- Chiplunkar Laboratory, Advanced Centre for Treatment, Research and Education in Cancer (ACTREC), Tata Memorial Centre, Navi Mumbai, India
The tumor microenvironment is an important aspect of cancer biology that contributes to tumor initiation, tumor progression and responses to therapy. The composition and characteristics of the tumor microenvironment vary widely and are important in determining the anti-tumor immune response. Successful immunization requires activation of both innate and adaptive immunity. Generally, immune system is compromised in patients with cancer due to immune suppression, loss of tumor antigen expression and dysfunction of antigen presenting cells (APC). Thus, therapeutic immunization leading to cancer regression remains a significant challenge. Certain cells of the immune system, including dendritic cells (DCs) and gamma delta (γδ) T cells are capable of driving potent anti-tumor responses. The property of MHC-unrestricted cytotoxicity, high potential of cytokine release, tissue tropism and early activation in infections and malignant disease makes γδ T cells as an emerging candidate for immunotherapy. Various strategies are being developed to enhance anti-tumor immune responses of γδ T cells and DCs one of them is the use of novel adjuvants like toll like receptors (TLR) agonists, which enhance γδ T cell function directly or through DC activation, which has ability to prime γδ T cells. TLR agonists are being used clinically either alone or in combination with tumor antigens and has shown initial success in both enhancing immune responses and eliciting anti-tumor activity. TLR activated γδ T cells and DCs nurture each other’s activation. This provides a potent base for first line of defense and manipulation of the adaptive response against pathogens and cancer. The available data provides a strong rationale for initiating combinatorial therapy for the treatment of diseases and this review will summarize the application of adjuvants (TLRs) for boosting immune response of γδ T cells to treat cancer and infectious diseases and their use in combinatorial therapy.
Introduction
Innate and adaptive immune responses are sentinels of host against the diverse repertoire of infectious agents (viruses and bacteria) and cancer. Both components of immune system identify invading microorganisms or damaged tissues as non-self and activate immune responses to eliminate them. Efficient immune responses depend upon how close an interaction is between the innate and adaptive immune system. γδ T cells and toll like receptors (TLR) serve as an important link between the innate and adaptive immune responses (1–3). Extensive studies have suggested that γδ T cells play important roles in host defense against microbial infections, tumorigenesis, immunoregulation and development of autoimmunity. γδ T cells also have several innate cell-like characters that allow their early and rapid activation following recognition of cellular stress and infection (4, 5). However to accomplish these functions, γδ T cells use both the T cell receptor (TCR) and additional activating receptors (notably NKG2D, NOTCH, and TLR) to respond to stress-induced ligands and infection. γδ T cells express TLRs and modulate early immune responses against different pathogens (6). In this review, we summarize and discuss some of the recent advances of the γδ T cell biology and how direct control of γδ T lymphocyte function and activation is monitored by TLR receptors and ligands. The review highlights involvement of TLR signaling in γδ T cell functions and their implications in harnessing γδ T cells for cancer immunotherapy.
γδ T Cells, Anatomical Distribution and Antigenic Diversity
Based on the type of TCR they express, T lymphocytes can be divided into two major subsets, αβ and γδ T cells. γδ T cell represents a small subset of T lymphocytes (1–10%) in peripheral blood. While in anatomical locations like small intestine, γδ T cells comprise a major bulk of T cells (25–60% in human gut) (7). γδ T cells are the first T cells to appear in thymus during T cell ontogeny in every vertebrate (8), which suggests that their primary contribution could be neonatal protection because at this point conventional αβ T cell responses are severely functionally impaired and DCs are immature (9). In neonates, the Vδ2+ cells derived from human cord blood showed early signs of activation. These cells secrete IFN-γ and express perforin after short-term in vitro stimulation (10). In comparison to the neonate derived αβ T cells of peripheral blood, γδ T cell subset produces copious amount of IFN-γ and are precociously active (11). Hence, γδ T cells are well engaged in newborns to contribute to immune-protection, immune-regulation and compensate for impaired αβ T cell compartment.
γδ T cells are unconventional CD3+ T cells and differ from the conventional αβ T cells in their biology and function (Table 1). Although a sizeable fraction of γδ T cells in the intraepithelial lymphocyte compartments of human and mice are CD8αα+ but the peripheral blood γδ T cells are predominantly double negative (CD4−CD8−) T cells. The absence of CD4 or CD8 expression on majority of the circulating γδ T cells is well in line with the fact that antigen recognition is not MHC restricted (12, 13). Crystal structure analysis of the γδ TCR revealed that γδ TCR is highly variable in length resembling immuno-globulins (Ig) more than the αβ TCR. The antigen recognition property of γδ T cells is fundamentally different from αβ T cells but similar to antigen–antibody binding, which is more likely to occur independent of MHC cross presentation (14). However, recently butyrophilin BTN3A1, a non-polymorphic ubiquitously expressed molecule was identified as an antigen presenting molecule of Vγ9Vδ2 T cells. Soluble BTN3A1 binds (Isopentenyl diphosphate) IPP and (E)-4-hydroxy-3-methyl-but-2-enyl diphosphate (HMBPP) with different affinities in 1:1 ratio to stimulate γδ T cells (15).
The important feature of γδ T cells is their tropism to epithelial tissues. With respect to anatomical localization, γδ T cell population can be divided into two groups: lymphoid-homing γδ T cells that can be primed in the circulation and clonally expand in a conventional “adaptive” manner; and innate-like cells that respond rapidly and at a relatively high frequency in many tissue sites. Migration and anatomical localization of T lymphocytes is crucial for their antigen specificity and maintaining homeostasis in the mammalian immune system. Although γδ T cells are well represented among peripheral blood mononuclear cells (PBMC) and in afferent and efferent lymph, they are rarely found in lymph node parenchyma, spleen, Peyer’s patches and thymus. Moreover, unlike αβ T cells, splenic γδ T cells, if present, are not confined to the lymphoid areas (the white pulp) but are also found throughout the red pulp of spleen and marginal zones of cell trafficking (16). γδ T cells are abundantly present in the epithelia of skin, genital and intestinal tract (17). In the small intestines of humans, mice, chickens and cattle, γδ T cells comprise a substantial fraction of intestinal intraepithelial lymphocytes (IELs); in mice γδ+ IELs constitute 50–60% of the IEL pool (18–20). The epidermal γδ+ IELs of mice and cattle (but not humans) have a marked dendritic morphology and are hence known as dendritic epidermal T cells (DETCs) (21). DETCs are maintained at steady state in normal adult murine skin but on activation execute specialized functions like tissue repair (22). DETCs also maintain keratinocyte homeostasis, which along with Langerhan cells forms its neighborhood (23). Under pathological conditions, γδ T cells quickly expand and infiltrate into lymphoid compartments and other tissues.
Another striking difference between αβ and γδ T cells is the range of antigens or ligands that are recognized by the respective TCRs. Unlike αβ T cells, which recognize protein antigen processed inside the cell and presented by MHC molecules, γδ T cells recognize antigens like B cells as revealed by structural and functional studies (24).γδ T cells can respond to a variety of stimuli irrespective of their molecular or genetic nature. In mice, the non-classical MHC class I molecules T10 and T22 are recognized by γδ T cells (25–28). Similar to T10 and T20, murine class II MHC (IA) antigens IE and IA are identified to act as ligands for γδ T cell clones (29, 30). In addition, herpes glycoprotein GI-reactive γδ T cell clones protect mice from herpes simplex virus (HSV) induced lethal encephalitis (31, 32). γδ TCRs can also bind to an algal molecule, phycoerythrin inducing upregulation of CD44 and downregulation of CD62L in γδ T cells (33). B6 murine splenic and hepatic γδ T cells respond to cardiolipin (bacterial cell-wall phospholipid and endogenous component of mitochondria) presented by CD1d molecules (34). Insulin derived peptide B:9–23 is also recognized by the γδ T cell clones derived from non-obese diabetic mice (NOD mice) (35). SKINT1, a mouse immunoglobulin superfamily member, bears structural similarity to human CD277 (butyrophilin 3A1) and is expressed by medullary thymic epithelial cells (mTECs) and keratinocytes that is crucial for the development of Vγ5Vδ1+ DETCs (36).
In humans, majority of γδ T cells express a rearranged T cell receptor (TCR) composed of Vγ9 and Vδ2 domains; thus, this population is referred to as Vγ9Vδ2. The Vγ9Vδ2 T cells recognize self and microbial phosphorylated metabolites generated in eukaryotic mevalonate pathway and in the microbial 2-C-methyl-derythritol 4-phosphate (MEP) pathway (37). Initially, it was reported that the non-peptidic ligands isolated from mycobacterial cell lysates were stimulatory for Vγ9Vδ2 T cell clones. Later, IPP, an intermediate metabolite of the mevalonate pathway, was isolated and identified as a stimulatory molecule. Characterization of the microbial antigens recognized by human γδ T cells predicted that these are non-proteinaceous in nature and have critical phosphate residues (37, 38). Subsequent studies, conducted with M. tuberculosis, identified HMBPP, an intermediate metabolite of the MEP pathway, as a strong agonist of γδ TCR. The measured potencies of IPP and HMBPP show an enormous difference. The ED50 of IPP is ~20 μM, whereas that of HMBPP is ~70 pM, i.e., more than 105 times lower (38).
Another stimulatory molecule is Staphylococcus aureus enterotoxin A (SEA) that directly interacts with the TCR Vγ9 chain independently of the paired Vδ chain. The mechanism of recognition of this superantigen is different from that of phosphorylated metabolites and requires the interaction with MHC class II molecules. γδ T cells kill target cells and release cytokines upon interaction with SEA but do not proliferate (39).
Recently, the TCR from a γδ T cell clone derived from a cytomegalovirus (CMV)-infected transplant patient was shown to directly bind to endothelial protein C receptor (EPCR), which is a lipid carrier with a similar structure to CD1, showing again that γδ TCR engagement is cargo independent (40). ATP F1 synthase has been identified as stimulatory ligand of the TCR Vγ9Vδ2. ATP F1 synthase is an intracellular protein complex involved in ATP generation. However, optimal responses of Vγ9Vδ2 T cells by tumor target cell lines expressing F1-ATPase requires apolipoprotein A1. A monoclonal antibody interacting with apolipoprotein A1 was shown to inhibit TCR γδ activation as it disrupted the trimolecular complex of ApoA1, ATP F1 synthase, and γδ TCR required for optimal response (41).
The second major population of human γδ T cells utilizes the Vδ1 chain, which pairs with a variety of Vγ chains. This subset of Vδ1+ T cells is mainly found in tissues and is activated by CD1c and CD1d-expressing cells. The group 1 CD1 molecules have ability to present lipid A to human γδ T cells. The human γδ T cells also recognize the related group 2 CD1 molecule as CD1d/lipid complex. Phosphatidyl ethanol amine (PE), a phospholipid, activates γδ T cells in a CD1d manner dependent suggesting its CD1d restricted recognition (42). In addition, some populations of γδ T cells in normal human PBMCs also recognize lipid molecules such as cardiolipin (a marker of damaged mitochondria), sulfatide (a myelin glycosphingolipid), or α-galactosylceramide (α-GalCer) in association with CD1d, which are noted ligands of natural killer T (NKT) cells (34, 43–45). Human γδ T cells also recognize the stress-induced MHC class I-related MICA/MICB molecules and the UL16-binding proteins that are upregulated on malignant or stressed cells (46–48). Heat shock proteins (HSPs) expressed on the cell membrane play an important role in cancer immunity. Hsp60 expressed on oral tumors act as ligand for Vγ9Vδ2 T cells (49, 50). Hsp60 and Hsp70 expressing human oral and esophageal tumors are lysed by Vγ9Vδ2 T cells (49–51). Hsp72 expressing neutrophils were rapidly killed by γδ T cells through direct cell to cell contact, indicating that hsp72 expression on cell surface pre-disposes inflamed neutrophils to killing by γδ T cells (52). In Another study, hsp90 expression on EBV infected B cells rapidly promoted γδ T cell proliferation (53). This confirms that γδ T cells recognize qualitatively distinct antigens, which are profoundly regulated by their anatomical localization.
Co-Receptors and γδ T Cell Activation
Most γδ T cells respond to non-peptidic antigens even in the absence of antigen presenting cells (APCs). However, the presence of APCs can greatly enhance the γδ T cell response (54). This suggests that accessory molecules/receptors may be involved in effector functions of these cells. Some of important co-receptors used by γδ T cells include NOTCH, NKG2D, and TLR (55).
Our study has identified Notch as an additional signal contributing to antigen specific effector functions of γδ T cells. We have shown that γδ T cells express Notch1 and Notch2 at both mRNA and protein level. Inhibition of Notch signaling in anti-CD3 MAb stimulated γδ T cells resulted in marked decrease in proliferation, cytotoxic potential, and cytokine production by γδ T cells confirming the involvement of Notch signaling in regulating antigen specific responses of γδ T cells (55).
γδ T cells express NKG2D on their cell surface resulting in their activation. Treatment of PBMC with immobilized NKG2D-specific mAb or NKG2D ligand MHC class I related protein A (MICA) resulted in the up-regulation of CD69 and CD25 on Vγ9Vδ2. Furthermore, NKG2D increased the production of TNF-alpha and release of cytolytic granules by Vγ9Vδ2 T cells (56). Later, it was shown that the protein kinase C transduction pathway as a main regulator of the NKG2D-mediated costimulation of anti-tumor Vγ9Vδ2 T cell cytolytic response (57).
TLR agonists are also known to trigger the early activation and the IFN-γ secretion by Vγ9Vδ2T cells (58). TLR ligands indirectly increase the anti-tumoricidal activity of Vγ9Vδ2T cells (59). In this review, we will focus on TLR as an additional co-receptor modulating the function of immune cells with special focus on γδ T cells.
Toll Like Receptor and Immune Cells
The immune system functions in anti-microbial defense by recognizing groups of molecules unique to microorganisms (60). These unique microbial molecules are called pathogen-associated molecular patterns (PAMPs) and are recognized by a family of cellular receptors called pattern recognition receptors (PRRs) (61). TLRs along with retinoic acid-inducible gene (RIG)-I-like receptors (RLRs) and nucleotide-binding oligomerization domain (NOD)-like receptor (NLRs) are prototype PPRs, which recognize pathogen-associated molecular patterns (PAMPs) from microorganisms or danger-associated molecular patterns (DAMPs) from damaged tissues (62). Recognition of PAMPs by TLRs trigger release of inflammatory cytokines and type 1 interferon’s (IFN) for host defense (60, 63–65). The adaptive immune system, on the other hand, is responsible for elimination of pathogens in the late phase of infection and in the generation of immunological memory mediated by B and T cells (66).
TLRs derived their name from Drosophila melanogaster Toll protein based on their homology (67). In mammals, till date 13 members of TLR family has been identified (63, 68–71). TLR1-9 is conserved in humans and mice while TLR10 is non-functional in mice because of a retroviral insertion while TLR11-13 is lost from the human genome. The first TLR identified was TLR4 and recognizes bacterial lipopolysaccharide (LPS) from Gram-negative bacteria (67, 72, 73). TLRs are classified into several groups based on the types of PAMPs they recognize. TLR1, 2, 4 and 6 recognize lipids whereas the highly related TLR7, TLR8 and TLR9 recognize nucleic acids. Murine TLR11 recognizes a protozoan derived profilin-like protein while TLR13 recognizes Vesicular stomatitis virus (63). TLRs are localized in the distinct cellular compartments, for example; TLR1, TLR2, TLR4, TLR5, TLR6, and TLR11 are expressed on the cell surface whereas TLR3, TLR7, TLR8 TLR9, TLR11, TLR12 and TLR13 are expressed in intracellular vesicles such as the endosome and ER. The intracellular TLRs are transported to the intracellular vesicles via UNC93B1, a trans-membrane protein, which is localized in the ER of the cell (70, 71, 74–77). TLR family receptors have a common structural architecture. TLRs are type I integral membrane glycoproteins characterized by multiple extracellular leucine-rich repeats (LRRs) and a single intracellular Toll/interleukin-1 (IL-1) receptor (TIR). TLRs mostly form homo-dimers with a few exceptions, which form heterodimers to trigger a signal. For example, TLR2 forms heterodimers with TLR1 or TLR6 enabling differential recognition of lipopeptides. The TIR domain of TLRs is required for the interaction and recruitment of various adaptor molecules to activate downstream signaling pathway. After recognizing PAMPs, TLRs activate intracellular signaling pathways that lead to the induction of inflammatory cytokine genes such as TNF-α, IL-6, IL-1β and IL-12 through the recruitment of adaptors such as MyD88, TRIF, TRAM, TIRAP and SARM1 (78). MyD88 is a universal adaptor used by all TLRs, except TLR3, to induce inflammatory pathways through activation of MAP Kinases (ERK, JNK, p38) and transcriptional factor NF-κB (63, 79). TLR3 and TLR4 use TRIF to bring activation of alternative pathway (TRIF-dependent pathway) through transcription factors IRF3 and NF-κB to induce type 1 IFN and inflammatory cytokines (80–82). TRAM selectively participates in the activation of the TRIF-dependent pathway downstream of TLR4, but not TLR3 (83, 84). TIRAP functions to recruit MyD88 leading to activation of MyD88-dependent pathway downstream of TLR2 and TLR4 (85, 86).Sterile-α- and armadillo-motif-containing protein 1 (SARM1), was shown to inhibit TRIF and is also critical for TLR-independent innate immunity (87). Thus, signaling pathways can be broadly classified as either MyD88-dependent pathway or TRIF-dependent pathway.
Hornung et al. have showed differential expression of TLR1-10 on human APCs and lymphocytes including T cells and their functional discrepancy in recognition of specific TLR ligands (88). CD4+ T cells express almost all TLRs at mRNA levels but may not express all as functional protein (89, 90). Moreover, they do not respond to all TLR ligands. Stimulation with TLR5, 7, or 8 agonists combined with TCR activation of CD4+T cells resulted in increased proliferation and production of IL-2, IL-8, IL-10, IFN-γ and TNFα (91). There are other reports as well suggesting the functional modulation of subtypes of CD4+ T cells by TLR ligands. The mouse Th1 but not Th2 cells responded to TLR2 agonist and resulted in enhanced proliferation and IFN-γ production independent of TCR stimulation (92). This work validated that the TLR can regulate function of CD4+ T cells even in absence of TCR engagement. CD4+CD25+ regulatory T cells (Tregs) express majority of TLRs with selectively higher expression of TLR2, 4, 5, 7/8, and 10 compared to CD4+CD25− conventional T cells (93). Liu et al. showed that CD4+CD25+ regulatory T cells and CD4+CD25− conventional T cells express TLR2 and proliferated upon stimulation with its agonist. TLR2 stimulation also led to transient loss of Treg suppressive potential through suppression of FOXP3 (94, 95). However, Tregs also express TLR5 but upon stimulation with flagellin (ligand of TLR5), do not proliferate rather showed increased suppressive capacity and enhanced expression of FOXP3 (96). These reports suggest that the suppressive function of Treg can be either enhanced or dampened by the type of TLR ligand engaged. TLR2 stimulation not only abrogates suppressive functions of CD4+ Tregs but also drives naïve as well as effector Treg population toward IL17 producing Th17 phenotype (97). Th17 cells express TLR2 along with TLR6 compared to Th1 and Th2 subsets and promote Th17 differentiation upon Pam3Cys stimulation and accelerates experimental autoimmune encephalomyelitis (98). Like TLR2, TLR4 also regulate the functions of CD4+ T cells. In a mouse model of arthritis, mice lacking TLR2 showed enhanced histopathological scores of arthritis by a shift in T cell balance from Th2 and T regulatory cells toward pathogenic Th1 cells. TLR4, in contrast, contributes to more severe disease by modulating the Th17 cell population and IL-17 production (99, 100). Recently, Li et al. showed that high-mobility group box 1 (HMGB1) proteins decrease Treg/Th17 ratio by inhibiting FOXP3 and enhancing RORγt in CD4+ T cells via TLR4–IL6 axis in patients with chronic hepatitis B infections (101). This shows that HMGB1 (TLR4 ligand) act as a modulator of CD4+ T cells responses in chronic viral inflammation. CD4+ T cells also express intracellular TLRs such as TLR9 and TLR3. Both these TLRs promote T cell survival via activation of NF-κB and MAPK signaling (102). Although the effector functions of CD4+ T cells are regulated by TLRs but the molecular pathway involved in skewing of CD4+ T cell function is poorly understood.
Like CD4+ T cells, CD8+ T cells also show differential expression of TLRs with high expression of TLR3 but lower expression of TRL1,2,5,9,10 compared to CD4+ T cells at mRNA level. It is important to note that the expression of TLR2, TLR3 and TLR5 increases on CD8 T cells in infected tonsils compared to controls (89) indicating immune activating role of TLRs in infections. Stimulation of CD8+ T cells through TLR2 agonists enhances their proliferation and IFN-γ production (103, 104). It also promotes cytolytic activity of CD8+ T cells and enhances anti-tumor response mediated through MyD88-dependent TLR1/2 pathway (105). Recently, Mercier et al. showed that TLR2 cooperate with NOD-containing protein 1 (NOD1) to enhance TCR mediated activation and can serve as alternative co-stimulatory receptor in CD8+ T cells (106). CD8+ T cells also express intracellular TLRs such as TLR3, TLR9 which are more potent in inducing CD8+ T cell activation in vivo (107).
Natural killer (NK) cell is a vital player in innate immune system. They recognize infected and transformed cells with downregulated major histocompatibility complex (MHC) class 1 molecules. They are the primary producers of IFN-γ and are protective against infections. Unlike CD4 and CD8 T cells NK cells as well as CD56+CD3+ NKT cells constitutively express TLR 1–8 with high expression of TLR2 and 3 at mRNA level. They recognize bacterial PAMPs and respond by producing α-defensins (108–111). Human NK cells can also directly recognize Mycobacterium bovis via TLR2 and enhance their cytolytic activity against tumor cells (112). Tumor-associated macrophages induce NK cell IFN-γ production and cytolytic activity upon TLR engagement (113). TLRs modulate NK cell function directly or indirectly to promote antibody dependent cell mediated cytotoxicity and cross presentation of viral antigens to T lymphocytes (114, 115). This highlights that the cells of adaptive immune system do express TLRs and their function can be directly or indirectly modulated by TLR ligands.
Activation of γδ T Cells by TLR Ligands
In 1997, the first human homolog of Drosophila Toll protein was cloned and characterized. It was also established that γδ T cells also express hToll mRNA (67). Purified γδ T cells were found to respond to the E. coli native lipid A in a TCR-independent fashion and the LPS/lipid A-reactive γδ T cells strongly expressed TLR2 mRNA. TLR2 antisense oligonucleotide inhibited the proliferation of γδ T cells in response to the native lipid A as well as the TLR2-deficient mice showed an impaired response of the γδ T cells following injection of native lipid A. These results suggest that TLR2 is involved in the activation of canonical Vγ6/Vδ1 T cells by native lipid A (116). Again, functional presence of TLR2 on Vγ2Vδ2 T cells (also known as Vγ9Vδ2 T cells) was reported when the dual stimulation of Vγ2Vδ2 T cells with anti-TCR antibody and Pam3Cys increased synthesis and secretion of IFN-γ and elevated the levels of CD107a expression. IFN-γ secretion and cell surface CD107a levels are markers of increased effector function in Vγ2Vδ2 T cells (117). Similarly, Bruno et al. reported that IL-23 and TLR2 co-stimulation induces IL17 expression in γδ T cells. However, TLR1 and TLR2 expression was found only on CCR6+ IL-17 producing murine peritoneal γδ T cells but not others. Thus, γδ T cells with innate receptor expression coupled with IL-17 production establishes them as first line of defense that can orchestrate an inflammatory response to pathogen-derived and environmental signals long before Th17 can sense the bacterial invasion (118). Pam3CSK4, TLR2 agonist was able to stimulate only splenic γδ T cell proliferation but not the dermal γδ T cells demonstrating that TLR2 signaling shows tissue tropism. (19). Furthermore, a profound change in the circulating γδ T-cell population was observed in early burn injury (24 h). These γδ T-cells showed TLR2 and TLR4 expression, priming them for TLR reactivity, However TLR expression was specific to circulatory γδ T cell subset and was transient, since it was not observed after post-injury (7 days). Transient nature of the post-burn increase in γδ T-cell TLR expression is likely to be protective to the host, most likely via regulation of inflammation and initiation of healing processes (119).Mitochondrial danger-associated molecular patterns (MTDs) induce TLR2 and TLR4 expression on γδ T cells in dose dependent manner. MTDs also induced the production of IL-1β, IL-6, IL-10, RANTES, and vascular endothelial growth factor by γδ T-cells thereby resulting in initiation of sterile inflammation leading to tissue/cellular repair (120).
Different studies have reported that γδ T cells express TLR3 (121, 122). TLR3 recognizes viral dsRNA, synthetic analogs of dsRNA, polyinosinic–polycytidylic acid [poly (I:C)] and small interfering (si) RNA. The direct stimulation of freshly isolated γδ T cells via TCR and surrogate TLR3 ligand poly (I:C) dramatically increased IFN-γ production. Addition of neutralizing anti-TLR3 mAb inhibited the co-stimulatory effect of poly (I:C), presumably by antagonizing the TLR3 signaling (122). Thus, the integrated signals of TLR3 and TCR induce a strong antiviral effector function in γδ T cells supporting the decisive role of γδ T cells in early defense against viral infection. In other study, it has been reported that γδ cells of term babies and of adults express TLR3 and TLR7 while the preterm babies have reduced levels. The greater levels of IFN-γ protein was observed in adult and cord blood cells co-stimulated with anti-CD3 and poly(I:C) whereas this was not seen in γδ T cell clones of preterm babies. Thus, reduced level of TLR3 expression by preterm-derived clones had an overt functional consequence on IFN-γ levels (11). Interestingly, a primary role of TLR3 in humans appears to mediate resistance to HSV-induced encephalitis (123). Hence, premature babies are particularly susceptible to HSV infection because of reduced levels of TLR3 on γδ T cells.
TLR4 was reported to be absent in the γδ T cells but can become functional in γδ T cells depending on localization, environmental signals, or γδ TCR usage (19, 118, 124). However, our own data has shown that TLR4 is expressed on human γδ T cells. Stimulation of γδ T cells with LPS (TLR4 ligand) increased their proliferation, IFN-γ release, and cytotoxic potential (125). DETCs lack cell surface expression of TLR4–MD2. MD-2 physically associates with TLR4 on the cell surface and is required for LPS signaling. However, TLR4–MD2 expression was upregulated when DETCs emigrated from the epidermis during cutaneous inflammation. The migration signals of DETCs may promote the TLR4–MD2 expression (126). Cairns et al. showed that late post-burn injury increased expression of TLR-4 on splenic T-cells (127). However, Martin et al. reported transient TLR-4 expression post-burn in the circulation or spleen but were specific for the γδ T-cell subset (119). Several evidences suggest that murine γδ T cells recognize LPS/LA through TLR2 or TLR4 (128, 129). Importantly activated γδ T cells, especially Vδ2 T cells, in peripheral blood cells recognize LA, a major component of LPS, via TLR4 resulting in extensive proliferation and production of IFN-γ and TNF-α in vitro (130). The data suggest that γδ T cells play an important role in the control of infection induced by gram negative bacteria. Reynolds et al. showed that a heterogeneous population of γδ T cells responds to LPS via TLR4 dependent manner and demonstrate the crucial and innate role of TLR4 in promoting the activation of γδ T cells, which contributes to the initiation of autoimmune inflammation (100). Another study showed the indirect role of TLR4 in HMGB–TLR4–IL-23–IL17A axis between macrophages and γδ T cells, which contribute to the accumulation of neutrophils and liver inflammation. Necrotic hepatocytes release HMGB1, a damage-associated molecule or TLR4 ligand, which increased IL-23 production of macrophages in a TLR4 dependent manner. IL-23 aids γδ T cells in liver in the generation of IL-17A, which then recruits hepatic neutrophils (131).
Human γδ T cells were found to express appreciable levels of TLR7. Costimulation with poly I:C upregulated the TLR7 expression in TCR-cross linked freshly isolated γδ T cells (124). In addition, tumor-infiltrating γδ T cells also express TLR7 (132). In case of mouse dermal γδ T cells, both TLR7 and TLR9 signaling promoted IL-17 production, which could be synergistically enhanced with the addition of IL-23 (19).
The identification of dominant γδ T cells in the total population of tumor-infiltrating lymphocytes (TILs) in renal, breast, and prostate cancer suggested that these cells might have the potent negative immune regulatory function (132, 133). The breast tumor-derived bulk γδ T cell lines and clones efficiently suppressed the proliferation and IL-2 secretion of naïve/effector T cells and inhibited DC maturation and function. Hence, their depletion or the reversal of their suppressive function could enhance anti-tumor immune responses against breast cancer. Indeed as in CD4+ regulatory T cells (Tregs), the immunosuppressive activity of γδ T cells could be reversed by human TLR8 ligands both in vitro and in vivo. Study revealed that MyD88, TRAF6, IKKα, IKKβ and p38α molecules in γδ1 cells were required for these cells to respond to TLR8 ligands (132, 134, 135). Table 2 shows expression and co-stimulatory effects mediated by TLR activation of γδ T cells
TLRs Modulate Crosstalk between γδ T and Dendritic Cells
The functional fate of effector T cells is governed by antigen presentation and the cytokine milieu in the local environment. Dendritic cells (DCs) being professional APCs, recognize the danger signal, process it, and present it to the T lymphocytes thereby modulate adaptive immune response. γδ T cells influence the antigen presenting property of DCs. DCs pre-incubated with activated γδ T cells enhance the production of IFN-γ by alloreactive T cells in mixed lymphocyte reaction (136). Moreover, γδ T cells not only upregulated CD86 and MHC I expression on DC but themselves get activated, leading to up-regulation of CD25, CD69, and cytokine production (137). These studies showed how γδ T cell and DCs regulate each other’s function. There are reports, which have shown how γδ T cells interact with DC or vice versa via TLR ligands. Leslie et al. reported that stimulation with TLR ligands in γδ/DC cocultures enhanced the maturation and production of IL12p70 by DCs (138). TLR also regulate the γδ T cells and DC crosstalk in microbial context. TLR2-stimulated DCs enhanced IFN-γ production by Vδ2 T cells; conversely, phosphoantigen activated Vδ2 T cells enhanced TLR2-induced DC maturation via IFN-γ, which co-stimulated interleukin-12 (IL-12) p70 secretion by DCs (139). Further, γδ T cells stimulated with TLR7 (CL097) or TLR3 (poly I: C) agonists produce IFN-γ, TNFα and/or IL-6 thereby inducing DC maturation, which prime effector T cells against West Nile Virus (WNV) infection (140). This study confirmed that the antiviral effector immunity may be regulated by interplay of DCs, γδ T cells and TLRs. Similarly, in human’s γδ T cells and DCs regulate each other’s immunostimulatory functions. TLR3 and TLR4 ligands stimulation of human PBMCs induced a rapid and exclusive IFN-γ production by Vγ9Vδ2 subset dependent on type 1 IFN secreted by monocytic DC. TLR-induced IFN-γ response of Vγ9Vδ2 T cells led to efficient DC polarization into IL-12p70-producing cells (58). In another study, it was reported that Vδ2 cells are indirectly activated by BCG and IL-12p70 secreted by DCs. IL-12p70 production by DC is modulated by Toll like receptor 2/4 ligands from BCG and IFN-γ secreted by memory CD4 T cells (141). This study portrayed the complex interplay between cells of the innate and adaptive immune response in contributing to immunosurveillance against pathogenic infections.
TLRs Complement Cytotoxic Potential of γδ T Cells Against Tumor Cells
γδ T cells have capability to lyse different types of tumors and tumor-derived cell lines (49, 50, 142–145). Circulating as well as tumor-infiltrating γδ T cells have the ability to produce abundant proinflammatory cytokines like IFN-γ and TNF-α, cytotoxic mediators and MHC-independent recognition of antigens, render them as important players in cancer immunotherapy (143, 145). In addition to TCR, γδ T cells use additional stimulatory co-receptors or ligands including TLRs to execute effector functions and TLR agonists are considered as adjuvants in clinical trial of cancer immunotherapy (146). Kalyan et al. even quoted that “TLR signaling may perfectly complement the anti-tumor synergy of aminobisphosponates and activated γδ T cells and this combined innate artillery could provide the necessary ammunition to topple malignancy’s stronghold on the immune system” (147). Paradoxically, TLR agonists execute dual role of enhancing immune response (148) as well as increasing invasiveness of tumor cells (149–152). Hence, the tripartite cooperation of tumor cell, TLRs, and γδ T cells should be carefully analyzed. In concordance to this, Shojaei et al. reported that Toll like receptor 3 and 7 agonists enhanced the tumor cell lysis by human γδ T cells. The enhanced capability of γδ T cells to lyse tumor cells was attributed to increased expression of CD54 and downregulation of MHC class 1 on tumor cells. Poly(I:C) treatment of pancreatic adenocarcinomas resulted in overexpression of CD54 and concomitant coculture of tumor cells with γδ T cells led to interaction between CD54 and its ligand CD11a/CD18 triggering effector function in γδ T cells. However, TLR7 surrogate ligand induced downregulation of MHC class 1 molecule on tumor cells resulting in a reduced affinity for inhibitory receptor NKG2A on γδ T cells (59). Manipulation of TLR signaling by using TLR8 agonists reversed the suppressive potential of γδ Tregs found elevated in breast cancer (132). Polysaccharide K (PSK) known for its anti-tumor and immuno-modulatory function can also activate TLR2 leading to increased secretion of IFN-γ by γδ T cells on stimulation. The cell–cell contact between γδ T cells and DC was required for optimal activation of γδ T cells. However, PSK along with anti-TCR could co-activate γδ T cells even in the absence of DC. The study confirmed that the anti-tumor effect of PSK was through activation of γδ T cells (153).
Studies from our lab have shown that the TLR signaling in γδ T cells derived from the oral cancer (OC) patients may be dysfunctional. We reported that γδ T cells from healthy individuals (HI) and OC patients express higher levels of TLR2, TLR3, TLR4, and TLR9 than in αβT cells. Higher TLR expression was observed in HI compared to OC patients. Stimulation with IL2 and TLR agonists (Pam3CSK, Poly I:C, LPS, and CpG ODN) resulted in higher proliferative response of peripheral blood lymphocytes from HI compared to OC patients. However, the role of other immune cells that may influence the TLR ligand stimulation induced activation status of lymphocytes cannot be ignored (125). Impairment in TLR expression/signaling can be viewed as a strategy employed by tumor cells to avoid immune recognition.
TLRs and γδ T Cells in Diseases
Studies have demonstrated the protective role of γδ T cells in infection and inflammation (154–157). Inoue et al. showed that during mycobacterial infection, γδ T cells precedes the αβ T cells, indicating role of γδ T cells as first line of defense against infections (158). The conserved molecular patterns associated with pathogens are directly recognized by γδ T cells leading to rapid protective response against the danger signal. Unlike αβ TCR, γδ TCR acts as pattern recognition receptor providing advantage in anti-infection immunity by directly initiating cytotoxicity against infected cells or through production of cytokine to involve multiple immune system components to combat infection (159, 160). Activated γδ T cells through TLR3 and TLR4 ligands rescue the repressed maturation of virus-infected DCs and mount a potent antiviral response (58, 140). Malarial infection in MyD88 deficient mice resulted in impairment in CD27−IL-17A-producing γδ T cell without affecting the IFN-γ producing γδ T cells (161). This study specifies the role of TLR in promoting proliferation of proinflammatory γδ T cells. Another study by Martin et al. showed that IL17 producing γδ T cells express TLR1 and TLR2 and expand in response to their ligands and mount an adequate response against heat-killed M. tuberculosis or C. albicans infection (118). However, γδ T cell are also known to directly recognize the pathogen-derived molecules and mediate cytotoxic effector function either through secretion of perforin and granzyme B or by secretion of proinflammatory cytokine IL17 (162–164). The involvement of TLRs in regulating anti-microbial γδ T cell function should be investigated in depth to exploit it as a cell based therapy for infectious diseases.
Concluding Remarks
The characteristic copious IFN-γ or IL17 secretion, MHC-independent antigen recognition, tissue tropism, and potent cytotoxicity make γδ T cells promising targets for immunotherapy. Similar to αβ T cells, γδ T cells exhibit functional and phenotypic plasticity, which influences the nature of the downstream adaptive immune response. The adoptive transfer of ex vivo expanded Vγ9Vδ2 T cells or in vivo activation of Vγ9Vδ2 T cells (phosphoantigens or amino-bisphosphonates) can be utilized as adjuvant to conventional therapies. Clinical trials of Vγ9Vδ2 T cells as immunotherapeutic agents have shown encouraging results that could be attributed to its low toxicity grade. Combinations of cellular immune-based therapies with chemotherapy and other anti-tumor agents may be of clinical benefit in the treatment of malignancies. Combinatorial treatment using, chemotherapeutic agents or bisphosphonate zoledronate (ZOL) sensitizes tumor-derived cell lines to rapid γδ T cells killing. Vγ9Vδ2 T cell triggering may be also enhanced by combining TCR stimulation with engagement of TLRs. Various TLR agonists are currently under investigation in clinical trials for their ability to orchestrate anti-tumor immunity. In one study, simultaneous use of both Imiquimod (TLR7 agonist) and CpG–ODN (TLR9 agonist) loaded onto virus like nanoparticles was found to be effective in triggering effector and memory CD8+ T cell response (165). Similarly, combination of γδ T cells and DCs along with nanoparticle loaded TLR agonists can be employed for developing effective immunotherapeutic strategies. The direct or indirect stimulation of γδ T cells by TLR agonists could be a strategy to optimize Th1-mediated immune responses as adjuvant in vaccines against infectious or malignant diseases.
Administration of an “immunogenic chemotherapy” (such as oxaliplatin or anthracycline or an X-ray-based regimen) or local delivery of TLR surrogates in the tumor microenvironment (which stimulate local DCs and provides a source of IL-1β) may be also instrumental in polarization of γδ TILs into IL17 producing cells. Tγδ17 cells play a crucial role in anti-microbial immunity but their role in tumor immunity remains controversial. Tγδ17 have both pro and anti-tumor properties. TLR use in combinatorial therapy, therefore, could be a double edged sword. Careful use of TLR agonists in combinatorial γδ T cell based therapy is needed to strike the balance between pro and anti-tumor effects (Figure 1).
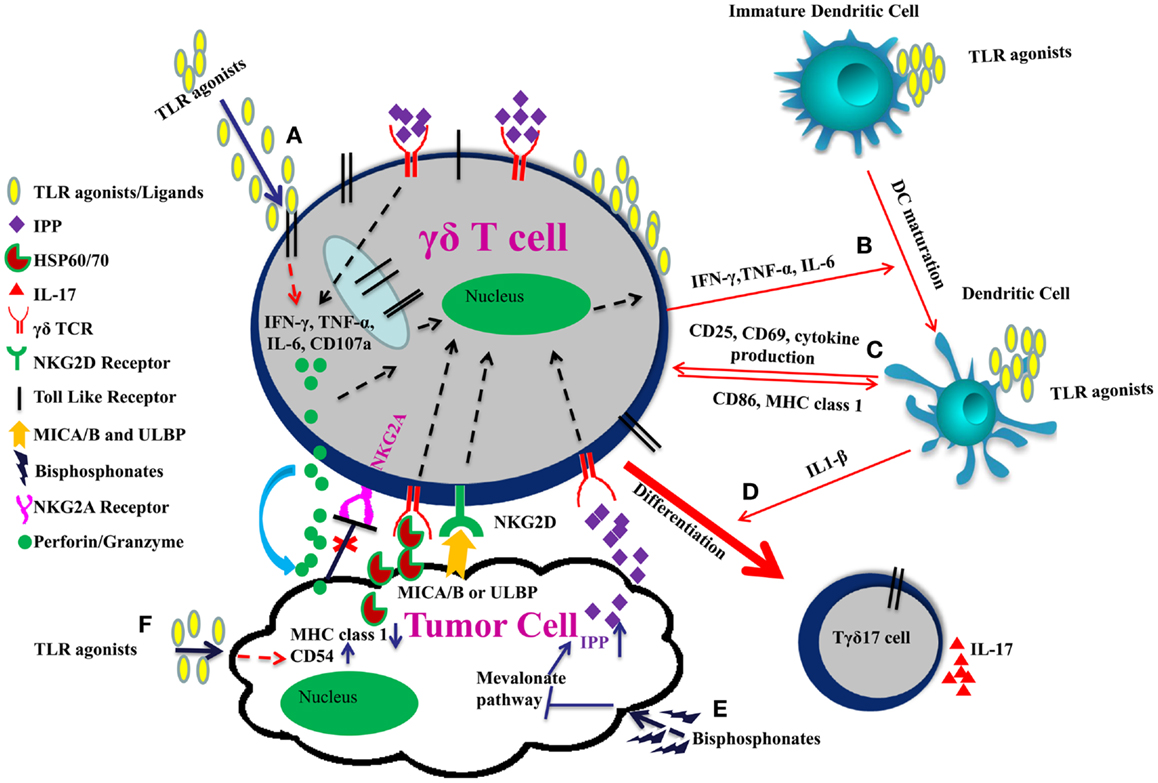
Figure 1. Improving γδ T cell functions by TLRs in combinatorial therapy. (A) TLR agonists induce effector function of γδ T cells through IFN-γ, TNF-α, IL-6 secretion, and increased expression of CD107a. (B) IFN-γ, TNF-α, and IL-6 secreted by γδ T cells and TLR agonists promote the maturation of dendritic cell. (C) γδ T cells upregulate CD86 and MHC I expression on DCs and are themselves activated through up-regulation of CD25, CD69, and cytokine production thereby modulating each other’s function. (D) Co-stimulation of γδ T cells with TLR agonists and IL-1β secreted by dendritic cells promote their polarization toward IL17 producing cells. (E) γδ TCR also recognizes the specific molecular patterns such as IPP, which are induced upon inhibition of mevalonate pathway by bisphosphonates. Moreover, NKG2D receptor on γδ T cells recognizes MICA/B or ULBP expressed on tumor cells. This binding enhances release of perforins and granzymes by the γδ T cells leading to tumor cell lysis. (F) TLR agonists act as adjuvants and can induce CD54 expression and downregulation of MHC class 1 on tumor cells. Interaction between CD54 and its ligand CD11a/CD18 trigger effector functions in γδ T cells. Downregulation of MHC class 1 molecule on tumor cells result in reduced signaling through the inhibitory receptor NKG2A on γδ T cells, which enhances the cytotoxic potential of γδ T cell.
Conflict of Interest Statement
The authors declare that the research was conducted in the absence of any commercial or financial relationships that could be construed as a potential conflict of interest.
References
1. Tipping PG. Toll-like receptors: the interface between innate and adaptive immunity. J Am Soc Nephrol (2006) 17(7):1769–71. doi: 10.1681/ASN.2006050489
2. Holtmeier W, Kabelitz D. Gammadelta T cells link innate and adaptive immune responses. Chem Immunol Allergy (2005) 86:151–83. doi:10.1159/000086659
3. Akira S, Takeda K, Kaisho T. Toll-like receptors: critical proteins linking innate and acquired immunity. Nat Immunol (2001) 2(8):675–80. doi:10.1038/90609
4. Bonneville M, O’Brien RL, Born WK. Gammadelta T cell effector functions: a blend of innate programming and acquired plasticity. Nat Rev Immunol (2010) 10(7):467–78. doi:10.1038/nri2781
5. Hayday AC. Gammadelta T cells and the lymphoid stress-surveillance response. Immunity (2009) 31(2):184–96. doi:10.1016/j.immuni.2009.08.006
6. Wesch D, Peters C, Oberg HH, Pietschmann K, Kabelitz D. Modulation of gammadelta T cell responses by TLR ligands. Cell Mol Life Sci (2011) 68(14):2357–70. doi:10.1007/s00018-011-0699-1
7. Hayday AC. [Gamma][delta] cells: a right time and a right place for a conserved third way of protection. Annu Rev Immunol (2000) 18:975–1026. doi:10.1146/annurev.immunol.18.1.975
8. Starr TK, Jameson SC, Hogquist KA. Positive and negative selection of T cells. Annu Rev Immunol (2003) 21:139–76. doi:10.1146/annurev.immunol.21.120601.141107
9. Velilla PA, Rugeles MT, Chougnet CA. Defective antigen-presenting cell function in human neonates. Clin Immunol (2006) 121(3):251–9. doi:10.1016/j.clim.2006.08.010
10. De Rosa SC, Andrus JP, Perfetto SP, Mantovani JJ, Herzenberg LA, Herzenberg LA, et al. Ontogeny of gamma delta T cells in humans. J Immunol (2004) 172(3):1637–45. doi:10.4049/jimmunol.172.3.1637
11. Gibbons DL, Haque SF, Silberzahn T, Hamilton K, Langford C, Ellis P, et al. Neonates harbour highly active gammadelta T cells with selective impairments in preterm infants. Eur J Immunol (2009) 39(7):1794–806. doi:10.1002/eji.200939222
12. Shin S, El-Diwany R, Schaffert S, Adams EJ, Garcia KC, Pereira P, et al. Antigen recognition determinants of gammadelta T cell receptors. Science (2005) 308(5719):252–5. doi:10.1126/science.1106480
13. Kalyan S, Kabelitz D. Defining the nature of human gammadelta T cells: a biographical sketch of the highly empathetic. Cell Mol Immunol (2013) 10(1):21–9. doi:10.1038/cmi.2012.44
14. Li H, Lebedeva MI, Llera AS, Fields BA, Brenner MB, Mariuzza RA. Structure of the Vdelta domain of a human gammadelta T-cell antigen receptor. Nature (1998) 391(6666):502–6. doi:10.1038/35172
15. Vavassori S, Kumar A, Wan GS, Ramanjaneyulu GS, Cavallari M, El Daker S, et al. Butyrophilin 3A1 binds phosphorylated antigens and stimulates human gammadelta T cells. Nat Immunol (2013) 14(9):908–16. doi:10.1038/ni.2665
16. Bordessoule D, Gaulard P, Mason DY. Preferential localisation of human lymphocytes bearing gamma delta T cell receptors to the red pulp of the spleen. J Clin Pathol (1990) 43(6):461–4. doi:10.1136/jcp.43.6.461
17. Itohara S, Farr AG, Lafaille JJ, Bonneville M, Takagaki Y, Haas W, et al. Homing of a gamma delta thymocyte subset with homogeneous T-cell receptors to mucosal epithelia. Nature (1990) 343(6260):754–7. doi:10.1038/343754a0
18. Goodman T, Lefrancois L. Intraepithelial lymphocytes. Anatomical site, not T cell receptor form, dictates phenotype and function. J Exp Med (1989) 170(5):1569–81. doi:10.1084/jem.170.5.1569
19. Cai Y, Shen X, Ding C, Qi C, Li K, Li X, et al. Pivotal role of dermal IL-17-producing gammadelta T cells in skin inflammation. Immunity (2011) 35(4):596–610. doi:10.1016/j.immuni.2011.08.001
20. Gray EE, Suzuki K, Cyster JG. Cutting edge: identification of a motile IL-17-producing gammadelta T cell population in the dermis. J Immunol (2011) 186(11):6091–5. doi:10.4049/jimmunol.1100427
21. Havran WL, Allison JP. Origin of Thy-1+ dendritic epidermal cells of adult mice from fetal thymic precursors. Nature (1990) 344(6261):68–70. doi:10.1038/344068a0
22. Jameson J, Ugarte K, Chen N, Yachi P, Fuchs E, Boismenu R, et al. A role for skin gammadelta T cells in wound repair. Science (2002) 296(5568):747–9. doi:10.1126/science.1069639
23. Sharp LL, Jameson JM, Cauvi G, Havran WL. Dendritic epidermal T cells regulate skin homeostasis through local production of insulin-like growth factor 1. Nat Immunol (2005) 6(1):73–9. doi:10.1038/ni1152
24. Chien YH, Jores R, Crowley MP. Recognition by gamma/delta T cells. Annu Rev Immunol (1996) 14:511–32. doi:10.1146/annurev.immunol.14.1.511
25. Adams EJ, Chien YH, Garcia KC. Structure of a gammadelta T cell receptor in complex with the nonclassical MHC T22. Science (2005) 308(5719):227–31. doi:10.1126/science.1106885
26. Bluestone JA, Cron RQ, Cotterman M, Houlden BA, Matis LA. Structure and specificity of T cell receptor gamma/delta on major histocompatibility complex antigen-specific CD3+, CD4-, CD8- T lymphocytes. J Exp Med (1988) 168(5):1899–916. doi:10.1084/jem.168.5.1899
27. Crowley MP, Reich Z, Mavaddat N, Altman JD, Chien Y. The recognition of the nonclassical major histocompatibility complex (MHC) class I molecule, T10, by the gammadelta T cell, G8. J Exp Med (1997) 185(7):1223–30. doi:10.1084/jem.185.7.1223
28. Bonneville M, Ito K, Krecko EG, Itohara S, Kappes D, Ishida I, et al. Recognition of a self major histocompatibility complex TL region product by gamma delta T-cell receptors. Proc Natl Acad Sci U S A (1989) 86(15):5928–32. doi:10.1073/pnas.86.15.5928
29. Matis LA, Fry AM, Cron RQ, Cotterman MM, Dick RF, Bluestone JA. Structure and specificity of a class II MHC alloreactive gamma delta T cell receptor heterodimer. Science (1989) 245(4919):746–9. doi:10.1126/science.2528206
30. Schild H, Mavaddat N, Litzenberger C, Ehrich EW, Davis MM, Bluestone JA, et al. The nature of major histocompatibility complex recognition by gamma delta T cells. Cell (1994) 76(1):29–37. doi:10.1016/0092-8674(94)90170-8
31. Sciammas R, Kodukula P, Tang Q, Hendricks RL, Bluestone JA. T cell receptor-gamma/delta cells protect mice from herpes simplex virus type 1-induced lethal encephalitis. J Exp Med (1997) 185(11):1969–75. doi:10.1084/jem.185.11.1969
32. Johnson RM, Lancki DW, Sperling AI, Dick RF, Spear PG, Fitch FW, et al. A murine CD4-, CD8- T cell receptor-gamma delta T lymphocyte clone specific for herpes simplex virus glycoprotein I. J Immunol (1992) 148(4):983–8.
33. Zeng X, Wei YL, Huang J, Newell EW, Yu H, Kidd BA, et al. Gammadelta T cells recognize a microbial encoded B cell antigen to initiate a rapid antigen-specific interleukin-17 response. Immunity (2012) 37(3):524–34. doi:10.1016/j.immuni.2012.06.011
34. Dieudé M, Striegl H, Tyznik AJ, Wang J, Behar SM, Piccirillo CA, et al. Cardiolipin binds to CD1d and stimulates CD1d-restricted gammadelta T cells in the normal murine repertoire. J Immunol (2011) 186(8):4771–81. doi:10.4049/jimmunol.1000921
35. Zhang L, Jin N, Nakayama M, O’Brien RL, Eisenbarth GS, Born WK. Gamma delta T cell receptors confer autonomous responsiveness to the insulin-peptide B:9-23. J Autoimmun (2010) 34(4):478–84. doi:10.1016/j.jaut.2009.12.008
36. Havran WL, Chien YH, Allison JP. Recognition of self antigens by skin-derived T cells with invariant gamma delta antigen receptors. Science (1991) 252(5011):1430–2. doi:10.1126/science.1828619
37. Tanaka Y, Sano S, Nieves E, De Libero G, Rosa D, Modlin RL, et al. Nonpeptide ligands for human gamma delta T cells. Proc Natl Acad Sci U S A (1994) 91(17):8175–9. doi:10.1073/pnas.91.17.8175
38. Tanaka Y, Morita CT, Tanaka Y, Nieves E, Brenner MB, Bloom BR. Natural and synthetic non-peptide antigens recognized by human gamma delta T cells. Nature (1995) 375(6527):155–8. doi:10.1038/375155a0
39. Rust CJ, Verreck F, Vietor H, Koning F. Specific recognition of staphylococcal enterotoxin A by human T cells bearing receptors with the V gamma 9 region. Nature (1990) 346(6284):572–4. doi:10.1038/346572a0
40. Willcox CR, Pitard V, Netzer S, Couzi L, Salim M, Silberzahn T, et al. Cytomegalovirus and tumor stress surveillance by binding of a human gammadelta T cell antigen receptor to endothelial protein C receptor. Nat Immunol (2012) 13(9):872–9. doi:10.1038/ni.2394
41. Scotet E, Martinez LO, Grant E, Barbaras R, Jenö P, Guiraud M, et al. Tumor recognition following Vgamma9Vdelta2 T cell receptor interactions with a surface F1-ATPase-related structure and apolipoprotein A-I. Immunity (2005) 22(1):71–80. doi:10.1016/j.immuni.2004.11.012
42. Russano AM, Agea E, Corazzi L, Postle AD, De Libero G, Porcelli S, et al. Recognition of pollen-derived phosphatidyl-ethanolamine by human CD1d-restricted gamma delta T cells. J Allergy Clin Immunol (2006) 117(5):1178–84. doi:10.1016/j.jaci.2006.01.001
43. Bai L, Picard D, Anderson B, Chaudhary V, Luoma A, Jabri B, et al. The majority of CD1d-sulfatide-specific T cells in human blood use a semiinvariant Vdelta1 TCR. Eur J Immunol (2012) 42(9):2505–10. doi:10.1002/eji.201242531
44. Uldrich AP, Le Nours J, Pellicci DG, Gherardin NA, McPherson KG, Lim RT, et al. CD1d-lipid antigen recognition by the gammadelta TCR. Nat Immunol (2013) 14(11):1137–45. doi:10.1038/ni.2713
45. Luoma AM, Castro CD, Mayassi T, Bembinster LA, Bai L, Picard D, et al. Crystal structure of Vdelta1 T cell receptor in complex with CD1d-sulfatide shows MHC-like recognition of a self-lipid by human gammadelta T cells. Immunity (2013) 39(6):1032–42. doi:10.1016/j.immuni.2013.11.001
46. Groh V, Steinle A, Bauer S, Spies T. Recognition of stress-induced MHC molecules by intestinal epithelial gammadelta T cells. Science (1998) 279(5357):1737–40. doi:10.1126/science.279.5357.1737
47. Wu J, Groh V, Spies T. T cell antigen receptor engagement and specificity in the recognition of stress-inducible MHC class I-related chains by human epithelial gamma delta T cells. J Immunol (2002) 169(3):1236–40. doi:10.4049/jimmunol.169.3.1236
48. Kong Y, Cao W, Xi X, Ma C, Cui L, He W. The NKG2D ligand ULBP4 binds to TCRgamma9/delta2 and induces cytotoxicity to tumor cells through both TCRgammadelta and NKG2D. Blood (2009) 114(2):310–7. doi:10.1182/blood-2008-12-196287
49. Laad AD, Thomas ML, Fakih AR, Chiplunkar SV. Human gamma delta T cells recognize heat shock protein-60 on oral tumor cells. Int J Cancer (1999) 80(5):709–14. doi:10.1002/(SICI)1097-0215(19990301)80:5<709::AID-IJC14>3.0.CO;2-R
50. Thomas ML, Samant UC, Deshpande RK, Chiplunkar SV. Gammadelta T cells lyse autologous and allogenic oesophageal tumours: involvement of heat-shock proteins in the tumour cell lysis. Cancer Immunol Immunother (2000) 48(11):653–9. doi:10.1007/s002620050014
51. Zhang H, Hu H, Jiang X, He H, Cui L, He W. Membrane HSP70: the molecule triggering gammadelta T cells in the early stage of tumorigenesis. Immunol Invest (2005) 34(4):453–68. doi:10.1080/08820130500265349
52. Hirsh MI, Hashiguchi N, Chen Y, Yip L, Junger WG. Surface expression of HSP72 by LPS-stimulated neutrophils facilitates gammadeltaT cell-mediated killing. Eur J Immunol (2006) 36(3):712–21. doi:10.1002/eji.200535422
53. Kotsiopriftis M, Tanner JE, Alfieri C. Heat shock protein 90 expression in Epstein-Barr virus-infected B cells promotes gammadelta T-cell proliferation in vitro. J Virol (2005) 79(11):7255–61. doi:10.1128/JVI.79.11.7255-7261.2005
54. Morita CT, Beckman EM, Bukowski JF, Tanaka Y, Band H, Bloom BR, et al. Direct presentation of nonpeptide prenyl pyrophosphate antigens to human gamma delta T cells. Immunity (1995) 3(4):495–507. doi:10.1016/1074-7613(95)90178-7
55. Gogoi D, Dar AA, Chiplunkar SV. Involvement of Notch in activation and effector functions of gammadelta T cells. J Immunol (2014) 192(5):2054–62. doi:10.4049/jimmunol.1300369
56. Rincon-Orozco B, Kunzmann V, Wrobel P, Kabelitz D, Steinle A, Herrmann T. Activation of V gamma 9V delta 2 T cells by NKG2D. J Immunol (2005) 175(4):2144–51. doi:10.4049/jimmunol.175.4.2144
57. Nedellec S, Sabourin C, Bonneville M, Scotet E. NKG2D costimulates human V gamma 9V delta 2 T cell antitumor cytotoxicity through protein kinase C theta-dependent modulation of early TCR-induced calcium and transduction signals. J Immunol (2010) 185(1):55–63. doi:10.4049/jimmunol.1000373
58. Devilder MC, Allain S, Dousset C, Bonneville M, Scotet E. Early triggering of exclusive IFN-gamma responses of human Vgamma9Vdelta2 T cells by TLR-activated myeloid and plasmacytoid dendritic cells. J Immunol (2009) 183(6):3625–33. doi:10.4049/jimmunol.0901571
59. Shojaei H, Oberg HH, Juricke M, Marischen L, Kunz M, Mundhenke C, et al. Toll-like receptors 3 and 7 agonists enhance tumor cell lysis by human gammadelta T cells. Cancer Res (2009) 69(22):8710–7. doi:10.1158/0008-5472.CAN-09-1602
60. Janeway CA Jr, Medzhitov R. Innate immune recognition. Annu Rev Immunol (2002) 20:197–216. doi:10.1146/annurev.immunol.20.083001.084359
61. Mogensen TH. Pathogen recognition and inflammatory signaling in innate immune defenses. Clin Microbiol Rev (2009) 22(2):240–73. doi:10.1128/CMR.00046-08
62. Janeway CA Jr. Approaching the asymptote? Evolution and revolution in immunology. Cold Spring Harb Symp Quant Biol (1989) 54(Pt 1):1–13. doi:10.1101/SQB.1989.054.01.003
63. Akira S, Uematsu S, Takeuchi O. Pathogen recognition and innate immunity. Cell (2006) 124(4):783–801. doi:10.1016/j.cell.2006.02.015
64. Beutler BA. TLRs and innate immunity. Blood (2009) 113(7):1399–407. doi:10.1182/blood-2008-07-019307
65. Medzhitov R. Recognition of microorganisms and activation of the immune response. Nature (2007) 449(7164):819–26. doi:10.1038/nature06246
66. Pasare C, Medzhitov R. Toll-like receptors: linking innate and adaptive immunity. Microbes Infect (2004) 6(15):1382–7. doi:10.1016/j.micinf.2004.08.018
67. Medzhitov R, Preston-Hurlburt P, Janeway CA Jr. A human homologue of the Drosophila toll protein signals activation of adaptive immunity. Nature (1997) 388(6640):394–7. doi:10.1038/41131
68. Takeda K, Akira S. Toll-like receptors in innate immunity. Int Immunol (2005) 17(1):1–14. doi:10.1093/intimm/dxh186
69. Shi Z, Cai Z, Sanchez A, Zhang T, Wen S, Wang J, et al. A novel toll-like receptor that recognizes vesicular stomatitis virus. J Biol Chem (2011) 286(6):4517–24. doi:10.1074/jbc.M110.159590
70. Oldenburg M, Krüger A, Ferstl R, Kaufmann A, Nees G, Sigmund A, et al. TLR13 recognizes bacterial 23S rRNA devoid of erythromycin resistance-forming modification. Science (2012) 337(6098):1111–5. doi:10.1126/science.1220363
71. Koblansky AA, Jankovic D, Oh H, Hieny S, Sungnak W, Mathur R, et al. Recognition of profilin by toll-like receptor 12 is critical for host resistance to Toxoplasma gondii. Immunity (2013) 38(1):119–30. doi:10.1016/j.immuni.2012.09.016
72. Rock FL, Hardiman G, Timans JC, Kastelein RA, Bazan JF. A family of human receptors structurally related to Drosophila toll. Proc Natl Acad Sci U S A (1998) 95(2):588–93. doi:10.1073/pnas.95.2.588
73. Poltorak A, He X, Smirnova I, Liu MY, Van Huffel C, Du X, et al. Defective LPS signaling in C3H/HeJ and C57BL/10ScCr mice: mutations in Tlr4 gene. Science (1998) 282(5396):2085–8. doi:10.1126/science.282.5396.2085
74. Tabeta K, Georgel P, Janssen E, Du X, Hoebe K, Crozat K, et al. Toll-like receptors 9 and 3 as essential components of innate immune defense against mouse cytomegalovirus infection. Proc Natl Acad Sci U S A (2004) 101(10):3516–21. doi:10.1073/pnas.0400525101
75. Brinkmann MM, Spooner E, Hoebe K, Beutler B, Ploegh HL, Kim YM. The interaction between the ER membrane protein UNC93B and TLR3, 7, and 9 is crucial for TLR signaling. J Cell Biol (2007) 177(2):265–75. doi:10.1083/jcb.200612056
76. Kim YM, Brinkmann MM, Paquet ME, Ploegh HL. UNC93B1 delivers nucleotide-sensing toll-like receptors to endolysosomes. Nature (2008) 452(7184):234–8. doi:10.1038/nature06726
77. Ewald SE, Lee BL, Lau L, Wickliffe KE, Shi GP, Chapman HA, et al. The ectodomain of toll-like receptor 9 is cleaved to generate a functional receptor. Nature (2008) 456(7222):658–62. doi:10.1038/nature07405
78. O’Neill LA, Golenbock D, Bowie AG. The history of toll-like receptors – redefining innate immunity. Nat Rev Immunol (2013) 13(6):453–60. doi:10.1038/nri3446
79. Wesche H, Henzel WJ, Shillinglaw W, Li S, Cao Z. MyD88: an adapter that recruits IRAK to the IL-1 receptor complex. Immunity (1997) 7(6):837–47. doi:10.1016/S1074-7613(00)80402-1
80. Yamamoto M, Sato S, Mori K, Hoshino K, Takeuchi O, Takeda K, et al. Cutting edge: a novel toll/IL-1 receptor domain-containing adapter that preferentially activates the IFN-beta promoter in the toll-like receptor signaling. J Immunol (2002) 169(12):6668–72. doi:10.4049/jimmunol.169.12.6668
81. Oshiumi H, Matsumoto M, Funami K, Akazawa T, Seya T. TICAM-1, an adaptor molecule that participates in toll-like receptor 3-mediated interferon-beta induction. Nat Immunol (2003) 4(2):161–7. doi:10.1038/ni886
82. Yamamoto M, Sato S, Hemmi H, Hoshino K, Kaisho T, Sanjo H, et al. Role of adaptor TRIF in the MyD88-independent toll-like receptor signaling pathway. Science (2003) 301(5633):640–3. doi:10.1126/science.1087262
83. Fitzgerald KA, Rowe DC, Barnes BJ, Caffrey DR, Visintin A, Latz E, et al. LPS-TLR4 signaling to IRF-3/7 and NF-kappaB involves the toll adapters TRAM and TRIF. J Exp Med (2003) 198(7):1043–55. doi:10.1084/jem.20031023
84. Yamamoto M, Sato S, Hemmi H, Uematsu S, Hoshino K, Kaisho T, et al. TRAM is specifically involved in the toll-like receptor 4-mediated MyD88- independent signaling pathway. Nat Immunol (2003) 4(11):1144–50. doi:10.1038/ni986
85. Fitzgerald KA, Palsson-McDermott EM, Bowie AG, Jefferies CA, Mansell AS, Brady G, et al. Mal (MyD88-adapter-like) is required for toll-like receptor-4 signal transduction. Nature (2001) 413(6851):78–83. doi:10.1038/35092578
86. Horng T, Barton GM, Medzhitov R. TIRAP: an adapter molecule in the toll signaling pathway. Nat Immunol (2001) 2(9):835–41. doi:10.1038/ni0901-835
87. Carty M, Goodbody R, Schröder M, Stack J, Moynagh PN, Bowie AG. The human adaptor SARM negatively regulates adaptor protein TRIF-dependent toll-like receptor signaling. Nat Immunol (2006) 7(10):1074–81. doi:10.1038/ni1382
88. Hornung V, Rothenfusser S, Britsch S, Krug A, Jahrsdörfer B, Giese T, et al. Quantitative expression of toll-like receptor 1-10 mRNA in cellular subsets of human peripheral blood mononuclear cells and sensitivity to CpG oligodeoxynucleotides. J Immunol (2002) 168(9):4531–7. doi:10.4049/jimmunol.168.9.4531
89. Mansson A, Adner M, Cardell LO. Toll-like receptors in cellular subsets of human tonsil T cells: altered expression during recurrent tonsillitis. Respir Res (2006) 7:36. doi:10.1186/1465-9921-7-36
90. Kabelitz D. Expression and function of toll-like receptors in T lymphocytes. Curr Opin Immunol (2007) 19(1):39–45. doi:10.1016/j.coi.2006.11.007
91. Caron G, Duluc D, Frémaux I, Jeannin P, David C, Gascan H, et al. Direct stimulation of human T cells via TLR5 and TLR7/8: flagellin and R-848 up-regulate proliferation and IFN-gamma production by memory CD4+ T cells. J Immunol (2005) 175(3):1551–7. doi:10.4049/jimmunol.175.3.1551
92. Imanishi T, Hara H, Suzuki S, Suzuki N, Akira S, Saito T. Cutting edge: TLR2 directly triggers Th1 effector functions. J Immunol (2007) 178(11):6715–9. doi:10.4049/jimmunol.178.11.6715
93. Dai J, Liu B, Li Z. Regulatory T cells and toll-like receptors: what is the missing link? Int Immunopharmacol (2009) 9(5):528–33. doi:10.1016/j.intimp.2009.01.027
94. Liu H, Komai-Koma M, Xu D, Liew FY. Toll-like receptor 2 signaling modulates the functions of CD4+ CD25+ regulatory T cells. Proc Natl Acad Sci U S A (2006) 103(18):7048–53. doi:10.1073/pnas.0601554103
95. Sutmuller RP, den Brok MH, Kramer M, Bennink EJ, Toonen LW, Kullberg BJ, et al. Toll-like receptor 2 controls expansion and function of regulatory T cells. J Clin Invest (2006) 116(2):485–94. doi:10.1172/JCI25439
96. Crellin NK, Garcia RV, Hadisfar O, Allan SE, Steiner TS, Levings MK. Human CD4+ T cells express TLR5 and its ligand flagellin enhances the suppressive capacity and expression of FOXP3 in CD4+CD25+ T regulatory cells. J Immunol (2005) 175(12):8051–9. doi:10.4049/jimmunol.175.12.8051
97. Nyirenda MH, Sanvito L, Darlington PJ, O’Brien K, Zhang GX, Constantinescu CS, et al. TLR2 stimulation drives human naive and effector regulatory T cells into a Th17-like phenotype with reduced suppressive function. J Immunol (2011) 187(5):2278–90. doi:10.4049/jimmunol.1003715
98. Reynolds JM, Pappu BP, Peng J, Martinez GJ, Zhang Y, Chung Y, et al. Toll-like receptor 2 signaling in CD4(+) T lymphocytes promotes T helper 17 responses and regulates the pathogenesis of autoimmune disease. Immunity (2010) 32(5):692–702. doi:10.1016/j.immuni.2010.04.010
99. Abdollahi-Roodsaz S, Joosten LA, Koenders MI, Devesa I, Roelofs MF, Radstake TR, et al. Stimulation of TLR2 and TLR4 differentially skews the balance of T cells in a mouse model of arthritis. J Clin Invest (2008) 118(1):205–16. doi:10.1172/JCI32639
100. Reynolds JM, Martinez GJ, Chung Y, Dong C. Toll-like receptor 4 signaling in T cells promotes autoimmune inflammation. Proc Natl Acad Sci U S A (2012) 109(32):13064–9. doi:10.1073/pnas.1120585109
101. Li J, Wang FP, She WM, Yang CQ, Li L, Tu CT, et al. Enhanced high-mobility group box 1 (HMGB1) modulates regulatory T cells (Treg)/T helper 17 (Th17) balance via toll-like receptor (TLR)-4-interleukin (IL)-6 pathway in patients with chronic hepatitis B. J Viral Hepat (2014) 21(2):129–40. doi:10.1111/jvh.12152
102. Reynolds JM, Dong C. Toll-like receptor regulation of effector T lymphocyte function. Trends Immunol (2013) 34(10):511–9. doi:10.1016/j.it.2013.06.003
103. Mercier BC, Cottalorda A, Coupet CA, Marvel J, Bonnefoy-Bérard N. TLR2 engagement on CD8 T cells enables generation of functional memory cells in response to a suboptimal TCR signal. J Immunol (2009) 182(4):1860–7. doi:10.4049/jimmunol.0801167
104. Cottalorda A, Mercier BC, Mbitikon-Kobo FM, Arpin C, Teoh DY, McMichael A, et al. TLR2 engagement on memory CD8(+) T cells improves their cytokine-mediated proliferation and IFN-gamma secretion in the absence of Ag. Eur J Immunol (2009) 39(10):2673–81. doi:10.1002/eji.200939627
105. Asprodites N, Zheng L, Geng D, Velasco-Gonzalez C, Sanchez-Perez L, Davila E. Engagement of toll-like receptor-2 on cytotoxic T-lymphocytes occurs in vivo and augments antitumor activity. FASEB J (2008) 22(10):3628–37. doi:10.1096/fj.08-108274
106. Mercier BC, Ventre E, Fogeron ML, Debaud AL, Tomkowiak M, Marvel J, et al. NOD1 cooperates with TLR2 to enhance T cell receptor-mediated activation in CD8 T cells. PLoS One (2012) 7(7):e42170. doi:10.1371/journal.pone.0042170
107. Mandraju R, Murray S, Forman J, Pasare C. Differential ability of surface and endosomal TLRs to induce CD8 T cell responses in vivo. J Immunol (2014) 192(9):4303–15. doi:10.4049/jimmunol.1302244
108. Chalifour A, Jeannin P, Gauchat JF, Blaecke A, Malissard M, N’Guyen T, et al. Direct bacterial protein PAMP recognition by human NK cells involves TLRs and triggers alpha-defensin production. Blood (2004) 104(6):1778–83. doi:10.1182/blood-2003-08-2820
109. Sivori S, Falco M, Della Chiesa M, Carlomagno S, Vitale M, Moretta L, et al. CpG and double-stranded RNA trigger human NK cells by toll-like receptors: induction of cytokine release and cytotoxicity against tumors and dendritic cells. Proc Natl Acad Sci U S A (2004) 101(27):10116–21. doi:10.1073/pnas.0403744101
110. Hart OM, Athie-Morales V, O’Connor GM, Gardiner CM. TLR7/8-mediated activation of human NK cells results in accessory cell-dependent IFN-gamma production. J Immunol (2005) 175(3):1636–42. doi:10.4049/jimmunol.175.3.1636
111. Souza-Fonseca-Guimaraes F, Adib-Conquy M, Cavaillon JM. Natural killer (NK) cells in antibacterial innate immunity: angels or devils? Mol Med (2012) 18:270–85. doi:10.2119/molmed.2011.00201
112. Marcenaro E, Ferranti B, Falco M, Moretta L, Moretta A. Human NK cells directly recognize Mycobacterium bovis via TLR2 and acquire the ability to kill monocyte-derived DC. Int Immunol (2008) 20(9):1155–67. doi:10.1093/intimm/dxn073
113. Bellora F, Castriconi R, Dondero A, Pessino A, Nencioni A, Liggieri G, et al. TLR activation of tumor-associated macrophages from ovarian cancer patients triggers cytolytic activity of NK cells. Eur J Immunol (2014) 44(6):1814–22. doi:10.1002/eji.201344130
114. Kim M, Osborne NR, Zeng W, Donaghy H, McKinnon K, Jackson DC, et al. Herpes simplex virus antigens directly activate NK cells via TLR2, thus facilitating their presentation to CD4 T lymphocytes. J Immunol (2012) 188(9):4158–70. doi:10.4049/jimmunol.1103450
115. Lu H, Dietsch GN, Matthews MA, Yang Y, Ghanekar S, Inokuma M, et al. VTX-2337 is a novel TLR8 agonist that activates NK cells and augments ADCC. Clin Cancer Res (2012) 18(2):499–509. doi:10.1158/1078-0432.CCR-11-1625
116. Mokuno Y, Matsuguchi T, Takano M, Nishimura H, Washizu J, Ogawa T, et al. Expression of toll-like receptor 2 on gamma delta T cells bearing invariant V gamma 6/V delta 1 induced by Escherichia coli infection in mice. J Immunol (2000) 165(2):931–40. doi:10.4049/jimmunol.165.2.931
117. Deetz CO, Hebbeler AM, Propp NA, Cairo C, Tikhonov I, Pauza CD. Gamma interferon secretion by human Vgamma2Vdelta2 T cells after stimulation with antibody against the T-cell receptor plus the toll-like receptor 2 agonist Pam3Cys. Infect Immun (2006) 74(8):4505–11. doi:10.1128/IAI.00088-06
118. Martin B, Hirota K, Cua DJ, Stockinger B, Veldhoen M. Interleukin-17-producing gammadelta T cells selectively expand in response to pathogen products and environmental signals. Immunity (2009) 31(2):321–30. doi:10.1016/j.immuni.2009.06.020
119. Schwacha MG, Daniel T. Up-regulation of cell surface toll-like receptors on circulating gammadelta T-cells following burn injury. Cytokine (2008) 44(3):328–34. doi:10.1016/j.cyto.2008.09.001
120. Schwacha MG, Rani M, Zhang Q, Nunez-Cantu O, Cap AP. Mitochondrial damage-associated molecular patterns activate gammadelta T-cells. Innate Immun (2014) 20(3):261–8. doi:10.1177/1753425913488969
121. Hedges JF, Lubick KJ, Jutila MA. Gamma delta T cells respond directly to pathogen-associated molecular patterns. J Immunol (2005) 174(10):6045–53. doi:10.4049/jimmunol.174.10.6045
122. Wesch D, Beetz S, Oberg HH, Marget M, Krengel K, Kabelitz D. Direct costimulatory effect of TLR3 ligand poly(I:C) on human gamma delta T lymphocytes. J Immunol (2006) 176(3):1348–54. doi:10.4049/jimmunol.176.3.1348
123. Zhang SY, Jouanguy E, Ugolini S, Smahi A, Elain G, Romero P, et al. TLR3 deficiency in patients with herpes simplex encephalitis. Science (2007) 317(5844):1522–7. doi:10.1126/science.1139522
124. Pietschmann K, Beetz S, Welte S, Martens I, Gruen J, Oberg HH, et al. Toll-like receptor expression and function in subsets of human gammadelta T lymphocytes. Scand J Immunol (2009) 70(3):245–55. doi:10.1111/j.1365-3083.2009.02290.x
125. Paleja B, Anand A, Chaukar D, D’Cruz A, Chiplunkar S. Decreased functional response to toll like receptor ligands in patients with oral cancer. Hum Immunol (2013) 74(8):927–36. doi:10.1016/j.humimm.2013.04.018
126. Shimura H, Nitahara A, Ito A, Tomiyama K, Ito M, Kawai K. Up-regulation of cell surface toll-like receptor 4-MD2 expression on dendritic epidermal T cells after the emigration from epidermis during cutaneous inflammation. J Dermatol Sci (2005) 37(2):101–10. doi:10.1016/j.jdermsci.2004.11.006
127. Cairns B, Maile R, Barnes CM, Frelinger JA, Meyer AA. Increased toll-like receptor 4 expression on T cells may be a mechanism for enhanced T cell response late after burn injury. J Trauma (2006) 61(2):293–8; discussion 298–9. doi:10.1097/01.ta.0000228969.46633.bb
128. Chow JC, Young DW, Golenbock DT, Christ WJ, Gusovsky F. Toll-like receptor-4 mediates lipopolysaccharide-induced signal transduction. J Biol Chem (1999) 274(16):10689–92. doi:10.1074/jbc.274.16.10689
129. Yang RB, Mark MR, Gray A, Huang A, Xie MH, Zhang M, et al. Toll-like receptor-2 mediates lipopolysaccharide-induced cellular signalling. Nature (1998) 395(6699):284–8. doi:10.1038/26239
130. Cui Y, Kang L, Cui L, He W. Human gammadelta T cell recognition of lipid A is predominately presented by CD1b or CD1c on dendritic cells. Biol Direct (2009) 4:47. doi:10.1186/1745-6150-4-47
131. Wang X, Sun R, Wei H, Tian Z. High-mobility group box 1 (HMGB1)-toll-like receptor (TLR)4-interleukin (IL)-23-IL-17A axis in drug-induced damage-associated lethal hepatitis: interaction of gammadelta T cells with macrophages. Hepatology (2013) 57(1):373–84. doi:10.1002/hep.25982
132. Peng G, Wang HY, Peng W, Kiniwa Y, Seo KH, Wang RF. Tumor-infiltrating gammadelta T cells suppress T and dendritic cell function via mechanisms controlled by a unique toll-like receptor signaling pathway. Immunity (2007) 27(2):334–48. doi:10.1016/j.immuni.2007.05.020
133. Choudhary A, Davodeau F, Moreau A, Peyrat MA, Bonneville M, Jotereau F. Selective lysis of autologous tumor cells by recurrent gamma delta tumor-infiltrating lymphocytes from renal carcinoma. J Immunol (1995) 154(8):3932–40.
134. Peng G, Guo Z, Kiniwa Y, Voo KS, Peng W, Fu T, et al. Toll-like receptor 8-mediated reversal of CD4+ regulatory T cell function. Science (2005) 309(5739):1380–4. doi:10.1126/science.1113401
135. Ye J, Ma C, Hsueh EC, Eickhoff CS, Zhang Y, Varvares MA, et al. Tumor-derived gammadelta regulatory T cells suppress innate and adaptive immunity through the induction of immunosenescence. J Immunol (2013) 190(5):2403–14. doi:10.4049/jimmunol.1202369
136. Ismaili J, Olislagers V, Poupot R, Fournié JJ, Goldman M. Human gamma delta T cells induce dendritic cell maturation. Clin Immunol (2002) 103(3 Pt 1):296–302. doi:10.1006/clim.2002.5218
137. Conti L, Casetti R, Cardone M, Varano B, Martino A, Belardelli F, et al. Reciprocal activating interaction between dendritic cells and pamidronate-stimulated gammadelta T cells: role of CD86 and inflammatory cytokines. J Immunol (2005) 174(1):252–60. doi:10.4049/jimmunol.174.1.252
138. Leslie DS, Vincent MS, Spada FM, Das H, Sugita M, Morita CT, et al. CD1-mediated gamma/delta T cell maturation of dendritic cells. J Exp Med (2002) 196(12):1575–84. doi:10.1084/jem.20021515
139. Shrestha N, Ida JA, Lubinski AS, Pallin M, Kaplan G, Haslett PA. Regulation of acquired immunity by gamma delta T-cell/dendritic-cell interactions. Ann N Y Acad Sci (2005) 1062:79–94. doi:10.1196/annals.1358.011
140. Fang H, Welte T, Zheng X, Chang GJ, Holbrook MR, Soong L, et al. Gammadelta T cells promote the maturation of dendritic cells during West Nile virus infection. FEMS Immunol Med Microbiol (2010) 59(1):71–80. doi:10.1111/j.1574-695X.2010.00663.x
141. Fowler DW, Copier J, Dalgleish AG, Bodman-Smith MD. Tripartite immune cell co-operation in the bacillus Calmette Guerin-induced activation of gammadelta T cells. Immunol Cell Biol (2013) 91(7):461–8. doi:10.1038/icb.2013.30
142. Dhar S, Chiplunkar SV. Lysis of aminobisphosphonate-sensitized MCF-7 breast tumor cells by Vgamma9Vdelta2 T cells. Cancer Immun (2010) 10:10.
143. D’Asaro M, La Mendola C, Di Liberto D, Orlando V, Todaro M, Spina M, et al. V gamma 9V delta 2 T lymphocytes efficiently recognize and kill zoledronate-sensitized, imatinib-sensitive, and imatinib-resistant chronic myelogenous leukemia cells. J Immunol (2010) 184(6):3260–8. doi:10.4049/jimmunol.0903454
144. Chargui J, Combaret V, Scaglione V, Iacono I, Péri V, Valteau-Couanet D, et al. Bromohydrin pyrophosphate-stimulated Vgamma9delta2 T cells expanded ex vivo from patients with poor-prognosis neuroblastoma lyse autologous primary tumor cells. J Immunother (2010) 33(6):591–8. doi:10.1097/CJI.0b013e3181dda207
145. Gogoi D, Chiplunkar SV. Targeting gamma delta T cells for cancer immunotherapy: bench to bedside. Indian J Med Res (2013) 138(5):755–61.
146. Weeratna RD, Makinen SR, McCluskie MJ, Davis HL. TLR agonists as vaccine adjuvants: comparison of CpG ODN and resiquimod (R-848). Vaccine (2005) 23(45):5263–70. doi:10.1016/j.vaccine.2005.06.024
147. Kalyan S, Wesch D, Kabelitz D. Aminobisphosphonates and toll-like receptor ligands: recruiting Vgamma9Vdelta2 T cells for the treatment of hematologic malignancy. Curr Med Chem (2011) 18(34):5206–16. doi:10.2174/092986711798184280
148. Paulos CM, Kaiser A, Wrzesinski C, Hinrichs CS, Cassard L, Boni A, et al. Toll-like receptors in tumor immunotherapy. Clin Cancer Res (2007) 13(18 Pt 1):5280–9. doi:10.1158/1078-0432.CCR-07-1378
149. Smits EL, Ponsaerts P, Berneman ZN, Van Tendeloo VF. The use of TLR7 and TLR8 ligands for the enhancement of cancer immunotherapy. Oncologist (2008) 13(8):859–75. doi:10.1634/theoncologist.2008-0097
150. Yu L, Chen S. Toll-like receptors expressed in tumor cells: targets for therapy. Cancer Immunol Immunother (2008) 57(9):1271–8. doi:10.1007/s00262-008-0459-8
151. Conroy H, Marshall NA, Mills KH. TLR ligand suppression or enhancement of Treg cells? A double-edged sword in immunity to tumours. Oncogene (2008) 27(2):168–80. doi:10.1038/sj.onc.1210910
152. Ridnour LA, Cheng RY, Switzer CH, Heinecke JL, Ambs S, Glynn S, et al. Molecular pathways: toll-like receptors in the tumor microenvironment – poor prognosis or new therapeutic opportunity. Clin Cancer Res (2013) 19(6):1340–6. doi:10.1158/1078-0432.CCR-12-0408
153. Inatsuka C, Yang Y, Gad E, Rastetter L, Disis ML, Lu H. Gamma delta T cells are activated by polysaccharide K (PSK) and contribute to the anti-tumor effect of PSK. Cancer Immunol Immunother (2013) 62(8):1335–45. doi:10.1007/s00262-013-1436-4
154. Hiromatsu K, Yoshikai Y, Matsuzaki G, Ohga S, Muramori K, Matsumoto K, et al. A protective role of gamma/delta T cells in primary infection with Listeria monocytogenes in mice. J Exp Med (1992) 175(1):49–56. doi:10.1084/jem.175.1.49
155. Hara T, Mizuno Y, Takaki K, Takada H, Akeda H, Aoki T, et al. Predominant activation and expansion of V gamma 9-bearing gamma delta T cells in vivo as well as in vitro in Salmonella infection. J Clin Invest (1992) 90(1):204–10. doi:10.1172/JCI115837
156. Kühl AA, Pawlowski NN, Grollich K, Loddenkemper C, Zeitz M, Hoffmann JC. Aggravation of intestinal inflammation by depletion/deficiency of gammadelta T cells in different types of IBD animal models. J Leukoc Biol (2007) 81(1):168–75. doi:10.1189/jlb.1105696
157. Poccia F, Agrati C, Martini F, Capobianchi MR, Wallace M, Malkovsky M. Antiviral reactivities of gammadelta T cells. Microbes Infect (2005) 7(3):518–28. doi:10.1016/j.micinf.2004.12.009
158. Inoue T, Yoshikai Y, Matsuzaki G, Nomoto K. Early appearing gamma/delta-bearing T cells during infection with Calmette Guerin bacillus. J Immunol (1991) 146(8):2754–62.
159. Beetz S, Wesch D, Marischen L, Welte S, Oberg HH, Kabelitz D. Innate immune functions of human gammadelta T cells. Immunobiology (2008) 213(3–4):173–82. doi:10.1016/j.imbio.2007.10.006
160. Zheng J, Liu Y, Lau YL, Tu W. Gammadelta-T cells: an unpolished sword in human anti-infection immunity. Cell Mol Immunol (2013) 10(1):50–7. doi:10.1038/cmi.2012.43
161. Ribot JC, Chaves-Ferreira M, d’Orey F, Wencker M, Gonçalves-Sousa N, Decalf J, et al. Cutting edge: adaptive versus innate receptor signals selectively control the pool sizes of murine IFN-gamma- or IL-17-producing gammadelta T cells upon infection. J Immunol (2010) 185(11):6421–5. doi:10.4049/jimmunol.1002283
162. Born WK, Zhang L, Nakayama M, Jin N, Chain JL, Huang Y, et al. Peptide antigens for gamma/delta T cells. Cell Mol Life Sci (2011) 68(14):2335–43. doi:10.1007/s00018-011-0697-3
163. Nedellec S, Bonneville M, Scotet E. Human Vgamma9Vdelta2 T cells: from signals to functions. Semin Immunol (2010) 22(4):199–206. doi:10.1016/j.smim.2010.04.004
164. Qin G, Mao H, Zheng J, Sia SF, Liu Y, Chan PL, et al. Phosphoantigen-expanded human gammadelta T cells display potent cytotoxicity against monocyte-derived macrophages infected with human and avian influenza viruses. J Infect Dis (2009) 200(6):858–65. doi:10.1086/605413
Keywords: immunotherapy, γδ T cells, toll like receptors, tumors, dendritic cells
Citation: Dar AA, Patil RS and Chiplunkar SV (2014) Insights into the relationship between toll like receptors and gamma delta T cell responses. Front. Immunol. 5:366. doi: 10.3389/fimmu.2014.00366
Received: 27 May 2014; Accepted: 15 July 2014;
Published online: 31 July 2014.
Edited by:
Anton G. Kutikhin, Russian Academy of Medical Sciences, RussiaReviewed by:
Isaias Glezer, Universidade Federal de São Paulo, BrazilRajesh Kumar Sharma, University of Louisville, USA
Copyright: © 2014 Dar, Patil and Chiplunkar. This is an open-access article distributed under the terms of the Creative Commons Attribution License (CC BY). The use, distribution or reproduction in other forums is permitted, provided the original author(s) or licensor are credited and that the original publication in this journal is cited, in accordance with accepted academic practice. No use, distribution or reproduction is permitted which does not comply with these terms.
*Correspondence: Shubhada Vivek Chiplunkar, Chiplunkar Laboratory, Advanced Centre for Treatment, Research and Education in Cancer (ACTREC), Tata Memorial Centre, Sector 22 Kharghar, Navi Mumbai, Maharashtra 410210, India e-mail:c2NoaXBsdW5rYXJAYWN0cmVjLmdvdi5pbg==