- 1University of Cambridge, Girton College, Cambridgeshire, United Kingdom
- 2Singapore Eye Research Institute, Singapore National Eye Centre, Singapore, Singapore
- 3Tissue Engineering and Cell Therapy Group, Singapore Eye Research Institute, Singapore, Singapore
- 4Cornea and Refractive Surgery Group, Singapore Eye Research Institute, Singapore, Singapore
- 5Ophthalmology and Visual Sciences Academic Clinical Program, Duke-NUS Medical School, Singapore, Singapore
- 6Department of Ophthalmology, National Taiwan University, Taipei, Taiwan
Diabetes mellitus is a global public health problem with both macrovascular and microvascular complications, such as diabetic corneal neuropathy (DCN). Using in-vivo confocal microscopy, corneal nerve changes in DCN patients can be examined. Additionally, changes in the morphology and quantity of corneal dendritic cells (DCs) in diabetic corneas have also been observed. DCs are bone marrow-derived antigen-presenting cells that serve both immunological and non-immunological roles in human corneas. However, the role and pathogenesis of corneal DC in diabetic corneas have not been well understood. In this article, we provide a comprehensive review of both animal and clinical studies that report changes in DCs, including the DC density, maturation stages, as well as relationships between the corneal DCs, corneal nerves, and corneal epithelium, in diabetic corneas. We have also discussed the associations between the changes in corneal DCs and various clinical or imaging parameters, including age, corneal nerve status, and blood metabolic parameters. Such information would provide valuable insight into the development of diagnostic, preventive, and therapeutic strategies for DM-associated ocular surface complications.
1. Diabetes mellitus and diabetic corneal neuropathy
Diabetes mellitus (DM), characterised by elevated levels of blood glucose resulting from defective insulin secretion and/or action, has emerged to become a major global public health problem (1). In 2021, 537 million adults were living with diabetes, and estimably 6.7 million adults have died because of DM or its complications (2). The estimated global cost of diabetes was projected to increase from US$1.31 trillion in 2015 to $2.1 trillion in 2030 (3). DM is associated with both macrovascular complications, such as cardiovascular disorders, and microvascular complications, including diabetic peripheral neuropathy (DPN) (4, 5). The manifestation of DPN in the cornea is referred to as diabetic corneal neuropathy, leading to diabetic keratopathy.
DCN is characterized by changes in corneal nerve fibres and occurs in 47-64% of patients during their clinical course of DM (6, 7). When evaluating corneal nerve changes in DCN, in-vivo confocal microscopy (IVCM) has been considered the gold standard. In vivo cell imaging uses light reflected from within the tissue, gathering information to aid the recognition of inter- and intracellular details (8). Different from conventional microscopy where the image can be observed directly, confocal microscopes obtain increased resolution by limiting the illumination and observation systems to a single point. Hence, to reconstruct a full field of view and allow for “real-time” viewing, rapid scanning is used for IVCM (8, 9). IVCM produces high-resolution images at a cellular level with a magnification of 600-800 times, a lateral image resolution of 1-2μm, and an axial resolution of 5-10 μm (10). Post-imaging quantitative evaluations of corneal nerve plexus can be done manually, in a semi-automated manner, or a completely automated manner using certain analytic software (5, 11). Numerous studies have reported IVCM findings of reduced corneal nerve fibre density (CNFD), corneal nerve fibre length (CNFL), and corneal nerve branch density (CNBD) in patients with type 1 diabetes mellitus (T1D) or type 2 diabetes mellitus (T2D) (Figures 1A, B) (5). A reduction in nerve beading frequency is also observed, indicating a decrease in nerve metabolic activity and an increase in the risk of neuronal damage (7). In addition, patients with T1D or T2D present with an increase in nerve fibre tortuosity, reflecting a degenerative and subsequent attempted regenerative nerve response (Figure 1C) (12, 13). Besides nerve changes in the central and peripheral cornea, an earlier reduction in CNFL and CNBD of the subbasal inferior whorl of the corneal nerves, located in the inferonasal cornea, is also reported, serving as an imaging site for early detection of DCN (Figures 1D, E) (5, 7, 14). Moreover, patients with T1D have a lower corneal nerve fractal dimension (CNFrD) compared to control subjects, suggesting a less healthy and less evenly-distributed nerve fibre network in patients with T1D (7). Changes in the morphology and quantity of corneal dendritic cells (DCs) in diabetic corneas were also observed in several studies (15). However, the role and pathogenesis of the accumulation of the DCs have been not well understood.
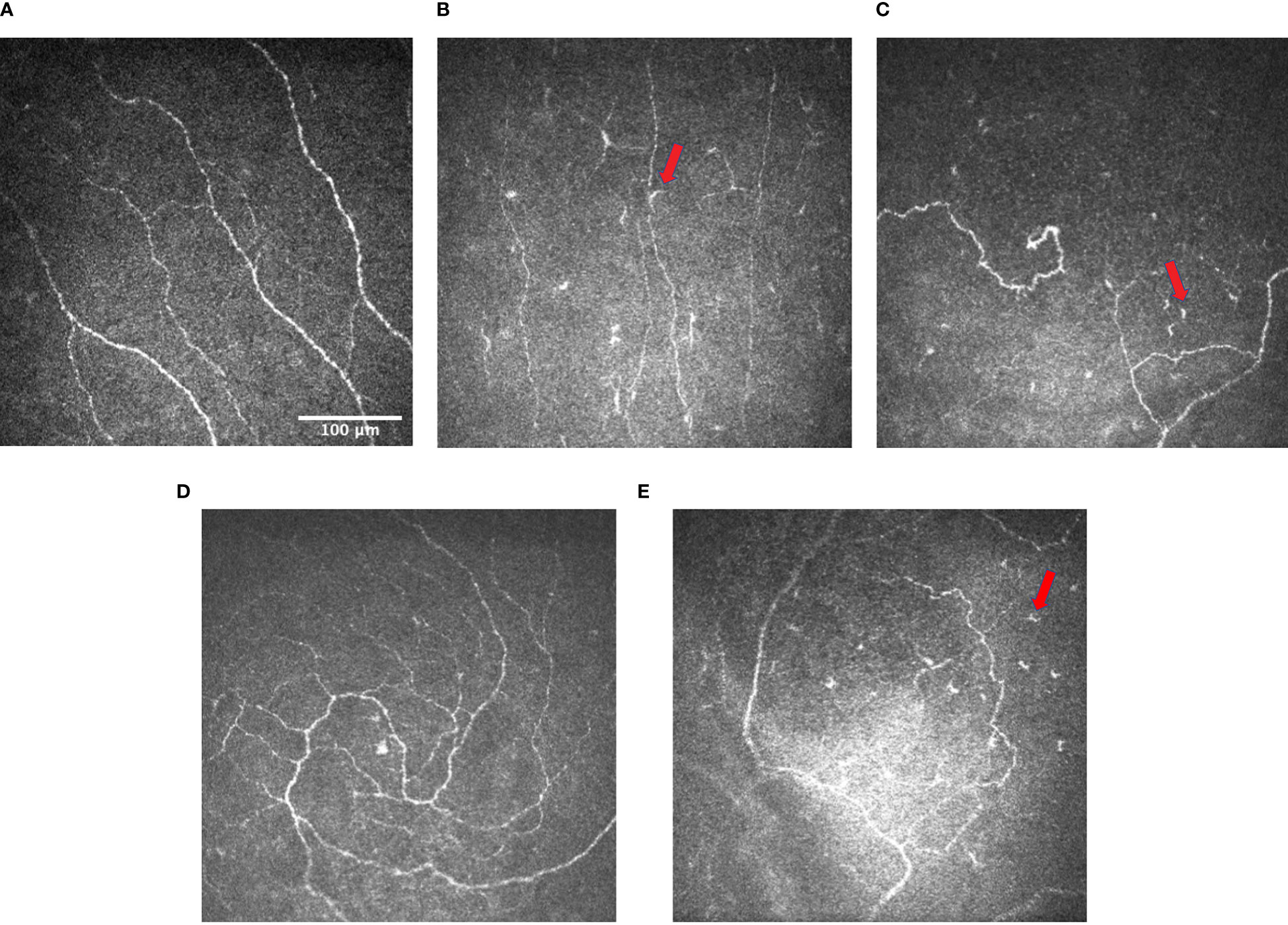
Figure 1 Representative IVCM images of (A) subbasal nerve plexus in normal controls (image taken from the subtemporal quadrant of the cornea of a middle-aged patient) (CNBD: 12.4992 no./mm2); (B) subbasal nerve plexus with decreased corneal nerve fiber length and density in patients with DM, and the presence of dendritic cells (arrows) (CNBD: 0 no./mm2) (C) subbasal nerve plexus with increased tortuosity and the presence of dendritic cells (arrows) in patients with DM (CNBD: 18.7488 no./mm2) (D) inferior whorl of corneal nerves in normal controls (CNBD: 43.7472 no./mm2); and (E) inferior whorl of corneal nerves in patients with DM showing the reduction in corneal nerve fiber length and density and the presence of dendritic cells (arrows) at the inferior whorl (CNBD: 6.2496 no./mm2). Images were produced via the Heidelberg retina tomograph (HRT) Corneal Module (Heidelberg Engineering, Heidelberg, Germany), laser scanning confocal microscopy.
2. Dendritic cells in normal corneas
DCs are bone marrow-derived antigen-presenting cells (APCs) that act as the initiator and modulator of immune responses (16). They are distinguished from other immune cell types through their cytoplasmic extensions (the dendrites), poor phagocytic activity, and the scarcity of their intracellular organelles (16). The most notable function of the DC family is to initiate primary T-lymphocyte-mediated immunity in response to an antigenic stimulus (17). This is achieved mainly through three functions of DCs: (a) capturing and presentation of antigens as sentinel cells; (b) migrating and binding to the antigen-specific T cells in lymphoid organs, and (c) activating T-cells and inducing their growth and proliferation (17).
2.1. Distributions of corneal dendritic cells
Naïve corneas were originally considered to lack the antigen-presenting system of DCs, contributing to their immune-privileged nature (18). However, more recent studies have shown a significant population of different subtypes of DCs residing in the cornea, with the number of which decreasing from the periphery towards the centre (19–22). Among the peripheral regions of the cornea, the inferior region has the highest density of DCs, followed by the superior region and the nasal region, while the temporal region has the lowest (22). In general, DCs can be subdivided into three main groups: the conventional DCs (cDCs), the plasmacytoid DCs (pDCs), and the monocyte-derived DCs (moDCs) (23). Such DCs subpopulations are defined based on their ontogeny, functional specialisation, and the requirement of specific transcription factors (TF) for the development (24). Different subtypes of corneal DCs are found in corneal epithelium and anterior stroma respectively (25, 26). Langerhans Cells (LCs), historically considered a subtype of conventional DCs (cDC), are observed in the periphery and centre of both human and murine corneal epithelium (25–29). However, the classification of LCs remains a topic with ongoing debate, since LCs were found to share properties with both DCs and macrophages. It has been argued by some that LCs may be considered a pecialized subset of tissue-resident macrophages based on their shared developmental origin (30, 31). Indeed, common DC precursors were found not to give rise to epidermal LCs. However, LCs share a remarkable number of functions with DCs, including migration to lymph nodes, and T-cell stimulation (31). The use of the term “LCs” has not been consistent across IVCM studies, and some other terms, such as APCs, dendritiform cells, or immune cells have also been used (28). Besides corneal epithelium, the anterior corneal stroma is also endowed with a different population of cDCs, namely the interstitial DCs. The interstitial DCs are primarily located in peripheral and paracentral regions of the anterior stroma with some toward the central anterior stroma in both murine and human cornea (25, 26, 29, 32). More recently, plasmacytoid dendritic cells (pDCs) have also been observed in the anterior stroma as well as epithelium in both the central and peripheral cornea of mice and human cadaver (29, 33–35).
2.2. Functions of corneal dendritic cells
Normally, mature DCs have developed dendrites that are absent in immature DCs (28). Unlike mature DCs, immature DCs lack the requisite accessory signals for T-cell activation, such as CD40, CD80, and CD86. To induce maturation of the dormant immature DCs, signals in the extracellular milieu through inflammatory mediators are needed (32). The distribution of corneal DCs at different maturation stages in the human cornea remains an issue of ongoing discussion. Some are consistent with the murine studies, which reported immature LCs in the centre of corneal epithelium, and both mature and immature LCs in the peripheral corneal epithelium (22, 27). Others demonstrated few mature LCs and interstitial DCs in epithelium and stroma respectively in both the peripheral and central cornea (25). The differences may have arisen from several reasons, potentially including different maturation markers and different models (in-vivo or ex-vivo) used (25, 27).
DCs serve both immunological and non-immunological roles in human cornea. The primary function of DCs in the cornea is to induce and amplify immunoinflammatory responses (18, 36). During the inflammatory process triggered by infection or allergy, the release of pro-inflammatory cytokines, such as interleukin (IL)-1, tumour necrosis factor (TNF)-α, CD40L, and lipopolysaccharide, or heat-shock proteins from dying cells, facilitates the activation of LCs/DCs in the cornea (20, 21). Resultingly, surface expression of co-stimulatory molecules (CD80/CD86) and CD40 is increased by DCs/LCs in the peripheral cornea, as well as acquired de novo by immature DCs/LCs in the central cornea (37). The activated corneal LCs/DCs function as APCs by transporting the antigens to lymphoid organs and presenting them to effector or memory T cells, priming the T cells for the antigen-specific adaptive immune response (18, 36, 37). Resident corneal DCs are considered long-lived, though it is still uncertain whether during the steady state, the corneal DCs self-regenerate through mitosis, emerge from tissue-resident precursors, or are recruited from the circulating blood (38–40). Nonetheless, in the presence of inflammatory stimuli and increased chemokine/cytokine levels in the cornea, corneal DCs are increased, at least partially through the recruitment of DC precursors from the blood (26, 38).
The non-immunological function of LCs/DCs is associated with tissue repair, through partnering with surrounding corneal epithelial cells. Upon injury, corneal intraepithelial LCs/DCs are activated either directly through recognition of danger signals, or indirectly from cytokines and chemokines secreted by epithelial cells in the injury site. The activated LCs/DCs modulate the migration, proliferation, and survival of epithelial cells in the wounding area via either cell-to-cell contact or the release of survival and growth factors (41). The epithelial cells, in turn, further activate corneal LCs/DCs and recruit them into the wound bed via epithelia-generated mediators (41).
It Is worth noting that although DCs and macrophages were historically regarded as two distinct types of immune cells, the classifications of DCs and macrophages have recently been challenged and remain a topic of ongoing discussion (42, 43). Due to some shared surface markers and functional parameters between renal DCs and macrophages in both acute renal injury and chronic immune-mediated kidney disease (42–46). It was argued that the functional and phenotypic definitions of these two cell types, especially in the kidney, overlap greatly (42). Therefore, an improved classification system may be needed to better facilitate future research work (42, 44).
3. In-vivo confocal microscopy (IVCM) evaluation on corneal DCs
As IVCM can provide images at the cellular level, it has been used to evaluate the DC morphology and distribution (27, 47). Using IVCM, changes in corneal DCs have been observed in ocular surface diseases including dry eye disease and infectious keratitis, as well as systemic disorders including DM, multiple sclerosis, rheumatoid arthritis, ankylosing spondylitis, and systemic lupus erythematosus (15, 48–51). On IVCM evaluation, corneal epithelial DCs present as bright corpuscular particles and a diameter of up to 15μm (27). The presence of Birbeck granules, a type of cytoplasmic marker granules, distinguishes LCs from other DCs (27). Currently, phenotypic classification of corneal epithelial DCs is achieved mainly through morphological differences (49). The DCs morphology can be evaluated according to a 0-3 scale based on the size of the dendrites compared to the largest diameter of the cell body (Figure 2): A score 0 indicates an absence of DCs; a score 1 indicates the presence of DCs without processes; a score 2 indicates the presence of DCs with small processes, the length of which does not exceed the largest diameter of the cell body; a score 3 indicates the presence of DCs with long processes, the length of which exceeds the largest diameter of the cell body (48, 52). Longer processes and smaller cell bodies in DCs indicate a higher level of maturation and potential activity (48, 52).
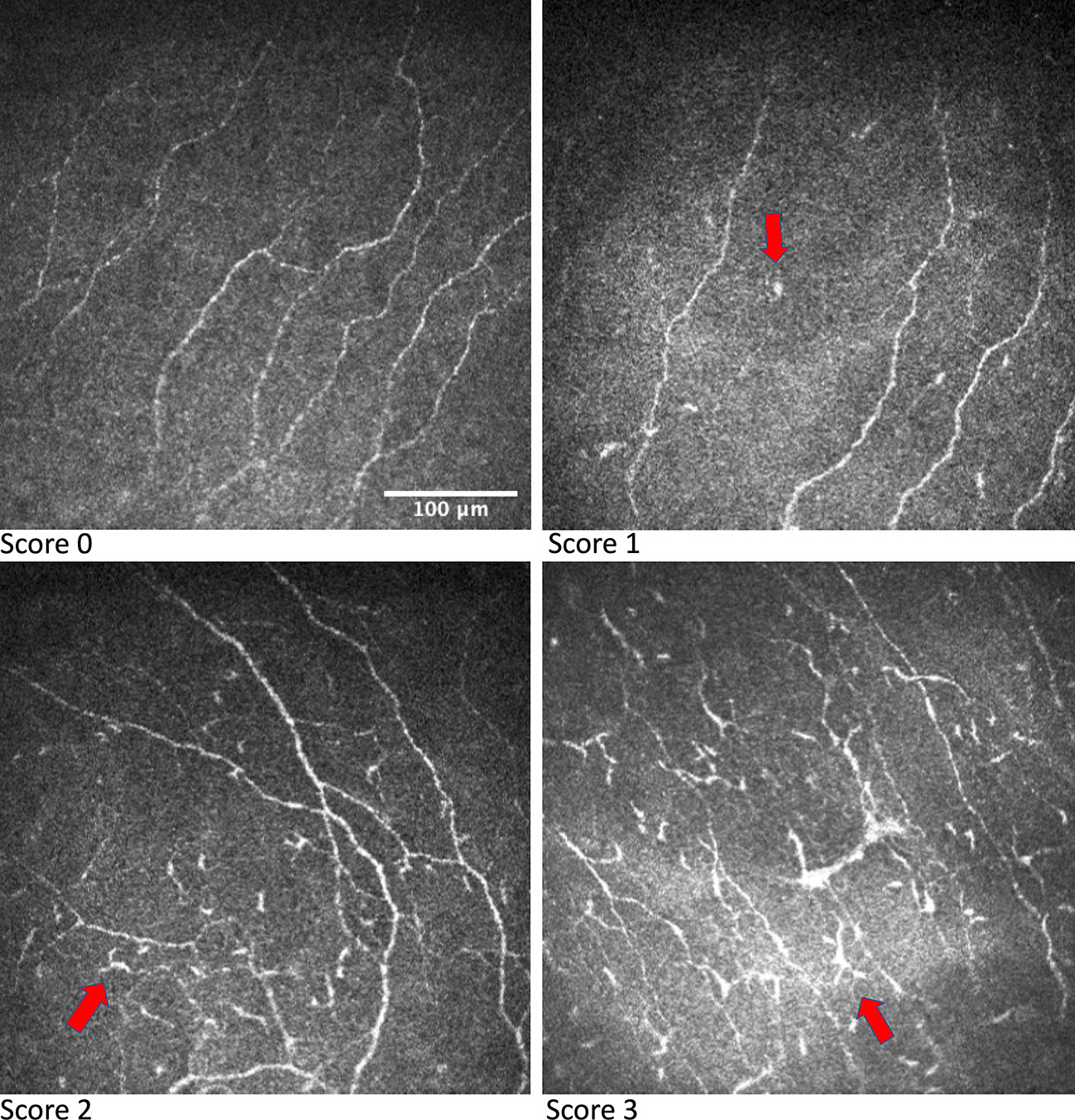
Figure 2 IVCM images showing grading of DC morphology. DCs are indicated by the arrow in respective IVCM images. Images were produced via the Heidelberg retina tomograph (HRT) Corneal Module (Heidelberg Engineering, Heidelberg, Germany), laser scanning confocal microscopy.
4. Search strategy and selection criteria
The authors conducted a search on the online database PubMed Central, Google Scholar, and Science Direct for relevant articles that describe the changes in corneal dendritic cells in subjects with T1D/T2D or the relationship between corneal DCs and clinical or corneal imaging parameters in patients with T1D/T2D.
Articles were included up to May 2022. Keywords included but were not limited to “diabetes” AND “hyperglycaemia” AND “corneal dendritic cells” OR “corneal Langerhans cells” AND “corneal nerve” AND “corneal neuropathy” AND “corneal epithelial cells” AND “ag”“ AND “diabetes duration” AND “blood metabolic profile”. Our review only examined papers written in English, and we restricted the date of publication to the most recent ten years as much as possible. We have also extracted relevant articles from the bibliographies of the existing articles. The authors then manually screened the abstracts and shortlisted papers based on our inclusion criteria. The full-text version of all selected articles was further examined.
5. Changes in corneal dendritic cells in patients with DM
DM affects multiple ocular tissues, including the cornea (49). Studies have examined the changes in DCs, including the DC density, maturation stages, as well as relationships between the corneal DCs, corneal nerves, and corneal epithelium, in subjects with T1D/T2D (15, 53–60). Corneal DCs may serve as a biomarker for DM-associated ocular surface complications, such as diabetic corneal neuropathy (54).
5.1. Changes of corneal DC density in DM
Changes in DCs density in subjects with T1D/T2D have been investigated and remain a topic of ongoing discussion. The majority of animal and clinical studies reported an increase in DCs density in subjects with T1D/T2D, while a few presented the opposite (15, 53–59). The literature review on this topic is summarised in Table 1.
When mice models are used to investigate T1D, streptozotocin (STZ) is commonly used to induce hyperglycemia through the destruction of pancreatic β-cells (15, 59, 62). Via corneal IVCM and corneal in vitro whole-mounts confocal microscopy (WMCM), a constant increase of corneal DC density over 9 weeks upon diabetes induction was observed in one murine study (15). Contrastingly, another study using WMCM observed a significantly lower number of DCs in corneas from STZ-induced mice with T1D compared to controls (59). On the other hand, Lepob/ob mice are often used as mouse models of T2D (62). One murine study investigating mice with T2D reported that the dendritic cell density in type 2 diabetic mice was 3-fold higher than in non-diabetic mice (15).
In clinical studies, the DC density was quantified in patients with T1D or T2D using IVCM. Compared to healthy controls, significantly higher DC density was observed in patients with T1D or T2D, as well as in patients with T1D/T2D and peripheral/somatic neuropathy or corneal punctate epitheliopathy (53–58). Among patients with T1D/T2D and peripheral/somatic neuropathy, DC density was significantly higher in patients with no or mild peripheral/somatic neuropathy compared to non-diabetic controls. However, with the progression of peripheral neuropathy, the DC density was reduced in patients with T1D/T2D and moderate or severe neuropathy, though remained above control values. The authors proposed that DCs might be only involved in the early phases of nerve degeneration whereas the later phase of nerve damage in DM may be maintained by other factors, including glucose neurotoxicity (55, 63). The DC density in patients with T2D and corneal punctate epitheliopathy was also investigated (57, 58). A significantly higher DC density in all corneal areas was observed in both groups of type 2 diabetic patients with and without punctate epitheliopathy compared to healthy controls. In another study where the authors compared the DC density in patients with punctate epitheliopathy resulting from T2D or other causes, a significantly higher DC density was found in the former group, suggesting an association between T2D status and DC density (58).
There are several mechanisms proposed for the increase of DC density in DM populations. Though DM is characterised by elevated levels of blood glucose, it is suggested that the increase in DC might be unrelated to hyperglycaemia as no correlation between DC density and glycaemic control was observed (57). Instead, the increase of DCs in patients with T1D/T2D may be deemed as a cellular response to inflammation. Diabetes, especially T2D, has been suggested to be a pro-inflammatory cytokine-associated disease, involving both the innate and adaptive immune systems (60). There are several pathogeneses involved in the inflammatory state of T2D, including tissue hypoxia, cell death of expanding adipose tissue, activation of interleukins, and nuclear factor (NF)-κB pathways, contributing to the recruitment and activation of immune cells (5, 7, 64). For example, the NF-κB signaling pathway may be activated via the interaction of advanced glycation end-products (AGEs) and its cognate receptor for advanced glycation end-products (RAGE), subsequently promoting the secretion of TNF-α, IL-1β, IL-6, and other pro-inflammatory cytokines (65–68). Significantly increased levels of various AGEs compounds have been reported in both type 1 and type 2 diabetic patients, resulting from non-enzymatic glycation and oxidation of proteins and lipids (65, 69–75). The mechanism for the increased DC density is supported by the observations that corneal DC infiltration and maturation are induced when inflammatory stimuli like electric cautery, lipopolysaccharide, and tumour necrosis factor-α are applied to the ocular surface (26, 76). Findings by another study are also in line with this proposed mechanism where the DC density in the cornea increased by a factor of approximately eight during immune-mediated corneal inflammation secondary to an infection, allergy, or corneal graft rejection (77).
On the contrary, some literature showed the opposite findings in which decreased corneal DCs were observed in both animal models and patients with T1D/T2D (59, 78, 79). One proposed explanation is that prolonged exposure to hyperglycaemia may cause DC apoptosis, reducing the DCs density (80). Similar observations in other immune cells, such as increased apoptosis in neutrophils as well as impaired antigen presentation by monocytes, have also been reported under chronic hyperglycaemic conditions (81). Such observation might also be attributed to the different imaging techniques used.
5.2. Changes in maturation stages of corneal DCs in DM
Besides the density changes, changes in the maturation stages of DCs are reported in DM. Through wide-area three-dimensional mosaic projections of the corneal subbasal nerve plexus, a doubling in mature DCs (mDCs) proportion, as well as a proportional decrease in immature DCs (imDCs), were observed in patients with T2D (60). This finding suggests that the maturation of corneal DCs occurs as T2D develops. This is also supported by another study where the authors reported a higher percentage of patients with T1D/T2D/latent autoimmune diabetes of adults (LADA) (95%) with mature DCs in their central cornea compared to healthy controls (65%), while immature DCs can be found in all participants, including patients with T1D/T2D/LADA and controls (54).
It is proposed that tumour necrosis factor receptor super family member 9 (TNFRSF9) acts as a key contributor to the changes in the maturation stages of corneal DCs, by promoting the maturation and survival of DCs (60). Out of 92 plasma proteins analysed in a clinical study, TNFRSF9 was associated with the observed maturation of DCs from an immature to mature antigen-presenting phenotype. There was a significant association between TNFRSF9 and the proportion of mDC, and TNFRSF9 was also inversely correlated with the imDC proportion (60). TNFRSF9 is found to be expressed on immune cells including activated and regulatory T-cells and activated natural killer (NK) cells (82–84). Hence, when T cells are activated with the onset of T2D, it subsequently induces the expression of TNFRSF9, which further promotes the maturation of the DCs (60). Besides TNFRSF9, the involvement of AGEs in regulating the maturation of DCs has also been reported in both in vitro studies of human tissue and in vivo studies of diabetic mice with myocardial infarction (85, 86). The maturation of DCs in patients with T1D/T2D may be induced by the increased level of AGE through promoting the expressions of scavenger receptor-A (SR-A) and RAGE, via the Jnk pathway. Such a mechanism has been proposed in patients with atherosclerosis (86).
6. Relationship between corneal DCs and clinical or corneal imaging parameters
Studies have reported the associations between the changes in corneal DCs and various clinical or corneal nerve imaging parameters, including age, corneal nerve status, and blood metabolic parameters (15, 53–58, 87). Such associations may contribute to the current understanding of DM, further helping the development of diagnostic measures and biomarkers, as well as preventive and therapeutic strategies (54, 88, 89).
6.1. Relationship between corneal DCs and age
In healthy individuals, corneal DC density was reported to be independent of age by a meta-regression analysis (90). On the contrary, a significant and positive correlation between the DC density and age was observed in patients with T1D/T2D (55) (56). Moreover, specific to children with T1D, a significant positive correlation was found between the pubertal stage and the mature DC density, immature DC density, as well as to total DC density (56). These findings indicate that age may be a potential differential risk of DM and DM-associated ocular surface complications (56).
Besides the DC density, the age of the patients with T1D was also reported to be inversely correlated with the displacement of DCs without dendrites (woDCs), as well as the woDCs’ persistence ratio (53). DC displacement was calculated as the straight-line distance between the start and end positions of a DC divided by the total time of movement. The authors further proposed that faster DC movements represent healthier DC behaviour and that reduced DC migration in older patients may contribute to age-associated immune dysfunction (53, 91, 92).
However, it is not entirely known how or whether the observed correlations between age and the corneal DC parameters in patients with T1D/T2D are involved or if they are influenced by the pathogenesis of diabetes. Aging has been linked to diabetes through several mechanisms, including age-associated insulin resistance and age-dependent disruption of insulin production (93, 94). Given that both the prevalence and incidence of T2D have been reported to increase dramatically as a function of age, further understanding of the mechanisms underpinning this differential risk is of great importance in the development of age-appropriate preventive and therapeutic strategies (88, 89).
6.2. Associations between corneal DCs and corneal nerves
Diabetes may perturb the interaction between DCs and other structures, especially corneal nerves (80). In the confocal images of mouse corneas stained with CD11c (inflammatory marker) and β-tubulin 3 (neuronal marker), intimate contacts between the DC body and its processes with sensory nerve endings were observed (59). It has also been demonstrated that DCs may be involved in diabetic nerve degeneration, yet whether DCs are neuroprotective or neurotoxic remains unclear with contrasting findings (49, 55).
Corneal nerve degeneration in DM may be associated with an increased DCs density (15). In STZ-induced type 1 diabetic mice, a significant negative correlation was reported between the corneal nerve fiber length and DC density (15). It was also observed that the density of DCs was higher in patients with T1D/T2D and no or mild peripheral neuropathy compared to those with moderate and severe peripheral neuropathy. The authors then proposed that DCs might be involved in the initial phase of nerve damage (55). This theory was further evidenced by the findings of other clinical studies. In patients with T1D/T2D, a significant negative correlation between increased DC density and corneal nerve fibre density, branch density, as well as fibre length was observed, suggesting a potential interaction between activated DCs and corneal nerve fibre degeneration (54, 58). Moreover, in adults with T1D or T2D with or without punctate epitheliopathy, a significant negative correlation was reported between the corneal nerve fiber length and DC density, specifically immature DC density for type 1 diabetic patients (15, 54, 57–59). An inverse correlation between the total DC density and corneal nerve total branch density was also reported in patients with T1D (54). In the immune-neuron crosstalk between nerves and DCs, cells from the neuroendocrine systems recognise the cytokines produced by immune cells. Reciprocally, the immune cells recognise the neurotransmitters and neuropeptides produced by the corneal nerves (95). It is speculated that the DC-nerve interaction in the cornea may be analogous to the neuro-immune axis in the skin and the gut (49, 80). For example, it was demonstrated that calcitonin gene-related peptide (CGRP)-containing nerve fibres were intimately associated with DCs and that CGRP could inhibit antigen presentation by epidermal DCs (96). The tolerogenic and immunomodulatory effects of many neuropeptides have also been previously indicated, which, in their absence due to damages to corneal nerves could lead to enhanced immune response, including increased DC density (97).
Contrary to the previous discussion, some studies found that nerve degeneration in DM may be associated with reduced DCs density (59). In STZ-induced type 1 diabetic mice with corneal epithelial debridement wounds, a reduced number of infiltrating DCs, as well as delayed sensory nerve regeneration, were observed (59). Though these observations were opposite from the findings of most other studies reporting on the same matter, the authors suggested a possible explanation (15, 54, 58). It is postulated that DCs may mediate corneal nerve innervation and regeneration through ciliary neurotrophic factor (CNTF). In the cornea, DCs are the major source of CNTF (59). It was demonstrated in mice with T1D that injection of CNTF-neutralising antibodies delayed nerve-ending regeneration, while exogenous CNTF accelerated nerve regeneration in corneas with local DCs depleted (59). Moreover, blocking the CNTF-specific receptor, CNTFα, induced corneal sensory nerve degeneration and delayed nerve regeneration, demonstrating the importance of CNTFα in the maintenance and regeneration of subbasal nerve plexus (59). Hence, in the case of the STZ-induced type 1 diabetic mice with corneal epithelial debridement wounds, decreased number of DCs on the cornea would lead to a decreased CNTF level, impairing corneal sensory nerve innervation and regeneration (59). Besides the involvement of CNTF, DCs may also be involved in the regeneration of neurons through the clearance of axonal debris. It was suggested that the clearance of axonal debris is a critical process in axonal regeneration in the peripheral nervous system (98). Besides murine studies, a clinical study has also reported similar observations where in children with T1D, a significant positive correlation was observed between the density of mature DCs and the corneal nerve fiber density (56).
However, the relationship between the DC density and corneal nerve parameters in subjects with T1D/T2D remains a topic for more investigation. There were also studies reporting no significant correlation to exist between the DC density and corneal nerve morphology in either T1D or T2D (54, 55). The analysis of the relationship may be confounded by several factors, such as variations in the type, stage, and duration of DM. It is also possible that corneal nerve fibre changes and DC density are different and independent phenomena that occur coincidentally at the same time, and other cells also play a role (15). For example, vascularisation that develops after denervation may also lead to the influx of DCs (15). Moreover, in patients with T1D/T2D, increased levels of AGE/RAGE signaling in neurons may induce the activation of inflammatory and oxidative stress pathways, including the NF-κB pathway, potentially causing damage and death of neuronal cells (99–101).
The associations reported may further help explore surrogate imaging markers for diabetic corneal neuropathy (54). For example, a significant correlation between DC density and the severity of diabetic peripheral neuropathy has been described (55). Moreover, the reported associations between the DC density and various nerve parameters may provide evidence for a potential therapeutic strategy to promote corneal nerve regeneration. For example, given the fact that DCs are the major source of CNTF, using DCs as therapeutic targets for the repair of injured corneal nerves in patients with T1D/T2D may open a new avenue for treatment (49, 59).
The literature review on the association between corneal DCs and corneal nerve parameters is summarised in Table 2.
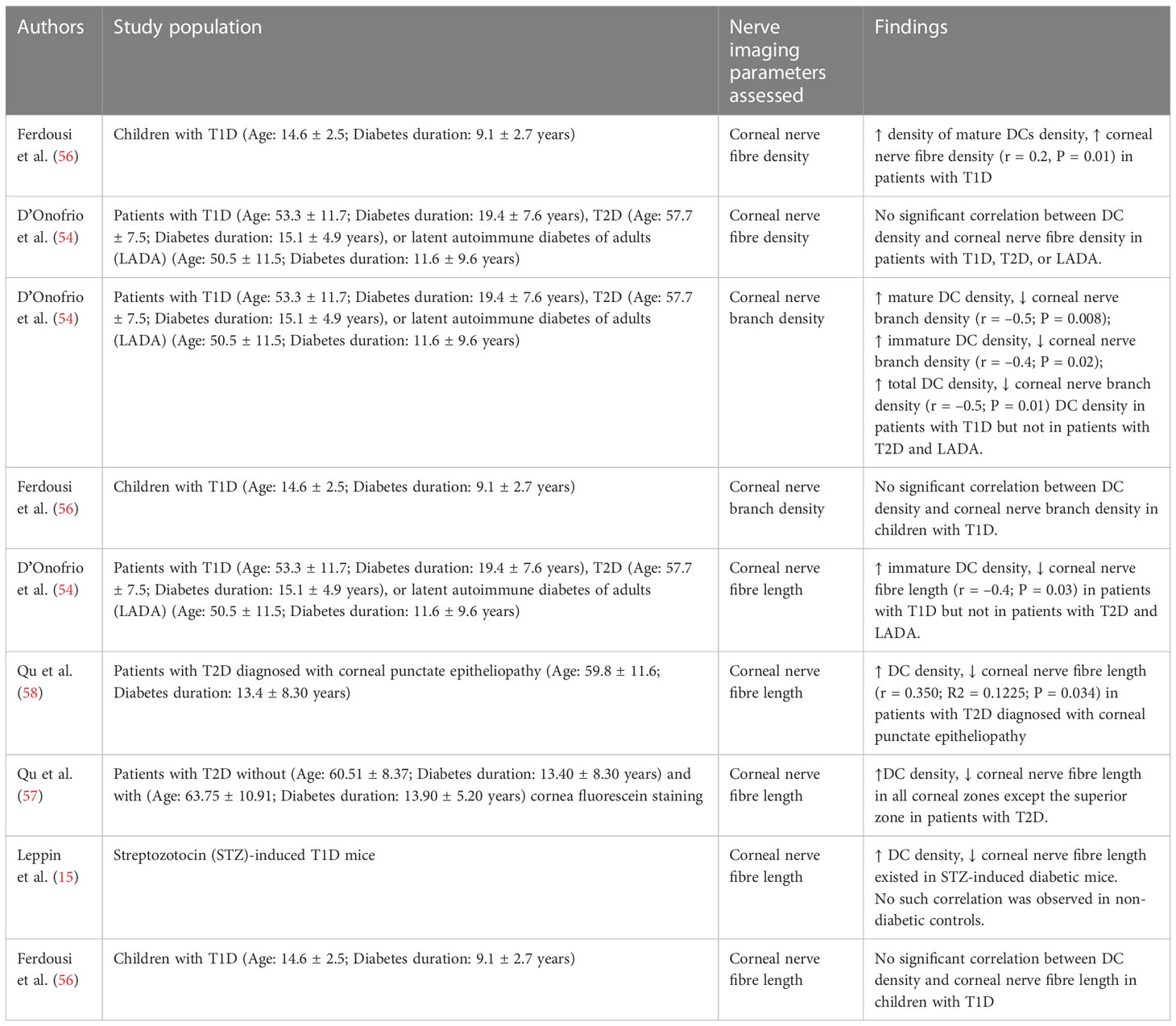
Table 2 Studies reporting on the correlation between DC density and various corneal nerve imaging parameters.
6.3. Interaction between corneal DCs and corneal epithelium in DM
Besides the interaction with the nerve, the interaction between DCs and epithelial cells may be perturbed in subjects with T1D/T2D, potentially affecting the corneal wound healing (80).
In both murine and clinical studies, it was observed that subjects with T1D/T2D had delayed corneal wound healing compared to healthy controls (59, 102–105). A decrease in basal epithelial cell (BEC) density in patients with T2D has also been reported by several clinical studies (57, 106, 107). Furthermore, a negative correlation between the BEC density and DC density in the cornea was observed in patients with T2D (57). Hence, it was speculated that DCs may be involved in the early stages of BEC proliferation and differentiation in DM (57). Epithelial wound closure requires cell reverse differentiation of wing cells to basal cell like cells, cell migration, and cell proliferation to replenish the lost cells (108, 109). Besides epithelial cells, immune cells, such as DCs, were also directly involved in accelerating epithelial wound healing (80). The anatomical proximity and structural intertwinements between DCs and epithelial cells have led to the suggestion that corneal epithelial cells and corneal intra-epithelial DCs interact with each other to form coordinated actions against adverse challenges, such as tissue injury and infection (41, 80). It was demonstrated in non-diabetic corneas that migratory epithelial cells during wound healing would express an elevated level of DC-targeting cytokines, to activate DCs around the injury site (41). Reciprocally, DCs would secrete growth factors, cytokines, and/or through cell-to-cell contact to facilitate migration and proliferation of epithelial cells, modulating wound healing (41, 59). However, the specific role of DCs in delayed epithelial wound healing in patients T1D/T2D remains unclear, and several explanations have been proposed (41).
One explanation is that prolonged corneal wound healing response in subjects with T1D/T2D may lead to increased recruitment of DCs to the corneal wound through chemokines released by the injured site (41, 103). Several factors were reported to contribute to the delayed recovery of corneal epithelial wounds in DM, including nerve degeneration, accumulation of AGEs, and direct damage caused by hyperglycaemia to the corneal epithelial basement membrane (110). In particular, it was suggested that AGEs may delay corneal epithelial wound healing through the production of reactive oxygen species (111). In a wounded cornea, the corneal epithelial cells can further facilitate wound healing through the release of various cytokines, including the C-X-C motif chemokine ligand 10 (CXCL10) (41). CXCL10 acts as a chemokine to DCs, activated T cells, and NK cells, and it was reported to be highly expressed in migrating epithelial during corneal wound healing (41, 112). Hence, it was suggested that epithelia-released CXCL10 may facilitate the recruitment of resident corneal epithelial DCs and even the circulating DCs to the wound bed in the cornea (41). It is possible that, in diabetic cornea where the wound healing process is altered and prolonged, more chemokines may be released by the epithelia, resulting in increased recruitment of DCs into the cornea (103, 113). Similarly, clinical studies on the epidermis of diabetic foot ulcers have reported an accumulation of DCs at the edge of diabetic foot ulcers (113, 114).
Contrary to the aforementioned explanation of increased DCs in diabetic wound healing, a murine study examining subjects with T1D has reported a decreased number of infiltrating DCs in diabetic healing cornea compared to healthy controls. It was proposed that such a decrease in DCs population may hinder the proliferation of the epithelial cells, contributing to the impaired wound healing process (59, 115). As discussed previously, CNTF originates from DCs and is involved in sensory nerve survival and regeneration (59). Recently, CNTF was also discovered to be able to promote epithelial wound healing by stimulating the mitogenic activation of corneal epithelial stem/progenitor cells (115). In the corneas of mice with T1D, the level of CNTF was significantly downregulated, potentially due to the decreased infiltrating DCs population, contributing to the impaired proliferation of epithelial cells during wound healing in diabetic corneas (59, 115).
6.4. Correlations between corneal DCs and blood metabolomic profiles
Associations between the DC density and several metabolic parameters, including lipid profiles, glycaemic control, as well as renal function, have also been assessed in patients with T1D/T2D, as shown in Table 3 (55, 56, 87).
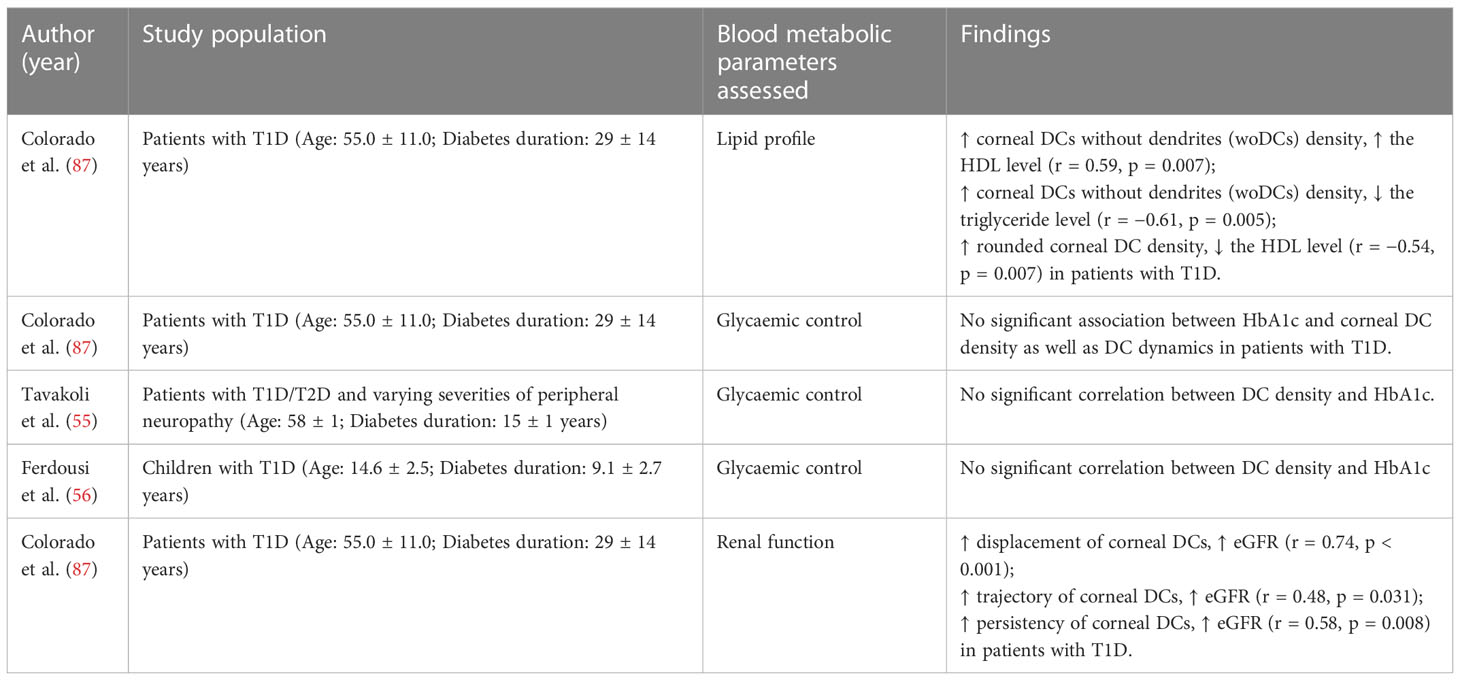
Table 3 Studies reporting on the correlation between corneal DC parameters and blood metabolic parameters.
In patients with T1D, significant associations were found between the DC density and lipid parameters (87). The density of corneal DCs without dendrites (woDCs) was positively correlated with the HDL cholesterol level and was inversely correlated with the triglycerides level (87). Such observations suggest that woDC may be associated with better health since both a higher HDL level and a lower triglyceride level potentially indicate lower cardiovascular risks (116, 117). However, another clinical study demonstrated that rounded corneal DC density was correlated inversely with the HDL level in patients with T1D (87). Such disparity reported may signal that different DC subsets exert different immune activities on the cornea in patients with T1D (87).
For the renal function of patients with T1D, a significant positive correlation was detected between the eGFR and the displacement, trajectory, and persistency of corneal DCs in patients with T1D (87). These three parameters are indicative of DCs’ mobilisation capacities which are critical for the role of the DCs in activating and mediating immune responses (87, 118). It has been proposed that the resident DCs in the cornea may function similarly to those in the kidney. eGFR was also negatively correlated with the number of DCs in the kidney for both healthy individuals and those with chronic kidney disease (119).
Contrasting to lipid parameters and renal function, glycaemic control has not been reported to be significantly associated with DCs parameters (density and dynamics) (55, 56, 87). The increase in DC density observed in patients with T1D/T2D may be independent of hyperglycaemia (55).
7. Future work
The changes in corneal DCs in patients with T1D/T2D have attracted much attention and discussion in recent years. Despite the current progress toward understanding the DC changes and underlying mechanisms, many questions remain and are to be addressed.
Firstly, continued improvement in imaging technologies, as well as the identification and quantification techniques used for corneal DCs on IVCM images are required, to ensure accurate analysis across the studies. Secondly, it is possible that the identification and characterisation of corneal DCs in vivo may be further refined, to contribute to a deeper understanding of corneal DCs changes, as well as the roles played by corneal DCs in the diabetic corneas (49). Thirdly, DCs, corneal nerves, and corneal epithelium were previously considered to form an “epineuroimmune” function unit (80). However, it remains unclear which of the three components is the initial “sentinel” that detects the physiological changes in patients with T1D/T2D and subsequently induces the changes in the other two components of the “epineuroimmune” function unit. Furthermore, the initial “trigger” (e.g. hyperglycaemia, intracellular reactive oxygen species, or extracellular AGEs) in the diabetic cornea that causes the physiological and functional changes in the “epineuroimmune” function unit also requires elucidation (80). Hence, further mechanistic studies are needed to define the basis of the changes in the “epineuroimmune” function unit in the diabetic cornea, potentially adding value to the development of preventive and treatment strategies for DM-associated ocular surface complications (55).
8. Conclusions
This article has reviewed current clinical and animal studies reporting the changes in corneal DCs in diabetic corneas, as well as the potential mechanisms underlying the changes. For the changes in DC density, the majority of animal and clinical studies reported an increase in corneal DCs density in DM, while a few presented the opposite (15, 53–59). The increase in DC density may be explained as a cellular response to inflammation while the decreased density may be explained as a result of apoptosis caused by prolonged exposure to hyperglycaemia (60, 80). The maturation of corneal DCs in tandem with the disease course of T2D was indicated (60). DCs were also found to be involved in diabetic nerve degeneration, yet whether DCs are neuroprotective or neurotoxic remains unclear with contrasting findings (49, 55). The association between increased DCs density and corneal nerve degeneration in DM may be explained by an enhanced immune response caused by the absence of tolerogenic and immunomodulatory neuropeptides following corneal nerve damage (95). On the other hand, the association between decreased DCs density and corneal nerve degeneration in diabetic corneas may be explained by the decreased CNTF level expressed by the corneal DCs (59). Corneal DCs are also involved in delayed epithelial wound healing in diabetic corneas (80). One suggested mechanism is that prolonged corneal wound healing response leads to increased recruitment of DCs to the corneal wound bed through chemokines released by epithelia around the injury site (41, 103). We also further reviewed the association between the changes in the corneal DCs and various clinical or corneal nerve imaging parameters, including age, corneal nerve status, and metabolic parameters (15, 53–58, 87). Such associations contribute to our current understanding of DM-associated ocular surface complications, potentially further assisting the development of diagnostic, preventive, and therapeutic strategies.
Author contributions
FL and LYC were responsible for conducting the search, screening potentially eligible studies, and writing the manuscript. CL was responsible for the synthesis of images. IL and ML were involved in conducting the search. LYC provided the direction of the review and overall supervision of this manuscript.
Funding
The study is supported by the Clinician Scientist Award Grant from the Singapore National Medical Research Council (MOH-CSAINV21jun-0001).
Conflict of interest
The authors declare that the research was conducted in the absence of any commercial or financial relationships that could be construed as a potential conflict of interest.
Publisher’s note
All claims expressed in this article are solely those of the authors and do not necessarily represent those of their affiliated organizations, or those of the publisher, the editors and the reviewers. Any product that may be evaluated in this article, or claim that may be made by its manufacturer, is not guaranteed or endorsed by the publisher.
References
1. Al-Lawati JA. Diabetes mellitus: A local and global public health emergency! Oman Med J (2017) 32(3):177–9. doi: 10.5001/omj.2017.34
2. Magliano DJ, Boyko EJ, Balkau B, Barengo N, Barr E, Basit A, et al. IDF_Atlas_10th_Edition_2021, 10th edition. Edward DJM, Boyko J., Suvi Karuranga LP, Riley P, Saeedi P, Sun H (Brussels: The International Diabetes Federation), editors (2021).
3. Bommer C, Sagalova V, Heesemann E, Manne-Goehler J, Atun R, Bärnighausen T, et al. Global economic burden of diabetes in adults: Projections from 2015 to 2030. Diabetes Care (2018) 41(5):963–70. doi: 10.2337/dc17-1962
4. Zhou T, Lee A, Lo ACY, Kwok JSWJ. Diabetic corneal neuropathy: Pathogenic mechanisms and therapeutic strategies. Front Pharmacol (2022) 13. doi: 10.3389/fphar.2022.816062
5. Mansoor H, Tan HC, Lin MT, Mehta JS, Liu YC. Diabetic corneal neuropathy. J Clin Med (2020) 9(12):3956. doi: 10.3390/jcm9123956
6. Barsegian A, Lee J, Salifu MO, McFarlane SI. Corneal neuropathy: An underrated manifestation of diabetes mellitus. J Clin Endocrinol Diabetes (2018) 2(1):JCED-111. doi: 10.29011/JCED-111/100011
7. So WZ, Qi Wong NS, Tan HC, Yu Lin MT, Yu Lee IX, Mehta JS, et al. Diabetic corneal neuropathy as a surrogate marker for diabetic peripheral neuropathy. Neural Regener Res (2022) 17(10):2172–8. doi: 10.4103/1673-5374.327364
8. Guthoff RF, Zhivov A, Stachs O. In vivo confocal microscopy, an inner vision of the cornea – a major review. Clin Exp Ophthalmol (2009) 37(1):100–17. doi: 10.1111/j.1442-9071.2009.02016.x
9. Jalbert I, Stapleton F, Papas E, Sweeney DF, Coroneo M. In vivo confocal microscopy of the human cornea. Br J Ophthalmol (2003) 87(2):225–36. doi: 10.1136/bjo.87.2.225
10. Liu YC, Lin MT, Mehta JS. Analysis of corneal nerve plexus in corneal confocal microscopy images. Neural Regener Res (2021) 16(4):690–1. doi: 10.4103/1673-5374.289435
11. Liu Y-C, Jung ASJ, Chin JY, Yang LWY, Mehta JS. Cross-sectional study on corneal denervation in contralateral eyes following SMILE versus LASIK. J Refractive Surg (2020) 36(10):653–60. doi: 10.3928/1081597X-20200730-01
12. Kallinikos P, Berhanu M, O'Donnell C, Boulton AJ, Efron N, Malik RA. Corneal nerve tortuosity in diabetic patients with neuropathy. Invest Ophthalmol Vis Sci (2004) 45(2):418–22. doi: 10.1167/iovs.03-0637
13. Teo CHY, Lin MT, Lee IXY, Koh SK, Zhou L, Goh DS, et al. Oral Peroxisome Proliferator-Activated Receptor-Alpha (PPAR)-α Agonist Enhances Corneal Nerve Regeneration in Patients with Type II Diabetes Mellitus. Diabetes (2022) db220611. doi: 10.2337/db22-0611
14. Petropoulos IN, Ferdousi M, Marshall A, Alam U, Ponirakis G, Azmi S, et al. The inferior whorl for detecting diabetic peripheral neuropathy using corneal confocal microscopy. Invest Ophthalmol Vis Sci (2015) 56(4):2498–504. doi: 10.1167/iovs.14-15919
15. Leppin K, Behrendt AK, Reichard M, Stachs O, Guthoff RF, Baltrusch S, et al. Diabetes mellitus leads to accumulation of dendritic cells and nerve fiber damage of the subbasal nerve plexus in the cornea. Invest Ophthalmol Vis Sci (2014) 55(6):3603–15. doi: 10.1167/iovs.14-14307
16. Banchereau J, Steinman RM. Dendritic cells and the control of immunity. Nature (1998) 392(6673):245–52. doi: 10.1038/32588
17. Steinman RM. The dendritic cell system and its role in immunogenicity. Annu Rev Immunol (1991) 9:271–96. doi: 10.1146/annurev.iy.09.040191.001415
18. Knickelbein JE, Watkins SC, McMenamin PG, Hendricks RL. Stratification of antigen-presenting cells within the normal cornea. Ophthalmol Eye Dis (2009) 1:45–54. doi: 10.4137/OED.S2813
19. Hattori T, Chauhan SK, Lee H, Ueno H, Dana R, Kaplan DH. Characterization of langerin-expressing dendritic cell subsets in the normal cornea. Invest Ophthalmol Vis Sci (2011) 52(7):4598–604. doi: 10.1167/iovs.10-6741
20. Hamrah P, Dana MR. Corneal antigen-presenting cells. Chem Immunol Allergy (2007) 92:58–70. doi: 10.1159/000099254
21. Forrester JV, Xu H, Kuffová L, Dick AD, McMenamin PG. Dendritic cell physiology and function in the eye. Immunol Rev (2010) 234(1):282–304. doi: 10.1111/j.0105-2896.2009.00873.x
22. Akhlaq A, Colón C, Cavalcanti BM, Aggarwal S, Qazi Y, Cruzat A, et al. Density and distribution of dendritiform cells in the peripheral cornea of healthy subjects using in vivo confocal microscopy. Ocul Surf (2022) 26:157–65. doi: 10.1016/j.jtos.2022.07.008
23. Sichien D, Lambrecht BN, Guilliams M, Scott CL. Development of conventional dendritic cells: from common bone marrow progenitors to multiple subsets in peripheral tissues. Mucosal Immunol (2017) 10(4):831–44. doi: 10.1038/mi.2017.8
24. Musumeci A, Lutz K, Winheim E, Krug AB. What makes a pDC: Recent advances in understanding plasmacytoid DC development and heterogeneity. Front Immunol (2019) 10. doi: 10.3389/fimmu.2019.01222
25. Mayer WJ, Irschick UM, Moser P, Wurm M, Huemer HP, Romani N, et al. Characterization of antigen-presenting cells in fresh and cultured human corneas using novel dendritic cell markers. Invest Ophthalmol Vis Sci (2007) 48(10):4459–67. doi: 10.1167/iovs.06-1184
26. Hamrah P, Huq SO, Liu Y, Zhang Q, Dana MR. Corneal immunity is mediated by heterogeneous population of antigen-presenting cells. J Leukoc Biol (2003) 74(2):172–8. doi: 10.1189/jlb.1102544
27. Zhivov A, Stave J, Vollmar B, Guthoff R. In vivo confocal microscopic evaluation of langerhans cell density and distribution in the normal human corneal epithelium. Graefes Arch Clin Exp Ophthalmol (2005) 243(10):1056–61. doi: 10.1007/s00417-004-1075-8
28. Chinnery HR, Zhang XY, Wu CY, Downie LE. Corneal immune cell morphometry as an indicator of local and systemic pathology: A review. Clin Exp Ophthalmol (2021) 49(7):729–40. doi: 10.1111/ceo.13972
29. Jamali A, Kenyon B, Ortiz G, Abou-Slaybi A, Sendra VG, Harris DL, et al. Plasmacytoid dendritic cells in the eye. Prog Retin Eye Res (2021) 80:100877. doi: 10.1016/j.preteyeres.2020.100877
30. Ginhoux F, Tacke F, Angeli V, Bogunovic M, Loubeau M, Dai X-M, et al. Langerhans cells arise from monocytes in vivo. Nat Immunol (2006) 7(3):265–73. doi: 10.1038/ni1307
31. Doebel T, Voisin B, Nagao K. Langerhans cells – the macrophage in dendritic cell clothing. Trends Immunol (2017) 38(11):817–28. doi: 10.1016/j.it.2017.06.008
32. Hamrah P, Liu Y, Zhang Q, Dana MR. The corneal stroma is endowed with a significant number of resident dendritic cells. Invest Ophthalmol Vis Sci (2003) 44(2):581–9. doi: 10.1167/iovs.02-0838
33. Zheng L, Andrian U, Hamrah P. Identification of novel subsets of plasmacytoid and conventional dendritic cells in the cornea. Invest Ophthalmol Visual Sci (2010) 51(13):1544–4.
34. Lužnik Z, Kopitar AN, Lapajne L, Pižem J, Ferrari S, Ihan A, et al. Identification and characterization of dendritic cell subtypes in preserved and cultured cadaveric human corneolimbal tissue on amniotic membrane. Acta Ophthalmologica (2019) 97(2):e184–93. doi: 10.1111/aos.13854
35. Blanco T, Jamali A, Sendra VG, Lopez MJ, Moein H-R, Hamrah P. Plasmacytoid dendritic cells in the mouse cornea: a multiphoton intravital microscopy study. Invest Ophthalmol Visual Sci (2017) 58(8):980–0.
36. Hattori T, Takahashi H, Dana R. Novel insights into the immunoregulatory function and localization of dendritic cells. Cornea (2016) 35(1):S49–s54. doi: 10.1097/ICO.0000000000001005
37. Dana MR. Corneal antigen-presenting cells: diversity, plasticity, and disguise: the cogan lecture. Invest Ophthalmol Vis Sci (2004) 45(3):722–7. doi: 10.1167/iovs.03-0803
38. Jamali A, Seyed-Razavi Y, Chao C, Ortiz G, Kenyon B, Blanco T, et al. Intravital multiphoton microscopy of the ocular surface: Alterations in conventional dendritic cell morphology and kinetics in dry eye disease. Front Immunol (2020) 11. doi: 10.3389/fimmu.2020.00742
39. Hao R, Ding Y, Li X. Alterations in corneal epithelial dendritic cell in sjogren’s syndrome dry eye and clinical correlations. Sci Rep (2022) 12(1):11167. doi: 10.1038/s41598-022-15537-4
40. Hamrah P, Liu Y, Zhang Q, Dana MR. Alterations in corneal stromal dendritic cell phenotype and distribution in inflammation. Arch Ophthalmol (2003) 121(8):1132–40. doi: 10.1001/archopht.121.8.1132
41. Gao N, Yin J, Yoon GS, Mi QS, Yu FS. Dendritic cell-epithelium interplay is a determinant factor for corneal epithelial wound repair. Am J Pathol (2011) 179(5):2243–53. doi: 10.1016/j.ajpath.2011.07.050
42. Gottschalk C, Kurts C. The debate about dendritic cells and macrophages in the kidney. Front Immunol (2015) 6. doi: 10.3389/fimmu.2015.00435
43. Cao Q, Wang Y, Wang XM, Lu J, Lee VW, Ye Q, et al. Renal F4/80+ CD11c+ mononuclear phagocytes display phenotypic and functional characteristics of macrophages in health and in adriamycin nephropathy. J Am Soc Nephrol (2015) 26(2):349–63. doi: 10.1681/ASN.2013121336
44. Dong X, Swaminathan S, Bachman LA, Croatt AJ, Nath KA, Griffin MD. Resident dendritic cells are the predominant TNF-secreting cell in early renal ischemia–reperfusion injury. Kidney Int (2007) 71(7):619–28. doi: 10.1038/sj.ki.5002132
45. Dong X, Bachman LA, Miller MN, Nath KA, Griffin MD. Dendritic cells facilitate accumulation of IL-17 T cells in the kidney following acute renal obstruction. Kidney Int (2008) 74(10):1294–309. doi: 10.1038/ki.2008.394
46. Krüger T, Benke D, Eitner F, Lang A, Wirtz M, Hamilton-Williams EE, et al. Identification and functional characterization of dendritic cells in the healthy murine kidney and in experimental glomerulonephritis. J Am Soc Nephrol (2004) 15(3):613–21. doi: 10.1097/01.ASN.0000114553.36258.91
47. Chin JY, Yang LWY, Ji AJS, Nubile M, Mastropasqua L, Allen JC, et al. Validation of the use of automated and manual quantitative analysis of corneal nerve plexus following refractive surgery. Diagnostics (2020) 10(7):493. doi: 10.3390/diagnostics10070493
48. Resch MD, Marsovszky L, Németh J, Bocskai M, Kovács L, Balog A, et al. Dry eye and corneal langerhans cells in systemic lupus erythematosus. J Ophthalmol (2015) 2015:543835. doi: 10.1155/2015/543835
49. Wu M, Hill LJ, Downie LE, Chinnery HR. Neuroimmune crosstalk in the cornea: The role of immune cells in corneal nerve maintenance during homeostasis and inflammation. Prog Retin Eye Res (2022) 91:101105. doi: 10.1016/j.preteyeres.2022.101105
50. Choi EY, Kang HG, Lee CH, Yeo A, Noh HM, Gu N, et al. Langerhans cells prevent subbasal nerve damage and upregulate neurotrophic factors in dry eye disease. PloS One (2017) 12(4):e0176153. doi: 10.1371/journal.pone.0176153
51. McLeish W, Rubsamen P, Atherton SS, Streilein JW. Immunobiology of langerhans cells on the ocular surface. II. role of central corneal langerhans cells in stromal keratitis following experimental HSV-1 infection in mice. Reg Immunol (1989) 2(4):236–43.
52. Csorba A, Maneschg OA, Resch MD, Nagy ZZ. Examination of corneal microstructure in the quiescent phase of vernal keratoconjunctivitis using in vivo confocal microscopy. Eur J Ophthalmol (2022) 33:11206721221099778. doi: 10.1177/11206721221099778
53. Colorado LH, Beecher L, Pritchard N, Al Rashah K, Dehghani C, Russell A, et al. Corneal dendritic cell dynamics are associated with clinical factors in type 1 diabetes. J Clin Med (2022) 11(9):2611. doi: 10.3390/jcm11092611
54. D'Onofrio L, Kalteniece A, Ferdousi M, Azmi S, Petropoulos IN, Ponirakis G, et al. Small nerve fiber damage and langerhans cells in type 1 and type 2 diabetes and LADA measured by corneal confocal microscopy. Invest Ophthalmol Vis Sci (2021) 62(6):5. doi: 10.1167/iovs.62.6.5
55. Tavakoli M, Boulton AJ, Efron N, Malik RA. Increased langerhan cell density and corneal nerve damage in diabetic patients: role of immune mechanisms in human diabetic neuropathy. Cont Lens Anterior Eye (2011) 34(1):7–11. doi: 10.1016/j.clae.2010.08.007
56. Ferdousi M, Romanchuk K, Mah JK, Virtanen H, Millar C, Malik RA, et al. Early corneal nerve fibre damage and increased langerhans cell density in children with type 1 diabetes mellitus. Sci Rep (2019) 9(1):8758. doi: 10.1038/s41598-019-45116-z
57. Qu JH, Tian L, Zhang XY, Sun XG. Early central and peripheral corneal microstructural changes in type 2 diabetes mellitus patients identified using in vivo confocal microscopy: A case-control study. Med (Baltimore) (2017) 96(38):e7960. doi: 10.1097/MD.0000000000007960
58. Qu JH, Li L, Tian L, Zhang XY, Thomas R, Sun XG. Epithelial changes with corneal punctate epitheliopathy in type 2 diabetes mellitus and their correlation with time to healing. BMC Ophthalmol (2018) 18(1):1. doi: 10.1186/s12886-017-0645-6
59. Gao N, Yan C, Lee P, Sun H, Yu FS. Dendritic cell dysfunction and diabetic sensory neuropathy in the cornea. J Clin Invest (2016) 126(5):1998–2011. doi: 10.1172/JCI85097
60. Lagali NS, Badian RA, Liu X, Feldreich TR, Arnlov J, Utheim TP, et al. Dendritic cell maturation in the corneal epithelium with onset of type 2 diabetes is associated with tumor necrosis factor receptor superfamily member 9. Sci Rep (2018) 8(1):14248. doi: 10.1038/s41598-018-32410-5
61. Chao C, Wang R, Jones M, Karson N, Jussel A, Smith J, et al. The relationship between corneal nerve density and hemoglobin A1c in patients with prediabetes and type 2 diabetes. Invest Ophthalmol Vis Sci (2020) 61(12):26. doi: 10.1167/iovs.61.12.26
62. King AJ. The use of animal models in diabetes research. Br J Pharmacol (2012) 166(3):877–94. doi: 10.1111/j.1476-5381.2012.01911.x
63. Tomlinson DR, Gardiner NJ. Glucose neurotoxicity. Nat Rev Neurosci (2008) 9(1):36–45. doi: 10.1038/nrn2294
64. Donath MY, Shoelson SE. Type 2 diabetes as an inflammatory disease. Nat Rev Immunol (2011) 11(2):98–107. doi: 10.1038/nri2925
65. Wu X-Q, Zhang D-D, Wang Y-N, Tan Y-Q, Yu X-Y, Zhao Y-Y, et al. AGE/RAGE in diabetic kidney disease and ageing kidney. Free Radical Biol Med (2021) 171:260–71. doi: 10.1016/j.freeradbiomed.2021.05.025
66. Rungratanawanich W, Qu Y, Wang X, Essa MM, Song B-J. Advanced glycation end products (AGEs) and other adducts in aging-related diseases and alcohol-mediated tissue injury. Exp Mol Med (2021) 53(2):168–88. doi: 10.1038/s12276-021-00561-7
67. Yamagishi S-i, Matsui T. Advanced glycation end products, oxidative stress and diabetic nephropathy. Oxid Med Cell Longevity (2010) 3:938285. doi: 10.4161/oxim.3.2.11148
68. Yue Q, Song Y, Liu Z, Zhang L, Yang L, Li J. Receptor for advanced glycation end products (RAGE): A pivotal hub in immune diseases. Molecules (2022) 27(15)4922. doi: 10.3390/molecules27154922
69. Makita Z, Radoff S, Rayfield EJ, Yang Z, Skolnik E, Delaney V, et al. Advanced glycosylation end products in patients with diabetic nephropathy. N Engl J Med (1991) 325(12):836–42. doi: 10.1056/NEJM199109193251202
70. Kilhovd BK, Giardino I, Torjesen PA, Birkeland KI, Berg TJ, Thornalley PJ, et al. Increased serum levels of the specific AGE-compound methylglyoxal-derived hydroimidazolone in patients with type 2 diabetes. Metabolism (2003) 52(2):163–7. doi: 10.1053/meta.2003.50035
71. Schalkwijk CG, Baidoshvili A, Stehouwer CDA, van Hinsbergh VWM, Niessen HWM. Increased accumulation of the glycoxidation product nϵ-(carboxymethyl)lysine in hearts of diabetic patients: generation and characterisation of a monoclonal anti-CML antibody. Biochim Biophys Acta (BBA) - Mol Cell Biol Lipids (2004) 1636(2):82–9. doi: 10.1016/j.bbalip.2003.07.002
72. Schleicher ED, Wagner E, Nerlich AG. Increased accumulation of the glycoxidation product n(epsilon)-(carboxymethyl)lysine in human tissues in diabetes and aging. J Clin Invest (1997) 99(3):457–68. doi: 10.1172/JCI119180
73. Kilhovd BK, Berg TJ, Birkeland KI, Thorsby P, Hanssen KF. Serum levels of advanced glycation end products are increased in patients with type 2 diabetes and coronary heart disease. Diabetes Care (1999) 22(9):1543–8. doi: 10.2337/diacare.22.9.1543
74. Nowotny K, Jung T, Höhn A, Weber D, Grune T. Advanced glycation end products and oxidative stress in type 2 diabetes mellitus. Biomolecules (2015) 5(1):194–222. doi: 10.3390/biom5010194
75. Kobayashi M, Zochodne DW. Diabetic neuropathy and the sensory neuron: New aspects of pathogenesis and their treatment implications. J Diabetes Invest (2018) 9(6):1239–54. doi: 10.1111/jdi.12833
76. Lee EJ, Rosenbaum JT, Planck SR. Epifluorescence intravital microscopy of murine corneal dendritic cells. Invest Ophthalmol Visual Sci (2010) 51(4):2101–8. doi: 10.1167/iovs.08-2213
77. Mastropasqua L, Nubile M, Lanzini M, Carpineto P, Ciancaglini M, Pannellini T, et al. Epithelial dendritic cell distribution in normal and inflamed human cornea: in vivo confocal microscopy study. Am J Ophthalmol (2006) 142(5):736–44. doi: 10.1016/j.ajo.2006.06.057
78. Vuckovic S, Withers G, Harris M, Khalil D, Gardiner D, Flesch I, et al. Decreased blood dendritic cell counts in type 1 diabetic children. Clin Immunol (2007) 123(3):281–8. doi: 10.1016/j.clim.2007.03.002
79. Seifarth CC, Hinkmann C, Hahn EG, Lohmann T, Harsch IA. Reduced frequency of peripheral dendritic cells in type 2 diabetes. Exp Clin Endocrinol Diabetes (2008) 116(3):162–6. doi: 10.1055/s-2007-990278
80. Yu FX, Lee PSY, Yang L, Gao N, Zhang Y, Ljubimov AV, et al. The impact of sensory neuropathy and inflammation on epithelial wound healing in diabetic corneas. Prog Retin Eye Res (2022) 89:101039. doi: 10.1016/j.preteyeres.2021.101039
81. Blondet JJ, Beilman GJ. Glycemic control and prevention of perioperative infection. Curr Opin Crit Care (2007) 13(4):421–7. doi: 10.1097/MCC.0b013e32826388a1
82. Vinay DS, Kwon BS. 4-1BB signaling beyond T cells. Cell Mol Immunol (2011) 8(4):281–4. doi: 10.1038/cmi.2010.82
83. Wilcox RA, Tamada K, Strome SE, Chen L. Signaling through NK cell-associated CD137 promotes both helper function for CD8+ cytolytic T cells and responsiveness to IL-2 but not cytolytic activity. J Immunol (2002) 169(8):4230–6. doi: 10.4049/jimmunol.169.8.4230
84. Bartkowiak T, Curran MA. 4-1BB agonists: Multi-potent potentiators of tumor immunity. Front Oncol (2015) 5:117. doi: 10.3389/fonc.2015.00117
85. Cao W, Chen J, Chen Y, Chen S, Chen X, Huang H, et al. Advanced glycation end products induced immune maturation of dendritic cells controls heart failure through NF-κB signaling pathway. Arch Biochem Biophysics (2015) 580:112–20. doi: 10.1016/j.abb.2015.07.003
86. Ge J, Jia Q, Liang C, Luo Y, Huang D, Sun A, et al. Advanced glycosylation end products might promote atherosclerosis through inducing the immune maturation of dendritic cells. Arteriosclerosis Thrombosis Vasc Biol (2005) 25(10):2157–63. doi: 10.1161/01.ATV.0000181744.58265.63
87. Colorado LH, Edwards K, Chinnery HR, Bazan HE. In vivo immune cell dynamics in the human cornea. Exp Eye Res (2020) 199:108168. doi: 10.1016/j.exer.2020.108168
88. Group T.D.P.P.R The influence of age on the effects of lifestyle modification and metformin in prevention of diabetes. Journals Gerontology: Ser A (2006) 61(10):1075–81. doi: 10.1093/gerona/61.10.1075
89. Harris MI, Flegal KM, Cowie CC, Eberhardt MS, Goldstein DE, Little RR. Prevalence of diabetes, impaired fasting glucose, and impaired glucose tolerance in U.S. adults: The third national health and nutrition examination survey, 1988–1994. Diabetes Care (1998) 21(4):518–24. doi: 10.2337/diacare.21.4.518
90. Mobeen R, Stapleton F, Chao C, Madigan MC, Briggs N, Golebiowski B. Corneal epithelial dendritic cell density in the healthy human cornea: A meta-analysis of in-vivo confocal microscopy data. Ocular Surface (2019) 17(4):753–62. doi: 10.1016/j.jtos.2019.07.001
91. Choi KL, Sauder DN. Epidermal langerhans cell density and contact sensitivity in young and aged BALB/c mice. Mech Ageing Dev (1987) 39(1):69–79. doi: 10.1016/0047-6374(87)90087-X
92. Bhushan M, Cumberbatch M, Dearman RJ, Andrew SM, Kimber I, Griffiths CE. Tumour necrosis factor-alpha-induced migration of human langerhans cells: the influence of ageing. Br J Dermatol (2002) 146(1):32–40. doi: 10.1046/j.1365-2133.2002.04549.x
93. Petersen KF, Shulman GI. Etiology of insulin resistance. Am J Med (2006) 119(5, Supplement 1):S10–6. doi: 10.1016/j.amjmed.2006.01.009
94. Tschen S-I, Dhawan S, Gurlo T, Bhushan A. Age-dependent decline in β-cell proliferation restricts the capacity of β-cell regeneration in mice. Diabetes (2009) 58(6):1312–20. doi: 10.2337/db08-1651
95. Cruzat A, Witkin D, Baniasadi N, Zheng L, Ciolino JB, Jurkunas UV, et al. Inflammation and the nervous system: The connection in the cornea in patients with infectious keratitis. Invest Ophthalmol Visual Sci (2011) 52(8):5136–43. doi: 10.1167/iovs.10-7048
96. Hosoi J, Murphy GF, Egan CL, Lerner EA, Grabbe S, Asahina A, et al. Regulation of langerhans cell function by nerves containing calcitonin gene-related peptide. Nature (1993) 363(6425):159–63. doi: 10.1038/363159a0
97. Gonzalez-Rey E. Keeping the balance between immune tolerance and pathogen immunity with endogenous neuropeptides. Neuroimmunomodulation (2010) 17(3):161–4. doi: 10.1159/000258713
98. Dubový P. Wallerian degeneration and peripheral nerve conditions for both axonal regeneration and neuropathic pain induction. Ann Anat - Anatomischer Anzeiger (2011) 193(4):267–75. doi: 10.1016/j.aanat.2011.02.011
99. Dong H, Zhang Y, Huang Y, Deng H. Pathophysiology of RAGE in inflammatory diseases. Front Immunol (2022) 13. doi: 10.3389/fimmu.2022.931473
100. Wang X, Yu S, Hu J-P, Wang C-Y, Wang Y, Liu H-X. Streptozotocin-induced diabetes increases amyloid plaque deposition in AD transgenic mice through modulating AGEs/RAGE/NF-κB pathway. Int J Neurosci (2014) 124(8):601–8. doi: 10.3109/00207454.2013.866110
101. Piras S, Furfaro AL, Domenicotti C, Traverso N, Marinari UM, Pronzato MA, et al. RAGE expression and ROS generation in neurons: Differentiation versus damage. Oxid Med Cell Longev (2016) 2016:9348651. doi: 10.1155/2016/9348651
102. Yamamoto T, Otake H, Hiramatsu N, Yamamoto N, Taga A, Nagai N. A proteomic approach for understanding the mechanisms of delayed corneal wound healing in diabetic keratopathy using diabetic model rat. Int J Mol Sci (2018) 19(11):3635. doi: 10.3390/ijms19113635
103. Bu Y, Shih KC, Kwok SS, Chan YK, Lo AC, Chan TCY, et al. Experimental modeling of cornea wound healing in diabetes: clinical applications and beyond. BMJ Open Diabetes Res Care (2019) 7(1):e000779. doi: 10.1136/bmjdrc-2019-000779
104. Xu K, Yu F-SX. Impaired epithelial wound healing and EGFR signaling pathways in the corneas of diabetic rats. Invest Ophthalmol Visual Sci (2011) 52(6):3301–8. doi: 10.1167/iovs.10-5670
105. Chen W-L, Lin C-T, Ko P-S, Yeh P-T, Kuan Y-H, Hu F-R, et al. In vivo confocal microscopic findings of corneal wound healing after corneal epithelial debridement in diabetic vitrectomy. Ophthalmology (2009) 116(6):1038–47. doi: 10.1016/j.ophtha.2009.01.002
106. Quadrado MJ, Popper M, Morgado AM, Murta JN, Van Best JA. Diabetes and corneal cell densities in humans by in vivo confocal microscopy. Cornea (2006) 25(7):761–8. doi: 10.1097/01.ico.0000224635.49439.d1
107. Chang PY, Carrel H, Huang JS, Wang IJ, Hou YC, Chen WL, et al. Decreased density of corneal basal epithelium and subbasal corneal nerve bundle changes in patients with diabetic retinopathy. Am J Ophthalmol (2006) 142(3):488–90. doi: 10.1016/j.ajo.2006.04.033
108. Xu KP, Li Y, Ljubimov AV, Yu FS. High glucose suppresses epidermal growth factor receptor/phosphatidylinositol 3-kinase/Akt signaling pathway and attenuates corneal epithelial wound healing. Diabetes (2009) 58(5):1077–85. doi: 10.2337/db08-0997
109. Dua HS, Gomes JA, Singh A. Corneal epithelial wound healing. Br J Ophthalmol (1994) 78(5):401–8. doi: 10.1136/bjo.78.5.401
110. Han SB, Yang HK, Hyon JY. Influence of diabetes mellitus on anterior segment of the eye. Clin Interv Aging (2019) 14:53–63. doi: 10.2147/CIA.S190713
111. Shi L, Chen H, Yu X, Wu X. Advanced glycation end products delay corneal epithelial wound healing through reactive oxygen species generation. Mol Cell Biochem (2013) 383(1):253–9. doi: 10.1007/s11010-013-1773-9
112. Padovan E, Spagnoli GC, Ferrantini M, Heberer M. IFN-alpha2a induces IP-10/CXCL10 and MIG/CXCL9 production in monocyte-derived dendritic cells and enhances their capacity to attract and stimulate CD8+ effector T cells. J Leukoc Biol (2002) 71(4):669–76. doi: 10.1189/jlb.71.4.669
113. Stojadinovic O, Yin N, Lehmann J, Pastar I, Kirsner RS, Tomic-Canic M. Increased number of langerhans cells in the epidermis of diabetic foot ulcers correlates with healing outcome. Immunologic Res (2013) 57(1):222–8. doi: 10.1007/s12026-013-8474-z
114. Galkowska H, Olszewski WL, Wojewodzka U. Expression of natural antimicrobial peptide beta-defensin-2 and langerhans cell accumulation in epidermis from human non-healing leg ulcers. Folia Histochemica Cytobiologica (2005) 43(3):133–6.
115. Zhou Q, Chen P, Di G, Zhang Y, Wang Y, Qi X, et al. Ciliary neurotrophic factor promotes the activation of corneal epithelial Stem/Progenitor cells and accelerates corneal epithelial wound healing. Stem Cells (2015) 33(5):1566–76. doi: 10.1002/stem.1942
116. Bardagjy AS, Steinberg FM. Relationship between HDL functional characteristics and cardiovascular health and potential impact of dietary patterns: A narrative review. Nutrients (2019) 11(6):1231. doi: 10.3390/nu11061231
117. Austin MA, Hokanson JE, Edwards KL. Hypertriglyceridemia as a cardiovascular risk factor. Am J Cardiol (1998) 81(4a):7b–12b. doi: 10.1016/S0002-9149(98)00031-9
118. Liu J, Zhang X, Cheng Y, Cao X. Dendritic cell migration in inflammation and immunity. Cell Mol Immunol (2021) 18(11):2461–71. doi: 10.1038/s41423-021-00726-4
119. Kassianos AJ, Wang X, Sampangi S, Muczynski K, Healy H, Wilkinson R. Increased tubulointerstitial recruitment of human CD141hi CLEC9A+ and CD1c+ myeloid dendritic cell subsets in renal fibrosis and chronic kidney disease. Am J Physiology-Renal Physiol (2013) 305(10):F1391–401. doi: 10.1152/ajprenal.00318.2013
Keywords: corneal dendritic cell, diabetic mellitus, corneal nerves, corneal epithelial cells, in vivo confocal microscopy, diabetic corneal neuropathy, diabetic microvascular complications, ocular surface
Citation: Liu F, Liu C, Lee IXY, Lin MTY and Liu Y-C (2023) Corneal dendritic cells in diabetes mellitus: A narrative review. Front. Endocrinol. 14:1078660. doi: 10.3389/fendo.2023.1078660
Received: 24 October 2022; Accepted: 12 January 2023;
Published: 27 January 2023.
Edited by:
Sobha Sivaprasad, NHS Foundation Trust, United KingdomReviewed by:
Norbert Nass, Städtische Klinikum Dessau, GermanyQingjun Zhou, Shandong Eye Institute, China
Helen Jiao, The University of Melbourne, Australia
Luca D’Onofrio, Sapienza University of Rome, Italy
Copyright © 2023 Liu, Liu, Lee, Lin and Liu. This is an open-access article distributed under the terms of the Creative Commons Attribution License (CC BY). The use, distribution or reproduction in other forums is permitted, provided the original author(s) and the copyright owner(s) are credited and that the original publication in this journal is cited, in accordance with accepted academic practice. No use, distribution or reproduction is permitted which does not comply with these terms.
*Correspondence: Yu-Chi Liu, liuchiy@gmail.com