- 1Department of Nephrology, The Second Xiangya Hospital of Central South University, Changsha, China
- 2Hunan Key Laboratory of Kidney Disease and Blood Purification, Changsha, Hunan, China
Metabolic syndrome is a complex metabolic disorder, its main clinical manifestations are obesity, hyperglycemia, hypertension and hyperlipidemia. Although metabolic syndrome has been the focus of research in recent decades, it has been proposed that the occurrence and development of metabolic syndrome is related to pathophysiological processes such as insulin resistance, adipose tissue dysfunction and chronic inflammation, but there is still a lack of favorable clinical prevention and treatment measures for metabolic syndrome. Multiple studies have shown that myostatin (MSTN), a member of the TGF-β family, is involved in the development and development of obesity, hyperlipidemia, diabetes, and hypertension (clinical manifestations of metabolic syndrome), and thus may be a potential therapeutic target for metabolic syndrome. In this review, we describe the transcriptional regulation and receptor binding pathway of MSTN, then introduce the role of MSTN in regulating mitochondrial function and autophagy, review the research progress of MSTN in metabolic syndrome. Finally summarize some MSTN inhibitors under clinical trial and proposed the use of MSTN inhibitor as a potential target for the treatment of metabolic syndrome.
1 Brief review of MSTN
Myostatin (MSTN), a member of TGF-β family, also known as growth differentiation factor 8 (GDF8), is a potent inhibitor of skeletal muscle development (1–3). It was first identified by McPherron et al. in 1997 and it was found MSTN is exclusively expressed in the myotome compartment of developing somites in the early stages of embryogenesis (4). Targeting mutant MSTN resulted in large and extensive increases in skeletal muscle mass in mice, with the muscle mass of mutant animals being 2-3 times heavier than that of wild-type animals (4). This suggests that MSTN is a negative regulator of skeletal muscle growth.
Early studies suggested that it is mainly expressed in skeletal muscle. However, with the deepening of research on it, it is also expressed in tissues other than skeletal muscle, such as adipose tissue (5), kidney (6) and heart (7). Before being secreted, its precursor is synthesized, which consists of an N-terminal signal sequence, an N-prodomain region, and a biologically active C-terminal domain (8, 9). The precursor needs to be cleaved twice to generate active MSTN. The first is the removal of a 24-amino acid signal peptide by Furin family enzymes (10), and the second is the cleavage by bone morphogenetic protein 1 (BMP-1)/Tolloid matrix metalloproteinases to generate mature myostatin dimers (11). This allows the active myostatin ligand to dissociate from the inhibitory N-terminal propeptide domain, allowing interaction with the receptor (12, 13).
MSTN is mostly present in the circulation as a bound inactive form (14, 15). Two activin type II receptors, ActRIIA and ActRIIB, are receptors that mediate the physiological effects of MSTN. The binding of MSTN to ActRIIA and ActRIIB requires the involvement of activin-like kinase (ALK) 4/5, which subsequently leads to the phosphorylation of the downstream Smad2/3 complex, which in turn recruits Smad4 (16, 17). Meanwhile, phosphorylated Smad2/3 also inhibited AKT activation and promoted the dephosphorylated of FOXO (18). The Smad complex and dephosphorylated FOXO can enter the nucleus and act as transcription factors to regulate the expression of MuRF1 and Atrogin1 muscle atrophy-related proteins. MuRF1 and Atrogin1 could promote ubiquitination of muscle proteins, thereby accelerating their degradation through the proteasome pathway and ultimately inhibiting muscle growth (Figure 1) (19, 20).
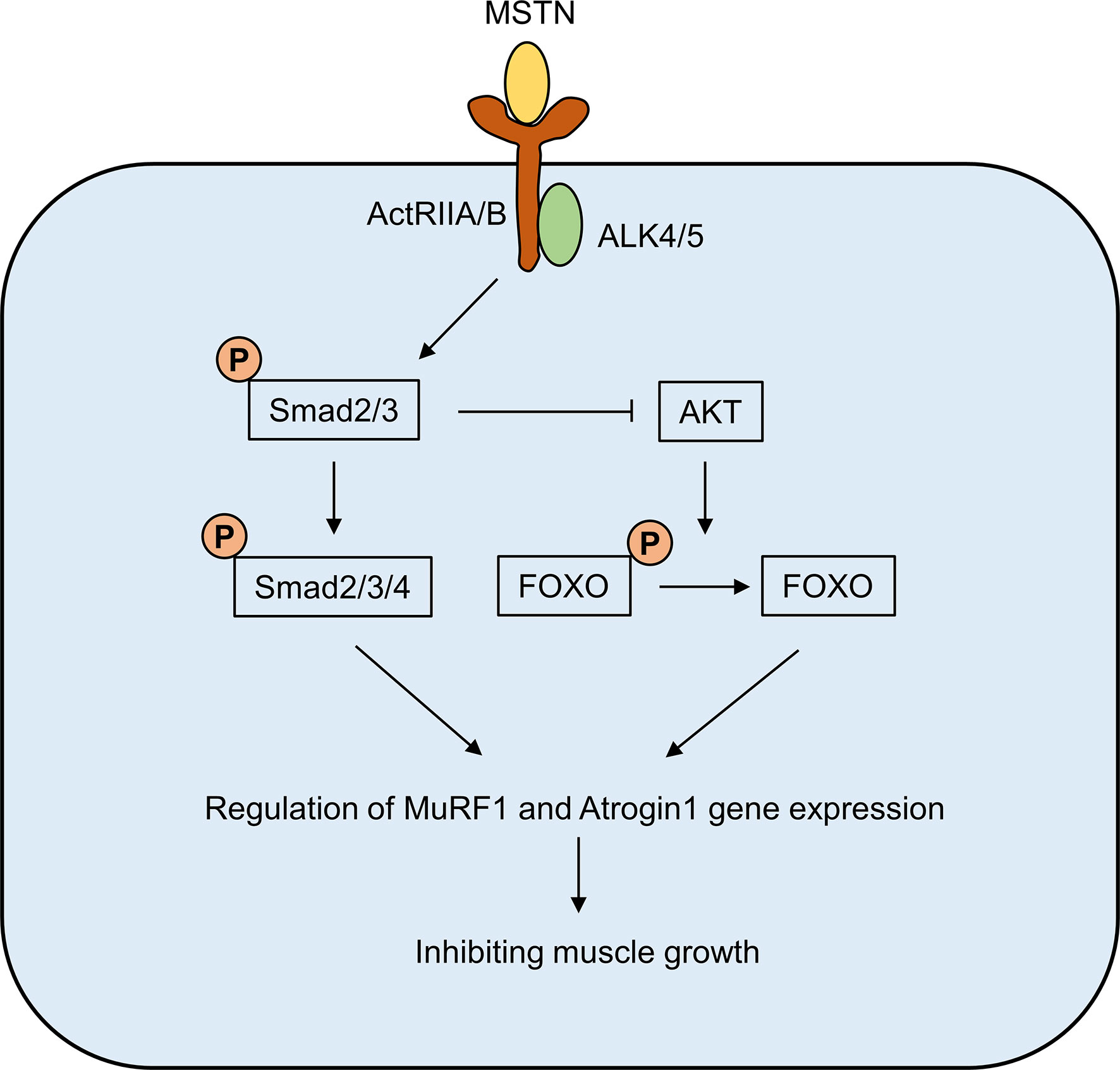
Figure 1 The signaling pathway of MSTN. The binding of MSTN to ActRIIA and ActRIIB requires the involvement of activin-like kinase (ALK) 4/5, which subsequently leads to the phosphorylation of the downstream Smad2/3 complex, which in turn recruits Smad4. Meanwhile, phosphorylated Smad2/3 also inhibited AKT activation and promoted the dephosphorylated of FOXO. The Smad complex and dephosphorylated FOXO can enter the nucleus and act as transcription factors to regulate the expression of MuRF1 and Atrogin1 muscle atrophy-related proteins.
In addition to the classical Smad signaling pathway, MSTN has also been reported to regulate cell growth by activating the c-Jun N-terminal kinase (JNK) signaling pathway. When ActRIIB was knocked out, MSTN-mediated JNK activation was significantly reduced and further intervention with JNK specific inhibitor SP600125 notably inhibited the MSTN-induced p21 up-regulation and differentiation marker gene expression down-regulation (21). In addition, MSTN has also been reported to be involved in the regulation of cell growth possibly through extracellular signal-regulated kinase (ERKs), p38 mitogen-activated protein kinases and Wnt signaling pathway (22–24).
2 The regulation of MSTN expression
Many proteins/compounds are involved in the regulation of MSTN expression. IGF-1 is a growth factor that plays a crucial role in regulating cell proliferation and it has also been reported to be involved in the process of transcriptional regulation of MSTN. Yang et al. have shown that IGF-1 intervention could upregulate myostatin expression through the phosphatidylinositol 3-kinase pathway (25). Further research showed that IGF-1 could activate the Ca2+-dependent nuclear factor of activated T cells (NFAT) transcription factor to bind to the MSTN promoter through the phospholipase C gamma (PLCγ)/inositol 1,4,5-triphosphate (IP3) signaling pathways, thereby regulating the transcription of MSTN (26).
In addition, a CCAAT box and a C/EBP-binding element (CBE) in the MSTN promoter are the two potential C/EBP-binding sequences (27). When treated with glucocorticoids, the activity of C/EBP-δ promoter was notably up-regulated after 1 hour of dexamethasone treatment, while the activity MSTN promoter was not significantly increased until 24 hours or more of intervention (27). Furthermore, by cotransfection with the C/EBP-δ expression vector, the myostatin promoter-reporter construct showed a significant increase in activity. Mutation of the CCAAT box in the MSTN promoter partially blocked the MSTN promoter activity, whereas mutation of the CBE completely abrogated glucocorticoid regulation of the MSTN promoter activity (27). Moreover, FOXO1 has also been reported to be a transcription factor of MSTN, and FOXO1 could increase myostatin mRNA levels and up-regulate myostatin promoter activity, and the mutations in the binding site to FOXO1 in the MSTN promoter significantly reduced the activity of the MSTN promoter (28). Moreover, The NF-κB p65 subunit can also bind to the myostatin promoter to stimulate MSTN gene transcription (29). In order to realize MSTN as a target for disease treatment, its specific agonists or inhibitors need to be identified, which will be described in detail in the following part.
3 MSTN in regulating cell metabolism
3.1 Mitochondria
Mitochondria are energy factories in cells, synthesizing large amounts of ATP to provide energy for the body’s activities (30–32). In the case of metabolic disorder, the morphology, number and function of mitochondria in tissues will change. On the one hand, damaged and abnormal mitochondria can be cleared through a process known as mitophagy, and when damaged mitochondria exceed their own clearance, mitochondrial contents are released into the cell, leading to oxidative stress, inflammation and apoptosis (33–35). Therefore, maintaining the stability of mitochondrial function is an important way to prevent disease progression. Interestingly, studies have also reported that MSTN is closely related to mitochondrial function.
Ploquin et al. have demonstrated that when MSTN was knocked out in mice, the respiratory coupling of mitochondria was reduced in intermyofibrillar, and basal oxygen consumption was significantly increased, lipid peroxidation levels were significantly reduced and the antioxidant glutathione system was notably upregulated (36). Moreover, when mice lack MSTN, the rate of ATP production from oxidative phosphorylation (OXPHOS) is inhibited in skeletal muscle, and the activity of respiratory chain complex is also decreased (37). The molecular mechanism may be that Smad2/3 is insufficiently bound to the promoter region of Idh2 and Idh3a (key rate-limiting enzymes associated with the TCA cycle), thus affecting OXPHOS of the cell (37). Similar result was also observed that MSTN knockout may inhibit mitochondrial function by inhibiting AMPK/SIRT1/PGC1α signaling pathway (38).
Furthermore, MSTN may also regulate mitochondrial function by affecting lipid metabolism. Compared with the control group, the expression levels of lipid membrane transporters (CD36, FABP3, FATP1, and FATP4) and proteins related to lipid oxidation pathways were significantly decreased in the muscle of MSTN-deficient mice. Further analysis of the composition differences of phospholipids and fatty acids in mitochondrial membrane by chromatography showed that the ratio of mitochondrial cardiolipin proportion of MSTN knockout mice was reduced compared with other phospholipids (39). This suggests that MSTN may affect mitochondrial function by regulating lipid composition in mitochondrial membrane. Interestingly, the abnormal cardiolipin content in muscle mitochondrial membrane caused by MSTN deficiency can be corrected by endurance training (40).
3.2 Autophagy
Autophagy is a process of maintaining cellular homeostasis through lysosome degradation of cellular damage or excessive organelles, proteins and other endogenous substances (41–43). Abnormalities in autophagy are closely related to the occurrence and development of a variety of metabolic diseases such as obesity, diabetes and non-alcoholic fatty liver disease (NAFLD) (44–46). Interestingly, MSTN has also been reported to be involved in the regulation of autophagy homeostasis in cells. The decreased of basal autophagy flux and ATP content were observed in muscle of MSTN knockout mice (47), while the treatment of MSTN can increase the expression of autophagy associated protein LC3II and autophagosome formation in skeletal muscle (48) and the increased autophagic flux induced by MSTN was blocked by elevating levels of G protein-coupled receptor kinase 2 (GRK2) (49). Furthermore, Anand et al. have shown that the mRNA expressions of MSTN and BECN1 (autophagy associated proteins) were increased in skeletal muscle of patients with sarcopenia compared with controls (50). These results imply that MSTN inhibits muscle hypertrophy by enhancing autophagy levels in skeletal muscle cells. However, MSTN regulates autophagy differently in cells with myocardial hypertrophy. There is an over-activated autophagy level in myocardial cells during myocardial hypertrophy, while in mice lacking MSTN, abdominal aortic coarctation (AAC) was aggravated and accompanied by an increase in angiotensin II-induced autophagy, which was reversed in vivo and in vitro by MSTN treatment (51). Mechanically, MSTN-mediated anti-autophagy mediated by inhibition of AMPK/mTOR and activation of the PPARγ/NF-κB signaling pathway (51). This suggests that MSTN regulates autophagy in different tissues and diseases in different ways. However, whether MSTN has an effect on autophagy in tissues other than skeletal and cardiac muscles needs to be observed in future studies.
4 MSTN and metabolic syndrome
4.1 MSTN in obesity and hyperlipemia
As a manifestation of metabolic disorder, obesity has been shown to be associated with increased myostatin expression. The mRNA levels of MSTN and its receptor ActRIIb were increased by more than 50-to 100-fold in subcutaneous and visceral fat of ob/ob mice (a mouse model of obesity) compared with wild-type mice (52). Similarly, the concentrations of myostatin were increased in obese nondiabetic subjects compared with lean subjects and were positively correlated with the insulin resistance index and negatively correlated with the insulin sensitivity index (53). Moreover, high-fat feeding could significantly increase the body weight and the expression of MSTN in muscle of high-fat diet induced obesity susceptible mice models, but the expression of MSTN in muscle of high-fat diet induced obesity resistant mice does not change significantly (54). These studies imply that MSTN may play a key role in obesity, and subsequent studies have demonstrated that altering MSTN expression can affect the development of obesity.
There were decreased muscle mass, decreased myocardial mass, and increased epididymal fat mass were observed in MSTN overexpressing mice (55). Similar result was also observed that the depletion of MSTN could reduce the age-related adipose tissue mass increase and partially reduce the obesity and diabetic phenotypes in agouti lethal yellow (Ay) and obese (Lepob/ob) mouse models of obesity and diabetes (56). In addition, Zhang et al. also demonstrated that the absence of MSTN induces reduced fat accumulation in mice fed a high-calorie diet (57). Mechanistically, loss of MSTN mediates fat accumulation suppression through two independent mechanisms. On the one hand, deficiency of MSTN upregulates enzymes involved in lipolysis and mitochondrial fatty acid oxidation (e.g., CPT1a and CPT2), thereby increasing fatty acid oxidation in peripheral tissues and reducing lipid accumulation. On the other hand, its absence also promoted the formation of brown fat in white adipose tissue in mice (white adipose tissue is an energy storage organ, while brown fat is rich in mitochondria and is involved in thermogenesis by consuming fat) (Figure 2) (57). Furthermore, intervention with the MSTN antagonist sActRIIB in wild-type HFD-fed mice significantly reduced obesity in mice (57). Moreover, compared with wild-type HFD-fed mice, HFD-fed male MSTN-deficient mice had reduced plasma cholesterol and triglyceride levels and reduced plasma TNF-α levels by approximately 40 percent, and reduced insulin resistance (58). These results suggest that anti-MSTN therapy may be a potential target for delaying obesity and hyperlipemia. However, there is still disagreement about the role of MSTN in obesity. Zhu et al. have shown that the content of lipids in 3T3-L1 preadipocytes treated with myostatin significantly decreased compared with untreated cells (59). This difference may be due to different cell lines’ different responses to MSTN. However, in future studies, the molecular mechanism of MSTN in obesity needs to be further revealed, which is conducive to the development of anti-obesity drugs targeting MSTN.
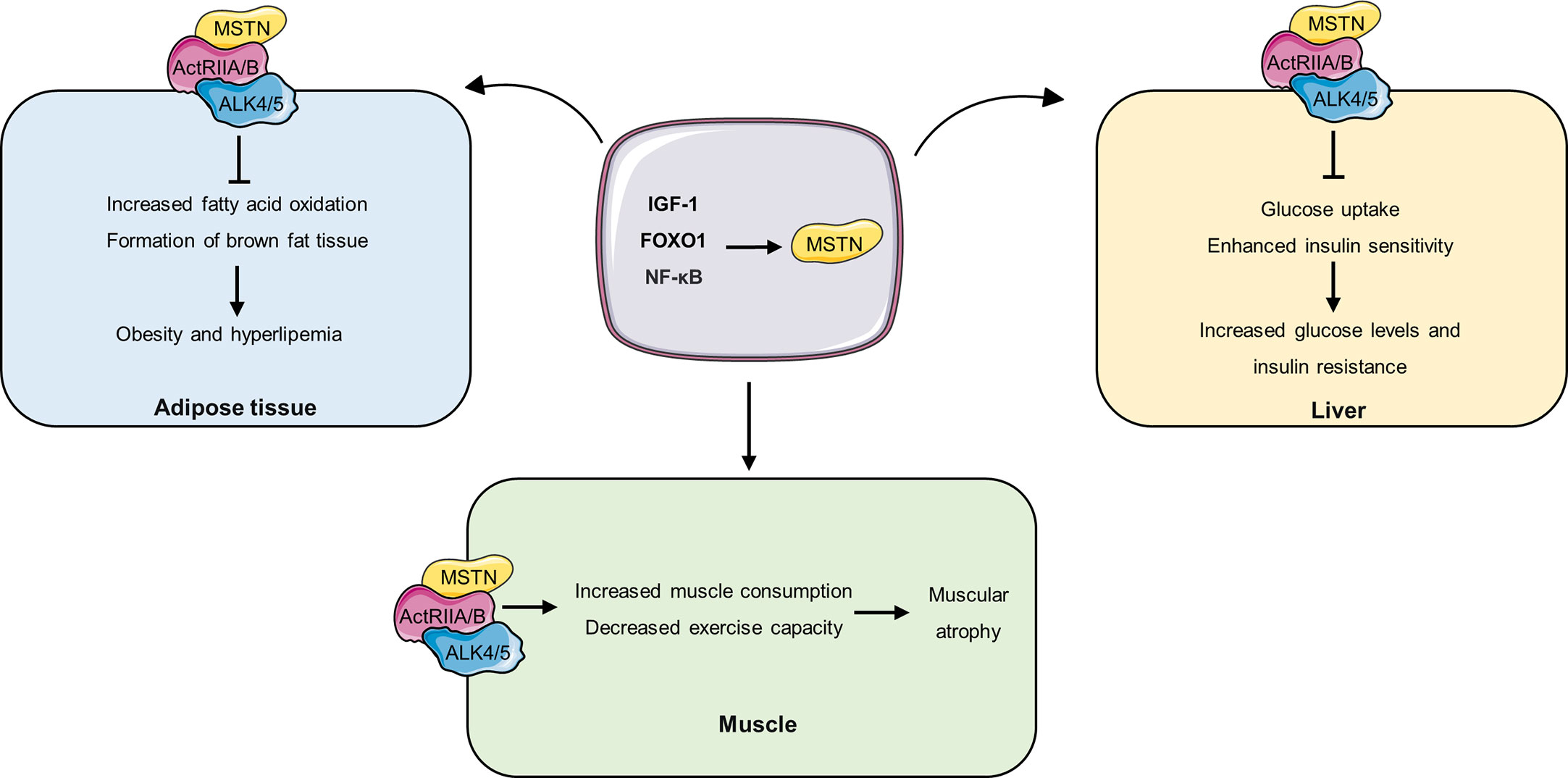
Figure 2 The role of MSTN in different tissues. IGF-1, FOXO1 and NF promote the expression and secretion of MSTN, and MSTN circulates to different tissues and binds to ActRIIA/B receptors on the cell. In adipose tissue, MSTN inhibits fatty acid oxidation and brown adipose tissue formation, thereby promoting obesity and hyperlipidemia. In the liver, MSTN inhibits hepatic glucose uptake and decreases insulin sensitivity, thereby increasing blood glucose levels and insulin resistance. In muscle, MSTN accelerates muscle consumption and decreased exercise capacity, resulting in muscle atrophy.
4.2 MSTN in diabetes
The relationship between diabetes mellitus and MSTN has also been partially revealed. The concentration of MSTN is closely related to the occurrence and development of diabetes or insulin resistance. Dial et al. shown that serum myostatin levels were significantly higher in type 1 diabetes (T1D) patients than in the control group and were higher in T1D women than in T1D men (60). Similar results have been observed in type 2 diabetes (T2D) that the mRNA levels of MSTN were higher in the muscles of patients with T2D than in the control group and MSTN mRNA was correlated with homeostasis model assessment of insulin resistance (HOMA2-IR) and plasma IL-6 level (61). Similar results have been observed that compared with the control group, mRNA expression of MSTN was increased in muscle and subcutaneous adipose tissue of diabetic rats, and mRNA expression of MSTN receptor (ActRIIB) was increased in brown adipose tissue (62). Moreover, Hittel et al. found a strong correlation between plasma MSTN levels and insulin sensitivity, and that injection of myostatin induced insulin resistance in mice (63).
Interestingly, inhibiting the level of MSTN could relieve insulin resistance and diabetes. The elderly men with T2D had significantly higher circulating MSTN concentration and lower muscle strength, while RT training can significantly reduce circulating MSTN levels and increase muscle strength (64). The increased MSTN expression was detected in in skeletal muscle of type 2 diabetic KKAy mice or palmitate treated C2C12 cells, while astragalus polysaccharide could downregulated the expression of MSTN and improve insulin sensitivity (65). By crossing lipodystrophy mice (A-ZIP/F1) with mice expressing a dominant negative MSTN receptor (activin receptor type IIB) in muscle, inhibition of MSTN action in A-ZIP/F1 mice notably lower the levels of blood glucose, serum insulin, triglyceride, and triglyceride synthesis rate, and enhanced insulin sensitivity (66). Moreover, by crossing Akita diabetic mice with myostatin knockout mice, the resulting diabetic myostatin knockout mice had upregulated Glut1 and Glut4 proteins and increased glucose uptake capacity, which in turn resulted in significantly down-regulated resting blood glucose levels and significantly reduced associated diabetes symptoms (67). Similarly, the intervention of MSTN significantly reduced basal and insulin-induced phosphorylation of IRS-1 tyrosine (Tyr495), as well as PI3K expression and activation (68). In addition, MSTN also inhibited AMPK activation and down-regulated Glut4 protein expression, which impaired systemic glucose homeostasis (68). Similar result was observed that myostatin significantly inhibits insulin-stimulated glucose uptake and Akt phosphorylation in hepatocytes (69). The changes of Mss51 were most pronounced in the muscle transcription profiles of MSTN-knockout mice or mice treated with a myostatin/activin inhibitor (ActRIIB-Fc). Furthermore, compared with the control group, muscle fibers isolated from Mss51 knockout mice showed a higher rate of oxygen consumption, up-regulated expression of genes related to oxidative phosphorylation and fatty acid β-oxidation in muscle, and increased systemic glucose turnover and glycolysis rates, as well as enhanced systemic insulin sensitivity (70). This suggests that the antidiabetic effect of inhibition of MSTN may be achieved through MSS51 and enhancing mitochondrial oxidation.
In addition to its direct relationship with diabetes, MSTN is also involved in the occurrence and development of diabetes complications. In diabetic muscle atrophy rats, MSTN expression is increased in skeletal muscle and pulsed electromagnetic field intervention can decrease MSTN expression and increase the cross-sectional area of muscle fibers (71). Diabetic nephropathy is also a common microvascular disease in diabetes. In the kidney, MSTN is mainly expressed in the renal tubules and interstitium, and is colocalized with CD45+. In diabetic nephropathy patients, the expression level of MSTN in renal tubules is increased, and MSTN induced the release of ROS and up-regulation of NADPH oxidase in cells through the ERK pathway, thus aggravate the progression of tubule cell fibrosis (72).
4.3 MSTN in hypertension
MSTN have also been reported to be involved in another important clinical manifestation of metabolic syndrome: hypertension. Pucci et al. showed that individual with above-median serum MSTN concentrations had higher brachial diastolic blood pressure and higher carotid - femoral pulse velocity compared to controls (73). Similarly, compared to non-hypertensive healthy donors, the expression of MSTN was notably increased in structural cardiomyopathy patients (74). However, in the spontaneously hypertensive rat model, the expression of myostatin protein decreased in chronically hypertrophic myocardium and was significantly negatively correlated with left ventricular diastolic diameter/body weight ratio and left ventricular systolic diameter, but positively correlated with partial shortening of the middle wall (75). Although these parts reveal an association between MSTN and hypertension, further mechanisms need to be uncovered. In addition, whether the increase in MSTN levels in hypertensive patients is a primary or secondary increase also needs to be determined in the future.
4.4 MSTN-mediated tissue crosstalk in diseases
The expression of MSTN is not limited to skeletal muscle, it is also expressed in myocardium, adipose tissue, brain, kidney and circulating white blood cells (4, 72). Although current studies on MSTN focus on skeletal muscle, adipose tissere-derived MSTN is also essential for maintaining homeostasis. Adipose tissue is not only a storage of energy, but also secretes a series of proteins called adipokines that regulate the function of distal organs through endocrine processes (76). Recently, MSTN has also been identified as an adipokine. Steculorum et al. have shown that myostatin is secreted in brown adipose tissue and reduces local insulin sensitivity in the form of autocrine (77). In addition, MSTN also mediates the crosstalk between adipose tissue and muscle. Kong et al. have showed that loss of the transcription factor IRF4 in mouse brown adipose tissue (BATI4KO) reduced mitochondrial function and athletic ability in muscle (5). Furthermore, RNA-seq analysis showed upregulation of MSTN in adipose tissue of BATI4KO mice compared with control mice, while reducing circulating MSTN levels by neutralizing antibodies or soluble receptors restored exercise ability in BATI4KO mice (5). Moreover, overexpression of IRF4 in mouse brown adipose tissue reduces circulating MSTN levels, thereby increasing muscle athletic ability (5). This evidence reveals the role of MSTN as an adipokine in maintaining muscle homeostasis. However, whether adipose tissue-derived MSTN also affects the function of other organs needs to be further investigated in the future.
5 The inhibitor of MSTN
In view of the importance of MSTN in regulating muscle homeostasis, the inhibitors targeting the MSTN signaling pathway have been developed for clinical use to improve patients with sarcopenia and muscular dystrophy. Currently, a variety of compounds have been found to improve muscle function by inhibiting MSTN. A variety of MSTN-neutralizing antibodies have been used to inhibit MSTN, such as MYO-029 (78), SOD1 (79), AMG745 (80), Regn647 (81), GYM329 (82), which have been observed to improve muscle homeostasis by inhibiting MSTN levels. In addition, the development of drugs targeting the MSTN receptor is also one of the ideas to block the MSTN signaling pathway and several compounds are also being tested in animals or in clinical trials. Dumonceaux et al. shown that there was increased muscle mass in the mice once effectively down-regulated activin receptor IIb mRNA by intramuscular injection of adeno-associated virus (AAV) encoding a specific shRNA (83). Moreover, ACE-031 is a soluble ActRIIB that binds to circulating MSTN to inhibit its action (84). It has been shown to increase muscle mass in the interveners. Multiple studies have demonstrated that ACE-031 interventions increase muscle mass in subjects (85–87). In addition, a variety of natural compounds were observed to inhibit MSTN expression, including Epicatechin (88), Fructus Schisandrae (89), Sulforaphane (90) and Fructus Schisandrae (91). Here we summarize some inhibitors and part agonists of MSTN to use MSTN as a therapeutic target for diseases (Table 1).
6 Conclusion and perspective
Although MSTN is well known for its role as a key protein that regulates muscle homeostasis and prevents excessive muscle growth, its role in regulating metabolic processes, especially in metabolic syndrome, is also attracting increasing attention. It is involved in the development of obesity, diabetes, lipid disorders and hypertension, while gene knockout or drug inhibition of MSTN can effectively reduce the symptoms of metabolic syndrome. However, the benefits of MSTN therapy in metabolic syndrome still need to be validated in clinical patients. What needs to be determined is whether the circulating MSTN mainly comes from muscle, adipose tissue or other tissues. In addition, the baseline level of MSTN in the cycle at rest needs to be determined. Furthermore, the molecular mechanism of MSTN therapy against metabolic syndrome also needs to be further explored in the future. Although there are still many questions to be addressed, anti-MSTN therapy could be a potential target for metabolic syndrome.
Author contributions
MY the first draft of the manuscript. CBL, NJ, YL, HZ, CRL,YH, WC, LL, and LX, provided consultations on the preparation of the work. SL contributed to manuscript revision, read, and approved the submitted version.
Funding
This work was supported by the Natural Science Foundation of Hunan Province (2021JC0003).
Conflict of interest
The authors declare that the research was conducted in the absence of any commercial or financial relationships that could be construed as a potential conflict of interest.
Publisher’s note
All claims expressed in this article are solely those of the authors and do not necessarily represent those of their affiliated organizations, or those of the publisher, the editors and the reviewers. Any product that may be evaluated in this article, or claim that may be made by its manufacturer, is not guaranteed or endorsed by the publisher.
References
1. Meloux A, Rochette L, Maza M, Bichat F, Tribouillard L, Cottin Y, et al. Growth differentiation factor-8 (GDF8)/Myostatin is a predictor of troponin i peak and a marker of clinical severity after acute myocardial infarction. J Clin Med (2019) 9:116. doi: 10.3390/jcm9010116
2. Tsuchida K. Activins, myostatin and related TGF-beta family members as novel therapeutic targets for endocrine, metabolic and immune disorders. Curr Drug Targets Immune Endocr Metabol Disord (2004) 4:157–66. doi: 10.2174/1568008043339901
3. Lee SJ. Myostatin: a skeletal muscle chalone. Annu Rev Physiol (2023) 85:269–91. doi: 10.1146/annurev-physiol-012422-112116
4. McPherron AC, Lawler AM, Lee SJ. Regulation of skeletal muscle mass in mice by a new TGF-beta superfamily member. NATURE (1997) 387:83–90. doi: 10.1038/387083a0
5. Kong X, Yao T, Zhou P, Kazak L, Tenen D, Lyubetskaya A, et al. Brown adipose tissue controls skeletal muscle function via the secretion of myostatin. Cell Metab (2018) 28:631–43. doi: 10.1016/j.cmet.2018.07.004
6. Verzola D, Barisione C, Picciotto D, Garibotto G, Koppe L. Emerging role of myostatin and its inhibition in the setting of chronic kidney disease. Kidney Int (2019) 95:506–17. doi: 10.1016/j.kint.2018.10.010
7. Breitbart A, Auger-Messier M, Molkentin JD, Heineke J. Myostatin from the heart: local and systemic actions in cardiac failure and muscle wasting. Am J Physiol Heart Circ Physiol (2011) 300:H1973–82. doi: 10.1152/ajpheart.00200.2011
8. Day JW, Howell K, Place A, Long K, Rossello J, Kertesz N, et al. Advances and limitations for the treatment of spinal muscular atrophy. BMC Pediatr (2022) 22:632. doi: 10.1186/s12887-022-03671-x
9. Sharma M, McFarlane C, Kambadur R, Kukreti H, Bonala S, Srinivasan S. Myostatin: expanding horizons. IUBMB Life (2015) 67:589–600. doi: 10.1002/iub.1392
10. Lee SJ. Regulation of muscle mass by myostatin. Annu Rev Cell Dev Biol (2004) 20:61–86. doi: 10.1146/annurev.cellbio.20.012103.135836
11. Ge G, Greenspan DS. Developmental roles of the BMP1/TLD metalloproteinases. Birth Defects Res C Embryo Today (2006) 78:47–68. doi: 10.1002/bdrc.20060
12. Lee SJ, McPherron AC. Regulation of myostatin activity and muscle growth. Proc Natl Acad Sci USA (2001) 98:9306–11. doi: 10.1073/pnas.151270098
13. Thies RS, Chen T, Davies MV, Tomkinson KN, Pearson AA, Shakey QA, et al. GDF-8 propeptide binds to GDF-8 and antagonizes biological activity by inhibiting GDF-8 receptor binding. Growth FACTORS (2001) 18:251–9. doi: 10.3109/08977190109029114
14. Hill JJ, Davies MV, Pearson AA, Wang JH, Hewick RM, Wolfman NM, et al. The myostatin propeptide and the follistatin-related gene are inhibitory binding proteins of myostatin in normal serum. J Biol Chem (2002) 277:40735–41. doi: 10.1074/jbc.M206379200
15. Zimmers TA, Davies MV, Koniaris LG, Haynes P, Esquela AF, Tomkinson KN, et al. Induction of cachexia in mice by systemically administered myostatin. SCIENCE (2002) 296:1486–8. doi: 10.1126/science.1069525
16. Rodgers BD, Ward CW. Myostatin/Activin receptor ligands in muscle and the development status of attenuating drugs. Endocr Rev (2022) 43:329–65. doi: 10.1210/endrev/bnab030
17. Tsuchida K, Nakatani M, Uezumi A, Murakami T, Cui X. Signal transduction pathway through activin receptors as a therapeutic target of musculoskeletal diseases and cancer. Endocr J (2008) 55:11–21. doi: 10.1507/endocrj.KR-110
18. Esposito P, Verzola D, Picciotto D, Cipriani L, Viazzi F, Garibotto G. Myostatin/Activin-a signaling in the vessel wall and vascular calcification. CELLS-BASEL (2021) 10:2070. doi: 10.3390/cells10082070
19. Glass DJ. PI3 kinase regulation of skeletal muscle hypertrophy and atrophy. Curr Top Microbiol Immunol (2010) 346:267–78. doi: 10.1007/82_2010_78
20. Brooks NE, Myburgh KH. Skeletal muscle wasting with disuse atrophy is multi-dimensional: the response and interaction of myonuclei, satellite cells and signaling pathways. Front Physiol (2014) 5:99. doi: 10.3389/fphys.2014.00099
21. Huang Z, Chen D, Zhang K, Yu B, Chen X, Meng J. Regulation of myostatin signaling by c-jun n-terminal kinase in C2C12 cells. Cell Signal (2007) 19:2286–95. doi: 10.1016/j.cellsig.2007.07.002
22. Gui T, Sun Y, Shimokado A, Muragaki Y. The roles of mitogen-activated protein kinase pathways in TGF-beta-Induced epithelial-mesenchymal transition. J Signal Transduct (2012) 2012:289243. doi: 10.1155/2012/289243
23. Steelman CA, Recknor JC, Nettleton D, Reecy JM. Transcriptional profiling of myostatin-knockout mice implicates wnt signaling in postnatal skeletal muscle growth and hypertrophy. FASEB J (2006) 20:580–2. doi: 10.1096/fj.05-5125fje
24. Chen MM, Zhao YP, Zhao Y, Deng SL, Yu K. Regulation of myostatin on the growth and development of skeletal muscle. Front Cell Dev Biol (2021) 9:785712. doi: 10.3389/fcell.2021.785712
25. Yang W, Zhang Y, Li Y, Wu Z, Zhu D. Myostatin induces cyclin D1 degradation to cause cell cycle arrest through a phosphatidylinositol 3-kinase/AKT/GSK-3 beta pathway and is antagonized by insulin-like growth factor 1. J Biol Chem (2007) 282:3799–808. doi: 10.1074/jbc.M610185200
26. Valdes JA, Flores S, Fuentes EN, Osorio-Fuentealba C, Jaimovich E, Molina A. IGF-1 induces IP3 -dependent calcium signal involved in the regulation of myostatin gene expression mediated by NFAT during myoblast differentiation. J Cell Physiol (2013) 228:1452–63. doi: 10.1002/jcp.24298
27. Allen DL, Cleary AS, Hanson AM, Lindsay SF, Reed JM. CCAAT/enhancer binding protein-delta expression is increased in fast skeletal muscle by food deprivation and regulates myostatin transcription in vitro. Am J Physiol Regul Integr Comp Physiol (2010) 299:R1592–601. doi: 10.1152/ajpregu.00247.2010
28. Allen DL, Unterman TG. Regulation of myostatin expression and myoblast differentiation by FoxO and SMAD transcription factors. Am J Physiol Cell Physiol (2007) 292:C188–99. doi: 10.1152/ajpcell.00542.2005
29. Qiu J, Thapaliya S, Runkana A, Yang Y, Tsien C, Mohan ML, et al. Hyperammonemia in cirrhosis induces transcriptional regulation of myostatin by an NF-kappaB-mediated mechanism. Proc Natl Acad Sci USA (2013) 110:18162–7. doi: 10.1073/pnas.1317049110
30. Nunnari J, Suomalainen A. Mitochondria: in sickness and in health. CELL (2012) 148:1145–59. doi: 10.1016/j.cell.2012.02.035
31. Roger AJ, Munoz-Gomez SA, Kamikawa R. The origin and diversification of mitochondria. Curr Biol (2017) 27:R1177–92. doi: 10.1016/j.cub.2017.09.015
32. Burke PJ. Mitochondria, bioenergetics and apoptosis in cancer. Trends Cancer (2017) 3:857–70. doi: 10.1016/j.trecan.2017.10.006
33. Bhatti JS, Bhatti GK, Reddy PH. Mitochondrial dysfunction and oxidative stress in metabolic disorders - a step towards mitochondria based therapeutic strategies. Biochim Biophys Acta Mol Basis Dis (2017) 1863:1066–77. doi: 10.1016/j.bbadis.2016.11.010
34. Green DR, Galluzzi L, Kroemer G. Mitochondria and the autophagy-inflammation-cell death axis in organismal aging. SCIENCE (2011) 333:1109–12. doi: 10.1126/science.1201940
35. Abate M, Festa A, Falco M, Lombardi A, Luce A, Grimaldi A, et al. Mitochondria as playmakers of apoptosis, autophagy and senescence. Semin Cell Dev Biol (2020) 98:139–53. doi: 10.1016/j.semcdb.2019.05.022
36. Ploquin C, Chabi B, Fouret G, Vernus B, Feillet-Coudray C, Coudray C, et al. Lack of myostatin alters intermyofibrillar mitochondria activity, unbalances redox status, and impairs tolerance to chronic repetitive contractions in muscle. Am J Physiol Endocrinol Metab (2012) 302:E1000–8. doi: 10.1152/ajpendo.00652.2011
37. Wang X, Wei Z, Gu M, Zhu L, Hai C, Di A, et al. Loss of myostatin alters mitochondrial oxidative phosphorylation, TCA cycle activity, and ATP production in skeletal muscle. Int J Mol Sci (2022) 23:15707. doi: 10.3390/ijms232415707
38. Gu M, Wei Z, Wang X, Gao Y, Wang D, Liu X, et al. Myostatin knockout affects mitochondrial function by inhibiting the AMPK/SIRT1/PGC1alpha pathway in skeletal muscle. Int J Mol Sci (2022) 23:13703. doi: 10.3390/ijms232213703
39. Baati N, Feillet-Coudray C, Fouret G, Vernus B, Goustard B, Coudray C, et al. Myostatin deficiency is associated with lipidomic abnormalities in skeletal muscles. Biochim Biophys Acta Mol Cell Biol Lipids (2017) 1862:1044–55. doi: 10.1016/j.bbalip.2017.06.017
40. Baati N, Feillet-Coudray C, Fouret G, Vernus B, Goustard B, Jollet M, et al. New evidence of exercise training benefits in myostatin-deficient mice: effect on lipidomic abnormalities. Biochem Biophys Res Commun (2019) 516:89–95. doi: 10.1016/j.bbrc.2019.06.014
41. Debnath J, Gammoh N, Ryan KM. Autophagy and autophagy-related pathways in cancer. Nat Rev Mol Cell Biol (2023) 2:1–16. doi: 10.1038/s41580-023-00585-z
42. Kwon J, Kim J, Kim KI. Crosstalk between endoplasmic reticulum stress response and autophagy in human diseases. Anim Cells Syst (Seoul) (2023) 27:29–37. doi: 10.1080/19768354.2023.2181217
43. Rahman MA, Rahman MS, Parvez M, Kim B. The emerging role of autophagy as a target of environmental pollutants: an update on mechanisms. Toxics (2023) 11:135. doi: 10.3390/toxics11020135
44. Tao T, Xu H. Autophagy and obesity and diabetes. Adv Exp Med Biol (2020) 1207:445–61. doi: 10.1007/978-981-15-4272-5_32
45. Sarparanta J, Garcia-Macia M, Singh R. Autophagy and mitochondria in obesity and type 2 diabetes. Curr Diabetes Rev (2017) 13:352–69. doi: 10.2174/1573399812666160217122530
46. Wu W, Zhang L, Chan M. Autophagy, NAFLD and NAFLD-related HCC. Adv Exp Med Biol (2018) 1061:127–38. doi: 10.1007/978-981-10-8684-7_10
47. Nassar R, Vernus B, Carnac G, Fouret G, Goustard B, Casas F, et al. Myostatin gene inactivation increases post-mortem calpain-dependent muscle proteolysis in mice. MEAT Sci (2022) 185:108726. doi: 10.1016/j.meatsci.2021.108726
48. Lee JY, Hopkinson NS, Kemp PR. Myostatin induces autophagy in skeletal muscle in vitro. Biochem Biophys Res Commun (2011) 415:632–6. doi: 10.1016/j.bbrc.2011.10.124
49. Manfredi LH, Ang J, Peker N, Dagda RK, McFarlane C. G Protein-coupled receptor kinase 2 regulates mitochondrial bioenergetics and impairs myostatin-mediated autophagy in muscle cells. Am J Physiol Cell Physiol (2019) 317:C674–86. doi: 10.1152/ajpcell.00516.2018
50. Anand A, Nambirajan A, Kumar V, Agarwal S, Sharma S, Mohta S, et al. Alterations in autophagy and mammalian target of rapamycin (mTOR) pathways mediate sarcopenia in patients with cirrhosis. J Clin Exp Hepatol (2022) 12:510–8. doi: 10.1016/j.jceh.2021.05.004
51. Qi H, Ren J, Ba L, Song C, Zhang Q, Cao Y, et al. MSTN attenuates cardiac hypertrophy through inhibition of excessive cardiac autophagy by blocking AMPK /mTOR and miR-128/PPARgamma/NF-kappaB. Mol Ther Nucleic Acids (2020) 19:507–22. doi: 10.1016/j.omtn.2019.12.003
52. Allen DL, Cleary AS, Speaker KJ, Lindsay SF, Uyenishi J, Reed JM, et al. Myostatin, activin receptor IIb, and follistatin-like-3 gene expression are altered in adipose tissue and skeletal muscle of obese mice. Am J Physiol Endocrinol Metab (2008) 294:E918–27. doi: 10.1152/ajpendo.00798.2007
53. Amor M, Itariu BK, Moreno-Viedma V, Keindl M, Jurets A, Prager G, et al. Serum myostatin is upregulated in obesity and correlates with insulin resistance in humans. Exp Clin Endocrinol Diabetes (2019) 127:550–6. doi: 10.1055/a-0641-5546
54. Lyons JA, Haring JS, Biga PR. Myostatin expression, lymphocyte population, and potential cytokine production correlate with predisposition to high-fat diet induced obesity in mice. PloS One (2010) 5:e12928. doi: 10.1371/journal.pone.0012928
55. Reisz-Porszasz S, Bhasin S, Artaza JN, Shen R, Sinha-Hikim I, Hogue A, et al. Lower skeletal muscle mass in male transgenic mice with muscle-specific overexpression of myostatin. Am J Physiol Endocrinol Metab (2003) 285:E876–88. doi: 10.1152/ajpendo.00107.2003
56. McPherron AC, Lee SJ. Suppression of body fat accumulation in myostatin-deficient mice. J Clin Invest (2002) 109:595–601. doi: 10.1172/JCI0213562
57. Zhang C, McFarlane C, Lokireddy S, Masuda S, Ge X, Gluckman PD, et al. Inhibition of myostatin protects against diet-induced obesity by enhancing fatty acid oxidation and promoting a brown adipose phenotype in mice. DIABETOLOGIA (2012) 55:183–93. doi: 10.1007/s00125-011-2304-4
58. Wilkes JJ, Lloyd DJ, Gekakis N. Loss-of-function mutation in myostatin reduces tumor necrosis factor alpha production and protects liver against obesity-induced insulin resistance. DIABETES (2009) 58:1133–43. doi: 10.2337/db08-0245
59. Zhu HJ, Pan H, Zhang XZ, Li NS, Wang LJ, Yang HB, et al. The effect of myostatin on proliferation and lipid accumulation in 3T3-L1 preadipocytes. J Mol Endocrinol (2015) 54:217–26. doi: 10.1530/JME-15-0038
60. Dial AG, Monaco C, Grafham GK, Romanova N, Simpson JA, Tarnopolsky MA, et al. Muscle and serum myostatin expression in type 1 diabetes. Physiol Rep (2020) 8:e14500. doi: 10.14814/phy2.14500
61. Brandt C, Nielsen AR, Fischer CP, Hansen J, Pedersen BK, Plomgaard P. Plasma and muscle myostatin in relation to type 2 diabetes. PloS One (2012) 7:e37236. doi: 10.1371/journal.pone.0037236
62. Dutra DB, Bueno PG, Silva RN, Nakahara NH, Selistre-Araujo HS, Nonaka KO, et al. Expression of myostatin, myostatin receptors and follistatin in diabetic rats submitted to exercise. Clin Exp Pharmacol Physiol (2012) 39:417–22. doi: 10.1111/j.1440-1681.2012.05690.x
63. Hittel DS, Axelson M, Sarna N, Shearer J, Huffman KM, Kraus WE. Myostatin decreases with aerobic exercise and associates with insulin resistance. Med Sci Sports Exerc (2010) 42:2023–9. doi: 10.1249/MSS.0b013e3181e0b9a8
64. Shabkhiz F, Khalafi M, Rosenkranz S, Karimi P, Moghadami K. Resistance training attenuates circulating FGF-21 and myostatin and improves insulin resistance in elderly men with and without type 2 diabetes mellitus: a randomised controlled clinical trial. Eur J SPORT Sci (2021) 21:636–45. doi: 10.1080/17461391.2020.1762755
65. Liu M, Qin J, Hao Y, Liu M, Luo J, Luo T, et al. Astragalus polysaccharide suppresses skeletal muscle myostatin expression in diabetes: involvement of ROS-ERK and NF-kappaB pathways. Oxid Med Cell LONGEV (2013) 2013:782497. doi: 10.1155/2013/782497
66. Guo T, Bond ND, Jou W, Gavrilova O, Portas J, McPherron AC. Myostatin inhibition prevents diabetes and hyperphagia in a mouse model of lipodystrophy. DIABETES (2012) 61:2414–23. doi: 10.2337/db11-0915
67. Coleman SK, Rebalka IA, D'Souza DM, Deodhare N, Desjardins EM, Hawke TJ. Myostatin inhibition therapy for insulin-deficient type 1 diabetes. Sci Rep (2016) 6:32495. doi: 10.1038/srep32495
68. Liu XH, Bauman WA, Cardozo CP. Myostatin inhibits glucose uptake via suppression of insulin-dependent and -independent signaling pathways in myoblasts. Physiol Rep (2018) 6:e13837. doi: 10.14814/phy2.13837
69. Watts R, Ghozlan M, Hughey CC, Johnsen VL, Shearer J, Hittel DS. Myostatin inhibits proliferation and insulin-stimulated glucose uptake in mouse liver cells. Biochem Cell Biol (2014) 92:226–34. doi: 10.1139/bcb-2014-0004
70. Rovira GY, Moyer AL, LeTexier NJ, Bratti AD, Feng S, Sun C, et al. Mss51 deletion enhances muscle metabolism and glucose homeostasis in mice. JCI Insight (2019) 4:e122247. doi: 10.1172/jci.insight.122247
71. Yang J, Sun L, Fan X, Yin B, Kang Y, An S, et al. Pulsed electromagnetic fields alleviate streptozotocin−induced diabetic muscle atrophy. Mol Med Rep (2018) 18:1127–33. doi: 10.3892/mmr.2018.9067
72. Verzola D, Milanesi S, Viazzi F, Ansaldo F, Saio M, Garibaldi S, et al. Enhanced myostatin expression and signalling promote tubulointerstitial inflammation in diabetic nephropathy. Sci Rep (2020) 10:6343. doi: 10.1038/s41598-020-62875-2
73. Pucci G, Ministrini S, Nulli ME, Nunziangeli L, Battista F, D'Abbondanza M, et al. Relationship between serum myostatin levels and carotid-femoral pulse wave velocity in healthy young male adolescents: the MACISTE study. J Appl Physiol (1985) (2021) 130:987–92. doi: 10.1152/japplphysiol.00782.2020
74. Fernandez-Sola J, Borrisser-Pairo F, Antunez E, Tobias E. Myostatin and insulin-like growth factor-1 in hypertensive heart disease: a prospective study in human heart donors. J Hypertens (2015) 33:851–858, 859. doi: 10.1097/HJH.0000000000000493
75. Damatto RL, Lima AR, Martinez PF, Cezar MD, Okoshi K, Okoshi MP. Myocardial myostatin in spontaneously hypertensive rats with heart failure. Int J Cardiol (2016) 215:384–7. doi: 10.1016/j.ijcard.2016.04.101
76. Yang M, Song P, Zhao L, Wang X. Adipose-renal axis in diabetic nephropathy. Curr Med Chem (2023) 30:1860–74. doi: 10.2174/0929867329666220806115518
77. Steculorum SM, Ruud J, Karakasilioti I, Backes H, Engstrom RL, Timper K, et al. AgRP neurons control systemic insulin sensitivity via myostatin expression in brown adipose tissue. CELL (2016) 165:125–38. doi: 10.1016/j.cell.2016.02.044
78. Wagner KR, Fleckenstein JL, Amato AA, Barohn RJ, Bushby K, Escolar DM, et al. A phase I/IItrial of MYO-029 in adult subjects with muscular dystrophy. Ann Neurol (2008) 63:561–71. doi: 10.1002/ana.21338
79. Holzbaur EL, Howland DS, Weber N, Wallace K, She Y, Kwak S, et al. Myostatin inhibition slows muscle atrophy in rodent models of amyotrophic lateral sclerosis. Neurobiol Dis (2006) 23:697–707. doi: 10.1016/j.nbd.2006.05.009
80. Padhi D, Higano CS, Shore ND, Sieber P, Rasmussen E, Smith MR. Pharmacological inhibition of myostatin and changes in lean body mass and lower extremity muscle size in patients receiving androgen deprivation therapy for prostate cancer. J Clin Endocrinol Metab (2014) 99:E1967–75. doi: 10.1210/jc.2014-1271
81. Omosule CL, Joseph D, Weiler B, Gremminger VL, Silvey S, Jeong Y, et al. Combinatorial inhibition of myostatin and activin a improves femoral bone properties in the G610C mouse model of osteogenesis imperfecta. J Bone MINER Res (2022) 37:938–53. doi: 10.1002/jbmr.4529
82. Muramatsu H, Kuramochi T, Katada H, Ueyama A, Ruike Y, Ohmine K, et al. Novel myostatin-specific antibody enhances muscle strength in muscle disease models. Sci Rep (2021) 11:2160. doi: 10.1038/s41598-021-81669-8
83. Dumonceaux J, Marie S, Beley C, Trollet C, Vignaud A, Ferry A, et al. Combination of myostatin pathway interference and dystrophin rescue enhances tetanic and specific force in dystrophic mdx mice. Mol Ther (2010) 18:881–7. doi: 10.1038/mt.2009.322
84. Campbell C, McMillan HJ, Mah JK, Tarnopolsky M, Selby K, McClure T, et al. Myostatin inhibitor ACE-031 treatment of ambulatory boys with duchenne muscular dystrophy: results of a randomized, placebo-controlled clinical trial. Muscle Nerve (2017) 55:458–64. doi: 10.1002/mus.25268
85. Attie KM, Borgstein NG, Yang Y, Condon CH, Wilson DM, Pearsall AE, et al. A single ascending-dose study of muscle regulator ACE-031 in healthy volunteers. Muscle Nerve (2013) 47:416–23. doi: 10.1002/mus.23539
86. Li C, Yue C, Liu ZC, Gong J, Wei XS, Yang H, et al. The relationship between myodural bridges, hyperplasia of the suboccipital musculature, and intracranial pressure. PloS One (2022) 17:e273193. doi: 10.1371/journal.pone.0273193
87. Thevis M, Schanzer W. Emerging drugs affecting skeletal muscle function and mitochondrial biogenesis - potential implications for sports drug testing programs. Rapid Commun Mass Spectrom (2016) 30:635–51. doi: 10.1002/rcm.7470
88. Mafi F, Biglari S, Ghardashi AA, Gaeini AA. Improvement in skeletal muscle strength and plasma levels of follistatin and myostatin induced by an 8-week resistance training and epicatechin supplementation in sarcopenic older adults. J Aging Phys Act (2019) 27:384–91. doi: 10.1123/japa.2017-0389
89. Kim JW, Ku SK, Han MH, Kim KY, Kim SG, Kim GY, et al. The administration of fructus schisandrae attenuates dexamethasone-induced muscle atrophy in mice. Int J Mol Med (2015) 36:29–42. doi: 10.3892/ijmm.2015.2200
90. Fan H, Zhang R, Tesfaye D, Tholen E, Looft C, Holker M, et al. Sulforaphane causes a major epigenetic repression of myostatin in porcine satellite cells. EPIGENETICS-US (2012) 7:1379–90. doi: 10.4161/epi.22609
91. Lee EJ, Shaikh S, Ahmad K, Ahmad SS, Lim JH, Park S, et al. Isolation and characterization of compounds from glycyrrhiza uralensis as therapeutic agents for the muscle disorders. Int J Mol Sci (2021) 22:876. doi: 10.3390/ijms22020876
92. St AM, Johnson M, Bansal PN, Wellen J, Robertson A, Opsahl A, et al. A mouse anti-myostatin antibody increases muscle mass and improves muscle strength and contractility in the mdx mouse model of duchenne muscular dystrophy and its humanized equivalent, domagrozumab (PF-06252616), increases muscle volume in cynomolgus monkeys. SKELET Muscle (2017) 7:25. doi: 10.1186/s13395-017-0141-y
93. Camporez JP, Petersen MC, Abudukadier A, Moreira GV, Jurczak MJ, Friedman G, et al. Anti-myostatin antibody increases muscle mass and strength and improves insulin sensitivity in old mice. Proc Natl Acad Sci USA (2016) 113:2212–7. doi: 10.1073/pnas.1525795113
94. Barrett D, Bilic S, Chyung Y, Cote SM, Iarrobino R, Kacena K, et al. A randomized phase 1 safety, pharmacokinetic and pharmacodynamic study of the novel myostatin inhibitor apitegromab (SRK-015): a potential treatment for spinal muscular atrophy. Adv Ther (2021) 38:3203–22. doi: 10.1007/s12325-021-01757-z
95. Tauer JT, Rauch F. Novel ActRIIB ligand trap increases muscle mass and improves bone geometry in a mouse model of severe osteogenesis imperfecta. BONE (2019) 128:115036. doi: 10.1016/j.bone.2019.115036
96. Tinklenberg J, Meng H, Yang L, Liu F, Hoffmann RG, Dasgupta M, et al. Treatment with ActRIIB-mFc produces myofiber growth and improves lifespan in the acta1 H40Y murine model of nemaline myopathy. Am J Pathol (2016) 186:1568–81. doi: 10.1016/j.ajpath.2016.02.008
97. Nielsen C, Potter RM, Borowy C, Jacinto K, Kumar R, Carlson CG. Postnatal hyperplasic effects of ActRIIB blockade in a severely dystrophic muscle. J Cell Physiol (2017) 232:1774–93. doi: 10.1002/jcp.25694
98. Hill JJ, Qiu Y, Hewick RM, Wolfman NM. Regulation of myostatin in vivo by growth and differentiation factor-associated serum protein-1: a novel protein with protease inhibitor and follistatin domains. Mol Endocrinol (2003) 17:1144–54. doi: 10.1210/me.2002-0366
99. Kumagai H, Coelho AR, Wan J, Mehta HH, Yen K, Huang A, et al. MOTS-c reduces myostatin and muscle atrophy signaling. Am J Physiol Endocrinol Metab (2021) 320:E680–90. doi: 10.1152/ajpendo.00275.2020
100. Sun Z, Xu D, Zhao L, Li X, Li S, Huang X, et al. A new therapeutic effect of fenofibrate in duchenne muscular dystrophy: the promotion of myostatin degradation. Br J Pharmacol (2022) 179:1237–50. doi: 10.1111/bph.15678
101. Qin J, Du R, Yang YQ, Zhang HQ, Li Q, Liu L, et al. Dexamethasone-induced skeletal muscle atrophy was associated with upregulation of myostatin promoter activity. Res Vet Sci (2013) 94:84–9. doi: 10.1016/j.rvsc.2012.07.018
102. Kang MJ, Moon JW, Lee JO, Kim JH, Jung EJ, Kim SJ, et al. Metformin induces muscle atrophy by transcriptional regulation of myostatin via HDAC6 and FoxO3a. J Cachexia Sarcopenia Muscle (2022) 13:605–20. doi: 10.1002/jcsm.12833
103. Fernandez-Sola J, Lluis M, Sacanella E, Estruch R, Antunez E, Urbano-Marquez A. Increased myostatin activity and decreased myocyte proliferation in chronic alcoholic cardiomyopathy. Alcohol Clin Exp Res (2011) 35:1220–9. doi: 10.1111/j.1530-0277.2011.01456.x
104. Zhang L, Li D, Chang C, Sun Y. Myostatin/HIF2alpha-mediated ferroptosis is involved in skeletal muscle dysfunction in chronic obstructive pulmonary disease. Int J Chron Obstruct Pulmon Dis (2022) 17:2383–99. doi: 10.2147/COPD.S377226
105. Wang BW, Chang H, Kuan P, Shyu KG. Angiotensin II activates myostatin expression in cultured rat neonatal cardiomyocytes via p38 MAP kinase and myocyte enhance factor 2 pathway. J Endocrinol (2008) 197:85–93. doi: 10.1677/JOE-07-0596
Keywords: myostatin, metabolic syndrome, obesity, diabetes, lipid
Citation: Yang M, Liu C, Jiang N, Liu Y, Luo S, Li C, Zhao H, Han Y, Chen W, Li L, Xiao L and Sun L (2023) Myostatin: a potential therapeutic target for metabolic syndrome. Front. Endocrinol. 14:1181913. doi: 10.3389/fendo.2023.1181913
Received: 08 March 2023; Accepted: 04 May 2023;
Published: 23 May 2023.
Edited by:
Rosalia Rodriguez-Rodriguez, International University of Catalonia, SpainReviewed by:
Abir Mukherjee, Royal Veterinary College (RVC), United KingdomNarendra Bharathy, Ohayo Valley, United States
Copyright © 2023 Yang, Liu, Jiang, Liu, Luo, Li, Zhao, Han, Chen, Li, Xiao and Sun. This is an open-access article distributed under the terms of the Creative Commons Attribution License (CC BY). The use, distribution or reproduction in other forums is permitted, provided the original author(s) and the copyright owner(s) are credited and that the original publication in this journal is cited, in accordance with accepted academic practice. No use, distribution or reproduction is permitted which does not comply with these terms.
*Correspondence: Lin Sun, sunlin@csu.edu.cn