- 1Institute for Bioengineering, School of Engineering, The University of Edinburgh, Edinburgh, United Kingdom
- 2Centre for Regenerative Medicine, Institute for Regeneration and Repair, The University of Edinburgh, Edinburgh, United Kingdom
- 3Department of ENT, Head and Neck Surgery, NHS Lothian, Edinburgh, United Kingdom
Primary hypothyroidism severely impacts the quality of life of patients through a decrease in the production of the thyroid hormones T3 and T4, leading to symptoms affecting cardiovascular, neurological, cognitive, and metabolic function. The incidence rate of primary hypothyroidism is expected to increase in the near future, partially due to increasing survival of patients that have undergone radiotherapy for head and neck cancer, which induces this disease in over half of those treated. The current standard of care encompasses thyroid hormone replacement therapy, traditionally in the form of synthetic T4. However, there is mounting evidence that this is unable to restore thyroid hormone signaling in all tissues due to often persistent symptoms. Additional complications are also present in the form of dosage difficulties, extensive drug interactions and poor patience compliance. The alternative therapeutic approach employed in the past is combination therapy, which consists of administration of both T3 and T4, either synthetic or in the form of desiccated thyroid extract. Here, issues are present regarding the lack of regulation concerning formulation and lack of data regarding safety and efficacy of these treatment methods. Tissue engineering and regenerative medicine have been applied in conjunction with each other to restore function of various tissues. Recently, these techniques have been adapted for thyroid tissue, primarily through the fabrication of regenerative scaffolds. Those currently under investigation are composed of either biopolymers or native decellularized extracellular matrix (dECM) in conjunction with either primary thyrocytes or stem cells which have undergone directed thyroid differentiation. Multiple of these scaffolds have successfully restored an athyroid phenotype in vivo. However, further work is needed until clinical translation can be achieved. This is proposed in the form of exploration and combination of materials used to fabricate these scaffolds, the addition of peptides which can aid restoration of tissue homeostasis and additional in vivo experimentation providing data on safety and efficacy of these implants.
1 Introduction
Hypothyroidism, caused by the underproduction of thyroid hormone, is a debilitating condition which severely impacts quality of life (1). It presents with a wide range of symptoms, displayed in Table 1, most commonly including depression, fatigue, weight gain, dry skin, and bradycardia, amongst others (16, 36–39). Untreated, hypothyroidism can result in a life-threatening myxedema coma (40). The prevalence of hypothyroidism is thought to be around 9.3% in women and 1.3% in men, but it is related to iodine intake and therefore varies widely in between countries (41). Additionally, the incidence of primary hypothyroidism has been suggested to increase with age; and specifically, a Danish study has indicated this increase to be around eightfold (42).
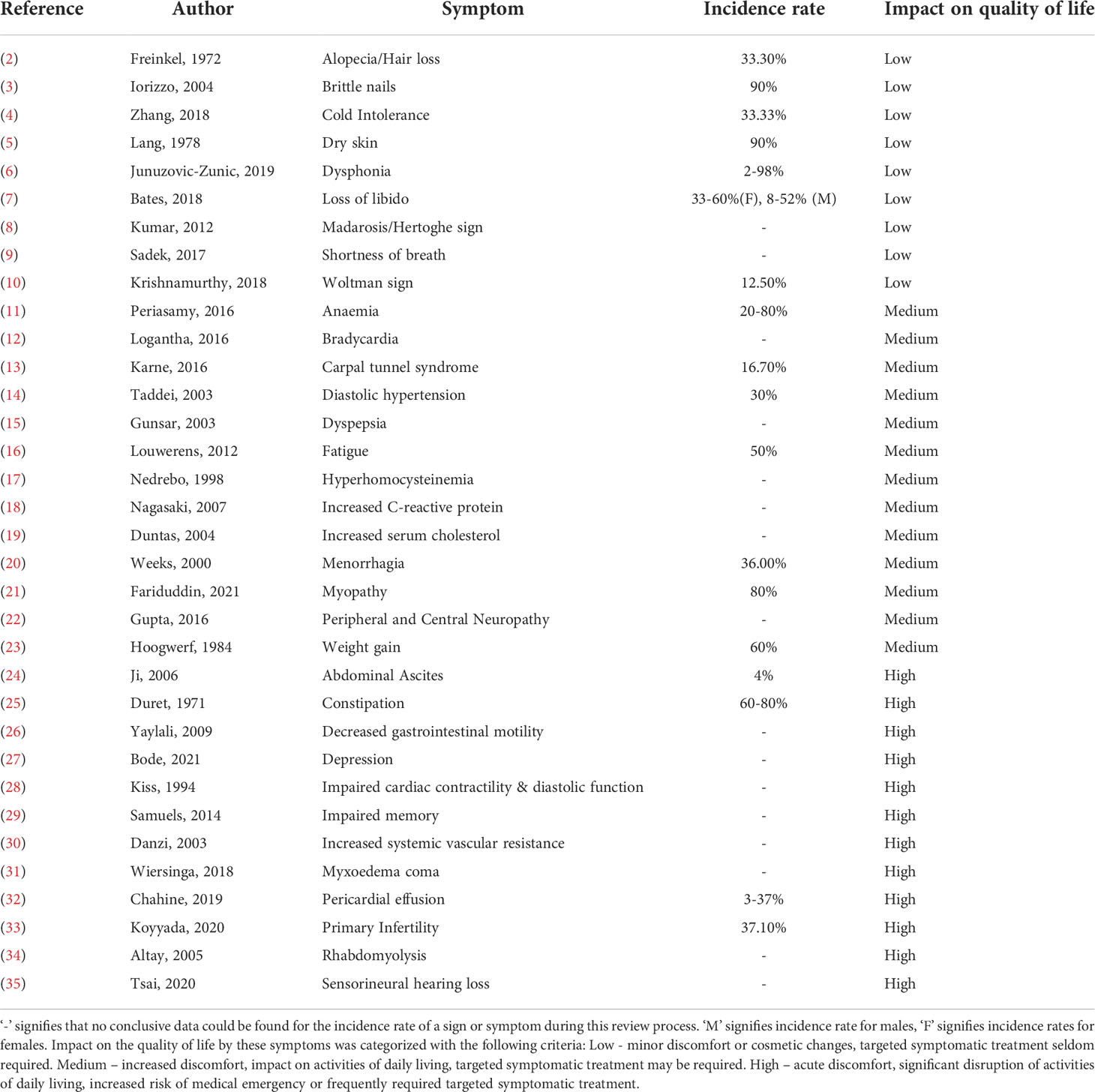
Table 1 Signs & Symptoms of Hypothyroidism and their impact on quality of life: This table details the signs and symptoms of hypothyroidism, along with the incidence rate and perceived impact on the quality of life of patients.
In accordance with its aetiology, hypothyroidism can be divided into three subtypes: primary; central, comprised of secondary and tertiary; and peripheral (42). Primary hypothyroidism, which accounts for 99% of all hypothyroidism cases, arises due to an inability of the thyroid to produce the thyroid hormones triiodothyronine (T3) and thyroxine (T4) (41). This can be attributed to a variety of reasons including medication and iodine deficiency (43, 44). However, the majority of causes in developed countries are instead due to direct damage of the thyroid gland through processes like inflammation, autoimmune disease, or radiation (45–47).
For example, the most common clinically late effect of radiation, when applied at a therapeutic dose (30-70Gy) within the cervical region, is primary hypothyroidism (46, 48). The overall incidence of hypothyroidism following irradiation in the head or neck region varies widely between studies, ranging up to 92%, but most frequently lays between 20-60% (49). The exact pathological mechanism through which radiation induces primary hypothyroidism remains to be eluded but radiation is known to directly cause gland damage through induction of apoptosis, fibrosis of the gland capsule, atherosclerosis, thyroiditis, thyroglobulin antibodies (50–54).
Considering the direct correlation between primary hypothyroidism and radiotherapy for head and neck cancer, as well as the observed significant increase in survival rates of cancer, rising from 24% to 50% over the last 40 years, and a latency period of up to 27 years for radiation-induced hypothyroidism, we can expect to see a significant increase in the incidence of primary hypothyroidism (55). In addition, the correlation between age and an increased prevalence of hypothyroidism, in a steadily ageing population, will contribute to an overall higher incidence of hypothyroidism in the near future.
Here, we will review current primary hypothyroidism treatment methods and novel therapeutic approaches. The novel approaches currently under investigation employ regenerative medicine and tissue engineering strategies, primarily focusing on regenerative scaffolds. This type of therapeutic may be applicable against various conditions affecting the thyroid gland. Thus, we have taken care to specifically select therapeutics produced with a purpose for application against primary hypothyroidism and qualities needed to treat this condition.
2 Treatment of primary hypothyroidism
2.1 Current approaches
Currently, there is no distinction between the treatments associated with primary hypothyroidism based on causality. As with the majority of endocrinological conditions induced by a lack of hormones, primary hypothyroidism is treated through hormone replacement therapy (HRT) (56). This is required to be taken for life if the disease cannot be treated through different methods.
2.1.1 Desiccated thyroid extract
The first regimen of thyroid HRT was described in 1891 by Murray and included the subcutaneous injection of sheep thyroid extract (57). Soon after, it was shown that the oral administration of thyroid extract proved to be just as effective (58). Desiccated thyroid extract (DTE) is thyroid gland tissue which has been lyophilized; it contains both T4 and T3 (59).
The majority of DTE used today is generated from pigs. However, there is an extensive dispute around the usage of DTE to treat any form of hypothyroidism, following the introduction of synthetic hormones (56). An argument against the use of pig DTE is the T4:T3 ratio, commonly around 4:1, in contrast to the human physiological ratio of 14:1 (60, 61). The treatment may therefore lead to a supraphysiological concentration of T3, which can lead to severe adverse effects such as those associated with thyrotoxicosis, including palpitations, heat intolerance and in severe circumstance loss of consciousness (62). Long-term, double-blind studies regarding the safety of DTE use for hypothyroidism are also lacking. Additionally, the contents of DTE preparations, excluding hormone concentrations, remain unregulated in many parts of the world (63). The use of DTE preparations is not currently approved by the Food and Drug Administration (FDA). However, as DTE was introduced into the market before FDA approval was implemented (“grandfathered in”), this is not a legal obligation and the contents and quality of DTE remains unregulated by authorities. Furthermore, clinical studies did not find a difference between the effectiveness of DTE and alternate HRTs, but most patients preferred DTE due to improvement of subjective symptoms and adverse effects (64, 65). This evidence highlights the need for further research regarding the use of DTE for hypothyroidism treatment.
2.1.2 Synthetic T4 monotherapy
T4 crystals were originally purified by Kendall in 1914 and became commercially available soon after (66). 12 years later, Harrington and Barger were able to delineate the structure of T4 and synthetic T4 was available for clinical use by the 1930s (67). However, it was not commonly used until almost 20 years later, likely due to its high cost, when it was marketed similar to a completely new drug (68, 69).
Today, levothyroxine (L-T4) is the treatment of choice for primary hyperthyroidism (70, 71). Whilst the safety and effectiveness of L-T4 are well established, there are multiple drawbacks and issues associated with its therapeutic use. There is no one-size-fits-all approach to treatment with L-T4; monitoring of serum thyroid stimulating hormone (TSH) levels, which regulates thyroid hormone secretion, must be undertaken for the entire duration of therapy. It is recommended that TSH levels are measured upon starting treatment and following every dose change, as well as yearly following stabilization of the L-T4 dose (71). If too little L-T4 is administered, serum TSH levels remain high and symptoms of hypothyroidism persist, whilst if the dosage is too high, serum TSH levels may decrease excessively, and symptoms of thyrotoxicosis may be encountered. This includes parlous cardiac issues such as tachycardia, arrhythmias, palpitations (72). Overtreatment with L-T4, which leads to TSH levels below 0.1 mlU/L, has also been shown to be associated with adverse skeletal health, in the form of bone mineral density loss increasing risk for fractures and osteoporosis, especially in elderly patients (73, 74). Additionally, due to the positive inotropic and chronotropic effects of thyroid hormone on the heart, patients with pre-existing ischemic heart disease will need to be started on a low dose of L-T4, which is gradually titrated upwards (75, 76). Cardiac medication will need to be adjusted in some of these patients so that the adequate dose of L-T4 needed to maintain a euthyroid state can be tolerated (75).
Furthermore, several medications, supplements and foods can interfere with the absorption, metabolism, and efficacy of L-T4. A comprehensive list of common drugs and minerals which fall into the category of interacting with L-T4, but do not induce hypothyroidism by themselves, can be seen in Table 2. Estrogen and methadone increase the bound fraction of T4 indirectly through raising circulating levels of thyroxine-binding globulin (TBG), leading to a higher treatment dose of L-T4 required to achieve satisfactory thyroid hormone levels (79, 80). Glucocorticoids and anabolic steroids have the opposite effect, decreasing thyroid binding globulin concentrating, often requiring a reduction of the L-T4 dose (84, 85). Many antacids and foods or dietary supplements rich in iron, calcium, and magnesium, as well as fiber and caffeine decrease the gastric absorption of L-T4 (91, 94, 95). Multiple seizure medications and drugs with agonistic β-adrenoreceptor activities, are known to increase the clearance of L-T4, as they act as enzyme inducers, directly altering thyroid hormone metabolism (100, 101, 106, 111, 112). Lastly, some drugs alter the secretion of thyroid stimulating hormone, also leading to an increased dose of L-T4 being required (108, 110, 113). In some cases, instead of a therapeutic L-T4 dose increase, the interacting drugs or foods may also be taken 4 hours apart to minimize any adverse events (71).
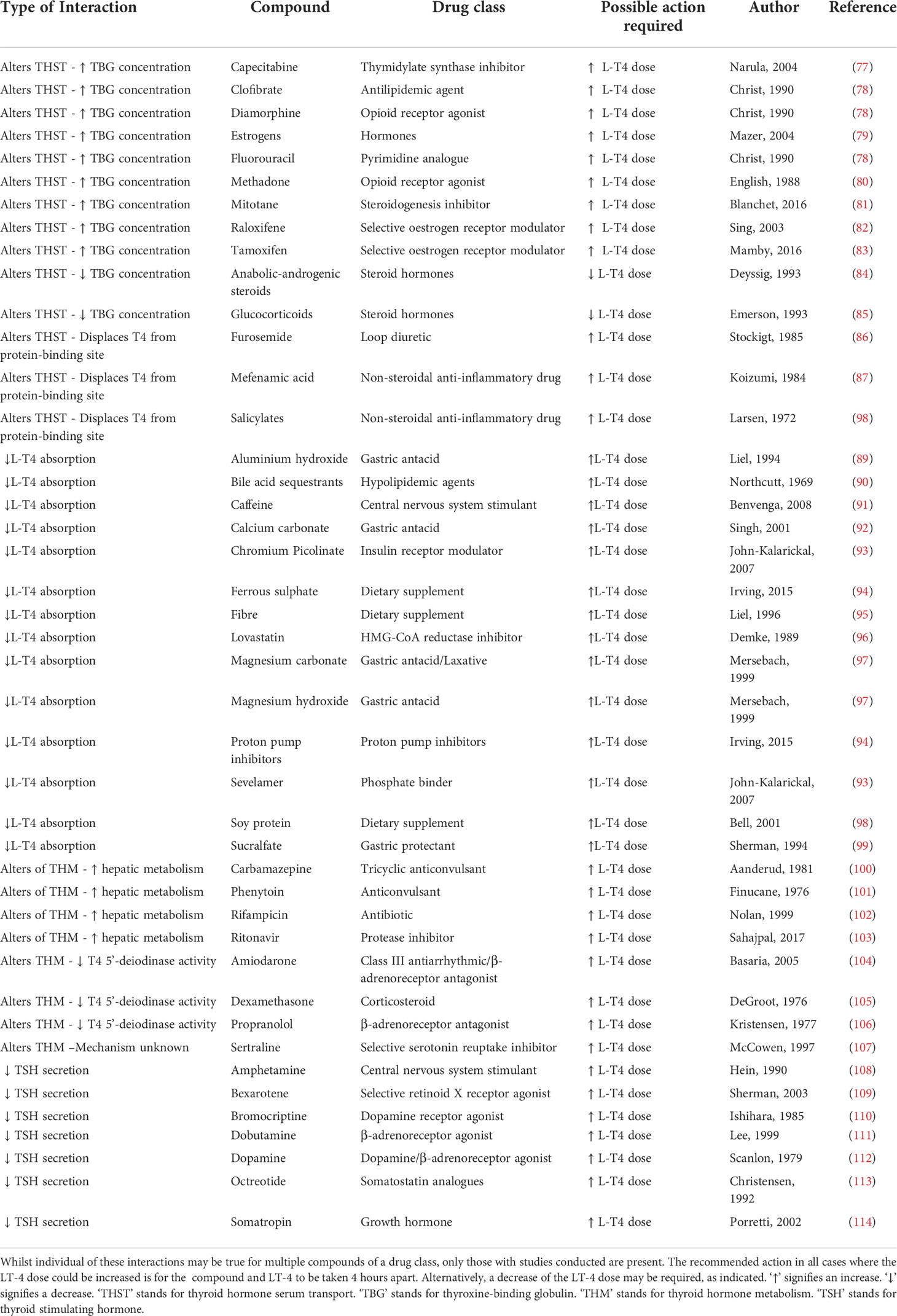
Table 2 Overview of interactions with levothyroxine (LT4): Compounds which have been recorded to interact with LT-4 and their effect on LT-4 metabolism, distribution and absorption are listed.
Most failings of L-T4 therapy can be attributed to poor patient compliance (115, 116). This is often due to the limitations and inconvenience imposed by the therapy such as avoidance of the above discussed medications, daily administration of the medication, and food intake prior to as well as 30-60 minutes after ingesting L-T4 (117). Non-compliance with the prescribed therapy can lead to adverse metabolic and cardiovascular effects, as well as psychological events such as paranoia and depression, further impairing quality of life (118). Whilst it has been proposed that weekly and intermittent administrations of L-T4 could prevent these issues, a dose sevenfold higher would be required. High doses of L-T4 may lead to a condition known as iatrogenic thyrotoxicosis factitia, when tissue is exposed to high levels of circulating thyroid hormone, causing the patient to experience symptoms of thyrotoxicosis such as palpitations, sweating and anxiety (72).
These symptoms of iatrogenic thyrotoxicosis were correlated with increased levels of plasma hydroperoxides, which act as markers of oxidative stress. Additionally, these symptoms were relieved using physiological modulators which expressed antioxidant activities (119, 120). Together, this evidence suggests that chronic use of L-T4 provokes a hypermetabolic state which leads to an increased mitochondrial production of reactive oxygen species (ROS). This excessive production of free radicals gives rise to oxidative stress; a harmful process which can negatively affect several cellular structures including membranes, lipids, proteins, lipoproteins, and DNA (121). The resulting consequences of oxidative stress have been associated with adverse consequences including the promotion of tumorigenesis, cardiovascular disease, neurological disease, renal disease, and chronic inflammatory diseases (122–127). Whilst there are other synthetic T4 alternatives available to L-T4, none of these circumvent the above discussed issues encountered with L-T4 therapy.
2.1.3 Synthetic combination therapy
In contrast to T4, serum T3 was discovered in 1952 by Gross and Pitt-Rivers (128, 129). A therapy with the combination of ‘natural’ T3 and T4 was originally employed as treatment for hypothyroidism through the use of DTE. As the use of DTE started being questioned, mainly due to discontent with variable potency, mixtures of synthetic T3, commonly liothyronine (L-T3) and L-T4 were employed instead (130). However, the landmark discovery that T4 can be peripherally converted into T3 in thyroid-deficient patients led to the replacement of LT3:LT4 combination therapy with L-T4 monotherapy as a first-line therapy (131).
Nevertheless, a significant minority of 5-10% of patients experience persistent symptoms related to hypothyroidism despite treatment with L-T4 (71, 132). Multiple studies have shown that many of these patients do not achieve the physiological free T3:T4 ratio, despite presenting with TSH serum level within the normal range (133, 134). This suggests an impairment of hepatic or renal conversion of T4 to T3, which may account for the persistence of the symptoms. Additionally, studies show that cerebral, hepatic, and skeletal muscle tissue of rats treated with L-T4 exhibit markers of localized hypothyroidism (135). Together this evidence indicates that L-T4/L-T3 combination therapy may be preferential.
Several studies have indeed evaluated the effect of T3/T4 combination therapy and obtained mixed results. A meta-analysis involving 1216 patients in 11 randomized controlled trials indicated no significant improvement in hypothyroid-related symptoms between combination and monotherapy (136). Another meta-analysis showed that 48% of patients preferred combination therapy, despite no improvement of objective symptoms, whilst 25% preferred T4 monotherapy, and 27% had no preference (71). It has been indicated that the results of these studies may be divergent due to single nucleotide polymorphisms (SNP) in genes involved in the thyroid hormone axis. This especially includes Thr92Ala in type 2 deiodinase (DIO2), essential for the peripheral conversion of T4 into T3 and present in the brain, pituitary, skeletal muscle, heart, brown adipose tissue, and thyroid (60, 137, 138). Indeed, studies have indicated that this particular SNP is associated with a significantly enhanced response to combination therapy and impaired baseline psychological well-being when on T4 monotherapy (139, 140). Multiple other SNPs have been indicated in genes associated with the regulation of the thyroid hormone axis (141–143). This implies that personalized medicine, based on genotype, may be the most ideal way of deciding the type of thyroid HRT needed. Further trials defining the influence of SNPs on and the safety and efficacy of T3:T4 combination therapy will need to be conducted before this can be implemented. The cost effectiveness of precision medicine approaches like this, and the restructuring of implemented healthcare systems required to implement these for a disease as common as hypothyroidism, may add considerable hurdles (144).
3 Experimental bioengineering and regenerative medicine approaches
The idea of transplantation to replace the thyroid gland and recover normal function, was originally proposed in the late 1800s by Sir Victor Horsley, through the suggestion that grafting a portion of healthy thyroid tissue may be a viable treatment method for myxoedema – now known to result from advanced hypothyroidism (145). Whilst this treatment initially showed success, it did not last long and with the recognition of the issues associated with organ transplantations, further attempts at utilizing this treatment method quickly diminished. However, with the advances in stem cell biology, thyroid progenitor cells were identified, revitalizing the idea of cell transplantation to rescue thyroid function. The harvesting and culturing of thyroid progenitor cells has proven to be a challenging task, hence other approaches to obtaining transplantable thyroid cells have been pursued. These have mainly focused on the utilization of embryonic stem cells (ESCs) or induced pluripotent stem cells (iPSCs), but also on primary thyrocytes. A list of studies employing tissue engineering and regenerative medicine approaches as treatment for primary hypothyroidism is displayed in Table 3.
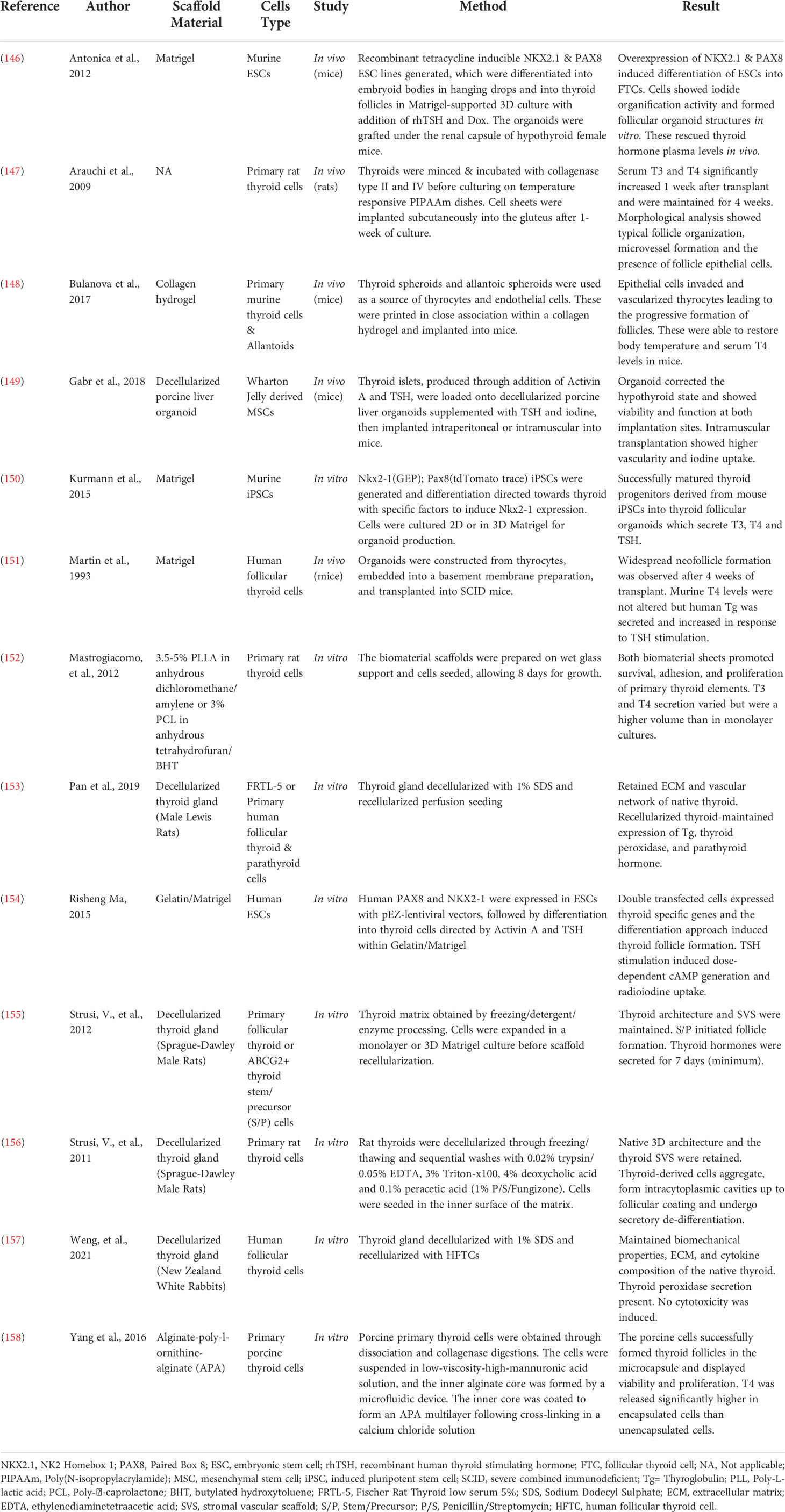
Table 3 Novel bioengineering and regenerative medicine approaches to produce treatments for hypothyroidism.
3.1 Biopolymer scaffolds
The use of biopolymer scaffolds has received much attention within regenerative medicine and bioengineering. They have been applied for the purpose of restoration and reconstruction of organ function in various tissues (159–162). Scaffolds are constructed of three-dimensional porous, fibrous, or permeable biomaterials with the intent of permitting transport of liquids and gases, promoting cell interaction, viability, and extracellular matrix (ECM) deposition whilst eliciting minimal inflammatory or cytotoxic responses (163). These scaffolds do not only provide mechanical support, but also serve as delivery systems for bioactive molecules and templates for cell adhesion, acting as an initiator for tissue neogenesis (164).
One of the earliest instances of utilizing biopolymer scaffolding to rescue thyroid function perhaps includes the transplantation of thyroid organoids, produced through culture of human thyrocytes in Engelbreth-Holm-Swarm mouse sarcoma – commercially known as Matrigel® –, into severe combined immunodeficiency (SCID) mice (151). Whilst this transplantation did not alter murine serum T4 levels, evidence of thyroid follicle reconstitution along with neovascularization was found. Furthermore, human thyroglobulin was detected in the serum, which increased upon human TSH stimulation, indicating functionality and TSH responsiveness of the transplanted organoids.
Subsequent experiments indicated the importance of the expression of transcription factors NK2 homebox 1 (NKX2-1, previously TTF-1) and paired box 8 (Pax8) in thyroid development as well as the specific thyroid gene expression program (165). Consequent overexpression of Pax8 and NKX2-1 proved to be sufficient to direct mouse ESC differentiation into thyrocyte-like cells (146). Remarkably, transient expression of these transcription factors was able to induce long-term thyroid cell development, negating the need for outside induction of these genes. These induced thyrocytes formed follicular aggregates when cultured in 3D Matrigel and stimulated with TSH, which demonstrated polarized characteristics consistent with natural thyroid follicles. Upon engraftment into athyroid mice, these follicles rescued serum thyroid hormone levels and promoted symptomatic recovery. A similar approach involving overexpression of Pax8 and NKX2-1 in human ESCs cultured in a Gelatine/Matrigel system produced functional thyroid follicles upon Activin A and TSH stimulation (154).
Alternatively, to primary cells, iPSCs, generated from human dermal fibroblasts, have successfully been used to produce T3 and T4 secreting thyroid progenitors in Matrigel (150). This was achieved in NKX2-1 haploinsufficient lines, through stimulation with Activin A, transient inhibition of transforming growth factor (TGF) β and bone-morphogenetic proteins (BMP) signalling, followed by stimulation with fibroblast growth factor (FGF) 2 and BMP4 and maturation in Matrigel.
Notably, the above-described approaches all employed Matrigel or Matrigel-combination gels as a scaffold. To date, only one study has been published using an alternative biomaterial with the aim of rescuing a hypothyroidism phenotype. This involved the use of either poly-L-lactic acid (PLLA) or poly-caprolactone (PCL) polymerized on a wet glass support and seeded with primary rat thyroid cells (152). Both biomaterials increased survival, adhesion, proliferation, and thyroid hormone (T3 and T4) secretion of the thyroid cells when compared to a monolayer cell culture.
Other biomaterials which may be of interest include a vast range of hydrogels as they are soft and elastic, reflecting the intrinsic properties of native thyroid (163). Indeed, a study bioprinted a mouse thyroid construct within a collagen hydrogel (148). The endothelial cells derived from allantoic spheroids included in this construct established vascularization of the gland. Following engraftment below the kidney capsule, the construct was able to restore blood thyroxine levels and body temperature in athyroid mice. Another group utilized alginate–poly-L-ornithine-alginate to produce encapsulated thyroid follicles from porcine thyroid cells (158). This tri-layered scaffold significantly increased the level of T4 released from the follicles, indicating these may be applied to rescue a hypothyroid state.
3.2 ECM scaffolds
One of the main components of native tissue is the ECM which provides the microenvironment cells need to thrive. The majority of ECM, including the thyroid ECM, is composed of collagen, fibronectin, laminin and perlecan, forming collagen fibers and proteoglycan filaments, responsible for supplying tissue-reflective durability and tensile strength (166). As opposed to biomaterial scaffolds, the majority of ECM scaffolds are produced through the decellularization of native tissue, a process which utilizes detergents or mechanical manipulation to remove components that may elicit an inflammatory or immune response if implanted in a host (163). This causes the ECM to retain its biomechanical properties, composition, architecture, and biological activity, allowing it to direct cell migration and cell fate. The resulting decellularized ECM (dECM) scaffold can then be recellularized with the cell type of choice. Whilst the advantages of dECM scaffolds compared to biomaterial scaffolds include that it more closely mimics the required microenvironment, dECM scaffolds still have a potential for immunogenicity (167). Additionally, the tissue for these scaffolds needs to be obtained from healthy animals, proving to be less ethical than the production of some synthetic biomaterials.
There have been multiple attempts at producing a dECM scaffold for the regeneration of thyroid function. These most commonly utilize a decellularization process involving 1% sodium dodecyl sulfate (SDS), which is cytotoxic and requires extensive washing procedures as it is difficult to remove from the tissue due to its ionic nature (168). SDS is also known to cause alteration of the microstructure in some instances but no evidence of this was found in studies utilizing this decellularization approach on the thyroid (153, 157). Alternatively, one study by Strusi et al. used an approach combining trypsin, Triton-x100, peracetic acid, deoxycholic acid and ethylenediaminetetraacetic acid (EDTA) (156). Whilst this approach perfectly preserved the delicate stromal-vascular scaffold of the thyroid, peracetic acid and EDTA are known to increase the stiffness of the ECM and decrease the salt- and acid-soluble proteins (168). However, the mechanical properties and protein contents of the produced dECM scaffold were not assessed in this study.
A rat dECM scaffold which has been reseeded with the spontaneously immortalized rat thyroid cell line FRTL-5 has shown generation of three-dimensional structures resembling thyroid follicles in vitro, as well as synthesis of thyroglobulin and thyroid peroxidase, indicating thyroid-specific function (153). Whilst immortalized cell lines may not fully reflect the functionality of native tissue, these results have been confirmed in the same study by successful reseeding of the scaffold with primary human thyrocytes and maintenance of thyroglobulin and TPO gene expression. Additionally, another study involving the generation of a rabbit dECM scaffold reseeded with human thyrocytes indicated a functional model which did not induce cytotoxicity in vitro (157).
Instead, stem cells have been utilized to attempt the production of a functional dECM scaffold. One study focused on the recellularization of a stromal/vascular rat matrix with thyroid stem/precursor cells (155). Analysis of these indicated the formation of thyrocytes and heterotopic migration of the stem cells into the matrix septa where they orthotopically aggregated and gave rise to intracytoplasmic cavities, similar to those seen in native thyroid follicles. This scaffold secreted thyroid hormones for a minimum of seven days. Another study utilized thyroid islets derived from mesenchymal stem cells to recellularize a porcine liver organoid, which, upon implantation into mice, successfully rescued a hypothyroid state (149). Together these indicate that a stem cell approach can be used to produce a dECM scaffold which secretes thyroid hormone and can be used as treatment for hypothyroidism. However, it is noteworthy that only one of the dECM scaffolds has been tested in vivo (149).
Lastly, one study focused on the generation of an ECM scaffold without the use of decellularized tissue. This involved mincing of whole thyroid tissue, followed by digestions with collagenase and culturing on thermo-responsive Poly(N-isopropylacrylamide) (PIPAAm) dishes (147). Following culture of the cells, a slight change in the external temperature allowed controlled detachment of a thyroid cell sheet which, upon implantation in athyroid mice, significantly increased T3 and T4 serum levels.
4 Future outlook
Primary hypothyroidism is known to be more prevalent in females than in males, particularly during puberty and menopause (169). This has been partially attributed to an increased presence of estrogen in females which exhibits indirect effects on the thyroid economy, increasing thyroxine binding globulin, as discussed above (170). Additionally, excessive estrogen enhances inflammatory processes within the immune system, which increases the likelihood of autoimmune mediated thyroid damage occurring (171). These are factors which should be taken into consideration during the development of a scaffold. Estrogen also exhibits direct effects on thyroid cells, stimulating proliferation and migration (172). Through addition of this hormone to a bioengineered scaffold, this has potential to be exploited to enhance thyroid regeneration following gland damage.
Whilst the transplantation of primary thyroid cells presents as a reality in mice, the potential for immune attack on these cells remains, which would prevent this to be clinically applied as treatment for primary hypothyroidism. However, the discovery of iPSCs circumvents this issue. This technique allows any cell type to be reprogrammed into a pluripotent state through the induction of the genes Oct3/4, Sox2, c-myc and Klf4 (173). A vast number of studies describe the derivation of iPSCs from human tissue and as discussed above, these have been successfully differentiated into thyroid-like cells, able to produce thyroid hormones. This approach is most likely to be clinically beneficial as it negates the need to isolate thyroid cells from human tissue before damage occurs to the organ and would not require immunosuppressant therapy which would severely decrease quality of life of the patients.
It is not only the cellular component which may elicit an immunological response from the recipient; the materials used to fabricate these scaffolds also provide immunogenic potential and may lead to the development of an acute sterile inflammatory reaction termed a foreign body reaction (FBR). Scaffolds may induce a chronic neutrophil infiltrate, often leading to fibrosis around the site of implantation, decreasing the regenerative efficacy of the scaffold. However, immune responses are known to diverge between biopolymers and dECM scaffolds (174). Overall, synthetic biopolymers have a high potential for inducing an innate response whilst no risk is present for an adaptive response. Conversely, natural biopolymers present a low risk for both an innate and adaptive host immune response arising (175). Alternatively, clinically employed ECM material has been shown to prevent the formation of a fibrous capsule and instead induce a type-2-like immune response, through production of M2 macrophage and Th2 T cell, which has been correlated with an improved outcome of scaffold integration and tissue regeneration (174, 176, 177). However, many other scaffold properties including surface chemistry related to moiety and charge, and topography determine the immunomodulatory function of, hence immune response to the scaffold (178, 179). Furthermore, as with any invasive medical procedure a risk of infection is present. This, however, is reduced by the multiple injectable scaffolds currently under development, which make the scaffold implantation procedure minimally invasive (180, 181).
The functional activity of thyrocytes closely relates to their structural organization into follicles, with polarization being a key factor for hormone production. It was previously shown that monolayer culture of thyrocytes may cause loss of their functional activity (182). Hence, multiple three-dimensional in vitro approaches have been employed to maintain essential properties of thyroid tissue including cell polarization, intracellular contact, and presence of the intrafollicular lumens (183). The production of a scaffold which allows thyroid hormone secretion in vivo is therefore more likely to succeed if the material used allows the cells to assume the follicle confirmation required to achieve this. Taken together with the intrinsic elasticity of thyroid tissue, based on its relatively low young’s modulus, a softer material mirroring these properties may be most beneficial for this purpose (184).
Whilst the use of scaffolds composed of either decellularized tissue or natural polymers - although with the majority using Matrigel - has been extensively explored for the use of thyroid tissue engineering, this is not the case for synthetic polymers. Indeed, only one study has explored these by investigating the thyroid regenerative abilities of PCL or PLLA scaffolds (152). Synthetic polymers offer advantages in the form of tuneable properties and consistent, predictable mechanical-chemical properties (185, 186). However, while this indicates further exploration of their use in thyroid scaffolding may be of worth, utilized on their own they often display poor cell adhesion due to a lack of adhesion sites (187). The formulation of composite scaffolds would circumvent the issues associated with the use of either natural polymers, synthetic polymers or decellularized tissue as a single scaffold component. This may involve the incorporation of decellularized tissue into biopolymer scaffolds, which has been successfully used in other tissues and allows for recapitulation of the complex native ECM properties of the thyroid, adding increased cell viability, without compromising advantages of biopolymer scaffolds (188–190).
5 Conclusion
The application of bioengineering and regenerative medicine approaches to produce novel primary hypothyroidism therapeutics is promising. Investigations have been made into scaffolds fabricated using primary thyrocytes, but these prove difficult to culture. Additionally, a fully formed thyroid follicle is needed to produce thyroid hormones. Therefore, the utilization of stem cells may provide a better direction for further research, especially with the formation of thyroid organoids derived from these being well established. Both biopolymers and decellularized scaffolds have been used successfully to construct implants for the purpose of rescuing a hypothyroid phenotype. However, little diversity has been explored with regards to biopolymers, with the focus remaining on hydrogels, especially Matrigel. Future studies should address the usage of a wider variety of biopolymers, seeing as these have been applied with much success in other tissues. The recent advances in the application of composite scaffolds within tissue engineering also act as promising future avenues for thyroid applications. The amalgamation of various polymers with dECM should be further investigated as it has the possibility of providing a more favorable niche for cell growth due to the presence of native proteins, despite not losing any tuning potential. Lastly, only few of these constructed scaffolds have been investigated in vivo. Studies should be conducted regarding the implantation of these scaffolds in vivo as this will give us more insight into the physiological effects in a whole system. Whilst the scaffolding approach provides an auspicious outlook for primary hypothyroidism treatment, further work is required until clinical application.
Author contributions
MH performed the literature review, drafted the manuscript and tables, and edited the manuscript. AC contributed to hypothesis generation and edited the manuscript. IN and EE edited the manuscript. All authors contributed to the article and approved the submitted version.
Funding
This research was funded in whole by the Engineering and Physical Sciences Research Council (EPSRC) grant EP/T517884/1. This funding source had no role in the design and execution of this review or decision to submit results. For the purpose of open access the author has applied a creative commons attribution (CC BY) licence to any author accepted manuscript version arising. Additional funding was provided by the University of Edinburgh UKRI Open Access Fund.
Conflict of interest
The authors declare that the research was conducted in the absence of any commercial or financial relationships that could be construed as a potential conflict of interest.
Publisher’s note
All claims expressed in this article are solely those of the authors and do not necessarily represent those of their affiliated organizations, or those of the publisher, the editors and the reviewers. Any product that may be evaluated in this article, or claim that may be made by its manufacturer, is not guaranteed or endorsed by the publisher.
References
1. Li Qian L, Hopkins ME, Nixon IJ, Hay A. Thyroid function post laryngectomy and hemithyroidectomy – do all laryngectomy patients need thyroid replacement? Clin Otolaryngol (2022) 47(2):323–7. doi: 10.1111/coa.13883
2. Freinkel RK, Freinkel N. Hair growth and alopecia in hypothyroidism. Arch Dermatol (1972) 106(3):349–52. doi: 10.1001/archderm.1972.01620120037007
3. Iorizzo M, Pazzaglia M, Piraccini BM, Tullo S, Tosti A. Brittle nails. J Cosmet Dermatol (2004) 3(3):138–44. doi: 10.1111/j.1473-2130.2004.00084.x
4. Zhang Z, Boelen A, Kalsbeek A, Fliers E. TRH neurons and thyroid hormone coordinate the hypothalamic response to cold. Eur Thyroid J (2018) 7(6):279–88. doi: 10.1159/000493976
6. Junuzović-Žunić L, Ibrahimagić A, Altumbabić S. Voice characteristics in patients with thyroid disorders. Eurasian J Med (2019) 51(2):101.
7. Bates JN, Kohn TP, Pastuszak AW. Effect of thyroid hormone derangements on sexual function in men and women. Sex Med Rev (2020) 8(2):217–30. doi: 10.1016/j.sxmr.2018.09.005
8. Kumar A, Karthikeyan K. Madarosis: A marker of many maladies. Int J Trichol (2012) 4(1):3. doi: 10.4103/0974-7753.96079
9. Sadek S, Khalifa W, Azoz A. Pulmonary consequences of hypothyroidism. Ann Thorac Med (2017) 12(3):204. doi: 10.4103/atm.ATM_364_16
10. Krishnamurthy A, Vishnu VY, Hamide A. Clinical signs in hypothyroidism–myoedema and woltman sign. QJM: Int J Med (2018) 111(3):193–3. doi: 10.1093/qjmed/hcx205
11. Periasamy S, Prabhu G, Balamurugan S, Aleemsarwar A. STUDY OF PREVALENCE AND PATTERN OF ANAEMIA IN HYPOTHYROIDISM. J Evol Med Dental Sci (2016) 5(76):5654–6. doi: 10.14260/jemds/2016/1275
12. Logantha SR, Ozbeyli D, Yanni J, D’souza A, Dobrzynski H, Kasimay O, et al. Bradycardia in hypothyroidism is caused by intrinsic remodelling of the sinus node - the physiological society. Thomas R. editor Paper presented at the Physiology Conference (2016) Dublin, Ireland.
13. Karne SS, Bhalerao NS. Carpal tunnel syndrome in hypothyroidism. J Clin Diagn Res: JCDR (2016) 10(2):OC36. doi: 10.7860/JCDR/2016/16464.7316
14. Taddei S, Caraccio N, Virdis A, Dardano A, Versari D, Ghiadoni L, et al. Impaired endothelium-dependent vasodilatation in subclinical hypothyroidism: Beneficial effect of levothyroxine therapy. J Clin Endocrinol Metab (2003) 88(8):3731–7. doi: 10.1210/jc.2003-030039
15. Gunsar F, Yilmaz S, Bor S, Kumanlioˇglukumanlioˇ Kumanlioˇglu K, Kabalak T, Ahmet Ozutemiz O. Effect of hypo-and hyperthyroidism on gastric myoelectrical activity. Digest Dis Sci (2003) 48(4):706–12. doi: 10.1023/A:1022876423487
16. Louwerens M, Appelhof BC, Verloop H, Medici M, Peeters RP, Visser TJ, et al. Fatigue and fatigue-related symptoms in patients treated for different causes of hypothyroidism. Eur J Endocrinol (2012) 167(6):809–15. doi: 10.1530/EJE-12-0501
17. Nedrebø BG, Ericsson UB, Nygård O, Refsum H, Ueland PM, Aakvaag A, et al. Plasma total homocysteine levels in hyperthyroid and hypothyroid patients. Metab - Clin Exp (1998) 47(1):89–93. doi: 10.1016/S0026-0495(98)90198-6
18. Nagasaki T, Inaba M, Shirakawa K, Hiura Y, Tahara H, Kumeda Y, et al. Increased levels of c-reactive protein in hypothyroid patients and its correlation with arterial stiffness in the common carotid artery. Biomed Pharmacother (2007) 61(2–3):167–72. doi: 10.1016/j.biopha.2006.10.008
19. Duntas LH. Thyroid disease and lipids (2004). Available at: https://home.liebertpub.com/thy.
20. Weeks AD, Prentice A. Menorrhagia and hypothyroidism: Evidence supports association between hypothyroidism and menorrhagia. BMJ: Br Med J (2000) 320(7235):649.
22. Gupta N, Arora M, Sharma R, Arora K. Peripheral and central nervous system involvement in recently diagnosed cases of hypothyroidism: An electrophysiological study. Ann Med Health Sci Res (2016) 6(5):261. doi: 10.4103/amhsr.amhsr_39_16
23. Hoogwerf BJ, Nuttall FQ. Long-term weight regulation in treated hyperthyroid and hypothyroid subjects. Am J Med (1984) 76(6):963–70. doi: 10.1016/0002-9343(84)90842-8
24. Ji JS, Chae HS, Cho YS, Kim HK, Kim SS, Kim CW, et al. Myxedema ascites: Case report and literature review. J Korean Med Sci (2006) 21(4):761–4. doi: 10.3346/jkms.2006.21.4.761
25. Duret RL, Bastenie PA. Intestinal disorders in hypothyroidism clinical and manometric study. Am J Dig Dis (1971) 16(8):723–7. doi: 10.1007/BF02239597
26. Yaylali O, Kirac S, Yilmaz M, Akin F, Yuksel D, Demirkan N, et al. Does hypothyroidism affect gastrointestinal motility? Gastroenterol Res Pract (2010) 2009:529802–7. doi: 10.1155/2009/529802
27. Bode H, Ivens B, Bschor T, Schwarzer G, Henssler J, Baethge C. Association of hypothyroidism and clinical depression: A systematic review and meta-analysis. JAMA Psychiatry (2021) 78(12):1375–83. doi: 10.1001/jamapsychiatry.2021.2506
28. Kiss E, Brittsan AG, Edes I, Grupp IL, Grupp G, Kranias EG. Thyroid hormone–induced alterations in phospholamban-deficient mouse hearts. Circ Res (1998) 83(6):608–13. doi: 10.1161/01.RES.83.6.608
29. Samuels MH. Psychiatric and cognitive manifestations of hypothyroidism. Curr Opin Endocrinol Diabetes Obes (2014) 21(5):377. doi: 10.1097/MED.0000000000000089
30. Danzi S, Klein I. Thyroid hormone and blood pressure regulation. Curr Hypertens Rep (2003) 5(6):513–20. doi: 10.1007/s11906-003-0060-7
31. Wiersinga WM. Myxedema and coma (Severe hypothyroidism). Endotext (2003) 4(2):137–41. doi: 10.1023/A:1022985902253
32. Chahine J, Ala CK, Gentry JL, Pantalone KM, Klein AL. Pericardial diseases in patients with hypothyroidism. Heart (2019) 105(13):1027–33. doi: 10.1136/heartjnl-2018-314528
33. Koyyada A, Orsu P. Role of hypothyroidism and associated pathways in pregnancy and infertility: Clinical insights. Tzu-Chi Med J (2020) 32(4):312. doi: 10.4103/tcmj.tcmj_255_19
34. Altay M, Duranay M, Ceri M. Rhabdomyolysis due to hypothyroidism. Nephrol Dialysis Transplant (2005) 20(4):847–8. doi: 10.1093/ndt/gfh745
35. Tsai Y, Chang IJ, Hsu CM, Yang YH, Liu CY, Tsai MS, et al. Association between sudden sensorineural hearing loss and preexisting thyroid diseases: A nationwide case-control study in Taiwan. Int J Environ Res Public Health (2020) 17(3):834. doi: 10.3390/ijerph17030834
36. Hage MP, Azar ST. The link between thyroid function and depression. J Thyroid Res (2012) 2012 :590648–8. doi: 10.1155/2012/590648
37. Song RH, Wang B, Yao QM, Li Q, Jia X, Zhang JA. The impact of obesity on thyroid autoimmunity and dysfunction: A systematic review and meta-analysis. Front Immunol (2019) 10:2349. doi: 10.3389/fimmu.2019.02349
38. Safer JD. Thyroid hormone action on skin. Dermatoendocrinol. (2011) 3(3):211. doi: 10.4161/derm.17027
39. Udovcic M, Pena RH, Patham B, Tabatabai L, Kansara A. Hypothyroidism and the heart. Methodist DeBakey Cardiovasc J (2017) 13(2):55. doi: 10.14797/mdcj-13-2-55
40. Chaker L, Bianco AC, Jonklaas J, Peeters RP. Hypothyroidism. Lancet (2017) 390(10101):1550. doi: 10.1016/S0140-6736(17)30703-1
41. Chiovato L, Magri F, Carlé A. Hypothyroidism in context: Where we’ve been and where we’re going. Adv Ther (2019) 36(Suppl 2):47. doi: 10.1007/s12325-019-01080-8
42. Nygaard B. Primary hypothyroidism. Lin K. editor American Family Physician London, UK: BMJ Publishing Group (2015), p. 359–60.
43. Zimmermann MB, Boelaert K. Iodine deficiency and thyroid disorders. Lancet Diabetes Endocrinol (2015) 3(4):286–95. doi: 10.1016/S2213-8587(14)70225-6
45. Slatosky J, Shipton B, Wahba H. Thyroiditis: Differential diagnosis and management. Am Family Phys (2000) 61(4):1047–52.
46. Jereczek-Fossa BA, Alterio D, Jassem J, Gibelli B, Tradati N, Orecchia R. Radiotherapy-induced thyroid disorders. Cancer Treat Rev (2004) 30(4):369–84. doi: 10.1016/j.ctrv.2003.12.003
47. Peng CCH, Chang RHE, Pennant M, Huang HK, Munir KM. A literature review of painful hashimoto thyroiditis: 70 published cases in the past 70 years. J Endocr Soc (2020) 4(2). doi: 10.1210/jendso/bvz008
48. Hancock SL, McDougall IR, Constine LS. Thyroid abnormalities after therapeutic external radiation. Int J Radiat Oncol Biol Phys (1995) 31(5):1165–70. doi: 10.1016/0360-3016(95)00019-U
49. Zhou L, Chen J, Tao CJ, Chen M, Yu ZH, Chen YY. Research progress of radiation-induced hypothyroidism in head and neck cancer. J Cancer (2021) 12(2):451. doi: 10.7150/jca.48587
50. Little JB. Principal cellular and tissue effects of radiation. In:Kufe DW, Pollock RE, Weichselbaum RR. editors Holland-Frei Cancer Medicine. 6th edition Hamilton, ON:B.C. Decker (2003).
51. Eckert CT, Probstein JG, Galinson S. Radiation of the thyroid. Radiology (1937) 29(1):40–4. doi: 10.1148/29.1.40
52. Feehs RS, Mcguirt WF, Bond MG, Strickland HL, Craven TE, Hiltbrand JB. Irradiation: A significant risk factor for carotid atherosclerosis. Arch Otolaryngol–Head Neck Surg (1991) 117(10):1135–7. doi: 10.1001/archotol.1991.01870220083014
53. DeGroot LJ. 14 radiation and thyroid disease. Baillière’s Clin Endocrinol Metab (1988) 2(3):777–91. doi: 10.1016/S0950-351X(88)80065-X
54. Lin Z, Chen L, Fang Y, Cai A, Zhang T, Wu VWC. Longitudinal study on the correlations of thyroid antibody and thyroid hormone levels after radiotherapy in patients with nasopharyngeal carcinoma with radiation-induced hypothyroidism. Head Neck (2014) 36(2):171–5. doi: 10.1002/hed.23285
55. Cancer survival statistics for all cancers combined | cancer research UK . Available at: https://www.cancerresearchuk.org/health-professional/cancer-statistics/survival/all-cancers-combined#heading-Zero.
56. McAninch EA, Bianco AC. The swinging pendulum in treatment for hypothyroidism: From (and toward?) combination therapy. Front Endocrinol (2019) 10:446. doi: 10.3389/fendo.2019.00446
57. Murray GR. Note on the treatment of myxœdema by hypodermic injections of an extract of the thyroid gland of a sheep. Br Med J (1891) 2(1606):796. doi: 10.1136/bmj.2.1606.796
58. Fox EL. A case of myxœdema treated by taking extract of thyroid by the mouth. Br Med J (1892) 2(1661):941. doi: 10.1136/bmj.2.1661.941
59. Mangier CN, Lund MH. Potency of united states pharmacopeia desiccated thyroid tablets as determined by the antigoitrogenic assay in rats. J Clin Endocrinol Metab (1970) 30(1):102–4. doi: 10.1210/jcem-30-1-102
60. Pilo A, Iervasi G, Vitek F, Ferdeghini M, Cazzuola F, Bianchi R. Thyroidal and peripheral production of 3,5,3’-triiodothyronine in humans by multicompartmental analysis. Am J Physiol (1990) 258(4 Pt 1):715–26. doi: 10.1152/ajpendo.1990.258.4.E715
61. Chakera AJ, Pearce SHS, Vaidya B. Treatment for primary hypothyroidism: current approaches and future possibilities. Drug Des Dev Ther (2012) 6:1. doi: 10.2147/DDDT.S12894
62. De Leo S, Lee SY, Braverman LE. Hyperthyroidism. Lancet (2016) 388(10047):906. doi: 10.1016/S0140-6736(16)00278-6
63. Weissel M. Role of desiccated thyroid extracts in the treatment of hypothyroidism: an update. Austrian J Clin Endocrinol Metab (2019) 12(4):159–64. doi: 10.1007/s41969-019-0071-x
64. Hoang TD, Olsen CH, Mai VQ, Clyde PW, Shakir MKM. Desiccated thyroid extract compared with levothyroxine in the treatment of hypothyroidism: A randomized, double-blind, crossover study. J Clin Endocrinol Metab (2013) 98(5):1982–90. doi: 10.1210/jc.2012-4107
65. Toloza FJK, Suarez NRE, El Kawkgi O, Golembiewski EH, Ponce OJ, Yao L, et al. Patient experiences and perceptions associated with the use of desiccated thyroid extract. Medicina (2020) 56(4):161. doi: 10.3390/medicina56040161
66. Kendall EC. The isolation in crystalline form of the compound containing iodin, which occurs in the thyroid. J Am Med Assoc (1915) LXIV(25):2042–3. doi: 10.1001/jama.1915.02570510018005
67. Harrington CR, Barger G. Thyroxine III: Constitution and synthesis of thyroxine. Biochem J (1927) 21:169–83. doi: 10.1042/bj0210169
68. Hart FD, Maclagan NF. Oral thyroxine in the treatment of myxoedema. Br Med J (1950) 1(4652):512. doi: 10.1136/bmj.1.4652.512
69. Lindholm J, Laurberg P. Hypothyroidism and thyroid substitution: historical aspects. J Thyroid Res (2011) 2011:809341–10. doi: 10.4061/2011/809341
70. Jonklaas J, Bianco AC, Bauer AJ, Burman KD, Cappola AR, Celi FS, et al. Guidelines for the treatment of hypothyroidism: prepared by the american thyroid association task force on thyroid hormone replacement. Thyroid (2014) 24(12):1670–751. doi: 10.1089/thy.2014.0028
71. Wiersinga WM, Duntas L, Fadeyev V, Nygaard B, Vanderpump MPJ. 2012 ETA guidelines: The use of l-T4 + l-T3 in the treatment of hypothyroidism. Eur Thyroid J (2012) 1(2):55–71. doi: 10.1159/000339444
72. Roomi S, Ullah W, Iqbal I, Ahmad A, Saleem S, Sattar Z. Thyrotoxicosis factitia: a rare cause of junctional rhythm and cardiac arrest. J Community Hosp Internal Med Perspect (2019) 9(3):258. doi: 10.1080/20009666.2019.1618668
73. Uzzan B, Campos J, Cucherat M, Nony P, Boissel JP, Perret GY. Effects on bone mass of long term treatment with thyroid hormones: a meta-analysis. J Clin Endocrinol Metab (1996) 81(12):4278–89. doi: 10.1210/jc.81.12.4278
74. Bauer DC, Ettinger B, Nevitt MC, Stone KL. Risk for fracture in women with low serum levels of thyroid-stimulating hormone. Ann Intern Med (2001) 134(7):561–8. doi: 10.7326/0003-4819-134-7-200104030-00009
75. Ellyin FM, Kumar Y, Somberg JC. Hypothyroidism complicated by angina pectoris: therapeutic approaches. J Clin Pharmacol (1992) 32(9):843–7. doi: 10.1002/j.1552-4604.1992.tb03893.x
76. Levine HD. Compromise therapy in the patient with angina pectoris and hypothyroidism. a clinical assessment. Am J Med (1980) 69(3):411–8. doi: 10.1016/0002-9343(80)90013-3
77. Narula HS, Carlson HE. Capecitabine-induced abnormalities in thyroid function tests. Am J Med (2004) 116(12):855–6. doi: 10.1016/j.amjmed.2004.02.025
78. Christ ER, Burger AG. Thyroid function tests. In:Huhtaniemi I, Martini L. editors Encyclopedia of endocrine dseases. 2nd ed Oxford:Academic Press (2015), 477–85.
79. Mazer NA. Interaction of estrogen therapy and thyroid hormone replacement in postmenopausal women. Thyroid (2004) 14(1):S27–S34. doi: 10.1089/105072504323024561
80. English T, Ruxton D, Eastman. CJ. Abnormalities in thyroid function associated with chronic therapy with methadone - PubMed. Clin Chem (1988) 34(11):2202–40. doi: 10.1093/clinchem/34.11.2202
81. Blanchet B, Clemence LM, Le MA, Habbas H, Claude MM, Groussin L, et al. Does mitotane influence free thyroid hormones levels? a possible explanation in vivo and in vitro. Endocr Abstr (2016) 41. doi: 10.1530/endoabs.41.GP202
82. Siraj ES, Gupta MK, Reddy S, Sethu K. Raloxifene causing malabsorption of levothyroxine. Arch Internal Med (2003) 163(11):1367–70. doi: 10.1001/archinte.163.11.1367
83. Mamby CC, Love RR, Lee KE. Thyroid function test changes with adjuvant tamoxifen therapy in postmenopausal women with breast cancer. (2016) 13(4):854–7. doi: 10.1200/JCO.1995.13.4.854
84. Deyssig R, Weissel M. Ingestion of androgenic-anabolic steroids induces mild thyroidal impairment in male body builders. J Clin Endocrinol Metab (1993) 76(4):1069–71. doi: 10.1210/jc.76.4.1069
85. Emerson CH, Seiler CM, Alex S, Fang SL, Mori Y, Devito WJ. Gene expression and serum thyroxine-binding globulin are regulated by adrenal status and corticosterone in the rat. Endocrinology (1993) 133(3):1192–6. doi: 10.1210/endo.133.3.8365361
86. Stockigt JR, Lim CF, Barlow JW, Wynne KN, Mohr VS, Topliss DJ, et al. Interaction of furosemide with serum thyroxine binding sites: In vivo and in vitro studies and comparison with other inhibitors. J Clin Endocrinol Metab (1985) 60(5):1025–31. doi: 10.1210/jcem-60-5-1025
87. Koizumi Y, Sato A, Yamada T, Inada M. Effect pf mefenamic acid on plasma protein-thyroid hormone interaction, monodeiodination of thyroxine, urinary excretion of tri-iodothyronine and thyrotropin regulation. Clin Exp Pharmacol Physiol (1984) 11(3):291–9. doi: 10.1111/j.1440-1681.1984.tb00267.x
88. Larsen PR. Salicylate-induced increases in free triiodothyronine in human serum: Evidence of inhibition of triiodothyronine binding to thyroxine-binding globulin and thyroxine-binding prealbumin. J Clin Invest (1972) 51(5):1125–34. doi: 10.1172/JCI106905
89. Liel Y, Sperber AD, Shany S. Nonspecific intestinal adsorption of levothyroxine by aluminum hydroxide. Am J Med (1994) 97(4):363–5. doi: 10.1016/0002-9343(94)90303-4
90. Northcutt R, Stiel J, Hollifield J, Stant E. The influence of cholestyramine on thyroxine absorption - PubMed. JAMA (1969) 208(10):1857–61. doi: 10.1001/jama.1969.03160100047012
91. Benvenga S, Bartolone L, Pappalardo MA, Russo A, Lapa D, Giorgianni G, et al. Altered intestinal absorption of l-thyroxine caused by coffee. Thyroid (2008) 18(3):293–301. doi: 10.1089/thy.2007.0222
92. Singh N, Weisler SL, Hershman JM. The acute effect of calcium carbonate on the intestinal absorption of levothyroxine. Thyroid (2001) 11(10):967–71. doi: 10.1089/105072501753211046
93. John-Kalarickal J, Pearlman G, Carlson HE. New medications which decrease levothyroxine absorption. Thyroid (2007) 17(8):763–5. doi: 10.1089/thy.2007.0060
94. Irving SA, Vadiveloo T, Leese GP. Drugs that interact with levothyroxine: an observational study from the thyroid epidemiology, audit and research study (TEARS). Clin Endocrinol (2015) 82(1):136–41. doi: 10.1111/cen.12559
95. Liel Y, Harman-Boehm I, Shany S. Evidence for a clinically important adverse effect of fiber-enriched diet on the bioavailability of levothyroxine in adult hypothyroid patients. J Clin Endocrinol Metab (1996) 81(2):857–9. doi: 10.1210/jc.81.2.857
96. Demke DM. Drug interaction between thyroxine and lovastatin. N Engl J Med (1989) 321(19):1341–2. doi: 10.1056/NEJM198911093211914
97. Mersebach H, Rasmussen ÅK, Kirkegaard L, Feldt-Rasmussen U. Intestinal adsorption of levothyroxine by antacids and laxatives: case stories and in vitro experiments. Pharmacol Toxicol (1999) 84(3):107–9. doi: 10.1111/j.1600-0773.1999.tb00883.x
98. Bell DSH, Ovalle F. Use of soy protein supplement and resultant need for increased dose of levothyroxine. Endocr Pract (2001) 7(3):193–4. doi: 10.4158/EP.7.3.193
99. Sherman SI, Tielens ET, Ladenson PW. Sucralfate causes malabsorption of l-thyroxine. Am J Med (1994) 96(6):531–5. doi: 10.1016/0002-9343(94)90093-0
100. Aanderud S, Myking OL, Strandjord RE. The influence of carbamazepine on thyroid hormones and thyroxine binding globulin in hypothyroid patients substituted with thyroxine. Clin Endocrinol (Oxf) (1981) 15(3):247–52. doi: 10.1111/j.1365-2265.1981.tb00662.x
101. Finucane JF, Griffiths RS. Effect of phenytoin therapy on thyroid function. Br J Clin Pharmacol (1976) 3(6):1041–4. doi: 10.1111/j.1365-2125.1976.tb00355.x
102. Nolan SR, Self TH, Norwood JM. Interaction between rifampin and levothyroxine. South Med J (1999) 92(5):529–31. doi: 10.1097/00007611-199905000-00018
103. Sahajpal R, Ahmed RA, Hughes CA, Foisy MM. Probable interaction between levothyroxine and ritonavir: Case report and literature review. Am J Health Syst Pharm (2017) 74(8):587–92. doi: 10.2146/ajhp160200
104. Basaria S, Cooper DS. Amiodarone and the thyroid. Am J Med (2005) 118(7):706–14. doi: 10.1016/j.amjmed.2004.11.028
105. DeGroot LJ, Hoye K. Dexamethasone suppression of serum T3 and T4. J Clin Endocrinol Metab (1976) 42(5):976–8. doi: 10.1210/jcem-42-5-976
106. Kristensen B, Weeke J. Propranolol-induced increments in total and free serum thyroxine in patients with essential hypertension. Clin Pharmacol Ther (1977) 22(6):864–7. doi: 10.1002/cpt1977226864
107. McCowen K, Garber J, Spark R. Elevated serum thyrotropin in thyroxine-treated patients with hypothyroidism given sertraline. N Engl J Med (1997) 337(14):1010–1. doi: 10.1056/NEJM199710023371415
108. Hein MD, Jackson IMD. Review: Thyroid function in psychiatric illness. Gen Hosp Psychiatry (1990) 12(4):232–44. doi: 10.1016/0163-8343(90)90060-P
109. Sherman SI. Etiology, diagnosis, and treatment recommendations for central hypothyroidism associated with bexarotene therapy for cutaneous T-cell lymphoma. Clin Lymphoma (2003) 3(4):249–52. doi: 10.3816/CLM.2003.n.006
110. Ishihara T, Waseda N, Ikekubo K, Ishihara T, Akamizu T, Imura H, et al. Effect of bromocriptine on serum TSH in euthyroid patients with endocrine disorders. Endocrinol Jpn (1985) 32(5):745–51. doi: 10.1507/endocrj1954.32.745
111. Lee E, Chen P, Rao H, Lee J, Burmelster LA. Effect of acute high dose dobutamine administration on serum thyrotrophin (TSH). Clin Endocrinol (Oxf) (1999) 50(4):487–92. doi: 10.1046/j.1365-2265.1999.00678.x
112. Scanlon MF, Weightman DR, Shale DJ, Mora B, Heath M, Snow MH, et al. Dopamine is a physiological regulator of thyrotrophin (TSH) secretion in normal man. Clin Endocrinol (1979) 10(1):7–15. doi: 10.1111/j.1365-2265.1979.tb03028.x
113. Christensen SE, Weeke J, Ørskov H, Kaal A, Lund E, Jørgensen J, et al. Long-term efficacy and tolerability of octreotide treatment in acromegaly. Metabolism (1992) 41(9):44–50. doi: 10.1016/0026-0495(92)90030-E
114. Porretti S, Giavoli C, Ronchi C, Lombardi G, Zaccaria M, Valle D, et al. Recombinant human GH replacement therapy and thyroid function in a Large group of adult GH-deficient patients: When does l-T4 therapy become mandatory? J Clin Endocrinol Metab (2002) 87(5):2042–5. doi: 10.1210/jcem.87.5.8479
115. Vita R, Saraceno G, Trimarchi F, Benvenga S. A novel formulation of l-thyroxine (L-T4) reduces the problem of l-T4 malabsorption by coffee observed with traditional tablet formulations. Endocrine (2013) 43(1):154–60. doi: 10.1007/s12020-012-9772-2
116. Cappelli C, Castello R, Marini F, Paoletta A, Marchetti M, Saullo M, et al. Adherence to levothyroxine treatment among patients with hypothyroidism: A northeastern Italian survey. Front Endocrinol (201) 9:699. doi: 10.3389/fendo.2018.00699
117. Wiersinga WM. Thyroid hormone replacement therapy. Horm Res Paediatr (2001) 56(Suppl. 1):74–81. doi: 10.1159/000048140
118. Watt T, Groenvold M, Rasmussen ÅK, Bonnema SJ, Hegedüs L, Bjorner JB, et al. Quality of life in patients with benign thyroid disorders. a review. Eur J Endocrinol (2006) 154(4):501–10. doi: 10.1530/eje.1.02124
119. Venditti P, Di Meo S. Thyroid hormone-induced oxidative stress. Cell Mol Life Sci CMLS (2006) 63(4):414–34. doi: 10.1007/s00018-005-5457-9
120. Hennessey JV, Malabanan AO, Haugen BR, Levy EG. Adverse event reporting in patients treated with levothyroxine: Results of the pharmacovigilance task force survey of the American thyroid association, American association of clinical endocrinologists, and the endocrine society. Endocr Pract (2010) 16(3):357–70. doi: 10.4158/EP0362.OR
121. Auten RL, Davis JM. Oxygen toxicity and reactive oxygen species: The devil is in the details. Pediatr Res (2009) 66(2):121–7. doi: 10.1203/PDR.0b013e3181a9eafb
122. Valko M, Izakovic M, Mazur M, Rhodes CJ, Telser J. Role of oxygen radicals in DNA damage and cancer incidence. Mol Cell Biochem (2004) 266(1–2):37–56. doi: 10.1023/B:MCBI.0000049134.69131.89
123. Pacher P, Beckman JS, Liaudet L. Nitric oxide and peroxynitrite in health and disease. Physiol Rev (2007) 87(1):315–424. doi: 10.1152/physrev.00029.2006
124. Halliwell B. Role of free radicals in the neurodegenerative diseases: therapeutic implications for antioxidant treatment. Drugs Aging (2001) 18(9):685–716. doi: 10.2165/00002512-200118090-00004
125. Galle J. Oxidative stress in chronic renal failure. Nephrol Dial Transplant (2001) 16(11):2135–7. doi: 10.1093/ndt/16.11.2135
126. MacNee W. Oxidative stress and lung inflammation in airways disease. Eur J Pharmacol (2001) 429(1–3):195–207. doi: 10.1016/S0014-2999(01)01320-6
127. Kumar V, Prakash J, Khan MY. Antioxidant enzymes in rheumatoid arthritis. J Arthritis (2016) 54(4):206. doi: 10.4172/2167-7921.1000206
128. Gross J, Pitt-Rivers R. The identification of 3:5:3’-l-triiodothyronine in human plasma. Lancet (1952) 1(6705):439–41. doi: 10.1016/S0140-6736(52)91952-1
129. Gross J, Pitt-Rivers R. Physiological activity of 3:5:3’-l-triiodothyronine. Lancet (1952) 1(6708):593–4. doi: 10.1016/S0140-6736(52)90104-9
130. McAninch EA, Bianco AC. The history and future of treatment of hypothyroidism. Ann Intern Med (2016) 164(1):50. doi: 10.7326/M15-1799
131. Braverman LE, Ingbar SH, Sterling K. Conversion of thyroxine (T4) to triiodothyronine (T3) in athyreotic human subjects. J Clin Invest (1970) 49(5):855–64. doi: 10.1172/JCI106304
132. Saravanan P, Chau WF, Roberts N, Vedhara K, Greenwood R, Dayan CM. Psychological well-being in patients on ‘adequate’ doses of l-thyroxine: results of a large, controlled community-based questionnaire study. Clin Endocrinol (2002) 57(5):577–85. doi: 10.1046/j.1365-2265.2002.01654.x
133. Jonklaas J, Davidson B, Bhagat S, Soldin SJ. Triiodothyronine levels in athyreotic individuals during levothyroxine therapy. JAMA (2008) 299(7):769–77. doi: 10.1001/jama.299.7.769
134. Gullo D, Latina A, Frasca F, Moli R, Pellegriti G, Vigneri R. Levothyroxine monotherapy cannot guarantee euthyroidism in all athyreotic patients. PloS One (2011) 6(8):e22552. doi: 10.1371/journal.pone.0022552
135. Escobar-Morreale HF, del Rey FE, Obregón MJ, de Escobar GM. Only the combined treatment with thyroxine and triiodothyronine ensures euthyroidism in all tissues of the thyroidectomized rat. Endocrinology (1996) 137(6):2490–502. doi: 10.1210/endo.137.6.8641203
136. Grozinsky-Glasberg S, Fraser A, Nahshoni E, Weizman A, Leibovici L. Thyroxine-triiodothyronine combination therapy versus thyroxine monotherapy for clinical hypothyroidism: meta-analysis of randomized controlled trials. J Clin Endocrinol Metab (2006) 91(7):2592–9. doi: 10.1210/jc.2006-0448
137. Bianco AC, Salvatore D, Gereben B, Berry MJ, Larsen PR. Biochemistry, cellular and molecular biology, and physiological roles of the iodothyronine selenodeiodinases. Endocr Rev (2002) 23(1):38–89. doi: 10.1210/edrv.23.1.0455
138. Torlontano M, Durante C, Torrente I, Crocetti U, Augello G, Ronga G, et al. Type 2 deiodinase polymorphism (threonine 92 alanine) predicts l-thyroxine dose to achieve target thyrotropin levels in thyroidectomized patients. J Clin Endocrinol Metab (2008) 93(3):910–3. doi: 10.1210/jc.2007-1067
139. Panicker V, Saravanan P, Vaidya B, Evans J, Hattersley AT, Frayling TM, et al. Common variation in the DIO2 gene predicts baseline psychological well-being and response to combination thyroxine plus triiodothyronine therapy in hypothyroid patients. J Clin Endocrinol Metab (2009) 94(5):1623–9. doi: 10.1210/jc.2008-1301
140. Carlé A, Faber J, Steffensen R, Laurberg P, Nygaard B. Hypothyroid patients encoding combined MCT10 and DIO2 gene polymorphisms may prefer l-T3 + l-T4 combination treatment - data using a blind, randomized, clinical study. Eur Thyroid J (2017) 6(3):143–51. doi: 10.1159/000469709
141. Peeters RP, Van Toor H, Klootwijk W, De Rijke YB, Kuiper GGJM, Uitterlinden AG, et al. Polymorphisms in thyroid hormone pathway genes are associated with plasma TSH and iodothyronine levels in healthy subjects. J Clin Endocrinol Metab (2003) 88(6):2880–8. doi: 10.1210/jc.2002-021592
142. Medici M, Van Der Deure WM, Verbiest M, Vermeulen SH, Hansen PS, Kiemeney LA, et al. A large-scale association analysis of 68 thyroid hormone pathway genes with serum TSH and FT4 levels. Eur J Endocrinol (2011) 164(5):781–8. doi: 10.1530/EJE-10-1130
143. Roef GL, Rietzschel ER, De Meyer T, Bekaert S, De Buyzere ML, Van daele C, et al. Associations between single nucleotide polymorphisms in thyroid hormone transporter genes (MCT8, MCT10 and OATP1C1) and circulating thyroid hormones. Clin Chim Acta (2013) 425:227–32. doi: 10.1016/j.cca.2013.08.017
144. Kasztura M, Richard A, Bempong NE, Loncar D, Flahault A. Cost-effectiveness of precision medicine: a scoping review. Int J Public Health (2019) 64(9):1261–71. doi: 10.1007/s00038-019-01298-x
145. Ginn SR, Vilensky JA. Experimental confirmation by sir victor horsley of the relationship between thyroid gland dysfunction and myxedema (2006). Available at: https://home.liebertpub.com/thy.
146. Antonica F, Kasprzyk DF, Opitz R, Iacovino M, Liao XH, Dumitrescu AM, et al. Generation of functional thyroid from embryonic stem cells. Nature (2012) 491(7422):66–71. doi: 10.1038/nature11525
147. Arauchi A, Shimizu T, Yamato M, Obara T, Okano T. Tissue-engineered thyroid cell sheet rescued hypothyroidism in rat models after receiving total thyroidectomy comparing with nontransplantation models (2009). Available at: https://home.liebertpub.com/tea.
148. Bulanova EA, Koudan EV, Degosserie J, Heymans C, Pereira FDAS, Parfenov VA, et al. Bioprinting of a functional vascularized mouse thyroid gland construct. Biofabrication (2017) 9(3):034105. doi: 10.1088/1758-5090/aa7fdd
149. Gabr H, Abo Elkheir WM, Zaki R, Amin A. Thyroid islets tissue engineering: Thyroid organoid implanted intramuscular secretes thyroid hormones in post-thyroidectomy mice - ClinicalKey. Cytotherapy (2018) 20(5):82–3. doi: 10.1016/j.jcyt.2018.02.233
150. Kurmann AA, Serra M, Hawkins F, Rankin SA, Mori M, Astapova I, et al. Regeneration of thyroid function by transplantation of differentiated pluripotent stem cells. Cell Stem Cell (2015) 17(5):527–42. doi: 10.1016/j.stem.2015.09.004
151. Martin A, Valentine M, Unger P, Lichtenstein C, Schwartz AE, Friedman EW, et al. Preservation of functioning human thyroid organoids in the scid mouse: 1. system characterization. J Clin Endocrinol Metab (1993) 77(2):305–10. doi: 10.1210/jcem.77.2.8345031
152. Mastrogiacomo S, Strusi V, Dallatana D, Barbaro F, Zini N, Zamparelli A, et al. Poly-l-lactic acid and poly-ϵ-caprolactone as biomaterials for ex-situ bioengineering of the rat thyroid tissue. Ital J Anat Embryol (2012) 117(2):120–0.
153. Pan J, Li H, Fang Y, Shen YB, Zhou XY, Zhu F, et al. Regeneration of a bioengineered thyroid using decellularized thyroid matrix. Thyroid (2019) 29(1):142–52. doi: 10.1089/thy.2018.0068
154. Ma R, Latif R, Davies TF. Human embryonic stem cells form functional thyroid follicles. Thyroid (2015) 25(4):455. doi: 10.1089/thy.2014.0537
155. Strusi V, Zini N, Dallatana D, Mastrogiacomo S, Parrilli A. Endocrine bioengineering: reconstruction of a bioartificial thyroid lobe using its natural, three-dimensional (3D) stromal / vascular matrix as a scaffold. In 15th international ∓ 14th European congress of endocrinology Florence, Italy. Bristol:Endocrine Abstracts (2012):1586.
156. Strusi V, Zini N, Dallatana D, Parrilli A, Giardino R, Lippi G, et al. Ex situ bioengineering of the rat thyroid using as a scaffold the three-dimensional (3D) decellularized matrix of the glandular lobe: clues to the organomorphic principle. Ital J Anat Embryol (2011) 116(1):180–0.
157. Weng J, Chen B, Xie M, Wan X, Wang P, Zhou X, et al. Rabbit thyroid extracellular matrix as a 3D bioscaffold for thyroid bioengineering: a preliminary in vitro study. Biomed Eng Online (2021) 20(1):1–13. doi: 10.1186/s12938-021-00856-w
158. Yang Y, Opara EC, Liu Y, Atala A, Zhao W. Microencapsulation of porcine thyroid cell organoids within a polymer microcapsule construct. Exp Biol Med (Maywood, NJ) (2017) 242(3):286–96. doi: 10.1177/1535370216673746
159. Yu LM, Liu T, Ma YL, Zhang F, Huang YC, Fan ZH. Fabrication of silk-hyaluronan composite as a potential scaffold for tissue repair. Front Bioeng Biotechnol (2020) 8:1400. doi: 10.3389/fbioe.2020.578988
160. Burton TP, Corcoran A, Callanan A. The effect of electrospun polycaprolactone scaffold morphology on human kidney epithelial cells. Biomed Mater (2017) 13(1):015006. doi: 10.1088/1748-605X/aa8dde
161. Sturtivant A, Callanan A. The use of antifreeze proteins to modify pore structure in directionally frozen alginate sponges for cartilage tissue engineering. Biomed Phys Eng Express (2020) 6(5):055016. doi: 10.1088/2057-1976/aba7aa
162. Gao Y, Callanan A. Influence of surface topography on PCL electrospun scaffolds for liver tissue engineering. J Mater Chem B (2021) 9(38):8081–93. doi: 10.1039/D1TB00789K
163. Nikolova MP, Chavali MS. Recent advances in biomaterials for 3D scaffolds: A review. Bioact Mater (2019) 4:271. doi: 10.1016/j.bioactmat.2019.10.005
164. Chan BP, Leong KW. Scaffolding in tissue engineering: general approaches and tissue-specific considerations. Eur Spine J (2008) 17(Suppl 4):467. doi: 10.1007/s00586-008-0745-3
165. De Felice M, Di Lauro R. Minireview: Intrinsic and extrinsic factors in thyroid gland development: an update. Endocrinology (2011) 152(8):2948–56. doi: 10.1210/en.2011-0204
166. Bürgi-Saville ME, Gerber H, Peter HJ, Paulsson M, Aeschlimann D, Glaser C, et al. Expression patterns of extracellular matrix components in native and cultured normal human thyroid tissue and in human toxic adenoma tissue (2009). Available at: https://home.liebertpub.com/thy.
167. Liao J, Xu B, Zhang R, Fan Y, Xie H, Li X. Applications of decellularized materials in tissue engineering: advantages, drawbacks and current improvements, and future perspectives. J Mater Chem B (2020) 8(44):10023–49. doi: 10.1039/D0TB01534B
168. Gilpin A, Yang Y. Decellularization strategies for regenerative medicine: From processing techniques to applications. BioMed Res Int (2017) 2017:9831534–13. doi: 10.1155/2017/9831534
169. Vanderpump MPJ, Tunbridge WMG, French JM, Appleton D, Bates D, Clark F, et al. The incidence of thyroid disorders in the community: a twenty-year follow-up of the whickham survey. Clin Endocrinol (Oxf) (1995) 43(1):55–68. doi: 10.1111/j.1365-2265.1995.tb01894.x
170. Santin AP, Furlanetto TW. Role of estrogen in thyroid function and growth regulation. J Thyroid Res (2011) 2011:875125–7. doi: 10.4061/2011/875125
171. Okamura K, Nakashima T, Ueda K, Inoue K, Omae T, Fujishima M. Thyroid disorders in the general population of hisayama Japan, with special reference to prevalence and sex differences. Int J Epidemiol (1987) 16(4):545–9. doi: 10.1093/ije/16.4.545
172. ALtucci L, Addeo R, Cicatello L, Dauvois S, Parker M, Truss M, et al. 17beta-estradiol induces cyclin D1 gene transcription, p36D1-p34cdk4 complex activation and p105Rb phosphorylation during mitogenic stimulation of G(1)-arrested human breast cancer cells. Oncogene (1996) 12(11):2315–24.
173. Okita K, Ichisaka T, Yamanaka S. Generation of germline-competent induced pluripotent stem cells. Nature (2007) 448(7151):313–7. doi: 10.1038/nature05934
174. Sadtler K, Wolf MT, Ganguly S, Moad CA, Chung L, Majumdar S, et al. Divergent immune responses to synthetic and biological scaffolds. Biomaterials (2019) 192:405–15. doi: 10.1016/j.biomaterials.2018.11.002
175. Mariani E, Lisignoli G, Borzì RM, Pulsatelli L. Biomaterials: Foreign bodies or tuners for the immune response? int J mol sci (2019). Available at: https://pubmed.ncbi.nlm.nih.gov/30717232/.
176. Turner NJ, Badylak SF. The use of biologic scaffolds in the treatment of chronic nonhealing wounds. Adv Wound Care (2015) 4(8):490. doi: 10.1089/wound.2014.0604
177. Badylak SF, Freytes DO, Gilbert TW. Extracellular matrix as a biological scaffold material: Structure and function. Acta Biomater (2009) 5(1):1–13. doi: 10.1016/j.actbio.2008.09.013
178. Hotaling NA, Tang L, Irvine DJ, Babensee JE. BIOMATERIAL STRATEGIES FOR IMMUNOMODULATION. Annu Rev BioMed Eng (2015) 17:317. doi: 10.1146/annurev-bioeng-071813-104814
179. Keselowsky BG, Collard DM, García AJ. Surface chemistry modulates focal adhesion composition and signaling through changes in integrin binding. biomaterials (2004). Available at: https://pubmed.ncbi.nlm.nih.gov/15183609/.
180. Babu S, Albertino F, Omidinia Anarkoli A, de Laporte L. Controlling structure with injectable biomaterials to better mimic tissue heterogeneity and anisotropy. advanced healthcare materials (2021). Available at: https://onlinelibrary.wiley.com/doi/full/10.1002/adhm.202002221.
181. Tang G, Tan Z, Zeng W, Wang X, Shi C, Liu Y, et al. Recent advances of chitosan-based injectable hydrogels for bone and dental tissue regeneration. Front Bioeng Biotechnol (2020) 8:1084. doi: 10.3389/fbioe.2020.587658
182. Bocanera LB, Aphalo P, Pisarev MA, Gärtner R, Silberschmidt D, Juvenal GJ, et al. Presence of a soluble inhibitor of thyroid iodination in primary cultures of thyroid cells. Eur J Endocrinol (1999) 141(1):55–60. doi: 10.1530/eje.0.1410055
183. Samimi H, Atlasi R, Parichehreh-Dizaji S, Khazaei S, Rahnama MA, Seifirad S, et al. A systematic review on thyroid organoid models: time-trend and its achievements. Am J Physiol Endocrinol Metab (2021) 320(3):581–90. doi: 10.1152/ajpendo.00479.2020
184. Prabhune M, Belge G, Dotzauer A, Bullerdiek J, Radmacher M. Comparison of mechanical properties of normal and malignant thyroid cells. Micron. (2012) 43(12):1267–72. doi: 10.1016/j.micron.2012.03.023
185. Gervaso F, Sannino A, Peretti GM. The biomaterialist’s task: scaffold biomaterials and fabrication technologies. Joints (2013) 1(3):130. doi: 10.11138/jts/2013.1.3.130
186. Boccaccini AR, Blaker JJ. Bioactive composite materials for tissue engineering scaffolds. Expert Rev Med Devices (2005) 2(3):303–17. doi: 10.1586/17434440.2.3.303
187. Reddy MSB, Ponnamma D, Choudhary R, Sadasivuni KK. A comparative review of natural and synthetic biopolymer composite scaffolds. Polym (Basel) (2021) 13(7):1105. doi: 10.3390/polym13071105
188. Kort-Mascort J, Bao G, Elkashty O, Flores-Torres S, Munguia-Lopez JG, Jiang T, et al. Decellularized extracellular matrix composite hydrogel bioinks for the development of 3D bioprinted head and neck in vitro tumor models. ACS Biomater Sci Eng (2021) 7(11):5288–300. doi: 10.1021/acsbiomaterials.1c00812
189. Cheung HK, Han TTY, Marecak DM, Watkins JF, Amsden BG, Flynn LE. Composite hydrogel scaffolds incorporating decellularized adipose tissue for soft tissue engineering with adipose-derived stem cells. Biomaterials (2014) 35(6):1914–23. doi: 10.1016/j.biomaterials.2013.11.067
Keywords: thyroid, hypothyroidism, regenerative medicine, tissue engineering, levothyroxine
Citation: Heim M, Nixon IJ, Emmerson E and Callanan A (2022) From hormone replacement therapy to regenerative scaffolds: A review of current and novel primary hypothyroidism therapeutics. Front. Endocrinol. 13:997288. doi: 10.3389/fendo.2022.997288
Received: 18 July 2022; Accepted: 09 September 2022;
Published: 05 October 2022.
Edited by:
Rauf Latif, Icahn School of Medicine at Mount Sinai, United StatesReviewed by:
Yuji Nagayama, Nagasaki University, JapanShioko Kimura, National Institutes of Health (NIH), United States
Copyright © 2022 Heim, Nixon, Emmerson and Callanan. This is an open-access article distributed under the terms of the Creative Commons Attribution License (CC BY). The use, distribution or reproduction in other forums is permitted, provided the original author(s) and the copyright owner(s) are credited and that the original publication in this journal is cited, in accordance with accepted academic practice. No use, distribution or reproduction is permitted which does not comply with these terms.
*Correspondence: Anthony Callanan, QW50aG9ueS5jYWxsYW5hbkBlZC5hYy51aw==