- 1Instituto de Salud y Ambiente del Litoral (ISAL), Universidad Nacional del Litoral (UNL)- Consejo Nacional de Investigaciones Científicas y Técnicas (CONICET), Facultad de Bioquímica y Ciencias Biológicas, Universidad Nacional del Litoral, Santa Fe, Argentina
- 2Departamento de Bioquímica Clínica y Cuantitativa, Facultad de Bioquímica y Ciencias Biológicas, Universidad Nacional del Litoral, Santa Fe, Argentina
- 3Cátedra de Patología Humana, Facultad de Bioquímica y Ciencias Biológicas, Universidad Nacional del Litoral, Santa Fe, Argentina
- 4Cátedra de Fisiología Humana, Facultad de Bioquímica y Ciencias Biológicas, Universidad Nacional del Litoral, Santa Fe, Argentina
Glyphosate is a phosphonomethyl amino acid derivative present in a number of non-selective and systemic herbicides. During the last years the use of glyphosate-based herbicide (GBH) has been increasing exponentially around the world, including Argentina. This fact added to the detection of glyphosate, and its main metabolite, amino methylphosphonic acid (AMPA), in environmental matrices such as soil, sediments, and food, has generated great concern about its risks for humans, animals, and environment. During the last years, there were controversy and intense debate regarding the toxicological effects of these compounds associated with the endocrine system, cancer, reproduction, and development. The mechanisms of action of GBH and their metabolites are still under investigation, although recent findings have shown that they could comprise epigenetic modifications. These are reversible mechanisms linked to tissue-specific silencing of gene expression, genomic imprinting, and tumor growth. Particularly, glyphosate, GBH, and AMPA have been reported to produce changes in global DNA methylation, methylation of specific genes, histone modification, and differential expression of non-coding RNAs in human cells and rodents. Importantly, the epigenome could be heritable and could lead to disease long after the exposure has ended. This mini-review summarizes the epigenetic changes produced by glyphosate, GBHs, and AMPA in humans and rodents and proposes it as a potential mechanism of action through which these chemical compounds could alter body functions.
Introduction
Epigenetics is defined as ‘molecular factors and processes around DNA that regulate genome activity, independent of DNA sequence, and are mitotically stable’ (1). Epigenetic modifications include DNA methylation, post-translational modifications of histones, and differential expression of non-coding RNAs. Epigenetic processes may be related to silencing/activating of gene expression, genomic imprinting, and pathology development (2–6). Moreover, epigenetic marks could be maintained over time and be transmitted transgenerationally in second, third, and fourth generations (7).
The epigenome is influenced by both genetic (e.g. single nucleotide polymorphisms) and environmental factors (8–10). In this sense, several studies indicate that pesticides can exert toxicity through epigenetic changes [reviewed in (11)]. Among environmental chemicals, glyphosate-based herbicides (GBHs) have been one of the most intensively used pollutants over the last two decades. The herbicide glyphosate, N-(phosphonomethyl) glycine, is a biocide with a broad-spectrum activity since its mode of action is by inhibiting the enzyme 5-enolpyruvylshikimate-3- phosphate synthase, involved in the biosynthesis of aromatic compounds in plants and microorganisms (12). Monitoring studies have evidenced the presence of glyphosate residues and its main metabolite, (aminomethyl) phosphonic acid (AMPA), in surface water, sediments, and soil (13–16), respirable dust emitted by agricultural soil (17), a variety of crops at harvest and processed food (18–20), human urine samples (21, 22), maternal and umbilical cord serum (23), and breast milk samples (24). The widespread presence of these compounds shows that there is a risk of environmental exposure and concern about their possible effects on the environment and human health.
Several studies have reported adverse effects of GBH and glyphosate exposure on female and male murine reproductive systems, at both low and environmentally relevant doses, including disruption of the hypothalamic–pituitary-axis (25), uterine and ovary abnormalities, pre- and post-implantation embryo losses [reviewed in (26)] and testicular lesions (27). Supporting these results, in vitro studies found alterations in sperm motility and mitochondrial functions in human sperm cells (28, 29), as well as increased death of TM4 Sertoli cells (30) and disruption of blood–testis barrier integrity (31). However, there have been controversy and debate regarding the toxicological effects of these compounds. While the International Agency for Research on Cancer (IARC) concluded in March 2015 that the herbicide and its formulated products are probably carcinogenic in humans (IARC Group 2A) (IARC 2015, https://www.iarc.fr); the European Food Safety Authority (EFSA) decided that ‘glyphosate is unlikely to pose a carcinogenic risk to humans’ (EFSA 2015) (32). In 2017, the Environmental Protection Agency (EPA) issued a Draft Human Risk Assessment for Glyphosate, which concluded that glyphosate is not likely to be carcinogenic in humans. In 2019, the EPA released a Glyphosate Proposed Interim Registration Review Decision for public comments and, in 2020, released the Interim Registration Review Decision. The EPA continues to find that there are no risks to public health when glyphosate is used in accordance with its current label (EPA 2020 https://www.epa.gov).
The mechanisms of action of GBH and their metabolites are still under investigation. It has been reported that they could comprise interference with Ca+ ion-channels and peptide/steroid hormone response [reviewed in (11)]. More recently, epigenetic mechanisms have been also proposed as possible mediator of the action of these compounds (Table 1). This mini-review summarizes the current evidence about glyphosate-, GBH- and AMPA-induced epigenetic modifications in humans and rodents and proposes them as potential mechanisms through which these compounds could alter body functions.
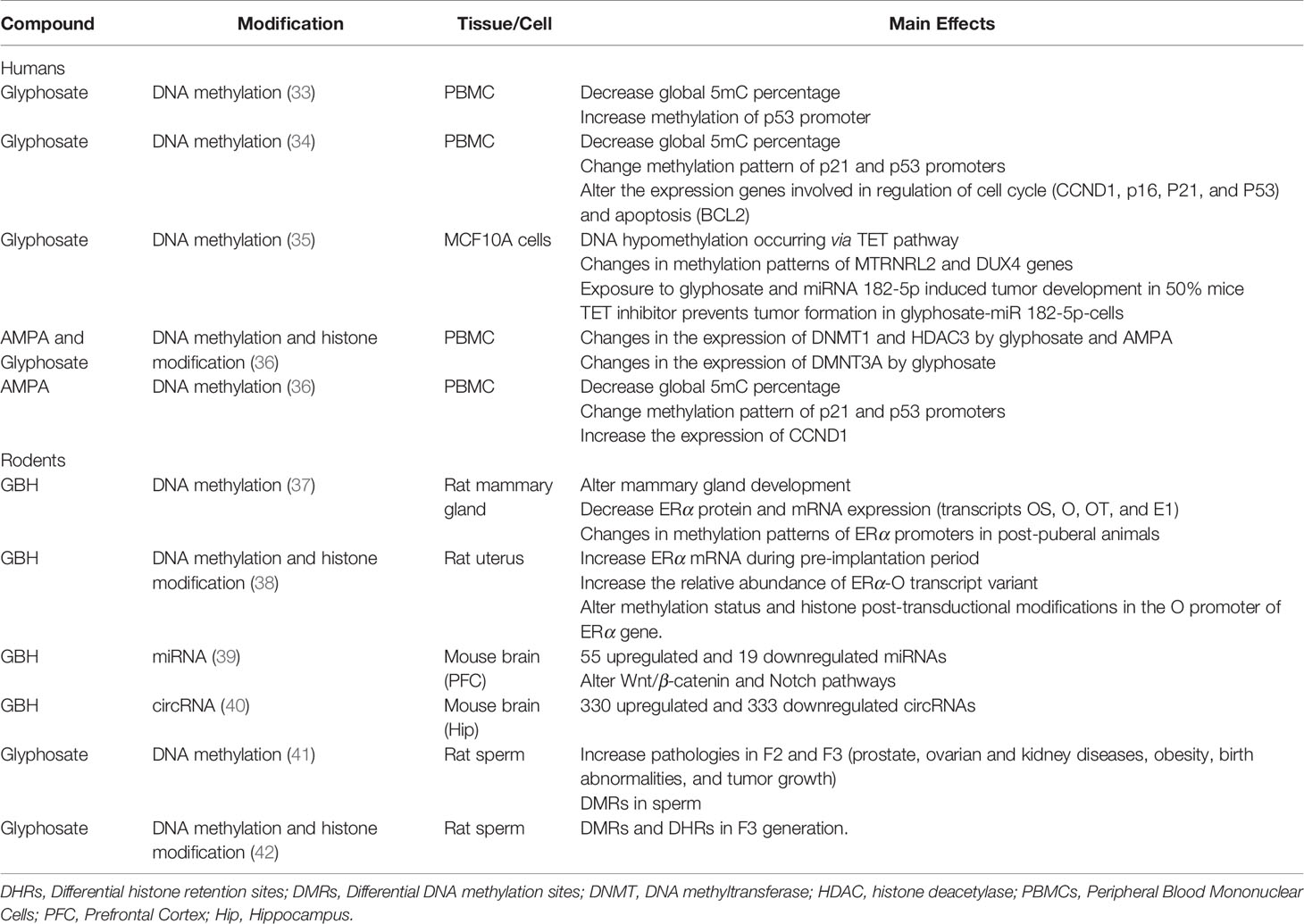
Table 1 Epigenetic modifications induced by glyphosate, glyphosate-based herbicides (GBH) and amino methylphosphonic acid (AMPA).
Epigenetic Mechanisms
DNA methylation is one of the most studied epigenetic modification. It occurs when DNA methyltransferases (DNMTs) transfer, in a reversible way, methyl groups from S-adenyl methionine to the fifth carbon of a cytosine residue, that is followed by a guanosine (CpG site), to form 5-methylcytosine (5mC) (43). The frequency of CpG sites is higher than expected in clusters known as CpG Island that are generally located in the promoter regions of genes. When these sites are methylated, transcription factors are not able to bind to the promoter regions, and the gene expression decreased. On the contrary, if these sites are demethylated, transcription is activated and gene expression is increased (9, 44).
Histone methylation could help to direct DNA methylation patterns, and DNA methylation seems to serve as a template for rebuilding histone modification patterns following DNA replication (45). Histone modification occurs on specific amino acid residues, changing the structure of chromatin and leading, together with the DNA methylation, to the activation or suppression of gene transcription (46). These biomarkers are known to be involved in the regulation of a broad range of biological processes, including DNA double-strand break repair (47). Trimethylated histone H3K9 (H3K9me3) and H3K27me3 are associated with decreased transcriptional activity and heterochromatin condensation (48). On the contrary, histone acetylation, in particular, increased acetylation of the H4 tail, is strongly linked to open, transcriptionally active regions of the chromatin (47).
DNA methylation also regulates microRNA (miRNA) biogenesis (49). miRNAs are small, non-coding RNAs that are recognized as endogenous regulators of post-transcriptional gene expression (5). Under normal physiological conditions, miRNAs function by safeguarding biological processes including cell cycle, proliferation, differentiation, and apoptosis. De-regulation of a single or small subset of miRNAs was reported to have a profound effect on the expression pattern of several hundred mRNAs which propels the cells towards transformation (6), leading to the development and progression of pathological conditions. Both hyper- and hypomethylation of miRNAs represent new levels of complexity in gene regulation (50).
Epigenetic Changes and Glyphosate-Based Herbicides
Several studies indicate that epigenetic mechanisms could mediate toxicity from pesticides. For example, methoxychlor induced changes in DNA methylation in rat ovary (51) and sperm (52). Moreover, dichlorodiphenyltrichloroethane (DDT) modified DNA methylation in rat hypothalamus (53). Atrazine (ATZ) was reported to dysregulate histone modification in mouse sperm (54, 55) and miRNA levels in rat brain and blood (56).
More recently, epigenetic processes in mammals have been also described after the exposure of glyphosate, GBH, and AMPA. To analyze the published data, we conducted a review of scientific publications on PubMed and Google Scholar searches using the following search terms: “glyphosate” or “GBH” or “AMPA” AND “epigenetic” or “DNA methylation” or “histone modification” or “miRNA” AND “human” or “rat” or “mice”. From these searches, eleven articles were found. Below, these works are described and discussed in detail.
Glyphosate and AMPA Induced DNA Methylation Changes in Human Cells
Tumor suppressor genes and proto-oncogenes play critical roles in cell cycle regulation, apoptosis, and cell senescence. Moreover, p16, p53, and p21 have important functions in the DNA-damage repair pathways which are among the most frequently compromised pathways in pathological conditions such as tumor growth (57). In this sense, most cancers have inactivating mutations in one or more proteins that normally function to restrict progression through the G1 stage of the cell cycle (e.g.: p16), and in proteins such as p53 that normally function at crucial cell-cycle checkpoints, stopping the cycle if a previous step has occurred incorrectly or if DNA has been damaged (58). In fact, the hypermethylation of p16 and p53 promoter regions is an epigenetic pattern frequently observed in human cancer development, and this condition is generally associated with reduced methylation level of global genomic DNA (59).
The effect of glyphosate on DNA methylation was first reported by Kwiatkowska et al. (33) in vitro (Table 1). These authors showed that high concentrations of glyphosate (from 84.54 to 1690 μg/ml) induce DNA lesions in peripheral blood mononuclear cells (PBMCs), decreased global 5mC percentage, and increased methylation of p53 promoter. Recently, similar results were reported, even at lower doses of glyphosate and AMPA (100–1,000 times lesser), in PBMC cells (34, 60) (Table 1). Importantly, they found that the hypermethylation of p16, p53, and p21 genes was able to downregulate their mRNA expression and activate proto-oncogenes, which could lead to genomic alterations, downstream function dysregulation, and cancer development risk. Supporting these possible effects, it was later reported by Santovito et al. (61) that human lymphocytes exposed to lower glyphosate concentrations (0.025–0.500 μg/ml) increased the frequency of chromosomal aberration and micronuclei. Later, Woźniak et al. (36) found that glyphosate changes the expression of DNMT1, DMNT3A, and histone deacetylase (HDAC) 3, while AMPA changes the expression of DNMT1 and HDAC3 in PBMCs. These enzymes are involved in the regulation of chromatin architecture and, thus, could affect methylation patterns and histone modification, leading to changes in gene expression. On the other hand, Duforestel et al. (35) found that glyphosate triggered a significant reduction in DNA methylation and increased ten-eleven translocation (TET) 3 activity in MCF10A cells. TET enzymes oxidize 5-methylcytosines and reverse methylation. Combining glyphosate with enhanced expression of miRNA 182-5p (associated with breast cancer) induced tumor development in mice, suggesting that DNA hypomethylation occurring via the TET pathway primes cells for oncogenic response in the presence of another potential risk factor (35).
Although controversies have grown about the carcinogenicity and toxicity consequence of glyphosate, effects have been shown on skin cancer promotion in mice and proliferation of human breast cells (62, 63). Taking into account the role of this herbicide as a “probable human carcinogen”, it would be interesting to analyze if the epigenetic changes of tumor suppressor genes observed in vitro could be replicated in in vivo models and if these molecular alterations could explain, at least in part, some of the adverse effects produce by glyphosate.
GBH Modifies the Methylation Status of the Estrogen Receptor α in Rats
Estrogens, a class of steroid hormones, regulate the growth, development, and physiology of the human reproductive system (64). They are produced principally by the gonads and placenta, but have multiple physiological functions on target organs such as the uterus, hypothalamus, pituitary, bone, mammary tissue, and liver (65). Estrogen signaling is mainly mediated through the classic nuclear receptor, estrogen receptor (ER) α. The expression of ERα occurs through different promoters depending on the tissue and physiological or developmental stages. In rats, five promoters have been described that result in transcripts with different 5′ untranslated regions derived from exons OS, ON, O, OT, and E1 (66). Importantly, the loss of expression, which is frequently observed in breast cancer, and the presence of triple negative tumors are often associated with hypermethylation of ER regulatory regions (37).
Several works report how the exposure to glyphosate alter the ER expression in vivo (67, 68) and in vitro (69–72), although the regulation mechanisms involved are still under study. Recently, Gomez et al. (37) and Lorenz et al. (38) found that developmental exposure to GBH (Filial 0, F0) from gestational day (GD) 9 until weaning induces epigenetic changes in ERα of F1 rats (Table 1). The first group reported that standard diet supplemented with a GBH in two doses, 3.50 and 350 mg of glyphosate/kg bw/day, decreased the expression of OS, O, OT, and E1 transcripts in male mammary glands at postnatal day (PND) 60. These changes were accompanied by an increased in the DNA methylation of their promoter regions (73). Along the same line, Lorenz et al. (38) found, in a similar model, that perinatal exposure to GBH in a dose of 350 mg of glyphosate/kg bw/day, upregulated the expression of ERα mRNA in the pregnant rat uterus (F1) at GD5 (preimplantation period). This change was associated with an increase in the abundance of the O transcript variant and a decrease in DNA methylation of its promoter. Supporting these transcriptional changes, histone H4 acetylation and H3K9me3 were enriched in the O promoter in GBH-exposed rats, whereas H3K27me3 was decreased.
These studies proposed that the adverse observed effects of GBH on mammary gland growth (37, 73–75), embryo implantation (76), uterine development and reproduction (67, 68, 77), could be mediated, at least in part, by aberrant DNA methylation and histone acetylation/methylation of ERα gene. In this sense, the disruption of ER was previously related to male and female outcomes, including infertility, abnormal uterine and sperm maturation, atypical ovarian functions, and implantation deficits (78). These results require particular attention since all the epigenetic alterations mentioned above were observed after the GBH exposure has ended, suggesting that this exposure during sensitive periods of development (gestation) could perturb epigenetic programming and could have a long-lasting impact later in life. So far, it is necessary to clarify whether these effects could be due to the active principle (glyphosate), the co-formulants, or a combination of both, since previous studies have shown that commercial formulations are more toxic than glyphosate alone (79). In addition, it would be interesting to consider different administration routes, timings of exposure, and time points as conditioning factors.
Glyphosate Induced Epigenetic Transgenerational Inheritance
Epigenetic transgenerational inheritance is a non-genetic form of inheritance that allows environmental factors to produce epigenetic alterations in the germline (sperm or egg) at critical periods of development that could be passed to subsequent generations, leading to pathologies or phenotypic variation in the absence of continued direct exposures (41). DNA methylation reprogramming could occur in the early embryo following fertilization (80), in the primordial germ cells in early gonadal development (81) or, even, during adult spermatogenesis in the testis (1).
Recently, Deepika Kubsad et al. (41) studied the transgenerational effect of glyphosate exposure (25 mg/kg bw/day) on pregnant rats (F0) during GD8 to 14. They found that this exposure produced in F1, F2, and F3 differential DNA methylation regions (DMRs) in the sperm (Table 1). DMR associated gene categories were mainly related to transcription, signaling, metabolism, receptors, and cytokines and include metabolic and cancer pathways. Negligible pathology was observed in the F0 and F1 generations, while a significant increase in prostate, kidney and ovarian diseases, obesity, and parturition (birth) abnormalities was observed in the F2 generation grand-offspring and F3 generation great-grand-offspring. Tumor development was also monitored in males and females and found to increase in the F2 generation glyphosate female lineage; the most predominant were mammary adenomas. In another work from the same group, sperm from F3 generation was studied for DMRs and differential histone retention sites (DHRs), that were correlated with known pathology specific-associated genes (42) (Table 1). Interestingly, overlapping sets of DMRs and DHRs were identified that were common for all the pathologies. These results support previous works that found adverse effects related to fetal parameters and structural congenital anomalies after perinatal exposure of GBH (F0) in second-generation of rats (F2) (76, 82, 83). However, Deepika Kubsad et al. (41) and Maamar et al. (42) reported for the first time that transgenerational inheritance of disease in rodents could be produced by DMRs and DHRs in the male germline, and these sites could potentially act as a biomarkers for specific diseases. Based on these findings, further studies are needed to deepen on the generational toxicology of glyphosate, in the disease etiology of the future generations.
Maternal Exposure to GBH Alter the Non-Coding RNAs Profile in the Rat Offspring
The effect of GBH on miRNAs was recently reported by Hua ji et al. (39) who studied the effect of glyphosate exposure (1% Roundup; equivalent to 50 mg/kg bw/day) during pregnancy and lactation (GD14 to PND7) in the offspring brain (Table 1). A miRNA microarray detected 55 upregulated (i.e: miR-711, miR-27b-3p, miR-142a-3p) and 19 downregulated (i.e: miR-34b-5p) miRNAs in the prefrontal cortex of mice at PND28 after maternal exposure. In addition, they reported abnormalities of the Wnt/β-catenin and Notch pathways in these animals that correspond with the dysregulation found in miRNA patterns. This support previous works that showed a disruption of Wnt proteins by neonatal exposure to GBH (2 mg/kg bw/day) from PND1 to PND7, in rat uterus (PND21) and implantation sites (GD9) (68, 77). In addition, exposure to glyphosate also produced a downregulation of these pathways in neuron cultures (84). In a second work and using the same model of exposure, these authors also found that circular RNA (circRNA) profile was significantly altered in the hippocampus of perinatal glyphosate exposure group (40) (Table 1). circRNAs are a special class of non-coding RNAs which may interact with miRNAs to regulate gene expression. The altered miRNA and circRNAs were related to biological functions, including neurogenesis, neuron differentiation, brain development, stress-associated steroid metabolism pathways, among others.
Some of the miRNAs and their target genes disrupted by glyphosate exposure were also reported to be involved in pathological conditions, such as neurological disorders, prostate cancer and breast cancer (40, 85–88). Particularly, miR-34b-5p affects Numbl and Notch1 genes, which are involved in the Notch signaling pathway. In addition, the 3′-untranslated regions of β-catenin and Lef-1, which are involved in the Wnt signaling, contain miR-34 binding sites and are sensitive to miR-34b-dependent regulation. Abnormal activation of the Wnt/β-catenin or Notch pathways may serve an important role in the pathogenesis of various reproductive outcomes, including preeclampsia (85), embryo implantation (86), endometriosis (87), and ovarian tumors (88). Taking all together, these findings provide a new basis for identifying the mechanism of action of glyphosate-induced neurotoxicity in the developing brain and could serve as a beginning for elucidating the more general mechanisms of GBH toxicity in human and animal models. In addition, more investigations are needed to clarify the interaction between circRNAs, miRNAs, and genes as possible target of glyphosate exposure.
Conclusions and Future Perspectives
Recent findings have shown that the exposure of glyphosate, GBH, or AMPA could affect epigenetic mechanisms. These include the decrease of global DNA methylation, alterations in the methylation pattern of specific regions, including ER and tumor suppressor genes, histone modifications, and differential expression of non-coding RNAs involved in, for example, Wnt and Notch pathways. These epigenetic markers have been involved in several physiological and pathological processes that were also reported after glyphosate, GBH, or AMPA exposure in animal models. In this sense, several lines of evidence indicate that the exposure to these compounds could alter the epigenome, disrupting the mRNA expression and protein levels of key genes involved in normal functions and thus, producing negative consequences (Figure 1). These epigenetic alterations could be heritable and could have a manifestation in health impacts and disease after the exposure has ended. Overall, more studies are needed to identify epigenetic targets, to define how they are dysregulated in human disease and their functional role, and to determine the critical windows of vulnerability by herbicide exposures. These points would influence environmental risk assessment and contribute to the development of prevention strategies for health outcomes.
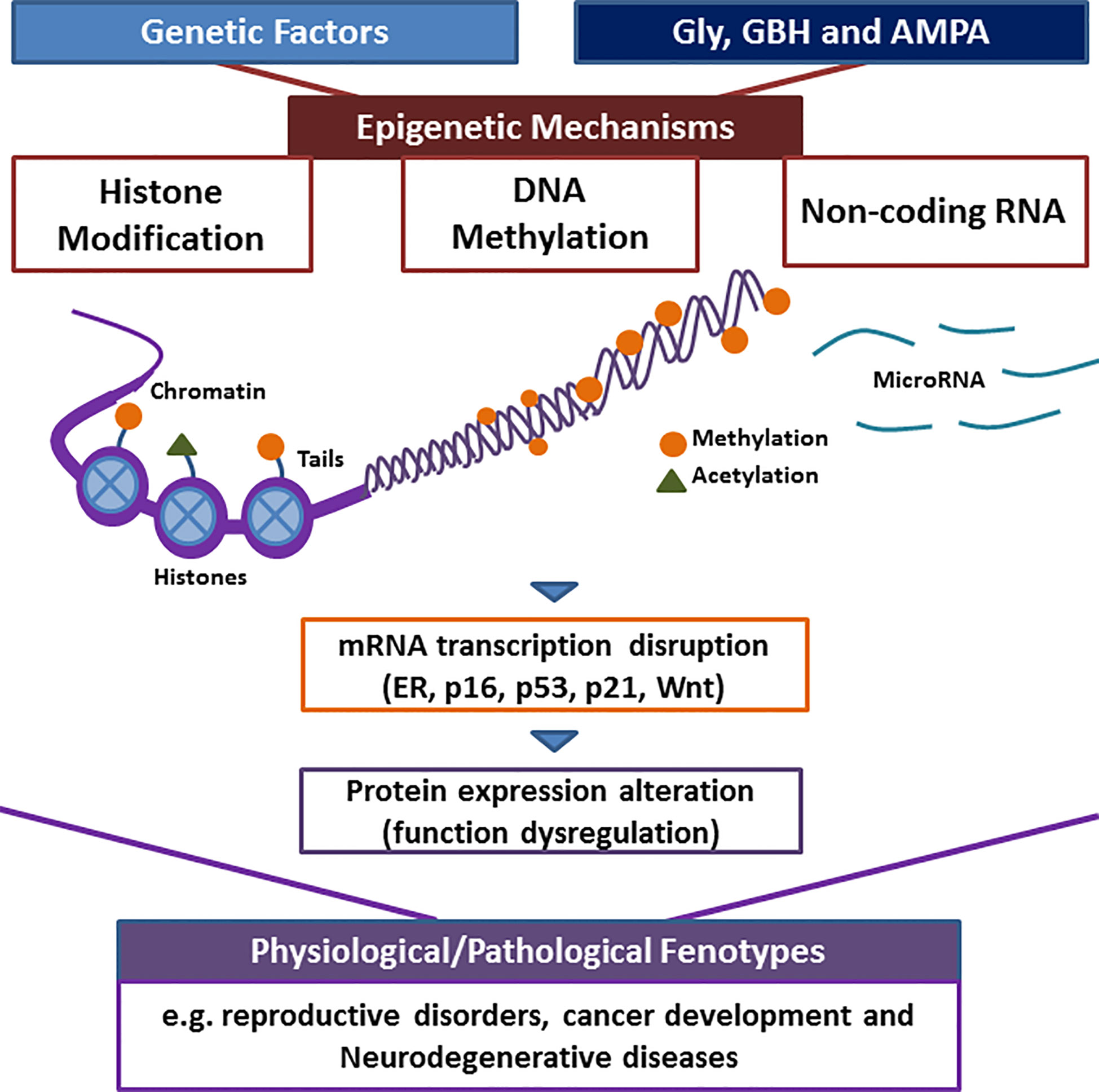
Figure 1 Possible mechanism that links the exposure to herbicides, the epigenome modification and the observed phenotypes. Chromosomes are composed of chromatin wrapped around proteins called histone; modifications of histone tails and DNA methylation control transcriptional access to DNA. Non-coding RNAs also regulate transcription. The exposure of herbicides, such as glyposhate (Gly), glyphosate-based herbicides (GBH) and, its main metabolite, (aminomethyl) phosphonic acid (AMPA) could alter the epigenome and could produce the silencing/activating of numerous genes, including estrogen receptor (ER), p16, p21, p53, and Wnt. This could result in the disruption of physiological functions and the promotion of health outcomes.
Author’s Note
MR, GC, and VL are fellows, and MM, JV, and JR are Career Investigators of the Consejo Nacional de Investigaciones Científicas y Técnicas (CONICET), Argentina.
Author Contributions
MR, GC, VL, MM, JV, and JR contributed equally to the literature search and to manuscript writing, revising, and editing. All authors contributed to the article and approved the submitted version.
Conflict of Interest
The authors declare that there is no conflict of interest that could be perceived as prejudicing the impartiality of the research reported.
Abbreviations
5mC, 5-methylcytosine; AMPA, (aminomethyl)phosphonic acid; ATZ, atrazine; circRNAs, circular RNAs; DNMT, DNA methyltransferase; EFSA, European Food Safety Authority; EPA, Environmental Protection Agency; ER, estrogen receptor; F, Filial; GBHs, glyphosate-based herbicides; GD, gestational day; HDAC, histone deacetylase; miRNA, microRNA; PBMC, peripheral blood mononuclear cell; PND, postnatal day.
References
1. Ben Maamar M, Nilsson E, Sadler-Riggleman I, Beck D, McCarrey JR, Skinner MK. Developmental Origins of Transgenerational Sperm DNA Methylation Epimutations Following Ancestral DDT Exposure. Dev Biol (2019) 445:280–93. doi: 10.1016/j.ydbio.2018.11.016
2. Murphy SK, Huang Z, Wen Y, Spillman MA, Whitaker RS, Simel LR, et al. Frequent IGF2/H19 Domain Epigenetic Alterations and Elevated Igf2 Expression in Epithelial Ovarian Cancer. Mol Cancer Res (2006) 4:283–92. doi: 10.1158/1541-7786.MCR-05-0138
3. Carvalho S, Freitas M, Antunes L, Monteiro-Reis S, Vieira-Coimbra M, Tavares A, et al. Prognostic Value of Histone Marks H3K27me3 and H3K9me3 and Modifying Enzymes EZH2, SETDB1 and LSD-1 in Colorectal Cancer. J Cancer Res Clin Oncol (2018) 144:2127–37. doi: 10.1007/s00432-018-2733-2
4. Hemmatzadeh M, Shomali N, Yousefzadeh Y, Mohammadi H, Ghasemzadeh A, Yousefi M. Micrornas: Small Molecules With a Large Impact on Pre-Eclampsia. J Cell Physiol (2020) 235:3235–48. doi: 10.1002/jcp.29286
5. Nelson PT, Wang WX, Rajeev BW. MicroRNAs (miRNAs) in Neurodegenerative Diseases. Brain Pathol (2008) 18:130–8. doi: 10.1111/j.1750-3639.2007.00120.x
6. Reddy KB. MicroRNA (miRNA) in Cancer. Cancer Cell Int (2015) 15:38. doi: 10.1186/s12935-015-0185-1
7. Anway MD, Cupp AS, Uzumcu M, Skinner MK. Epigenetic Transgenerational Actions of Endocrine Disruptors and Epigenetic Transgenerational Actions of Endocrine Disruptors and Male Fertility Male Fertility. Science (2005) 308:1466–9. doi: 10.1126/science.1108190
8. Rossetti MF, Varayoud J, Moreno-Piovano GS, Luque EH, Ramos JG. Environmental Enrichment Attenuates the Age-Related Decline in the mRNA Expression of Steroidogenic Enzymes and Reduces the Methylation State of the Steroid 5α-Reductase Type 1 Gene in the Rat Hippocampus. Mol Cell Endocrinol (2015) 412:330–8. doi: 10.1016/j.mce.2015.05.024
9. Rossetti MF, Schumacher R, Gastiazoro MP, Lazzarino GP, Andreoli MF, Stoker C, et al. Epigenetic Dysregulation of Dopaminergic System by Maternal Cafeteria Diet During Early Postnatal Development. Neuroscience (2020) 424:12–23. doi: 10.1016/j.neuroscience.2019.09.016
10. Shah S, McRae AF, Marioni RE, Harris SE, Gibson J, Henders AK, et al. Genetic and Environmental Exposures Constrain Epigenetic Drift Over the Human Life Course. Genome Res (2014) 24:1725–33. doi: 10.1101/gr.176933.114
11. Warner GR, Mourikes VE, Neff AM, Brehm E, Flaws JA. Mechanisms of Action of Agrochemicals Acting as Endocrine Disrupting Chemicals. Physiol Behav (2019) 63:1–18. doi: 10.1016/j.mce.2019.110680.Mechanisms
12. Martinez A, Al-Ahmad AJ. Effects of Glyphosate and Aminomethylphosphonic Acid on an Isogeneic Model of the Human Blood-Brain Barrier. Toxicol Lett (2019) 304:39–49. doi: 10.1016/j.toxlet.2018.12.013
13. Gunarathna S, Gunawardana B, Jayaweera M, Manatunge J, Zoysa K. Glyphosate and AMPA of Agricultural Soil, Surface Water, Groundwater and Sediments in Areas Prevalent With Chronic Kidney Disease of Unknown Etiology, Sri Lanka. J Environ Sci Heal - Part B Pestic Food Contam Agric Wastes (2018) 53:729–37. doi: 10.1080/03601234.2018.1480157
14. Reynoso EC, Peña RD, Reyes D, Chavarin-Pineda Y, Palchetti I, Torres E. Determination of Glyphosate in Water From a Rural Locality in México and its Implications for the Population Based on Water Consumption and Use Habits. Int J Environ Res Public Health (2020) 17:1–16. doi: 10.3390/ijerph17197102
15. Montiel-León JM, Munoz G, Vo Duy S, Do DT, Vaudreuil MA, Goeury K, et al. Widespread Occurrence and Spatial Distribution of Glyphosate, Atrazine, and Neonicotinoids Pesticides in the St. Lawrence and Tributary Rivers. Environ Pollut (2019) 250:29–39. doi: 10.1016/j.envpol.2019.03.125
16. Primost JE, Marino DJG, Aparicio VC, Costa JL, Carriquiriborde P. “Pseudo-Persistent” Pollutants Under Real-World Agricultural Management Practices in the Mesopotamic Pampas Agroecosystem, Argentina. Environ Pollut (2017) 229:771–9. doi: 10.1016/j.envpol.2017.06.006
17. Mendez MJ, Aimar SB, Aparicio VC, Ramirez Haberkon NB, Buschiazzo DE, De Gerónimo E, et al. Glyphosate and Aminomethylphosphonic Acid (AMPA) Contents in the Respirable Dust Emitted by an Agricultural Soil of the Central Semiarid Region of Argentina. Aeolian Res (2017) 29:23–9. doi: 10.1016/j.aeolia.2017.09.004
18. Myers JP, Antoniou MN, Blumberg B, Carroll L, Colborn T, Everett LG, et al. Concerns Over Use of Glyphosate-Based Herbicides and Risks Associated With Exposures: A Consensus Statement. Environ Heal A Glob Access Sci Source (2016) 15:19. doi: 10.1186/s12940-016-0117-0
19. Granby K, Johannesen S, Vahl M. Analysis of Glyphosate Residues in Cereals Using Liquid Chromatography-Mass Spectrometry (LC-MS/MS). Food Addit Contam (2003) 20:692–8. doi: 10.1080/0265203031000109477
20. Zoller O, Rhyn P, Rupp H, Zarn JA, Geiser C. Glyphosate Residues in Swiss Market Foods: Monitoring and Risk Evaluation. Food Addit Contam Part B Surveill (2018) 11:83–91. doi: 10.1080/19393210.2017.1419509
21. Niemann L, Sieke C, Pfeil R, Solecki R. A Critical Review of Glyphosate Findings in Human Urine Samples and Comparison With the Exposure of Operators and Consumers. J Fur Verbraucherschutz Und Leb (2015) 10:3–12. doi: 10.1007/s00003-014-0927-3
22. Parvez S, Gerona RR, Proctor C, Friesen M, Ashby JL, Reiter JL, et al. Glyphosate Exposure in Pregnancy and Shortened Gestational Length: A Prospective Indiana Birth Cohort Study. Environ Heal A Glob Access Sci Source (2018) 17:1–12. doi: 10.1186/s12940-018-0367-0
23. Kongtip P, Nankongnab N, Phupancharoensuk R, Palarach C, Sujirarat D, Sangprasert S, et al. Glyphosate and Paraquat in Maternal and Fetal Serums in Thai Women. J Agromedicine (2017) 22:282–9. doi: 10.1080/1059924X.2017.1319315
24. Steinborn A, Alder L, Michalski B, Zomer P, Bendig P, Martinez SA, et al. Determination of Glyphosate Levels in Breast Milk Samples From Germany by LC-MS/MS and GC-MS/MS. J Agric Food Chem (2016) 64:1414–21. doi: 10.1021/acs.jafc.5b05852
25. Pandey A, Rudraiah M. Analysis of Endocrine Disruption Effect of Roundup® in Adrenal Gland of Male Rats. Toxicol Rep (2015) 2:1075–85. doi: 10.1016/j.toxrep.2015.07.021
26. Ingaramo P, Alarcón R, Muñoz-de-Toro M, Luque EH. Are Glyphosate and Glyphosate-Based Herbicides Endocrine Disruptors That Alter Female Fertility? Mol Cell Endocrinol (2020) 518:110934. doi: 10.1016/j.mce.2020.110934
27. Owagboriaye FO, Dedeke GA, Ademolu KO, Olujimi OO, Ashidi JS, Adeyinka AA. Reproductive Toxicity of Roundup Herbicide Exposure in Male Albino Rat. Exp Toxicol Pathol (2017) 69:461–8. doi: 10.1016/j.etp.2017.04.007
28. Anifandis G, Katsanaki K, Lagodonti G, Messini C, Simopoulou M, Dafopoulos K, et al. The Effect of Glyphosate on Human Sperm Motility and Sperm Dna Fragmentation. Int J Environ Res Public Health (2018) 15:1117. doi: 10.3390/ijerph15061117
29. Anifandis G, Amiridis G, Dafopoulos K, Daponte A, Dovolou E, Gavriil E, et al. The In Vitro Impact of the Herbicide Roundup on Human Sperm Motility and Sperm Mitochondria. Toxics (2017) 6:1–9. doi: 10.3390/toxics6010002
30. Vanlaeys A, Dubuisson F, Seralini GE, Travert C. Formulants of Glyphosate-Based Herbicides Have More Deleterious Impact Than Glyphosate on TM4 Sertoli Cells. Toxicol Vitr (2018) 52:14–22. doi: 10.1016/j.tiv.2018.01.002
31. Gorga A, Rindone GM, Centola CL, Sobarzo C, Pellizzari EH, Camberos M del C, et al. In Vitro Effects of Glyphosate and Roundup on Sertoli Cell Physiology. Toxicol Vitr (2020) 62:104682. doi: 10.1016/j.tiv.2019.104682
32. Tarazona JV, Court-Marques D, Tiramani M, Reich H, Pfeil R, Istace F, et al. Glyphosate Toxicity and Carcinogenicity: A Review of the Scientific Basis of the European Union Assessment and its Differences With IARC. Arch Toxicol (2017) 91:2723–43. doi: 10.1007/s00204-017-1962-5
33. Kwiatkowska M, Reszka E, Woźniak K, Jabłońska E, Michałowicz J, Bukowska B, et al. DNA Damage and Methylation Induced by Glyphosate in Human Peripheral Blood Mononuclear Cells (In Vitro Study). Food Chem Toxicol (2017) 105:93–8. doi: 10.1016/j.fct.2017.03.051
34. Woźniak E, Reszka E, Jabłońska E, Balcerczyk A, Broncel M, et al. Glyphosate Affects Methylation in the Promoter Regions of Selected Tumor Suppressors as Well as Expression of Major Cell Cycle and Apoptosis Drivers in PBMCs (In Vitro Study). Toxicol Vitr (2020) 63:104736. doi: 10.1016/j.tiv.2019.104736
35. Duforestel M, Nadaradjane A, Bougras-Cartron G, Briand J, Olivier C, Frenel JS, et al. Glyphosate Primes Mammary Cells for Tumorigenesis by Reprogramming the Epigenome in a TET3-Dependent Manner. Front Genet (2019) 10:885. doi: 10.3389/fgene.2019.00885
36. Woźniak E, Reszka E, Jabłońska E, Michałowicz J, Huras B, et al. Glyphosate and Ampa Induce Alterations in Expression of Genes Involved in Chromatin Architecture in Human Peripheral Blood Mononuclear Cells (In Vitro). Int J Mol Sci (2021) 22:1–13. doi: 10.3390/ijms22062966
37. Gomez AL, Altamirano GA, Leturia J, Bosquiazzo VL, Muñoz-de-Toro M, Kass L. Male Mammary Gland Development and Methylation Status of Estrogen Receptor Alpha in Wistar Rats Are Modified by the Developmental Exposure to a Glyphosate-Based Herbicide. Mol Cell Endocrinol (2019) 481:14–25. doi: 10.1016/j.mce.2018.11.005
38. Lorenz V, Milesi MM, Schimpf MG, Luque EH, Varayoud J. Epigenetic Disruption of Estrogen Receptor Alpha Is Induced by a Glyphosate-Based Herbicide in the Preimplantation Uterus of Rats. Mol Cell Endocrinol (2019) 480:133–41. doi: 10.1016/j.mce.2018.10.022
39. Ji H, Xu L, Wang Z, Fan X, Wu L. Differential microRNA Expression in the Prefrontal Cortex of Mouse Offspring Induced by Glyphosate Exposure During Pregnancy and Lactation. Exp Ther Med (2018) 15:2457–67. doi: 10.3892/etm.2017.5669
40. Yu N, Tong Y, Zhang D, Zhao S, Fan X, Wu L, et al. Circular RNA Expression Profiles in Hippocampus From Mice With Perinatal Glyphosate Exposure. Biochem Biophys Res Commun (2018) 501:838–45. doi: 10.1016/j.bbrc.2018.04.200
41. Kubsad D, Nilsson EE, King SE, Sadler-Riggleman I, Beck D, Skinner MK. Assessment of Glyphosate Induced Epigenetic Transgenerational Inheritance of Pathologies and Sperm Epimutations: Generational Toxicology. Sci Rep (2019) 9:1–17. doi: 10.1038/s41598-019-42860-0
42. Ben Maamar M, Beck D, Nilsson EE, Kubsad D, Skinner MK. Epigenome-Wide Association Study for Glyphosate Induced Transgenerational Sperm DNA Methylation and Histone Retention Epigenetic Biomarkers for Disease. Epigenetics (2020) 00:1–18. doi: 10.1080/15592294.2020.1853319
43. Jin Z, Liu Y. DNA Methylation in Human Diseases. Genes Dis (2018) 5:1–8. doi: 10.1016/j.gendis.2018.01.002
44. Rossetti MF, Varayoud J, Lazzarino GP, Luque EH, Ramos JG. Pregnancy and Lactation Differentially Modify the Transcriptional Regulation of Steroidogenic Enzymes Through DNA Methylation Mechanisms in the Hippocampus of Aged Rats. Mol Cell Endocrinol (2016) 429:73–83. doi: 10.1016/j.mce.2016.03.037
45. Cedar H, Bergman Y. Linking DNA Methylation and Histone Modification: Patterns and Paradigms. Nat Rev Genet (2009) 10:295–304. doi: 10.1038/nrg2540
46. Venkatesh S, Workman JL. Histone Exchange, Chromatin Structure and the Regulation of Transcription. Nat Rev Mol Cell Biol (2015) 3:178–89. doi: 10.1038/nrm3941
47. Price BD, Dhar S, Gursoy-Yuzugullu O, Parasuram R. The Tale of a Tail: Histone H4 Acetylation and the Repair of DNA Breaks. Philos Trans R Soc B (2017) 372:20160284. doi: 10.1098/rstb.2016.0284
48. Lee MY, Lee J, Hyeon SJ, Cho H, Hwang YJ, Shin JY, et al. Epigenome Signatures Landscaped by Histone H3K9me3 Are Associated With the Synaptic Dysfunction in Alzheimer’s Disease. Aging Cell (2020) 19:e13153. doi: 10.1111/acel.13153
49. Glaich O, Parikh S, Bell RE, Mekahel K, Donyo M, Leader Y, et al. DNA Methylation Directs microRNA Biogenesis in Mammalian Cells. Nat Commun (2019) 10:1–11. doi: 10.1038/s41467-019-13527-1
50. Wang S, Wu W, Claret FX. Mutual Regulation of microRNAs and DNA Methylation in Human Cancers. Epigenetics (2017) 12:187–97. doi: 10.1080/15592294.2016.1273308
51. Zama AM, Uzumcu M. Fetal and Neonatal Exposure to the Endocrine Disruptor Methoxychlor Causes Epigenetic Alterations in Adult Ovarian Genes. Endocrinology (2009) 150:4681–91. doi: 10.1210/en.2009-0499
52. Manikkam M, Haque MM, Guerrero-Bosagna C, Nilsson EE, Skinner MK. Pesticide Methoxychlor Promotes the Epigenetic Transgenerational Inheritance of Adult-Onset Disease Through the Female Germline. PloS One (2014) 9:e102091. doi: 10.1371/journal.pone.0102091
53. Shutoh Y, Takeda M, Ohtsuka R, Haishima A, Yamaguchi S, Fujie H, et al. Low Dose Effects of Dichlorodiphenyltrichloroethane (DDT) on Gene Transcription and DNA Methylation in the Hypothalamus of Young Male Rats: Implication of Hormesis-Like Effects. J Toxicol Sci (2009) 34:469–82. doi: 10.2131/jts.34.469
54. Hao C, Gely-Pernot A, Kervarrec C, Boudjema M, Becker E, Khil P, et al. Exposure to the Widely Used Herbicide Atrazine Results in Deregulation of Global Tissue-Specific RNA Transcription in the Third Generation and is Associated With a Global Decrease of Histone Trimethylation in Mice. Nucleic Acids Res (2016) 44:9784–802. doi: 10.1093/nar/gkw840
55. Gely-Pernot A, Hao C, Becker E, Stuparevic I, Kervarrec C, Chalmel F, et al. The Epigenetic Processes of Meiosis in Male Mice Are Broadly Affected by the Widely Used Herbicide Atrazine. BMC Genomics (2015) 16:1–22. doi: 10.1186/s12864-015-2095-y
56. Li B, Jiang Y, Xu Y, Li Y, Li B. Identification of miRNA-7 as a Regulator of Brain-Derived Neurotrophic Factor/α-Synuclein Axis in Atrazine-Induced Parkinson’s Disease by Peripheral Blood and Brain microRNA Profiling. Chemosphere (2019) 233:542–8. doi: 10.1016/j.chemosphere.2019.05.064
57. Kulaberoglu Y, Gundogdu R, Hergovich A. The Role of p53/p21/p16 in DNA-Damage Signaling and DNA Repair. In: Genome Stability: From Virus to Human Application. United Kingdom: Elsevier Inc (2016). p. 243–56. doi: 10.1016/B978-0-12-803309-8.00015-X
58. Lodish H, Berk A, Zipursky SL, Matsudaira P, Baltimore D, Darnell J. Proto- Oncogenes and Tumor-Suppressor Genes. In: Molecular Cell Biology, 4th Edition Section 24.2. New York: W. H. Freeman (2000). Available at: https://www.ncbi.nlm.nih.gov/books/NBK21662.
59. Geraldes C, Gonçalves AC, Cortesão E, Pereira MI, Roque A, Paiva A, et al. Aberrant p15, p16, p53, and DAPK Gene Methylation in Myelomagenesis: Clinical and Prognostic Implications. Clin Lymphoma Myeloma Leuk (2017) 17:78–82. doi: 10.1016/j.clml.2016.08.016
60. Woźniak E, Reszka E, Jabłońska E, Mokra K, Balcerczyk A, et al. The Selected Epigenetic Effects of Aminomethylphosphonic Acid, a Primary Metabolite of Glyphosate on Human Peripheral Blood Mononuclear Cells (In Vitro). Toxicol In Vitro (2020) 66:104878. doi: 10.1016/j.tiv.2020.104878
61. Santovito A, Ruberto S, Gendusa C, Cervella P. In Vitro Evaluation of Genomic Damage Induced by Glyphosate on Human Lymphocytes. Environ Sci Pollut Res (2018) 25:34693–700. doi: 10.1007/s11356-018-3417-9
62. George J, Prasad S, Mahmood Z, Shukla Y. Studies on Glyphosate-Induced Carcinogenicity in Mouse Skin: A Proteomic Approach. J Proteomics (2009) 73:951–64. doi: 10.1016/j.jprot.2009.12.008
63. Thongprakaisang S, Thiantanawat A, Rangkadilok N, Suriyo T, Satayavivad J, Satayavivad AJ. Glyphosate Induces Human Breast Cancer Cells Growth Via Estrogen Receptors. Food Chem Toxicol (2013) 59:129–36. doi: 10.1016/j.fct.2013.05.057
64. Mueller SO, Korach KS. Estrogen Receptors and Endocrine Diseases: Lessons From Estrogen Receptor Knockout Mice. Curr Opin Pharmacol (2001) 1:613–9. doi: 10.1016/S1471-4892(01)00105-9
65. Findlay JK, Liew SH, Simpson ER, Korach KS. Estrogen Signaling in the Regulation of Female Reproductive Functions. Handb Exp Pharmacol (2010) 198:29–35. doi: 10.1007/978-3-642-02062-9_2
66. Monje L, Varayoud J, Luque EH, Ramos JG. Neonatal Exposure to Bisphenol A Modifies the Abundance of Estrogen Receptor α Transcripts With Alternative 5′-Untranslated Regions in the Female Rat Preoptic Area. J Endocrinol (2007) 194:201–12. doi: 10.1677/JOE-07-0014
67. Ingaramo PI, Guerrero Schimpf M, Milesi MM, Luque EH, Varayoud J. Acute Uterine Effects and Long-Term Reproductive Alterations in Postnatally Exposed Female Rats to a Mixture of Commercial Formulations of Endosulfan and Glyphosate. Food Chem Toxicol (2019) 134:110832. doi: 10.1016/j.fct.2019.110832
68. Guerrero Schimpf M, Milesi MM, Ingaramo PI, Luque EH, Varayoud J. Neonatal Exposure to a Glyphosate Based Herbicide Alters the Development of the Rat Uterus. Toxicology (2017) 376:2–14. doi: 10.1016/j.tox.2016.06.004
69. Gastiazoro MP, Durando M, Milesi MM, Lorenz V, Vollmer G, Varayoud J, et al. Glyphosate Induces Epithelial Mesenchymal Transition-Related Changes in Human Endometrial Ishikawa Cells Via Estrogen Receptor Pathway. Mol Cell Endocrinol (2020) 510:110841. doi: 10.1016/j.mce.2020.110841
70. Thongprakaisang S, Thiantanawat A, Rangkadilok N, Suriyo T, Satayavivad J. Glyphosate Induces Human Breast Cancer Cells Growth Via Estrogen Receptors. Food Chem Toxicol (2013) 59:129–36. doi: 10.1016/j.fct.2013.05.057
71. Mesnage R, Phedonos A, Biserni M, Arno M, Balu S, Corton JC, et al. Evaluation of Estrogen Receptor Alpha Activation by Glyphosate-Based Herbicide Constituents. Food Chem Toxicol (2017) 108:30–42. doi: 10.1016/j.fct.2017.07.025
72. Sritana N, Suriyo T, Kanitwithayanun J, Songvasin BH, Thiantanawat A, Satayavivad J. Glyphosate Induces Growth of Estrogen Receptor Alpha Positive Cholangiocarcinoma Cells Via Non-Genomic Estrogen Receptor/ERK1/2 Signaling Pathway. Food Chem Toxicol (2018) 118:595–607. doi: 10.1016/j.fct.2018.06.014
73. Gomez AL, Altamirano GA, Tschopp MV, Bosquiazzo VL, Muñoz-de-Toro M, Kass L. Exposure to a Glyphosate-Based Herbicide Alters the Expression of Key Regulators of Mammary Gland Development on Pre-Pubertal Male Rats. Toxicology (2020) 439:152477. doi: 10.1016/j.tox.2020.152477
74. Altamirano GA, Delconte MB, Gomez AL, Ingaramo PI, Bosquiazzo VL, Luque EH, et al. Postnatal Exposure to a Glyphosate-Based Herbicide Modifies Mammary Gland Growth and Development in Wistar Male Rats. Food Chem Toxicol (2018) 118:111–8. doi: 10.1016/j.fct.2018.05.011
75. Zanardi MV, Schimpf MG, Gastiazoro MP, Milesi MM, Muñoz-de-Toro M, Varayoud J, et al. Glyphosate-Based Herbicide Induces Hyperplastic Ducts in the Mammary Gland of Aging Wistar Rats. Mol Cell Endocrinol (2020) 501:110658. doi: 10.1016/j.mce.2019.110658
76. Milesi MM, Lorenz V, Pacini G, Repetti MR, Demonte LD, Varayoud J, et al. Perinatal Exposure to a Glyphosate-Based Herbicide Impairs Female Reproductive Outcomes and Induces Second-Generation Adverse Effects in Wistar Rats. Arch Toxicol (2018) 92:2629–43. doi: 10.1007/s00204-018-2236-6
77. Ingaramo PI, Varayoud J, Milesi MM, Schimpf MG, Muñoz-De-toro M, Luque EH. Effects of Neonatal Exposure to a Glyphosate-Based Herbicide on Female Rat Reproduction. Reproduction (2016) 152:403–15. doi: 10.1530/REP-16-0171
78. Lee H-R, Kim T-H, Choi K-C. Functions and Physiological Roles of Two Types of Estrogen Receptors, Erα and Erβ, Identified by Estrogen Receptor Knockout Mouse. Lab Anim Res (2012) 28:71. doi: 10.5625/lar.2012.28.2.71
79. Mesnage R, Defarge N, Spiroux de Vendômois J, Séralini GE. Potential Toxic Effects of Glyphosate and Its Commercial Formulations Below Regulatory Limits. Food Chem Toxicol (2015) 84:133–53. doi: 10.1016/j.fct.2015.08.012
80. Hackett JA, Azim Surani M. DNA Methylation Dynamics During the Mammalian Life Cycle. Philos Trans R Soc B Biol Sci (2013) 368:20110328. doi: 10.1098/rstb.2011.0328
81. von Meyenn F, Berrens RV, Andrews S, Santos F, Collier AJ, Krueger F, et al. Comparative Principles of DNA Methylation Reprogramming During Human and Mouse in Vitro Primordial Germ Cell Specification. Dev Cell (2016) 39:104–15. doi: 10.1016/j.devcel.2016.09.015
82. Milesi MM, Lorenz V, Beldomenico PM, Vaira S, Varayoud J, Luque EH. Response to Comments on: Perinatal Exposure to a Glyphosate-Based Herbicide Impairs Female Reproductive Outcomes and Induces Second-Generation Adverse Effects in Wistar Rats. Arch Toxicol (2019) 93:3635–8. doi: 10.1007/s00204-019-02609-0
83. Milesi MM, Lorenz V, Beldomenico PM, Vaira S, Varayoud J, Luque EH. Correction to: Response to Comments on: Perinatal Exposure to a Glyphosate-Based Herbicide Impairs Female Reproductive Outcomes and Induces Second-Generation Adverse Effects in Wistar Rats (Archives of Toxicology, (2019), 93, 12, (3635-3638), 10.1007/S002. Arch Toxicol (2020) 94:2897–8. doi: 10.1007/s00204-020-02814-2
84. Coullery R, Pacchioni AM, Rosso SB. Exposure to Glyphosate During Pregnancy Induces Neurobehavioral Alterations and Downregulation of Wnt5a-CaMKII Pathway. Reprod Toxicol (2020) 96:390–8. doi: 10.1016/j.reprotox.2020.08.006
85. Zhang Z, Wang X, Zhang L, Shi Y, Wang J, Yan H. Wnt/β-Catenin Signaling Pathway in Trophoblasts and Abnormal Activation in Preeclampsia (Review). Mol Med Rep (2017) 16:1007–13. doi: 10.3892/mmr.2017.6718
86. Xie H, Tranguch S, Jia X, Zhang H, Das SK, Dey SK, et al. Inactivation of Nuclear Wnt-β-Catenin Signaling Limits Blastocyst Competency for Implantation. Development (2008) 135:717–27. doi: 10.1242/dev.015339
87. González-Foruria I, Santulli P, Chouzenoux S, Carmona F, Chapron C, Batteux F. Dysregulation of the ADAM17/Notch Signalling Pathways in Endometriosis: From Oxidative Stress to Fibrosis. Mol Hum Reprod (2017) 23:488–99. doi: 10.1093/molehr/gax028
Keywords: glyphosate, amino methylphosphonic acid (AMPA), epigenetic, DNA methylation, histone modifications, miRNA
Citation: Rossetti MF, Canesini G, Lorenz V, Milesi MM, Varayoud J and Ramos JG (2021) Epigenetic Changes Associated With Exposure to Glyphosate-Based Herbicides in Mammals. Front. Endocrinol. 12:671991. doi: 10.3389/fendo.2021.671991
Received: 24 February 2021; Accepted: 03 May 2021;
Published: 21 May 2021.
Edited by:
Anderson Martino-Andrade, Federal University of Paraná, BrazilReviewed by:
Oleg Sergeyev, Lomonosov Moscow State University, RussiaPatricia Cristina Lisboa, Rio de Janeiro State University, Brazil
Copyright © 2021 Rossetti, Canesini, Lorenz, Milesi, Varayoud and Ramos. This is an open-access article distributed under the terms of the Creative Commons Attribution License (CC BY). The use, distribution or reproduction in other forums is permitted, provided the original author(s) and the copyright owner(s) are credited and that the original publication in this journal is cited, in accordance with accepted academic practice. No use, distribution or reproduction is permitted which does not comply with these terms.
*Correspondence: Jorge Guillermo Ramos, Z3JhbW9zQGZiY2IudW5sLmVkdS5hcg==