- 1 Department of Hematology, Erasmus University Medical Center, Rotterdam, Netherlands
- 2 Tytgat Institute for Liver and Intestinal Research, Academic Medical Center of the University of Amsterdam, Amsterdam, Netherlands
- 3 Department of Surgery, Erasmus University Medical Center, Rotterdam, Netherlands
Human RORC+ lymphoid tissue inducer cells are part of a rapidly expanding family of innate lymphoid cells (ILC) that participate in innate and adaptive immune responses as well as in lymphoid tissue (re) modeling. The assessment of a potential role for innate lymphocyte-derived cytokines in human homeostasis and disease is hampered by a poor characterization of RORC+ innate cell subsets and a lack of knowledge on the distribution of these cells in adults. Here we show that functionally distinct subsets of human RORC+ innate lymphoid cells are enriched for secretion of IL-17a or IL-22. Both subsets have an activated phenotype and can be distinguished based on the presence or absence of the natural cytotoxicity receptor NKp44. NKp44+ IL-22 producing cells are present in tonsils while NKp44− IL-17a producing cells are present in fetal developing lymph nodes. Development of human intestinal NKp44+ ILC is a programmed event that is independent of bacterial colonization and these cells colonize the fetal intestine during the first trimester. In the adult intestine, NKp44+ ILC are the main ILC subset producing IL-22. NKp44− ILC remain present throughout adulthood in peripheral non-inflamed lymph nodes as resting, non-cytokine producing cells. However, upon stimulation lymph node ILC can swiftly initiate cytokine transcription suggesting that secondary human lymphoid organs may function as a reservoir for innate lymphoid cells capable of participating in inflammatory responses.
Introduction
There is an increasing awareness that early innate response involve several types of RORC+ and RORC− lymphoid-like cells that combine aspects of innate and adaptive immunity including the production of cytokines traditionally associated with adaptive immune cells. These innate cells are collectively referred to as innate lymphocytes (ILC) and are on the one hand important in early immunity to pathogenic bacteria and helminthes as well as tissue integrity after infection, while these cells on the other hand can be part of pathology during experimental colitis and are specifically expanding in Crohn’s disease patients and allergic rhinosinusitis patients (Satoh-Takayama et al., 2008; Sanos et al., 2009; Buonocore et al., 2010; Moro et al., 2010; Neill et al., 2010; Mjosberg et al., 2011; Spits and Di Santo, 2011).
The best studied population of RORC+ ILC are lymphoid tissue inducer cells (LTi cells) that are present in secondary lymphoid organs and whose prime function is to control fetal development of lymph nodes and Peyer’s patches in mouse and man (Cupedo et al., 2009; van de Pavert and Mebius, 2010). Even though lymphoid organogenesis occurs in utero, cells that phenotypically resemble LTi cells have been found in adult mice (Mebius et al., 1997; Kim et al., 2006). Similar to fetal LTi cells, adult cells express molecules involved in LN development such as lymphotoxinα1β2 (LT) and TNFα and depend on expression of Rorγt and Id2 for their development (Yokota et al., 1999; Kurebayashi et al., 2000; Sun et al., 2000; Kim et al., 2006). Although it remains to be determined to what extent adult LTi cells are similar to their fetal counterparts in terms of the ability to induce lymphoid organs, adult LTi cells were shown to have additional functions in the support of adaptive immune responses by facilitating memory T cell generation in the spleen (Kim et al., 2003; Lane et al., 2008). In humans, ILC that resemble the mouse LTi cells are present in developing fetal lymph nodes (Cupedo et al., 2009). In adult mice and humans, RORC+ ILC are found in secondary lymphoid organs, in the skin, and in the intestines. In mice, RORC+ ILC are essential for the first wave of defense against attaching and effacing intestinal pathogens like Citrobacter rodentium (Cella et al., 2008; Satoh-Takayama et al., 2008). IL-22 produced by ILC activates the epithelium via the IL-22 receptor to produce antimicrobial products (Cella et al., 2008; Ouyang et al., 2008; Satoh-Takayama et al., 2008). In humans, several mucosal ILC subsets that produce IL-22 were described. We have previously identified a population of CD56+RORC+ ILC in the tonsil that constitutively produce IL-22 (Cupedo et al., 2009). At the same time, a population of NKp44+RORC+ ILC were described in tonsils, Peyer’s patches, and small intestines and named NK22 (Cella et al., 2008). Clonal analysis of tonsil-derived RORC+ ILC has since shown that CD56+RORC+ ILC and NKp44+ NK22 cells are the same population of ILC (Crellin et al., 2010).
NKp44 belongs to a family of activating receptors found on human NK cells. These receptors are collectively termed natural cytotoxicity receptors (NCRs) and next to NKp44 include NKp30 and NKp46 (Moretta et al., 2001). All three are Ig-like transmembrane receptors that convey activating signals to NK cells. NKp46 and NKp30 are expressed constitutively, while expression of NKp44 is induced upon NK cell activation. NKp46 is also expressed on mouse NK cells, as well as on mouse ILC in the intestines. NKp30 and NKp44 are not expressed in Mus musculus (Moretta et al., 2001; Hollyoake et al., 2005).
Besides production of IL-22, RORC+ ILC in mice and man have been reported to produce IL-17a. In man, RORC+ ILC in fetal lymph nodes (Cupedo et al., 2009) and inflamed intestines (Geremia et al., 2011) contain IL-17a transcripts and in mice, stimulated splenic RORC+ ILC and RORC+ ILC from the small intestines were shown to produce IL-17a (Takatori et al., 2009; Sawa et al., 2011).
In sum, RORC+ ILC from tonsils and fetal lymph nodes differ strikingly in cytokine production: tonsil ILC produce IL-22 while fetal lymph node ILC contain only transcripts for IL-17a (Cupedo et al., 2009). Whether in vivo IL-17 and IL-22 are produced by the same population of cells or by different ILC populations is still subject to debate (Sawa et al., 2010; Vonarbourg et al., 2010). Clarifying the cellular sources of these functionally distinct cytokines will increase our understanding of ILC biology and activation. In this report we determined the cellular source of ILC-derived IL-17a and IL-22 and show that these cytokines are preferentially transcribed by distinct subsets of ILC located in peripheral or mucosal lymphoid organs respectively.
Results
Differential NCR Expression on Tonsil- and Fetal LN-Derived ILC
We hypothesized that expression of Natural Cytotoxicity Receptors could be used to distinguish ILC subpopulations biased toward production of either IL-17a or IL-22. To test this we first compared the ex vivo expression of NKp30, NKp44, and NKp46 on freshly isolated human ILC from fetal lymph nodes vs tonsils. The following phenotypic definition was used for ILC: lineage (CD3; CD19; CD14; CD34) negative cells expressing intermediate levels of CD45 and high levels of both CD127 (IL7Rα) and CD117 (c-Kit). Cells within this gate were uniformly RORC positive (Figure 1A). In fetal lymph nodes, the majority of RORC+ ILC lacked expression of NKp46 (Figure 1B). Approximately half of the cells expressed NKp30 and a very small fraction of these NKp30+ ILC co-expressed NKp44 (Figure 1B). In contrast, the majority of tonsil-derived RORC+ ILC expressed NKp44 as well as NKp30. In addition, a substantial fraction of tonsil RORC+ ILC expressed NKp46 (Figure 1B). The biggest differences in NCR expression between fetal lymph node and tonsils were observed for NKp44 and upon enumeration the percentage of NKp44-expressing RORC+ ILC was indeed significantly increased in tonsils compared to fetal lymph nodes (Figure 1C). These data show that the relative distribution of NCR-positive vs NCR-negative ILC is different between tonsils and fetal lymph nodes and follows the pattern described for IL-22 (Cupedo et al., 2009).
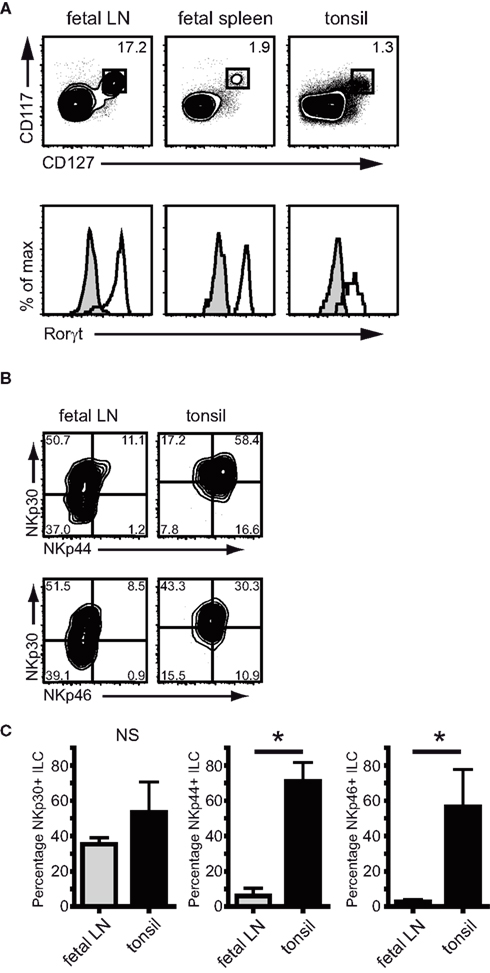
Figure 1. NCR expression on ILC from fetal LN and tonsil. (A) Lineage-negative cells in fetal lymph nodes, fetal spleen, and tonsil labeled for CD117 and CD127 are RORC positive. Shaded histogram indicates isotype control staining (representative example of three independent experiments). (B) Flow cytometric analysis of NKp30 (p = 0.07), NKp44 (p = 0.00), and NKp46 (p = 0.03) expression on ILC and fetal lymph nodes and tonsil. (C) Percentage of NKp30, NKp44, and NKp46 expressing RORC+ ILC in fetal lymph nodes and tonsil (n > 5; average ± SD.)
Next, we assessed the proportion of CD56 expressing ILC in fetal lymph nodes and tonsils. The percentage of CD56+RORC+ ILC was low in fetal lymph nodes, but was increased in tonsils where on average half of the RORC+ ILC expressed CD56 (Figure 2A). This implies that CD56 expression follows a pattern similar but not identical to NKp44, and indeed in tonsils only half of the NKp44+ ILC population expressed CD56 (Figure 2B). In summary, NKp44, and not CD56, is the most prominent cell surface marker differentiating between RORC+ ILC from fetal lymph nodes and adult tonsils.
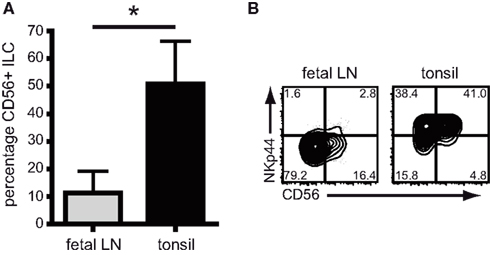
Figure 2. CD56 expression on ILC from fetal LN and tonsil. (A) Percentage of CD56 expressing RORC+ ILC in fetal lymph nodes and tonsil (n > 5; Av ± SD, p = 0.007). (B) Flow cytometric analysis of CD56 and NKp44 expression on RORC+ ILC in fetal lymph nodes and tonsil (representative example of five independent experiments).
NKp44 Expression Allows Identification of IL-17 and IL-22 Biased ILC
On NK cells, NKp44 is only expressed upon activation. In addition, production of IL-17a in T cells is restricted to activated or memory cells (Fossiez et al., 1996). This led us to hypothesize that ILC which contain transcripts for either IL17A or IL22 might also be cells with an activated phenotype. To test this hypothesis, we analyzed the expression of surface proteins known to be regulated during cellular activation on RORC+ ILC cells from fetal lymph nodes and tonsils. The early activation marker CD69 was expressed on part of RORC+ ILC, and the combination of CD69 with NKp44 revealed discrete ILC subpopulations (Figure 3A). In fetal lymph nodes, where the majority of cells lack expression of NKp44, CD69 divided the ILC population into a CD69− and a CD69+ population. In tonsils, NKp44+ ILC were most abundant, and all NKp44+ cells co-expressed CD69. In addition, a small population of CD69+NKp44− cells was present (Figure 3A).
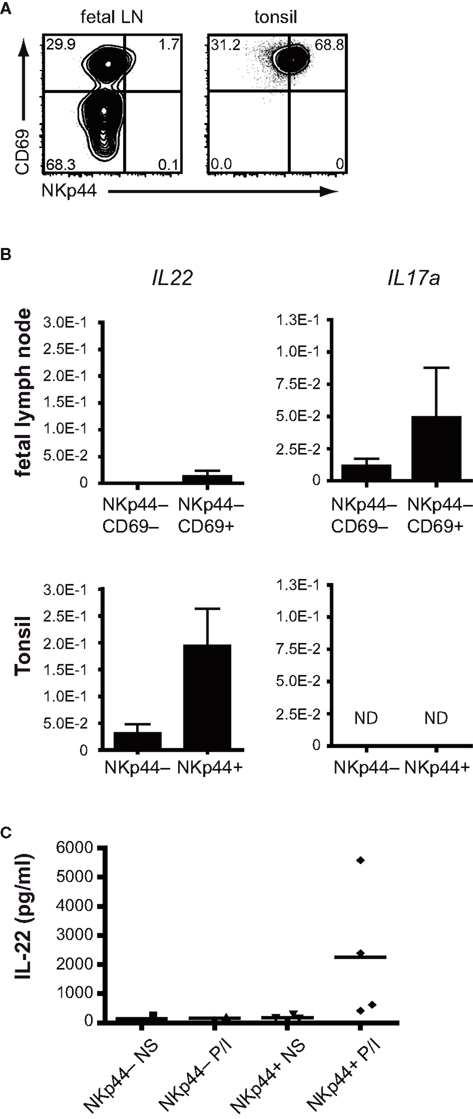
Figure 3. Differential cytokine production by NKp44+ and NKp44− ILC. (A) Flow cytometric analysis of CD69 and NKp44 expression on RORC+ ILC from fetal LN and tonsil (representative example of three independent experiments). (B) Expression of IL22 and IL17A mRNA in sorted ILC populations from tonsils and fetal LN (representative of two individual experiments with three to five donors per experiment). (C) IL-22 protein production by NKp44− and NKp44+ ILC from tonsils stimulated overnight with PMA + ionomycin (n = 3).
We next set out to determine the presence of IL22 and IL17A transcripts in these ILC subpopulations. To this end, the NKp44− ILC were sorted from fetal lymph nodes and separated in CD69− and CD69+ populations. From tonsils, ILC were purified and divided in NKp44− and NKp44+ fractions. Transcripts for IL17A and IL22 were determined without ex vivo stimulation. Within the fetal lymph nodes, IL17A transcripts were enriched within the CD69+NKp44− population (Figure 3B, top right). IL22 transcripts were very low to absent in the fetal lymph nodes (Figure 3B, top left). In tonsil RORC+ ILC, IL22 transcripts were easily detectable within the CD69+NKp44+ population as expected (Cella et al., 2008) but were much lower in the NKp44− population (Figure 3B, bottom left). Transcripts for IL17A were undetectable in tonsil ILC (Figure 3B, bottom right).
In order to assess whether the divergent cytokine patterns observed directly ex vivo are maintained after in vitro stimulation we isolated tonsil NKp44+ and NKp44− ILC and stimulated these overnight with PMA and ionomycin. Similar to freshly isolated ILC, overnight stimulation induces IL-22 secretion from NKp44+ ILC, but not NKp44− ILC (Figure 3C), confirming the cytokine bias seen directly ex vivo. The IL22 induction in NKp44+ ILC by PMA/ionomycin stimulation was observed in all donors analyzed, but did however show a high degree of variability.
These findings imply that tonsil-derived NKp44 positive ILC preferentially transcribe IL-22, while IL-17 is preferentially transcribed by fetal lymph node-derived NKp44+CD69+ ILC, enriched within the ILC that lack expression of NKp44, but do express CD69.
Human Mucosal NKp44+ ILC are Developmentally Programmed
In the mouse, NKp46+ ILC develop in the absence of microbiota, and appear in the intestines after birth (Sanos et al., 2009; Sawa et al., 2010; Reynders et al., 2011). Since fetal human intestines are not yet colonized by bacteria or exposed to ingested antigens (DiGiulio et al., 2008; Mshvildadze et al., 2010; Agarwal et al., 2011), this allowed us to asses the development of human fetal NKp44+ ILC in the absence of external stimuli. We analyzed ILC in first and second trimester fetal small intestines and determined their expression of CD69 and NKp44. First trimester small intestinal-derived ILC (7 weeks gestation) did not express CD69 or NKp44 and resembled putative LTi cells (Figure 4A). CD69+NKp44+ ILC appeared during late first trimester, between weeks 7 and 10 of gestation and with increasing age replaced the LTi-like cells. From week 12 onward, the majority of ILC in the small intestines were expressing both CD69 and NKp44 (Figure 4B). This was specific for the fetal small intestines and was not found in paired mesentery samples containing developing lymph nodes (Figure 4C). Since our analysis of NKp44+ ILC from tonsils had shown IL22 transcription in these cells, we also probed fetal intestine-derived NKp44+ ILC for IL22 transcripts. We consistently found low levels of IL-22 transcripts in NKp44+ ILC from second trimester fetal small intestines (Figure 4D). It was technically impossible to purify sufficient NKp44− ILC from fetal intestines as a comparison. Therefore, CD56+CD127+ NK cells sorted from fetal lymph nodes were used as a negative control. These data show that human NKp44+ ILC develop in utero in the absence of microbial colonization and can be found in the small intestine.
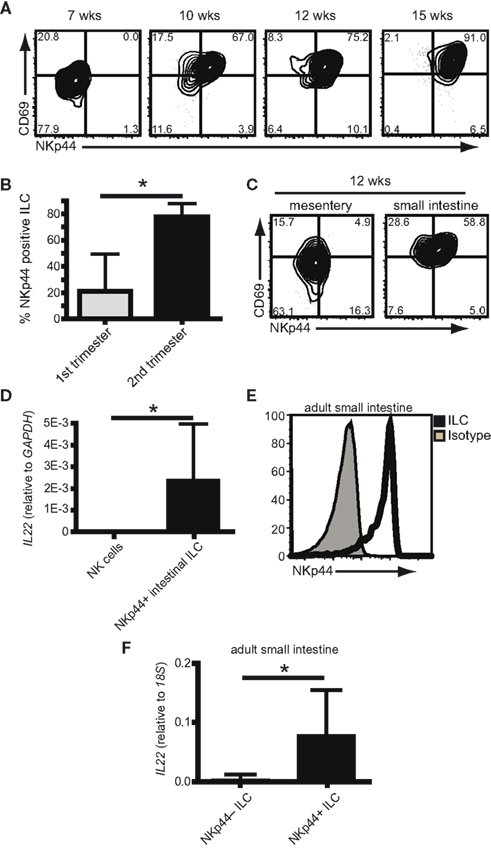
Figure 4. Human NKp44+ ILC are developmentally programmed. (A) Flow cytometric analysis of ILC from fetal intestines of indicated ages (weeks gestation) (representative examples of at least three independent experiments per time point). (B) percentage of ILC expressing NKp44 in the fetal small intestine during first trimester (<12 weeks) or second trimester (>12 weeks) (n > 6; average ± SD; p = 0.003). (C) Flow cytometric analysis of ILC in fetal small intestines and mesentery of the same donor at 12 weeks gestation (representative example of three independent experiments). (D) Expression of IL22 transcripts in NKp44+ ILC from fetal intestine compared to NK cells from fetal lymph node (five independent samples per condition, average ± SD; p = 0.009). (E) Flow cytometric analysis of NKp44 expression on ILC from adult intestine. Filled histogram shows isotype control staining (representative example of five independent experiments). (F) Expression of IL22 transcripts in NKp44+ and NKp44− ILC from adult intestine (n = 7, average ± SD; p = 0.005).
To be able to compare intestinal NKp44+ and NKp44− ILC we next isolated these cells from non-affected adult ileal mucosa acquired during resection procedures for colon cancer. In the adult human intestine, the majority of ILC express NKp44 (Figure 4E). In line with our data from tonsil-derived ILC, intestinal NKp44+ ILC preferentially transcribe IL-22, while NKp44− ILC only contain very low levels of IL-22 trancripts (Figure 4F).
Resting ILC Are Present in Adult Non-Inflamed Human Lymph Nodes
The presence of IL-17 transcribing ILC in fetal human lymph nodes raised the question whether these cells might also be found in adult-lymph nodes. Therefore we determined the presence of RORC+ ILC in adult peripheral non-inflamed lymph nodes. Lymph nodes were collected during multi-organ donation procedures and were liver-draining hepatic lymph nodes unless stated otherwise. Donor age ranged from 24 to 68 years. In adult-lymph nodes a population of Lineage−CD45intCD117+CD127+ ILC was found that uniformly expressed RORC (Figure 5A). Based on expression of NCRs, adult LN-derived RORC+ ILC resembled ILC from fetal lymph nodes rather then from tonsils in that they were mostly negative for NKp46 and NKp44 and only a part of the cells expressed CD56 and NKp30 (Figures 5A,B). There was no difference in the percentage of NKp30 or NKp44-expressing ILC between fetal and adult LN, while slightly more adult ILC expressed NKp46 (Figure 5B). These data show that NKp30 is the only NCR that is consistently expressed on approximately half of all ILC, regardless of whether they were isolated from fetal LN, adult LN or tonsil.
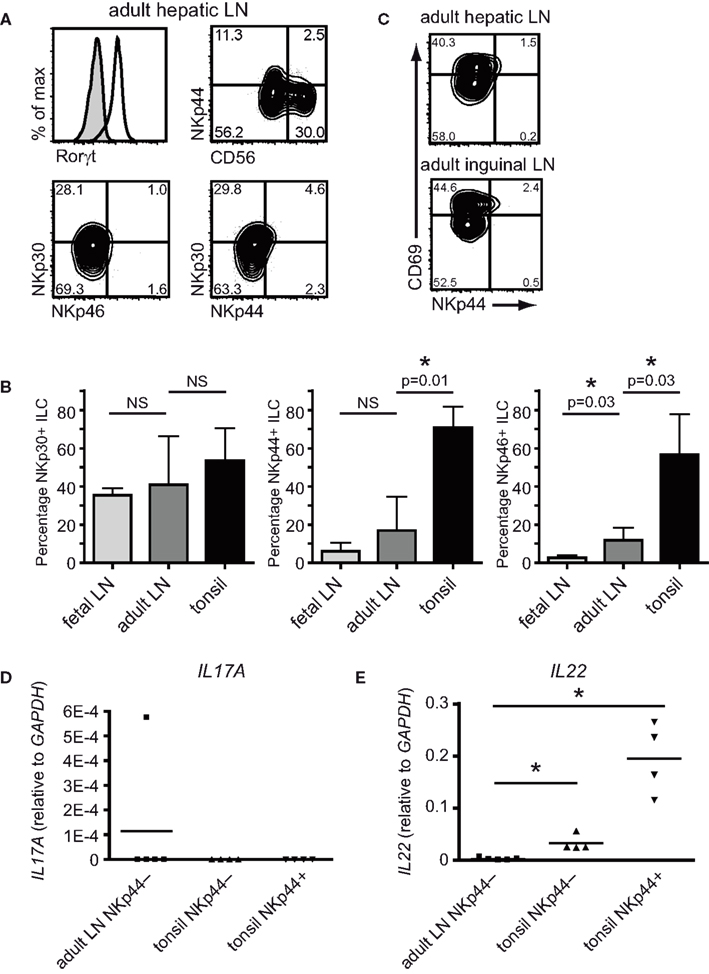
Figure 5. NKp44− ILC in adult non-inflamed lymph nodes. (A) Flow cytometric analysis of ILC from adult hepatic lymph nodes for expression of RORC, NKp44, NKp30, NKp46, and CD56 (representative example of three independent experiments). (B) Flow cytometric analysis of CD69 and NKp44 expression on ILC from adult hepatic and inguinal lymph nodes (representative example of at least three independent experiments). (C) Percentage of NKp30, NKp44 and NKp46 expressing RORC+ ILC in fetal lymph nodes, adult-lymph nodes and tonsil (representative example of at least three independent experiments). (D) PCR analysis for IL17A and (E) IL22 transcripts of sorted ILC from adult hepatic lymph nodes compared to NKp44− and NKp44+ ILC from tonsil (adult LN vs NKp44−p = 0.02; adult LN vs NKp44+p = 0.02; n = 4).
Upon staining for CD69, the presence of CD69+ and CD69− ILC became apparent, very much alike the fetal LN-derived ILC. This phenotype was not restricted to hepatic lymph nodes as a similar distribution of NCR was seen in adult inguinal nodes (Figure 5C). To determine cytokine production by the ILC from adult-lymph nodes, total NKp44− ILC were purified and analyzed for IL22 and IL17A transcripts directly ex vivo. Transcript levels for these cytokines were compared to the levels found in NKp44− and NKp44+ ILC from tonsil. Strikingly, adult-lymph node ILC lacked detectable IL22 or IL17A transcripts (Figures 5D,E). These data suggest that, in contrast to fetal lymph node-derived ILC, adult non-inflamed lymph node-derived ILC are not actively transcribing detectable levels of IL17A in vivo.
To test whether these peripheral ILC were biased toward the production of either IL17A or IL22 upon activation, we stimulated adult-lymph node ILC overnight with PMA and ionomycin (Figure 6). After overnight stimulation we could detect low levels of both IL17A and IL22 transcripts in adult ILC. Induction of IL-17 was specific for adult-lymph node ILC as tonsil-derived ILC did not contain IL17A transcripts after stimulation (Figure 6A). However, the levels of IL17A transcripts were much lower then those found in freshly isolated fetal LN-derived CD69+ ILC. Even more so, the IL22 transcripts found in adult ILC after overnight stimulation were very low in comparison to the levels found in either stimulated or unstimulated tonsil ILC. These findings indicate that throughout adulthood human lymph nodes contain a resting ILC population that is characterized by the absence of NKp44 and the absence of active transcription of IL17A or IL22.
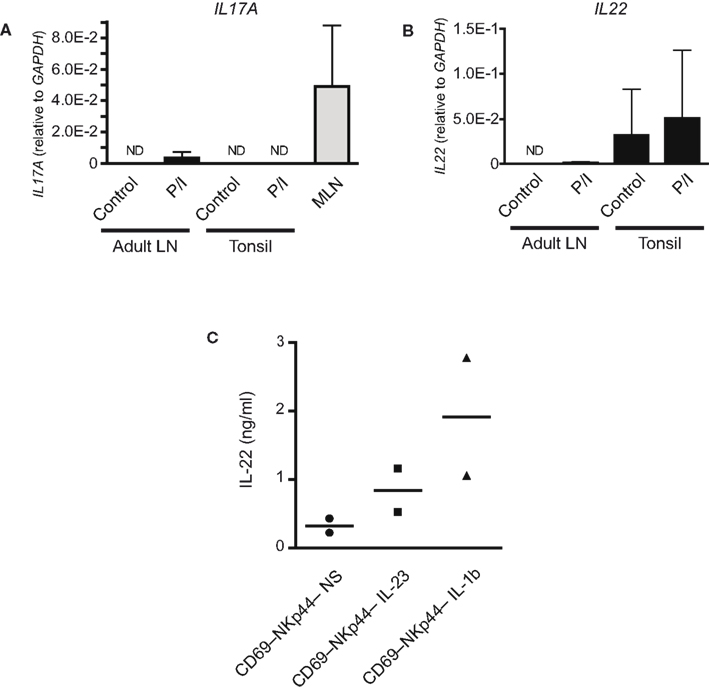
Figure 6. Stimulated adult CD69+NKp44− ILC produce IL-22. (A) IL17A transcripts in NKp44− ILC from adult hepatic lymph nodes and in NKp44+ ILC from tonsils stimulated overnight with PMA + ionomycin as well as in freshly isolated fetal LN-derived CD69+ ILC (n = 3). (B) IL22 transcripts NKp44− ILC from adult hepatic lymph nodes and in NKp44+ ILC from tonsils stimulated overnight with PMA + ionomycin (n = 3). (C) IL-22 protein production by CD69−NKp44− ILC from adult hepatic lymph nodes after 3 days culture with IL-23 or IL-1β (n = 2).
Finally, IL-17a and IL-22 protein levels produced by adult-lymph node-derived NKp44− ILC stimulated with physiological stimuli were determined by ELISA. Unfortunately, the scarcity of adult human tissue precluded the analysis of sufficient donors to draw statistical conclusions. This notwithstanding, in two independent donors 3 days culture in the presence of either IL-23 or IL-1β did induce detectable levels of IL-22 protein (Figure 6C). These culture conditions did not induce IL-17a secretion to levels detectable by our ELISA (detection limit = 31 pg/ml; data not shown). This suggests that even though low level transcription of IL17A can be initiated, no detectable protein is secreted. The reason for this is presently unknown. These data suggest that resting adult-lymph node-derived ILC retain the capacity to secrete IL-22 upon in vitro stimulation with IL-23 or IL-1β. Further work is needed to substantiate these findings.
Discussion
RORC+ ILC are increasingly recognized as important mediators of early innate immunological defenses against mucosal pathogens and as essential for safeguarding tissue integrity during infections. Both effects are mediated by ILC-derived cytokines, and especially IL-22 and IL-17a have been studied in this respect. In mice, IL-22 secreting ILC mediate the innate response to C. rodentium and IL-22 is an important mediator of epithelial homeostasis (Vivier et al., 2009). Conversely, IL-17a producing ILC are pathogenic in a T cell-independent mouse model of intestinal inflammation (Buonocore et al., 2010). Most importantly, the balance between IL-22 and IL-17a secreting ILC was shown to be skewed toward IL-17a in patients with Crohn’s disease, suggesting that ILC-derived cytokines might be involved in human disease (Geremia et al., 2011).
The systematic analysis of ILC subsets in humans and the detection of any changes in subset distribution as a result of disease are hampered by the poor characterization of functionally distinct human ILC. In this study we show that expression of the NCR NKp44 is a good predictor for ILC that are actively transcribing IL-22, and that these cells are mainly found in mucosal tissues. In contrast, our data suggest that throughout adulthood, non-inflamed lymph nodes may function as a reservoir of resting, RORC+ non-cytokine secreting ILC that lack NKp44 expression, yet retain the capacity to secrete IL-22 after appropriate cytokine activation in vitro.
Production of IL-17a by T cells is normally tightly controlled to avoid unwanted or excessive inflammation (Eyerich et al., 2010) and the fact that freshly isolated CD69+NKp44− ILC in non-inflamed lymph nodes lack IL17A transcripts suggests that similar levels of restraint are operational for ILC. Unraveling the exact signals that can either induce or inhibit ILC activation is needed to understand regulation and control of innate IL-17 secretion.
IL-17a has been associated with several human diseases (Ouyang et al., 2008) and ILC-derived IL-17a has been reported in experimental models of colitis (Buonocore et al., 2010). In addition, an increase in IL-17 producing ILC has now also been found in the intestines of some Crohn’s disease patients (Geremia et al., 2011). The IL-17 producing ILC in Crohn’s disease patients were mainly found within the CD56− ILC fraction, while the CD56+ ILC were more prominent producers of IL-22 (Geremia et al., 2011). Our current data would predict that a dissection based on NKp44 expression, rather then expression of CD56, could further enhance the exact enumeration of IL-22 vs IL-17a producing ILC in humans.
The biological function of IL-17a produced by fetal LN-derived ILC remain enigmatic. Development of lymphoid organs has many similarities to a controlled inflammation and the inflammation-related cytokine IL-17a would fit that concept (Nishikawa et al., 2000; Drayton et al., 2006). However, IL-17a is unlikely to have an essential function during organogenesis as IL-17Rα deficient mice display no gross abnormalities in LN development (Kerim Hoorweg and Tom Cupedo, unpublished observations), suggesting that IL-17a serves another yet to be determined function.
In vivo, distinct subsets of RORC+ ILC seem biased toward either IL17A (CD69+NKp44− ILC) or IL22 (CD69+NKp44+ ILC) transcription. It is not clear whether these subsets belong to different ILC lineages, or whether they represent different activation or differentiation states of cells within a single lineage. In mice, conflicting data exists on the relationship between NKp46+ and NKp46− ILC. Genetic approaches indicated that these two cell types belong to separate lineages and do not have a precursor–progeny relationship (Sawa et al., 2010). Conversely, transfer of purified populations of ILC showed that NKp46− cells could give rise to NKp46+ ILC in vivo (Vonarbourg et al., 2010). In humans, these relationships are even less clear. After in vitro culture, freshly isolated NKp44− fetal LN-derived ILC acquired NKp30, NKp44, and NKp46 (Cupedo et al., 2009). In line with this, the adult-lymph node-derived ILC also upregulate NKp44 when cultured (Kerim Hoorweg and Tom Cupedo, unpublished). Whether the observed IL-22 production by lymph node ILC is functionally linked to this expression of NKp44 is currently under investigation. Our current results indicate that in ex vivo isolated ILC, NKp44 predicts for IL-22 production whereas in mice NKp46 is a marker for IL-22 producing ILC, again adding to the complexity of comparing data gathered in human and mouse studies.
NKp44+ ILC are preferentially found in mucosal tissues like tonsil and the intestines (this report and Cella et al., 2008). Within this mucosal environment NKp44+ ILC constitutively produce IL-22 that, based on mouse models, acts on intestinal epithelial cells and conditions these cells to cope with the hostile environment to which they are exposed (Satoh-Takayama et al., 2008; Sanos et al., 2009; Vivier et al., 2009).
Here we show that the appearance of ILC in the human intestine is developmentally programmed and NKp44+ ILC are present from early second trimester. In mice NKp46+ ILC is also a programmed event independent of microflora, yet these cells only appear after birth (Sawa et al., 2010; Reynders et al., 2011). The observed differences between mouse and man in this respect might be due to the longer pregnancy in humans. NKp44+ ILC in the developing human intestine initiate low level IL22 transcription during second trimester pregnancy. However, these IL22 levels are much lower then those found in NKp44+ ILC from pediatric tonsils or adult intestines. Current it is unknown whether this difference reflects a role for microbial colonization of the intestines in inducing a postnatal increase in IL-22 levels. This would be different from the observations done in mice, where microbial colonization was not essential for IL-22 induction but actually induces a decrease in intestinal IL-22 postnatal (Sawa et al., 2010, 2011; Reynders et al., 2011). Again, an alternative and perhaps more likely explanation is that due to the longer gestation time in humans compared to mice, the low levels of IL22 transcription found during the second trimester will steadily increase throughout the third trimester, reaching levels comparable to postnatal ILC just before birth. Even thought the intestines are culture sterile, they are by no means devoid of potential immune stimuli such as TLR ligands and cytokines and these could still be a factor in ILC development and activation (Mshvildadze et al., 2010; Agarwal et al., 2011).
NKp44− ILC reside within peripheral lymph nodes throughout life but in the absence of activating signals do not transcribe detectable levels of IL17A or IL22. However, upon stimulation NKp44− ILC can produce at least IL-22, and also initiate low level IL17A transcription. This raises the hypothesis that secondary lymphoid organs are a reservoir for ILC that can participate in inflammatory responses in an antigen independent manner. Detailed analysis of human lymph node biopsies from inflammatory diseases or the study of relevant animal models is needed to determine the contribution of LN-derived ILC to systemic immune responses.
Materials and Methods
Human Tissues
The use of all human tissues was approved by the Medical Ethical Commission of the Erasmus University Medical Center Rotterdam, and use was contingent on informed consent. Fetal tissues were obtained from elective abortions and gestational age was determined by ultrasonic measurement of the diameter of the skull or the femur and ranged from 7 to 22 weeks gestation. Tonsils were obtained from routine pediatric tonsillectomies. Adult hepatic and inguinal lymph nodes were collected post-mortem during multi-organ donation procedures. Adult small intestine, ileum, was collected as residual material after intestinal surgery for colon carcinoma. Healthy ileum was obtained from a clear distance from the tumor. Patients undergoing radiotherapy or chemotherapy were excluded. Informed consent was obtained from all patients, according to the Medical Ethical Commission of the Academic Medical Center, Amsterdam, the Netherlands.
Cell Preparation
Fetal lymph nodes were dissected from the mesentery using dissecting microscopes, and cell suspensions were prepared by digestion with 0.5 mg/ml collagenase type IV (Sigma, St. Louis, MO, USA) in PBS for 30 min at 37°C, while stirring continuously, and subsequently filtered through a 70-μm nylon mesh. Tonsils and adult-lymph nodes were cut into small pieces and cell suspensions were prepared by disrupting the tissue with a GentleMacs (Miltenyi Biotech) in the presence of 0.5 mg/ml collagenase type IV (Sigma, St. Louis, MO, USA). Mononuclear cells were isolated from ficoll gradients. Tonsils were further enriched for ILC by labeling with CD117 microbeads (Miltenyi Biotech) and positive selection using MidiMacs (Miltenyi Biotech).
Adult intestinal ILC were isolated as described (Ruijter et al., 2009) ileum was incubated with HBSS (Gibco) containing DTT (154 μg/ml), 0.1% β-mercaptoethanol, and 5 mM EDTA at 37°C to eliminate mucus and epithelial cells. Thereafter mucosa was cut into small pieces and digested for 30 min at 37°C with Liberase TM (125 μg/ml) and DNaseI (200 μg/ml; Roche). Cell suspensions were filtered through a 70-μm nylon mesh and lamina propria mononuclear cells (LPMC) were isolated by Ficoll-Paque PLUS (GE, Healthcare).
Flow Cytometry
The following antibodies were used: CD117–PercP.Cy5.5, NKp44–AlexaFluor647, NKp46–AlexaFluor647, NKp30–Pe (Biolegend, San Diego, CA, USA); CD127–APC-eFluor780, CD45–PeCy7, RORγt-Pe (eBioscience, San Diego, CA, USA); CD56–Pacific Blue, CD69–Pe, CD19–PeDY590, CD3–PeDY590, CD14–PeDY590, and CD34–PeDY590 (Exbio, Praha, CZ, USA). Flow cytometry was performed on a BD LSRII and cell sorting on a BD ARIA (Becton Dickinson). Analysis were performed using FlowJo software (Tree Star Inc., Stanford, CA, USA).
Cell Culture
Sorted ILC subsets were cultured overnight in the presence of IL-7 (Peprotech) and SCF R&D Systems; both at 10 ng/ml) in the presence or absence of PMA (50 ng/ml; Sigma) and Ionomycin (100 ng/ml; Sigma) or for 3 days with IL-7, SCF, and either IL-23 or IL-1b. Cells were cultured in 200 μl DMEM supplemented with 10% FCS (Hyclone). IL-17a and IL-22 production was measured using the DuoSet ELISA development kit (R&D systems). ELISAs were performed according to the manufacturers’ instructions.
PCR
From fetal samples, adult-lymph nodes, and tonsil, RNA was extracted using the RNA-XS kit (Machery Nagel) followed by reverse-transcription with random hexamer primers. For quantitative PCR, a Neviti Thermal Cycler (Applied Biosystems) and DyNAmo Flash SYBR Green qPCR kit (Finnzymes) were used, with the addition of MgCl2 to a final concentration of 4 mM. All reactions were done in duplicate and are normalized to the expression of GAPDH (glyceraldehyde phosphate dehydrogenase). Relative expression was calculated by the cycling threshold (CT) method as 2−Δt.
Primers used were:
GAPDH (Accession: NG_007073.2) FW GTC GGA GTC AAC GGA TT; Rev AAG CTT CCC GTT CTC AG
IL22 (Accession: NM_020525.4) FW CCC ATC AGC TCC CAC TGC; Rev GGC ACC ACC TCC TGC ATA TA
IL17A (Accession: NM_002190.2) FW GAA GGC AGG AAT CAC AAT C; Rev GCC TCC CAG ATC ACA GA
For cDNA synthesis of the adult ileum samples, the high-capacity cDNA archive kit (Applied Biosystems) was used. PCR was performed using the SYBR Green I master mix (Roche) for LightCycler 480 Instrument II (Roche).
Quantification of expression levels was performed with LinRegPCR software (Ruijter et al., 2009). All reactions were performed in duplicate were normalized using 18S rRNA expression levels and expressed in arbitrary units.
Specific primers were as follows:
18S rRNA FW: AAT CTG GAG CTG GCC TTT CA; Rev CTG GAA GAT CTG CAG CCT TT
Statistical Analysis
Samples were analyzed by Mann–Whitney U tests in SPSS PASW 17.0.2. p-Values <0.05 were considered significant.
Conflict of Interest Statement
The authors declare that the research was conducted in the absence of any commercial or financial relationships that could be construed as a potential conflict of interest.
Acknowledgments
We want to thank the staff of the CASA clinics in Leiden and Rotterdam for collection of fetal tissues and Dr. Jaap Kwekkeboom of the Department of Gastroenterology and Hepatology, Erasmus University Medical Center Rotterdam for coordinating the acquisition of adult-lymph nodes. Drs. Reina Mebius (VU Medical Center Amsterdam), Mark Coles (University of York), Janneke Samsom, and Rogier Reijmers (Erasmus University Medical Center) are thanked for critical reading of the manuscript. Kerim Hoorweg was supported by an Erasmus MC grant from the Erasmus University Medical Center. Tom Cupedo was supported by Innovational Research Incentives Scheme Vidi grant #91710377 from the Netherlands Organization for Scientific Research (Zon-MW).
References
Agarwal, S., Karmaus, W., Davis, S., and Gangur, V. (2011). Review: immune markers in breast milk and fetal and maternal body fluids: a systematic review of perinatal concentrations. J. Hum. Lact. 27, 171–186.
Buonocore, S., Ahern, P. P., Uhlig, H. H., Ivanov, Ii., Littman, D. R., Maloy, K. J., and Powrie, F. (2010). Innate lymphoid cells drive interleukin-23-dependent innate intestinal pathology. Nature 464, 1371–1375.
Cella, M., Fuchs, A., Vermi, W., Facchetti, F., Otero, K., Lennerz, J. K., Doherty, J. M., Mills, J. C., and Colonna, M. (2008). A human natural killer cell subset provides an innate source of IL-22 for mucosal immunity. Nature 457, 722–725.
Crellin, N. K., Trifari, S., Kaplan, C. D., Cupedo, T., and Spits, H. (2010). Human NKp44+IL-22+ cells and LTi-like cells constitute a stable RORC+ lineage distinct from conventional natural killer cells. J. Exp. Med. 207, 281–290.
Cupedo, T., Crellin, N. K., Papazian, N., Rombouts, E. J., Weijer, K., Grogan, J. L., Fibbe, W. E., Cornelissen, J. J., and Spits, H. (2009). Human fetal lymphoid tissue-inducer cells are interleukin 17-producing precursors to RORC+ CD127+ natural killer-like cells. Nat. Immunol. 10, 66–74.
DiGiulio, D. B., Romero, R., Amogan, H. P., Kusanovic, J. P., Bik, E. M., Gotsch, F., Kim, C. J., Erez, O., Edwin, S., and Relman, D. A. (2008). Microbial prevalence, diversity and abundance in amniotic fluid during preterm labor: a molecular and culture-based investigation. PLoS ONE 3, e3056. doi:10.1371/journal.pone.0003056
Drayton, D. L., Liao, S., Mounzer, R. H., and Ruddle, N. H. (2006). Lymphoid organ development: from ontogeny to neogenesis. Nat. Immunol. 7, 344–353.
Eyerich, S., Eyerich, K., Cavani, A., and Schmidt-Weber, C. (2010). IL-17 and IL-22: siblings, not twins. Trends Immunol. 31, 354–361.
Fossiez, F., Djossou, O., Chomarat, P., Flores-Romo, L., Ait-Yahia, S., Maat, C., Pin, J. J., Garrone, P., Garcia, E., Saeland, S., Blanchard, D., Gaillard, C., Das Mahapatra, B., Rouvier, E., Golstein, P., Banchereau, J., and Lebecque, S. (1996). T cell interleukin-17 induces stromal cells to produce proinflammatory and hematopoietic cytokines. J. Exp. Med. 183, 2593–2603.
Geremia, A., Arancibia-Carcamo, C. V., Fleming, M. P., Rust, N., Singh, B., Mortensen, N. J., Travis, S. P., and Powrie, F. (2011). IL-23-responsive innate lymphoid cells are increased in inflammatory bowel disease. J. Exp. Med. 208, 1127–1133.
Hollyoake, M., Campbell, R. D., and Aguado, B. (2005). NKp30 (NCR3) is a pseudogene in 12 inbred and wild mouse strains, but an expressed gene in Mus caroli. Mol. Biol. Evol. 22, 1661–1672.
Kim, M. Y., Gaspal, F. M., Wiggett, H. E., Mcconnell, F. M., Gulbranson-Judge, A., Raykundalia, C., Walker, L. S., Goodall, M. D., and Lane, P. J. (2003). CD4(+)CD3(-) accessory cells costimulate primed CD4 T cells through OX40 and CD30 at sites where T cells collaborate with B cells. Immunity 18, 643–654.
Kim, M. Y., Toellner, K. M., White, A., Mcconnell, F. M., Gaspal, F. M., Parnell, S. M., Jenkinson, E., Anderson, G., and Lane, P. J. (2006). Neonatal and adult CD4+ CD3- cells share similar gene expression profile, and neonatal cells up-regulate OX40 ligand in response to TL1A (TNFSF15). J. Immunol. 177, 3074–3081.
Kurebayashi, S., Ueda, E., Sakaue, M., Patel, D. D., Medvedev, A., Zhang, F., and Jetten, A. M. (2000). Retinoid-related orphan receptor gamma (RORgamma) is essential for lymphoid organogenesis and controls apoptosis during thymopoiesis. Proc. Natl. Acad. Sci. U.S.A. 97, 10132–10137.
Lane, P., Kim, M. Y., Withers, D., Gaspal, F., Bekiaris, V., Desanti, G., Khan, M., Mcconnell, F., and Anderson, G. (2008). Lymphoid tissue inducer cells in adaptive CD4 T cell dependent responses. Semin. Immunol. 20, 159–163.
Mebius, R. E., Rennert, P., and Weissman, I. L. (1997). Developing lymph nodes collect CD4+CD3-LTβ+ cells that can differentiate to APC, NK cells, and follicular cells but not T or B cells. Immunity 7, 493–504.
Mjosberg, J. M., Trifari, S., Crellin, N. K., Peters, C. P., Van Drunen, C. M., Piet, B., Fokkens, W. J., Cupedo, T., and Spits, H. (2011). Human IL-25- and IL-33-responsive type 2 innate lymphoid cells are defined by expression of CRTH2 and CD161. Nat. Immunol. 12, 1055–1062.
Moretta, A., Bottino, C., Vitale, M., Pende, D., Cantoni, C., Mingari, M. C., Biassoni, R., and Moretta, L. (2001). Activating receptors and coreceptors involved in human natural killer cell-mediated cytolysis. Annu. Rev. Immunol. 19, 197–223.
Moro, K., Yamada, T., Tanabe, M., Takeuchi, T., Ikawa, T., Kawamoto, H., Furusawa, J., Ohtani, M., Fujii, H., and Koyasu, S. (2010). Innate production of T(H)2 cytokines by adipose tissue-associated c-Kit(+)Sca-1(+) lymphoid cells. Nature 463, 540–544.
Mshvildadze, M., Neu, J., Shuster, J., Theriaque, D., Li, N., and Mai, V. (2010). Intestinal microbial ecology in premature infants assessed with non-culture-based techniques. J. Pediatr. 156, 20–25.
Neill, D. R., Wong, S. H., Bellosi, A., Flynn, R. J., Daly, M., Langford, T. K., Bucks, C., Kane, C. M., Fallon, P. G., Pannell, R., Jolin, H. E., and Mckenzie, A. N. (2010). Nuocytes represent a new innate effector leukocyte that mediates type-2 immunity. Nature 464, 1367–1370.
Nishikawa, S. I., Hashi, H., Honda, K., Fraser, S., and Yoshida, H. (2000). Inflammation, a prototype for organogenesis of the lymphopoietic/hematopoietic system. Curr. Opin. Immunol. 12, 342–345.
Ouyang, W., Kolls, J. K., and Zheng, Y. (2008). The biological functions of T helper 17 cell effector cytokines in inflammation. Immunity 28, 454–467.
Reynders, A., Yessaad, N., Vu Manh, T. P., Dalod, M., Fenis, A., Aubry, C., Nikitas, G., Escaliere, B., Renauld, J. C., Dussurget, O., Cossart, P., Lecuit, M., Vivier, E., and Tomasello, E. (2011). Identity, regulation and in vivo function of gut NKp46+RORgammat+ and NKp46+ RORgammat- lymphoid cells. EMBO J. 30, 2934–2947.
Ruijter, J. M., Ramakers, C., Hoogaars, W. M., Karlen, Y., Bakker, O., Van Den Hoff, M. J., and Moorman, A. F. (2009). Amplification efficiency: linking baseline and bias in the analysis of quantitative PCR data. Nucleic Acids Res. 37, e45.
Sanos, S. L., Bui, V. L., Mortha, A., Oberle, K., Heners, C., Johner, C., and Diefenbach, A. (2009). ROR[gamma]t and commensal microflora are required for the differentiation of mucosal interleukin 22-producing NKp46+ cells. Nat. Immunol. 10, 83–91.
Satoh-Takayama, N., Vosshenrich, C. A., Lesjean-Pottier, S., Sawa, S., Lochner, M., Rattis, F., Mention, J. J., Thiam, K., Cerf-Bensussan, N., Mandelboim, O., Eberl, G., and Di Santo, J. P. (2008). Microbial flora drives interleukin 22 production in intestinal NKp46+ cells that provide innate mucosal immune defense. Immunity 29, 958–970.
Sawa, S., Cherrier, M., Lochner, M., Satoh-Takayama, N., Fehling, H. J., Langa, F., Di Santo, J. P., and Eberl, G. (2010). Lineage relationship analysis of ROR{gamma}t+ Innate Lymphoid Cells. Science 330, 665–669.
Sawa, S., Lochner, M., Satoh-Takayama, N., Dulauroy, S., Berard, M., Kleinschek, M., Cua, D., Di Santo, J. P., and Eberl, G. (2011). RORgammat(+) innate lymphoid cells regulate intestinal homeostasis by integrating negative signals from the symbiotic microbiota. Nat. Immunol. 12, 320–326.
Spits, H., and Di Santo, J. P. (2011). The expanding family of innate lymphoid cells: regulators and effectors of immunity and tissue remodeling. Nat. Immunol. 12, 21–27.
Sun, Z., Unutmaz, D., Zou, Y. R., Sunshine, M. J., Pierani, A., Brenner-Morton, S., Mebius, R. E., and Littman, D. R. (2000). Requirement for RORgamma in thymocyte survival and lymphoid organ development. Science 288, 2369–2373.
Takatori, H., Kanno, Y., Watford, W. T., Tato, C. M., Weiss, G., Ivanov, Ii., Littman, D. R., and O’Shea, J. J. (2009). Lymphoid tissue inducer-like cells are an innate source of IL-17 and IL-22. J. Exp. Med. 206, 35–41.
van de Pavert, S. A., and Mebius, R. E. (2010). New insights into the development of lymphoid tissues. Nat. Rev. Immunol. 10, 664–674.
Vivier, E., Spits, H., and Cupedo, T. (2009). Interleukin-22-producing innate immune cells: new players in mucosal immunity and tissue repair? Nat. Rev. Immunol. 9, 229–234.
Vonarbourg, C., Mortha, A., Bui, V. L., Hernandez, P. P., Kiss, E. A., Hoyler, T., Flach, M., Bengsch, B., Thimme, R., Holscher, C., Honig, M., Pannicke, U., Schwarz, K., Ware, C. F., Finke, D., and Diefenbach, A. (2010). Regulated expression of nuclear receptor RORgammat confers distinct functional fates to NK cell receptor-expressing RORgammat(+) innate lymphocytes. Immunity 33, 736–751.
Keywords: innate lymphoid cells, human, IL-22, IL-17a, lymph node, tonsil, fetal, RORC
Citation: Hoorweg K, Peters CP, Cornelissen F, Aparicio-Domingo P, Papazian N, Kazemier G, Mjösberg JM, Spits H and Cupedo T (2012) Functional differences between human NKp44− and NKp44+ RORC+ innate lymphoid cells. Front. Immun. 3:72. doi: 10.3389/fimmu.2012.00072
Received: 19 December 2011; Paper pending published: 11 January 2012;
Accepted: 22 March 2012; Published online: 09 April 2012.
Edited by:
Brigitta Stockinger, MRC National Institute for Medical Research, UKReviewed by:
Henrique Veiga-Fernandes, Instituto de Medicina Molecular, PortugalTroy Randall, University of Rochester, USA
Copyright: © 2012 Hoorweg, Peters, Cornelissen, Aparicio-Domingo, Papazian, Kazemier, Mjösberg, Spits and Cupedo. This is an open-access article distributed under the terms of the Creative Commons Attribution Non Commercial License, which permits non-commercial use, distribution, and reproduction in other forums, provided the original authors and source are credited.
*Correspondence: Tom Cupedo, Department of Hematology, Erasmus University Medical Center, PO Box 2040, 3000 CA Rotterdam, Netherlands. e-mail:dC5jdXBlZG9AZXJhc211c21jLm5s
†Kerim Hoorweg and Charlotte P. Peters have contributed equally to this work.