- 1Department of Pharmacology, Faculty of Medicine, School of Health Sciences, University of Ioannina, Ioannina, Greece
- 2Department of Anatomy, School of Medicine, European University of Cyprus, Nicosia, Cyprus
Accumulating clinical evidence indicates extensive inter-individual variations in the effectiveness and adverse effects of standard treatment protocols, which are largely attributed to the multifactorial regulation of the hepatic CYP-dependent drug metabolism that is connected with either transcriptional or post-translational modifications. Age and stress belong to the most important factors in CYP gene regulation. Alterations in neuroendocrine responses to stress, which are associated with modified hypothalamo-pituitary-adrenal axis function, usually accompany ageing. In this light, ageing followed by a decline of the functional integrity of organs, including liver, a failure in preserving homeostasis under stress, increased morbidity and susceptibility to stress, among others, holds a determinant role in the CYP-catalyzed drug metabolism and thus, in the outcome and toxicity of pharmacotherapy. Modifications in the drug metabolizing capacity of the liver with age have been reported and in particular, a decline in the activity of the main CYP isoforms in male senescent rats, indicating decreased metabolism and higher levels of the drug-substrates in their blood. These factors along with the restricted experience in the use of the most medicines in childhood and elderly, could explain at an extent the inter-individual variability in drug efficacy and toxicity outcomes, and underscore the necessity of designing the treatment protocols, accordingly.
Introduction
To date, the outcome of pharmacotherapy remains a complex and challenging issue. Over the years, accumulating clinical evidence indicates that each patient represents an individual case in drug treatment. This notion is mainly based on the extensive inter-individual variations observed in the efficacy of standard protocols used in the treatment of diseases, such as depression, cancer, hypertension, epilepsy and diabetes, as well as in the drug-related adverse reactions and toxicity outcomes (1–4). In particular, when a multi-drug therapeutic scheme is followed, the failure of pharmacotherapy and drug toxicity is more likely to occur. The diversity in the drug response among individuals is largely attributed to the multi-factorial machinery that regulates the biological activity and fate of a drug in the body. Genes that encode factors holding determinant roles in cell signaling, metabolic and transport processes, participate in this machinery. It is well defined that these genes are regulated by various factors including age, gender, race, stress, disease, drugs, diet, lipidemic and endocrinological state, among others (2, 5–8). It should be noted also that important modifications in the functional integrity of the cardiovascular, immune, respiratory, gastrointestinal, central nervous and endocrinological systems take place as we grow older, which have a decisive impact on the factors controlling drug activity and therefore, the outcome and toxicity of pharmacotherapy (1–3, 5, 8–10). This is mainly due to the determinant roles these factors play in the regulation of the absorption, distribution, metabolism, excretion and activation of the drugs. In this regulatory loop, stress among other factors, holds a key role in the regulation of several enzymes catalyzing the metabolism of the majority of prescribed medicines (8, 11) (2, 4, 12–15). The age-related modifications in vital functions of the body and stress perception over the years along with the lack of experience in the use of drugs in children and old people, may explain at least in part, the important deviations in drug efficacy and toxicity observed in childhood and elderly (16); (https://www.msdmanuals.com/professional/geriatrics/drug-therapy-in-older-adults/pharmacokinetics-in-older-adults).
Drug Metabolism
Every drug entering the body is recognized as a threat of homeostasis and the detoxifying systems are activated (2, 17). The liver is the major site of drug metabolism, where various enzymes catalyze specific metabolic reactions including oxidation, reduction, hydrolysis, hydration, conjugation, condensation, or isomerization aiming at formation of water soluble molecules that can be readily excreted in urine and bile (2, 18).
During drug metabolism of Phase I, cytochrome P450s (CYPs), flavin-containing monoxygenases (FMO) and epoxide hydrolases (EH) are the main families of enzymes that catalyze the biotransformation of xenobiotics including drugs. In Phase II, the metabolic products of Phase I are conjugated with glucuronic acid, glutathione, sulphate and acetyl groups to form highly water soluble complexes, a process that facilitates and accelerates the detoxification of the organism (19). These conjugation reactions are catalyzed by glucuronosyl-transferases (UGT), glutathione S-transferases (GST), UDP- sulfo-transferases (SULT) and N-acetyl-transferases (NAT), respectively (19). Long-term disturbances of these metabolic processes usually result in intracellular accumulation of metabolites and free radicals that could trigger toxic manifestations (18).
The structure of the drug determines whether one or more of these enzymes will be involved in its metabolism during Phase I. Usually, metabolic biotransformation of a drug modifies drastically the drug’s pharmacokinetic, pharmacodynamic and potentially, toxicity profiles (2, 5, 19–22). In most cases, the metabolism of drugs at Phase I leads to their inactivation. Nonetheless, it is also possible that some metabolites are pharmacologically active, and sometimes they are even more active than the parent compound (2, 8). It should be noted that several active metabolic products may have the potential to induce toxic manifestations including cell death, oxidative stress, tumor initiation and teratogenesis, among others (4, 19, 20, 23–26). There are also inactive or weakly active drugs called pro-drugs, which are converted to pharmacologically active metabolites within the body through metabolic reactions predominantly catalyzed by cytochromes (8, 19, 27–30).
The extensive variation among different patients and populations in the hepatic drug metabolic rate is of paramount clinical interest. The spectrum of factors holding major roles in this diversity includes genetic factors, comorbidities, such as chronic liver disorders, diabetes, advanced heart failure and cancer, as well as drug interactions and mainly those resulting in the acceleration or inhibition of drug metabolism (2, 4, 8).
CYP-catalyzed drug metabolism
CYPs are hemoproteins, which are considered as the most important enzymes in animals and humans that catalyze during Phase I the metabolic biotransformation of the majority of prescribed drugs. They can recognize and subsequently metabolize numerous structures, as they have broad and overlapping substrate specificities. The main CYP isozymes are expressed in all tissues, and predominantly in the liver, where they display the highest capacity. Based on their amino acid sequence homology, the main CYP isozymes that are involved in the metabolism of drugs are arranged into three gene families (CYP1, CYP2 and CYP3) (19, 21, 31). Among the hundreds of CYPs, the CYP1A2, CYP2A6, CYP2B6, CYP2C8/9/19, CYP2D6, CYP2E1 and CYP3A4 are considered as the most important human CYP isoforms (21, 32–34) that catalyze diverse oxidation reactions, such as heteroatom oxidations, hydroxylations, epoxidations, heteroatom dealkylations, oxidative group transfer, cleavage of esters, and dehydrogenations (22, 35). CYPs including CYP2D6, also catalyze the biosynthesis or catabolism of steroid hormones, fat-soluble vitamins, bile acids, fatty acids, eicosanoids and neurotransmitters (23, 36).
Genetic polymorphism and mutations of CYP genes including CYP1A1, CYP2A6, CYP2A13, CYP2C8/9/19, CYP2D6, CYP2B6 and CYP3A4 (19, 37, 38) and the variations in the distribution of the common CYP gene allelic variants that are observed among several ethnic populations, such as Africans, White and African Americans, Asians, Caucasians and Europeans, are among the predominant factors determining to a great extent, the inter-individual and inter-ethnic deviations in drug response and adverse reactions (39, 40). Based on CYP polymorphism, populations could be divided into four phenotypes: the ultra-rapid metabolizers (UM), who display more than two active CYP genes, the extensive metabolizers (EM), who carry two functional CYP genes, the poor-metabolizers (PM), who lack a functional CYP isozyme due to deleted or defective CYP gene and the intermediate metabolizers (IM), who carry one functional and one defective CYP gene or two defective CYP genes. UM cannot reach therapeutic concentrations of drugs in blood and tissues following the usual dosing regime, because they metabolize rapidly the drugs-substrates, whereas PM display slow drug-substrate metabolism that results in the accumulation of the drug in the blood even at usual doses, a condition that may favor toxic manifestations (2). Apparently, CYP gene polymorphism determines a drug’s pharmacokinetic and pharmacodynamic profile (18). From a clinical perspective, the pharmacogenetic profile of a patient in terms of CYP polymorphism could be implemented in improving the outcome of pharmacotherapy and reducing drug toxicity (5, 9, 39).
Stress impact on CYP-catalyzed drug metabolism
Various external and internal factors can modify the regulation of most CYP genes. They can either induce or inhibit the expression of a particular CYP resulting in acceleration or inhibition respectively, of the metabolism of its drug-substrates thus modifying accordingly, the drug’s pharmacokinetic and toxicity outcomes. Among these factors, stress holds a determinant role in the expression and activity of the main CYP isozymes that catalyze the metabolism of the majority of drugs in the market. In our preclinical studies several animal models of stress were employed, such as the mild unpredictable stress for 7 days, the repeated restraint stress (2hrs per day x 4 days) and the maternal deprivation stress for 24hrs. The findings indicated that mainly restraint stress and to a lesser extent maternal deprivation stress can modify constitutive and induced expression levels of CYP3A, CYP2C, CYP2D and CYP1A at an extent that could affect a drug’s pharmacokinetic profile (2, 4, 8, 11); https://www.fda.gov/drugs/drug-interactions-labeling/drug-development-and-drug-interactions-table-substrates-inhibitors-and-inducers).
It is well documented that the stress-mediated effect on each particular CYP gene is stress-specific involving various mechanisms, such as transcriptional regulation by ligand-activated nuclear receptors including PXR (pregnane X receptor), CAR (constitutive androstane receptor) and AhR (aromatic hydrocarbon receptor) (2, 8, 11, 19, 41). In the stress-mediated regulation of CYP genes, the stress-system effectors, epinephrine and norepinephrine released from adrenal medulla and glucocorticoids released from adrenal cortex, hold central roles by activating major signal transduction pathways in the liver, such as the AR/cAMP/PKA, JNK, growth hormone (GH)/signal transducer and activator of transcription 5b (STAT5b), PI3K/AKT/FOXO1β and Glucocorticoid/GR-linked pathways. Activation of these pathways usually results in up-regulation of several transcription factors including hepatocyte nuclear factor 4α (HNF4α), hepatocyte nuclear factor 1α (HNF1α), CAR, PXR, RXR, AhR and peroxisome proliferator activated receptor α (PPARα) that hold determinant roles in CYP regulation (Figure 1; (2, 13, 14, 42–46). It should be noted that prolonged activation of these pathways can result in accumulation of free radicals and other toxic metabolic products in tissues, which along with the stress-mediated reduction of glutathione content, constitute a condition that usually favors the development of severe disease states (2, 4, 19, 47). Within the spectrum of the stress-induced events, alterations in the secretion of hormones, such as GH, prolactin (PRL), thyroid hormones and insulin, the increased release of cytokines/NF-kB and oxidative stress, play determinant roles in CYP regulation by stress (2, 7, 8, 48, 49), (Figure 1). In particular, activation of the hypothalamo-pituitary-adrenal (HPA) axis by stress results in the somatostatin-mediated inhibition of thyroid-releasing hormone (TRH) and thyroid-stimulating hormone (TSH) release and in reduced conversion of thyroid T4 to T3 (active hormone) that in humans, displays a negative control on various CYP genes including those belonging to the CYP3A subfamily (50). It is well defined that the stress-induced somatostatin release and adrenergic receptor stimulation inhibit GH secretion (51–54), an effect of paramount significance in drug metabolism, because GH, among other effects, positively regulates various CYPs, such as CYP3A4 (55), CYP2C and CYP2D (7, 48, 56, 57) and its up-regulating effect is mediated by the GH-pulse activated transcription factor, STAT5b (7, 23, 56, 57). Notably, GH suppression is followed by down-regulation of the insulin-like growth factor (IGF-1), which holds a determinant role in the insulin-mediated down-regulation of various CYPs including CYP3A4, CYP2C, CYP1A and CYP2D (2, 46, 49, 58–60). It is known that stress reduces PRL release (61), which down-regulates several CYPs including those of CYP1 family (62, 63). The effects of glucocorticoids on drug-metabolizing enzymes derive from a combination of distinct mechanisms involving their stimulating effects on the synthesis and activity of several transcription factors, such as CAR, PXR, RXR, AhR, HNF4α and STAT5b, critical CYP regulators (64, 65). It is of interest also to note that the stress-induced release of adrenaline stimulates alpha- and beta-adrenergic receptor-linked pathways and in turn, the release of cytokines, IL-1β, IL-6 and TNFα, that down-regulate hepatic CYP3A and CYP2C (66–68).
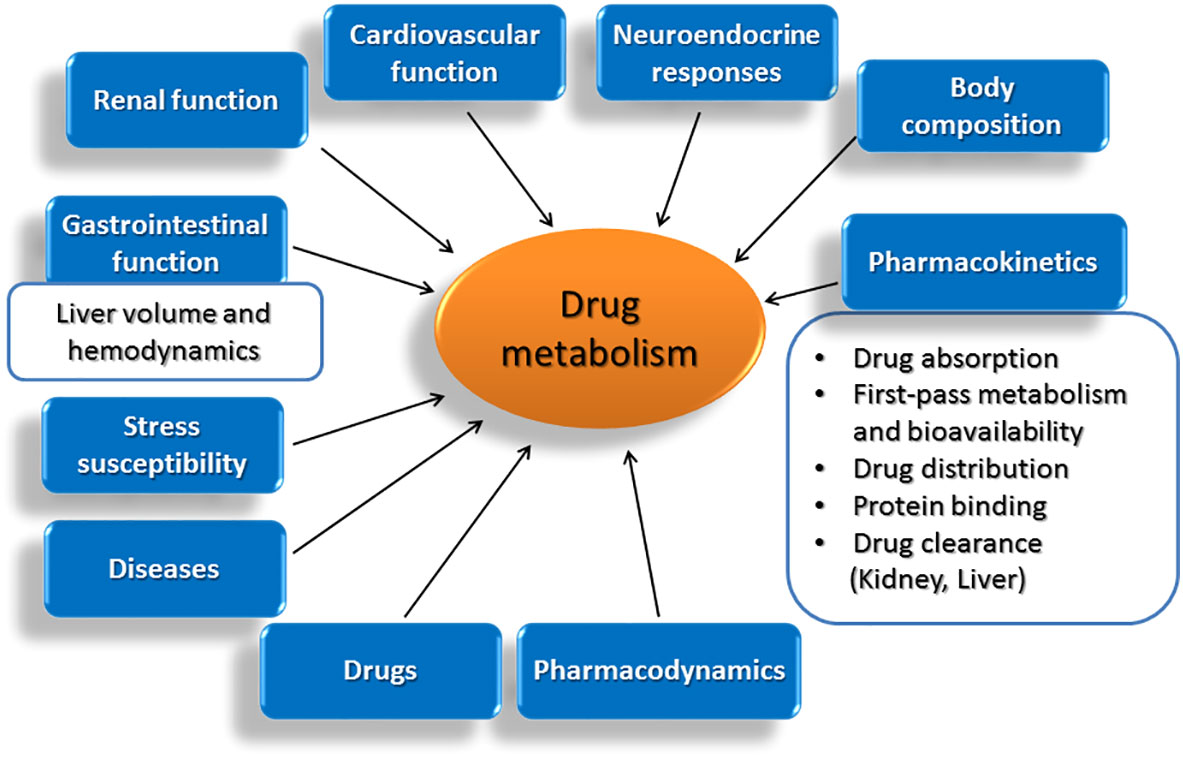
Figure 1 Age-related modifications in vital functions of the body and in other parameters that affect decisively the drug metabolizing capacity of the liver.
The impact of stress on drug metabolism is unique and distinct from that of medicines that usually display dose- and time-dependent specificities (2, 8, 11). Specifically, repeated restraint stress repressed hepatic CYP2E1 and CYP2B constitutive expression, whereas it up-regulated most of the other CYPs including those belonging to CYP1A, CYP2A, CYP2C, CYP2D and CYP3A subfamilies and metabolize over 70% of prescribed drugs (2, 8, 22, 69). It is of note that CYP1A1 and CYP1A2 substrates including several drugs and pre-carcinogens, such as polycyclic aromatic hydrocarbons (PAHs), act as ligands of AhR and induce these CYP1A isozymes (31) thus accelerating their metabolism. On the other hand, inhibition of these isozymes can result in reduced metabolism of their drug-substrates and restricted bioactivation of inactive pre-carcinogens (70–72). In terms of the impact of stress on CYP2D expression, it appears that repeated restraint stress induced CYP2D that to date was considered as a non-inducible enzyme. CYP2D catalyzes the metabolism of most antidepressants, anxiolytic, antipsychotic and antiepileptic drugs along with several antiarrythmic drugs, beta- and calcium channel-blockers (69, 73, 74). The role of CYP2D in the synthesis of dopamine, serotonin and neurosteroids in the brain is well defined and the reason of the current intensive investigation on its role in Parkinson’s disease and other neurodegenerative disorders (4, 75–78). From a clinical perspective, the stress-induced up-regulating effect on the afore-mentioned CYPs could result in acceleration of the metabolism of their drug-substrates and therefore, in sub-therapeutic drug levels in blood and tissues (2, 4, 8). On the other hand though, the stress-induced CYP up-regulation could increase the activation of pro-drug-substrates and therefore, the drug efficacy, whereas the opposite is true for the stress-mediated CYP down-regulation (2, 4, 8).
There is also an indirect involvement of stress in drug metabolism as long-term exposure to uncontrolled stress may trigger several pathophysiological states including cancer, depression, inflammatory diseases, diabetes mellitus and other diseases within the spectrum of metabolic syndrome, which comprise modified hormonal, nutritional, immune and psychological states compared to healthy population (43, 44), condition that has been largely associated with the patient’s hepatic drug metabolizing capacity (2, 4, 8). Based on the accumulating evidence that stress and the major effectors of the stress response, epinephrine and glucocorticoids, play significant and distinct roles in the regulation of the metabolism of numerous drugs in the market, clinicians should consider the stress profile of the patient when designing treatment protocols and dosing regimes. Although stress can not be included in any treatment algorithm, as it does not display the dose- and time- dependent properties of a drug, it is suggested that stress should be alleviated in patients, in order to assure the highest drug efficacy with the less possible adverse reactions (2, 4, 8). Furthermore, drugs with sympathomimetic properties or adrenergic receptor blockers, or those who modify GH, thyroid hormone, insulin and glucocorticoid hormone status should be considered in treatment protocols, because they can decisively modify the pharmacokinetic and pharmacodynamic profiles of drugs and therefore, the efficacy and toxicity of pharmacotherapy (2, 4, 8).
Age-related modifications of critical parameters determining hepatic drug metabolism
Although a comprehensive definition of ageing is not possible, several characteristic alterations including the time-related loss of functional units of organs, the disruption of functional integration between cells and organs and the failure in preserving homeostasis under stress are recognized. In this light, ageing is followed by a progressive accumulation of random modifications in the function of vital systems in the body, which take place at molecular, cellular and tissue level and are followed by decreased viability and increased morbidity (79). Within the spectrum of modifications observed with age, a decline in the functional integrity of organs, such as heart, kidneys and liver, holds central role. Among others, ageing is followed by a progressive reduction in liver volume and blood flow, parameters that drastically affect hepatic drug metabolism. Within pharmacokinetic implications occurring with age and affect the fate and activity of drugs in the body, the composition of the body is included (80), along with alterations in drug absorption, first-pass metabolism and bioavailability (81–84), drug distribution (80, 85), protein binding (79, 86) and drug clearance (79, 87–91). Furthermore, age-related diseases including congestive heart failure and hypertension and the drugs used for their treatment, can also modify drug pharmacokinetics (79, 85, 92–95).
It should be noted that alterations in neuroendocrine responses to stress, which are associated with modified HPA axis function, usually accompany ageing (79). In the aged-brain and in particular, the hippocampus, increased oxidative stress and reduced synthesis of neurotrophins are observed (96, 97). These ageing-dependent alterations in the limbic system can affect critical biobehavioral parameters, such as emotions and coping with stress (98). Notably, susceptibility to stress increases with age via up-regulation of the NADPH oxidase in the hippocampus (99). It should be also taken into account the fact that aged people exhibit increased plasma glucocorticoid levels (GC), which along with epinephrine and norepinephrine organize the response of the stress system to stress stimuli (42, 43, 45, 53, 99). Interestingly, the dysfunction of the stress-regulating neuroendocrine system in elderly people along with the ageing-dependent oxidative stress are equivalent to those in humans exposed to chronic stress (99, 100). Apparently, elderly people are more vulnerable to chronic stress that determines among other parameters, the age-related dynamics in the hepatic CYP-dependent drug metabolism and therefore, the drug efficacy and toxicity (2, 99).
Age-related modifications in the CYP-catalyzed drug metabolism
It is worth noting that about 50% of drugs are prescribed by clinicians without following age-related guidelines and in only 10% of drugs administered in neonates and infants the safety and efficacy has been evaluated. The age-related variations in the activity and toxicity of drugs depend largely on the metabolic capacity of the liver of patients, which is clinically very important, because it determines a drug’s pharmacokinetic and pharmacodynamic profile. Immaturity of drug-metabolizing systems in children and their decline in elderly, have been associated with increased risk of drug toxicity (101).
The drug metabolizing systems are under continuous modifications during our lifespan and this fact should be considered when prescribing drugs in children and the elderly. In particular, ageing is followed by significant modifications in the hepatic CYP isozyme expression pattern in humans, which are CYP isozyme-specific (102) and these modifications can affect drastically the oxidative biotransformation of the majority of drugs in the market, and numerous other xenobiotics including environmental pollutants, pre-carcinogens, carcinogens, and toxicants along with the biosynthesis of several endogenous compounds (101, 102).
There are several studies reporting considerable variations in the hepatic ontogenic gene expression pattern of the major CYPs in children and the elderly. These variations may underlie the fact that children are often less responsive to drugs and are exposed to higher risk in developing adverse reactions related to drugs (103). Of particular interest is the fact that maturational changes in CYP3A ontogeny may affect the clinical outcome of many drugs (~50% of drugs in the market) (104). Therefore, the impact of ontogeny on CYP3A and other CYP isozymes should be considered by the clinicians when prescribing drugs-substrates, inducers or inhibitors of these CYPs (104). But, variations in CYP-catalyzed drug metabolism among individuals at different ages is not only related to ontogeny, but also to exposure to environmental pollutants, the diet, the gender and genetic polymorphism often observed within different ethnicities (2, 6, 103).
Several studies reported an age-related decline in the clearance of medicines undergoing CYP-catalyzed biotransformation in the liver (102, 105–118). In particular, preclinical studies indicated that the CYP-dependent drug metabolism is reduced by about 37-60% in the liver of senescent rats (102, 115, 116, 118, 119). It is also of interest to note that constitutive CYP1A1 expression was detected only in the livers of 3-week-old rats, whereas it was not detectable in older rats (120–122). In humans also, CYP1A1 is constitutively expressed only at early stages of development, while it is not detectable in adults (121).
CYP1A2, CYP2B1 and CYP2E1 were detected at their highest basal protein expression levels in 3-week-old rats, while they were decreased in older rats aged 12- and 26-weeks (122). Hepatic CYP2B1 was further repressed in old rats aged 104 weeks. Similar age-related variations were observed in the CYP1A1-dependent EROD, the CYP1A2-dependent MROD, the CYP2B1/2-dependent PROD and the CYP2E1-dependent PNP activity levels (102, 122, 123).
Hepatic CYP2C11 and CYP3A2 constitutive protein expression and the CYP3A1/2-dependent midazolam activity were drastically induced after puberty in the adulthood and started declining with ageing (122). It was also reported that CYP2C11, the main gender-specific steroid 2α- and 16α-hydroxylase, was not detectable in newborn rat livers. Nonetheless, it was induced at puberty only in males and not in females (124–126). In contrast, the CYP3A1/2-dependent steroid 6β-hydroxylase activity was detectable in the liver of pre-pubertal male and female rats and CYP3A2 protein expression increased until the age of 12-weeks, when it started declining. Interestingly, CYP3A2 expression levels were lower in female rat livers at puberty compared to those at a pre-pubertal state. Apparently, there is an age- and gender-dependent regulation of both, CYP2C11 and CYP3A1/2 expression patterns in the liver of rats (122, 124–126). Notably, the developmental expression pattern of CYP2C11 in males and females is determined by the gender differentiated GH secretion pattern, but this is not the case for CYP3A2 (57, 102, 125).
There are controversial reports on the effect of ageing on the CYP-dependent drug metabolism in humans. In several studies, no significant modifications of specific CYP isozyme expression patterns were observed in the liver of subjects aged between 12 and 73 years (102, 127, 128). In contrast, there are studies reporting that hepatic CYP2E1 and CYP3A content decreased with age (102, 129). Based on data coming from studies employing specific CYP isozyme substrates, it appeared that the rate of CYP1A2 and CYP2C19 drug-substrate metabolism decreases with age, whereas the CYP3A4-, CYP2A6-, CYP2C9- and CYP2D6-dependent substrate metabolism is slightly or not modified (102, 130, 131). As previously mentioned, hepatic CYP1A1 and CYP1A2 expression patterns are similarly modified with age in rats and humans (122).
Fluctuations in hepatic CYP expression and activity patterns observed during development and with age occur at post-transcriptional level and could be profoundly associated with alterations in GH, gonadal hormones, PRL, thyroid hormone, insulin, glucagon and glucocorticoid levels, because these hormones hold determinant roles in CYP regulation. In particular, GH and gonadal hormones determine sex-differentiation in the CYP expression patterns (2, 8, 11, 37, 48, 57, 102, 125, 127, 132–136). In this light, the modified CYP expression patterns observed in pregnancy, at different phases of the estrous cycle (137, 138), in menopause or in subjects following hormonal replacement therapy (137, 138) and in diabetes could be explained (102). The age-related alterations in the hepatic CYP expression pattern may also take place at transcriptional level and are often associated with oxidative stress and HNF1α levels, factors that are induced during the metabolic biotransformation of drugs at Phase I (102, 118, 139, 140).
In drug metabolism, CYP inducibility is a significant parameter. Numerous structurally diverse foreign substances and endogenous compounds can induce several CYP genes, resulting in increased synthesis and activity of the corresponding enzymes and acceleration of the drug-substrates metabolism. Most of CYP genes belonging to families 1-4 can be transcriptionally induced by xenobiotics. In this process, cytosolic AhR and the nuclear receptors CAR, PXR and PPARα (peroxisome proliferator-activated receptor α) hold central roles (2, 11, 60, 102, 141–143).
Regarding the effect of age on CYP induction in humans and rats there are controversial reports (102, 144, 145). Several studies reported decreased (102) or no modified CYP induction by various compounds with age in rats (146). In particular, the phenobarbital-induced effect on several CYP genes including CYP3A and CYP2B, was either decreased with age (147) or remained unaffected (148). It is of note though that the CYP3A inducibility by dexamethasone was strongly reduced with ageing (149–151).
Discussion
Long-term clinical experience demonstrates the high frequency of failure in the treatment protocols followed in pediatric and geriatric patients. This is due in part, to the fact that clinical trials usually engage adults 18-65 years old (152) and thus, the results drown from these studies often do not reflect the potential activity and side effects of a drug in children and elderly patients. This review has focused mainly on alterations in drug metabolism in the elderly, because there is limited information about alterations in drug metabolism at early stages of life including childhood. In addition, there is markedly higher incidence of morbidity with age (over 80% of old people suffer at least from one chronic disease). In comparison to younger individuals, the elderly take about three times more medications and usually, they follow multi-drug treatments (polypharmacy) (153–155), a condition that constitutes a major challenge for health professionals and patients, who often experience memory impairment and either forget to take their medication or take it multiple times (156). It should be noted also that ageing is accompanied by significant alterations in the functional capacity of several organs, such as the liver, kidneys, heart, lungs and intestine, among others (157). All these parameters hold determinant roles in the regulation of drug metabolism in the elderly and may explain the higher incidence of drug-drug interactions and increased incidence of adverse effects and toxicity in old patients (158, 159). It is estimated that about 10% of hospital admissions in the elderly are associated with drug side effects (10, 102, 160–162).
The effects of a drug in the body depend on various factors including body composition, age, stress, gender, race, diet, medication, hormonal state, pathophysiological states, long-term alcohol consumption and smoking that modify fundamental processes determining a drug’s pharmacokinetic and pharmacodynamic profiles (2, 4, 137). In particular, hepatic drug metabolism, mainly at Phase I, is a determinant parameter for drug effectiveness and toxicity and hence, the inter-individual variability in drug metabolism is now considered as a crucial factor in the age-dependent variations in pharmacokinetics and idiosyncratic drug toxicities observed in patients (163, 164); (www.fda.gov/cber/gdens/popharm.pdf).
As previously mentioned, several hormones including GH, cortisol, insulin, PRL and thyroid hormones play important roles in CYP regulation (2, 7, 48, 51, 148) and therefore, the decline in the functional integrity and activity of the hormonal systems that follows ageing is of paramount significance for the pharmacotherapy in elderly patients. Among these age-related modifications, the impairment in HPA axis feedback inhibition with age, which is followed by increased plasma cortisol levels, holds a prevalent role and may explain the increased stress susceptibility in geriatric patients (165). All these modifications observed with age can drastically affect several drug metabolizing enzymes and mainly, several CYP isozymes that catalyze the metabolism of numerous prescribed drugs (2, 4, 11, 51).
In conclusion, the restricted experience in the use of most drugs in children and elderly patients, the decline in memory with age along with the increased stress susceptibility and the decreased activity of various hormonal systems, which follow ageing and largely determine the CYP-dependent drug metabolism, are exceptionally important parameters that should be taken into account when designing treatment regimes in pediatric and geriatric patients, in order to provide them with the appropriate drug dosing regimes and assure the optimal effectiveness and restricted adverse reactions of pharmacotherapy.
Author contributions
MK drafted the original manuscript and EJ critically revised it. Both authors agree for the content of the work and approved it for publication. All authors contributed to the article and approved the submitted version.
Conflict of interest
The authors declare that the research was conducted in the absence of any commercial or financial relationships that could be construed as a potential conflict of interest.
Publisher’s note
All claims expressed in this article are solely those of the authors and do not necessarily represent those of their affiliated organizations, or those of the publisher, the editors and the reviewers. Any product that may be evaluated in this article, or claim that may be made by its manufacturer, is not guaranteed or endorsed by the publisher.
References
1. Zhou SF, Liu JP, Chowbay B. Polymorphism of human cytochrome P450 enzymes and its clinical impact. Drug Metab Rev (2009) 41(2):89–295. doi: 10.1080/03602530902843483
2. Konstandi M, Johnson EO, Lang MA. Consequences of psychophysiological stress on cytochrome P450-catalyzed drug metabolism. Neurosci Biobehav Rev (2014) 45:149–67. doi: 10.1016/j.neubiorev.2014.05.011
3. Thummel KE, Lin YS. Sources of interindividual variability. Methods Mol Biol (2014) 1113:363–415. doi: 10.1007/978-1-62703-758-7_17
4. Konstandi M, Johnson EO, Lang MA. Stress as a potential regulatory factor in the outcome of pharmacotherapy. Front Neurosci (2022) 16:737716. doi: 10.3389/fnins.2022.737716
5. Ingelman-Sundberg M. Pharmacogenetics of cytochrome P450 and its applications in drug therapy: the past, present and future. Trends Pharmacol Sci (2004) 25(4):193–200. doi: 10.1016/j.tips.2004.02.007
6. Pelkonen O, Turpeinen M, Hakkola J, Honkakoski P, Hukkanen J, Raunio H. Inhibition and induction of human cytochrome P450 enzymes: Current status. Arch Toxicol (2008) 82(10):667–715. doi: 10.1007/s00204-008-0332-8
7. Waxman DJ, Holloway MG. Sex differences in the expression of hepatic drug metabolizing enzymes. Mol Pharmacol (2009) 76(2):215–28. doi: 10.1124/mol.109.056705
8. Konstandi M. Psychophysiological stress: a significant parameter in drug pharmacokinetics. Expert Opin Drug Metab Toxicol (2013) 9(10):1317–34. doi: 10.1517/17425255.2013.816283
9. Ingelman-Sundberg M. Human drug metabolising cytochrome P450 enzymes: properties and polymorphisms. Naunyn Schmiedebergs Arch Pharmacol (2004) 369(1):89–104. doi: 10.1007/s00210-003-0819-z
10. Roughead EE, Pratt NL. Impact on drug safety of variation in adherence: the need for routinely reporting measures of dose intensity in medication safety studies using electronic health data. Drug Saf (2015) 38(12):1145–52. doi: 10.1007/s40264-015-0347-z
11. Daskalopoulos EP, Lang MA, Marselos M, Malliou F, Konstandi M. D2-dopaminergic receptor-linked pathways: critical regulators of CYP3A, CYP2C, and CYP2D. Mol Pharmacol (2012) 82(4):668–78. doi: 10.1124/mol.112.078709
12. Konstandi M, Johnson E, Lang MA, Camus-Radon AM, Marselos M. Stress modulates the enzymatic inducibility by benzo[alpha]pyrene in the rat liver. Pharmacol Res (2000) 42(3):205–11. doi: 10.1006/phrs.2000.0675
13. Konstandi M, Johnson EO, Marselos M, Kostakis D, Fotopoulos A, Lang MA. Stress-mediated modulation of B(alpha)P-induced hepatic CYP1A1: role of catecholamines. Chem Biol Interact (2004) 147(1):65–77. doi: 10.1016/j.cbi.2003.10.007
14. Konstandi M, Kostakis D, Harkitis P, Marselos M, Johnson EO, Adamidis K, et al. Role of adrenoceptor-linked signaling pathways in the regulation of CYP1A1 gene expression. Biochem Pharmacol (2005) 69(2):277–87. doi: 10.1016/j.bcp.2004.09.024
15. Konstandi M, Lang MA, Kostakis D, Johnson EO, Marselos M. Predominant role of peripheral catecholamines in the stress-induced modulation of CYP1A2 inducibility by benzo(alpha)pyrene. Bas. Clin Pharmacol Toxicol (2008) 102(1):35–44. doi: 10.1111/j.1742-7843.2007.00154
16. Stephenson T. How children’s responses to drugs differ from adults. Br J Clin Pharmacol (2005) 59(6):670–3. doi: 10.1111/j.1365-2125.2005.02445.x
17. Handschin C, Meyer UA. Induction of drug metabolism: the role of nuclear receptors. Pharmacol Rev (2003) 55(4):649–73. doi: 10.1124/pr.55.4.2
18. Naik A, Belič A, Zanger UM, Rozman D. Molecular interactions between NAFLD and xenobiotic metabolism. Front Genet (2013) 4:2. doi: 10.3389/fgene.2013.00002
19. Gonzalez FJ. Role of cytochromes P450 in chemical toxicity and oxidative stress: studies with CYP2E1. Mutat Res (2005) 569(1-2):101–10. doi: 10.1016/j.mrfmmm.2004.04.021
20. Gonzalez FJ, Gelboin HV. Role of human cytochromes P450 in the metabolic activation of chemical carcinogens and toxins. Drug Metab Rev (1994) 26(1-2):165–83. doi: 10.3109/03602539409029789
21. Nebert DW, Russell DW. Clinical importance of the cytochromes P450. Lancet (2002) 360(9340):1155–62. doi: 10.1016/S0140-6736(02)11203-7
22. Guengerich FP. Cytochrome p450 and chemical toxicology. Chem Res Toxicol (2008) 21(1):70–83. doi: 10.1021/tx700079z
23. Guengerich FP. Cytochromes P450, drugs, and diseases. Mol Interv (2003) 3(4):194–204. doi: 10.1124/mi.3.4.194
24. Cribb AE, Peyrou M, Muruganandan S, Schneider L. The endoplasmic reticulum in xenobiotic toxicity. Drug Metab Rev (2005) 37(3):405–42. doi: 10.1080/03602530500205135
25. Ingelman-Sundberg M, Rodriguez-Antona C. Pharmacogenetics of drug-metabolizing enzymes: implications for a safer and more effective drug therapy. Philos Trans R Soc Lond B Biol Sci (2005) 360(1460):1563–70. doi: 10.1098/rstb.2005.1685
26. Gonzalez FJ, Yu AM. Cytochrome P450 and xenobiotic receptor humanized mice. Annu Rev Pharmacol Toxicol (2006) 46:41–64. doi: 10.1146/annurev.pharmtox.45.120403.100007
27. Sjövall J, Magni L, Vinnars E. Bioavailability of bacampicillin and talampicillin, two oral prodrugs of ampicillin. Antimicrob Agents Chemother (1981) 20(6):837–8. doi: 10.1128/AAC.20.6.837
28. Chen CS, Lin JT, Goss KA, He YA, Halpert JR, Waxman DJ. Activation of the anticancer prodrugs cyclophosphamide and ifosfamide: identification of cytochrome P450 2B enzymes and site-specific mutants with improved enzyme kinetics. Mol Pharmacol (2004) 65(5):1278–85. doi: 10.1124/mol.65.5.1278
29. Rooseboom M, Commandeur JN, Vermeulen NP. Enzyme-catalyzed activation of anticancer prodrugs. Pharmacol Rev (2004) 56(1):53–102. doi: 10.1124/pr.56.1.3
30. Sozio P, Cerasa LS, Abbadessa A, Di Stefano A. Designing prodrugs for the treatment of parkinson’s disease. Expert Opin Drug Discovery (2012) 7(5):385–406. doi: 10.1517/17460441.2012.677025
31. Nebert DW. Suggestions for the nomenclature of human alleles: relevance to ecogenetics, pharmacogenetics and molecular epidemiology. Pharmacogenetics (2000) 10(4):279–90. doi: 10.1097/00008571-200006000-00001
32. Wormhoudt LW, Commandeur JN, Vermeulen NP. Genetic polymorphisms of human n-acetyltransferase, cytochrome P450, glutathione-s-transferase, and epoxide hydrolase enzymes: relevance to xenobiotic metabolism and toxicity. Crit Rev Toxicol (1999) 29(1):59–124. doi: 10.1080/10408449991349186
33. Lin Y, Lu P, Tang C, Mei Q, Sandig G, Rodrigues AD, et al. Substrate inhibition kinetics for cytochrome P450-catalyzed reactions. Drug Metab Disposit.: Biol fate Chem. (2001) 29(4 Pt 1):368–74.
34. Turpeinen M, Zanger UM. Cytochrome P450 2B6: function, genetics, and clinical relevance. Drug Metabol Drug Interact (2012) 27(4):185–97. doi: 10.1515/dmdi-2012-0027
35. Hollenberg PF. Mechanisms of cytochrome P450 and peroxidase-catalyzed xenobiotic metabolism. FASEB journal: Off Publ Fed Am Societies Exp Biol (1992) 6(2):686–94. doi: 10.1096/fasebj.6.2.1537457
36. Spatzenegger M, Jaeger W. Clinical importance of hepatic cytochrome P450 in drug metabolism. Drug Metab Rev (1995) 27(3):397–417. doi: 10.3109/03602539508998329
37. Lamba V, Lamba J, Yasuda K, Strom S, Davila J, Hancock ML, et al. Hepatic CYP2B6 expression: gender and ethnic differences and relationship to CYP2B6 genotype and CAR (constitutive androstane receptor) expression. J Pharmacol Exp Ther (2003) 307(3):906–22. doi: 10.1124/jpet.103.054866
38. Bahar MA, Hak E, Bos JHJ, Borgsteede SD, Wilffert B. The burden and management of cytochrome P450 2D6 (CYP2D6)-mediated drug-drug interaction (DDI): co-medication of metoprolol and paroxetine or fluoxetine in the elderly. Pharmacoepidemiol Drug Saf (2017) 26(7):752–65. doi: 10.1002/pds.4200
39. Ingelman-Sundberg M, Sim SC, Gomez A, Rodriguez-Antona C. Influence of cytochrome P450 polymorphisms on drug therapies: pharmacogenetic, pharmacoepigenetic and clinical aspects. Pharmacol Ther (2007) 116(3):496–526. doi: 10.1016/j.pharmthera.2007.09.004
40. Jaja C, Burke W, Thummel K, Edwards K, Veenstra DL. Cytochrome P450 enzyme polymorphism frequency in indigenous and native American populations: a systematic review. Public Health Genomics (2008) 11(3):141–9. doi: 10.1159/000113876
41. Nebert DW, Gonzalez FJ. P450 genes and evolutionary genetics. Hosp Pract (Off Ed) (1987) 22(3):63–74.
42. Chrousos GP, Gold PW. The concepts of stress and stress system disorders. overview of physical and behavioral homeostasis. Jama (1992) 267(9):1244–52. doi: 10.1001/jama.1992.03480090092034
43. Johnson EO, Kamilaris TC, Chrousos GP, Gold PW. Mechanisms of stress: A dynamic overview of hormonal and behavioral homeostasis. Neurosci Biobehav Rev (1992) 16(2):115–30. doi: 10.1016/S0149-7634(05)80175-7
44. Chrousos GP. Stress and disorders of the stress system. Nat Rev Endocrinol (2009) 5(7):374–81. doi: 10.1038/nrendo.2009.106
45. Chrousos GP, Kino T. Glucocorticoid signaling in the cell. expanding clinical implications to complex human behavioral and somatic disorders. Ann N Y Acad Sci (2009) 1179:153–66. doi: 10.1111/j.1749-6632.2009.04988.x
46. Daskalopoulos EP, Malliou F, Rentesi G, Marselos M, Lang MA, Konstandi M. Stress is a critical player in CYP3A, CYP2C, and CYP2D regulation: role of adrenergic receptor signaling pathways. Am J Physiol Endocrinol Metab (2012) 303(1):E40–54. doi: 10.1152/ajpendo.00545.2011
47. Konstandi M, Marselos M, Radon-Camus AM, Johnson E, Lang MA. The role of stress in the regulation of drug metabolizing enzymes in mice. Eur J Drug Metab Pharmacokinet (1998) 23(4):483–90. doi: 10.1007/BF03189999
48. Waxman DJ, Pampori NA, Ram PA, Agrawal AK, Shapiro BH. Interpulse interval in circulating growth hormone patterns regulates sexually dimorphic expression of hepatic cytochrome P450. Proc Natl Acad Sci USA (1991) 88(15):6868–72. doi: 10.1073/pnas.88.15.6868
49. Woodcroft KJ, Hafner MS, Novak RF. Insulin signaling in the transcriptional and posttranscriptional regulation of CYP2E1 expression. Hepatology (2002) 35(2):263–73. doi: 10.1053/jhep.2002.30691
50. Takahashi N, Inui N, Morita H, Takeuchi K, Uchida S, Watanabe H, et al. Effect of thyroid hormone on the activity of CYP3A enzyme in humans. J Clin Pharmacol (2010) 50(1):88–93. doi: 10.1177/0091270009344336
51. Tuomisto J, Männistö P. Neurotransmitter regulation of anterior pituitary hormones. Pharmacol Rev (1985) 37(3):249–332.
52. McMahon CD, Chapin LT, Radcliff RP, Lookingland KJ, Tucker HA. Somatostatin inhibits alpha-2-adrenergic-induced secretion of growth hormone-releasing hormone. Neuroendocrinology (2001) 73(6):417–25. doi: 10.1159/000054660
53. Tsigos C, Chrousos GP. Hypothalamic-pituitary-adrenal axis, neuroendocrine factors and stress. J Psychosom Res (2002) 53(4):865–71. doi: 10.1016/S0022-3999(02)00429-4
54. Charmandari E, Kino T, Souvatzoglou E, Chrousos GP. Pediatric stress: hormonal mediators and human development. Horm Res (2003) 59(4):161–79. doi: 10.1159/000069325
55. Flierl MA, Rittirsch D, Huber-Lang M, Sarma JV, Ward PA. Catecholamines-crafty weapons in the inflammatory arsenal of immune/inflammatory cells or opening pandora’s box? Mol Med (2008) 14(3-4):195–204. doi: 10.2119/2007-00105.Flierl
56. Ram PA, Waxman DJ. Hepatic P450 expression in hypothyroid rats: differential responsiveness of male-specific P450 forms 2a (IIIA2), 2c (IIC11), and RLM2 (IIA2) to thyroid hormone. Mol Endocrinol (1991) 5(1):13–20. doi: 10.1210/mend-5-1-13
57. Wiwi CA, Waxman DJ. Role of hepatocyte nuclear factors in growth hormone-regulated, sexually dimorphic expression of liver cytochromes P450. Growth Factors (2004) 22(2):79–88. doi: 10.1080/08977190410001715172
58. Woodcroft KJ, Novak RF. Insulin effects on CYP2E1, 2B, 3A, and 4A expression in primary cultured rat hepatocytes. Chem Biol Interact (1997) 107(1-2):75–91. doi: 10.1016/S0009-2797(97)00075-6
59. Kim SK, Novak RF. The role of intracellular signaling in insulin-mediated regulation of drug metabolizing enzyme gene and protein expression. Pharmacol Ther (2007) 113(1):88–120. doi: 10.1016/j.pharmthera.2006.07.004
60. Harkitis P, Daskalopoulos EP, Malliou F, Lang MA, Marselos M, Fotopoulos A, et al. Dopamine D2-receptor antagonists down-regulate CYP1A1/2 and CYP1B1 in the rat liver. PloS One (2015) 10(10):e0128708. doi: 10.1371/journal.pone.0128708
61. Bibi S, Shah M, Malik MO, Goosens KA. T3 is linked to stress-associated reduction of prolactin in lactating women. J Neuroendocrinol (2021) 33(8):e13003. doi: 10.1111/jne.13003
62. Yamazoe Y, Shimada M, Murayama N, Kato R. Suppression of levels of phenobarbital-inducible rat liver cytochrome p-450 by pituitary hormone. J Biol Chem (1987) 262(15):7423–8. doi: 10.1016/S0021-9258(18)48254-3
63. Fitzgerald P, Dinan TG. Prolactin and dopamine: what is the connection? a review article. J Psychopharmacol (2008) 22(2 Suppl):12–9. doi: 10.1177/0269216307087148
64. Gross KL, Cidlowski JA. Tissue-specific glucocorticoid action: a family affair. Trends Endocrinol Metab (2008) 19(9):331–9. doi: 10.1016/j.tem.2008.07.009
65. Dvorak Z, Pavek P. Regulation of drug-metabolizing cytochrome P450 enzymes by glucocorticoids. Drug Metab Rev (2010) 42(4):621–35. doi: 10.3109/03602532.2010.484462
66. Carlson TJ, Billings RE. Role of nitric oxide in the cytokine-mediated regulation of cytochrome p-450. Mol Pharmacol (1996) 49(5):796–801.
67. Jover R, Bort R, Gómez-Lechón MJ, Castell JV. Down-regulation of human CYP3A4 by the inflammatory signal interleukin-6: molecular mechanism and transcription factors involved. FASEB journal: Off Publ Fed Am Societies Exp Biol (2002) 16(13):1799–801. doi: 10.1096/fj.02-0195fje
68. Assenat E, Gerbal-Chaloin S, Larrey D, Saric J, Fabre J-M, Maurel P, et al. Interleukin 1β inhibits CAR-induced expression of hepatic genes involved in drug and bilirubin clearance. Hepatology (2004) 40(4):951–60. doi: 10.1002/hep.20387
69. Rendic S, Guengerich FP. Update information on drug metabolism systems–2009, part II: summary of information on the effects of diseases and environmental factors on human cytochrome P450 (CYP) enzymes and transporters. Curr Drug Metab (2010) 11(1):4–84. doi: 10.2174/138920010791110917
70. Tardiff RG, Lohman PHM, Wogan GN, International Council of Scientific Unions, Scientific Committee on Problems of the E, United Nations Environment P, et al. Methods to assess DNA damage and repair: interspecies comparisons. Chichester: Wiley (1994).
71. Shou M, Krausz KW, Gonzalez FJ, Gelboin HV. Metabolic activation of the potent carcinogen dibenzo[a,l]pyrene by human recombinant cytochromes P450, lung and liver microsomes. Carcinogenesis (1996) 17(11):2429–33. doi: 10.1093/carcin/17.11.2429
73. Niwa T, Okada K, Hiroi T, Imaoka S, Narimatsu S, Funae Y. Effect of psychotropic drugs on the 21-hydroxylation of neurosteroids, progesterone and allopregnanolone, catalyzed by rat CYP2D4 and human CYP2D6 in the brain. Biol Pharm Bull (2008) 31(3):348–51. doi: 10.1248/bpb.31.348
74. Wang X, Li J, Dong G, Yue J. The endogenous substrates of brain CYP2D. Eur J Pharmacol (2014) 724:211–8. doi: 10.1016/j.ejphar.2013.12.025
75. Tsuneoka Y, Matsuo Y, Higuchi R, Ichikawa Y. Characterization of the cytochrome p-450IID subfamily in bovine liver. nucleotide sequences and microheterogeneity. Eur J Biochem (1992) 208(3):739–46. doi: 10.1111/j.1432-1033.1992.tb17242.x
76. Mann A, Miksys S, Lee A, Mash DC, Tyndale RF. Induction of the drug metabolizing enzyme CYP2D in monkey brain by chronic nicotine treatment. Neuropharmacology (2008) 55(7):1147–55. doi: 10.1016/j.neuropharm.2008.07.017
77. Anna Haduch A, Bromek E, Daniel WA. Role of brain cytochrome P450 (CYP2D) in the metabolism of monoaminergic neurotransmitters. Pharmacol Rep (2013) 65(6):1519–28. doi: 10.1016/S1734-1140(13)71513-5
78. Ur Rasheed MS, Mishra AK, Singh MP. Cytochrome P450 2D6 and parkinson’s disease: polymorphism, metabolic role, risk and protection. Neurochemical Res (2017) 42(12):3353–61. doi: 10.1007/s11064-017-2384-8
79. Mangoni AA, Jackson SH. Age-related changes in pharmacokinetics and pharmacodynamics: basic principles and practical applications. Br J Clin Pharmacol (2004) 57(1):6–14. doi: 10.1046/j.1365-2125.2003.02007.x
80. Fülöp T Jr., Wórum I, Csongor J, Fóris G, Leövey A. Body composition in elderly people. i. determination of body composition by multiisotope method and the elimination kinetics of these isotopes in healthy elderly subjects. Gerontology (1985) 31(1):6–14. doi: 10.1159/000212676
81. Castleden CM, George CF. The effect of ageing on the hepatic clearance of propranolol. Br J Clin Pharmacol (1979) 7(1):49–54. doi: 10.1111/j.1365-2125.1979.tb00896.x
82. Robertson DR, Waller DG, Renwick AG, George CF. Age-related changes in the pharmacokinetics and pharmacodynamics of nifedipine. Br J Clin Pharmacol (1988) 25(3):297–305. doi: 10.1111/j.1365-2125.1988.tb03307.x
83. Greenblatt DJ, Harmatz JS, Shapiro L, Engelhardt N, Gouthro TA, Shader RI. Sensitivity to triazolam in the elderly. N Engl J Med (1991) 324(24):1691–8. doi: 10.1056/NEJM199106133242403
84. Anantharaju A, Feller A, Chedid A. Aging liver. a review. Gerontology (2002) 48(6):343–53. doi: 10.1159/000065506
85. Cusack B, Kelly J, O’Malley K, Noel J, Lavan J, Horgan J. Digoxin in the elderly: pharmacokinetic consequences of old age. Clin Pharmacol Ther (1979) 25(6):772–6. doi: 10.1002/cpt1979256772
86. Fu A, Nair KS. Age effect on fibrinogen and albumin synthesis in humans. Am J Physiol (1998) 275(6):E1023–1030. doi: 10.1152/ajpendo.1998.275.6.E1023
87. Lumholtz B, Kampmann TJ, Siersbaek-Nielsen K, Hansen JM. Dose regimen of kanamycin and gentamicin. Acta Med Scand (1974) 196(6):521–4. doi: 10.1111/j.0954-6820.1974.tb01054.x
88. Portnoi VA. Digitalis delirium in elderly patients. J Clin Pharmacol (1979) 19(11-12):747–50. doi: 10.1002/j.1552-4604.1979.tb01646.x
89. Triggs EJ, Johnson JM, Learoyd B. Absorption and disposition of ampicillin in the elderly. Eur J Clin Pharmacol (1980) 18(2):195–8. doi: 10.1007/BF00561590
90. Somogyi A, Hewson D, Muirhead M, Bochner F. Amiloride disposition in geriatric patients: importance of renal function. Br J Clin Pharmacol (1990) 29(1):1–8. doi: 10.1111/j.1365-2125.1990.tb03595.x
91. Fliser D, Bischoff I, Hanses A, Block S, Joest M, Ritz E, et al. Renal handling of drugs in the healthy elderly. creatinine clearance underestimates renal function and pharmacokinetics remain virtually unchanged. Eur J Clin Pharmacol (1999) 55(3):205–11. doi: 10.1007/s002280050619
92. Ewy GA, Kapadia GG, Yao L, Lullin M, Marcus FI. Digoxin metabolism in the elderly. Circulation (1969) 39(4):449–53. doi: 10.1161/01.CIR.39.4.449
93. Kerremans AL, Tan Y, van Baars H, van Ginneken CA, Gribnau FW. Furosemide kinetics and dynamics in aged patients. Clin Pharmacol Ther (1983) 34(2):181–9. doi: 10.1038/clpt.1983.150
94. Cody RJ, Torre S, Clark M, Pondolfino K. Age-related hemodynamic, renal, and hormonal differences among patients with congestive heart failure. Arch Intern Med (1989) 149(5):1023–8. doi: 10.1001/archinte.1989.00390050029006
95. Kelly JG, O’Malley K. Clinical pharmacokinetics of the newer ACE inhibitors. a review. Clin Pharmacokinet (1990) 19(3):177–96. doi: 10.2165/00003088-199019030-00003
96. Berk M, Kapczinski F, Andreazza AC, Dean OM, Giorlando F, Maes M, et al. Pathways underlying neuroprogression in bipolar disorder: focus on inflammation, oxidative stress and neurotrophic factors. Neurosci Biobehav Rev (2011) 35(3):804–17. doi: 10.1016/j.neubiorev.2010.10.001
97. Rowland T, Perry BI, Upthegrove R, Barnes N, Chatterjee J, Gallacher D, et al. Neurotrophins, cytokines, oxidative stress mediators and mood state in bipolar disorder: systematic review and meta-analyses. Br J Psychiatry (2018) 213(3):514–25. doi: 10.1192/bjp.2018.144
98. McEwen BS, Gianaros PJ. Central role of the brain in stress and adaptation: links to socioeconomic status, health, and disease. Ann N Y Acad Sci (2010) 1186:190–222. doi: 10.1111/j.1749-6632.2009.05331.x
99. Lee J-E, Kwon H-J, Choi J, Seo J-S, Han P-L. Aging increases vulnerability to stress-induced depression via upregulation of NADPH oxidase in mice. Commun Biol (2020) 3(1):292. doi: 10.1038/s42003-020-1010-5
100. Yiallouris A, Tsioutis C, Agapidaki E, Zafeiri M, Agouridis AP, Ntourakis D, et al. Adrenal aging and its implications on stress responsiveness in humans. Front Endocrinol (Lausanne) (2019) 10:54. doi: 10.3389/fendo.2019.00054
101. Thakur A, Parvez MM, Leeder JS, Prasad B. Ontogeny of drug-metabolizing enzymes. In: Nagar S, Argikar UA, Tweedie D, editors. Enzyme kinetics in drug metabolism: fundamentals and applications. New York, NY: Springer US (2021). p. 551–593.
102. Wauthier V, Verbeeck RK, Calderon PB. The effect of ageing on cytochrome p450 enzymes: consequences for drug biotransformation in the elderly. Curr Med Chem (2007) 14(7):745–57. doi: 10.2174/092986707780090981
103. Hart SN, Cui Y, Klaassen CD, Zhong X-b. Three patterns of cytochrome P450 gene expression during liver maturation in mice. Drug Metab Disposit. (2009) 37(1):116–21. doi: 10.1124/dmd.108.023812
104. de Wildt SN, Kearns GL, Leeder JS, van den Anker JN. Cytochrome P450 3A. Clin Pharmacokinet (1999) 37(6):485–505. doi: 10.2165/00003088-199937060-00004
105. Kato R, Takanaka A. Effect of phenobarbital on electron transport system, oxidation and reduction of drugs in liver microsomes of rats of different age. J Biochem (1968) 63(3):406–8.
106. Bach B, Hansen JM, Kampmann JP, Rasmussen SN, Skovsted L. Disposition of antipyrine and phenytoin correlated with age and liver volume in man. Clin Pharmacokinet (1981) 6(5):389–96. doi: 10.2165/00003088-198106050-00005
107. Kamataki T, Maeda K, Shimada M, Kitani K, Nagai T, Kato R. Age-related alteration in the activities of drug-metabolizing enzymes and contents of sex-specific forms of cytochrome p-450 in liver microsomes from male and female rats. J Pharmacol Exp Ther (1985) 233(1):222–8.
108. Morgan ET, MacGeoch C, Gustafsson JA. Hormonal and developmental regulation of expression of the hepatic microsomal steroid 16 alpha-hydroxylase cytochrome p-450 apoprotein in the rat. J Biol Chem (1985) 260(22):11895–8. doi: 10.1016/S0021-9258(17)38963-9
109. Watkins PB, Murray SA, Winkelman LG, Heuman DM, Wrighton SA, Guzelian PS. Erythromycin breath test as an assay of glucocorticoid-inducible liver cytochromes p-450. studies in rats and patients. J Clin Invest (1989) 83(2):688–97.
110. Belpaire FM, de Smet F, Vynckier LJ, Vermeulen AM, Rosseel MT, Bogaert MG, et al. Effect of aging on the pharmcokinetics of atenolol, metoprolol and propranolol in the rat. J Pharmacol Exp Ther (1990) 254(1):116–22.
111. Imaoka S, Fujita S, Funae Y. Age-dependent expression of cytochrome p-450s in rat liver. Biochim Biophys Acta (1991) 1097(3):187–92. doi: 10.1016/0925-4439(91)90034-7
112. Hämmerlein A, Derendorf H, Lowenthal DT. Pharmacokinetic and pharmacodynamic changes in the elderly. clinical implications. Clin Pharmacokinet (1998) 35(1):49–64. doi: 10.2165/00003088-199835010-00004
113. Le Couteur DG, McLean AJ. The aging liver. drug clearance and an oxygen diffusion barrier hypothesis. Clin Pharmacokinet (1998) 34(5):359–73. doi: 10.2165/00003088-199834050-00003
114. Tanaka E. In vivo Age-related changes in hepatic drug-oxidizing capacity in humans. J Clin Pharm Ther (1998) 23(4):247–55. doi: 10.1046/j.1365-2710.1998.00164.x
115. Dhir RN, Shapiro BH. Interpulse growth hormone secretion in the episodic plasma profile causes the sex reversal of cytochrome P450s in senescent male rats. Proc Natl Acad Sci U.S.A. (2003) 100(25):15224–8. doi: 10.1073/pnas.2434273100
116. Warrington JS, Court MH, Greenblatt DJ, von Moltke LL. Phenacetin and chlorzoxazone biotransformation in aging male Fischer 344 rats. J Pharm Pharmacol (2004) 56(6):819–25.
117. Wauthier V, Verbeeck RK, Buc Calderon P. Age-related changes in the protein and mRNA levels of CYP2E1 and CYP3A isoforms as well as in their hepatic activities in wistar rats. what role for oxidative stress? Arch Toxicol (2004) 78(3):131–8. doi: 10.1007/s00204-003-0526-z
118. Wauthier V, Verbeeck RK, Calderon PB. Decreased CYP3A2 expression and activity in senescent male wistar rats: is there a role for HNF4alpha? Exp Gerontol (2006) 41(9):846–54. doi: 10.1016/j.exger.2006.06.046
119. Wauthier V, Schenten V, Verbeeck RK, Calderon PB. Ageing is associated with increased expression but decreased activity of CYP2E1 in male wistar rats. Life Sci (2006) 79(20):1913–20. doi: 10.1016/j.lfs.2006.06.046
120. Omiecinski CJ, Redlich CA, Costa P. Induction and developmental expression of cytochrome P450IA1 messenger RNA in rat and human tissues: detection by the polymerase chain reaction. Cancer Res (1990) 50(14):4315–21.
121. Kitada M, Taneda M, Itahashi K, Kamataki T. Four forms of cytochrome p-450 in human fetal liver: purification and their capacity to activate promutagens. Jpn J Cancer Res (1991) 82(4):426–32. doi: 10.1111/j.1349-7006.1991.tb01866.x
122. Yun KU, Oh SJ, Oh JM, Kang KW, Myung CS, Song GY, et al. Age-related changes in hepatic expression and activity of cytochrome P450 in male rats. Arch Toxicol (2010) 84(12):939–46. doi: 10.1007/s00204-010-0520-1
123. Tani Y, Yamamoto H, Kawaji A, Mizuno H, Fukushige J, Hosokawa T, et al. Hepatic cytochrome P450 and flavin-containing monooxygenase in male nts : mini rat, a transgenic rat carrying antisense RNA transgene for rat growth hormone. Toxicol Lett (1999) 106(2-3):159–69. doi: 10.1016/S0378-4274(99)00055-7
124. Waxman DJ. Rat hepatic cytochrome p-450 isoenzyme 2c. identification as a male-specific, developmentally induced steroid 16 alpha-hydroxylase and comparison to a female-specific cytochrome p-450 isoenzyme. J Biol Chem (1984) 259(24):15481–90.
125. Waxman DJ, Dannan GA, Guengerich FP. Regulation of rat hepatic cytochrome p-450: age-dependent expression, hormonal imprinting, and xenobiotic inducibility of sex-specific isoenzymes. Biochemistry (1985) 24(16):4409–17. doi: 10.1021/bi00337a023
126. Gonzalez FJ, Song BJ, Hardwick JP. Pregnenolone 16 alpha-carbonitrile-inducible p-450 gene family: gene conversion and differential regulation. Mol Cell Biol (1986) 6(8):2969–76. doi: 10.1128/mcb.6.8.2969-2976.1986
127. Shimada T, Yamazaki H, Mimura M, Inui Y, Guengerich FP. Interindividual variations in human liver cytochrome p-450 enzymes involved in the oxidation of drugs, carcinogens and toxic chemicals: studies with liver microsomes of 30 Japanese and 30 caucasians. J Pharmacol Exp Ther (1994) 270(1):414–23.
128. Sotaniemi EA, Arranto AJ, Pelkonen O, Pasanen M. Age and cytochrome P450-linked drug metabolism in humans: an analysis of 226 subjects with equal histopathologic conditions. Clin Pharmacol Ther (1997) 61(3):331–9. doi: 10.1016/S0009-9236(97)90166-1
129. George J, Byth K, Farrell GC. Age but not gender selectively affects expression of individual cytochrome P450 proteins in human liver. Biochem Pharmacol (1995) 50(5):727–30. doi: 10.1016/0006-2952(95)00192-3
130. Kinirons MT, Crome P. Clinical pharmacokinetic considerations in the elderly. an update. Clin Pharmacokinet (1997) 33(4):302–12. doi: 10.2165/00003088-199733040-00005
131. Cusack BJ. Pharmacokinetics in older persons. Am J Geriatr Pharmacother (2004) 2(4):274–302. doi: 10.1016/j.amjopharm.2004.12.005
132. Noshiro M, Negishi M. Pretranslational regulation of sex-dependent testosterone hydroxylases by growth hormone in mouse liver. J Biol Chem (1986) 261(34):15923–7. doi: 10.1016/S0021-9258(18)66653-0
133. Legraverend C, Mode A, Westin S, Ström A, Eguchi H, Zaphiropoulos PG, et al. Transcriptional regulation of rat p-450 2C gene subfamily members by the sexually dimorphic pattern of growth hormone secretion. Mol Endocrinol (1992) 6(2):259–66. doi: 10.1210/mend.6.2.1569969
134. Shapiro BH, Agrawal AK, Pampori NA. Gender differences in drug metabolism regulated by growth hormone. Int J Biochem Cell Biol (1995) 27(1):9–20. doi: 10.1016/1357-2725(94)00056-5
135. Cheung C, Akiyama TE, Kudo G, Gonzalez FJ. Hepatic expression of cytochrome P450s in hepatocyte nuclear factor 1-alpha (HNF1alpha)-deficient mice. Biochem Pharmacol (2003) 66(10):2011–20. doi: 10.1016/S0006-2952(03)00586-0
136. Wolbold R, Klein K, Burk O, Nüssler AK, Neuhaus P, Eichelbaum M, et al. Sex is a major determinant of CYP3A4 expression in human liver. Hepatology (2003) 38(4):978–88. doi: 10.1002/hep.1840380424
137. Konstandi M, Cheng J, Gonzalez FJ. Sex steroid hormones regulate constitutive expression of Cyp2e1 in female mouse liver. Am J Physiol Endocrinol Metab (2013) 304(10):E1118–1128. doi: 10.1152/ajpendo.00585.2012
138. Konstandi M, Andriopoulou CE, Cheng J, Gonzalez FJ. Sex steroid hormones differentially regulate CYP2D in female wild-type and CYP2D6-humanized mice. J Endocrinol (2020) 245(2):301–14. doi: 10.1530/JOE-19-0561
139. Ueno T, Gonzalez FJ. Transcriptional control of the rat hepatic CYP2E1 gene. Mol Cell Biol (1990) 10(9):4495–505. doi: 10.1128/mcb.10.9.4495-4505.1990
140. Wauthier V, Verbeeck RK, Buc Calderon P. The use of precision-cut liver slices from male wistar rats as a tool to study age related changes in CYP3A induction and in formation of paracetamol conjugates. Toxicol In Vitro (2004) 18(6):879–85. doi: 10.1016/j.tiv.2004.04.013
141. Sonntag WE, Steger RW, Forman LJ, Meites J. Decreased pulsatile release of growth hormone in old male rats. Endocrinology (1980) 107(6):1875–9. doi: 10.1210/endo-107-6-1875
142. Nebert DW, Petersen DD, Puga A. Human AH locus polymorphism and cancer: inducibility of CYP1A1 and other genes by combustion products and dioxin. Pharmacogenetics (1991) 1(2):68–78. doi: 10.1097/00008571-199111000-00003
143. Konstandi M, Kostakis D, Harkitis P, Johnson EO, Marselos M, Adamidis K, et al. Benzo(alpha)pyrene-induced up-regulation of CYP1A2 gene expression: role of adrenoceptor-linked signaling pathways. Life Sci (2006) 79(4):331–41. doi: 10.1016/j.lfs.2006.01.012
144. Park KS, Sohn DH, Veech RL, Song BJ. Translational activation of ethanol-inducible cytochrome P450 (CYP2E1) by isoniazid. Eur J Pharmacol (1993) 248(1):7–14. doi: 10.1016/0926-6917(93)90019-M
145. Bardag-Gorce F, Li J, French BA, French SW. Ethanol withdrawal induced CYP2E1 degradation in vivo, blocked by proteasomal inhibitor PS-341. Free Radic Biol Med (2002) 32(1):17–21. doi: 10.1016/S0891-5849(01)00768-7
146. McMartin DN, O’Connor JA Jr., Fasco MJ, Kaminsky LS. Influence of aging and induction of rat liver and kidney microsomal mixed function oxidase systems. Toxicol Appl Pharmacol (1980) 54(3):411–9. doi: 10.1016/0041-008X(80)90168-4
147. Birnbaum LS, Baird MB. Induction of hepatic mixed function oxidases in senescent rodents. Exp Gerontol (1978) 13(5):299–303. doi: 10.1016/0531-5565(78)90038-4
148. Waxman DJ, Ram PA, Pampori NA, Shapiro BH. Growth hormone regulation of male-specific rat liver P450s 2A2 and 3A2: induction by intermittent growth hormone pulses in male but not female rats rendered growth hormone deficient by neonatal monosodium glutamate. Mol Pharmacol (1995) 48(5):790–7.
149. Kao J, Hudson P. Induction of the hepatic cytochrome p-450-dependent mono-oxygenase system in young and geriatric rats. Biochem Pharmacol (1980) 29(8):1191–4. doi: 10.1016/0006-2952(80)90417-7
150. Schmucker DL, Wang RK. Effects of aging and phenobarbital on the rat liver microsomal drug-metabolizing system. Mech Ageing Dev (1981) 15(2):189–202. doi: 10.1016/0047-6374(81)90074-9
151. Schmucker DL, Wang RK. Age-dependent alterations in rat liver microsomal NADPH-cytochrome c (P-450) reductase: a qualitative and quantitative analysis. Mech Ageing Dev (1983) 21(2):137–56. doi: 10.1016/0047-6374(83)90070-2
152. Shenoy P, Harugeri A. Elderly patients’ participation in clinical trials. Perspect Clin Res (2015) 6(4):184–9. doi: 10.4103/2229-3485.167099
153. Anderson G, Kerluke K. Distribution of prescription drug exposures in the elderly: description and implications. J Clin Epidemiol (1996) 49(8):929–35. doi: 10.1016/0895-4356(96)00055-8
154. Jörgensen T, Johansson S, Kennerfalk A, Wallander MA, Svärdsudd K. Prescription drug use, diagnoses, and healthcare utilization among the elderly. Ann Pharmacother (2001) 35(9):1004–9. doi: 10.1345/aph.10351
155. Kennerfalk A, Ruigómez A, Wallander MA, Wilhelmsen L, Johansson S. Geriatric drug therapy and healthcare utilization in the united kingdom. Ann Pharmacother (2002) 36(5):797–803. doi: 10.1345/aph.1A226
156. Maher RL, Hanlon J, Hajjar ER. Clinical consequences of polypharmacy in elderly. Expert Opin Drug Saf (2014) 13(1):57–65. doi: 10.1517/14740338.2013.827660
157. McLean AJ, Le Couteur DG. Aging biology and geriatric clinical pharmacology. Pharmacol Rev (2004) 56(2):163–84. doi: 10.1124/pr.56.2.4
158. Bordet R, Gautier S, Le Louet H, Dupuis B, Caron J. Analysis of the direct cost of adverse drug reactions in hospitalised patients. Eur J Clin Pharmacol (2001) 56(12):935–41. doi: 10.1007/s002280000260
159. Routledge PA, O’Mahony MS, Woodhouse KW. Adverse drug reactions in elderly patients. Br J Clin Pharmacol (2004) 57(2):121–6. doi: 10.1046/j.1365-2125.2003.01875.x
160. Roughead EE, Gilbert AL, Primrose JG, Sansom LN. Drug-related hospital admissions: a review of Australian studies published 1988-1996. Med J Aust (1998) 168(8):405–8. doi: 10.5694/j.1326-5377.1998.tb138996.x
161. Ebbesen J, Buajordet I, Erikssen J, Brørs O, Hilberg T, Svaar H, et al. Drug-related deaths in a department of internal medicine. Arch Intern Med (2001) 161(19):2317–23. doi: 10.1001/archinte.161.19.2317
162. Schmucker DL. Liver function and phase I drug metabolism in the elderly: a paradox. Drugs Aging (2001) 18(11):837–51. doi: 10.2165/00002512-200118110-00005
163. Thürmann PA, Hompesch BC. Influence of gender on the pharmacokinetics and pharmacodynamics of drugs. Int J Clin Pharmacol Ther (1998) 36(11):586–90.
164. Tanaka E. Gender-related differences in pharmacokinetics and their clinical significance. J Clin Pharm Ther (1999) 24(5):339–46. doi: 10.1046/j.1365-2710.1999.00246.x
Keywords: drug metabolism, cytochrome, CYP3A4, CYP2D6, age, stress ageing and CYP-dependent drug metabolism
Citation: Konstandi M and Johnson EO (2023) Age-related modifications in CYP-dependent drug metabolism: role of stress. Front. Endocrinol. 14:1143835. doi: 10.3389/fendo.2023.1143835
Received: 13 January 2023; Accepted: 10 April 2023;
Published: 24 May 2023.
Edited by:
Nina Mohorko, University of Primorska, SloveniaReviewed by:
Kristin L. Gosselink, Burrell College of Osteopathic Medicine, United StatesMojca Kržan, University of Ljubljana, Slovenia
Copyright © 2023 Konstandi and Johnson. This is an open-access article distributed under the terms of the Creative Commons Attribution License (CC BY). The use, distribution or reproduction in other forums is permitted, provided the original author(s) and the copyright owner(s) are credited and that the original publication in this journal is cited, in accordance with accepted academic practice. No use, distribution or reproduction is permitted which does not comply with these terms.
*Correspondence: Maria Konstandi, bWtvbnN0YW5AdW9pLmdy