- Laboratory of Translational Immunology, Department of Hematology, University Medical Center Utrecht, Utrecht, Netherlands
The broad and potent tumor-reactivity of innate-like γδT cells makes them valuable additions to current cancer immunotherapeutic concepts based on adaptive immunity, such as monoclonal antibodies and αβT cells. However, clinical success using γδT cells to treat cancer has so far fallen short. Efforts of recent years have revealed a striking diversity in γδT cell functions and immunobiology, putting these cells forward as true “swiss army knives” of immunity. At the same time, however, this heterogeneity poses new challenges to the design of γδT cell-based therapeutic concepts and could explain their rather limited clinical efficacy in cancer patients. This review outlines the recent new insights into the different levels of γδT cell diversity, including the myriad of γδT cell-mediated immune functions, the diversity of specificities and affinities within the γδT cell repertoire, and the multitude of complex molecular requirements for γδT cell activation. A careful consideration of the diversity of antibodies and αβT cells has delivered great progress to their clinical success; addressing also the extraordinary diversity in γδT cells will therefore hold the key to more effective immunotherapeutic strategies with γδT cells as additional and valuable tools to battle cancer.
Immunotherapy to Treat Cancer: The Era is Now
Current treatment options to fight cancer heavily rely on pharmaceutical and radiological interventions that are accompanied by substantial off-tumor toxicity and lack of clinical efficacy. Cancer immunotherapy aims to capture the specificity and memory of the immune system and holds the promise of truly targeted treatment with durable clinical responses. Recent advances in clinical trials and the approval of more and more immunotherapeutic agents by international regulatory agencies have given the field considerable momentum, a fact that is mirrored by the announcement of cancer immunotherapy as the breakthrough of the year 2013 by Science (1).
So far, the vast majority of efforts aimed at utilizing the immune system to reject cancer have focused on components of adaptive immunity, including monoclonal antibodies and αβT cells. The human immune system can theoretically generate up to 1011 unique antibodies and some 1015 unique αβT cell receptors (αβTCRs) (2), and controlling this vast diversity in antigen specificity for targeted immune interventions has been a major challenge for clinical implementation. Although immunoglobulins are still used in clinical practice for untargeted protection against viral infections, such as in patients with general B-cell deficiencies, the real breakthrough in clinical immunotherapy came with mastering the genetic profile of defined monoclonal antibodies. Among the first therapeutic antibodies to directly target cancer were anti-CD20 (Rituxan or Rituximab) and anti-Her2 (Herceptin or Trastuzumab) antibodies to treat B cell leukemias and breast cancer, respectively. Treatment with these antibodies, recognizing one particular antigen with a defined affinity, has underscored the therapeutic potential of truly antigen-targeted immunotherapy, as impressive clinical benefit has been reported across studies covering the last decade (3, 4). The clinical success of these pioneering agents has in recent years led to the development and regulatory approval of additional antibodies to target various cancers (5), propelling antigen-specific antibody-based immunotherapy into mainstream cancer treatment. Similar to the evolution of clinical antibody treatment, first evidence for the anti-tumor potential of adoptively transferred αβT cells originated from the transfer of a very diverse immune population, the so called donor lymphocyte infusions, in the early 1990s, when allogeneic donor αβT cells that were infused in patients after allogeneic stem cell transplantation demonstrated potent anti-leukemia responses (6). By now, these data have been complemented by remarkable clinical results obtained with strategies that aim to mobilize the tumor-reactivity of autologous T cells in cancer patients, either by the adoptive transfer of ex vivo expanded tumor-infiltrating lymphocytes (TILs) (7, 8) or the infusion of monoclonal antibodies that stimulate T cell activity, such as the recently approved anti-CTLA4 antibody Ipilimumab (9, 10). Additionally, the genetic engineering of T cells with tumor-reactive αβTCRs (11, 12) or antibody-based chimeric antigen receptors (CARs) (13) has gained increasing interest in recent years, and the first clinical trials using adoptive transfer of such gene-modified T cells have demonstrated potent and lasting anti-tumor responses in selected patients (14–18).
Importantly, understanding the diversity of adaptive immune repertoires and utilizing very defined specificities for therapeutic interventions has so far been not only the success but also the downside of such therapies, resulting in highly personalized cancer care that depends on antibody-based strategies (including CAR-engineered T cells) with limited numbers of targetable tumor antigens and αβT cell products that are only clinically applicable to HLA-matched patient populations. Moreover, clinical anti-tumor efficacy of αβT cell-based approaches is so far mainly restricted to particularly immunogenic tumor types, such as melanoma. Thus, there is a compelling need to call to arms alternative immune components for novel cancer immunotherapeutic concepts.
γδT Cells: The Promising Outsiders
Unconventional γδT cells, a second lineage of T cells that express a unique somatically recombined γδTCR, possess unique features to confront the limitations of adaptive-based immunotherapeutic strategies. γδT cells are rapidly activated upon encounter of pathogen-derived antigens or self molecules that are upregulated on infected or stressed cells, resembling the activation of innate immune cells that sense molecular stress signatures (19, 20). Importantly, γδT cells are set apart from conventional αβT cells by the fact that activation of γδT cells does not depend on antigen presentation in the context of classical MHC molecules. A preferential usage of distinct TCR γ and δ chains, which together have the potential to form a tremendous repertoire of ~1020 uniquely recombined γδTCRs (2), has formed the basis for the identification of two major γδT cell subsets. γδT cells that carry Vγ9Vδ2+ TCRs are primarily found in peripheral blood, where they constitute a minor fraction of total T cells and respond to non-peptidic intermediates of the mevalonate pathway called phosphoantigens. Other γδT cells express mainly Vδ1+ or Vδ3+ chains paired with diverse γ chains (also called Vδ2neg γδT cells) and are highly enriched at mucosal sites and epithelial tissues. The effector mechanisms of γδT cells are highly similar to those of αβT cells and involve the secretion of high levels of cytokines and lysis of target cells by the release of granzymes and perforin and the engagement of FAS and TRAIL death receptors. Thus, by combining the potent effector functions of adaptive αβT cells with recognition modes that target unique classes of antigens in an innate-like manner, γδT cells are regarded as valuable sentinels that bridge innate and adaptive immunity.
Underlying the interest in γδT cells for use in cancer immunotherapy is a long-standing body of evidence indicating that γδT cells play important roles in tumor immunosurveillance. Human γδT cells display potent in vitro cytotoxicity toward a surprisingly large array of tumors, including cells derived from both solid and hematological origin (20–22). Importantly, γδT cells are also capable of targeting chemotherapy-resistant leukemic cells (23) and to kill leukemic and colon cancer stem cells (24) and Sebestyen and Kuball, unpublished observation). In vivo evidence for the non-redundant relevance of γδT cells in tumor immune surveillance stems from studies showing that γδT cell-deficient mice are more susceptible for developing cancer (25–27). Moreover, tumor-infiltrating γδT cells (γδTIL) have been observed in cancer patients with various cancers, and isolated γδTILs were shown to efficiently kill autologous tumors ex vivo, while leaving healthy cells unharmed (28–32). Important roles for γδT cells in tumor host defense are furthermore suggested by clinical data showing that high numbers of γδTILs in tumors of melanoma patients and elevated levels of circulating γδT cells in leukemia patients correlate with increased cancer-free survival (33, 34). Taken together, these studies have established a wealth of evidence for the broad tumor-targeting capabilities of γδT cells and have sparked great interest in their application in cancer immunotherapy.
Clinical Success of γδT Cells: Stuck in Diversity?
Given the broad recognition of unique classes of tumor antigens by γδT cells combined with their potent killing capacity, it is no surprise that γδT cells have been the focus of attempts to design novel cancer immunotherapeutic strategies. Of the two major γδT cell subsets, clinical trials conducted so far have exclusively focused on the stimulation of autologous Vγ9Vδ2+ γδT cells that were either activated in vivo using so-called aminobisphosphonate compounds that specifically activate Vγ9Vδ2+ γδT cells, or expanded ex vivo and reinfused into patients. Protocols for the in vivo mobilization of Vγ9Vδ2+ T cells generally involved repeated cycles of intravenous injection of synthetic phosphoantigen (35) or aminobisphosphonates such as pamidronate (36) or zoledronate (37–40), in combination with multiple IL2 injections per cycle. In trials that explored the adoptive transfer autologous Vγ9Vδ2+ T cells, patient PBMCs were cultured ex vivo for 2 weeks in the presence of aminobisphosphonates (41–43) or synthetic phosphoantigen (44, 45) in combination with IL2. Even though these conditions promoted the expansion of Vγ9Vδ2+ T cells, ex vivo expanded cell products contained rather low (on average 50–60%) and highly variable percentages of Vγ9Vδ2+ T cells, and no additional purification of Vγ9Vδ2+ T cells was performed prior to reinfusion into patients. Patients received repeated infusions of expanded cells, in some trials in combination with IL2. Treatment using γδT cells was generally found to be safe using both in vivo and ex vivo stimulation protocols, but clinical responses varied widely across trials and were generally limited, even in patients with cancers generally sensitive to immune responses such as renal cell carcinoma [reviewed in Ref. (46–48)]. Important limitations included (a) the need for a preselection of patients due to a wide variability in in vitro cytotoxicity of patient γδT cells against autologous tumor tissue (36, 41, 44), and (b) limited in vivo or ex vivo expansion potential of patient γδT cells (40, 41, 44, 45, 49). Moreover, anti-tumor efficacy of γδT cells showed only marginal improvement over standard treatment options (46). Thus, despite the fact that these trials have established the anti-tumor potential of γδT cells in cancer immunotherapy, current therapeutic strategies using these cells clearly suffer from major shortcomings that have so far prevented γδT cells to live up to their clinical promise.
A Remarkable Diversity Hampers Application of γδT Cells in Cancer Immunotherapy
Recent years have seen important progress in the understanding of γδT cell immunobiology and have uncovered a striking diversity in γδT cell functions and subsets. These new insights have important implications for the use of γδT cells in the treatment of cancer. To date, however, a profound appreciation of this γδT cell diversity has lacked from γδT cell-based clinical concepts and this is likely to contribute to the limited clinical results observed so far. At least three levels of γδT cell heterogeneity can be distinguished (Figure 1), including (a) a multitude of immune functions mediated by γδT cells, (b) a diverse γδTCR repertoire that, also for similar antigen-specificities, mediates different affinities, and (c) the complex and diverse molecular needs for target recognition within the same and across different γδT cell populations. A thorough consideration of these features will be of central importance to improving the clinical efficacy of γδT cells in treating cancer.
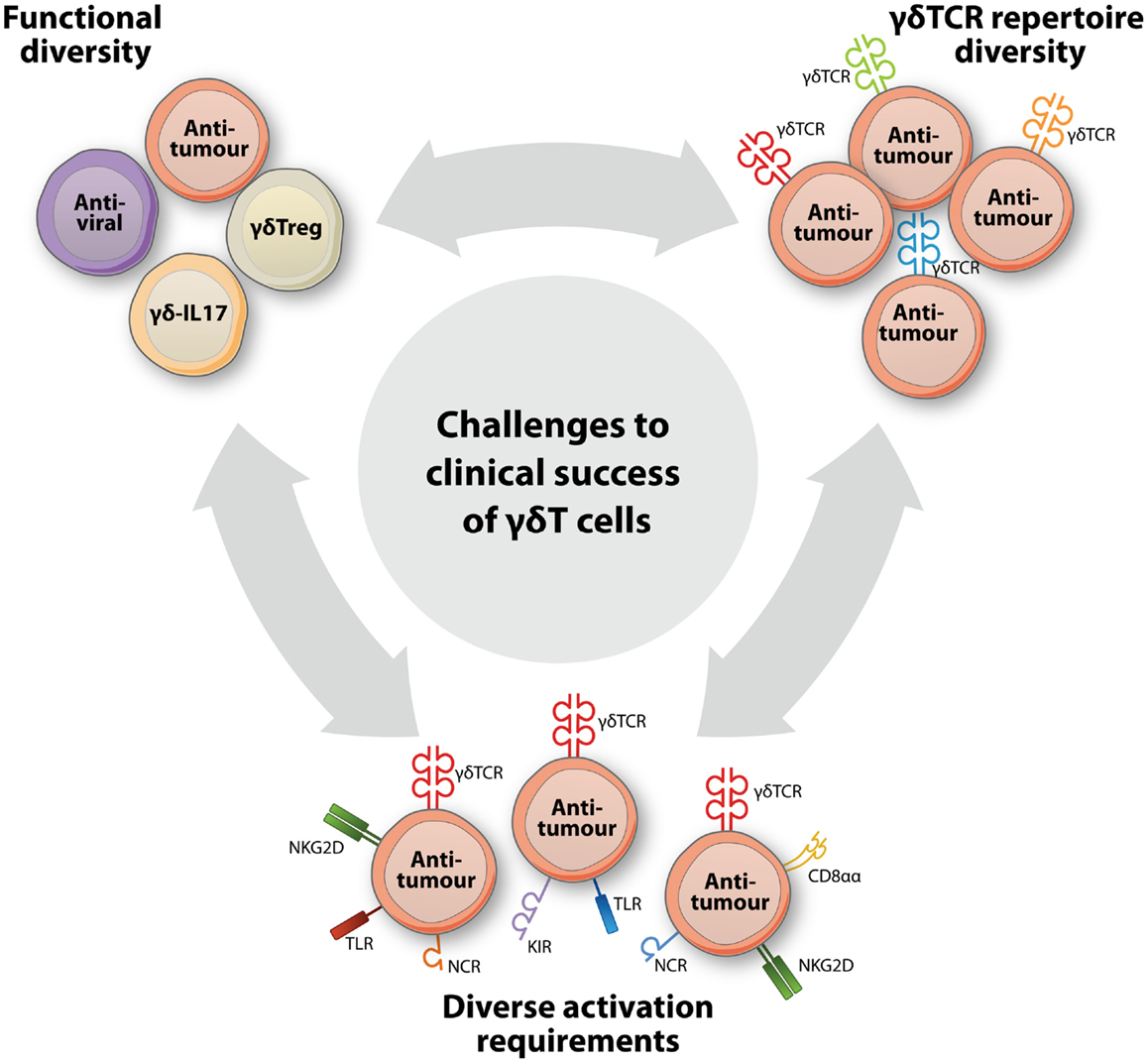
Figure 1. A broad functional and clonal diversity challenges the clinical success of γδT cells in cancer immunotherapy. New insights into γδT cell biology have pointed to at least three levels of diversity that each have a major impact on the design of successful γδT cell-based interventions to treat cancer. A striking functional diversity has come to light by the identification of new γδT cell subsets, such as regulatory (γδTreg) and IL17-producing (γδ-IL17) γδT cells, that now complement the well-established subsets with antiviral or anti-tumor functions. Within γδT cell populations that perform identical functions, another level of diversity is created by the extraordinarily diverse γδTCR repertoire that results in considerable variation in functional avidities of individual γδT cells. Additional diversity within and across γδT cell populations is represented by variable expression patterns of and complex activation requirements for additional immune receptors, including TLRs, CD8αα, and NK cell receptors such NKG2D, the natural cytotoxicity receptors (NCR) NKp30, NKp44, and NKp46, and activating and inhibitory killer cell immunoglobulin-like receptors (KIRs).
γδT Cell Functions: The More the Better?
γδT cells have, as discussed above, been attributed important and valuable functions in tumor immunosurveillance, but reactivity toward tumors is far from the only part that γδT cells play in immunity. By now, it is evident that γδT cells perform a plethora of functions that underline their involvement in diverse pathophysiological conditions other than cancer, including host defense against infectious pathogens such as bacteria, viruses, and parasites, the modulation of the activity of other immune cells, and promoting tissue regenerating after injury (20, 50).
Rapid expansions of γδT cells are observed in human beings infected with a variety of viruses or bacteria and γδT cells possess a potent capacity to directly kill infected cells (51). Moreover, a proportion of γδT cells contribute to pathogen clearance by the secretion of anti-microbial peptides such as granulysin and cathelicidin (52–54). Intriguingly, the recognition of pathogens may have important implications for γδT cell-mediated cytotoxicity against cancers, as subsets of γδT cells that respond to cytomegalovirus (CMV) infection have been reported to cross-recognize solid (55) as well as hematological (56) tumor cells in vitro. A role for virus-induced γδT cells in the protection from cancer in vivo is supported by observations that CMV infection in kidney transplant recipients was observed to associate with increased levels of γδT cells and concomitantly a reduced risk of developing cancer (57). Also in leukemia patients treated with hematopoietic stem cell transplantation, CMV infection associates with lower incidence of leukemic relapse after transplantation (58, 59) and work from our laboratory has demonstrated that tumor surveillance by CMV-induced γδT cells is likely to play a major role in this (56), emphasizing the clinical value of such dual-reactive γδT cells in immunotherapy.
In addition to their strong reactivity to a wide variety of tumors and pathogens, a valuable feature of γδT cells is their capability to broaden immune responses by recruiting and activating additional immune cell populations. For example, activated γδT cells have the potential to orchestrate adaptive αβT cell responses, both directly by functioning as antigen-presenting cells (60–62) as well as indirectly via the interaction with dendritic cells (56, 63, 64). In addition, γδT cells have been reported to secrete cytokines to provide B cell help in the production of antibodies (65, 66), to prime NK cells to kill tumor cells (67), to rapidly recruit neutrophils via the secretion of IL-17 (68, 69), and to synergize with monocytes to mount anti-microbial αβT cell responses (70). However, in addition to the immunostimulatory roles of γδT cells, their modulatory function may be of regulatory nature as well, suggesting complex implications of γδT cells in mediating broader immune responses. For example, depending on antigenic exposure, γδT cells may suppress rather than promote antibody production by B cells (71, 72). Similarly, γδT cells can strongly inhibit the proliferation of activated αβT cells (73, 74), and a suboptimal maturation of DCs by γδT cells (56) may induce tolerogenic rather than cytotoxic αβT cell responses. Importantly, human and mouse IL17-producing γδT cells have recently been demonstrated to facilitate tumor growth by recruiting myeloid-derived suppressor cells to tumor sites (75, 76). With the recent identification of bona fide Foxp3-expressing regulatory γδT cell subsets (77), it is thus becoming clear that, depending on their local or temporal cytokine milieu, activated γδT cells may suppress instead of activate local immune responses (78). Indeed, even though the presence of γδT cells may correlate with increased survival of cancer patients in some studies (see above), their infiltration into tumor sites may also associate with worse clinical outcome of patients due to a immunosuppressive phenotype of local γδT cells (79–81).
A Very Diverse γδTCR Repertoire Produces Receptors with Variable Anti-Tumor Affinities
Like αβTCRs and B cell receptors, γδTCRs are generated during T cell maturation through the somatic recombination of germline-encoded variable (V), diversity (D), and joining (J) gene segments. Despite the fact that the number of germline Vγ and Vδ genes is far more limited than the repertoire of Vα and Vβ genes, more extensive junctional diversification processes during TCR γ and δ chain rearrangement leads to a potential γδTCR repertoire that is roughly 105-fold larger than that of αβTCRs (2). Despite this extensive γδTCR repertoire, the diversity of antigens that are recognized by γδTCRs appears to be surprisingly limited. The vast majority of Vγ9Vδ2+ TCRs on circulating γδT cells are restricted to sensing elevated levels of phosphoantigens (22, 82), a process that has recently been demonstrated to involve the butyrophilin family member BTN3A1 (83, 84). Similarly, all antigens of Vδ2neg γδTCRs identified so far, including MICA/B (85), CD1 (86, 87), and EPCR (88), belong to the family of non-classical MHC homologs, although additional antigens are likely to still be identified and may include MHC-unrelated molecules.
An important question is why this rather narrow antigen restriction of γδT cells is confronted with such a broad γδTCR diversity, instead of a rather oligoclonal or invariant repertoire as expressed by for example NKT cells (89). One possible explanation may be that the extensive γδTCR repertoire of γδT cells allows an important fine-tuning of γδTCR-mediated target cell recognition. Indeed, we have shown recently that phosphoantigen-responsive Vγ9Vδ2+ γδT cell clones differed widely in their functional avidity toward tumor cells (90). γδTCR transfer and mutation experiments showed that this variability in the ability to respond to tumor cells was mediated primarily through diverse sequence compositions that dictate the affinities of individual clone-derived Vγ9Vδ2+ TCRs. A similar γδTCR-mediated heterogeneity in anti-tumor specificity can be observed in the Vδ2neg subset of γδT cells, as we recently demonstrated that individual Vδ1+ γδT cell clones display γδTCR-mediated reactivity against diverse arrays of tumor cells (56). Moreover, γδTCRs of other Vδ1+ clones were not involved in tumor recognition but mediated interactions with dendritic cells, demonstrating that a diverse γδTCR repertoire can mediate not only a fine-tuning of anti-tumor avidity but also different functions. Accordingly, diverse γδT cell functions that segregate with γδTCR composition have been observed for the human Vγ9Vδ2+ and Vδ2neg subsets, as Vγ9Vδ2 γδT cells have been generally ascribed potent cytotoxic effector functions, while Vδ2neg γδT cells rather have immunomodulatory roles (91, 92). However, these observations are contrasted by reports showing a superior tumor-homing and -killing capacity of Vδ2neg γδTILs over Vγ9Vδ2 γδTILs in some cancers (30, 93), further underlining the heterogeneous and context-dependent nature of both γδT cell subsets.
γδT Cell Activation: A Complex Interplay between Receptors
Alongside the γδTCR, γδT cells can be activated through a variety of activating and inhibitory NK receptors (48, 94) and toll-like receptors (TLR) (95), emphasizing the innate-like nature of these unconventional T cells. Depending on the pathophysiological context, these receptors can provide costimulation to γδTCR-mediated activation signals or can activate γδT cells independent of γδTCR triggering, adding yet another level of heterogeneity and complexity to γδT cell biology. The best-studied receptor with dualistic roles in γδT cell activation is NKG2D, a natural cytotoxicity receptor (NCR) that is expressed on NK cells, most γδT cells and CD8+ αβT cells. NKG2D recognizes the non-classical MHC homologs MICA/B and ULBPs, the expression of which is upregulated on many different tumors (96, 97). On Vγ9Vδ2+ γδT cells, NKG2D can amplify γδTCR-mediated effector functions in response to MICA/B-positive target cells (98, 99). In other cases, however, sole signaling through NKG2D has been proposed to be sufficient for activating γδT cells, without requiring γδTCR engagement (100, 101). However, as most of these studies have used TCR blocking antibodies and not receptor gene-transfer experiments, the impact of TCR affinity and signaling in NKG2D-triggered γδT cell activation might have been underestimated (Gründer and Kuball, unpublished observation). Factors that determine the directly stimulatory versus costimulatory function of NKG2D are not known, but may involve signaling by polymorphic receptors such as inhibitory NK receptors (100). Apart from serving as ligand for NKG2D, MICA/B is also recognized by selected Vδ1+ γδTCRs (85). In fact, overlapping binding epitopes for NKG2D and γδTCRs on MICA/B result in competitive binding of both receptors for MIC ligands, suggestive of complex, temporally regulated interactions of both receptors for MIC ligands (102). Similarly, engagement of the NCRs NKp30, NKp44, and NKp46 on γδT cells can be sufficient for eliciting anti-tumor cytotoxicity, but interestingly only after expression of these receptors on γδT cells has been induced via triggering of the γδTCR (103). Differential involvement of the γδTCR and additional receptors has also been reported in pathophysiological processes other than cancer, as work by us and others has demonstrated that reactivity of γδT cells against CMV-infected cells may involve γδTCR-dependent (55, 104) and -independent (56) pathways, suggesting multimodal pathogen-sensing mechanisms that may involve NK receptors (48).
Recently, we have found additional evidence for a complex interplay between receptors in the response of γδT cells against tumor cells by demonstrating that CD8αα, that serves as coreceptor for selected γδTCRs as reported by us recently (56), mediates γδTCR costimulation in a manner that depends on the particular tumor cell target (Scheper and Kuball, unpublished observation). Expression of CD8αα on T cells engineered to express a tumor-reactive γδTCR was a prerequisite for recognition of all tested tumor cell lines, but coexpression of signaling-deficient CD8α variants or mutants with single residue substitutions in the extracellular domain of CD8α alongside the γδTCR differentially impacted T cell reactivity toward the different tumor targets. Even though CD8αα+ γδT cells were first identified over 20 years ago, when CD8αα was found to be commonly expressed on Vδ1+ γδT cells in the intestine but not circulating Vγ9Vδ2+ T cells (105, 106), the functional implications of CD8αα expression on γδT cells have remained rather controversial. A number of studies have reported regulatory functions for CD8αα+ γδT cells, being capable of for example inhibiting inflammatory responses in celiac disease (107) but also to suppress αβT cell-mediated responses against tumor cells (80). On the other hand, and in line with our data (56), stimulated CD8αα+ γδT cells have been reported to be as capable as CD8αα−γδT cells of secreting high levels of Th1 cytokines such as IFNγ (108). Moreover, cytokines produced by CD8αα+ but not CD8αα− γδT cells have been implicated in the controlling of R5-tropic HIV replication and persistence (109). Thus, CD8αα+ γδT cells appear to perform diverse functions depending on the context in which they are activated.
Taken together, the emerging insights into the molecular requirements for γδT cell activation and the interplay between different receptors in this process have substantially furthered our understanding of the response of γδT cells against cancer cells, but also unveil substantial challenges to the design of uniform γδT cell-based strategies for cancer immunotherapy.
Successful Translation Using γδT Cells: Picking the Right Ones
Beyond doubt, the implications of the functional and clonal heterogeneity of γδT cells for their application in the treatment of cancer are substantial, and a failure to fully recognize this diversity in clinical concepts and trial designs is likely the most important contributing factor in the limited clinical results observed with γδT cells to date. Current clinical protocols based on the broad activation of unselected γδT cells are likely to induce γδT cell populations with diverse specificities, avidities, and functions, including regulatory. Consequently, high-avidity γδT cells with strong tumor-reactivity and a desired functional profile may represent only a relatively minor population of such cell products. In addition, stimulation of γδT cells using agents that primarily depend on strong γδTCR-mediated activation, such as the use of aminobisphosphonate and phoshoantigen compounds to expand Vγ9Vδ2+ γδT cells in trials pursued to date, most likely selects for γδT cells with low affinity Vγ9Vδ2+ γδTCRs and thus, low activity on primary tumor cells. Moreover, γδTCR-based activation strategies do not necessarily mobilize γδT cells that express a repertoire of NK receptors and TLRs required to potently respond to the multimolecular stress signature of tumor cells. Thus, the selection of optimally tumor-reactive γδT cell populations will likely be a critical parameter in the design of improved cancer immunotherapeutic concepts. In principal, this would favor strategies aimed at ex vivo rather than in vivo expansion of γδT cells, since the first allows a careful monitoring and culture-dependent skewing of γδT cell phenotype and functionality that is far more challenging to accomplish using in vivo stimulation protocols. With the clinical data available so far, it is difficult to corroborate this by comparing clinical responses observed in both types of trials, as studies using adoptive transfer of ex vivo generated γδT cells have so far relied on similar stimulation protocols (aminobisphosphonate or phosphoantigen in combination with IL-2) and the potential for extended in vitro manipulation for enhanced anti-tumor efficacy has not yet been investigated (41–45, 49, 110). Importantly, ex vivo manipulation of patient γδT cells could also include a valuable enrichment of tumor-specific γδT cells with high functional avidity, for instance using selection techniques based on the upregulation of activation markers or the production of cytokines such as IFNγ by γδT cells after in vitro coculture with autologous tumor cells. Nevertheless, γδTCR repertoires vary widely among individuals (111, 112), and generating sufficient numbers of γδT cells that recognize tumors with high avidity may therefore be challenging in certain patients. Similarly, NK receptor and TLR repertoires as well as CD8α expression levels differ considerably between γδT cell subsets (56, 103, 105, 113) and between individuals (95, 114, 115), putting additional constraints on the generation of γδT cell products potently capable of rejecting cancer.
To overcome the limitations of patient γδT cell repertoires, γδTCRs with broad tumor-specificity could be identified in vitro and genetically introduced into patient-derived immune cells. Recent work by our group has demonstrated that gene-transfer of tumor-specific Vγ9Vδ2+ and Vδ1+ γδTCRs can be used to efficiently reprogram conventional αβT cells to recognize a wide variety of tumor cells (56, 90, 97). By exploiting the abundance and superior proliferation potential of αβT cells, large numbers of autologous γδTCR-engineered T cells with defined tumor-specificity can be generated ex vivo and subsequently reinfused into cancer patients. In contrast to αβTCR gene-transfer strategies, introduced TCR γ and δ chains do not dimerize with endogenous αβTCR chains (97) and therefore do not lead to the formation of unwanted TCRs with unpredictable, and potentially dangerous, specificities. Moreover, since antigen recognition by γδTCRs does not depend on classical MHC molecules, well-characterized γδTCRs that mediate superior anti-tumor functional avidities can be applied to a broad patient population without the requirement for HLA matching. Additionally, transgenic expression of γδTCRs downregulates surface expression of endogenous αβTCR chains (56, 90, 97), enabling the use of engineered cell product even in an allogeneic “off-the-shelf” fashion. The ex vivo generation of γδTCR-engineered T cells furthermore allows additional manipulation of cell products, such as the selection of T cells with highest γδTCR expression levels or T cells which express beneficial TLRs or NK receptors. Importantly, such strategies can take advantage of the valuable lessons that have been learned from efforts to apply conventional αβT cells and their receptors in cancer immunotherapy, such as evidence for the effect of the differentiation status on in vivo persistence and function of clinical T cells (116). Our group has initiated the first clinical trial using γδTCR-gene-modified T cells to treat cancer patients (scheduled to start in 2015). Donor T cells engineered with a well-characterized tumor-reactive Vγ9Vδ2+ γδTCR (90) will be administered to leukemia patients after allogeneic stem cell transplantation as part of an engineered donor lymphocyte infusion. Ex vivo manipulations of gene-modified T cell products will include the depletion of cells that express only low levels of the clinical γδTCR and adapted culturing conditions to prevent terminal differentiation of engineered T cells before infusion into patients.
Closing Remarks
Even though γδT cells have traditionally been regarded as a homogeneous immune population, important advances in the understanding of γδT cell immunobiology have revealed a striking diversity in functionality and molecular activation modes. These new insights are generally met with great enthusiasm as they give acclaim to γδT cells for their non-redundant involvement in so many pathophysiological and homeostatic processes. However, this pleiotropy of γδT cells is likely an important factor that stifles the clinical success of their application to treat cancer. As for adaptive immune interventions, it may be absolutely mandatory to carefully consider the plethora of γδT cell functions, the diversity in γδTCR specificities and affinities as well as the complex requirements for proper γδT cell activation. At the end, such broadly tumor-reactive γδT cells might be highly effective only under very defined molecular and pathophysiological conditions and therefore less broadly applicable as initially thought, though a valuable addition to current therapeutic options. This new concept represents a major challenge in the design of next generation γδT cell-based immunotherapies, and clinical trials that incorporate these exciting insights will need to be pursued to confirm the clinical potential of γδT cells in the treatment of cancer.
Author Contributions
Wouter Scheper, Zsolt Sebestyen, and Jürgen Kuball wrote the manuscript; all authors agreed on the final manuscript.
Conflict of Interest Statement
The scheduled clinical trial using γδTCR-gene engineered T cells is supported by Miltenyi Biotec.
Acknowledgments
We thank Mariëtte Kranendonk for critical reading of the manuscript. This work was supported by grants of ZonMW 43400003, VIDI-ZonMW 917.11.337, LSBR 0902, AICR 10-736, KWF UU-2010-4669, UU-2013-6426, and Vrienden van het UMC Utrecht.
References
1. Couzin-Frankel J. Breakthrough of the year 2013. Cancer immunotherapy. Science (2013) 342(6165):1432–3. doi: 10.1126/science.342.6165.1432
2. Carding SR, Egan PJ. Gammadelta T cells: functional plasticity and heterogeneity. Nat Rev Immunol (2002) 2(5):336–45. doi:10.1038/nri797
Pubmed Abstract | Pubmed Full Text | CrossRef Full Text | Google Scholar
3. Bedard PL, Piccart-Gebhart MJ. Current paradigms for the use of HER2-targeted therapy in early-stage breast cancer. Clin Breast Cancer (2008) 8(Suppl 4):S157–65. doi:10.3816/CBC.2008.s.012
Pubmed Abstract | Pubmed Full Text | CrossRef Full Text | Google Scholar
4. Maloney DG. Anti-CD20 antibody therapy for B-cell lymphomas. N Engl J Med (2012) 366(21):2008–16. doi:10.1056/NEJMct1114348
5. Vacchelli E, Aranda F, Eggermont A, Galon J, Sautes-Fridman C, Zitvogel L, et al. Trial Watch: tumor-targeting monoclonal antibodies in cancer therapy. Oncoimmunology (2014) 3(1):e27048. doi:10.4161/onci.27048
Pubmed Abstract | Pubmed Full Text | CrossRef Full Text | Google Scholar
6. van den Brink MR, Porter DL, Giralt S, Lu SX, Jenq RR, Hanash A, et al. Relapse after allogeneic hematopoietic cell therapy. Biol Blood Marrow Transplant (2010) 16(1 Suppl):S138–45. doi:10.1016/j.bbmt.2009.10.023
Pubmed Abstract | Pubmed Full Text | CrossRef Full Text | Google Scholar
7. Dudley ME, Wunderlich JR, Robbins PF, Yang JC, Hwu P, Schwartzentruber DJ, et al. Cancer regression and autoimmunity in patients after clonal repopulation with antitumor lymphocytes. Science (2002) 298(5594):850–4. doi:10.1126/science.1076514
Pubmed Abstract | Pubmed Full Text | CrossRef Full Text | Google Scholar
8. Rosenberg SA, Restifo NP, Yang JC, Morgan RA, Dudley ME. Adoptive cell transfer: a clinical path to effective cancer immunotherapy. Nature Rev Cancer (2008) 8(4):299–308. doi:10.1038/nrc2355
Pubmed Abstract | Pubmed Full Text | CrossRef Full Text | Google Scholar
9. Hodi FS, O’Day SJ, McDermott DF, Weber RW, Sosman JA, Haanen JB, et al. Improved survival with ipilimumab in patients with metastatic melanoma. N Engl J Med (2010) 363(8):711–23. doi:10.1056/NEJMoa1003466
Pubmed Abstract | Pubmed Full Text | CrossRef Full Text | Google Scholar
10. Lipson EJ, Drake CG. Ipilimumab: an anti-CTLA-4 antibody for metastatic melanoma. Clin Cancer Res (2011) 17(22):6958–62. doi:10.1158/1078-0432.CCR-11-1595
11. Stauss HJ, Morris EC. Immunotherapy with gene-modified T cells: limiting side effects provides new challenges. Gene Ther (2013) 20(11):1029–32. doi:10.1038/gt.2013.34
Pubmed Abstract | Pubmed Full Text | CrossRef Full Text | Google Scholar
12. Marcu-Malina V, van Dorp S, Kuball J. Re-targeting T-cells against cancer by gene-transfer of tumor-reactive receptors. Expert Opin Biol Ther (2009) 9(5):579–91. doi:10.1517/14712590902887018
Pubmed Abstract | Pubmed Full Text | CrossRef Full Text | Google Scholar
13. Maus MV, Grupp SA, Porter DL, June CH. Antibody-modified T cells: CARs take the front seat for hematologic malignancies. Blood (2014) 123(17):2625–35. doi:10.1182/blood-2013-11-492231
Pubmed Abstract | Pubmed Full Text | CrossRef Full Text | Google Scholar
14. Morgan RA, Dudley ME, Wunderlich JR, Hughes MS, Yang JC, Sherry RM, et al. Cancer regression in patients after transfer of genetically engineered lymphocytes. Science (2006) 314(5796):126–9. Epub 2006/09/02., doi:10.1126/science.1129003
Pubmed Abstract | Pubmed Full Text | CrossRef Full Text | Google Scholar
15. Robbins PF, Morgan RA, Feldman SA, Yang JC, Sherry RM, Dudley ME, et al. Tumor regression in patients with metastatic synovial cell sarcoma and melanoma using genetically engineered lymphocytes reactive with NY-ESO-1. J Clin Oncol (2011) 29(7):917–24. doi:10.1200/JCO.2010.32.2537
Pubmed Abstract | Pubmed Full Text | CrossRef Full Text | Google Scholar
16. Porter DL, Levine BL, Kalos M, Bagg A, June CH. Chimeric antigen receptor-modified T cells in chronic lymphoid leukemia. N Engl J Med (2011) 365(8):725–33. doi:10.1056/NEJMoa1103849
Pubmed Abstract | Pubmed Full Text | CrossRef Full Text | Google Scholar
17. Kochenderfer JN, Wilson WH, Janik JE, Dudley ME, Stetler-Stevenson M, Feldman SA, et al. Eradication of B-lineage cells and regression of lymphoma in a patient treated with autologous T cells genetically engineered to recognize CD19. Blood (2010) 116(20):4099–102. doi:10.1182/blood-2010-04-281931
Pubmed Abstract | Pubmed Full Text | CrossRef Full Text | Google Scholar
18. Grupp SA, Kalos M, Barrett D, Aplenc R, Porter DL, Rheingold SR, et al. Chimeric antigen receptor-modified T cells for acute lymphoid leukemia. N Engl J Med (2013) 368(16):1509–18. doi:10.1056/NEJMoa1215134
Pubmed Abstract | Pubmed Full Text | CrossRef Full Text | Google Scholar
19. Vantourout P, Hayday A. Six-of-the-best: unique contributions of gammadelta T cells to immunology. Nat Rev Immunol (2013) 13(2):88–100. doi:10.1038/nri3384
Pubmed Abstract | Pubmed Full Text | CrossRef Full Text | Google Scholar
20. Bonneville M, O’Brien RL, Born WK. Gammadelta T cell effector functions: a blend of innate programming and acquired plasticity. Nat Rev Immunol (2010) 10(7):467–78. doi:10.1038/nri2781
Pubmed Abstract | Pubmed Full Text | CrossRef Full Text | Google Scholar
21. Hannani D, Ma Y, Yamazaki T, Dechanet-Merville J, Kroemer G, Zitvogel L. Harnessing gammadelta T cells in anticancer immunotherapy. Trends Immunol (2012) 33(5):199–206. doi:10.1016/j.it.2012.01.006
22. Morita CT, Jin C, Sarikonda G, Wang H. Nonpeptide antigens, presentation mechanisms, and immunological memory of human Vgamma2Vdelta2 T cells: discriminating friend from foe through the recognition of prenyl pyrophosphate antigens. Immunol Rev (2007) 215:59–76. doi:10.1111/j.1600-065X.2006.00479.x
Pubmed Abstract | Pubmed Full Text | CrossRef Full Text | Google Scholar
23. D’Asaro M, La Mendola C, Di Liberto D, Orlando V, Todaro M, Spina M, et al. V gamma 9V delta 2 T lymphocytes efficiently recognize and kill zoledronate-sensitized, imatinib-sensitive, and imatinib-resistant chronic myelogenous leukemia cells. J Immunol (2010) 184(6):3260–8. doi:10.4049/jimmunol.0903454
Pubmed Abstract | Pubmed Full Text | CrossRef Full Text | Google Scholar
24. Todaro M, D’Asaro M, Caccamo N, Iovino F, Francipane MG, Meraviglia S, et al. Efficient killing of human colon cancer stem cells by gammadelta T lymphocytes. J Immunol (2009) 182(11):7287–96. doi:10.4049/jimmunol.0804288
Pubmed Abstract | Pubmed Full Text | CrossRef Full Text | Google Scholar
25. Girardi M, Oppenheim DE, Steele CR, Lewis JM, Glusac E, Filler R, et al. Regulation of cutaneous malignancy by gammadelta T cells. Science (2001) 294(5542):605–9. doi:10.1126/science.1063916
Pubmed Abstract | Pubmed Full Text | CrossRef Full Text | Google Scholar
26. Gao Y, Yang W, Pan M, Scully E, Girardi M, Augenlicht LH, et al. Gamma delta T cells provide an early source of interferon gamma in tumor immunity. J Exp Med (2003) 198(3):433–42. doi:10.1084/jem.20030584
Pubmed Abstract | Pubmed Full Text | CrossRef Full Text | Google Scholar
27. Street SE, Hayakawa Y, Zhan Y, Lew AM, MacGregor D, Jamieson AM, et al. Innate immune surveillance of spontaneous B cell lymphomas by natural killer cells and gammadelta T cells. J Exp Med (2004) 199(6):879–84. doi:10.1084/jem.20031981
Pubmed Abstract | Pubmed Full Text | CrossRef Full Text | Google Scholar
28. Groh V, Rhinehart R, Secrist H, Bauer S, Grabstein KH, Spies T. Broad tumor-associated expression and recognition by tumor-derived gamma delta T cells of MICA and MICB. Proc Natl Acad Sci USA (1999) 96(12):6879–84. doi:10.1073/pnas.96.12.6879
Pubmed Abstract | Pubmed Full Text | CrossRef Full Text | Google Scholar
29. Maeurer MJ, Martin D, Walter W, Liu K, Zitvogel L, Halusczcak K, et al. Human intestinal Vdelta1+ lymphocytes recognize tumor cells of epithelial origin. J Exp Med (1996) 183(4):1681–96. doi:10.1084/jem.183.4.1681
Pubmed Abstract | Pubmed Full Text | CrossRef Full Text | Google Scholar
30. Choudhary A, Davodeau F, Moreau A, Peyrat MA, Bonneville M, Jotereau F. Selective lysis of autologous tumor cells by recurrent gamma delta tumor-infiltrating lymphocytes from renal carcinoma. J Immunol (1995) 154(8):3932–40.
31. Ferrarini M, Heltai S, Pupa SM, Mernard S, Zocchi R. Killing of laminin receptor-positive human lung cancers by tumor infiltrating lymphocytes bearing gammadelta(+) t-cell receptors. J Natl Cancer Inst (1996) 88(7):436–41. doi:10.1093/jnci/88.7.436
Pubmed Abstract | Pubmed Full Text | CrossRef Full Text | Google Scholar
32. Viey E, Fromont G, Escudier B, Morel Y, Da Rocha S, Chouaib S, et al. Phosphostim-activated gamma delta T cells kill autologous metastatic renal cell carcinoma. J Immunol (2005) 174(3):1338–47. doi:10.4049/jimmunol.174.3.1338
Pubmed Abstract | Pubmed Full Text | CrossRef Full Text | Google Scholar
33. Bialasiewicz AA, Ma JX, Richard G. Alpha/beta- and gamma/delta TCR(+) lymphocyte infiltration in necrotising choroidal melanomas. Br J Ophthalmol (1999) 83(9):1069–73. doi:10.1136/bjo.83.9.1069
Pubmed Abstract | Pubmed Full Text | CrossRef Full Text | Google Scholar
34. Godder KT, Henslee-Downey PJ, Mehta J, Park BS, Chiang KY, Abhyankar S, et al. Long term disease-free survival in acute leukemia patients recovering with increased gammadelta T cells after partially mismatched related donor bone marrow transplantation. Bone Marrow Transplant (2007) 39(12):751–7. doi:10.1038/sj.bmt.1705650
Pubmed Abstract | Pubmed Full Text | CrossRef Full Text | Google Scholar
35. Bennouna J, Levy V, Sicard H, Senellart H, Audrain M, Hiret S, et al. Phase I study of bromohydrin pyrophosphate (BrHPP, IPH 1101), a Vgamma9Vdelta2 T lymphocyte agonist in patients with solid tumors. Cancer Immunol Immunother (2010) 59(10):1521–30. doi:10.1007/s00262-010-0879-0
Pubmed Abstract | Pubmed Full Text | CrossRef Full Text | Google Scholar
36. Wilhelm M, Kunzmann V, Eckstein S, Reimer P, Weissinger F, Ruediger T, et al. Gammadelta T cells for immune therapy of patients with lymphoid malignancies. Blood (2003) 102(1):200–6. doi:10.1182/blood-2002-12-3665
Pubmed Abstract | Pubmed Full Text | CrossRef Full Text | Google Scholar
37. Dieli F, Gebbia N, Poccia F, Caccamo N, Montesano C, Fulfaro F, et al. Induction of gammadelta T-lymphocyte effector functions by bisphosphonate zoledronic acid in cancer patients in vivo. Blood (2003) 102(6):2310–1. doi:10.1182/blood-2003-05-1655
38. Dieli F, Vermijlen D, Fulfaro F, Caccamo N, Meraviglia S, Cicero G, et al. Targeting human {gamma}delta} T cells with zoledronate and interleukin-2 for immunotherapy of hormone-refractory prostate cancer. Cancer Res (2007) 67(15):7450–7. doi:10.1158/0008-5472.CAN-07-0199
Pubmed Abstract | Pubmed Full Text | CrossRef Full Text | Google Scholar
39. Meraviglia S, Eberl M, Vermijlen D, Todaro M, Buccheri S, Cicero G, et al. In vivo manipulation of Vgamma9Vdelta2 T cells with zoledronate and low-dose interleukin-2 for immunotherapy of advanced breast cancer patients. Clin Exp Immunol (2010) 161(2):290–7. doi:10.1111/j.1365-2249.2010.04167.x
Pubmed Abstract | Pubmed Full Text | CrossRef Full Text | Google Scholar
40. Lang JM, Kaikobad MR, Wallace M, Staab MJ, Horvath DL, Wilding G, et al. Pilot trial of interleukin-2 and zoledronic acid to augment gammadelta T cells as treatment for patients with refractory renal cell carcinoma. Cancer Immunol Immunother (2011) 60(10):1447–60. doi:10.1007/s00262-011-1049-8
Pubmed Abstract | Pubmed Full Text | CrossRef Full Text | Google Scholar
41. Noguchi A, Kaneko T, Kamigaki T, Fujimoto K, Ozawa M, Saito M, et al. Zoledronate-activated Vgamma9gammadelta T cell-based immunotherapy is feasible and restores the impairment of gammadelta T cells in patients with solid tumors. Cytotherapy (2011) 13(1):92–7. doi:10.3109/14653249.2010.515581
Pubmed Abstract | Pubmed Full Text | CrossRef Full Text | Google Scholar
42. Nakajima J, Murakawa T, Fukami T, Goto S, Kaneko T, Yoshida Y, et al. A phase I study of adoptive immunotherapy for recurrent non-small-cell lung cancer patients with autologous gammadelta T cells. Eur J Cardiothorac Surg (2010) 37(5):1191–7. doi:10.1016/j.ejcts.2009.11.051
Pubmed Abstract | Pubmed Full Text | CrossRef Full Text | Google Scholar
43. Nicol AJ, Tokuyama H, Mattarollo SR, Hagi T, Suzuki K, Yokokawa K, et al. Clinical evaluation of autologous gamma delta T cell-based immunotherapy for metastatic solid tumours. Br J Cancer (2011) 105(6):778–86. doi:10.1038/bjc.2011.293
Pubmed Abstract | Pubmed Full Text | CrossRef Full Text | Google Scholar
44. Bennouna J, Bompas E, Neidhardt EM, Rolland F, Philip I, Galea C, et al. Phase-I study of Innacell gammadelta, an autologous cell-therapy product highly enriched in gamma9delta2 T lymphocytes, in combination with IL-2, in patients with metastatic renal cell carcinoma. Cancer Immunol Immunother (2008) 57(11):1599–609. doi:10.1007/s00262-008-0491-8
Pubmed Abstract | Pubmed Full Text | CrossRef Full Text | Google Scholar
45. Kobayashi H, Tanaka Y, Yagi J, Osaka Y, Nakazawa H, Uchiyama T, et al. Safety profile and anti-tumor effects of adoptive immunotherapy using gamma-delta T cells against advanced renal cell carcinoma: a pilot study. Cancer Immunol Immunother (2007) 56(4):469–76. doi:10.1007/s00262-006-0199-6
Pubmed Abstract | Pubmed Full Text | CrossRef Full Text | Google Scholar
46. Fisher JP, Heuijerjans J, Yan M, Gustafsson K, Anderson J. Gammadelta T cells for cancer immunotherapy: a systematic review of clinical trials. Oncoimmunology (2014) 3(1):e27572. doi:10.4161/onci.27572
Pubmed Abstract | Pubmed Full Text | CrossRef Full Text | Google Scholar
47. Fournie JJ, Sicard H, Poupot M, Bezombes C, Blanc A, Romagne F, et al. What lessons can be learned from gammadelta T cell-based cancer immunotherapy trials? Cell Mol Immunol (2013) 10(1):35–41. doi:10.1038/cmi.2012.39
Pubmed Abstract | Pubmed Full Text | CrossRef Full Text | Google Scholar
48. Scheper W, Grunder C, Straetemans T, Sebestyen Z, Kuball J. Hunting for clinical translation with innate-like immune cells and their receptors. Leukemia (2014) 28(6):1181–90. doi:10.1038/leu.2013.378
Pubmed Abstract | Pubmed Full Text | CrossRef Full Text | Google Scholar
49. Kondo M, Sakuta K, Noguchi A, Ariyoshi N, Sato K, Sato S, et al. Zoledronate facilitates large-scale ex vivo expansion of functional gammadelta T cells from cancer patients for use in adoptive immunotherapy. Cytotherapy (2008) 10(8):842–56. doi:10.1080/14653240802419328
Pubmed Abstract | Pubmed Full Text | CrossRef Full Text | Google Scholar
50. Hayday AC. Gammadelta T cells and the lymphoid stress-surveillance response. Immunity (2009) 31(2):184–96. doi:10.1016/j.immuni.2009.08.006
Pubmed Abstract | Pubmed Full Text | CrossRef Full Text | Google Scholar
51. Zheng J, Liu Y, Lau YL, Tu W. Gammadelta-T cells: an unpolished sword in human anti-infection immunity. Cell Mol Immunol (2013) 10(1):50–7. doi:10.1038/cmi.2012.43
Pubmed Abstract | Pubmed Full Text | CrossRef Full Text | Google Scholar
52. Agerberth B, Charo J, Werr J, Olsson B, Idali F, Lindbom L, et al. The human antimicrobial and chemotactic peptides LL-37 and alpha-defensins are expressed by specific lymphocyte and monocyte populations. Blood (2000) 96(9):3086–93.
53. Dieli F, Troye-Blomberg M, Ivanyi J, Fournie JJ, Krensky AM, Bonneville M, et al. Granulysin-dependent killing of intracellular and extracellular Mycobacterium tuberculosis by Vgamma9/Vdelta2 T lymphocytes. J Infect Dis (2001) 184(8):1082–5. doi:10.1086/323600
Pubmed Abstract | Pubmed Full Text | CrossRef Full Text | Google Scholar
54. Dudal S, Turriere C, Bessoles S, Fontes P, Sanchez F, Liautard J, et al. Release of LL-37 by activated human Vgamma9Vdelta2 T cells: a microbicidal weapon against Brucella suis. J Immunol (2006) 177(8):5533–9. doi:10.4049/jimmunol.177.8.5533
Pubmed Abstract | Pubmed Full Text | CrossRef Full Text | Google Scholar
55. Halary F, Pitard V, Dlubek D, Krzysiek R, de la Salle H, Merville P, et al. Shared reactivity of V{delta}2(neg) {gamma}{delta} T cells against cytomegalovirus-infected cells and tumor intestinal epithelial cells. J Exp Med (2005) 201(10):1567–78. doi:10.1084/jem.20041851
Pubmed Abstract | Pubmed Full Text | CrossRef Full Text | Google Scholar
56. Scheper W, van Dorp S, Kersting S, Pietersma F, Lindemans C, Hol S, et al. gammadeltaT cells elicited by CMV reactivation after allo-SCT cross-recognize CMV and leukemia. Leukemia (2013) 27(6):1328–38. doi:10.1038/leu.2012.374
Pubmed Abstract | Pubmed Full Text | CrossRef Full Text | Google Scholar
57. Couzi L, Levaillant Y, Jamai A, Pitard V, Lassalle R, Martin K, et al. Cytomegalovirus-induced gammadelta T cells associate with reduced cancer risk after kidney transplantation. J Am Soc Nephrol (2010) 21(1):181–8. doi:10.1681/ASN.2008101072
Pubmed Abstract | Pubmed Full Text | CrossRef Full Text | Google Scholar
58. Elmaagacli AH, Steckel NK, Koldehoff M, Hegerfeldt Y, Trenschel R, Ditschkowski M, et al. Early human cytomegalovirus replication after transplantation is associated with a decreased relapse risk: evidence for a putative virus-versus-leukemia effect in acute myeloid leukemia patients. Blood (2011) 118(5):1402–12. doi:10.1182/blood-2010-08-304121
Pubmed Abstract | Pubmed Full Text | CrossRef Full Text | Google Scholar
59. Green ML, Leisenring WM, Xie H, Walter RB, Mielcarek M, Sandmaier BM, et al. CMV reactivation after allogeneic HCT and relapse risk: evidence for early protection in acute myeloid leukemia. Blood (2013) 122(7):1316–24. doi:10.1182/blood-2013-02-487074
Pubmed Abstract | Pubmed Full Text | CrossRef Full Text | Google Scholar
60. Brandes M, Willimann K, Bioley G, Levy N, Eberl M, Luo M, et al. Cross-presenting human gammadelta T cells induce robust CD8+ alphabeta T cell responses. Proc Natl Acad Sci U S A (2009) 106(7):2307–12. doi:10.1073/pnas.0810059106
Pubmed Abstract | Pubmed Full Text | CrossRef Full Text | Google Scholar
61. Brandes M, Willimann K, Moser B. Professional antigen-presentation function by human gammadelta T Cells. Science (2005) 309(5732):264–8. doi:10.1126/science.1110267
62. Himoudi N, Morgenstern DA, Yan M, Vernay B, Saraiva L, Wu Y, et al. Human gammadelta T lymphocytes are licensed for professional antigen presentation by interaction with opsonized target cells. J Immunol (2012) 188(4):1708–16. doi:10.4049/jimmunol.1102654
Pubmed Abstract | Pubmed Full Text | CrossRef Full Text | Google Scholar
63. Leslie DS, Vincent MS, Spada FM, Das H, Sugita M, Morita CT, et al. CD1-mediated gamma/delta T cell maturation of dendritic cells. J Exp Med (2002) 196(12):1575–84. doi:10.1084/jem.20021515
Pubmed Abstract | Pubmed Full Text | CrossRef Full Text | Google Scholar
64. Conti L, Casetti R, Cardone M, Varano B, Martino A, Belardelli F, et al. Reciprocal activating interaction between dendritic cells and pamidronate-stimulated gammadelta T cells: role of CD86 and inflammatory cytokines. J Immunol (2005) 174(1):252–60. doi:10.4049/jimmunol.174.1.252
Pubmed Abstract | Pubmed Full Text | CrossRef Full Text | Google Scholar
65. Zheng B, Marinova E, Han J, Tan TH, Han S. Cutting edge: gamma delta T cells provide help to B cells with altered clonotypes and are capable of inducing Ig gene hypermutation. J Immunol (2003) 171(10):4979–83. doi:10.4049/jimmunol.171.10.4979
Pubmed Abstract | Pubmed Full Text | CrossRef Full Text | Google Scholar
66. Caccamo N, Battistini L, Bonneville M, Poccia F, Fournie JJ, Meraviglia S, et al. CXCR5 identifies a subset of Vgamma9Vdelta2 T cells which secrete IL-4 and IL-10 and help B cells for antibody production. J Immunol (2006) 177(8):5290–5. doi:10.4049/jimmunol.177.8.5290
Pubmed Abstract | Pubmed Full Text | CrossRef Full Text | Google Scholar
67. Maniar A, Zhang X, Lin W, Gastman BR, Pauza CD, Strome SE, et al. Human gammadelta T lymphocytes induce robust NK cell-mediated antitumor cytotoxicity through CD137 engagement. Blood (2010) 116(10):1726–33. doi:10.1182/blood-2009-07-234211
Pubmed Abstract | Pubmed Full Text | CrossRef Full Text | Google Scholar
68. Caccamo N, La Mendola C, Orlando V, Meraviglia S, Todaro M, Stassi G, et al. Differentiation, phenotype, and function of interleukin-17-producing human Vgamma9Vdelta2 T cells. Blood (2011) 118(1):129–38. doi:10.1182/blood-2011-01-331298
Pubmed Abstract | Pubmed Full Text | CrossRef Full Text | Google Scholar
69. Chien YH, Zeng X, Prinz I. The natural and the inducible: interleukin (IL)-17-producing gammadelta T cells. Trends Immunol (2013) 34(4):151–4. doi:10.1016/j.it.2012.11.004
Pubmed Abstract | Pubmed Full Text | CrossRef Full Text | Google Scholar
70. Eberl M, Roberts GW, Meuter S, Williams JD, Topley N, Moser B. A rapid crosstalk of human gammadelta T cells and monocytes drives the acute inflammation in bacterial infections. PLoS Pathog (2009) 5(2):e1000308. doi:10.1371/journal.ppat.1000308
Pubmed Abstract | Pubmed Full Text | CrossRef Full Text | Google Scholar
71. McMenamin C, Pimm C, McKersey M, Holt PG. Regulation of IgE responses to inhaled antigen in mice by antigen-specific gamma delta T cells. Science (1994) 265(5180):1869–71. doi:10.1126/science.7916481
Pubmed Abstract | Pubmed Full Text | CrossRef Full Text | Google Scholar
72. Huang Y, Jin N, Roark CL, Aydintug MK, Wands JM, Huang H, et al. The influence of IgE-enhancing and IgE-suppressive gammadelta T cells changes with exposure to inhaled ovalbumin. J Immunol (2009) 183(2):849–55. doi:10.4049/jimmunol.0804104
Pubmed Abstract | Pubmed Full Text | CrossRef Full Text | Google Scholar
73. Kuhl AA, Pawlowski NN, Grollich K, Blessenohl M, Westermann J, Zeitz M, et al. Human peripheral gammadelta T cells possess regulatory potential. Immunology (2009) 128(4):580–8. doi:10.1111/j.1365-2567.2009.03162.x
Pubmed Abstract | Pubmed Full Text | CrossRef Full Text | Google Scholar
74. Traxlmayr MW, Wesch D, Dohnal AM, Funovics P, Fischer MB, Kabelitz D, et al. Immune suppression by gammadelta T-cells as a potential regulatory mechanism after cancer vaccination with IL-12 secreting dendritic cells. J Immunother (2010) 33(1):40–52. doi:10.1097/CJI.0b013e3181b51447
Pubmed Abstract | Pubmed Full Text | CrossRef Full Text | Google Scholar
75. Ma S, Cheng Q, Cai Y, Gong H, Wu Y, Yu X, et al. IL-17A produced by gammadelta T cells promotes tumor growth in hepatocellular carcinoma. Cancer Res (2014) 74(7):1969–82. doi:10.1158/0008-5472.CAN-13-2534
Pubmed Abstract | Pubmed Full Text | CrossRef Full Text | Google Scholar
76. Wu P, Wu D, Ni C, Ye J, Chen W, Hu G, et al. GammadeltaT17 cells promote the accumulation and expansion of myeloid-derived suppressor cells in human colorectal cancer. Immunity (2014) 40(5):785–800. doi:10.1016/j.immuni.2014.03.013
Pubmed Abstract | Pubmed Full Text | CrossRef Full Text | Google Scholar
77. Casetti R, Agrati C, Wallace M, Sacchi A, Martini F, Martino A, et al. Cutting edge: TGF-beta1 and IL-15 Induce FOXP3+ gammadelta regulatory T cells in the presence of antigen stimulation. J Immunol (2009) 183(6):3574–7. doi:10.4049/jimmunol.0901334
Pubmed Abstract | Pubmed Full Text | CrossRef Full Text | Google Scholar
78. Kabelitz D, Peters C, Wesch D, Oberg HH. Regulatory functions of gammadelta T cells. Int Immunopharmacol (2013) 16(3):382–7. doi:10.1016/j.intimp.2013.01.022
79. Ma C, Zhang Q, Ye J, Wang F, Zhang Y, Wevers E, et al. Tumor-infiltrating gammadelta T lymphocytes predict clinical outcome in human breast cancer. J Immunol (2012) 189(10):5029–36. doi:10.4049/jimmunol.1201892
Pubmed Abstract | Pubmed Full Text | CrossRef Full Text | Google Scholar
80. Peng G, Wang HY, Peng W, Kiniwa Y, Seo KH, Wang RF. Tumor-infiltrating gammadelta T cells suppress T and dendritic cell function via mechanisms controlled by a unique toll-like receptor signaling pathway. Immunity (2007) 27(2):334–48. doi:10.1016/j.immuni.2007.05.020
Pubmed Abstract | Pubmed Full Text | CrossRef Full Text | Google Scholar
81. Ye J, Ma C, Hsueh EC, Eickhoff CS, Zhang Y, Varvares MA, et al. Tumor-derived gammadelta regulatory T cells suppress innate and adaptive immunity through the induction of immunosenescence. J Immunol (2013) 190(5):2403–14. doi:10.4049/jimmunol.1202369
Pubmed Abstract | Pubmed Full Text | CrossRef Full Text | Google Scholar
82. Gober HJ, Kistowska M, Angman L, Jeno P, Mori L, De Libero G. Human T cell receptor gammadelta cells recognize endogenous mevalonate metabolites in tumor cells. J Exp Med (2003) 197(2):163–8. doi:10.1084/jem.20021500
Pubmed Abstract | Pubmed Full Text | CrossRef Full Text | Google Scholar
83. Harly C, Guillaume Y, Nedellec S, Peigne CM, Monkkonen H, Monkkonen J, et al. Key implication of CD277/butyrophilin-3 (BTN3A) in cellular stress sensing by a major human gammadelta T-cell subset. Blood (2012) 120(11):2269–79. doi:10.1182/blood-2012-05-430470
Pubmed Abstract | Pubmed Full Text | CrossRef Full Text | Google Scholar
84. Sandstrom A, Peigne CM, Leger A, Crooks JE, Konczak F, Gesnel MC, et al. The intracellular B30.2 domain of butyrophilin 3A1 binds phosphoantigens to mediate activation of human Vgamma9Vdelta2 T cells. Immunity (2014) 40(4):490–500. doi:10.1016/j.immuni.2014.03.003
Pubmed Abstract | Pubmed Full Text | CrossRef Full Text | Google Scholar
85. Groh V, Steinle A, Bauer S, Spies T. Recognition of stress-induced MHC molecules by intestinal epithelial gammadelta T cells. Science (1998) 279(5357):1737–40. doi:10.1126/science.279.5357.1737
Pubmed Abstract | Pubmed Full Text | CrossRef Full Text | Google Scholar
86. Spada FM, Grant EP, Peters PJ, Sugita M, Melian A, Leslie DS, et al. Self-recognition of CD1 by gamma/delta T cells: implications for innate immunity. J Exp Med (2000) 191(6):937–48. doi:10.1084/jem.191.6.937
Pubmed Abstract | Pubmed Full Text | CrossRef Full Text | Google Scholar
87. Uldrich AP, Le Nours J, Pellicci DG, Gherardin NA, McPherson KG, Lim RT, et al. CD1d-lipid antigen recognition by the gammadelta TCR. Nat Immunol (2013) 14(11):1137–45. doi:10.1038/ni.2713
Pubmed Abstract | Pubmed Full Text | CrossRef Full Text | Google Scholar
88. Willcox CR, Pitard V, Netzer S, Couzi L, Salim M, Silberzahn T, et al. Cytomegalovirus and tumor stress surveillance by binding of a human gammadelta T cell antigen receptor to endothelial protein C receptor. Nat Immunol (2012) 13(9):872–9. doi:10.1038/ni.2394
Pubmed Abstract | Pubmed Full Text | CrossRef Full Text | Google Scholar
89. Bendelac A, Savage PB, Teyton L. The biology of NKT cells. Annu Rev Immunol (2007) 25:297–336. doi:10.1146/annurev.immunol.25.022106.141711
90. Grunder C, van Dorp S, Hol S, Drent E, Straetemans T, Heijhuurs S, et al. Gamma9 and delta2CDR3 domains regulate functional avidity of T cells harboring gamma9delta2TCRs. Blood (2012) 120(26):5153–62. doi:10.1182/blood-2012-05-432427
Pubmed Abstract | Pubmed Full Text | CrossRef Full Text | Google Scholar
91. O’Brien RL, Born WK. Gammadelta T cell subsets: a link between TCR and function? Semin Immunol (2010) 22(4):193–8. doi:10.1016/j.smim.2010.03.006
Pubmed Abstract | Pubmed Full Text | CrossRef Full Text | Google Scholar
92. Kress E, Hedges JF, Jutila MA. Distinct gene expression in human Vdelta1 and Vdelta2 gammadelta T cells following non-TCR agonist stimulation. Mol Immunol (2006) 43(12):2002–11. doi:10.1016/j.molimm.2005.11.011
Pubmed Abstract | Pubmed Full Text | CrossRef Full Text | Google Scholar
93. Zocchi MR, Ferrarini M, Migone N, Casorati G. T-cell receptor V delta gene usage by tumour reactive gamma delta T lymphocytes infiltrating human lung cancer. Immunology (1994) 81(2):234–9.
94. Correia DV, Lopes A, Silva-Santos B. Tumor cell recognition by gammadelta T lymphocytes: T-cell receptor vs. NK-cell receptors. Oncoimmunology (2013) 2(1):e22892. doi:10.4161/onci.22892
Pubmed Abstract | Pubmed Full Text | CrossRef Full Text | Google Scholar
95. Wesch D, Peters C, Oberg HH, Pietschmann K, Kabelitz D. Modulation of gammadelta T cell responses by TLR ligands. Cell Mol Life Sci (2011) 68(14):2357–70. doi:10.1007/s00018-011-0699-1
Pubmed Abstract | Pubmed Full Text | CrossRef Full Text | Google Scholar
96. Bauer S, Groh V, Wu J, Steinle A, Phillips JH, Lanier LL, et al. Activation of NK cells and T cells by NKG2D, a receptor for stress-inducible MICA. Science (1999) 285(5428):727–9. doi:10.1126/science.285.5428.727
Pubmed Abstract | Pubmed Full Text | CrossRef Full Text | Google Scholar
97. Marcu-Malina V, Heijhuurs S, van Buuren M, Hartkamp L, Strand S, Sebestyen Z, et al. Redirecting alphabeta T cells against cancer cells by transfer of a broadly tumor-reactive gammadeltaT-cell receptor. Blood (2011) 118(1):50–9. doi:10.1182/blood-2010-12-325993
Pubmed Abstract | Pubmed Full Text | CrossRef Full Text | Google Scholar
98. Das H, Groh V, Kuijl C, Sugita M, Morita CT, Spies T, et al. MICA engagement by human Vgamma2Vdelta2 T cells enhances their antigen-dependent effector function. Immunity (2001) 15(1):83–93. doi:10.1016/S1074-7613(01)00168-6
Pubmed Abstract | Pubmed Full Text | CrossRef Full Text | Google Scholar
99. Nedellec S, Sabourin C, Bonneville M, Scotet E. NKG2D costimulates human V gamma 9V delta 2 T cell antitumor cytotoxicity through protein kinase C theta-dependent modulation of early TCR-induced calcium and transduction signals. J Immunol (2010) 185(1):55–63. doi:10.4049/jimmunol.1000373
Pubmed Abstract | Pubmed Full Text | CrossRef Full Text | Google Scholar
100. Rincon-Orozco B, Kunzmann V, Wrobel P, Kabelitz D, Steinle A, Herrmann T. Activation of V gamma 9V delta 2 T cells by NKG2D. J Immunol (2005) 175(4):2144–51. doi:10.4049/jimmunol.175.4.2144
Pubmed Abstract | Pubmed Full Text | CrossRef Full Text | Google Scholar
101. Lanca T, Correia DV, Moita CF, Raquel H, Neves-Costa A, Ferreira C, et al. The MHC class Ib protein ULBP1 is a nonredundant determinant of leukemia/lymphoma susceptibility to gammadelta T-cell cytotoxicity. Blood (2010) 115(12):2407–11. doi:10.1182/blood-2009-08-237123
Pubmed Abstract | Pubmed Full Text | CrossRef Full Text | Google Scholar
102. Xu B, Pizarro JC, Holmes MA, McBeth C, Groh V, Spies T, et al. Crystal structure of a gammadelta T-cell receptor specific for the human MHC class I homolog MICA. Proc Natl Acad Sci U S A (2011) 108(6):2414–9. doi:10.1073/pnas.1015433108
Pubmed Abstract | Pubmed Full Text | CrossRef Full Text | Google Scholar
103. Correia DV, Fogli M, Hudspeth K, da Silva MG, Mavilio D, Silva-Santos B. Differentiation of human peripheral blood Vdelta1+ T cells expressing the natural cytotoxicity receptor NKp30 for recognition of lymphoid leukemia cells. Blood (2011) 118(4):992–1001. doi:10.1182/blood-2011-02-339135
Pubmed Abstract | Pubmed Full Text | CrossRef Full Text | Google Scholar
104. Vermijlen D, Brouwer M, Donner C, Liesnard C, Tackoen M, Van Rysselberge M, et al. Human cytomegalovirus elicits fetal gammadelta T cell responses in utero. J Exp Med (2010) 207(4):807–21. doi:10.1084/jem.20090348
Pubmed Abstract | Pubmed Full Text | CrossRef Full Text | Google Scholar
105. Deusch K, Luling F, Reich K, Classen M, Wagner H, Pfeffer K. A major fraction of human intraepithelial lymphocytes simultaneously expresses the gamma/delta T cell receptor, the CD8 accessory molecule and preferentially uses the V delta 1 gene segment. Eur J Immunol (1991) 21(4):1053–9. doi:10.1002/eji.1830210429
Pubmed Abstract | Pubmed Full Text | CrossRef Full Text | Google Scholar
106. Bucy RP, Chen CL, Cooper MD. Tissue localization and CD8 accessory molecule expression of T gamma delta cells in humans. J Immunol (1989) 142(9):3045–9.
107. Bhagat G, Naiyer AJ, Shah JG, Harper J, Jabri B, Wang TC, et al. Small intestinal CD8+TCRgammadelta+NKG2A+ intraepithelial lymphocytes have attributes of regulatory cells in patients with celiac disease. J Clin Invest (2008) 118(1):281–93. doi:10.1172/JCI30989
Pubmed Abstract | Pubmed Full Text | CrossRef Full Text | Google Scholar
108. Clemente A, Cambra A, Munoz-Saa I, Crespi C, Pallares L, Juan A, et al. Phenotype markers and cytokine intracellular production by CD8+ gammadelta T lymphocytes do not support a regulatory T profile in Behcet’s disease patients and healthy controls. Immunol Lett (2010) 129(2):57–63. doi:10.1016/j.imlet.2010.02.005
Pubmed Abstract | Pubmed Full Text | CrossRef Full Text | Google Scholar
109. Omi K, Shimizu M, Watanabe E, Matsumura J, Takaku C, Shinya E, et al. Inhibition of R5-tropic HIV type-1 replication in CD4(+) natural killer T cells by gammadelta T lymphocytes. Immunology (2014) 141(4):596–608. doi:10.1111/imm.12221
Pubmed Abstract | Pubmed Full Text | CrossRef Full Text | Google Scholar
110. Abe Y, Muto M, Nieda M, Nakagawa Y, Nicol A, Kaneko T, et al. Clinical and immunological evaluation of zoledronate-activated Vgamma9gammadelta T-cell-based immunotherapy for patients with multiple myeloma. Exp Hematol (2009) 37(8):956–68. doi:10.1016/j.exphem.2009.04.008
Pubmed Abstract | Pubmed Full Text | CrossRef Full Text | Google Scholar
111. Michishita Y, Hirokawa M, Guo YM, Abe Y, Liu J, Ubukawa K, et al. Age-associated alteration of gammadelta T-cell repertoire and different profiles of activation-induced death of Vdelta1 and Vdelta2 T cells. Int J Hematol (2011) 94(3):230–40. doi:10.1007/s12185-011-0907-7
Pubmed Abstract | Pubmed Full Text | CrossRef Full Text | Google Scholar
112. Giachino C, Granziero L, Modena V, Maiocco V, Lomater C, Fantini F, et al. Clonal expansions of V delta 1+ and V delta 2+ cells increase with age and limit the repertoire of human gamma delta T cells. Eur J Immunol (1994) 24(8):1914–8. doi:10.1002/eji.1830240830
Pubmed Abstract | Pubmed Full Text | CrossRef Full Text | Google Scholar
113. Dar AA, Patil RS, Chiplunkar SV. Insights into the relationship between toll like receptors and gamma delta T cell responses. Front Immunol (2014) 5:366. doi:10.3389/fimmu.2014.00366
Pubmed Abstract | Pubmed Full Text | CrossRef Full Text | Google Scholar
114. Pietschmann K, Beetz S, Welte S, Martens I, Gruen J, Oberg HH, et al. Toll-like receptor expression and function in subsets of human gammadelta T lymphocytes. Scand J Immunol (2009) 70(3):245–55. doi:10.1111/j.1365-3083.2009.02290.x
Pubmed Abstract | Pubmed Full Text | CrossRef Full Text | Google Scholar
115. Vilches C, Parham P. KIR: diverse, rapidly evolving receptors of innate and adaptive immunity. Annu Rev Immunol (2002) 20:217–51. doi:10.1146/annurev.immunol.20.092501.134942
Pubmed Abstract | Pubmed Full Text | CrossRef Full Text | Google Scholar
116. Crompton JG, Sukumar M, Restifo NP. Uncoupling T-cell expansion from effector differentiation in cell-based immunotherapy. Immunol Rev (2014) 257(1):264–76. doi:10.1111/imr.12135
Pubmed Abstract | Pubmed Full Text | CrossRef Full Text | Google Scholar
Keywords: γδT cells, cancer immunotherapy, γδT cell diversity, innate-like lymphocytes, γδTCR
Citation: Scheper W, Sebestyen Z and Kuball J (2014) Cancer immunotherapy using γδT cells: dealing with diversity. Front. Immunol. 5:601. doi: 10.3389/fimmu.2014.00601
Received: 11 September 2014; Paper pending published: 13 October 2014;
Accepted: 07 November 2014; Published online: 20 November 2014.
Edited by:
Dieter Kabelitz, Christian-Albrechts University Kiel, GermanyReviewed by:
Hansjörg Schild, University Medical Center of the Johannes Gutenberg-University, GermanyMaria L. Toribio, Spanish National Research Council (CSIC), Spain
Copyright: © 2014 Scheper, Sebestyen and Kuball. This is an open-access article distributed under the terms of the Creative Commons Attribution License (CC BY). The use, distribution or reproduction in other forums is permitted, provided the original author(s) or licensor are credited and that the original publication in this journal is cited, in accordance with accepted academic practice. No use, distribution or reproduction is permitted which does not comply with these terms.
*Correspondence: Jürgen Kuball, Laboratory of Translational Immunology, Department of Hematology, University Medical Center Utrecht, Room number Q05.4.301, PO Box 85500, Utrecht 3508GA, Netherlands e-mail:ai5oLmUua3ViYWxsQHVtY3V0cmVjaHQubmw=