- Institute of Basic Medical Sciences, Xi’an Medical University, Xi’an, China
Glucose metabolism is primarily controlled by pancreatic hormones, with the coordinated assistance of the hormones from gastrointestine and adipose tissue. Studies have unfolded a sophisticated hormonal gastrointestinal-pancreatic-adipose interaction network, which essentially maintains glucose homeostasis in response to the changes in substrates and nutrients. Free fatty acids (FFAs) are the important substrates that are involved in glucose metabolism. FFAs are able to activate the G-protein coupled membrane receptors including GPR40, GPR120, GPR41 and GPR43, which are specifically expressed in pancreatic islet cells, enteroendocrine cells as well as adipocytes. The activation of FFA receptors regulates the secretion of hormones from pancreas, gastrointestine and adipose tissue to influence glucose metabolism. This review presents the effects of the FFA receptors on glucose metabolism via the hormonal gastrointestinal-pancreatic-adipose interactions and the underlying intracellular mechanisms. Furthermore, the development of therapeutic drugs targeting FFA receptors for the treatment of abnormal glucose metabolism such as type 2 diabetes mellitus is summarized.
Introduction
Glucose homeostasis ensures continuous energy supply to all the cells of the body. It protects the body against hypoglycemic shock and hyperglycemia-induced damage to the cells such as vascular cells and neurons. In general, the uptake of glucose is intermittent while its consumption is a continual process. The fluctuation of blood glucose is well restricted to a limited range by the neuronal and hormonal regulatory molecules, which constitute a complex network to target on the organs that are critical for the intake, absorption, storage, conversion and consumption of glucose (1, 2). In this regulation network, the hormones from pancreatic islet cells locate in the central position, with the assistance of hormones from gastrointestinal enteroendocrine cells (EECs) and adipocytes (3–5).
Similarly, to glucose, free fatty acids (FFAs) are important substrates and their metabolism is entangled with glucose for energy supply. FFAs show diverse regulatory effects on glucose metabolism according to their length and saturation (6). It is well known that FFAs enter into cells for β-oxidation and generate acetyl-CoA to link glucose metabolism via tricarboxylic acid cycle in mitochondria. Increasing studies have demonstrated that FFAs also function as extracellular ligands to activate G protein-coupled receptors (GPCR) on the plasma membrane. GPR40, GPR120, GPR84, GPR41 and GPR43 are identified as FFA receptors, and they are differently activated by long-chain, medium-chain, and short-chain FFAs (7–9).
FFA receptors are expressed in the cells that are critical to glucose metabolism. Pancreatic islet cells, EECs and adipocytes are equipped with FFA receptors in a cell-specific manner. At present, studies have shown that FFA receptors activation regulates the endocrine function of pancreatic islet cells, EECs and adipocytes, which takes part in the regulation of glucose homeostasis (8, 10). The integrated effects of FFA receptors on glucose metabolism via the hormonal gastrointestinal-pancreatic-adipose (G-P-A) interactions and the underlying intracellular molecular mechanisms are summarized in this review. The drug development targeting FFA receptors for the therapy of abnormal glucose metabolism such as type 2 diabetes mellitus (T2DM) is also discussed.
G-P-A interactions and glucose homeostasis
Insulin is secreted from islet β-cells and plays vital role in lowering blood glucose levels by acting on insulin-sensitive tissues and organs such as liver, skeletal muscles and adipose tissue. It stimulates the synthesis of glycogen and triglyceride and inhibits lipolysis to force the entry of blood glucose into cells (11). In contrast, glucagon is secreted from islet α-cells to elevate blood glucose levels by stimulating glycogenolysis, gluconeogenesis and lipolysis (12). Somatostatin (SS) and pancreatic polypeptide (PP) that are respectively secreted by islet δ-cells and γ-cells modulate insulin and glucagon secretion in a paracrine manner (13). The secretion of insulin and glucagon is primarily regulated by blood glucose, while it is also finely modulated by gastrointestinal hormones (GI hormones) and adipokines (14, 15).
GI hormones are a number of peptides that are secreted by different EECs (16). Glucagon like peptide-1 (GLP-1), cholecystokinin (CCK), gastric inhibitory peptide (GIP), ghrelin, gastrin and secretin are the well-known hormones that related to metabolism, and most of them take part in glucose metabolism by regulating insulin secretion or by acting directly on adipose tissue, liver, skeletal muscle and hypothalamus in central nervous system (CNS) (14) (17, 18). Adipokines are another group of proteins that are released by adipocytes. Among adipokines, leptin and adiponectin are well-known for their role in glucose metabolism (19). Leptin acts on CNS to inhibit appetite and stimulate sympathetic system to increase thermogenesis, and it also acts on islet β-cells to inhibit insulin secretion (20, 21). Leptin induces the loss of fat mass and the improvement of insulin sensitivity, which is beneficial to blood glucose control (22–24). However, leptin resistance occurs in obese subjects, which may contribute to the development of obesity and insulin resistance (25, 26). Adiponectin protects pancreatic islet β-cells against apoptosis and prevents islets loss after transplantation (27–29). Adiponectin also increases insulin sensitivity to improve glucose metabolism (30). The expression of leptin and adiponectin is regulated by islets hormones and GI hormones. For instance, leptin expression is increased by insulin (31), and adiponectin expression is upregulated by GLP-1 but inhibited by GIP (32–34).
The hormonal signals link pancreatic islet cells, EECs and adipocytes together to constitute a regulatory system for glucose metabolism (Figure 1). The G-P-A network responds to the fluctuation of blood glucose through a negative feedback mechanism to maintain glucose homeostasis. When the blood glucose level elevates after ingestion, insulin and some GI hormones increase to lower blood glucose by stimulating glycogen synthesis, inhibiting appetite, and reducing gluconeogenesis (35). Thus, the blood glucose is finely controlled in the normal range. Adipokines influence appetite, insulin secretion, insulin sensitivity and glucose utilization, which may be involved in the long-term mechanism for glucose metabolism.
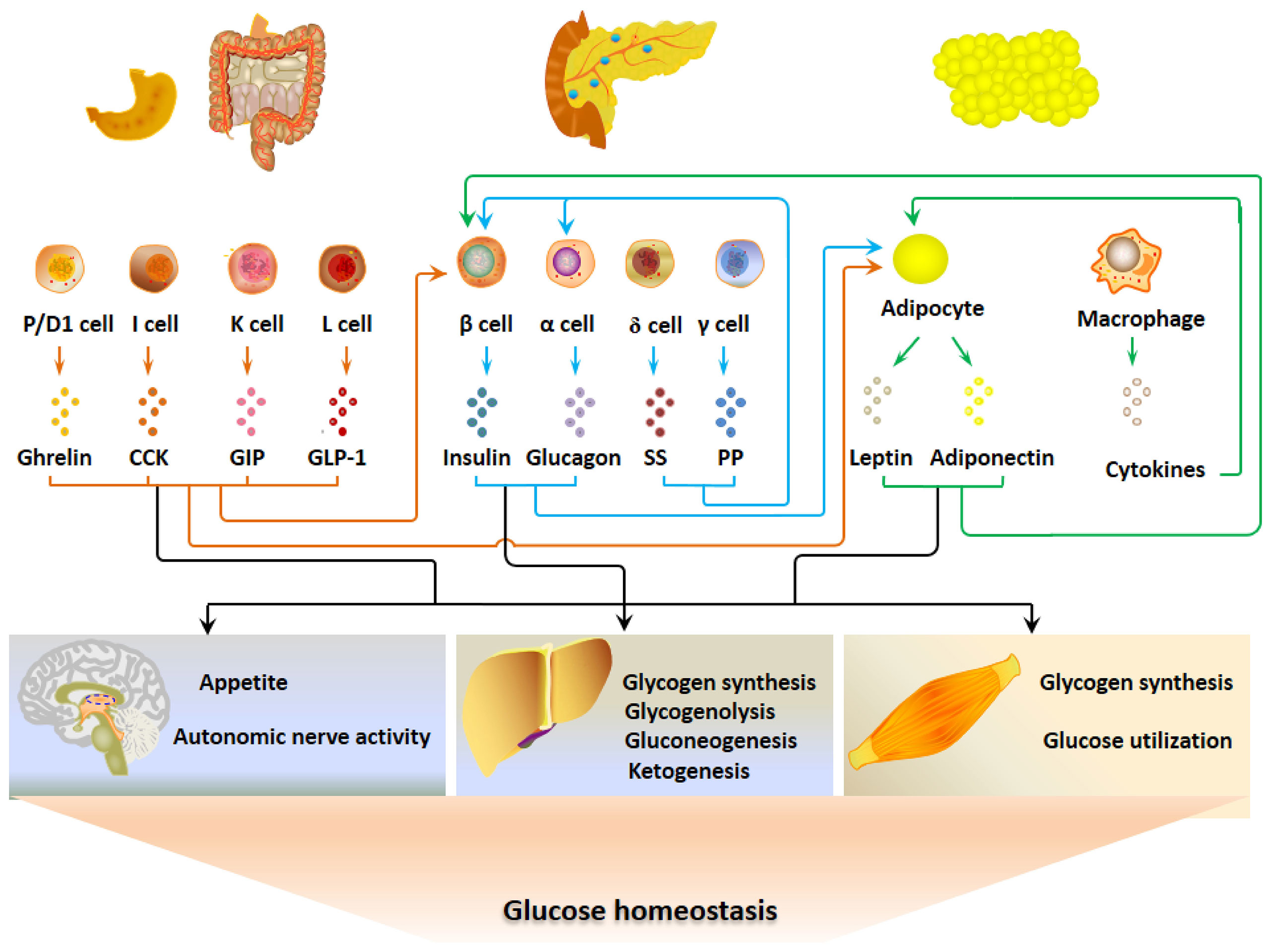
Figure 1 The gastrointestinal-pancreatic-adipose (G-P-A) interactions and hormonal regulation of glucose metabolism. When the blood glucose levels elevate after ingestion, insulin and certain GI hormones increase and act on the main target organs that include liver, skeletal muscle and central nervous system to lower blood glucose. Adipokines regulate glucose metabolism by altering appetite, insulin secretion, insulin sensitivity and glucose utilization, which may be a long-term mechanism for glucose metabolism.
The effects of FFAs on glucose metabolism
Glucose and FFAs are entangled in energy metabolism, and their interaction is crucial to the maintenance of glucose homeostasis. Fatty acids can be divided into short-chain (C2-C5), medium-chain (C6-C12) and long-chain fatty acids (C14-C26). All of them regulate insulin secretion. LCFFAs are divided into saturated and unsaturated FFAs, both of which are involved in insulin secretion. In general, FFAs exhibit rapid potentiation of glucose-stimulated insulin secretion (GSIS) on the basis of elevated blood glucose levels (6, 36, 37). Meanwhile, FFAs enhance the secretion of gastrointestinal hormones such as GLP-1, CCK and GIP, which are able to promote insulin secretion (38). The acute potentiation of insulin secretion may be helpful for the control of postprandial elevation of blood glucose. However, long-term elevation of FFAs in combination with glucose damages GSIS, induces insulin resistance, and leads to the elevation of basal blood glucose (39). In addition, FFAs in long-term incubation induce lipotoxic β-cell damage and contribute to the occurrence of T2DM (40, 41).
During fasting, FFAs show the beneficial effects on glucose metabolism. LCFFAs are used as the main energy fuel and go to β-oxidation to generate energy during fasting, and they are also converted to ketone bodies for energy supply. Then blood glucose is saved, and the hypoglycemia is prevented. Blood glucose can not remain stable without the usage of LCFFA during fasting and starvation. LCFFAs are also vital for the recovery of high insulin-secreting ability of pancreatic β-cells in response to glucose after ingestion (42, 43), which is crucial to the control of glucose homeostasis after refeeding.
In summary, the influence of FFAs on glucose metabolism can be physiologically beneficial to remain glucose homeostasis at low glucose levels. On the other hand, they lead to the pathological change and the occurrence of metabolic diseases at high concentrations in accompanying high glucose.
FFA receptors
It was previously considered that FFAs regulate glucose metabolism through intracellular metabolism. The discovery of FFA receptors that include GPR40 (FFA1), GPR120 (FFA4), GPR41 (FFA3) and GPR43 (FFA2) unveils a new mechanism of FFAs for their regulation of glucose metabolism. GPR40 and GPR120 are activated by LCFFA, while GPR43 and GPR41 are activated by SCFAs (8). Being members of the GPCR family, all the FFA receptors couple to heterotrimeric G proteins that are composed of α-subunit, β-subunit and γ-subunit (44). FFA receptors have been reported to activate multiple signaling pathways that are mediated by Gαs, Gαi/o, Gαq/11 subunits and β-arrestins (8). In a cell specific manner, GPR40 is coupled to Gαs, Gαi/o and Gαq/11 subunits, while GPR120 is coupled to Gαq/11, Gαi/o and β-arrestin2, respectively. GPR41 and GPR43 are coupled to Gαi/o, and GPR43 also couples to Gαq/11 subunit (8). The diversity of linkage between FFA receptors and G proteins enables the FFA receptor to execute flexible regulatory functions. The signaling pathways for FFA receptors have not been fully discovered, and the details of the relationship between intracellular signaling molecules and cellular responses needs to be further clarified.
It is well known that Gαs and Gαi/o affect the activity of adenylate cyclase (AC) and regulate intracellular cAMP levels and the relative signaling pathways (45). Gαq/11 is linked to phospholipase C (PLC) and activates the phosphatidylinositol signaling pathway (46). These signaling pathways regulate hormone secretion by altering the active state of many proteins including ion channels, vesicle trafficking proteins and exocytosis-related proteins. GPCR activation can recruit β-arrestins to the membrane for their binding to GPCR. β-Arrestins mediate the endocytosis of GPCR and negatively regulate GPCR signaling. Meanwhile, β-arrestins also interact with the intracellular signaling proteins (47). Mitogen-activated protein kinases (MAPK) cascade is an important signaling pathway for β-arrestin-activated intracellular signaling molecules (48).
GPR40 and glucose metabolism
GPR40 is distributed in pancreatic islet cells and EECs. The hormonal regulation of glucose metabolism by GPR40 is summarized in Figure 2. GPR40 activation by FFAs after fat ingestion potentiates the secretion of insulin, GLP-1, CCK and GIP, which restrain the elevation of blood glucose by acting on CNS, liver and skeletal muscle. Although GPR40 is not expressed in adipocytes, insulin and GI hormones act on adipocytes to improve glucose uptake and utilization as well as adipokine secretion.
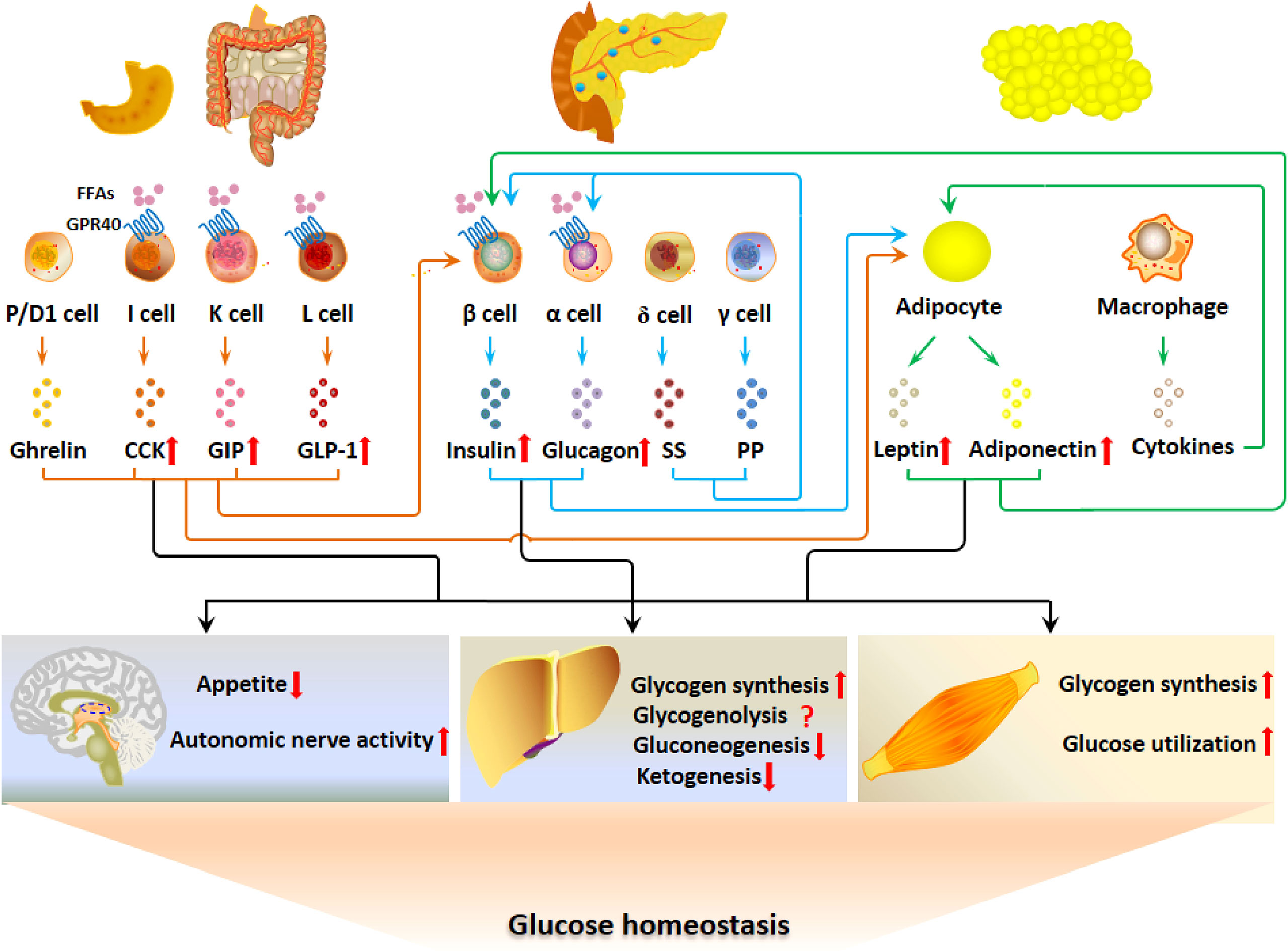
Figure 2 GPR40 regulates glucose metabolism via the G-P-A regulatory system. The activation of GPR40 by FFAs after fat ingestion potentiates the secretion of insulin, GLP-1, CCK and GIP, which contributes to the restrain of blood glucose elevation by acting on CNS, liver and skeletal muscle. Although GPR40 is not expressed in adipocytes, GPR40-potentiated insulin and GI hormones act on adipocytes to improve glucose uptake and utilization as well as adipokine secretion.
GPR40 and islet hormone secretion
GPR40 was first discovered as a FFA receptor in pancreatic islet β-cells (49). GPR40 activation was reported to potentiate insulin secretion in primary cultured murine β-cells, INS-1 cells, MIN6 cells and human islets in vitro as well as in human and rodents in vivo (50–53). GPR40 knockout mice show approximately 50% reduction in FFAs-induced insulin secretion in vivo. It is suggested that postprandial increase of blood FFAs activates GPR40 to potentiate GSIS. The activation of PLC via Gαq/11 subunit and the increase in intracellular Ca2+ concentrations ([Ca2+]i) mediate GPR40-potentiated insulin secretion (51, 54). The coupling of GPR40 to Gαs subunit and the activation of AC are also suggested to mediate the effects of GPR40 agonists on ion channels activities (55).
The potentiation of insulin secretion by GPR40 activation is glucose dependent. GPR40 activation increases insulin secretion at high glucose levels while it does not stimulate insulin secretion at low glucose levels (56). A conversion mechanism for GPR40 activation and insulin secretion may exist. Exocytosis of insulin granules is a complex process that is regulated by membrane potential, intracellular ATP levels, intracellular signaling molecules, and [Ca2+]i in β-cells (57, 58). Studies showed that GPR40 activation in rat pancreatic islet β-cells results in the opening of ATP-sensitive potassium channels (KATP channels) (59, 60). It is proposed that the intracellular environment of β-cells at low glucose levels facilitates the opening of KATP channels and GPR40 activation results in the opening of KATP channels. The opening of KATP channels hyperpolarizes membrane potential and then blocks insulin secretion. When the blood glucose level is up the stimulatory concentration for insulin secretion, there may be mechanism for the blockade of GPR40-induced opening of KATP channels. It is proposed that the state of KATP channels may be the reason for the glucose-dependence of GPR40 activation to stimulate insulin secretion.
GPR40 is also expressed in islet α-cells, and GPR40 activation potentiates glucagon secretion in rodent islets in vitro (61, 62). GPR40 agonists elicit the oscillatory increase in [Ca2+]i in α-cells by activating intracellular Ca2+ release from ER stores and the influx of extracellular Ca2+, and the increase in [Ca2+]i triggers exocytosis of glucagon granules (63, 64). When high fat diet (HFD) without sufficient glucose is consumed, hypoglycemia may occur, provided that glucagon does not elevate while insulin secretion increases. GPR40 activation by high FFAs promotes insulin secretion to store energy substrates. Meanwhile, GPR40 activation stimulates glucagon secretion to prevent hypoglycemia. Thus, the elevation of both insulin and glucagon after GPR40 activation may be a mechanism to harmonize between the uptake of energy in the forms of FFAs and the prevention of hypoglycemia under the intake of HFD.
GPR40 and GI hormone secretion
GPR40 is expressed in L cells, and its activation stimulates GLP-1 secretion, and the activation of Gαq/11-PLC-Ca2+ signaling pathway medicates GPR40-stimulated GLP-1 secretion (65). Some GPR40 agonists such as AM-1638 and AM-5262 also activate Gαs-AC-cAMP signaling pathway to potentiate GLP-1 secretion (65). GPR40 is also expressed in I cells in mouse small intestine, and GPR40 activation by LCFFAs induces the secretion of CCK in mice. The GPR40 knockout in mice leads to 50% reduction of linoleic acid-induced CCK secretion (66, 67). The Gαq/11/-PLC-Ca2+ signaling pathway mediates the effects of GPR40 activation on CCK secretion (66). Moreover, GPR40 is expressed in K cells and its agonists stimulate GIP secretion (68, 69).
GLP-1, CCK and GIP regulate glucose metabolism through multiple pathways. They potentiate insulin secretion by acting directly on β-cells in a glucose-dependent manner (70). Meanwhile, GLP-1 and CCK act on hypothalamus to inhibit food intake, which is a negative feedback mechanism for metabolic regulation (71). They also act on adipocytes. GLP-1 increases insulin sensitivity and promote fatty acid synthesis in adipocytes (72). Meanwhile, GLP-1 stimulates brown adipose tissue (BAT) thermogenesis and browning of white adipose tissue (WAT), which accelerate energy production and contributes to the lowering effects of GLP-1 on blood glucose levels (73–77). CCK and GIP promote fat deposit in adipocytes (78–81). The effect of CCK and GIP on fat deposit is a double-edged sword affecting glucose metabolism. To a certain extent, the induction of fat deposit may lower fatty acid levels and be beneficial to glucose metabolism. However, in the long run, it leads to obesity and insulin resistance and damage glucose metabolism. The action of GI hormones on adipocytes may be not involved in the acute regulation of blood glucose, but it may regulate glucose homeostasis in the long-term by changing the metabolic and secreting state of adipocytes.
Along with regulating adipocyte metabolism, GI hormones modulate the expression of adipokines. GLP-1 inhibits leptin expression in adipocytes. CCK antagonists increase leptin secretion from adipocytes (82). The physiological and pharmacological significance of GLP-1-inhibited leptin expression remains uncertain (83). GLP-1 and CCK upregulate adiponectin expression in adipocytes (33, 84, 85). Adiponectin exerts protective effects against inflammation and enhances insulin sensitivity in obese animals and humans (86, 87). Adiponectin also regulates glucose metabolism by stimulating fatty acids oxidation and glucose utilization in the skeletal muscle (88). The upregulation of adiponectin expression is suggested to be involved in GPR40-regulated glucose metabolism (86).
GPR120 and glucose metabolism
GPR120 is expressed in adipocytes, EECs, pancreatic islet cells, immune cells and pulmonary Clara cells (89). GPR120 was first found as an orphan receptor and later was identified as a FFA receptor in enteroendocrine L cells (7, 90). As shown in Figure 3, the postprandial activation of GPR120 by LCFFAs stimulates the secretion of GLP-1, CCK and GIP, with the inhibition of ghrelin secretion. The GI hormones, directly or indirectly though regulating the secretion of insulin and adipokines, regulate glucose homeostasis by acting on liver, skeletal muscle and CNS. GPR120 regulates the secretion of SS and PP in islets and then may bring about paracrine regulation of insulin and glucagon secretion. In addition, GPR120 regulates the function of adipocytes directly and indirectly via modulating the cytokine release from macrophages in adipose tissue. Thus, GPR120 activation excites the G-P-A regulatory system and regulates glucose metabolism.
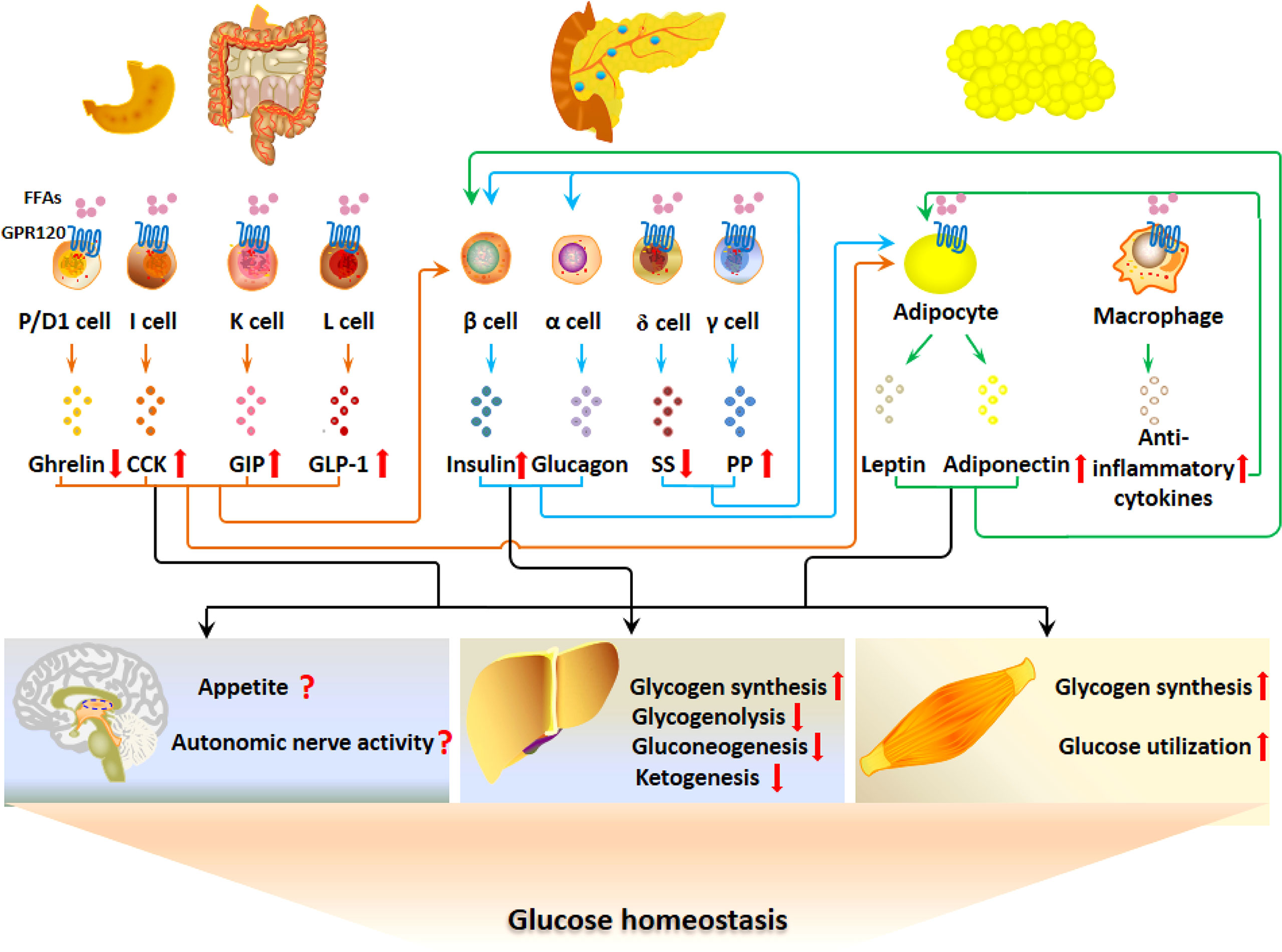
Figure 3 GPR120 regulates glucose metabolism via the G-P-A regulatory system. GPR120 activation after fat ingestion potentiates the secretion of GLP-1, CCK and GIP with the inhibition of ghrelin. Meanwhile, GPR120 activation increases adiponectin secretion. The GI hormones and adiponectin may act on CNS, liver and skeletal muscles to inhibit appetite, increase autonomous nerve activity, stimulate glycogen synthesis, and promote glucose utilization. GPR120 regulates the secretion of SS and PP in islets, which may influence the secretion of insulin in a paracrine manner. In addition, GPR120 regulates the function of adipocytes directly and indirectly via modulating the cytokine release from macrophages.
GPR120 and GI hormone secretion
GPR120-deficient mice developed more severe obesity and glucose intolerance when fed HFD compared with the wild type (WT) mice (91). GPR120 activation promotes GLP-1 secretion from enteroendocrine L cells in vitro (7). The increase in [Ca2+]i and the activation of extracellular signal-regulated kinase (ERK1/2) are involved in GPR120-stimulated GLP-1 secretion (7). It was suggested that GLP-1 is responsible for the acute blood glucose-lowering effects of GPR120 agonists (92). However, other reports showed that GLP-1 secretion after fat ingestion did not differ between WT mice and GPR120-knockout mice (93, 94). In GPR120 and GPR40-double knockout mice, GLP-1 secretion is not induced by oil ingestion, indicating GPR120 and GPR40 are essential for fat-induced GLP-1 secretion. These results also suggest that GPR40 activation may compensate for the defect of GPR120 in enteroendocrine L cells.
In intestine, GPR120 is also expressed in K cells and I cells, and its activation stimulates the secretion of GIP and CCK (93, 95). It has been known that FFAs stimulate CCK secretion in humans in vivo. Knockdown of GPR120 expression significantly attenuates FFA-induced CCK secretion (95), which indicates that GPR120 medicates the stimulatory effects of FFA on CCK secretion. Cellular analysis in STC-1 cells and GLUTag cells indicates that FFAs increase [Ca2+]i through the stimulation of intracellular Ca2+ release and extracellular Ca2+ influx (96). The activation of Gαq/11-PLC signaling pathway and the resultant opening of monovalent cation-specific transient receptor potential channel type 5 (TRPM5) to increase [Ca2+]i are responsible for GPR120-induced CCK secretion (97). GPR120 is expressed in K cells of the upper small intestine and mediate FFAs-stimulated GIP secretion in mice (93). Another study suggests that GPR120 activation stimulates CCK secretion and CCK acts on the K cells to stimulate GIP secretion (94, 98). Hormone secretion of endocrine cells such as K cells is finely controlled by many signal molecules. Some signal molecules are primary while the others are secondary, and they interrelate to regulate hormone secretion coordinately. Although the mechanism of GPR120-regulated GIP secretion is inconclusive, it is clear that GPR120 activation stimulates GIP secretion.
In stomach, GPR120 is expressed in ghrelin-secreting P/D1 cells, and its agonists inhibit ghrelin secretion in mice in vivo (99). GPR120 activation inhibited ghrelin secretion by activating the pertussis toxin-sensitive Gαi/o protein and inhibiting cAMP-mediated signaling pathways (100). Ghrelin is an orexigenic protein and its blood level increases during fasting to motivate ingestion. Ghrelin administration enhances appetite and increases food intake in humans and in rodents (101, 102). The inhibition of ghrelin secretion by GPR120 activation is suggested to be postprandial negative feedback to stop ingestion, which coordinates with the increase in the anorexigenic hormones such as GLP-1, CCK and GIP to regulate glucose metabolism.
Since GLP-1 and CCK inhibit appetite while ghrelin motivates ingestion, GPR120-induced changes in GI hormones are proposed to reduce appetite (103). However, GPR120 knockout mice did not show significant changes in food intake, indicating that GPR120 is physiologically dispensable for appetite regulation (91, 104). GPR120 is expressed in hypothalamus and maybe take part in unsaturated fatty acids-induced improvement of hypothalamic inflammation in obesity (105). Intracerebroventricular injection of GPR120 agonist (TUG1197) exerts anti-inflammatory activity but has no effect on body mass and caloric intake in 6-days treatment in obese mice (106). In another study, chronic intracerebroventricular injection of GPR120 agonist (GPR120 agonist III) also does not affect the intake of HFD in 15-days treatment in normal-weight mice (107). However, intracerebroventricular injection of GPR120 agonist acutely inhibits food intake in 4 hours after the administration (107). It is suggested that GPR120 plays a role in hypothalamus, but its pharmacological regulation of appetite remain to be fully investigated in the future.
GPR120 and adipocyte function
GPR120 is expressed in white adipose tissue including subcutaneous and visceral WAT as well as BAT (108). In adipose tissue, it is expressed both in adipocytes and in macrophages (109). The expression level of GPR120 increases with the differentiation of preadipocytes. It is previously considered that GPR120 is expressed in mature adipocytes but not in preadipocytes. A study showed that GPR120 is expressed in the ciliary structure of preadipocytes and senses the extracellular FFAs and activate cAMP/EPAC (the exchange protein activated by cAMP)/CTCF (CCCTC binding factor) signaling pathway, which results in remodeling of chromosome and promotion of expression of differentiation-related genes (110). 3T3-L1 cells exhibit a low differentiation rate when GPR120 is knocked down. Although GPR120-deficient mice exhibit an obese phenotype, they have decreased differentiation of adipocytes (91, 111). It is concluded that GPR120 activation in adipose tissue promotes the differentiation of preadipocytes. The adipocyte differentiation and triglyceride accumulation may benefit the decrease in blood glucose levels via the promotion of glucose transformation to triglyceride. Meanwhile, GPR120 activation in macrophages inhibits the release of inflammatory factors such as interleukin-6 (IL-6), tumor necrosis factor-α (TNF-α) and interleukin-1 (IL-1) to improve insulin sensitivity of adipocytes (112, 113), which promotes glucose entry into adipocytes and inhibits FFA release to favor the control of blood glucose (114, 115). Moreover, GPR120 activation promotes the browning of WAT via stimulating the secretion of fibroblast growth factor 21 (FGF21), and GPR120-deficient mice have impaired browning of WAT in response to cold exposure (116). The browning of WAT increases the thermogenesis and benefits the control of postprandial blood glucose levels. GPR120 is also highly expressed in BAT with upregulation by cold exposure in mice. A study showed that GPR120-deficient neonatal mice had reduced neonatal BAT activity and thermogenesis (117). GPR120 agonists have been shown to increase fatty acid uptake and oxidation, augment mitochondrial respiration, and reduce fat mass in mice (118). Thus, the promotion of thermogenesis is one mechanism of GPR120-regulated glucose metabolism.
GPR120 and islet hormone secretion
In pancreatic islets, GPR120 is not expressed in β-cells and α-cell. It is expressed in SS-secreting δ-cells PP-secreting γ-cells (119–122). GPR120 activation inhibits SS secretion but stimulates PP secretion in mouse islets (121, 122). The pertussis toxin-sensitive Gαi/o protein and its linked signaling pathway are suggested to mediate GPR120-inhibited SS secretion (121). The Gαq/11-PLC-Ca2+ signaling pathway is indicated to mediate GPR120-stimulated PP secretion (122). The physiological significance of this kind of cellular specificity of GPR120 expression in islets remains to be demonstrated. A recent study indicates the paracrine regulation of insulin secretion via GPR120-inhibited SS secretion (119).
GPR43/GPR41 and glucose metabolism
GPR43/GPR41 and hormone secretion
The SCFA receptor GPR43 and GPR41 are expressed in pancreatic β-cells and enteroendocrine L cells. GPR43-deficient mice showed a reduction of insulin secretion and developed more severe glucose intolerance when fed HFD compared with WT mice (123). GPR43 agonists increased insulin secretion via Gαq/11-PLC-Ca2+ signaling pathway in murine and human islets (123). Thus, GPR43 agonists directly act on β-cells to potentiate insulin secretion and regulate glucose metabolism. In addition, GPR43 agonists stimulate islet β-cell proliferation, and GPR43 deficiency caused a reduction in β-cell mass due to increased β-cell death (124). GPR43 agonists are suggested to enhance the compensatory capacity of β-cells to insulin resistance, which makes them potential therapeutic candidates for T2DM (123).
In contrary to GPR43, loss of GPR41 enhances glucose tolerance in mice, and GPR41 overexpression has opposite effects (125). The islets from GPR41-deficient mice have increased insulin secretion under high glucose although the islets from GPR41-overexpressing transgenic mice did not show significant changes in insulin secretion under high glucose (125). GPR41 is coupled to the Gαi/o subunit, and its activation leads to the inhibition of AC activity and the decrease in cAMP levels, which may be responsible for the reduction of insulin secretion. Therefore, although both GPR43 and GPR41 are activated by SCFAs, they mediate opposite effects on insulin secretion in β-cells. It is interesting to demonstrate the dominant type of receptors and the net effect of SCFAs on insulin secretion. GPR43/GPR41 double knockout improves glucose tolerance and insulin secretion (126). It is suggested that GPR41 has a negative but dominant effect over GPR43 and GPR43/GPR41 mediate a net inhibition on insulin secretion under normal conditions.
GPR43 is expressed in enteroendocrine L cells and mediates SCFAs-stimulated GLP-1 secretion in the mixed colonic cell cultures in vitro and in vivo (127, 128). GPR43-deficient mice show reduced SCFAs-induced GLP-1 secretion and impaired glucose tolerance (127). Gαq/11-PLC-Ca2+ signaling pathway was reported to mediate the effects of GPR43 activation on GLP-1 secretion in L cells (127). Although GPR41 is expressed in enteroendocrine L cells (129), its role in GLP-1 secretion remains to be demonstrated.
GPR43/GPR41 and adipocyte function
GPR43 is expressed in adipocytes, but its role in adipocytes is not clear (130). GPR43 expression levels in WAT are higher in HFD-induced obese mice than in normal chow-fed mice. It was reported that SCFAs treatment suppresses lipolysis in 3T3-L1 adipocytes and adipocytes isolated from mice adipose tissue and that GPR43 knockdown inhibits adipogenesis (131). However, another report showed that GPR43-deficient mice tend to become obese easier when fed HFD than WT mice and GPR43 overexpression in adipose tissue leads to the lean phenotype in mice (132). Further studies are needed to elucidate the role of GPR43 in white adipose tissue. As to BAT, the other type of adipose tissue, GPR43-deficient old age mice exhibit the increase in BAT activity and increased energy expenditure, which may be responsible for the improved insulin sensitivity in the mice (133). GPR43 mediates the stimulatory effects of SCFAs on adipogenesis and mitochondrial biogenesis in brown adipocytes (134). It is proposed that the stimulation of BAT contributes to the lean phenotype of GPR43 overexpression. However, GPR43 expression in adipose tissue is not different between obese patients and lean subjects, and GPR43 agonists do not induce the differentiation of human preadipocytes isolated from omental adipose tissue (135). This study indicates that a species difference has to be considered between humans and mice in the study of GPR43 actions.
The role of GPR41 in regulating adipocytes function is also unsettled. GPR41 expression was found in adipocytes, and its activation stimulated leptin secretion from adipose tissues (136). However, other studies did not detect GPR41 expression in mouse adipose tissue, and the stimulation of leptin secretion by SCFA is suggested to be mediated by GPR43 rather than GPR41 (137). Male GPR41-deficient mice show higher body fat mass and plasma leptin levels as well as higher glucose levels than WT mice (138). Although this study does not resolve the controversial about the expression of GPR41 in adipocytes, it demonstrates that GPR41 surely regulates fat and glucose metabolism via direct or indirect actions on adipocytes. Immune cells such as macrophages distribute in adipose tissue and modulate adipocyte function via paracrine signaling cytokines such as IL-6, IL-1, and TNF-α (139, 140). GPR41 and GPR43 are expressed in macrophages (141, 142). Therefore, the involvement of macrophages in the regulation of fat accumulation and adipokine secretion in adipocytes may complicate the observation of GPR43/GPR41-regulated adipocyte function.
Differences between SCFAs AND LCFFAs in metabolic regulation
SCFAs are very different to LCFFAs in characteristics and its source in human body. SCFAs in humans are mainly obtained from colon as the products of bacterial fermentation from insoluble fiber and proteins but not from food intake (143, 144). Thus, the physiological significance of SCFAs in regulating metabolism is surely different to LCFFAs. Although both SCFA receptors and LCFFA receptor are involved in the regulation of glucose metabolism through targeting the secretion of insulin and GLP-1 as well as the function of adipocytes, they should have distinct effects on glucose metabolism in physiological and pathophysiological conditions. The details in differences of FFA receptors in glucose metabolism in different metabolic states are worth of further exploration.
Targeting FFA receptors for drug development
The development of drugs targeting FFA receptors has been going on for decades, and the earliest is the development of GPR40 agonists for the treatment of T2DM. GPR40 agonist TAK-875 exhibits the ability to improve blood glucose control in patients with T2DM. However, it stopped in clinical trial III because of its hepatotoxicity (145). The other GPR40 agonists including LY2881835, AMG837, CPL207280, SCO-267, CPU-014 and AM-1638 are in the pipeline of drug development for T2DM treatment. Eli Lilly and Amgen initiated phase I/II clinical trials with LY2881835 and AMG837, respectively (146). Interestingly, GPR40 antagonists also have been developed for T2DM treatment. GPR40 antagonist DC260126 inhibits LCFA-stimulated increased in [Ca2+]i and protect β-cells against palmitate-induced ER stress and cell apoptosis (147, 148).
GPR120 agonists have been shown to improve insulin sensitivity in obese subjects. GPR120 agonist TUG-891 has been indicated as therapeutic agent of diabetes and obesity (8, 146). The other GPR120 agonists including NCG75, GSK137647A, AZ13581837 and CpdA all improve glucose tolerance in HFD-induced obese mice by increasing insulin sensitivity (146, 149, 150). Although a number of GPR120 agonists have been discovered, they have not moved to clinical trials. The insufficiency in both the understanding of GPR120 biology and the discovery of specific agonists with efficient effects in vivo may obstruct the development of drugs targeting GPR120.
GPR43 not only regulates glucose metabolism but also plays an important role in regulating immune function. GPR43 antagonists have been in development for anti-inflammation (151, 152). Since patients of T2DM are in the state of noninfectious microinflammation in multiple tissues such as adipose tissue, heart and liver (153), it is proposed that GPR43 antagonists may improve T2DM through anti-inflammation. GPR41 is also the therapeutic target for inflammatory diseases, but the development of drugs targeting GPR41 is relatively few compared with the other FFA receptors. There is still a long way to go for the development of drugs targeting GPR43/GPR41 for the treatment of metabolic diseases such as T2DM.
Conclusion and prospect
FFA receptors distribute in metabolism-related tissues to sense the fluctuation in extracellular FFAs and then regulate glucose metabolism through G-P-A regulatory system. The overlapping distribution of different types of FFA receptors in intestine indicates the importance of FFA receptors in nutrient sensing and metabolic regulation. This phenomenon also suggests that different FFA receptors may function differently and are distinguishingly activated in different nutritional states such as food intake, fasting, and obesity. Thus, the cells are able to response specifically to the changes in the level and composition of blood FFAs, which ensures the optimal fine-tuning of regulatory system for the maintenance of metabolism homeostasis. To date, the research in FFA receptor activation by different FFAs in vivo under different nutritional states is deficient. In the future, the detailed analysis of FFA receptor activation in different nutritional states will increase the understanding of FFA-regulated metabolism.
The regulatory effects of ligand-receptor interaction depend on not only the levels of ligands but also the levels of receptors. The changes in cellular expression of FFA receptors certainly influence the actions of FFAs on metabolism. It was found that HFD-induced obesity leads to the downregulation of GPR120 in intestine and in pancreatic islets in mice (122, 154). Due to the differentiation of adipocytes, GPR120 levels in subcutaneous fat and omental fat are increased in obese human subjects compared with those in lean subjects (91). However, morbidly obese human subjects (BMI 54.0 ± 5.7 kg/m2) have lower GPR120 levels in visceral adipose tissue than nonobese subjects, indicating that the enlarged adipocytes goes to the other side for GPR120 expression (154). Demonstration of the expression level of FFA receptors in different nutritional states is essential for understanding their physiological and pathophysiological role and the strategies to regulate metabolism through FFA receptors. It is suggested that compounds that upregulate the expression of FFA receptors may improve metabolic disorders synergistically with FFA receptor agonists. A recent study shows that PPAR-γ agonist upregulates GPR120 expression in adipocytes and synergistically enhances the effects of GPR120 agonists on metabolism (114). This study gives an example of how-to strength FFA receptors-FFAs interaction to regulate metabolism. More studies are expected to demonstrate the regulatory mechanism of the expression of FFA receptors in the future.
Author contributions
The author confirms being the sole contributor of this work and has approved it for publication.
Funding
This work was supported by the Funding from Innovative Group of Xi’an Medical University (No. 2021TD01).
Conflict of interest
The authors declares that the research was conducted in the absence of any commercial or financial relationships that could be construed as a potential conflict of interest.
Publisher’s note
All claims expressed in this article are solely those of the authors and do not necessarily represent those of their affiliated organizations, or those of the publisher, the editors and the reviewers. Any product that may be evaluated in this article, or claim that may be made by its manufacturer, is not guaranteed or endorsed by the publisher.
References
1. Wachsmuth HR, Weninger SN, Duca FA. Role of the gut-brain axis in energy and glucose metabolism. Exp Mol Med (2022) 54:377–92. doi: 10.1038/s12276-021-00677-w
2. Holst JJ, Gribble F, Horowitz M, Rayner CK. Roles of the gut in glucose homeostasis. Diabetes Care (2016) 39:884–92. doi: 10.2337/dc16-0351
3. Kim W, Egan JM. The role of incretins in glucose homeostasis and diabetes treatment. Pharmacol Rev (2008) 60:470–512. doi: 10.1124/pr.108.000604
4. Walker JT, Saunders DC, Brissova M, Powers AC. The human islet: Mini-organ with mega-impact. Endocrine Rev (2021) 42:605–57. doi: 10.1210/endrev/bnab010
5. Röder PV, Wu B, Liu Y, Han W. Pancreatic regulation of glucose homeostasis. Exp Mol Med (2016) 48:e219. doi: 10.1038/emm.2016.6
6. Chueire VB, Muscelli E. Effect of free fatty acids on insulin secretion, insulin sensitivity and incretin effect - a narrative review. Arch Endocrinol Metab (2021) 65:24–31. doi: 10.20945/2359-3997000000313
7. Hirasawa A, Tsumaya K, Awaji T, Katsuma S, Adachi T, Yamada M, et al. Free fatty acids regulate gut incretin glucagon-like peptide-1 secretion through GPR120. Nat Med (2005) 11:90–4. doi: 10.1038/nm1168
8. Kimura I, Ichimura A, Ohue-Kitano R, Igarashi M. Free fatty acid receptors in health and disease. Physiol Rev (2020) 100:171–210. doi: 10.1152/physrev.00041.2018
9. Miyamoto J, Hasegawa S, Kasubuchi M, Ichimura A, Nakajima A, Kimura I. Nutritional signaling via free fatty acid receptors. Int J Mol Sci (2016) 17:450. doi: 10.3390/ijms17040450
10. Kebede MA, Alquier T, Latour MG, Poitout V. Lipid receptors and islet function: therapeutic implications? Diabetes Obes Metab (2009) 11 Suppl 4:10–20. doi: 10.1111/j.1463-1326.2009.01114.x
11. Norton L, Shannon C, Gastaldelli A, DeFronzo RA. Insulin: The master regulator of glucose metabolism. Metabolism: Clin Exp (2022) 129:155142. doi: 10.1016/j.metabol.2022.155142
12. Gilon P. The role of α-cells in islet function and glucose homeostasis in health and type 2 diabetes. J Mol Biol (2020) 432:1367–94. doi: 10.1016/j.jmb.2020.01.004
13. Huising MO. Paracrine regulation of insulin secretion. Diabetologia (2020) 63:2057–63. doi: 10.1007/s00125-020-05213-5
14. Holst JJ, Gasbjerg LS, Rosenkilde MM. The role of incretins on insulin function and glucose homeostasis. Endocrinology (2021) 162:bqab065. doi: 10.1210/endocr/bqab065
15. Gerst F, Wagner R, Oquendo MB, Siegel-Axel D, Fritsche A, Heni M, et al. What role do fat cells play in pancreatic tissue? Mol Metab (2019) 25:1–10. doi: 10.1016/j.molmet.2019.05.001
16. Osinski C, Moret D, Clément K, Serradas P, Ribeiro A. Enteroendocrine system and gut barrier in metabolic disorders. Int J Mol Sci (2022) 23:3732. doi: 10.3390/ijms23073732
17. Rosendo-Silva D, Matafome P. Gut-adipose tissue crosstalk: A bridge to novel therapeutic targets in metabolic syndrome? Obes Rev an Off J Int Assoc Study Obes (2021) 22:e13130. doi: 10.1111/obr.13130
18. Guccio N, Gribble FM, Reimann F. Glucose-dependent insulinotropic polypeptide-a postprandial hormone with unharnessed metabolic potential. Annu Rev Nutr (2022) 42:21–44. doi: 10.1146/annurev-nutr-062320-113625
19. Kamohara S, Burcelin R, Halaas JL, Friedman JM, Charron MJ. Acute stimulation of glucose metabolism in mice by leptin treatment. Nature (1997) 389:374–7. doi: 10.1038/38717
20. Zhao YF, Feng DD, Chen C. Contribution of adipocyte-derived factors to beta-cell dysfunction in diabetes. Int J Biochem Cell Biol (2006) 38:804–19. doi: 10.1016/j.biocel.2005.11.008
21. Pereira S, Cline DL, Glavas MM, Covey SD, Kieffer TJ. Tissue-specific effects of leptin on glucose and lipid metabolism. Endocrine Rev (2021) 42:1–28. doi: 10.1210/endrev/bnaa027
22. Schwartz MW, Baskin DG, Bukowski TR, Kuijper JL, Foster D, Lasser G, et al. Specificity of leptin action on elevated blood glucose levels and hypothalamic neuropeptide y gene expression in ob/ob mice. Diabetes (1996) 45:531–5. doi: 10.2337/diab.45.4.531
23. Coppari R, Bjørbæk C. Leptin revisited: its mechanism of action and potential for treating diabetes. Nat Rev Drug Discovery (2012) 11:692–708. doi: 10.1038/nrd3757
24. Berglund ED, Vianna CR, Donato J Jr., Kim MH, Chuang JC, Lee CE, et al. Direct leptin action on POMC neurons regulates glucose homeostasis and hepatic insulin sensitivity in mice. J Clin Invest (2012) 122:1000–9. doi: 10.1172/jci59816
25. Cummings BP, Bettaieb A, Graham JL, Stanhope KL, Dill R, Morton GJ, et al. Subcutaneous administration of leptin normalizes fasting plasma glucose in obese type 2 diabetic UCD-T2DM rats. Proc Natl Acad Sci United States America (2011) 108:14670–5. doi: 10.1073/pnas.1107163108
26. Mendoza-Herrera K, Florio AA, Moore M, Marrero A, Tamez M, Bhupathiraju SN, et al. The leptin system and diet: A mini review of the current evidence. Front Endocrinol (2021) 12:749050. doi: 10.3389/fendo.2021.749050
27. Wang C, Du X, Fu F, Li X, Wang Z, Zhou Y, et al. Adiponectin gene therapy prevents islet loss after transplantation. J Cell Mol Med (2022) 26:4847–58. doi: 10.1111/jcmm.17515
28. Rakatzi I, Mueller H, Ritzeler O, Tennagels N, Eckel J. Adiponectin counteracts cytokine- and fatty acid-induced apoptosis in the pancreatic beta-cell line INS-1. Diabetologia (2004) 47:249–58. doi: 10.1007/s00125-003-1293-3
29. Holland WL, Miller RA, Wang ZV, Sun K, Barth BM, Bui HH, et al. Receptor-mediated activation of ceramidase activity initiates the pleiotropic actions of adiponectin. Nat Med (2011) 17:55–63. doi: 10.1038/nm.2277
30. Yanai H, Yoshida H. Beneficial effects of adiponectin on glucose and lipid metabolism and atherosclerotic progression: Mechanisms and perspectives. Int J Mol Sci (2019) 20:1190. doi: 10.3390/ijms20051190
31. Marques-Oliveira GH, Silva TM, Lima WG, Valadares HMS, Chaves VE. Insulin as a hormone regulator of the synthesis and release of leptin by white adipose tissue. Peptides (2018) 106:49–58. doi: 10.1016/j.peptides.2018.06.007
32. Li L, Miao Z, Liu R, Yang M, Liu H, Yang G. Liraglutide prevents hypoadiponectinemia-induced insulin resistance and alterations of gene expression involved in glucose and lipid metabolism. Mol Med (Cambridge Mass.) (2011) 17:1168–78. doi: 10.2119/molmed.2011.00051
33. Wang A, Li T, An P, Yan W, Zheng H, Wang B, et al. Exendin-4 upregulates adiponectin level in adipocytes via Sirt1/Foxo-1 signaling pathway. PloS One (2017) 12:e0169469. doi: 10.1371/journal.pone.0169469
34. Naitoh R, Miyawaki K, Harada N, Mizunoya W, Toyoda K, Fushiki T, et al. Inhibition of GIP signaling modulates adiponectin levels under high-fat diet in mice. Biochem Biophys Res Commun (2008) 376:21–5. doi: 10.1016/j.bbrc.2008.08.052
35. Huda MS, Wilding JP, Pinkney JH. Gut peptides and the regulation of appetite. Obes Rev an Off J Int Assoc Study Obes (2006) 7:163–82. doi: 10.1111/j.1467-789X.2006.00245.x
36. Warnotte C, Gilon P, Nenquin M, Henquin JC. Mechanisms of the stimulation of insulin release by saturated fatty acids. A study palmitate effects Mouse beta-cells. Diabetes (1994) 43:703–11. doi: 10.2337/diab.43.5.703
37. Haber EP, Procópio J, Carvalho CR, Carpinelli AR, Newsholme P, Curi R. New insights into fatty acid modulation of pancreatic beta-cell function. Int Rev cytology (2006) 248:1–41. doi: 10.1016/s0074-7696(06)48001-3
38. Dimitriadis GD, Maratou E, Kountouri A, Board M, Lambadiari V. Regulation of postabsorptive and postprandial glucose metabolism by insulin-dependent and insulin-independent mechanisms: An integrative approach. Nutrients (2021) 13:159. doi: 10.3390/nu13010159
39. Mason TM, Goh T, Tchipashvili V, Sandhu H, Gupta N, Lewis GF, et al. Prolonged elevation of plasma free fatty acids desensitizes the insulin secretory response to glucose in vivo in rats. Diabetes (1999) 48:524–30. doi: 10.2337/diabetes.48.3.524
40. Unger RH. Lipotoxicity in the pathogenesis of obesity-dependent NIDDM. Genet Clin implications. Diabetes (1995) 44:863–70. doi: 10.2337/diab.44.8.863
41. Shimabukuro M, Zhou YT, Levi M, Unger RH. Fatty acid-induced beta cell apoptosis: a link between obesity and diabetes. Proc Natl Acad Sci United States America (1998) 95:2498–502. doi: 10.1073/pnas.95.5.2498
42. Dobbins RL, Chester MW, Stevenson BE, Daniels MB, Stein DT, McGarry JD. A fatty acid- dependent step is critically important for both glucose- and non-glucose-stimulated insulin secretion. J Clin Invest (1998) 101:2370–6. doi: 10.1172/jci1813
43. Dobbins RL, Chester MW, Daniels MB, McGarry JD, Stein DT. Circulating fatty acids are essential for efficient glucose-stimulated insulin secretion after prolonged fasting in humans. Diabetes (1998) 47:1613–8. doi: 10.2337/diabetes.47.10.1613
44. Gilman AG. G Proteins: transducers of receptor-generated signals. Annu Rev Biochem (1987) 56:615–49. doi: 10.1146/annurev.bi.56.070187.003151
45. Simonds WF. G Protein regulation of adenylate cyclase. Trends Pharmacol Sci (1999) 20:66–73. doi: 10.1016/s0165-6147(99)01307-3
46. Rhee SG. Regulation of phosphoinositide-specific phospholipase c. Annu Rev Biochem (2001) 70:281–312. doi: 10.1146/annurev.biochem.70.1.281
47. Aydin Y, Coin I. Biochemical insights into structure and function of arrestins. FEBS J (2021) 288:2529–49. doi: 10.1111/febs.15811
48. Kim K, Han Y, Duan L, Chung KY. Scaffolding of mitogen-activated protein kinase signaling by β-arrestins. Int J Mol Sci (2022) 23:1000. doi: 10.3390/ijms23021000
49. Itoh Y, Kawamata Y, Harada M, Kobayashi M, Fujii R, Fukusumi S, et al. Free fatty acids regulate insulin secretion from pancreatic beta cells through GPR40. Nature (2003) 422:173–6. doi: 10.1038/nature01478
50. Kebede M, Alquier T, Latour MG, Semache M, Tremblay C, Poitout V. The fatty acid receptor GPR40 plays a role in insulin secretion in vivo after high-fat feeding. Diabetes (2008) 57:2432–7. doi: 10.2337/db08-0553
51. Fujiwara K, Maekawa F, Yada T. Oleic acid interacts with GPR40 to induce Ca2+ signaling in rat islet beta-cells: mediation by PLC and l-type Ca2+ channel and link to insulin release. Am J Physiol Endocrinol Metab (2005) 289:E670–677. doi: 10.1152/ajpendo.00035.2005
52. Shapiro H, Shachar S, Sekler I, Hershfinkel M, Walker MD. Role of GPR40 in fatty acid action on the beta cell line INS-1E. Biochem Biophys Res Commun (2005) 335:97–104. doi: 10.1016/j.bbrc.2005.07.042
53. Schnell S, Schaefer M, Schöfl C. Free fatty acids increase cytosolic free calcium and stimulate insulin secretion from beta-cells through activation of GPR40. Mol Cell Endocrinol (2007) 263:173–80. doi: 10.1016/j.mce.2006.09.013
54. Yamada H, Yoshida M, Ito K, Dezaki K, Yada T, Ishikawa SE, et al. Potentiation of glucose-stimulated insulin secretion by the GPR40-PLC-TRPC pathway in pancreatic β-cells. Sci Rep (2016) 6:25912. doi: 10.1038/srep25912
55. Feng DD, Luo Z, Roh SG, Hernandez M, Tawadros N, Keating DJ, et al. Reduction in voltage-gated k+ currents in primary cultured rat pancreatic beta-cells by linoleic acids. Endocrinology (2006) 147:674–82. doi: 10.1210/en.2005-0225
56. Sakuma K, Yabuki C, Maruyama M, Abiru A, Komatsu H, Negoro N, et al. Fasiglifam (TAK-875) has dual potentiating mechanisms via gαq-GPR40/FFAR1 signaling branches on glucose-dependent insulin secretion. Pharmacol Res Perspect (2016) 4:e00237. doi: 10.1002/prp2.237
57. Vakilian M, Tahamtani Y, Ghaedi K. A review on insulin trafficking and exocytosis. Gene (2019) 706:52–61. doi: 10.1016/j.gene.2019.04.063
58. Röder PV, Wong X, Hong W, Han W. Molecular regulation of insulin granule biogenesis and exocytosis. Biochem J (2016) 473:2737–56. doi: 10.1042/bcj20160291
59. Zhao YF, Wang L, Zha D, Qiao L, Lu L, Yu J, et al. GW9508 inhibits insulin secretion by activating ATP-sensitive potassium channels in rat pancreatic β-cells. J Mol Endocrinol (2013) 51:69–77. doi: 10.1530/jme-13-0019
60. Zhao YF, Pei J, Chen C. Activation of ATP-sensitive potassium channels in rat pancreatic beta-cells by linoleic acid through both intracellular metabolites and membrane receptor signalling pathway. J Endocrinol (2008) 198:533–40. doi: 10.1677/joe-08-0105
61. Ueno H, Ito R, Abe SI, Ookawara M, Miyashita H, Ogino H, et al. SCO-267, a GPR40 full agonist, improves glycemic and body weight control in rat models of diabetes and obesity. J Pharmacol Exp Ther (2019) 370:172–81. doi: 10.1124/jpet.118.255885
62. Kristinsson H, Sargsyan E, Manell H, Smith DM, Göpel SO, Bergsten P. Basal hypersecretion of glucagon and insulin from palmitate-exposed human islets depends on FFAR1 but not decreased somatostatin secretion. Sci Rep (2017) 7:4657. doi: 10.1038/s41598-017-04730-5
63. Flodgren E, Olde B, Meidute-Abaraviciene S, Winzell MS, Ahrén B, Salehi A. GPR40 is expressed in glucagon producing cells and affects glucagon secretion. Biochem Biophys Res Commun (2007) 354:240–5. doi: 10.1016/j.bbrc.2006.12.193
64. Wang L, Zhao Y, Gui B, Fu R, Ma F, Yu J, et al. Acute stimulation of glucagon secretion by linoleic acid results from GPR40 activation and [Ca2+]i increase in pancreatic islet {alpha}-cells. J Endocrinol (2011) 210:173–9. doi: 10.1530/joe-11-0132
65. Hauge M, Vestmar MA, Husted AS, Ekberg JP, Wright MJ, Di Salvo J, et al. GPR40 (FFAR1) - combined gs and gq signaling in vitro is associated with robust incretin secretagogue action ex vivo and in vivo. Mol Metab (2015) 4:3–14. doi: 10.1016/j.molmet.2014.10.002
66. Liou AP, Lu X, Sei Y, Zhao X, Pechhold S, Carrero RJ, et al. The G-protein-coupled receptor GPR40 directly mediates long-chain fatty acid-induced secretion of cholecystokinin. Gastroenterology (2011) 140:903–12. doi: 10.1053/j.gastro.2010.10.012
67. Kato T, Harada N, Ikeguchi-Ogura E, Sankoda A, Hatoko T, Lu X, et al. Gene expression of nutrient-sensing molecules in I cells of CCK reporter male mice. J Mol Endocrinol (2021) 66:11–22. doi: 10.1530/jme-20-0134
68. Sankoda A, Harada N, Kato T, Ikeguchi E, Iwasaki K, Yamane S, et al. Free fatty acid receptors, G protein-coupled receptor 120 and G protein-coupled receptor 40, are essential for oil-induced gastric inhibitory polypeptide secretion. J Diabetes Invest (2019) 10:1430–7. doi: 10.1111/jdi.13059
69. Edfalk S, Steneberg P, Edlund H. Gpr40 is expressed in enteroendocrine cells and mediates free fatty acid stimulation of incretin secretion. Diabetes (2008) 57:2280–7. doi: 10.2337/db08-0307
70. Rossetti L, Shulman GI, Zawalich WS. Physiological role of cholecystokinin in meal-induced insulin secretion in conscious rats. studies with l 364718, a specific inhibitor of CCK-receptor binding. Diabetes (1987) 36:1212–5. doi: 10.2337/diab.36.10.1212
71. Yu Y, Fernandez ID, Meng Y, Zhao W, Groth SW. Gut hormones, adipokines, and pro- and anti-inflammatory cytokines/markers in loss of control eating: A scoping review. Appetite (2021) 166:105442. doi: 10.1016/j.appet.2021.105442
72. Egan JM, Montrose-Rafizadeh C, Wang Y, Bernier M, Roth J. Glucagon-like peptide-1(7-36) amide (GLP-1) enhances insulin-stimulated glucose metabolism in 3T3-L1 adipocytes: one of several potential extrapancreatic sites of GLP-1 action. Endocrinology (1994) 135:2070–5. doi: 10.1210/endo.135.5.7956929
73. Beiroa D, Imbernon M, Gallego R, Senra A, Herranz D, Villarroya F, et al. GLP-1 agonism stimulates brown adipose tissue thermogenesis and browning through hypothalamic AMPK. Diabetes (2014) 63:3346–58. doi: 10.2337/db14-0302
74. López M, Diéguez C, Nogueiras R. Hypothalamic GLP-1: the control of BAT thermogenesis and browning of white fat. Adipocyte (2015) 4:141–5. doi: 10.4161/21623945.2014.983752
75. Xu F, Lin B, Zheng X, Chen Z, Cao H, Xu H, et al. GLP-1 receptor agonist promotes brown remodelling in mouse white adipose tissue through SIRT1. Diabetologia (2016) 59:1059–69. doi: 10.1007/s00125-016-3896-5
76. Li H, Donelan W, Wang F, Zhang P, Yang L, Ding Y, et al. GLP-1 induces the expression of FNDC5 derivatives that execute lipolytic actions. Front Cell Dev Biol (2021) 9:777026. doi: 10.3389/fcell.2021.777026
77. Zhou JY, Poudel A, Welchko R, Mekala N, Chandramani-Shivalingappa P, Rosca MG, et al. Liraglutide improves insulin sensitivity in high fat diet induced diabetic mice through multiple pathways. Eur J Pharmacol (2019) 861:172594. doi: 10.1016/j.ejphar.2019.172594
78. Plaza A, Merino B, Cano V, Domínguez G, Pérez-Castells J, Fernández-Alfonso MS, et al. Cholecystokinin is involved in triglyceride fatty acid uptake by rat adipose tissue. J Endocrinol (2018) 236:137–50. doi: 10.1530/joe-17-0580
79. Lo CM, King A, Samuelson LC, Kindel TL, Rider T, Jandacek RJ, et al. Cholecystokinin knockout mice are resistant to high-fat diet-induced obesity. Gastroenterology (2010) 138:1997–2005. doi: 10.1053/j.gastro.2010.01.044
80. Yip RG, Boylan MO, Kieffer TJ, Wolfe MM. Functional GIP receptors are present on adipocytes. Endocrinology (1998) 139:4004–7. doi: 10.1210/endo.139.9.6288
81. Weaver RE, Donnelly D, Wabitsch M, Grant PJ, Balmforth AJ. Functional expression of glucose-dependent insulinotropic polypeptide receptors is coupled to differentiation in a human adipocyte model. Int J Obes (2005) 2008) 32:1705–11. doi: 10.1038/ijo.2008.148
82. Attoub S, Levasseur S, Buyse M, Goïot H, Laigneau JP, Moizo L, et al. Physiological role of cholecystokinin b/gastrin receptor in leptin secretion. Endocrinology (1999) 140:4406–10. doi: 10.1210/endo.140.10.7079
83. Zhou B, Dong C, Zhao B, Su X, Luo Y, Xie L, et al. (E(X)-4)(2)-Fc, an effective long-acting GLP-1 receptor agonist, reduces obesity-related inflammation by inhibiting leptin expression. Biochem Biophys Res Commun (2020) 529:562–8. doi: 10.1016/j.bbrc.2020.06.054
84. Singh A, Fernandes JRD, Chhabra G, Krishna A, Banerjee A. Liraglutide modulates adipokine expression during adipogenesis, ameliorating obesity, and polycystic ovary syndrome in mice. Endocrine (2019) 64:349–66. doi: 10.1007/s12020-019-01891-3
85. Plaza A, Merino B, Del Olmo N, Ruiz-Gayo M. The cholecystokinin receptor agonist, CCK-8, induces adiponectin production in rat white adipose tissue. Br J Pharmacol (2019) 176:2678–90. doi: 10.1111/bph.14690
86. Khoramipour K, Chamari K, Hekmatikar AA, Ziyaiyan A, Taherkhani S, Elguindy NM, et al. Adiponectin: Structure, physiological functions, role in diseases, and effects of nutrition. Nutrients (2021) 13:1180. doi: 10.3390/nu13041180
87. Awazawa M, Ueki K, Inabe K, Yamauchi T, Kubota N, Kaneko K, et al. Adiponectin enhances insulin sensitivity by increasing hepatic IRS-2 expression via a macrophage-derived IL-6-dependent pathway. Cell Metab (2011) 13:401–12. doi: 10.1016/j.cmet.2011.02.010
88. Abou-Samra M, Selvais CM, Dubuisson N, Brichard SM. Adiponectin and its mimics on skeletal muscle: Insulin sensitizers, fat burners, exercise mimickers, muscling pills … or everything together? Int J Mol Sci (2020) 21:2620. doi: 10.3390/ijms21072620
89. Miyauchi S, Hirasawa A, Iga T, Liu N, Itsubo C, Sadakane K, et al. Distribution and regulation of protein expression of the free fatty acid receptor GPR120. Naunyn-Schmiedeberg's Arch Pharmacol (2009) 379:427–34. doi: 10.1007/s00210-008-0390-8
90. Fredriksson R, Höglund PJ, Gloriam DE, Lagerström MC, Schiöth HB. Seven evolutionarily conserved human rhodopsin G protein-coupled receptors lacking close relatives. FEBS Lett (2003) 554:381–8. doi: 10.1016/s0014-5793(03)01196-7
91. Ichimura A, Hirasawa A, Poulain-Godefroy O, Bonnefond A, Hara T, Yengo L, et al. Dysfunction of lipid sensor GPR120 leads to obesity in both mouse and human. Nature (2012) 483:350–4. doi: 10.1038/nature10798
92. Sundström L, Myhre S, Sundqvist M, Ahnmark A, McCoull W, Raubo P, et al. The acute glucose lowering effect of specific GPR120 activation in mice is mainly driven by glucagon-like peptide 1. PloS One (2017) 12:e0189060. doi: 10.1371/journal.pone.0189060
93. Iwasaki K, Harada N, Sasaki K, Yamane S, Iida K, Suzuki K, et al. Free fatty acid receptor GPR120 is highly expressed in enteroendocrine K cells of the upper small intestine and has a critical role in GIP secretion after fat ingestion. Endocrinology (2015) 156:837–46. doi: 10.1210/en.2014-1653
94. Sankoda A, Harada N, Iwasaki K, Yamane S, Murata Y, Shibue K, et al. Long-chain free fatty acid receptor GPR120 mediates oil-induced GIP secretion through CCK in Male mice. Endocrinology (2017) 158:1172–80. doi: 10.1210/en.2017-00090
95. Tanaka T, Katsuma S, Adachi T, Koshimizu TA, Hirasawa A, Tsujimoto G. Free fatty acids induce cholecystokinin secretion through GPR120. Naunyn-Schmiedeberg's Arch Pharmacol (2008) 377:523–7. doi: 10.1007/s00210-007-0200-8
96. Sidhu SS, Thompson DG, Warhurst G, Case RM, Benson RS. Fatty acid-induced cholecystokinin secretion and changes in intracellular Ca2+ in two enteroendocrine cell lines, STC-1 and GLUTag. J Physiol (2000) 528 Pt 1:165–76. doi: 10.1111/j.1469-7793.2000.00165.x
97. Shah BP, Liu P, Yu T, Hansen DR, Gilbertson TA. TRPM5 is critical for linoleic acid-induced CCK secretion from the enteroendocrine cell line, STC-1. American journal of physiology. Cell Physiol (2012) 302:C210–219. doi: 10.1152/ajpcell.00209.2011
98. Murata Y, Harada N, Kishino S, Iwasaki K, Ikeguchi-Ogura E, Yamane S, et al. Medium-chain triglycerides inhibit long-chain triglyceride-induced GIP secretion through GPR120-dependent inhibition of CCK. iScience (2021) 24:102963. doi: 10.1016/j.isci.2021.102963
99. Lu X, Zhao X, Feng J, Liou AP, Anthony S, Pechhold S, et al. Postprandial inhibition of gastric ghrelin secretion by long-chain fatty acid through GPR120 in isolated gastric ghrelin cells and mice. American journal of physiology. Gastroint liver Physiol (2012) 303:G367–376. doi: 10.1152/ajpgi.00541.2011
100. Engelstoft MS, Park WM, Sakata I, Kristensen LV, Husted AS, Osborne-Lawrence S, et al. Seven transmembrane G protein-coupled receptor repertoire of gastric ghrelin cells. Mol Metab (2013) 2:376–92. doi: 10.1016/j.molmet.2013.08.006
101. Tschöp M, Smiley DL, Heiman ML. Ghrelin induces adiposity in rodents. Nature (2000) 407:908–13. doi: 10.1038/35038090
102. Wren AM, Seal LJ, Cohen MA, Brynes AE, Frost GS, Murphy KG, et al. Ghrelin enhances appetite and increases food intake in humans. J Clin Endocrinol Metab (2001) 86:5992. doi: 10.1210/jcem.86.12.8111
103. Im DS. FFA4 (GPR120) as a fatty acid sensor involved in appetite control, insulin sensitivity and inflammation regulation. Mol aspects Med (2018) 64:92–108. doi: 10.1016/j.mam.2017.09.001
104. Croze ML, Guillaume A, Ethier M, Fergusson G, Tremblay C, Campbell SA, et al. Combined deletion of free fatty-acid receptors 1 and 4 minimally impacts glucose homeostasis in mice. Endocrinology (2021) 162:bqab002. doi: 10.1210/endocr/bqab002
105. Cintra DE, Ropelle ER, Moraes JC, Pauli JR, Morari J, Souza CT, et al. Unsaturated fatty acids revert diet-induced hypothalamic inflammation in obesity. PloS One (2012) 7:e30571. doi: 10.1371/journal.pone.0030571
106. Dragano NRV, Solon C, Ramalho AF, de Moura RF, Razolli DS, Christiansen E, et al. Polyunsaturated fatty acid receptors, GPR40 and GPR120, are expressed in the hypothalamus and control energy homeostasis and inflammation. J Neuroinflamm (2017) 14:91. doi: 10.1186/s12974-017-0869-7
107. Auguste S, Fisette A, Fernandes MF, Hryhorczuk C, Poitout V, Alquier T, et al. Central agonism of GPR120 acutely inhibits food intake and food reward and chronically suppresses anxiety-like behavior in mice. Int J Neuropsychopharmacol (2016) 19:pyw014. doi: 10.1093/ijnp/pyw014
108. Al Mahri S, Malik SS, Al Ibrahim M, Haji E, Dairi G, Mohammad S. Free fatty acid receptors (FFARs) in adipose: Physiological role and therapeutic outlook. Cells (2022) 11:750. doi: 10.3390/cells11040750
109. Oh DY, Talukdar S, Bae EJ, Imamura T, Morinaga H, Fan W, et al. GPR120 is an omega-3 fatty acid receptor mediating potent anti-inflammatory and insulin-sensitizing effects. Cell (2010) 142:687–98. doi: 10.1016/j.cell.2010.07.041
110. Hilgendorf KI, Johnson CT, Mezger A, Rice SL, Norris AM, Demeter J, et al. Omega-3 fatty acids activate ciliary FFAR4 to control adipogenesis. Cell (2019) 179:1289–1305 e1221. doi: 10.1016/j.cell.2019.11.005
111. Gotoh C, Hong YH, Iga T, Hishikawa D, Suzuki Y, Song SH, et al. The regulation of adipogenesis through GPR120. Biochem Biophys Res Commun (2007) 354:591–7. doi: 10.1016/j.bbrc.2007.01.028
112. Hidalgo MA, Carretta MD, Burgos RA. Long chain fatty acids as modulators of immune cells function: Contribution of FFA1 and FFA4 receptors. Front Physiol (2021) 12:668330. doi: 10.3389/fphys.2021.668330
113. Hasan AU, Ohmori K, Hashimoto T, Kamitori K, Yamaguchi F, Noma T, et al. GPR120 in adipocytes has differential roles in the production of pro-inflammatory adipocytokines. Biochem Biophys Res Commun (2017) 486:76–82. doi: 10.1016/j.bbrc.2017.03.001
114. Paschoal VA, Walenta E, Talukdar S, Pessentheiner AR, Osborn O, Hah N, et al. Positive reinforcing mechanisms between GPR120 and PPARγ modulate insulin sensitivity. Cell Metab (2020) 31:1173–1188.e1175. doi: 10.1016/j.cmet.2020.04.020
115. Oh DY, Walenta E. Omega-3 fatty acids and FFAR4. Front Endocrinol (2014) 5:115. doi: 10.3389/fendo.2014.00115
116. Quesada-López T, Cereijo R, Turatsinze JV, Planavila A, Cairó M, Gavaldà-Navarro A, et al. The lipid sensor GPR120 promotes brown fat activation and FGF21 release from adipocytes. Nat Commun (2016) 7:13479. doi: 10.1038/ncomms13479
117. Quesada-López T, Gavaldà-Navarro A, Morón-Ros S, Campderrós L, Iglesias R, Giralt M, et al. GPR120 controls neonatal brown adipose tissue thermogenic induction. Am J Physiol Endocrinol Metab (2019) 317:E742–50. doi: 10.1152/ajpendo.00081.2019
118. Schilperoort M, van Dam AD, Hoeke G, Shabalina IG, Okolo A, Hanyaloglu AC, et al. The GPR120 agonist TUG-891 promotes metabolic health by stimulating mitochondrial respiration in brown fat. EMBO Mol Med (2018) 10:e8047. doi: 10.15252/emmm.201708047
119. Du YQ, Sha XY, Cheng J, Wang J, Lin JY, An WT, et al. Endogenous lipid-GPR120 signaling modulates pancreatic islet homeostasis to different extents. Diabetes (2022) 71:1454–71. doi: 10.2337/db21-0794
120. Egerod KL, Engelstoft MS, Lund ML, Grunddal KV, Zhao M, Barir-Jensen D, et al. Transcriptional and functional characterization of the G protein-coupled receptor repertoire of gastric somatostatin cells. Endocrinology (2015) 156:3909–23. doi: 10.1210/en.2015-1388
121. Stone VM, Dhayal S, Brocklehurst KJ, Lenaghan C, Sörhede Winzell M, Hammar M, et al. GPR120 (FFAR4) is preferentially expressed in pancreatic delta cells and regulates somatostatin secretion from murine islets of langerhans. Diabetologia (2014) 57:1182–91. doi: 10.1007/s00125-014-3213-0
122. Zhao YF, Li XC, Liang XY, Zhao YY, Xie R, Zhang LJ, et al. GPR120 regulates pancreatic polypeptide secretion from Male mouse islets via PLC-mediated calcium mobilization. Endocrinology (2020) 161:bqaa157. doi: 10.1210/endocr/bqaa157
123. McNelis JC, Lee YS, Mayoral R, van der Kant R, Johnson AM, Wollam J, et al. GPR43 potentiates β-cell function in obesity. Diabetes (2015) 64:3203–17. doi: 10.2337/db14-1938
124. Villa SR, Priyadarshini M, Fuller MH, Bhardwaj T, Brodsky MR, Angueira AR, et al. Loss of free fatty acid receptor 2 leads to impaired islet mass and beta cell survival. Sci Rep (2016) 6:28159. doi: 10.1038/srep28159
125. Veprik A, Laufer D, Weiss S, Rubins N, Walker MD. GPR41 modulates insulin secretion and gene expression in pancreatic β-cells and modifies metabolic homeostasis in fed and fasting states. FASEB J Off Publ Fed Am Societies Exp Biol (2016) 30:3860–9. doi: 10.1096/fj.201500030R
126. Tang C, Ahmed K, Gille A, Lu S, Gröne HJ, Tunaru S, et al. Loss of FFA2 and FFA3 increases insulin secretion and improves glucose tolerance in type 2 diabetes. Nat Med (2015) 21:173–7. doi: 10.1038/nm.3779
127. Tolhurst G, Heffron H, Lam YS, Parker HE, Habib AM, Diakogiannaki E, et al. Short-chain fatty acids stimulate glucagon-like peptide-1 secretion via the G-protein-coupled receptor FFAR2. Diabetes (2012) 61:364–71. doi: 10.2337/db11-1019
128. Park BO, Kim SH, Kong GY, Kim DH, Kwon MS, Lee SU, et al. Selective novel inverse agonists for human GPR43 augment GLP-1 secretion. Eur J Pharmacol (2016) 771:1–9. doi: 10.1016/j.ejphar.2015.12.010
129. Nøhr MK, Pedersen MH, Gille A, Egerod KL, Engelstoft MS, Husted AS, et al. GPR41/FFAR3 and GPR43/FFAR2 as cosensors for short-chain fatty acids in enteroendocrine cells vs FFAR3 in enteric neurons and FFAR2 in enteric leukocytes. Endocrinology (2013) 154:3552–64. doi: 10.1210/en.2013-1142
130. Kimura I, Inoue D, Hirano K, Tsujimoto G. The SCFA receptor GPR43 and energy metabolism. Front Endocrinol (2014) 5:85. doi: 10.3389/fendo.2014.00085
131. Hong YH, Nishimura Y, Hishikawa D, Tsuzuki H, Miyahara H, Gotoh C, et al. Acetate and propionate short chain fatty acids stimulate adipogenesis via GPCR43. Endocrinology (2005) 146:5092–9. doi: 10.1210/en.2005-0545
132. Kimura I, Ozawa K, Inoue D, Imamura T, Kimura K, Maeda T, et al. The gut microbiota suppresses insulin-mediated fat accumulation via the short-chain fatty acid receptor GPR43. Nat Commun (2013) 4:1829. doi: 10.1038/ncomms2852
133. Bjursell M, Admyre T, Göransson M, Marley AE, Smith DM, Oscarsson J, et al. Improved glucose control and reduced body fat mass in free fatty acid receptor 2-deficient mice fed a high-fat diet. American journal of physiology. Endocrinol Metab (2011) 300:E211–220. doi: 10.1152/ajpendo.00229.2010
134. Hu J, Kyrou I, Tan BK, Dimitriadis GK, Ramanjaneya M, Tripathi G, et al. Short-chain fatty acid acetate stimulates adipogenesis and mitochondrial biogenesis via GPR43 in brown adipocytes. Endocrinology (2016) 157:1881–94. doi: 10.1210/en.2015-1944
135. Dewulf EM, Ge Q, Bindels LB, Sohet FM, Cani PD, Brichard SM, et al. Evaluation of the relationship between GPR43 and adiposity in human. Nutr Metab (2013) 10:11. doi: 10.1186/1743-7075-10-11
136. Xiong Y, Miyamoto N, Shibata K, Valasek MA, Motoike T, Kedzierski RM, et al. Short-chain fatty acids stimulate leptin production in adipocytes through the G protein-coupled receptor GPR41. Proc Natl Acad Sci United States America (2004) 101:1045–50. doi: 10.1073/pnas.2637002100
137. Zaibi MS, Stocker CJ, O'Dowd J, Davies A, Bellahcene M, Cawthorne MA, et al. Roles of GPR41 and GPR43 in leptin secretory responses of murine adipocytes to short chain fatty acids. FEBS Lett (2010) 584:2381–6. doi: 10.1016/j.febslet.2010.04.027
138. Bellahcene M, O'Dowd JF, Wargent ET, Zaibi MS, Hislop DC, Ngala RA, et al. Male Mice that lack the G-protein-coupled receptor GPR41 have low energy expenditure and increased body fat content. Br J Nutr (2013) 109:1755–64. doi: 10.1017/s0007114512003923
139. Altun I, Yan X, Ussar S. Immune cell regulation of white adipose progenitor cell fate. Front Endocrinol (2022) 13:859044. doi: 10.3389/fendo.2022.859044
140. Blaszczak AM, Jalilvand A, Hsueh WA. Adipocytes, innate immunity and obesity: A mini-review. Front Immunol (2021) 12:650768. doi: 10.3389/fimmu.2021.650768
141. Huang C, Du W, Ni Y, Lan G, Shi G. The effect of short-chain fatty acids on M2 macrophages polarization in vitro and in vivo. Clin Exp Immunol (2022) 207:53–64. doi: 10.1093/cei/uxab028
142. Nakajima A, Nakatani A, Hasegawa S, Irie J, Ozawa K, Tsujimoto G, et al. The short chain fatty acid receptor GPR43 regulates inflammatory signals in adipose tissue M2-type macrophages. PloS One (2017) 12:e0179696. doi: 10.1371/journal.pone.0179696
143. Cong J, Zhou P, Zhang R. Intestinal microbiota-derived short chain fatty acids in host health and disease. Nutrients (2022) 14:1977. doi: 10.3390/nu14091977
144. Koh A, De Vadder F, Kovatcheva-Datchary P, Bäckhed F. From dietary fiber to host physiology: Short-chain fatty acids as key bacterial metabolites. Cell (2016) 165:1332–45. doi: 10.1016/j.cell.2016.05.041
145. Ackerson T, Amberg A, Atzrodt J, Arabeyre C, Defossa E, Dorau M, et al. Mechanistic investigations of the liver toxicity of the free fatty acid receptor 1 agonist fasiglifam (TAK875) and its primary metabolites. J Biochem Mol Toxicol (2019) 33:e22345. doi: 10.1002/jbt.22345
146. Ghislain J, Poitout V. Targeting lipid GPCRs to treat type 2 diabetes mellitus - progress and challenges. Nat Rev Endocrinol (2021) 17:162–75. doi: 10.1038/s41574-020-00459-w
147. Sun P, Wang T, Zhou Y, Liu H, Jiang H, Zhu W, et al. DC260126: a small-molecule antagonist of GPR40 that protects against pancreatic β-cells dysfunction in db/db mice. PloS One (2013) 8:e66744. doi: 10.1371/journal.pone.0066744
148. Wu J, Sun P, Zhang X, Liu H, Jiang H, Zhu W, et al. Inhibition of GPR40 protects MIN6 β cells from palmitate-induced ER stress and apoptosis. J Cell Biochem (2012) 113:1152–8. doi: 10.1002/jcb.23450
149. Takeuchi M, Hirasawa A, Hara T, Kimura I, Hirano T, Suzuki T, et al. FFA1-selective agonistic activity based on docking simulation using FFA1 and GPR120 homology models. Br J Pharmacol (2013) 168:1570–83. doi: 10.1111/j.1476-5381.2012.02052.x
150. McCloskey AG, Miskelly MG, Flatt PR, McKillop AM. Pharmacological potential of novel agonists for FFAR4 on islet and enteroendocrine cell function and glucose homeostasis. Eur J Pharm Sci Off J Eur Fed Pharm Sci (2020) 142:105104. doi: 10.1016/j.ejps.2019.105104
151. Namour F, Galien R, Van Kaem T, van der Aa A, Vanhoutte F, Beetens J, et al. Safety, pharmacokinetics and pharmacodynamics of GLPG0974, a potent and selective FFA2 antagonist, in healthy male subjects. Br J Clin Pharmacol (2016) 82:139–48. doi: 10.1111/bcp.12900
152. Miyamoto J, Kasubuchi M, Nakajima A, Kimura I. Anti-inflammatory and insulin-sensitizing effects of free fatty acid receptors. Handb Exp Pharmacol (2017) 236:221–31. doi: 10.1007/164_2016_47
153. Cai B, Zhang J, Zhang M, Li L, Feng W, An Z, et al. Micro-inflammation characterized by disturbed Treg/Teff balance with increasing sIL-2R in patients with type 2 diabetes. Exp Clin Endocrinol Diabetes Off journal German Soc Endocrinol [and] German Diabetes Assoc (2013) 121:214–9. doi: 10.1055/s-0033-1333687
Keywords: free fatty acid receptors, glucose metabolism, pancreatic islet cells, gastrointestinal hormones, adipose tissue
Citation: Zhao Y-F (2022) Free fatty acid receptors in the endocrine regulation of glucose metabolism: Insight from gastrointestinal-pancreatic-adipose interactions. Front. Endocrinol. 13:956277. doi: 10.3389/fendo.2022.956277
Received: 30 May 2022; Accepted: 14 September 2022;
Published: 28 September 2022.
Edited by:
Erwin Ilegems, Karolinska Institutet (KI), SwedenReviewed by:
Ken-ichiro Nakajima, National Institute for Physiological Sciences (NIPS), JapanNorio Harada, Kyoto University, Japan
Copyright © 2022 Zhao. This is an open-access article distributed under the terms of the Creative Commons Attribution License (CC BY). The use, distribution or reproduction in other forums is permitted, provided the original author(s) and the copyright owner(s) are credited and that the original publication in this journal is cited, in accordance with accepted academic practice. No use, distribution or reproduction is permitted which does not comply with these terms.
*Correspondence: Yu-Feng Zhao, eXVmZW5nLnpoYW9AeGl5aS5lZHUuY24=
†ORCID: Yu-Feng Zhao, orcid.org/0000-0002-5296-3568