, Naqvi A, Yamamori T, Hoffman TA, Jung SB, et al. SIRT1 promotes endothelium-dependent vascular relaxation by activating endothelial nitric oxide synthase. Proc Natl Acad Sci U S A (2007) 104(37):14855–60. doi: 10.1073/pnas.0704329104
84. Sun Y, Hu X, Hu G, Xu C, Jiang H. Curcumin Attenuates Hydrogen Peroxide-Induced Premature Senescence viathe Activation of SIRT1 in Human Umbilical Vein Endothelial Cells. Biol Pharm Bull (2015) 38(8):1134–41. doi: 10.1248/bpb.b15-00012
85. Morrow VA, Foufelle F, Connell JM, Petrie JR, Gould GW, Salt IP. Direct activation of AMP-activated protein kinase stimulates nitric-oxide synthesis in human aortic endothelial cells. J Biol Chem (2003) 278(34):31629–39. doi: 10.1074/jbc.M212831200
86. Chen Z, Peng IC, Sun W, Su MI, Hsu PH, Fu Y, et al. AMP-activated protein kinase functionally phosphorylates endothelial nitric oxide synthase Ser633. Circ Res (2009) 104(4):496–505. doi: 10.1161/CIRCRESAHA.108.187567
87. Kondo M, Shibata R, Miura R, Shimano M, Kondo K, Li P, et al. Caloric restriction stimulates revascularization in response to ischemia via adiponectin-mediated activation of endothelial nitric-oxide synthase. J Biol Chem (2009) 284(3):1718–24. doi: 10.1074/jbc.M805301200
88. Csiszar A, Labinskyy N, Pinto JT, Ballabh P, Zhang H, Losonczy G, et al. Resveratrol induces mitochondrial biogenesis in endothelial cells. Am J Physiol Heart Circ Physiol (2009) 297(1):H13–20. doi: 10.1152/ajpheart.00368.2009
89. Zhang QJ, Wang Z, Chen HZ, Zhou S, Zheng W, Liu G, et al. Endothelium-specific overexpression of class III deacetylase SIRT1 decreases atherosclerosis in apolipoprotein E-deficient mice. Cardiovasc Res (2008) 80(2):191–9. doi: 10.1093/cvr/cvn224
90. Xia N, Strand S, Schlufter F, Siuda D, Reifenberg G, Kleinert H, et al. Role of SIRT1 and FOXO factors in eNOS transcriptional activation by resveratrol. Nitric Oxide (2013) 32:29–35. doi: 10.1016/j.niox.2013.04.001
91. Arumugam TV, Kennedy BK. H(2)S to Mitigate Vascular Aging: A SIRT1 Connection. Cell (2018) 173(1):8–10. doi: 10.1016/j.cell.2018.03.011
92. Testai L, Citi V, Martelli A, Brogi S, Calderone V. Role of hydrogen sulfide in cardiovascular ageing. Pharmacol Res (2020) 160:105125. doi: 10.1016/j.phrs.2020.105125
93. Jiang T, Yang W, Zhang H, Song Z, Liu T, Lv X. Hydrogen Sulfide Ameliorates Lung Ischemia-Reperfusion Injury Through SIRT1 Signaling Pathway in Type 2 Diabetic Rats. Front Physiol (2020) 11:596. doi: 10.3389/fphys.2020.00596
94. Ahmed HH, Taha FM, Omar HS, Elwi HM, Abdelnasser M. Hydrogen sulfide modulates SIRT1 and suppresses oxidative stress in diabetic nephropathy. Mol Cell Biochem (2019) 57(1-2):1–9. doi: 10.1007/s11010-019-03506-x
95. Wang CY, Zou W, Liang XY, Jiang ZS, Li X, Wei HJ, et al. Hydrogen sulfide prevents homocysteine−induced endoplasmic reticulum stress in PC12 cells by upregulating SIRT-1. Mol Med Rep (2017) 16(3):3587–93. doi: 10.3892/mmr.2017.7004
96. Du C, Lin X, Xu W, Zheng F, Cai J, Yang J, et al. Sulfhydrated Sirtuin-1 Increasing Its Deacetylation Activity Is an Essential Epigenetics Mechanism of Anti-Atherogenesis by Hydrogen Sulfide. Antioxid Redox Signal (2019) 30(2):184–97. doi: 10.1089/ars.2017.7195
97. Das A, Huang GX, Bonkowski MS, Longchamp A, Li C, Schultz MB, et al. Impairment of an Endothelial NAD(+)-H(2)S Signaling Network Is a Reversible Cause of Vascular Aging. Cell (2018) 173(1):74–89. doi: 10.1016/j.cell.2018.02.008
98. Zhou X, Cao Y, Ao G, Hu L, Liu H, Wu J, et al. CaMKKβ-dependent activation of AMP-activated protein kinase is critical to suppressive effects of hydrogen sulfide on neuroinflammation. Antioxid Redox Signal (2014) 21(12):1741–58. doi: 10.1089/ars.2013.5587
99. Shimizu Y, Polavarapu R, Eskla KL, Nicholson CK, Koczor CA, Wang R, et al. Hydrogen sulfide regulates cardiac mitochondrial biogenesis viathe activation of AMPK. J Mol Cell Cardiol (2018) 116:29–40. doi: 10.1016/j.yjmcc.2018.01.011
100. Tee AR. The Target of Rapamycin and Mechanisms of Cell Growth. Int J Mol Sci (2018) 19:E880. doi: 10.3390/ijms19030880
101. Cunningham JT, Rodgers JT, Arlow DH, Vazquez F, Mootha VK, Puigserver P. mTOR controls mitochondrial oxidative function through a YY1-PGC-1alpha transcriptional complex. Nature (2007) 450(7170):736–40. doi: 10.1038/nature06322
102. Vergès B, Cariou B. mTOR inhibitors and diabetes. Diabetes Res Clin Pract (2015) 110(2):101–8. doi: 10.1016/j.diabres.2015.09.014
103. Zhao D, Yang J, Yang L. Insights for Oxidative Stress and mTOR Signaling in Myocardial Ischemia/Reperfusion Injury under Diabetes. Oxid Med Cell Longev (2017) 2017:6437467. doi: 10.1155/2017/6437467
104. Igarashi M, Guarente L. mTORC1 and SIRT1 Cooperate to Foster Expansion of Gut Adult Stem Cells during Calorie Restriction. Cell (2016) 166:436–50. doi: 10.1016/j.cell.2016.05.044
105. Ma L, Dong W, Wang R, Li Y, Xu B, Zhang J, et al. Effect of caloric restriction on the SIRT1/mTOR signaling pathways in senile mice. Brain Res Bull (2015) 116:67–72. doi: 10.1016/j.brainresbull.2015.06.004
106. Wang RH, Kim HS, Xiao C, Xu X, Gavrilova O, Deng CX. Hepatic Sirt1 deficiency in mice impairs mTorc2/Akt signaling and results in hyperglycemia, oxidative damage, and insulin resistance. J Clin Invest (2011) 121:4477–90. doi: 10.1172/JCI46243
107. Maiese K. Programming apoptosis and autophagy with novel approaches for diabetes mellitus. Curr Neurovasc Res (2015) 12:173–88. doi: 10.2174/1567202612666150305110929
108. Maiese K. Erythropoietin and mTOR: A “One-Two Punch” for Aging-Related Disorders Accompanied by Enhanced Life Expectancy. Curr Neurovasc Res (2016) 13:329–40. doi: 10.2174/1567202613666160729164900
109. Ruiz MA, Chakrabarti S. MicroRNAs: the underlying mediators of pathogenetic processes in vascular complications of diabetes. Can J Diabetes (2013) 37:339–44. doi: 10.1016/j.jcjd.2013.07.003
110. Maiese K. Harnessing the Power of SIRT1 and Non-coding RNAs in Vascular Disease. Curr Neurovasc Res (2017) 14:82–8. doi: 10.2174/1567202613666161129112822
111. Li Q, Kim YR, Vikram A, Kumar S, Kassan M, Gabani M, et al. P66Shc-Induced MicroRNA-34a Causes Diabetic Endothelial Dysfunction by Downregulating Sirtuin1. Arterioscler Thromb Vasc Biol (2016) 36:2394–403. doi: 10.1161/ATVBAHA.116.308321
112. Arunachalam G, Lakshmanan AP, Samuel SM, Triggle CR, Ding H. Molecular Interplay between microRNA-34a and Sirtuin1 in Hyperglycemia-Mediated Impaired Angiogenesis in Endothelial Cells: Effects of Metformin. J Pharmacol Exp Ther (2016) 356:314–23. doi: 10.1124/jpet.115.226894
113. Zhou S, Chen HZ, Wan YZ, Zhang QJ, Wei YS, Huang S, et al. Repression of P66Shc expression by SIRT1 contributes to the prevention of hyperglycemia-induced endothelial dysfunction. Circ Res (2011) 109:639–48. doi: 10.1161/CIRCRESAHA.111.243592
114. Paneni F, Volpe M, Luscher TF, Cosentino F. SIRT1, p66(Shc), and Set7/9 in vascular hyperglycemic memory: bringing all the strands together. Diabetes (2013) 62:1800–7. doi: 10.2337/db12-1648
115. Guo Y, Li P, Gao L, Zhang J, Yang Z, Bledsoe G, et al. Kallistatin reduces vascular senescence and aging by regulating microRNA-34a-SIRT1 pathway. Aging Cell (2017) 16:837–46. doi: 10.1111/acel.12615
116. Carlomosti F, D’Agostino M, Beji S, Torcinaro A, Rizzi R, Zaccagnini G, et al. Oxidative Stress-Induced miR-200c Disrupts the Regulatory Loop Among SIRT1, FOXO1, and eNOS. Antioxid Redox Signal (2017) 27:328–44. doi: 10.1089/ars.2016.6643
117. Zhao S, Li T, Li J, Lu Q, Han C, Wang N, et al. miR-23b-3p induces the cellular metabolic memory of high glucose in diabetic retinopathy through a SIRT1-dependent signalling pathway. Diabetologia (2016) 59:644–54. doi: 10.1007/s00125-015-3832-0
118. Togliatto G, Trombetta A, Dentelli P, Gallo S, Rosso A, Cotogni P, et al. Unacylated ghrelin induces oxidative stress resistance in a glucose intolerance and peripheral artery disease mouse model by restoring endothelial cell miR-126 expression. Diabetes (2015) 64:1370–82. doi: 10.2337/db14-0991
119. Rane S, He M, Sayed D, Vashistha H, Malhotra A, Sadoshima J, et al. Downregulation of miR-199a derepresses hypoxia-inducible factor-1alpha and Sirtuin 1 and recapitulates hypoxia preconditioning in cardiac myocytes. Circ Res (2009) 104:879–86. doi: 10.1161/CIRCRESAHA.108.193102
120. Ramachandran D, Roy U, Garg S, Ghosh S, Pathak S, Kolthur-Seetharam U. Sirt1 and mir-9 expression is regulated during glucose-stimulated insulin secretion in pancreatic β-islets. FEBS J (2011) 278:1167–74. doi: 10.1111/j.1742-4658.2011.08042.x
121. Strum JC, Johnson JH, Ward J, Xie H, Feild J, Hester A, et al. MicroRNA 132 regulates nutritional stress-induced chemokine production through repression of SirT1. Mol Endocrinol (2009) 23:1876–84. doi: 10.1210/me.2009-0117
122. Chung S, Yao H, Caito S, Hwang JW, Arunachalam G, Rahman I. Regulation of SIRT1 in cellular functions: role of polyphenols. Arch Biochem Biophys (2010) 501:79–90. doi: 10.1016/j.abb.2010.05.003
123. Iside C, Scafuro M, Nebbioso A, Altucci L. SIRT1 Activation by Natural Phytochemicals: An Overview. Front Pharmacol (2020) 11:1225. doi: 10.3389/fphar.2020.01225
124. Milne JC, Lambert PD, Schenk S, Carney DP, Smith JJ, Gagne DJ, et al. Small molecule activators of SIRT1 as therapeutics for the treatment of type 2 diabetes. Nature (2007) 450:712–6. doi: 10.1038/nature06261
125. Kitada M, Ogura Y, Koya D. The protective role of Sirt1 in vascular tissue: its relationship to vascular aging and atherosclerosis. Aging (Albany NY) (2016) 8:2290–307. doi: 10.18632/aging.101068
126. Aditya R, Kiran AR, Varma DS, Vemuri R1, Gundamaraju R. A Review on SIRtuins in Diabetes. Curr Pharm Des (2017) 23:2299–307. doi: 10.2174/1381612823666170125153334
Keywords: SIRT1, oxidative stress, diabetic vascular complications, adenosine-monophosphate-activated protein kinase, mechanistic target of rapamycin, microRNAs
Citation: Meng T, Qin W and Liu B (2020) SIRT1 Antagonizes Oxidative Stress in Diabetic Vascular Complication. Front. Endocrinol. 11:568861. doi: 10.3389/fendo.2020.568861
Received: 11 June 2020; Accepted: 21 October 2020;
Published: 16 November 2020.
Edited by:
Pieter de Lange, University of Campania Luigi Vanvitelli, ItalyCopyright © 2020 Meng, Qin and Liu. This is an open-access article distributed under the terms of the Creative Commons Attribution License (CC BY). The use, distribution or reproduction in other forums is permitted, provided the original author(s) and the copyright owner(s) are credited and that the original publication in this journal is cited, in accordance with accepted academic practice. No use, distribution or reproduction is permitted which does not comply with these terms.
*Correspondence: Baohua Liu, cHBsaWV3QHN6dS5lZHUuY24=