- 1Endocrine Hypertension Research Centre, University of Queensland Diamantina Institute, Greenslopes and Princess Alexandra Hospitals, Brisbane, QLD, Australia
- 2Department of Nephrology, Royal Brisbane and Women’s Hospital, Brisbane, QLD, Australia
- 3Department of Biomedicine, Aarhus University, Aarhus, Denmark
The thiazide-sensitive sodium chloride cotransporter (NCC), expressed in the renal distal convoluted tubule, plays a major role in Na+, Cl- and K+ homeostasis and blood pressure as exemplified by the symptoms of patients with non-functional NCC and Gitelman syndrome. NCC activity is modulated by a variety of hormones, but is also influenced by the extracellular K+ concentration. The putative “renal-K+ switch” mechanism is a relatively cohesive model that links dietary K+ intake to NCC activity, and may offer new targets for blood pressure control. However, a remaining hurdle for full acceptance of this model is the lack of human data to confirm molecular findings from animal models. Extracellular vesicles (EVs) have attracted attention from the scientific community due to their potential roles in intercellular communication, disease pathogenesis, drug delivery and as possible reservoirs of biomarkers. Urinary EVs (uEVs) are an excellent sample source for the study of physiology and pathology of renal, urothelial and prostate tissues, but the diverse origins of uEVs and their dynamic molecular composition present both methodological and data interpretation challenges. This review provides a brief overview of the state-of-the-art, challenges and knowledge gaps in current uEV-based analyses, with a focus on the application of uEVs to study the “renal-K+ switch” and NCC regulation. We also provide recommendations regarding biospecimen handling, processing and reporting requirements to improve experimental reproducibility and interoperability towards the realisation of the potential of uEV-derived biomarkers in hypertension and clinical practice.
Introduction
Hypertension (using the cut-off of >139/89 mmHg) affects up to 40% of adults worldwide, and is a major risk factor for stroke, coronary heart disease, heart failure, chronic kidney disease and development of severe coronavirus infection complications (1–4). Excessive dietary sodium (Na+) intake is generally accepted to play a role in the development of hypertension (5). However growing evidence now spotlights an inverse association between dietary potassium (K+) and blood pressure (BP) (6–8). A recent population-wide salt substitution initiative which replaced regular salt with a K+- enriched salt substitute in Peru reported an average reduction of 1.29 in systolic BP and 0.76 in diastolic BP, and an over 50% reduction in hypertension incidence in normotensive people (9). The K+-enriched salt substitution trial in populations with history of stroke or hypertension demonstrated to lower the rates of stroke, major cardiovascular events and death, and participants demonstrated a mean reduction of 3.34 mmHg in systolic BP after five years (10). These observations indicate that the impact of dietary K+ may be even higher than that of dietary Na+ in regulation of body fluid volume and BP maintenance.
Renal Na+ handling has a profound effect on body fluid and BP maintenance as exemplified by the use of diuretic medications to treat states of volume expansion or hypertension. The thiazide-sensitive NaCl cotransporter (NCC, encoded by SLC12A3) plays a key role in the regulation of Na+, Cl- and K+ balance and BP, as underscored by Gitelman syndrome (loss-of-function mutation in SLC12A3) resulting in normal to low blood pressure, hypokalaemia and metabolic alkalosis, combined with significant hypomagnesaemia and hypocalciuria. Activation of the renin-angiotensin-aldosterone system (RAAS) was initially thought to be a primary driver of NCC activity, but this system could not easily explain the observations that NCC activity decreased during high aldosterone states subsequent to high dietary K+ intake (11). This conundrum was recently solved when it was demonstrated that a reduction in plasma K+ (for example due to reduced dietary K+ intake or prolonged aldosterone stimulation) increased NCC activity (12, 13). This mechanism may contribute to the volume expansion and hypertension associated with conditions associated with aldosterone excess (12, 13). The effects on NCC are dependent on a variety of K+ mediated alterations in cellular signaling, which together orchestrate the putative “renal-K+ switch”, a mechanism which may offer new targets for pharmaceutical or dietary manipulation in health and disease. However, although the renal-K+ switch has been robustly tested in various animal models, a hurdle that still exists is the lack of data to confirm the existence and relevance of this mechanism in humans.
Extracellular vesicles (EVs) are small vesicles derived from their cells of origin and have been detected in blood, urine and other biofluids. Urine is a favorable specimen for biomarker discovery and is used to diagnose and monitor kidney diseases. Proteome analyses reveal that EVs isolated from urine may represent a more targeted approach for biomarker discovery than unfractionated urine (14–20). EVs originating from cells lining the urinary tract contain molecules derived from glomerular, tubular and bladder cells (21), and EVs released from renal tubules carry protein channels from different segments that are responsible for Na+ and water reabsorption under hormonal regulation (22). Therefore, analysis of urinary EVs can serve as a non-invasive novel approach to study physiological and pathophysiological states and regulation of NCC in humans.
Urinary extracellular vesicles
Urinary extracellular vesicles (uEVs) are a heterogenous group of nanosized membrane vesicles excreted by cells lining the urinary tract (21) that function as a carrier of information (e.g. proteins (23), lipids (24) and nucleic acids (25–27)) for cell-to-cell communication and intercellular exchange. The uEV proteome and transcriptome contains multiple disease-associated proteins and transcripts (18, 28), indicating that uEVs are a non-invasive source of potential molecular biomarkers to mirror molecular processes as well as physiological and pathological conditions in the kidney and other urinary tract tissues (16, 21, 22). Although uEVs are an attractive research tool, their diverse origins and dynamic molecular composition present an enormous methodological challenge. This review aims to give a brief overview of the state-of-the-art, challenges and knowledge gaps in current uEV-based analyses, with a focus on the application of uEVs to study the “renal-K+ switch” and NCC regulation. We also provide recommendations regarding biospecimen handling, processing and reporting requirements to improve experimental reproducibility and interoperability towards the realization of the potential of uEV-derived biomarkers in hypertension and clinical practice.
Biology of EVs
EVs consist of exosomes, microvesicles and apoptotic bodies (Figure 1). The biogenesis of exosomes and microvesicles both involve membrane trafficking processes. Exosomes are generated as intraluminal vesicles in the lumen of multivesicular endosomes by inward budding of the endosomal membrane during the formation and maturation of multivesicular endosomes (MVEs). Their biogenesis pathways are intermediated within the endosomal system and released outside the cells upon fusion of MVEs with the cell surface for intercellular communication (35, 36). In contrast, accumulation of calcium-dependent enzymes and changes in the polarity of membrane phospholipids (37) cause outward budding and fission of the plasma membrane and the subsequent release of microvesicles into the extracellular space (38). Apoptotic bodies are formed during apoptosis when cells undergo characteristic outward blebbing caused by breaks in the cytoskeleton (33, 34). Once released by the cell, microvesicles and exosomes exhibit overlapping size and composition, which makes it difficult to determine their origin.
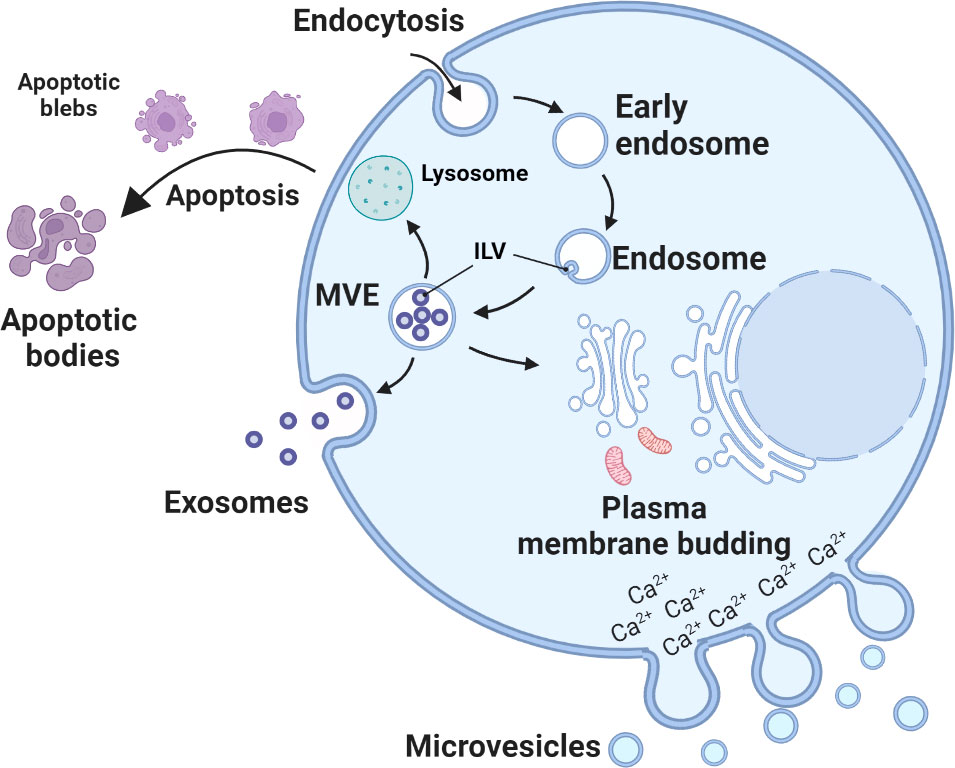
Figure 1 Biogenesis of urinary extracellular vesicles (uEVs). Exosome biogenesis starts from inward budding of the plasma membrane (endocytosis) and eventual formation of early endosomes. The membranes of early endosomes invaginate and bud into surrounding luminal space with cytoplasmic content to form intraluminal vesicles (ILVs) (29). Late endosomal structures containing ILVs are known as multivesicular endosomes (MVEs), which are eventually transported to the trans-Golgi network for endosome recycling, delivered to lysosomes for degradation of all carried material, or fuse with the plasma membrane and release exosomes outside the cell (30). Microvesicles arises through outward budding and fission of plasma membrane and is the result of dynamic interplay between phospholipid redistribution and cytoskeletal protein contraction (31, 32). Apoptotic bodies are formed during apoptosis. Apoptosis progresses through several stages, first nuclear chromatin condensation, then nuclear splitting and the frequent appearance of micronuclei, then membrane blebbing and finally splitting of the cellular content into distinct membrane-enclosed vesicles, termed apoptotic bodies (33, 34).
Origin of uEVs
uEVs originate from several parts of the urogenital tract, including the kidneys, bladder, prostate in males and utero-vaginal tract in females (Figure 2) (14, 39), and can be differentiated by characteristic proteins (14, 39, 40). However, the relative contributions of each part of the urogenital tract to the total population of uEVs has not been determined. Non-urinary tract material (proteins, microRNA etc.) or systemic EVs can also be detected in urine (41, 42), suggesting that EVs can cross the glomerular filtration barrier and basement membrane of the kidney. How this occurs is unclear, but recent studies in transgenic mice have emphasized that under physiological conditions the majority of uEVs are derived from the kidney with limited contribution of EVs from the circulation (43). In contrast, in pathophysiological states this contribution may be different. For example, during lung cancer, uEVs have been reported to contain tumour-specific proteins (44). Recent studies have demonstrated that cancer-cell derived exosomes can cause blood-brain-barrier leakiness by modulating actin dynamics of recipient endothelial cells, resulting in the breakdown of tight junctions, higher vascular permeability and metastasis (45–47). Exosome-mediated dysfunction of glomerular filtration has also been suggested, with glomerular endothelial cell-derived exosomes in a high glucose environment triggering epithelial-mesenchymal transition and dysfunction of podocytes (48). Therefore, it is likely that various pathophysiological states impair the glomerular filtration barrier allowing passage of systemic EVs into the urinary space. EVs in the urine can also be derived from residing immune cells and bacteria (49, 50). As urinary immune effectors, uEVs from healthy individuals are enriched in proteins involved in host defence and immunity with bacteriostatic/bactericidal functions, and proteins that bind to bacterial surface molecules (51).
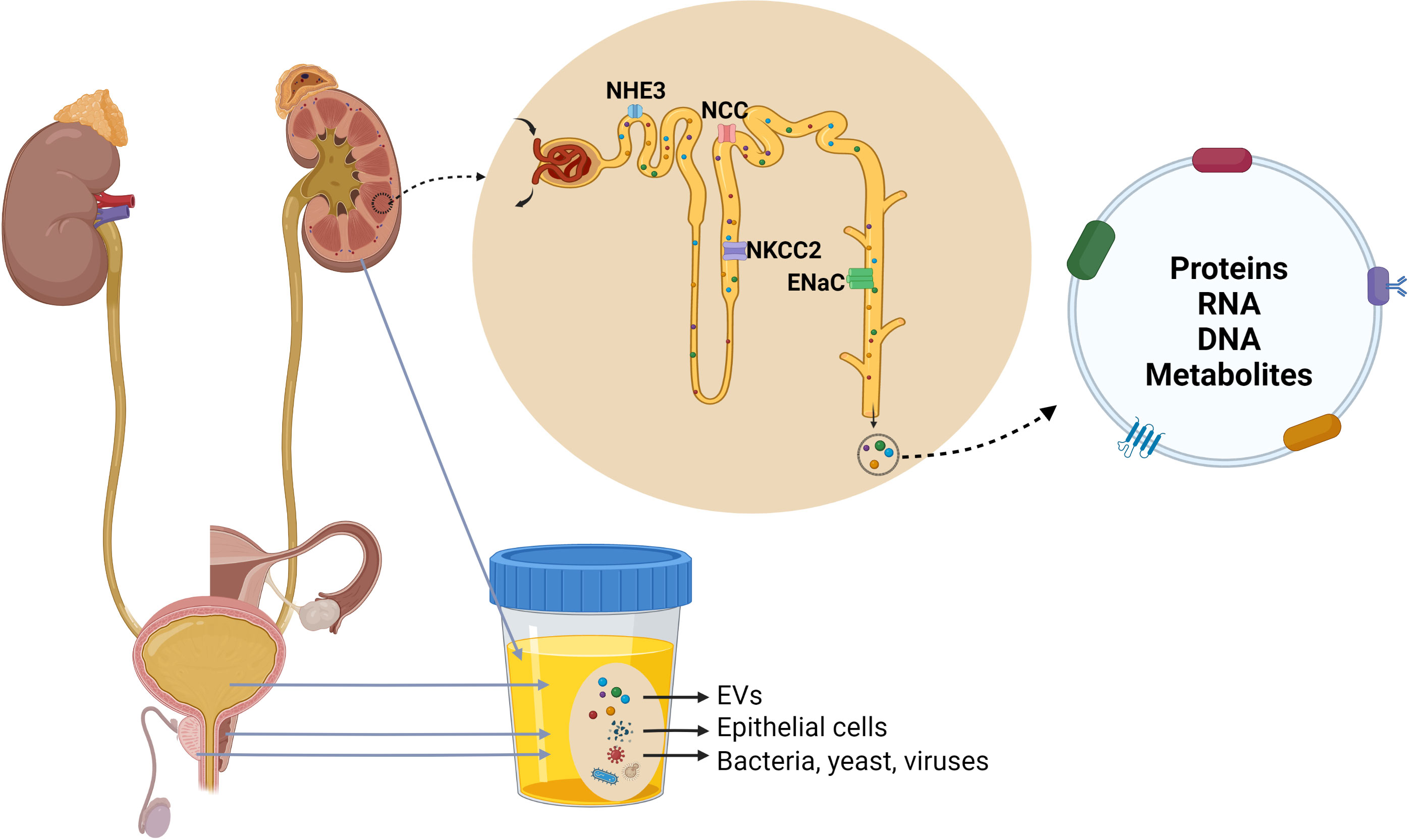
Figure 2 Origin and composition of urinary extracellular vesicles (uEVs). uEVs are generally considered to originate from several parts of the urogenital tract, including the kidneys, bladder, prostate in males and utero-vaginal tract in females. Urine can also contain a small quantity of EVs derived from other organs, epithelial cells, bacteria, yeast and viruses. uEVs carry proteins, nucleic acids, lipids and metabolites, and uEVs originating from cell lining nephron lumen contain marker proteins of nephron segments.
Molecular composition
uEVs carry proteins, nucleic acids, lipids and metabolites. However, because uEVs are a population of vesicles commonly obtained following multiple steps, the actual composition of each subtype of uEVs is unclear.
The protein composition of uEVs was originally studied in the context of the feasibility of AQP2 detection in low-density membrane vesicles isolated from human urine by ultracentrifugation (52, 53). Thereafter, several major Na+ transporters were identified in urine low density membrane fractions, such as Na/H exchanger 3(NHE3), Na-K-2Cl cotransporter 2 (NKCC2) and NCC (54). By 2011, studies using mass spectrometry techniques identified more than 1000 proteins in human uEVs (14, 39, 55, 56), including biomarkers for the renal and urogenital system in pathological conditions (57–60). Improvements in mass spectrometric techniques have expanded the number of proteins identified in uEVs to over 3000 (61, 62), enabling deeper analysis of EV biology and identification of additional biomarker candidates (63–66).
Various RNA species are present in uEVs, and include protein coding transcripts (mRNAs) and a prominent population of small non-coding RNAs. mRNAs in uEVs derive from all regions of the nephron and could facilitate the examination of kidney cellular transcriptional changes in health and disease (67). The small non-coding RNAs in uEVs include microRNAs (miRNAs), small nuclear RNAs (snRNAs), small nucleolar RNAs (snoRNAs), transfer RNAs (tRNAs) and long non-coding RNAs (lncRNAs) (68–71), which play an essential role in intracellular communication by transferring genetic information. Additionally, uEVs contain over 10,000 of the ~20,000 known protein-coding genes and ribosomal RNA (rRNA) transcripts, which is more abundant compared to EVs from other human biofluids (71, 72). Similar to kidney tissue, uEVs have an RNA integrity profile with prominent 18S and 28S rRNA peaks (67). This finding suggests that the EV structure protects the RNAs from degradation by ribonucleases during urine passage, thus increasing the potential of using uEVs as a source of reliable RNA-based biomarkers. However, the presence of 18S and 28S rRNA in uEVs is not consistent (69, 73, 74). It remains unclear whether DNA is present in the lumen of uEVs, but uEVs with and without DNase treatment showed similar trends in read distribution of nucleic acid cargo following deep sequencing (71).
Lipids and metabolites are relatively less investigated uEV components. However, in human uEVs over 100 lipid species have been detected by mass spectrometry, with cholesterol the most abundant lipid species, followed by phosphatidyl serine (75). Compared to EVs from other human biofluids, only in uEVs, all phosphatidylethanolamine species were identified as phosphatidylethanolamine-ether lipid species (75). The amount of ether lipids in EVs can be regulated, and higher levels of ether lipids in EVs have been associated with greater EV release and altered EV protein composition (76). A study that profiled metabolites in uEVs identified six main categories: organic acids and their derivatives, nucleotides, sugars and derivatives, carnitines, vitamin B/related metabolites, and amines. Among the most abundant metabolites were ornithine, D-ribose 5-phosphate, L-cystathionine, alanine and serine (77).
Current technologies for studying uEVs
The general steps for studying uEVs include pre-analytical urine handling, uEV isolation, uEV characterisation and normalisation, and downstream analyses of uEV content. Careful consideration of the applied technologies and approaches used at each step are necessary as these aspects are major source of data variability and can limit data reproducibility (16, 78–80).
Pre-analytical urine handling
Pre-analytical urine handling is a critical source of variability as preservation and storage methods have a major impact on outcomes. Important steps in urine handling include urine collection (e.g. time of voiding, use of protease inhibitors), processing and storage (e.g. immediate freezing at minus twenty or eighty degrees, short-term storage at 4°C, uEV isolation before storage), and urine handling after thawing (e.g. rapid or slow thawing, extensive vortexing). Huebner and colleagues summarized and compared currently applied methods for urine collection and storage for uEV utilization (16). Their studies concluded that the addition of protease inhibitors and preservatives such as phenylmethanesulfonyl fluoride, leupeptin, and sodium azide, long-term storage at -80°C, and extensive vortexing of urine after thawing provide the best quality uEVs for subsequent analyses (Table 1). Technical details of each step should be standardized for clinical and research purpose because data can be profoundly influenced by these pre-analytical variables.
uEV isolation
Ultracentrifugation was the first method used to reproducibly isolate uEVs (14). However, the resulting uEV pellet includes contamination with highly abundant urinary proteins such as Tamm-Horsfall protein (THP, also known as uromodulin) and albumin in pathological states. THP originates from a glycosylphosphatidylinositol-linked protein present in the apical plasma membrane of the thick ascending limb of the Henle loop, and is excreted into urine by proteolytic cleavage (85). THP forms large networks of fibre that can constrain uEVs in the urine and interfere with fractioning procedures (86). Hence, techniques to remove or eliminate THP in urine before uEV isolation are required to enhance final yield. Progressive ultracentrifugation is the most commonly used method (14). Initially, low speed centrifugation is used to remove cells and debris, followed by incubation of the resuspended low-density membrane pellet with reducing agents and subsequent repeated ultracentrifugation. This procedure denatures the zona pellucida domains in THP, thus inhibiting aggregation and allowing THP to be removed in the supernatant. Reducing agents, such as dithiothreitol (DTT) can depolymerize THP at 37°C by breaking disulfide bridges between individual THP monomers (87–90), which effectively eliminates polymeric entrapment of uEVs. However, DTT may also cause remodeling of the exosomal proteins, therefore hampering relevant functional studies and reducing the potential for using affected proteins as biomarkers (87). The detergent 3-[(3-cholamidopropyl) dimethylammonio]-1-propanesulfonic (CHAPS) has the capability of solubilising THP while at the same time preserving the conformation and the enzymatic activity of proteins contained within uEVs (88, 91), hence it can be considered as an optimised substitute of DTT if remaining physiological activity in the sample is of importance. Unfortunately, both uEV treatments with DTT and CHAPS did not completely remove THP or other abundant urinary proteins (92). Therefore modifications such as the use of double-cushioning (93), use of heavy water and a sucrose gradient in ultracentrifugation (94), or ultracentrifugation followed by size-exclusion chromatography (95) have been introduced to improve uEV purity. However, the long and laborious processing times and the requirement of expensive equipment have rendered them less attractive for clinical application.
New methodologies used for uEV isolation include EV filtration techniques, uEV precipitation, hydrostatic dialysis, acoustic trapping and immunocapture (Table 2). These methods have shown varying degrees of improved efficiency and yield, but further evaluation is required to determine optimal isolation methods for different analytical purposes (e.g., measurement of proteins, RNAs or lipids). In addition, the patient’s clinical condition should be considered in the isolation approach because, depending on the disease state involved, urine may contain various amounts of albumin, bacteria, erythrocytes, lymphocytes or other potentially interfering substances. For example, in glomerular disease, albumin and other proteins that leak into urine can bind to the surface of EVs or form a protein complex (101, 102). Therefore, isolation methods that can reduce albumin and other proteins in uEV isolates (e.g. ultracentrifugation followed by size-exclusion chromatography, the use of sucrose or other density gradients or hydrostatic filtration dialysis) are recommended (101, 103).
uEV characterisation
EV characterisation is not straightforward, because non-EV entities, such as argonaut 2 protein complex and lipoproteins also contain components that are present in EVs (104, 105), and the commonly used protocols and commercial kits that claim high quality EV or exosome isolation or purification cannot fully separate EVs from non-EV entities (106). In addition, variability in experimental systems, investigator experience and the instrumentation used contribute to the heterogeneity of protocols and composition of recovered EVs, leading to difficulties in interpretation of results. The International Society for Extracellular Vesicles has recently provided updated criteria to guide researchers in EV characterisation for the purposes of single EV characterisation, quantification, and global characterisation (107). Detailed requirements of EV characterisation are summarised in Table 3.
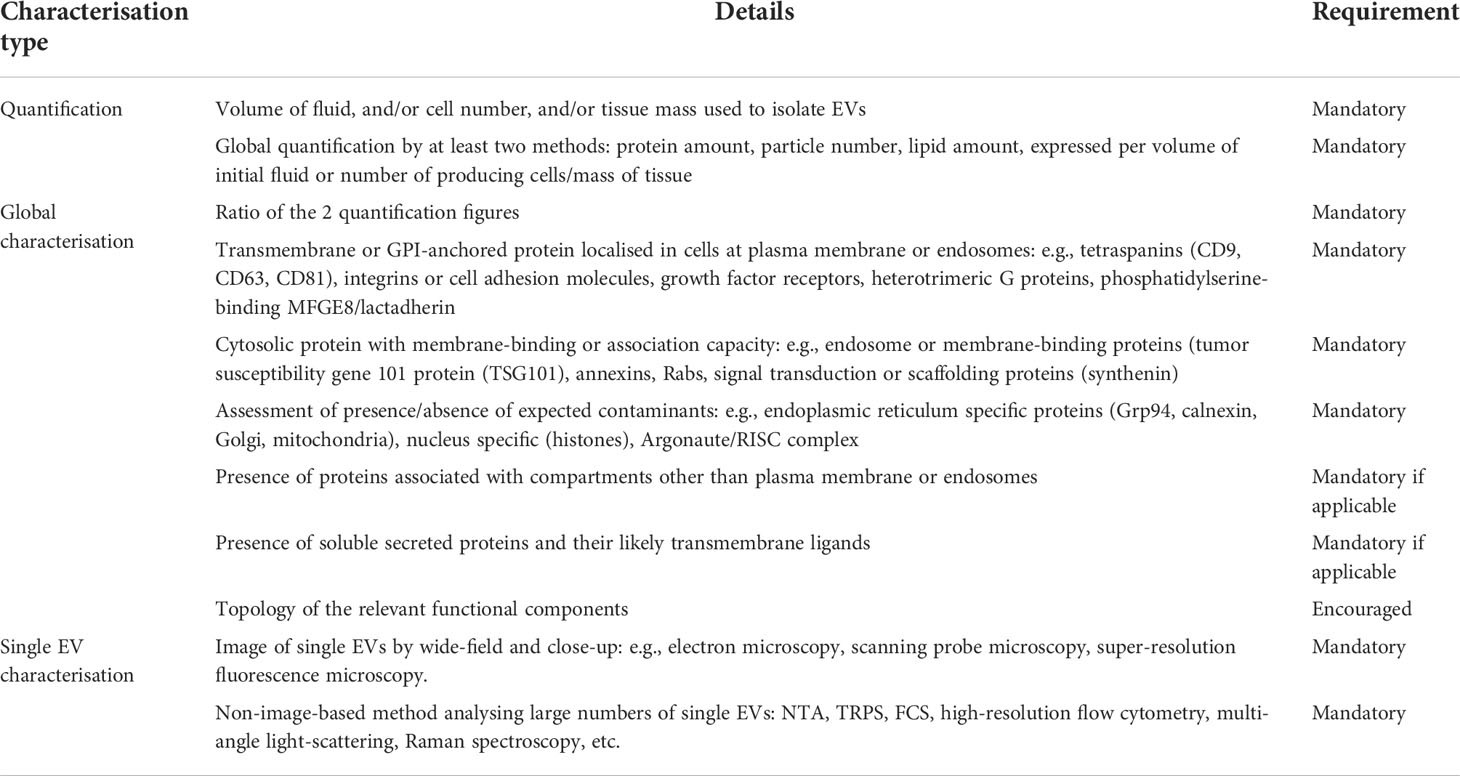
Table 3 EV characterisation methods (107).
Unfortunately, there is no single technique that can fulfill the requirements for describing EV morphology, size, number and content. Current techniques used for EV morphological characterisation include transmission electron microscopy (TEM), cryogenic electron microscopy (cryo-EM), atomic force microscopy (AFM) and super resolution fluorescence microscopy, among which TEM is the most commonly used. Because these techniques provide different information about EV structure and size distribution, they are not necessarily interchangeable or capable of providing images of comparable quality. Techniques used to measure uEV size distribution and count include nanoparticle tracking analysis (NTA) and tunable resistive pulse sensing (TRPS). On the basis of Brownian motion, NTA provides uEV particle size distribution and concentration within a specific detection range, however it cannot exclude non-EV entities and therefore NTA-based particle count may be overestimated. TRPS is a relatively more accurate method that measures particle size, number and surface charge (90). Complementing these approaches, assessment of the presence of EV-enriched/specific molecules (e.g. EV housekeeping genes/proteins) and absence of potential contaminants by standard methodologies are often utilised to describe EV content for the purpose of global characterisation. For example, uEV protein content can be measured by bulk analysis (western blotting, ELISA) or high-throughput flow cytometry for single EV surface protein analysis. Techniques currently used for uEV characterisation are listed in Table 4.
Normalisation
One of the greatest challenges in uEV-based research is the requirement for normalisation of uEV contents between samples or subjects, especially in clinical studies where spot urine is the most frequently used and simplest collection method. Current normalisation approaches, each with both benefits and limitations, include comparing uEV contents to THP abundance, urine creatinine concentration, known EV-enriched proteins, uEV concentration or uEV numbers. THP abundance highly correlates with EV markers such as ALG-2-interacting protein X (ALIX) and Tumor Susceptibility Gene 101 Protein (TSG101) (87), but the association between THP aggregation (polymerization) and the ionic strength and pH of urine needs to be taken into account (119). In addition, THP is less suitable for normalisation when DTT or other reducing reagents are used during uEV isolation.
Urine creatinine is widely used to normalize analytes (e.g. urine protein-to-creatinine ratio) in spot urine samples in routine clinical practice (120), but its use in uEV normalisation is less common (121–126). A recent study quantifying urine particle in dilute and concentrated urines randomly obtained from healthy volunteers and subjects with kidney disease demonstrated a strong correlation between uEV concentration and urine creatinine (127), indicating urine creatinine can potentially be used to normalize spot urines. However, normalisation for creatinine concentration in spot urines does not address the influence of variable uEV recovery/sedimentation rates during progressive ultracentrifugation or other isolation approaches (128).
The abundance of EV-enriched proteins and uEV concentration are considered relatively stable (129). As such, EV-enriched proteins such as tetraspanins surface markers (CD9, CD63) and proteins of exosomal biogenesis (ALIX, TSG101) are commonly used to characterize uEVs and for normalisation/correction of protein abundances in uEVs. However, some proteins may not be generally applicable for all urine samples. Blijdorp et al. reported that the commonly used uEV markers CD9 and CD63 are differentially expressed throughout the urogenital system, and therefore use of CD9 or CD63 as a normalizer can affect final outcomes (127). Importantly, for unbiased uEV characterisation methods using mass spectrometry or real-time quantitative polymerase chain reaction, no reliable normalisation or housekeeping target is currently available.
The uEV concentration depends not only on the isolation method used, but also the uEV production and excretion rate (secretion minus possible uptake) and the overall urine concentration at the sampling time point. uEV excretion has showed a circadian pattern that is independent of sex, fed-fasting/hydration status and kidney injury, and this circadian variation can be normalised by uEV particle number and its marker protein TSG101 (130). A more recent study demonstrated nephron mass determines uEV excretion rate, but nephrectomy reduces uEV excretion less than expected based on nephron loss due to compensatory hypertrophy (131). The findings imply that proxies for nephron mass including kidney function (e.g., eGFR and creatinine clearance), total kidney volume and kidney weight should also be included in uEV-based studies for inter-individual comparisons, but these indicators may not suit for studies in nephrectomy patients. The same authors furthermore identified a sex difference in uEV excretion that is likely due to greater nephron mass in men, which implies that when the excretion of a uEV biomarker is studied in males and females, the results should be corrected for uEV excretion rate to avoid false estimation of biomarker levels. Nonetheless, a validated normalisation method, or more specifically a normalisation variable, is urgently required to substitute for time in analysing the relative excretion rate of uEV proteins (87).
Reliability of uEVs in studying physiological and pathophysiological processes in kidney
Measurement of uEV protein abundances is frequently used to reflect ongoing changes in the kidney or identify biomarkers for specific diseases. For example, uEVs have been used for quantitative assessment of the levels of different proteins in the setting of patients with familial hyperkalaemic hypertension (132), Bartter syndrome (133), Gitelman syndrome (133, 134), primary aldosteronism (135–139), Cushing’s syndrome (140), polycystic kidney disease (49) and nephrogenic diabetes insipidus (123). However, matched kidney and uEV samples isolated from patients undergoing nephrectomy demonstrated no correlations in the abundance of nine proteins between the two sources (141). Although there were technical limitations, such as the correlations being based on a limited number of proteins and a significant period of time between urine and tissue collection, this study raised doubt as to whether changes in uEV protein content faithfully reflect changes within kidney tissue. In an attempt to address this concern, a recent study used large-scale proteomics to assess in an unbiased manner the correlation between protein levels in uEVs and kidney tissue from the same animal (142). The quantitative proteomic data identified an overall good positive correlation between uEV and kidney protein abundances, and furthermore provided a catalogue of uEV proteins that track the abundance of the parent protein in the kidney. Although this study supports the reliability of using uEV protein changes to monitor physiological responses and disease mechanisms, the use of a defined cohort of genetically identical animals is a limiting factor and similar large correlations in humans is still lacking. In addition, the usefulness of uEV analysis has to be taken in context of the numerous confounders that can affect results. Many factors, including glomerular filtration rate (GFR), tubular metabolism and reabsorption, dietary consumption, medical conditions, medications, gender and age, can affect an individual’s urine production and composition. In addition as described, multiple steps involved in the pre-analytical phase and isolation when studying uEVs also influence the results of subsequent downstream analyses, such as characterisation and assays to determine EV contents and function.
The putative “renal-K+ switch”
The distal tubule is critical in fine-tuning Na+ reabsorption and K+ excretion, and these processes are intimately related, as exemplified by amiloride induced hyperkalaemia and the presence of hypokalaemia in patients with thiazide induced hyponatraemia. The primary channels involved in distal tubular Na+ handling include ENaC expressed from the late distal convoluted tubule (DCT) through the connecting tubule (CNT) and the medullary collecting duct (CD), and NCC expressed only in the DCT. ENaC is thought to be the major target of aldosterone in the distal nephron. Although NCC activity was originally proposed to be increased by aldosterone, more recent studies indicate this largely occurs through low K+ induced increases in NCC phosphorylation (which activates the transporter) via activation of the Cl‐ -sensitive With-No-Lysine kinases (WNK) and SPS1-related proline/alanine-rich kinase (SPAK) network (11, 13, 143, 144) – a mechanism often referred to as the “renal-K+ switch” (Figure 3). The switch proposes that a low K+ intake acts as a trigger that links K+ and NCC regulation. The switch “turns on” NCC in response to a low dietary K+ intake, and “turns off” NCC in response to a high K+ intake, altering the amount of Na+ delivered to downstream ENaC and altering the degree of Na+/K+ exchange to modulate extracellular fluid (ECF) K+ concentrations (13, 144). Hence, although aldosterone stimulates ENaC to promote distal Na+ reabsorption and K+ excretion, the ECF K+ concentration itself regulates NCC abundance and phosphorylation. The switch pathway centers around the WNK-SPAK kinase network (11). Importantly, mutations of WNK1 and WNK4 or components of WNK regulatory ubiquitin ligase complex culin3 (CUL3) and kelch-like family member 3 (KLHL3) cause familial hyperkalaemic hypertension (FHHt, also known as Gordon’s syndrome) by increasing NCC activity. Inhibition of NCC by thiazide diuretics corrects the hypertension and hyperkalaemia in FHHt. These observations, and the use of thiazide diuretics as a BP lowering agent for hypertension, highlight the critical role of NCC in regulation of Na+ and K+ balance and BP. Unfortunately, difficulties in obtaining human kidney tissue have hampered studies of the “renal-K+ switch” model in the clinical setting. To navigate this roadblock, analysis of human uEVs has been used recently as an indirect readout to assess the roles of K+ and aldosterone in regulating NCC expression and activity.
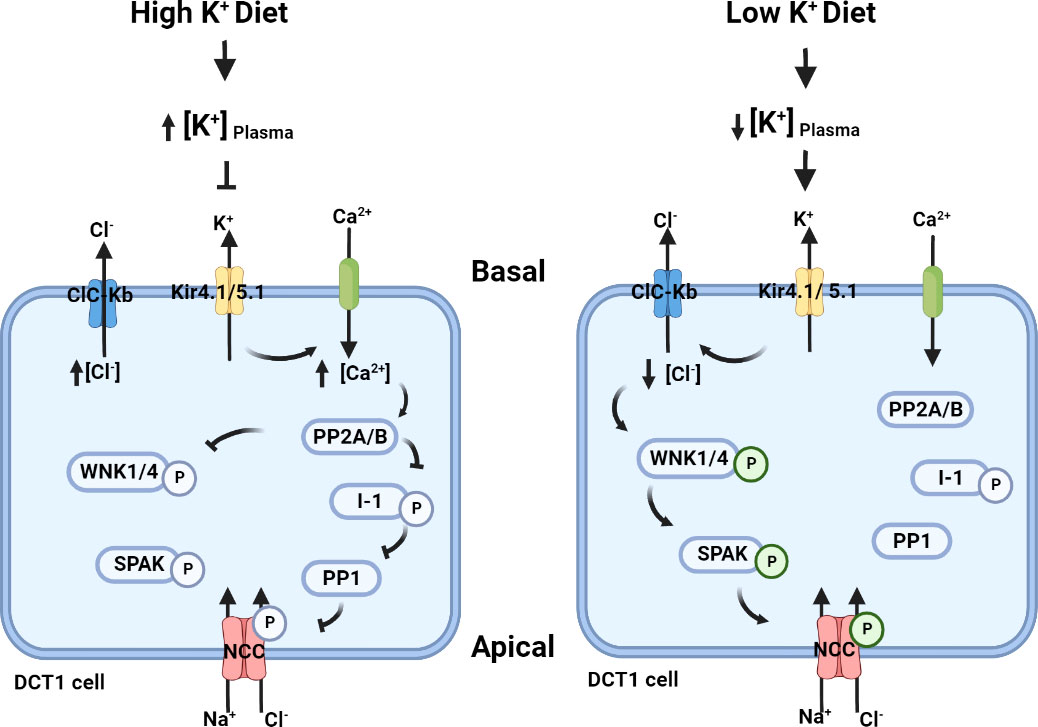
Figure 3 The putative “renal-K+ switch” mechanism. In the basolateral membrane, K+ channels Kir4.1/5.1 and a Cl- channel ClC-Kb can indirectly regulate NCC activity by modifying intracellular [Cl-] and hence the autophosphorylation of WNKs (145). High dietary K+ intake increases plasma [K+], resulting in reduced K+ extrusion by Kir4.1 and plasma membrane depolarisation of the early DCT (DCT1). This limits Cl- removal by ClC-Kb and hence intracellular Cl- mediated inhibition of the WNK-SPAK-NCC pathway remains (146–148). In addition, the effects on Kir4.1 may facilitate extracellular Ca2+ influx across the membrane via an unknown voltage-gated Ca2+ channel. This increased intracellular Ca2+ is proposed to activate protein phosphatase 2 (PP2A/B) to inhibit WNK (149, 150), and potentially PP2A/B may modulate the protein phosphatase 1 inhibitor (I-1) protein phosphatase 1 (PP1) pathway leading to NCC dephosphorylation (151–153). In contrast, a low K+ diet reduces the plasma K+ concentration. Low extracellular K+ results in cellular K+ extrusion by Kir4.1 leading to membrane hyperpolarization and release of Cl- from the cell through ClC-Kb. The subsequent reduction in intracellular Cl- relieves the inhibition of WNK4 autophosphorylation and allows the WNK-SPAK pathway to phosphorylate and activate NCC, leading to more NaCl reabsorption.
Use of uEVs for studying NCC
The presence of NCC in low-density membrane fractions from urine of normal rats first raised the possibility that analysis of urine samples may be useful in clinical settings (54). By utilising uEVs, Mayan and colleagues demonstrated a marked increase in NCC in FHHt subjects compared to controls, providing evidence of a central role of NCC in the pathophysiology of FHHt (132). The calcineurin inhibitors cyclosporine-A and tacrolimus, often used as anti-rejection drugs in transplant patients, can cause hypertension and hyperkalaemia, resembling FHHt. Calcineurin inhibitor treatment also increased NCC and phosphorylated NCC (pNCC) in human uEVs (154), concomitant with thiazides lowering the blood pressure in these patients (155). In Gitelman syndrome, NCC is absent or reduced in uEVs, consistent with the patients’ renal tissue (156). NCC in uEVs can also differentiate salt-sensitive and salt-resistant hypertensive patients (157). However, no reduction in NCC after high NaCl diet in salt-sensitive hypertensives while fractional sodium reabsorption significantly decreased, and the lack of association between changes in BP with NaCl intake and change in NCC in uEVs in the study suggests that the effect of NaCl intake on NCC may be altered renal sodium handling in salt-sensitive hypertension.
Primary aldosteronism is a common and potentially curable form of hypertension, characterized by excessive production of aldosterone by the adrenal glands that is partially or completely autonomous of the RAAS, and associated with suppressed renin activity and variable but not universal hypokalaemia. In patients with primary aldosteronism, pNCC in uEVs was higher than in patients with essential hypertension, a study which first utilized uEV pNCC as a clinical biomarker (135). Our group examined uEVs from patients with primary aldosteronism undergoing 4-day co-administration of fludrocortisone acetate and oral NaCl loading (fludrocortisone suppression testing, as a means of confirming or excluding primary aldosteronism), with variable amounts of KCl supplements to correct or prevent hypokalaemia during testing (136). There were marked increases in uEV levels of NCC and pNCC at the end of testing, providing evidence that NCC is mineralocorticoid-sensitive. However, an inverse correlation between abundances of NCC and pNCC and plasma K+ in the study suggested that the observed effect on NCC may be attributable to changes in plasma K+ rather than direct mineralocorticoid stimulation. Soon after, in another group of patients who underwent the same procedure but with a greater rise in plasma K+ due to KCl supplementation, the increase in uEV NCC was not apparent (139). This observation is consistent with recent mice studies that demonstrated that K+ supplementation reduced the degree of upregulation of NCC abundance induced by aldosterone infusion (158, 159). These observations raise the possibility that higher plasma K+ induced by KCl supplements during the testing may counterbalance mineralocorticoid-induced NCC stimulation. However, interpretation of the findings of the two uEV studies was complicated by the fact that they involved mineralocorticoid, KCl and NaCl supplementation. The effect of NaCl loading and volume expansion on the uEV NCC profile and a possible role (if any) of intravenous NaCl loading alone on NCC in patients with primary aldosteronism was recently examined (137). Despite a significant fall in plasma aldosterone in the patients, there were no changes in uEV NCC and pNCC after normalisation, suggesting aldosterone is not a major regulator of NCC abundance and phosphorylation. By contrast, the inverse association of plasma K+ with uEV NCC further highlights that ECF K+ concentration is a potent regulator of NCC.
In Cushing’s syndrome, NCC and pNCC are more abundant in uEVs of patients with a suppressed RAAS than those with non-suppressed RAAS and healthy controls (140). In this study, patients with Cushing’s syndrome and suppressed RAAS had similar blood pressure but significantly lower plasma K+ (although within the normal range) than those with non-suppressed RAAS, with inverse correlations of plasma K+ and uEV abundances of NCC and pNCC. These observations are similar to patients with primary aldosteronism, that uEV levels of pNCC are indeed a reliable readout for NCC activity and that plasma K+ may override any stimulatory effect of mineralocorticoid/aldosterone on NCC. The hypokalaemia in patients with primary aldosteronism or Cushing’s syndrome may contribute to hypertension through increased Na+ reabsorption via enhanced NCC activity. Recently, Kong et al. reported that differences in NCC and pNCC in uEVs can be used as biomarker for subtyping of primary aldosteronism and potentially for avoiding the need for adrenal venous sampling (AVS) in differentiating unilateral from bilateral forms (138). The use of uEVs as biomarkers for primary aldosteronism subtyping appears valid; however, uEVs cannot be used to avoid AVS because even if associated to unilateral forms, the side of lateralization cannot be identified. The study concluded that the observed differences were due to higher aldosterone in the unilateral group, but an effect of low K+ on NCC and pNCC may have been overlooked because they corrected hypokalaemia with KCl supplements.
Future perspectives
Use of uEVs as a tool to assess NCC abundance and activity in a variety of human studies has provided insight to the relative roles of K+ and mineralocorticoids in NCC regulation. The observations in uEVs support the notion that suppressive effects of K+ override stimulatory effects of mineralocorticoid on NCC in humans. Using uEVs to explore molecular mechanisms in humans with the intent to combine them with information from animal models and clinical assessment provides novel insights into the interactions between kidney Na and K handling, blood pressure and the RAAS. However, use of uEV NCC, pNCC or other molecules as biomarkers for diagnosis, prognosis and guidance for treatment is still methodologically challenging. Apart from the already known great differences in uEVs between patients in different gender (160, 161), age (162) and states of disease (131, 163, 164), limiting technical variations throughout the uEV analysis workflow and establishing standardised protocols are required before clinical application of uEV assessment. Moreover, the lack of robust molecular normalisation controls or normalisation controls for urine volume is still a major challenge in the field (165). Although the International Society of Extracellular Vesicles has provided recommendations for uEV research (107), new information derived from research in this rapidly growing field has evolved so that they are not universally accepted among different researchers. Nevertheless, with ongoing refinement and standardization, the study of uEVs remains a promising tool, for both research into disorders of BP regulation and possibly for incorporation into clinical practice.
Author contributions
AW drafted the paper and made the figures and tables. MJW, RAF and MS revised the paper. All authors contributed to the article and approved the submitted version.
Funding
This work is supported by a grant from the Leducq Foundation (Potassium in Hypertension Network 17CVD05).
Acknowledgments
Cartoons in Figures were created with BioRender.com.
Conflict of interest
The authors declare that the research was conducted in the absence of any commercial or financial relationships that could be construed as a potential conflict of interest.
Publisher’s note
All claims expressed in this article are solely those of the authors and do not necessarily represent those of their affiliated organizations, or those of the publisher, the editors and the reviewers. Any product that may be evaluated in this article, or claim that may be made by its manufacturer, is not guaranteed or endorsed by the publisher.
References
1. Rapsomaniki E, Timmis A, George J, Pujades-Rodriguez M, Shah AD, Denaxas S, et al. Blood pressure and incidence of twelve cardiovascular diseases: lifetime risks, healthy life-years lost, and age-specific associations in 1.25 million people. Lancet (2014) 383(9932):1899–911. doi: 10.1016/S0140-6736(14)60685-1
2. Lewington S, Prospective studies collaboration. Age-specific relevance of usual blood pressure to vascular mortality: a meta-analysis of individual data for one million adults in 61 prospective studies (vol 360, pg 1903, 2002). Lancet (2003) 361(9362):1060. doi: 10.1016/s0140-6736(02)11911-8
3. Klag MJ, Whelton PK, Randall BL, Neaton JD, Brancati FL, Ford CE, et al. Blood pressure and end-stage renal disease in men. N Engl J Med (1996) 334(1):13–8. doi: 10.1056/NEJM199601043340103
4. Hosseinzadeh R, Goharrizi MASB, Bahardoust M, Alvanegh AG, Ataee MR, Bagheri M, et al. Should all patients with hypertension be worried about developing severe coronavirus disease 2019 (COVID-19)? Clin Hypertens (2021) 27(1):3. doi: 10.1186/s40885-021-00161-7
5. Arcand J, Wong MMY, Santos JA, Leung AA, Trieu K, Thout SR, et al. More evidence that salt increases blood pressure and risk of kidney disease from the science of salt: A regularly updated systematic review of salt and health outcomes (April-July 2016). J Clin Hypertension (2017) 19(8):813–23. doi: 10.1111/jch.13049
6. Aburto NJ, Hanson S, Gutierrez H, Hooper L, Elliott P, Cappuccio FP. Effect of increased potassium intake on cardiovascular risk factors and disease: systematic review and meta-analyses. Bmj-British Med J (2013) 346:f1378. doi: 10.1136/bmj.f1378
7. Mente A, O’Donnell M, Rangarajan S, McQueen M, Dagenais G, Wielgosz A, et al. Urinary sodium excretion, blood pressure, cardiovascular disease, and mortality: a community-level prospective epidemiological cohort study. Lancet. (2018) 392(10146):496–506. doi: 10.1016/S0140-6736(18)31376-X
8. Messerli FH, Hofstetter L, Bangalore S. Salt and heart disease: a second round of “bad science”? Lancet (2018) 392(10146):456–8. doi: 10.1016/S0140-6736(18)31724-0
9. Bernabe-Ortiz A, Sal YRVG, Ponce-Lucero V, Cardenas MK, Carrillo-Larco RM, Diez-Canseco F, et al. Effect of salt substitution on community-wide blood pressure and hypertension incidence. Nat Med (2020) 26(3):374–8. doi: 10.1038/s41591-020-0754-2
10. Neal B, Wu Y, Feng X, Zhang R, Zhang Y, Shi J, et al. Effect of salt substitution on cardiovascular events and death. N Engl J Med (2021) 385(12):1067–77. doi: 10.1056/NEJMoa2105675
11. Hoorn EJ, Gritter M, Cuevas CA, Fenton RA. Regulation of the renal NaCl cotransporter and its role in potassium homeostasis. Physiol Rev (2020) 100(1):321–56. doi: 10.1152/physrev.00044.2018
12. Terker AS, Yarbrough B, Ferdaus MZ, Lazelle RA, Erspamer KJ, Meermeier NP, et al. Direct and indirect mineralocorticoid effects determine distal salt transport. J Am Soc Nephrol (2016) 27(8):2436–45. doi: 10.1681/ASN.2015070815
13. Ellison DH, Terker AS, Gamba G. Potassium and its discontents: New insight, new treatments. J Am Soc Nephrol (2016) 27(4):981–9. doi: 10.1681/ASN.2015070751
14. Pisitkun T, Shen RF, Knepper MA. Identification and proteomic profiling of exosomes in human urine. Proc Natl Acad Sci U S A. (2004) 101(36):13368–73. doi: 10.1073/pnas.0403453101
15. Alvarez ML, Khosroheidari M, Ravi RK, DiStefano JK. Comparison of protein, microRNA, and mRNA yields using different methods of urinary exosome isolation for the discovery of kidney disease biomarkers. Kidney Int (2012) 82(9):1024–32. doi: 10.1038/ki.2012.256
16. Huebner A, Somparn P, Benjachat T, Leelahavanichkul A, Avihingsanon Y, Fenton R, et al. Exosomes in urine biomarker discovery. In: Gao Y, editor. Adv Exp Med Biol. Springer Netherlands (2015). p. 43–58.
17. Karpman D, Stahl AL, Arvidsson I. Extracellular vesicles in renal disease. Nat Rev Nephrol. (2017) 13(9):545–62. doi: 10.1038/nrneph.2017.98
18. Salih M, Zietse R, Hoorn EJ. Urinary extracellular vesicles and the kidney: biomarkers and beyond. Am J Physiol Renal Physiol (2014) 306(11):F1251–9. doi: 10.1152/ajprenal.00128.2014
19. Street JM, Koritzinsky EH, Glispie DM, Star RA, Yuen PS. Urine exosomes: An emerging trove of biomarkers. Adv Clin Chem (2017) 78:103–22. doi: 10.1016/bs.acc.2016.07.003
20. Zhang W, Zhou X, Zhang H, Yao Q, Liu Y, Dong Z. Extracellular vesicles in diagnosis and therapy of kidney diseases. Am J Physiol Renal Physiol (2016) 311(5):F844–F51. doi: 10.1152/ajprenal.00429.2016
21. Spanu S, van Roeyen CR, Denecke B, Floege J, Muhlfeld AS. Urinary exosomes: a novel means to non-invasively assess changes in renal gene and protein expression. PloS One (2014) 9(10):e109631. doi: 10.1371/journal.pone.0109631
22. Barros ER, Carvajal CA. Urinary exosomes and their cargo: Potential biomarkers for mineralocorticoid arterial hypertension? Front Endocrinol (Lausanne) (2017) 8:230. doi: 10.3389/fendo.2017.00230
23. Choi DS, Kim DK, Kim YK, Gho YS. Proteomics of extracellular vesicles: Exosomes and ectosomes. Mass Spectrom Rev (2015) 34(4):474–90. doi: 10.1002/mas.21420
24. Record M, Carayon K, Poirot M, Silvente-Poirot S. Exosomes as new vesicular lipid transporters involved in cell-cell communication and various pathophysiologies. Biochim Et Biophys Acta-Molecular Cell Biol Lipids (2014) 1841(1):108–20. doi: 10.1016/j.bbalip.2013.10.004
25. Valadi H, Ekstrom K, Bossios A, Sjostrand M, Lee JJ, Lotvall JO. Exosome-mediated transfer of mRNAs and microRNAs is a novel mechanism of genetic exchange between cells. Nat Cell Biol (2007) 9(6):654–U72. doi: 10.1038/ncb1596
26. Williams C, Rodriguez-Barrueco R, Silva JM, Zhang WJ, Hearn S, Elemento O, et al. Double-stranded DNA in exosomes: a novel biomarker in cancer detection. Cell Res (2014) 24(6):766–9. doi: 10.1038/cr.2014.44
27. Ramachandran S, Palanisamy V. Horizontal transfer of RNAs: exosomes as mediators of intercellular communication. Wiley Interdiscip Rev RNA (2012) 3(2):286–93. doi: 10.1002/wrna.115
28. McKiernan J, Donovan MJ, Margolis E, Partin A, Carter B, Brown G, et al. A prospective adaptive utility trial to validate performance of a novel urine exosome gene expression assay to predict high-grade prostate cancer in patients with prostate-specific antigen 2-10ng/ml at initial biopsy. Eur Urol (2018) 74(6):731–8. doi: 10.1016/j.eururo.2018.08.019
29. McAndrews KM, Kalluri R. Mechanisms associated with biogenesis of exosomes in cancer. Mol Cancer (2019) 18(1):52. doi: 10.1186/s12943-019-0963-9
30. Williams RL, Urbe S. The emerging shape of the ESCRT machinery. Nat Rev Mol Cell Biol (2007) 8(5):355–68. doi: 10.1038/nrm2162
31. Cocucci E, Racchetti G, Meldolesi J. Shedding microvesicles: artefacts no more. Trends Cell Biol (2009) 19(2):43–51. doi: 10.1016/j.tcb.2008.11.003
32. Stein JM, Luzio JP. Ectocytosis caused by sublytic autologous complement attack on human neutrophils - the sorting of endogenous plasma-membrane proteins and lipids into shed vesicles. Biochem J (1991) 274:381–6. doi: 10.1042/bj2740381
33. Elmore S. Apoptosis: a review of programmed cell death. Toxicol Pathol (2007) 35(4):495–516. doi: 10.1080/01926230701320337
34. Povea-Cabello S, Oropesa-Avila M, de la Cruz-Ojeda P, Villanueva-Paz M, de la Mata M, Suarez-Rivero JM, et al. Dynamic reorganization of the cytoskeleton during apoptosis: The two coffins hypothesis. Int J Mol Sci (2017) 18(11):2393. doi: 10.3390/ijms18112393
35. Raposo G, Stoorvogel W. Extracellular vesicles: exosomes, microvesicles, and friends. J Cell Biol (2013) 200(4):373–83. doi: 10.1083/jcb.201211138
36. Kalluri R, LeBleu VS. The biology, function, and biomedical applications of exosomes. Science (2020) 367(6478):eaau6977. doi: 10.1126/science.aau6977
37. Malvezzi M, Chalat M, Janjusevic R, Picollo A, Terashima H, Menon AK, et al. Ca2+-dependent phospholipid scrambling by a reconstituted TMEM16 ion channel. Nat Commun (2013) 4:2367. doi: 10.1038/ncomms3367
38. Tricarico C, Clancy J, D’Souza-Schorey C. Biology and biogenesis of shed microvesicles. Small GTPases (2017) 8(4):220–32. doi: 10.1080/21541248.2016.1215283
39. Gonzales PA, Pisitkun T, Hoffert JD, Tchapyjnikov D, Star RA, Kleta R, et al. Large-Scale proteomics and phosphoproteomics of urinary exosomes. J Am Soc Nephrol (2009) 20(2):363–79. doi: 10.1681/ASN.2008040406
40. Erdbrugger U, Blijdorp CJ, Bijnsdorp IV, Borras FE, Burger D, Bussolati B, et al. Urinary extracellular vesicles: A position paper by the urine task force of the international society for extracellular vesicles. J Extracell Vesicles (2021) 10(7):e12093. doi: 10.1002/jev2.12093
41. Matthews O, Morrison EE, Tranter JD, Starkey Lewis P, Toor IS, Srivastava A, et al. Transfer of hepatocellular microRNA regulates cytochrome P450 2E1 in renal tubular cells. EBioMedicine (2020) 62:103092. doi: 10.1016/j.ebiom.2020.103092
42. Kang M, Jordan V, Blenkiron C, Chamley LW. Biodistribution of extracellular vesicles following administration into animals: A systematic review. J Extracellular Vesicles (2021) 10(8):e12085. doi: 10.1002/jev2.12085
43. Norgard MO, Steffensen LB, Hansen DR, Fuchtbauer EM, Engelund MB, Dimke H, et al. A new transgene mouse model using an extravesicular EGFP tag enables affinity isolation of cell-specific extracellular vesicles. Sci Rep-Uk (2022) 12(1):496. doi: 10.1038/s41598-021-04512-0
44. Li Y, Zhang Y, Qiu F, Qiu Z. Proteomic identification of exosomal LRG1: a potential urinary biomarker for detecting NSCLC. Electrophoresis (2011) 32(15):1976–83. doi: 10.1002/elps.201000598
45. Zhou W, Fong MY, Min Y, Somlo G, Liu L, Palomares MR, et al. Cancer-secreted miR-105 destroys vascular endothelial barriers to promote metastasis. Cancer Cell (2014) 25(4):501–15. doi: 10.1016/j.ccr.2014.03.007
46. Tominaga N, Kosaka N, Ono M, Katsuda T, Yoshioka Y, Tamura K, et al. Brain metastatic cancer cells release microRNA-181c-containing extracellular vesicles capable of destructing blood-brain barrier. Nat Commun (2015) 6:6716. doi: 10.1038/ncomms7716
47. Treps L, Edmond S, Harford-Wright E, Galan-Moya EM, Schmitt A, Azzi S, et al. Extracellular vesicle-transported Semaphorin3A promotes vascular permeability in glioblastoma. Oncogene (2016) 35(20):2615–23. doi: 10.1038/onc.2015.317
48. Wu X, Gao Y, Xu L, Dang W, Yan H, Zou D, et al. Exosomes from high glucose-treated glomerular endothelial cells trigger the epithelial-mesenchymal transition and dysfunction of podocytes. Sci Rep (2017) 7(1):9371. doi: 10.1038/s41598-017-09907-6
49. Salih M, Demmers JA, Bezstarosti K, Leonhard WN, Losekoot M, van Kooten C, et al. Proteomics of urinary vesicles links plakins and complement to polycystic kidney disease. J Am Soc Nephrol (2016) 27(10):3079–92. doi: 10.1681/ASN.2015090994
50. Li K, Sacks SH, Sheerin NS. The classical complement pathway plays a critical role in the opsonisation of uropathogenic escherichia coli. Mol Immunol (2008) 45(4):954–62. doi: 10.1016/j.molimm.2007.07.037
51. Hiemstra TF, Charles PD, Gracia T, Hester SS, Gatto L, Al-Lamki R, et al. Human urinary exosomes as innate immune effectors. J Am Soc Nephrology (2014) 25(9):2017–27. doi: 10.1681/ASN.2013101066
52. Kanno K, Sasaki S, Hirata Y, Ishikawa S, Fushimi K, Nakanishi S, et al. Urinary excretion of aquaporin-2 in patients with diabetes insipidus. N Engl J Med (1995) 332(23):1540–5. doi: 10.1056/NEJM199506083322303
53. Wen HJ, Frokiaer J, Kwon TH, Nielsen S. Urinary excretion of aquaporin-2 in rat is mediated by a vasopressin-dependent apical pathway. J Am Soc Nephrology (1999) 10(7):1416–29. doi: 10.1681/ASN.V1071416
54. McKee JA, Kumar S, Ecelbarger CA, Fernandez-Llama P, Terris J, Knepper MA. Detection of na+ transporter proteins in urine. J Am Soc Nephrology (2000) 11(11):2128–32. doi: 10.1681/ASN.V11112128
55. Moon PG, Lee JE, You S, Kim TK, Cho JH, Kim IS, et al. Proteomic analysis of urinary exosomes from patients of early IgA nephropathy and thin basement membrane nephropathy. Proteomics (2011) 11(12):2459–75. doi: 10.1002/pmic.201000443
56. Hogan MC, Manganelli L, Woollard JR, Masyuk AI, Masyuk TV, Tammachote R, et al. Characterization of PKD protein-positive exosome-like vesicles. J Am Soc Nephrol (2009) 20(2):278–88. doi: 10.1681/ASN.2008060564
57. Zhou H, Pisitkun T, Aponte A, Yuen PST, Hoffert JD, Yasuda H, et al. Exosomal fetuin-a identified by proteomics: A novel urinary biomarker for detecting acute kidney injury. Kidney Int (2006) 70(10):1847–57. doi: 10.1038/sj.ki.5001874
58. Mitchell PJ, Welton J, Staffurth J, Court J, Mason MD, Tabi Z, et al. Can urinary exosomes act as treatment response markers in prostate cancer? J Trans Med (2009) 7:4. doi: 10.1186/1479-5876-7-4
59. Zhou H, Cheruvanky A, Hu XZ, Matsumoto T, Hiramatsu N, Cho ME, et al. Urinary exosomal transcription factors, a new class of biomarkers for renal disease. Kidney Int (2008) 74(5):613–21. doi: 10.1038/ki.2008.206
60. Nilsson J, Skog J, Nordstrand A, Baranov V, Mincheva-Nilsson L, Breakefield XO, et al. Prostate cancer-derived urine exosomes: a novel approach to biomarkers for prostate cancer. Br J Cancer (2009) 100(10):1603–7. doi: 10.1038/sj.bjc.6605058
61. Wang Z, Hill S, Luther JM, Hachey DL, Schey KL. Proteomic analysis of urine exosomes by multidimensional protein identification technology (MudPIT). Proteomics (2012) 12(2):329. doi: 10.1002/pmic.201100477
62. Qi Y, Wang XJ, Rose KL, MacDonald WH, Zhang B, Schey KL, et al. Activation of the endogenous renin-Angiotensin-Aldosterone system or aldosterone administration increases urinary exosomal sodium channel excretion. J Am Soc Nephrology (2016) 27(2):646–56. doi: 10.1681/ASN.2014111137
63. Bijnsdorp IV, Maxouri O, Kardar A, Schelfhorst T, Piersma SR, Pham TV, et al. Feasibility of urinary extracellular vesicle proteome profiling using a robust and simple, clinically applicable isolation method. J Extracellular Vesicles (2017) 6(1):1313091. doi: 10.1080/20013078.2017.1313091
64. Fujita K, Kume H, Matsuzaki K, Kawashima A, Ujike T, Nagahara A, et al. Proteomic analysis of urinary extracellular vesicles from high Gleason score prostate cancer. J Urology (2017) 197(4):E1320–E. doi: 10.1016/j.juro.2017.02.3087
65. Fujita K, Kume H, Matsuzaki K, Kawashima A, Ujike T, Nagahara A, et al. Proteomic analysis of urinary extracellular vesicles from high Gleason score prostate cancer. Sci Rep-Uk (2017) 7:42961. doi: 10.1038/srep42961
66. Dhondt B, Geeurickx E, Tulkens J, Van Deun J, Vergauwen G, Lippens L, et al. Unravelling the proteomic landscape of extracellular vesicles in prostate cancer by density-based fractionation of urine. J Extracellular Vesicles (2020) 9(1):1736935. doi: 10.1080/20013078.2020.1736935
67. Miranda KC, Bond DT, McKee M, Skog J, Păunescu TG, Da Silva N, et al. Nucleic acids within urinary exosomes/microvesicles are potential biomarkers for renal disease. Kidney Int (2010) 78(2):191–9. doi: 10.1038/ki.2010.106
68. Barutta F, Tricarico M, Corbelli A, Annaratone L, Pinach S, Grimaldi S, et al. Urinary exosomal MicroRNAs in incipient diabetic nephropathy. PloS One (2013) 8(11):e73798. doi: 10.1371/journal.pone.0073798
69. Cheng L, Sun X, Scicluna BJ, Coleman BM, Hill AF. Characterization and deep sequencing analysis of exosomal and non-exosomal miRNA in human urine. Kidney Int (2014) 86(2):433–44. doi: 10.1038/ki.2013.502
70. Delic D, Eisele C, Schmid R, Baum P, Wiech F, Gerl M, et al. Urinary exosomal miRNA signature in type II diabetic nephropathy patients. PloS One (2016) 11(3):e0150154. doi: 10.1371/journal.pone.0150154
71. Miranda KC, Bond DT, Levin JZ, Adiconis X, Sivachenko A, Russ C, et al. Massively parallel sequencing of human urinary exosome/microvesicle RNA reveals a predominance of non-coding RNA. PLoS ONE (2014) 9(5):e96094. doi: 10.1371/journal.pone.0096094
72. Barreiro K, Dwivedi OP, Leparc G, Rolser M, Delic D, Forsblom C, et al. Comparison of urinary extracellular vesicle isolation methods for transcriptomic biomarker research in diabetic kidney disease. J Extracell Vesicles (2020) 10(2):e12038. doi: 10.1002/jev2.12038
73. Musante L, Tataruch D, Gu D, Benito-Martin A, Calzaferri G, Aherne S, et al. A simplified method to recover urinary vesicles for clinical applications, and sample banking. Sci Rep (2014) 4:7532. doi: 10.1038/srep07532
74. Lv LL, Cao Y, Liu D, Xu M, Liu H, Tang RN, et al. Isolation and quantification of microRNAs from urinary exosomes/microvesicles for biomarker discovery. Int J Biol Sci (2013) 9(10):1021–31. doi: 10.7150/ijbs.6100
75. Skotland T, Ekroos K, Kauhanen D, Simolin H, Seierstad T, Berge V, et al. Molecular lipid species in urinary exosomes as potential prostate cancer biomarkers. Eur J Cancer (2017) 70:122–32. doi: 10.1016/j.ejca.2016.10.011
76. Phuyal S, Skotland T, Hessvik NP, Simolin H, Overbye A, Brech A, et al. The ether lipid precursor hexadecylglycerol stimulates the release and changes the composition of exosomes derived from PC-3 cells. J Biol Chem (2015) 290(7):4225–37. doi: 10.1074/jbc.M114.593962
77. Clos-Garcia M, Loizaga-Iriarte A, Zuniga-Garcia P, Sanchez-Mosquera P, Rosa Cortazar A, Gonzalez E, et al. Metabolic alterations in urine extracellular vesicles are associated to prostate cancer pathogenesis and progression. J Extracell Vesicles. (2018) 7(1):1470442. doi: 10.1080/20013078.2018.1470442
78. Clayton A, Boilard E, Buzas EI, Cheng L, Falcon-Perez JM, Gardiner C, et al. Considerations towards a roadmap for collection, handling and storage of blood extracellular vesicles. J Extracellular Vesicles (2019) 8(1):1647027. doi: 10.1080/20013078.2019.1647027
79. Dhondt B, Van Deun J, Vermaerke S, de Marco A, Lumen N, De Wever O, et al. Urinary extracellular vesicle biomarkers in urological cancers: From discovery towards clinical implementation. Int J Biochem Cell B (2018) 99:236–56. doi: 10.1016/j.biocel.2018.04.009
80. Zhou H, Yuen PS, Pisitkun T, Gonzales PA, Yasuda H, Dear JW, et al. Collection, storage, preservation, and normalization of human urinary exosomes for biomarker discovery. Kidney Int (2006) 69(8):1471–6. doi: 10.1038/sj.ki.5000273
81. Overbye A, Skotland T, Koehler CJ, Thiede B, Seierstad T, Berge V, et al. Identification of prostate cancer biomarkers in urinary exosomes. Oncotarget (2015) 6(30):30357–76. doi: 10.18632/oncotarget.4851
82. Firsov D, Bonny O. Circadian rhythms and the kidney. Nat Rev Nephrol (2018) 14(10):626–35. doi: 10.1038/s41581-018-0048-9
83. Oosthuyzen W, Sime NEL, Ivy JR, Turtle EJ, Street JM, Pound J, et al. Quantification of human urinary exosomes by nanoparticle tracking analysis. J Physiology-London (2013) 591(23):5833–42. doi: 10.1113/jphysiol.2013.264069
84. Barreiro K, Dwivedi OP, Valkonen S, Groop PH, Tuomi T, Holthofer H, et al. Urinary extracellular vesicles: Assessment of pre-analytical variables and development of a quality control with focus on transcriptomic biomarker research. J Extracell Vesicles (2021) 10(12):e12158. doi: 10.1002/jev2.12158
85. Serafini-Cessi F, Malagolini N, Cavallone D. Tamm-horsfall glycoprotein: biology and clinical relevance. Am J Kidney Dis (2003) 42(4):658–76. doi: 10.1016/S0272-6386(03)00829-1
86. Jovine L, Qi HY, Williams Z, Litscher E, Wassarman PM. The ZP domain is a conserved module for polymerization of extracellular proteins. Nat Cell Biol (2002) 4(6):457–61. doi: 10.1038/ncb802
87. Fernandez-Llama P, Khositseth S, Gonzales PA, Star RA, Pisitkun T, Knepper MA. Tamm-horsfall protein and urinary exosome isolation. Kidney Int (2010) 77(8):736–42. doi: 10.1038/ki.2009.550
88. Musante L, Saraswat M, Duriez E, Byrne B, Ravida A, Domon B, et al. Biochemical and physical characterisation of urinary nanovesicles following CHAPS treatment. PloS One (2012) 7(7):e37279. doi: 10.1371/journal.pone.0037279
89. Puhka M, Nordberg ME, Valkonen S, Rannikko A, Kallioniemi O, Siljander P, et al. KeepEX, a simple dilution protocol for improving extracellular vesicle yields from urine. Eur J Pharm Sci (2017) 98:30–9. doi: 10.1016/j.ejps.2016.10.021
90. Musante L, Bontha SV, La Salvia S, Fernandez-Pineros A, Lannigan J, Le TH, et al. Rigorous characterization of urinary extracellular vesicles (uEVs) in the low centrifugation pellet - a neglected source for uEVs. Sci Rep (2020) 10(1):3701. doi: 10.1038/s41598-020-60619-w
91. Sun AL, Deng JT, Guan GJ, Chen SH, Liu YT, Cheng J, et al. Dipeptidyl peptidase-IV is a potential molecular biomarker in diabetic kidney disease. Diabetes Vasc Dis Res (2012) 9(4):301–8. doi: 10.1177/1479164111434318
92. Musante L, Tataruch DE, Holthofer H. Use and isolation of urinary exosomes as biomarkers for diabetic nephropathy. Front Endocrinol (2014) 5. doi: 10.3389/fendo.2014.00149
93. Raj DAA, Fiume I, Capasso G, Pocsfalvi G. A multiplex quantitative proteomics strategy for protein biomarker studies in urinary exosomes. Kidney Int (2012) 81(12):1263–72. doi: 10.1038/ki.2012.25
94. Chen CY, Hogan MC, Ward CJ. Purification of exosome-like vesicles from urine. Cilia Pt A (2013) 524:225–41. doi: 10.1016/B978-0-12-397945-2.00013-5
95. Rood IM, Deegens JKJ, Merchant ML, Tamboer WPM, Wilkey DW, Wetzels JFM, et al. Comparison of three methods for isolation of urinary microvesicles to identify biomarkers of nephrotic syndrome. Kidney Int (2010) 78(8):810–6. doi: 10.1038/ki.2010.262
96. Cheruvanky A, Zhou H, Pisitkun T, Kopp JB, Knepper MA, Yuen PS, et al. Rapid isolation of urinary exosomal biomarkers using a nanomembrane ultrafiltration concentrator. Am J Physiol-Renal (2007) 292(5):F1657–F61. doi: 10.1152/ajprenal.00434.2006
97. Merchant ML, Powell DW, Wilkey DW, Cummins TD, Deegens JK, Rood IM, et al. Microfiltration isolation of human urinary exosomes for characterization by MS. Proteomics Clin Applications (2010) 4(1):84–96. doi: 10.1002/prca.200800093
98. Ku A, Lim HC, Evander M, Lilja H, Laurell T, Scheding S, et al. Acoustic enrichment of extracellular vesicles from biological fluids. Anal Chem (2018) 90(13):8011–9. doi: 10.1021/acs.analchem.8b00914
99. Hammarstrom B, Skov NR, Olofsson K, Bruus H, Wiklund M. Acoustic trapping based on surface displacement of resonance modes. J Acoust Soc Am (2021) 149(3):1445. doi: 10.1121/10.0003600
100. Hildonen S, Skarpen E, Halvorsen TG, Reubsaet L. Isolation and mass spectrometry analysis of urinary extraexosomal proteins. Sci Rep (2016) 6:36331. doi: 10.1038/srep36331
101. Santucci L, Bruschi M, Del Zotto G, Antonini F, Ghiggeri GM, Panfoli I, et al. Biological surface properties in extracellular vesicles and their effect on cargo proteins. Sci Rep-Uk (2019) 9:13048. doi: 10.1038/s41598-019-47598-3
102. Merchant ML, Rood IM, Deegens JKJ, Klein JB. Isolation and characterization of urinary extracellular vesicles: implications for biomarker discovery. Nat Rev Nephrology (2017) 13(12):731–49. doi: 10.1038/nrneph.2017.148
103. Musante L, Tataruch D, Gu DF, Benito-Martin A, Calzaferri G, Aherne S, et al. A simplified method to recover urinary vesicles for clinical applications, and sample banking. Sci Rep-Uk (2014) 4:7532. doi: 10.1038/srep07532
104. Vickers KC, Palmisano BT, Shoucri BM, Shamburek RD, Remaley AT. MicroRNAs are transported in plasma and delivered to recipient cells by high-density lipoproteins (vol 13, pg 423, 2011). Nat Cell Biol (2015) 17(1):104. doi: 10.1038/ncb2210
105. Arroyo JD, Chevillet JR, Kroh EM, Ruf IK, Pritchard CC, Gibson DF, et al. Argonaute2 complexes carry a population of circulating microRNAs independent of vesicles in human plasma. Proc Natl Acad Sci U S A. (2011) 108(12):5003–8. doi: 10.1073/pnas.1019055108
106. Lotvall J, Hill AF, Hochberg F, Buzas EI, Di Vizio D, Gardiner C, et al. Minimal experimental requirements for definition of extracellular vesicles and their functions: a position statement from the international society for extracellular vesicles. J Extracellular Vesicles (2014) 3(1):26913. doi: 10.3402/jev.v3.26913
107. Thery C, Witwer KW, Aikawa E, Alcaraz MJ, Anderson JD, Andriantsitohaina R, et al. Minimal information for studies of extracellular vesicles 2018 (MISEV2018): A position statement of the international society for extracellular vesicles and update of the MISEV2014 guidelines. J Extracell Vesicles (2018) 7(1):1535750. doi: 10.1080/20013078.2018.1535750
108. Arraud N, Linares R, Tan S, Gounou C, Pasquet JM, Mornet S, et al. Extracellular vesicles from blood plasma: determination of their morphology, size, phenotype and concentration. J Thromb Haemost (2014) 12(5):614–27. doi: 10.1111/jth.12554
109. Hoog JL, Lotvall J. Diversity of extracellular vesicles in human ejaculates revealed by cryo-electron microscopy. J Extracell Vesicles (2015) 4:28680. doi: 10.3402/jev.v4.28680
110. Sharma S, Rasool HI, Palanisamy V, Mathisen C, Schmidt M, Wong DT, et al. Structural-mechanical characterization of nanoparticle exosomes in human saliva, using correlative AFM, FESEM, and force spectroscopy. ACS Nano (2010) 4(4):1921–6. doi: 10.1021/nn901824n
111. Saliba DG, Cespedes-Donoso PF, Balint S, Compeer EB, Korobchevskaya K, Valvo S, et al. Composition and structure of synaptic ectosomes exporting antigen receptor linked to functional CD40 ligand from helper T cells. Elife (2019) 8:e47528. doi: 10.7554/eLife.47528
112. Treps L, Perret R, Edmond S, Ricard D, Gavard J. Glioblastoma stem-like cells secrete the pro-angiogenic VEGF-a factor in extracellular vesicles. J Extracell Vesicles (2017) 6(1):1359479. doi: 10.1080/20013078.2017.1359479
113. Chen C, Zong S, Wang Z, Lu J, Zhu D, Zhang Y, et al. Imaging and intracellular tracking of cancer-derived exosomes using single-molecule localization-based super-resolution microscope. ACS Appl Mater Interfaces (2016) 8(39):25825–33. doi: 10.1021/acsami.6b09442
114. Wiklander OPB, Bostancioglu RB, Welsh JA, Zickler AM, Murke F, Corso G, et al. Systematic methodological evaluation of a multiplex bead-based flow cytometry assay for detection of extracellular vesicle surface signatures. Front Immunol (2018) 9. doi: 10.3389/fimmu.2018.01326
115. Welsh JA, van der Pol E, Arkesteijn GJA, Bremer M, Brisson A, Coumans F, et al. MIFlowCyt-EV: a framework for standardized reporting of extracellular vesicle flow cytometry experiments. J Extracellular Vesicles (2020) 9(1):1713526. doi: 10.1080/20013078.2020.1713526
116. Wu Q, Fenton RA. Urinary proteomics for kidney dysfunction: insights and trends. Expert Rev Proteomics (2021) 18(6):437–52. doi: 10.1080/14789450.2021.1950535
117. Everaert C, Helsmoortel H, Decock A, Hulstaert E, Van Paemel R, Verniers K, et al. Performance assessment of total RNA sequencing of human biofluids and extracellular vesicles. Sci Rep (2019) 9(1):17574. doi: 10.1038/s41598-019-53892-x
118. Williams C, Palviainen M, Reichardt NC, Siljander PR, Falcon-Perez JM. Metabolomics applied to the study of extracellular vesicles. Metabolites (2019) 9(11):276. doi: 10.3390/metabo9110276
119. Tamm I, Horsfall FL. A mucoprotein derived from human urine which reacts with influenza, mumps, and Newcastle disease viruses. J Exp Med (1952) 95(1):71–97. doi: 10.1084/jem.95.1.71
120. Ginsberg JM, Chang BS, Matarese RA, Garella S. Use of single voided urine samples to estimate quantitative proteinuria. N Engl J Med (1983) 309(25):1543–6. doi: 10.1056/NEJM198312223092503
121. Damkjaer M, Jensen PH, Schwammle V, Sprenger RR, Jacobsen IA, Jensen ON, et al. Selective renal vasoconstriction, exaggerated natriuresis and excretion rates of exosomic proteins in essential hypertension. Acta Physiol (Oxf) (2014) 212(1):106–18. doi: 10.1111/apha.12345
122. Esteva-Font C, Wang XY, Ars E, Guillen-Gomez E, Sans L, Saavedra IG, et al. Are sodium transporters in urinary exosomes reliable markers of tubular sodium reabsorption in hypertensive patients? Nephron Physiol (2010) 114(3):P25–34. doi: 10.1159/000274468
123. Hinrichs GR, Hansen LH, Nielsen MR, Fagerberg C, Dieperink H, Rittig S, et al. A novel mutation affecting the arginine-137 residue of AVPR2 in dizygous twins leads to nephrogenic diabetes insipidus and attenuated urine exosome aquaporin-2. Physiol Rep (2016) 4(8):e12764. doi: 10.14814/phy2.12764
124. Salih M, Fenton RA, Zietse R, Hoorn EJ. Urinary extracellular vesicles as markers to assess kidney sodium transport. Curr Opin Nephrol Hypertension (2016) 25(2):67–72. doi: 10.1097/MNH.0000000000000192
125. Coumans FA, van der Pol E, Boing AN, Hajji N, Sturk G, van Leeuwen TG, et al. Reproducible extracellular vesicle size and concentration determination with tunable resistive pulse sensing. J Extracell Vesicles (2014) 3:25922. doi: 10.3402/jev.v3.25922
126. Oosthuyzen W, Sime N, Ivy J, Turtle E, Street J, Pound J, et al. Quantification of human urinary exosomes by nanoparticle tracking analysis. Toxicol Letters (2014) 229:S247–S. doi: 10.1016/j.toxlet.2014.06.822
127. Blijdorp CJ, Tutakhel OAZ, Hartjes TA, van den Bosch TPP, van Heugten MH, Rigalli JP, et al. Comparing approaches to normalize, quantify, and characterize urinary extracellular vesicles. J Am Soc Nephrol (2021) 32(5):1210–26. doi: 10.1681/ASN.2020081142
128. Dhondt B, Geeurickx E, Tulkens J, Van Deun J, Vergauwen G, Lippens L, et al. Unravelling the proteomic landscape of extracellular vesicles in prostate cancer by density-based fractionation of urine. J Extracell Vesicles. (2020) 9(1):1736935. doi: 10.1080/20013078.2020.1736935
129. Colombo M, Moita C, van Niel G, Kowal J, Vigneron J, Benaroch P, et al. Analysis of ESCRT functions in exosome biogenesis, composition and secretion highlights the heterogeneity of extracellular vesicles. J Cell Sci (2013) 126(Pt 24):5553–65. doi: 10.1242/jcs.128868
130. Koritzinsky EH, Street JM, Chari RR, Glispie DM, Bellomo TR, Aponte AM, et al. Circadian variation in the release of small extracellular vesicles can be normalized by vesicle number or TSG101. Am J Physiol Renal Physiol (2019) 317(5):F1098–F110.0. doi: 10.1152/ajprenal.00568.2017
131. Blijdorp CJ, Hartjes TA, Wei KY, van Heugten MH, Bovee DM, Budde RPJ, et al. Nephron mass determines the excretion rate of urinary extracellular vesicles. J Extracell Vesicles (2022) 11(1):e12181. doi: 10.1002/jev2.12181
132. Mayan H, Attar-Herzberg D, Shaharabany M, Holtzman EJ, Farfel Z. Increased urinary Na-cl cotransporter protein in familial hyperkalaemia and hypertension. Nephrol Dial Transplant (2008) 23(2):492–6. doi: 10.1093/ndt/gfm641
133. Corbetta S, Raimondo F, Tedeschi S, Syrèn M-L, Rebora P, Savoia A, et al. Urinary exosomes in the diagnosis of gitelman and bartter syndromes. Nephrol Dial Transplant (2015) 30(4):621–30. doi: 10.1093/ndt/gfu362
134. Isobe K, Mori T, Asano T, Kawaguchi H, Nonoyama S, Kumagai N, et al. Development of enzyme-linked immunosorbent assays for urinary thiazide-sensitive Na-cl cotransporter measurement. Am J Physiol Renal Physiol (2013) 305(9):F1374–81. doi: 10.1152/ajprenal.00208.2013
135. van der Lubbe N, Jansen PM, Salih M, Fenton RA, van den Meiracker AH, Danser AJ, et al. The phosphorylated sodium chloride cotransporter in urinary exosomes is superior to prostasin as a marker for aldosteronism. Hypertension (2012) 60(3):741–8. doi: 10.1161/HYPERTENSIONAHA.112.198135
136. Wolley MJ, Wu A, Xu S, Gordon RD, Fenton RA, Stowasser M. In primary aldosteronism, mineralocorticoids influence exosomal sodium-chloride cotransporter abundance. J Am Soc Nephrol. (2017) 28(1):56–63. doi: 10.1681/ASN.2015111221
137. Wu A, Wolley MJ, Wu Q, Cowley D, Palmfeldt J, Welling PA, et al. Acute intravenous NaCl and volume expansion reduces NCC abundance and phosphorylation in urinary extracellular vesicles. Kidney360 (2022) 3(5):910–21. doi: 10.34067/KID.0000362022
138. Kong L, Tang X, Kang Y, Dong L, Tong J, Xu J, et al. The role of urinary extracellular vesicles sodium chloride cotransporter in subtyping primary aldosteronism. Front Endocrinol (Lausanne) (2022) 13:834409. doi: 10.3389/fendo.2022.834409
139. Wu A, Wolley MJ, Wu Q, Gordon RD, Fenton RA, Stowasser M. The cl-/HCO3- exchanger pendrin is downregulated during oral co-administration of exogenous mineralocorticoid and KCl in patients with primary aldosteronism. J Hum Hypertens (2021) 35(10):837–48. doi: 10.1038/s41371-020-00439-7
140. Salih M, Bovee DM, van der Lubbe N, Danser AHJ, Zietse R, Feelders RA, et al. Increased urinary extracellular vesicle sodium transporters in cushing syndrome with hypertension. J Clin Endocr Metab (2018) 103(7):2583–91. doi: 10.1210/jc.2018-00065
141. Sabaratnam R, Geertsen L, Skjodt K, Hojlund K, Dimke H, Lund L, et al. In human nephrectomy specimens, the kidney level of tubular transport proteins does not correlate with their abundance in urinary extracellular vesicles. Am J Physiol Renal Physiol (2019) 317(3):F560–F71. doi: 10.1152/ajprenal.00242.2019
142. Wu Q, Poulsen SB, Murali SK, Grimm PR, Su XT, Delpire E, et al. Large-Scale proteomic assessment of urinary extracellular vesicles highlights their reliability in reflecting protein changes in the kidney. J Am Soc Nephrol (2021) 32(9):2195–209. doi: 10.1681/ASN.2020071035
143. Wu A, Wolley M, Stowasser M. The interplay of renal potassium and sodium handling in blood pressure regulation: critical role of the WNK-SPAK-NCC pathway. J Hum Hypertens (2019) 33:508–23. doi: 10.1038/s41371-019-0170-6
144. Terker AS, Zhang C, Erspamer KJ, Gamba G, Yang CL, Ellison DH. Unique chloride-sensing properties of WNK4 permit the distal nephron to modulate potassium homeostasis. Kidney Int (2016) 89(1):127–34. doi: 10.1038/ki.2015.289
145. Wang MX, Cuevas CA, Su XT, Wu P, Gao ZX, Lin DH, et al. Potassium intake modulates the thiazide-sensitive sodium-chloride cotransporter (NCC) activity via the Kir4. 1 potassium channel. Kidney Int (2018) 93(4):893–902. doi: 10.1016/j.kint.2017.10.023
146. Argaiz ER, Chavez-Canales M, Ostrosky-Frid M, Rodriguez-Gama A, Vazquez N, Gonzalez-Rodriguez X, et al. Kidney-specific WNK1 isoform (KS-WNK1) is a potent activator of WNK4 and NCC. Am J Physiol Renal Physiol (2018) 315(3):F734–F45. doi: 10.1152/ajprenal.00145.2018
147. Boyd-Shiwarski CR, Shiwarski DJ, Roy A, Namboodiri HN, Nkashama LJ, Xie J, et al. Potassium-regulated distal tubule WNK bodies are kidney-specific WNK1 dependent. Mol Biol Cell (2018) 29(4):499–509. doi: 10.1091/mbc.E17-08-0529
148. Hadchouel J, Ellison DH, Gamba G. Regulation of renal electrolyte transport by WNK and SPAK-OSR1 kinases. Annu Rev Physiol (2016) 78:367–89. doi: 10.1146/annurev-physiol-021115-105431
149. Stewart AA, Ingebritsen TS, Manalan A, Klee CB, Cohen P. Discovery of a Ca2+- and calmodulin-dependent protein phosphatase: probable identity with calcineurin (CaM-BP80). FEBS Lett (1982) 137(1):80–4. doi: 10.1016/0014-5793(82)80319-0
150. Ishizawa K, Wang Q, Li J, Yamazaki O, Tamura Y, Fujigaki Y, et al. Calcineurin dephosphorylates kelch-like 3, reversing phosphorylation by angiotensin II and regulating renal electrolyte handling. Proc Natl Acad Sci U S A (2019) 116(8):3155–60. doi: 10.1073/pnas.1817281116
151. El-Armouche A, Bednorz A, Pamminger T, Ditz D, Didie M, Dobrev D, et al. Role of calcineurin and protein phosphatase-2A in the regulation of phosphatase inhibitor-1 in cardiac myocytes. Biochem Biophys Res Commun (2006) 346(3):700–6. doi: 10.1016/j.bbrc.2006.05.182
152. Picard N, Trompf K, Yang CL, Miller RL, Carrel M, Loffing-Cueni D, et al. Protein phosphatase 1 inhibitor-1 deficiency reduces phosphorylation of renal NaCl cotransporter and causes arterial hypotension. J Am Soc Nephrol (2014) 25(3):511–22. doi: 10.1681/ASN.2012121202
153. Penton D, Moser S, Wengi A, Czogalla J, Rosenbaek LL, Rigendinger F, et al. Protein phosphatase 1 inhibitor-1 mediates the cAMP-dependent stimulation of the renal NaCl cotransporter. J Am Soc Nephrol (2019) 30(5):737–50. doi: 10.1681/ASN.2018050540
154. Esteva-Font C, Guillen-Gomez E, Diaz JM, Guirado L, Facundo C, Ars E, et al. Renal sodium transporters are increased in urinary exosomes of cyclosporine-treated kidney transplant patients. Am J Nephrol (2014) 39(6):528–35. doi: 10.1159/000362905
155. Tutakhel OAZ, Moes AD, Valdez-Flores MA, Kortenoeven MLA, von den Vries M, Jelen S, et al. NaCl Cotransporter abundance in urinary vesicles is increased by calcineurin inhibitors and predicts thiazide sensitivity. PloS One (2017) 12(4):e0176220. doi: 10.1371/journal.pone.0176220
156. Joo KW, Lee JW, Jang HR, Heo NJ, Jeon US, Oh YK, et al. Reduced urinary excretion of thiazide-sensitive Na-cl cotransporter in gitelman syndrome: preliminary data. Am J Kidney Dis (2007) 50(5):765–73. doi: 10.1053/j.ajkd.2007.07.022
157. Esteva-Font C, Wang X, Ars E, Guillen-Gomez E, Sans L, Gonzalez Saavedra I, et al. Are sodium transporters in urinary exosomes reliable markers of tubular sodium reabsorption in hypertensive patients? Nephron Physiol (2010) 114(3):p25–34. doi: 10.1159/000274468
158. Xu N, Hirohama D, Ishizawa K, Chang WX, Shimosawa T, Fujita T, et al. Hypokalemia and pendrin induction by aldosterone. Hypertension (2017) 69(5):855. doi: 10.1161/HYPERTENSIONAHA.116.08519
159. Shibata S, Rinehart J, Zhang J, Moeckel G, Castaneda-Bueno M, Stiegler AL, et al. Mineralocorticoid receptor phosphorylation regulates ligand binding and renal response to volume depletion and hyperkalemia. Cell Metab (2013) 18(5):660–71. doi: 10.1016/j.cmet.2013.10.005
160. Duijvesz D, Versluis CYL, van der Fels CAM, Vredenbregt-van den Berg MS, Leivo J, Peltola MT, et al. Immuno-based detection of extracellular vesicles in urine as diagnostic marker for prostate cancer. Int J Cancer (2015) 137(12):2869–78. doi: 10.1002/ijc.29664
161. Ploussard G, de la Taille A. Urine biomarkers in prostate cancer. Nat Rev Urol. (2010) 7(2):101–9. doi: 10.1038/nrurol.2009.261
162. Turco AE, Lam W, Rule AD, Denic A, Lieske JC, Miller VM, et al. Specific renal parenchymal-derived urinary extracellular vesicles identify age-associated structural changes in living donor kidneys. J Extracell Vesicles (2016) 5:29642. doi: 10.3402/jev.v5.29642
163. Puelles VG, Hoy WE, Hughson MD, Diouf B, Douglas-Denton RN, Bertram JF. Glomerular number and size variability and risk for kidney disease. Curr Opin Nephrol Hypertens (2011) 20(1):7–15. doi: 10.1097/MNH.0b013e3283410a7d
164. Bertram JF, Douglas-Denton RN, Diouf B, Hughson MD, Hoy WE. Human nephron number: implications for health and disease. Pediatr Nephrol (2011) 26(9):1529–33. doi: 10.1007/s00467-011-1843-8
Keywords: urinary extracellular vesicles, sodium chloride cotransporter, potassium, aldosterone, hypertension, primary aldosteronism
Citation: Wu A, Wolley MJ, Fenton RA and Stowasser M (2022) Using human urinary extracellular vesicles to study physiological and pathophysiological states and regulation of the sodium chloride cotransporter . Front. Endocrinol. 13:981317. doi: 10.3389/fendo.2022.981317
Received: 29 June 2022; Accepted: 09 August 2022;
Published: 29 August 2022.
Edited by:
Cristian A. Carvajal, Pontificia Universidad Católica de Chile, ChileReviewed by:
Jacopo Burrello, University of Turin, ItalyFrancesca Raimondo, University of Milano Bicocca, Italy
Copyright © 2022 Wu, Wolley, Fenton and Stowasser. This is an open-access article distributed under the terms of the Creative Commons Attribution License (CC BY). The use, distribution or reproduction in other forums is permitted, provided the original author(s) and the copyright owner(s) are credited and that the original publication in this journal is cited, in accordance with accepted academic practice. No use, distribution or reproduction is permitted which does not comply with these terms.
*Correspondence: Michael Stowasser, bS5zdG93YXNzZXJAdXEuZWR1LmF1