- 1Department of Immunology, Medical Research Institute, Tokyo Medical and Dental University, Tokyo, Japan
- 2Department of Biomedical Science, Graduate School of Agricultural and Life Sciences, The University of Tokyo, Tokyo, Japan
- 3Department of Agrobioscience, Graduate School of Agricultural Science, Kobe University, Kobe, Japan
- 4Biomedical Research Institute, National Institute for Advanced Industrial Science and Technology (AIST), Tsukuba, Japan
- 5Department of Bio-science and Engineering, Shibaura Institute of Technology, Saitama, Japan
- 6Laboratory for Cell Function and Dynamics, Advanced Technology Development Group, Brain Science Institute, RIKEN, Saitama, Japan
- 7Department of Immune Regulation, Tokyo Medical and Dental University, Tokyo, Japan
- 8Laboratory of Recombinant Animals, Medical Research Institute, Tokyo Medical and Dental University, Tokyo, Japan
- 9NARO Institute of Livestock and Grassland Science, National Agriculture and Food Research Organization, Tsukuba, Ibaraki, Japan
- 10Laboratory of Veterinary Public Health, Graduate School of Agricultural and Life Sciences, The University of Tokyo, Tokyo, Japan
Probiotics, such as lactic acid bacteria (LAB) and Bacillus subtilis var. natto, have been shown to modulate immune responses. It is important to understand how probiotic bacteria impact intestinal epithelial cells (IECs), because IECs are the first line of defense at the mucosal surface barrier and their activities substantially affect the gut microenvironment and immunity. However, to date, their precise mechanism remains unknown due to a lack of analytical systems available for live animal models. Recently, we generated a conditional Ca2+ biosensor Yellow Cameleon (YC3.60) transgenic mouse line and established 5D (x, y, z, time, and Ca2+) intravital imaging systems of lymphoid tissues including those in Peyer’s patches and bone marrow. In the present study, we further advance our intravital imaging system for intestinal tracts to visualize IEC responses against orally administrated food compounds in real time. Using this system, heat-killed B. subtilis natto, a probiotic TTCC012 strain, is shown to directly induce Ca2+ signaling in IECs in mice housed under specific pathogen-free conditions. In contrast, this activation is not observed in the Lactococcus lactis strain C60; however, when we generate germ-free YC3.60 mice and observe the LAB stimulation of IECs in the absence of gut microbiota, C60 is capable of inducing Ca2+ signaling. This is the first study to successfully visualize the direct effect of probiotics on IECs in live animals. These data strongly suggest that probiotic strains stimulate IECs under physiological conditions and that their activity is affected by the microenvironment of the small intestine, such as commensal bacteria.
Introduction
Food compounds are digested and absorbed through the gastrointestinal tract for nutrition, and probiotic bacteria and polysaccharides affect immunological homeostasis in the gut (1–6). Fermentative lactic acid bacteria (LAB) are aerobic and abundant in the environment and are very often contained in the average diet, consequently composing a major part of small intestinal commensal flora (7–9). LAB, therefore, affect the maturation of host immune cells and intestinal immune homeostasis under normal steady-state conditions (10–12). Oral administration of some LAB strains has been shown to stimulate innate immunity at mucosal sites and to potentiate systemic immune responses against pathogenic bacteria or viruses (13–17). In addition to resident LAB, orally administrated LAB, although inactive, have a substantial effect on the regulation of immunity. We recently described an anti-inflammatory mechanism exclusive to LAB strains. Most LAB strains contain large amounts of double-stranded RNA and are sensed by the endosomal toll-like receptor (TLR) 3 on intestinal dendritic cells to produce interferon-β. This innate sensing procedure contributes to anti-inflammatory and protective immune responses both locally and systemically; therefore, both live and inactive LAB can be utilized as effective probiotics (12). Functional maturation of the immune system is largely dependent on mucosal biological events, and our findings suggest a co-evolutional process through a long-term mutualism between LAB and the immune system. We have demonstrated that Lactococci tolerates bile acids and low pH and adheres to human enterocyte-like Caco-2 cells (18). We have not, however, determined the mechanism of interaction between LAB and intestinal epithelial cells (IECs).
Intestinal epithelial cells communicate with commensal microbes and probiotics and potentiate immune responses via cytokines and antigen delivery (19). Probiotics trigger signaling pathways in IECs, such as NF-κB and MAP kinase, which affect the immune response and integrity of the mucosal surface barrier. However, it is difficult to monitor their biological events in real time in vivo. This issue became an obstacle in our initial study on the interaction between probiotics and IECs. Thus, it would be of great value to develop a reliable analytical system for intravital imaging of IECs.
Calcium ions (Ca2+) are universal second messengers performing multiple functions in most cells. In the immune system, stimulation of immunological receptors, including B-cell antigen and cytokine receptors, induces intracellular Ca2+ mobilization concomitant with other signaling events such as phosphorylation of cellular substrates (20–24). To visualize Ca2+ signaling in vivo, we generated a conditional Föster/fluorescent resonance energy transfer (FRET)-based calcium biosensor Yellow Cameleon 3.60 (YC3.60) transgenic mice (25). YC3.60 is a double-chromophore indicator that employs FRET between a cyan fluorescent protein (CFP) and a circularly permuted variant of the yellow fluorescent protein (YFP) Venus (26). Ca2+ signaling can be monitored by measuring the ratio of YFP to CFP (YFP/CFP). FRET-based ratiometric indicators including YC3.60 can be corrected for unequal sensor expression and motion-derived changes in fluorescent intensity. Therefore, ratiometric sensors, such as YC3.60, are suitable for in vivo whole-body imaging in mice. Accordingly, we have recently established 5D (x, y, z, time, and Ca2+ signal) live imaging of immunological tissues including those in bone marrow and Peyer’s patches (25).
Here, we applied our system to detect probiotic-mediated Ca2+ signaling in IECs in vivo and found differences between the two types of Gram-positive probiotic bacteria, Lactococcus lactis and Bacillus subtilis var. natto. Our results suggest, for the first time, that probiotic strains stimulate small IECs via intravital observations; in addition, these results facilitate the understanding of probiotic-mediated immunoregulatory mechanisms.
Materials and Methods
Mice
The conditional YC3.60 expression transgenic mouse line has been previously described (25). The floxed YC3.60 reporter (YC3.60flox) mouse line was crossed with a CD19-Cre mouse line (27), which resulted in CD19+ cell-specific YC3.60 expression in YC3.60flox/CD19-Cre mice due to the loss of the loxP-flanked neomycin cassette. The YC3.60flox mouse line was crossed with a CAG-Cre (28) mouse line, which expresses the Cre gene ubiquitously. These mice were maintained in our animal facility under specific pathogen-free (SPF) conditions in accordance with the guidelines of the Tokyo Medical and Dental University for animal care. These procedures have been approved by the Committee of the Tokyo Medical and Dental University for animal care.
Germ-free BALB/cA mice were bred at the Laboratory of Veterinary Public Health, the University of Tokyo, and were used as foster mothers. Germ-free animals were kept in flexible vinyl isolators in a room at 24°C, relative humidity of 60%, and 12 h periods of light and dark, and were fed a CMF-pelleted diet (Oriental Yeast Co., Tokyo, Japan) sterilized by γ-irradiation at a dose of 50 kGy. For the generation of germ-free mice with ubiquitous YC3.60 expression, in vitro fertilization and cesarean operation were performed as described below. Female mice with ubiquitous YC3.60 expression were superovulated by an intraperitoneal injection of 7.5 IU eCG followed by 7.5 IU hCG at an interval of 48 h. Eggs were collected from sacrificed female mice and fertilized with the sperm of male mice with ubiquitous YC3.60 expression in HTF medium (ARK Resource, Kumamoto, Japan). After overnight culture in the KSOM medium (ARK Resource), two-cell embryos were transferred into the oviducts of pseudopregnant female ICR mice. The estimated delivery date was controlled by a subcutaneous injection of Progehorrmon (Mochida Pharmaceutical Co., Ltd., Tokyo, Japan). The surrogate mothers were sacrificed at the fetal age of 19.5 days by cervical dislocation, and the uterus was aseptically removed with clamps at the top of each uterine horn and the base of the uterus close to the cervix. The uterus was introduced into an isolator for operation through a germicidal trap with 2% peracetic acid solution kept at 37°C. The uterus was cut with scissors, and pups were removed. Their breathing was stimulated, and they were cleaned with dry gauze. After the pups started breathing normally, they were transferred to the isolator with their foster mothers. The germ status was checked once a month. These procedures were approved by the Committee for Care of Laboratory Animals in the Graduate School of Agricultural and Life Sciences at the University of Tokyo.
Probiotic Bacteria
Lactococcus lactis subsp. cremoris C60 (29) was cultured in MRS broth (BD Difco) for 20 h at 30°C (late-log phase) at the National Institute of Advanced Industrial Science and Technology (AIST). The bacteria were harvested, washed two times, and resuspended in sterile saline. The suspensions were then heated for 30 min at 70°C (heat-killed) and were stored at −80°C. Heat-killed B. subtilis var. natto TTCC12 (late-log phase) were kindly provided from Takano Foods Co. Ltd. and were stored at −80°C.
Flow Cytometry
Calcium ions mobilization was analyzed using flow cytometry. Ca2+ mobilization in YC3.60-expressing cells was analyzed by flow cytometry using CyAn ADP™ (Beckman Coulter) as previously described (25). Antibodies with the following specificity of CD19-Alexa647 and B220-Alexa647 (BioLegend) were used.
Intravital and In Vitro Microscope
Intestinal epithelial cells from anesthetized mice were imaged. Small intestinal tracts were surgically opened lengthwise, placed on a cover glass, and immobilized on a microscope stage. For image acquisition, a Nikon A1 laser-scanning confocal microscope with a 20× objective and NIS-Elements AR software was used as previously described (25). We used a dichronic mirrors (DM457/514) and two bandpass emission filters (482/35 for CFP, 540/30 for YFP). YFP/CFP ratio was obtained by excitation at 458 nm. Images of purified spleen cells in PBS were also obtained as above. Acquired images were analyzed with NIS-Elements software (Nikon).
Results
Establishment of In Vivo Ca2+ Signaling Detection System in Intestinal Gut Epithelial Cells
We previously established an intravital imaging system of Ca2+ signaling in lymphoid tissues, such as in Peyer’s patches, spleen, and bone marrow (25). To visualize Ca2+ signaling in IECs, we surgically opened the small intestinal tract of the mice with ubiquitous YC3.60 expression, fixed a cover glass on it, and placed it on the stage of the confocal microscope (Figure 1A). Images of the villi in the middle of the small intestine of the mice with ubiquitous YC3.60 expression are shown in Figure 1B. Images of over 50 µm from top of the villi to the basal were obtained. Reconstructed 3D structures showed that almost the entire length of the small intestinal villi could be visualized (Figure 1C). There were no salient differences in intracellular Ca2+ concentration among the total epithelial cells, and they included heterogeneous minor subpopulations, such as goblet cells, enteroendocrine cells, and tuft cells (30, 31).
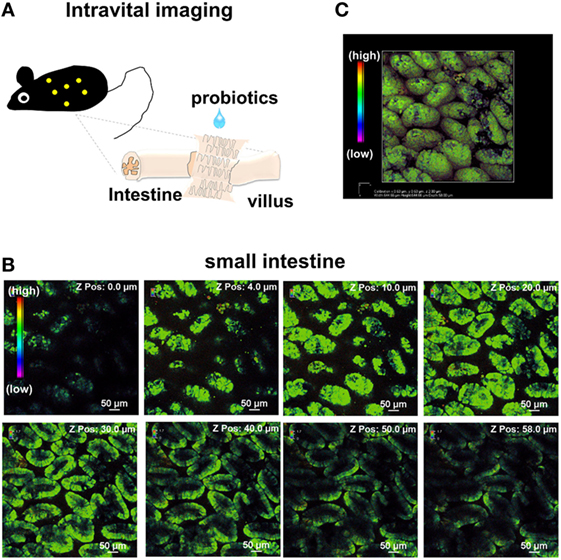
Figure 1. Structure of intestinal villi in a mouse under ubiquitous YC3.60 expression. (A) Schematic method of intravital imaging of intestinal epithelial cells (IECs). (B) Z-stack analysis of epithelial cells in the intestinal tract. Intravital imaging of small intestinal villi in the jejunum was performed using confocal lazar microscopy. Ratiometric images (yellow fluorescent protein/cyan fluorescent protein at excitation of 458 nm) are shown. Z-stack images of 2-µm intervals up to a depth of 58 µm were obtained. Only representative images are shown. A rainbow parameter indicates relative Ca2+ concentration; scale bar, 50 µm. (C) 3D structures of small IECs with intracellular Ca2+ concentrations. 3D images based on Z-stack images (B) were obtained using NIS-Elements software. Shown are representative results from three mice.
Intravital imaging of the IECs showed sporadic but relatively minute Ca2+ signaling in some regions under steady-state conditions (Figure 2A). Less than 1% IECs exhibited spontaneous Ca2+ signaling (Figure 2B). To determine if the perceptive Ca2+ signaling response is observed in this system, we first tested ionomycin, as a positive control, on the stimuli. Upon the addition of ionomycin, transient Ca2+ elevation was observed in many IECs (Figures 2C,D). Thus, a system was established to detect in vivo real-time Ca2+ signaling of IECs.
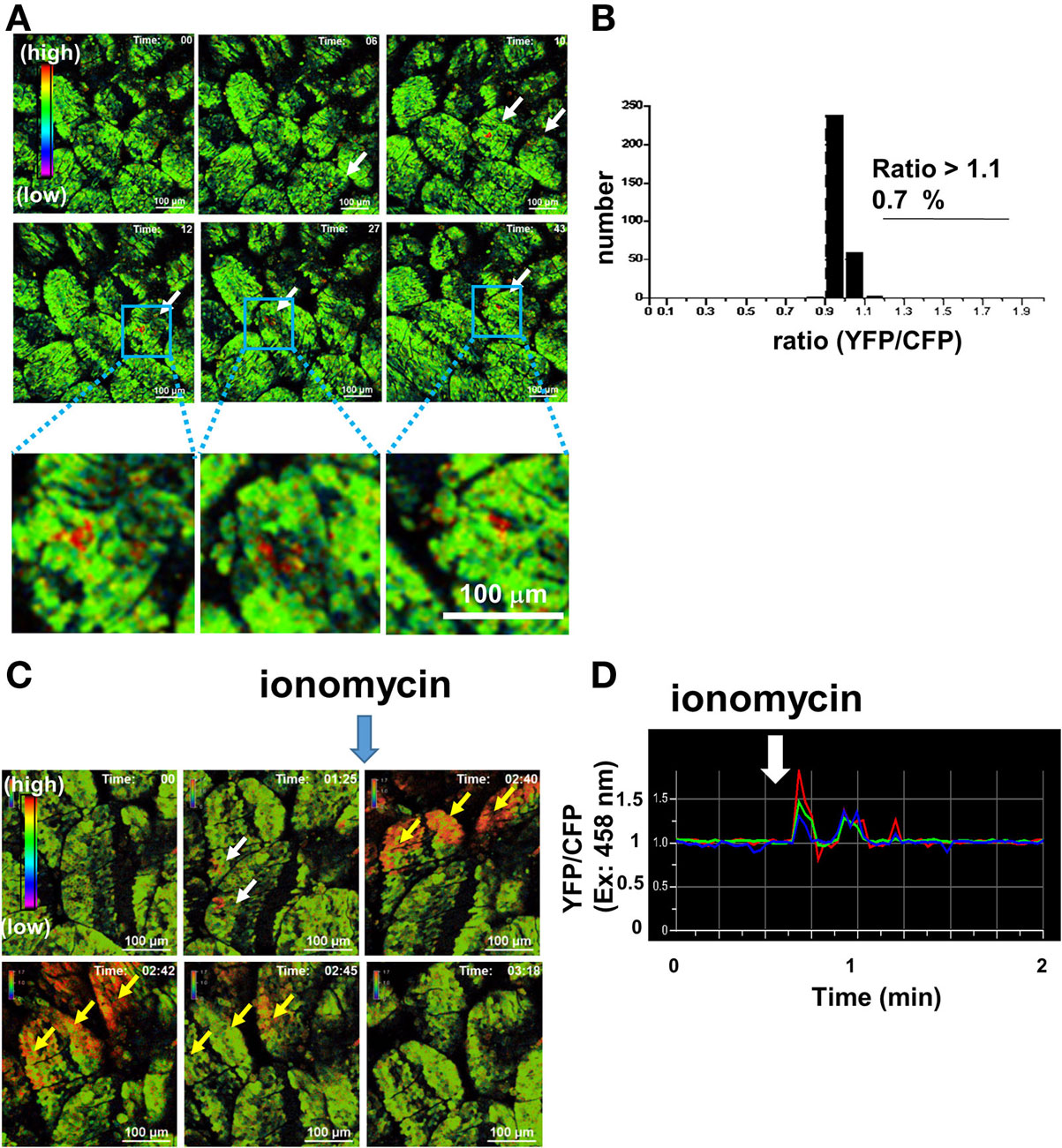
Figure 2. Intravital Ca2+ signaling images mediated by ionomycin in the intestinal tract of a mouse under ubiquitous YC3.60 expression. (A) Representative Ca2+ signaling images in the intestinal tract of a mouse under ubiquitous YC3.60 expression without any stimulation. Ratiometric images [yellow fluorescent protein (YFP)/cyan fluorescent protein (CFP) at excitation of 458 nm] are shown. The rainbow parameter indicates relative Ca2+ concentration. (B) Distribution of time-integrated intracellular Ca2+ concentrations of randomly selected regions. N = 10; frame = 30. (C) Representative Ca2+ signaling images in the intestinal tract of a mouse under ubiquitous YC3.60 expression with ionomycin. Ratiometric images (YFP/CFP at excitation of 458 nm) are shown. Five micromolars of ionomycin in PBS were added at the indicated time point. A rainbow parameter indicates relative Ca2+ concentration; scale bar, 100 µm; frame = 85. (D) Ratiometric intensities (YFP/CFP at excitation of 458 nm) of indicated regions, represented by yellow arrows in (C) (n = 3), were measured for 2 min at 2-s intervals. Spontaneous Ca2+ signals are indicated by white arrows. Shown are representative results from two mice.
Effect of Probiotics on Ca2+ Signaling in the IECs of the Mice with Ubiquitous YC3.60 Expression In Vivo
Lactococcus lactis (18, 29) regulate immune responses by inducing cytokines in dendritic cells and B. subtilis natto regulate gut flora and immunity (32–34). We tested whether these probiotics induce Ca2+ signaling in IECs. Intravital imaging of IECs exhibited Ca2+ signaling upon B. subtilis natto treatment (Figure 3A; Video S1 in Supplementary Material). Bacillus subtilis natto induced gradual and sustained elevation of intracellular Ca2+ concentration in most cells (Figures 3A,B). Figure 3C shows that intracellular Ca2+ concentration in IECs was strikingly increased after adding B. subtilis natto. Thus, the kinetics of B. subtilis natto-mediated Ca2+ signaling in IECs is clearly distinct from that observed under steady-state conditions (Figure 2A). One LAB strain, L. lactis C60, did not induce Ca2+ signaling in IECs except for spontaneous signals (Figures 3D–F). This result is surprising as both B. subtilis and LAB are Gram-positive bacteria and well-known probiotics; yet the responses of IECs in SPF mice were distinct in inducing Ca2+ signaling. LAB compose a major part of small intestinal commensal flora, and therefore, chronic exposure to the bacteria species may have induced hyporesponsiveness of IECs against LAB.
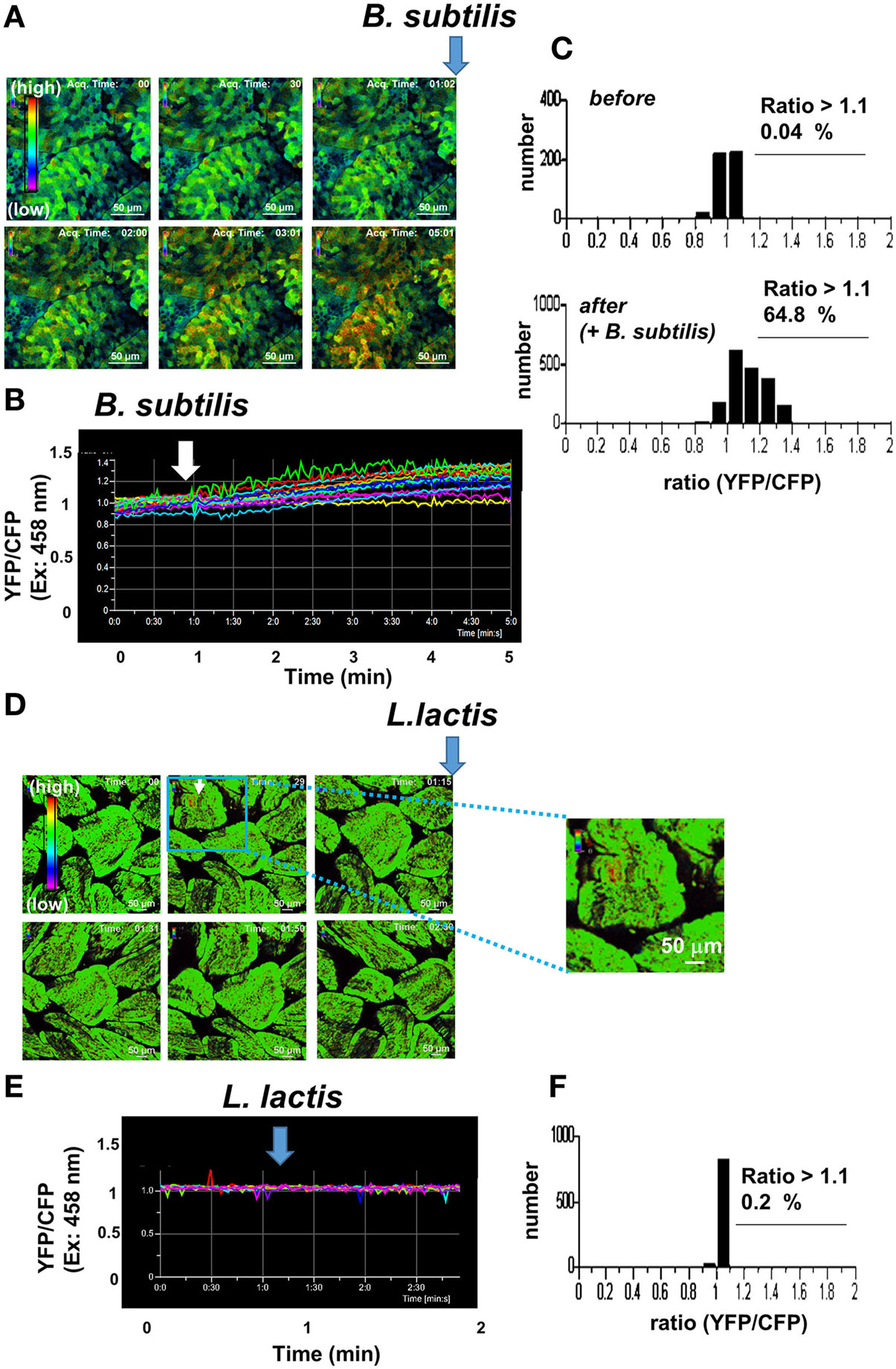
Figure 3. Intravital Ca2+ signaling images mediated by probiotics in the intestinal tract of a mouse under ubiquitous YC3.60 expression. (A) Representative Ca2+ signaling images in the intestinal tract of a mouse under ubiquitous YC3.60 expression and specific pathogen-free (SPF) conditions. Ratiometric images [yellow fluorescent protein (YFP)/cyan fluorescent protein (CFP) at excitation of 458 nm] are shown. The 0.1 ml of Bacillus subtilis natto in PBS (109 cells/ml) was added at the indicated time point. A rainbow parameter indicates relative Ca2+ concentration. (B) Time course for fluorescence intensities of YFP/CFP on excitation at 458 nm. Randomly selected regions (n = 10) were measured. Scale bar, 50 µm; frame = 151. Spontaneous Ca2+ signals are indicated by arrows. (C) Distribution of time-integrated intracellular Ca2+ concentrations of randomly selected regions before (upper panel) and after (lower panel) stimulation; n = 10. (D) Representative Ca2+ signaling images in the intestinal tract of a mouse under ubiquitous YC3.60 expression and SPF conditions. Ratiometric images (YFP/CFP at excitation of 458 nm) are shown. The 0.1 ml of Lactococcus lactis in PBS (109 cells/ml) was added at the indicated time point. (E) Time course for fluorescence intensities of YFP/CFP on excitation at 458 nm; frame = 85. (F) Distribution of time-integrated intracellular Ca2+ concentrations of randomly selected regions; n = 10. Shown are representative results from three mice.
LAB Induces Ca2+ Signaling in IECs under Germ-Free Conditions
As Lactococcus is a related genus of Enterococcus, a constituent of gut-resident LAB in the small intestine (7), it may constantly stimulate IECs under steady-state conditions. IECs may refrain from responding to heat-killed L. lactis C60 and induce Ca2+ signaling due to chronic microbial stimuli by Enterococci. We attempted to clarify whether small intestinal microenvironments, especially gut commensal flora, modulate the responsiveness of IECs against LAB.
To this end, we generated germ-free YC3.60 mice to determine whether L. lactis can induce Ca2+ signaling in IECs in the absence of gut microbiota. As shown in Figure 4 and Video S2 in Supplementary Material, L. lactis C60 induced sustained intracellular Ca2+ elevation as B. subtilis natto, indicating that L. lactis C60 can stimulate IECs directly in the absence of gut microbiota. Many IECs were stimulated by adding L. lactis under germ-free conditions (Figure 4B). Furthermore, the IECs in germ-free mice exhibited sporadic Ca2+ signals under steady-state conditions regardless of L. lactis stimulation (Figures 4A,C). The frequency of sporadic Ca2+ signals in germ-free mice (Figure 4B, left panel) is higher than that in the SPF mice (Figure 2A).
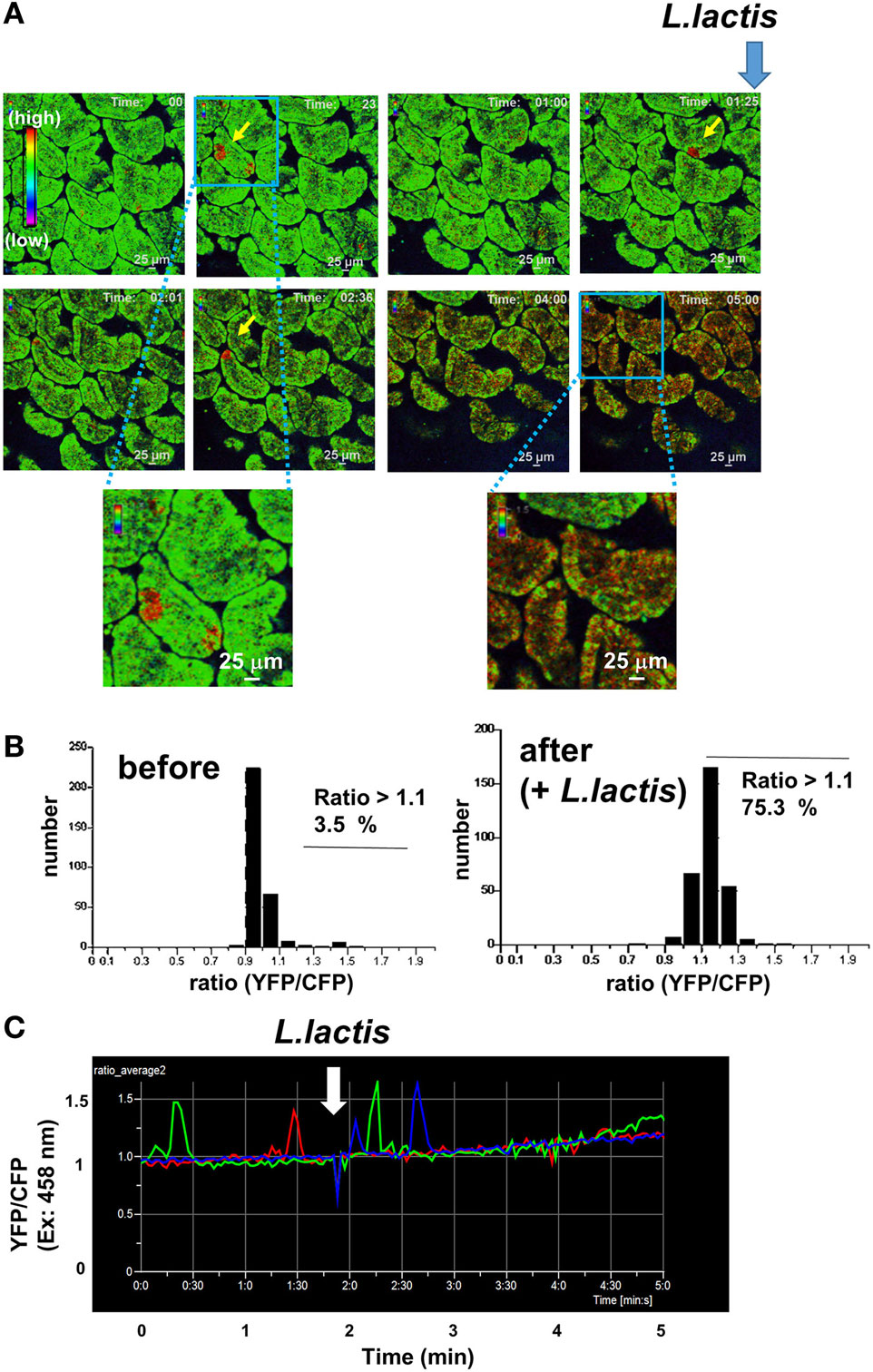
Figure 4. Intravital Ca2+ signaling images mediated by Lactococcus lactis in the intestinal tract of a mouse under ubiquitous YC3.60 expression. (A) Representative Ca2+ signaling images in the intestinal tract of a mouse under ubiquitous YC3.60 expression and germ-free conditions. Ratiometric images [yellow fluorescent protein (YFP)/cyan fluorescent protein (CFP) at excitation of 458 nm] are shown. The 0.1 ml of L. lactis in PBS (109 cells/ml) was added at the indicated time point. A rainbow parameter indicates relative Ca2+ concentration. Scale bar, 25 µm; frame = 145. Spontaneous Ca2+ signals are indicated by arrows. (B) Distribution of time-integrated intracellular Ca2+ concentrations of randomly selected regions before (left) and after (right) stimulation. (C) Time course for fluorescence intensities of YFP/CFP on excitation at 458 nm in the indicated region is represented by the yellow arrow in (A); n = 3. Shown are representative results from three mice.
L. Lactis Induces Ca2+ Signaling in B Cells In Vitro
Probiotics directly stimulate various immune cells such as dendritic cells, macrophages, NK cells, and T cells (11, 35). We prepared primary B cells from the spleens of YC3.60flox/CD19-Cre mice and stimulated with B. subtilis natto (Figures 5A,B) or L. lactis (Figures 5C,D). Upon stimulation, both B. subtilis natto and L. lactis induced Ca2+ mobilization in primary B cells (Figure 5), confirming their direct stimulation of B cells. Time-lapse observation of single cells was useful to clarify heterogeneity in the kinetics of Ca2+ signaling (Figures 5B,D).
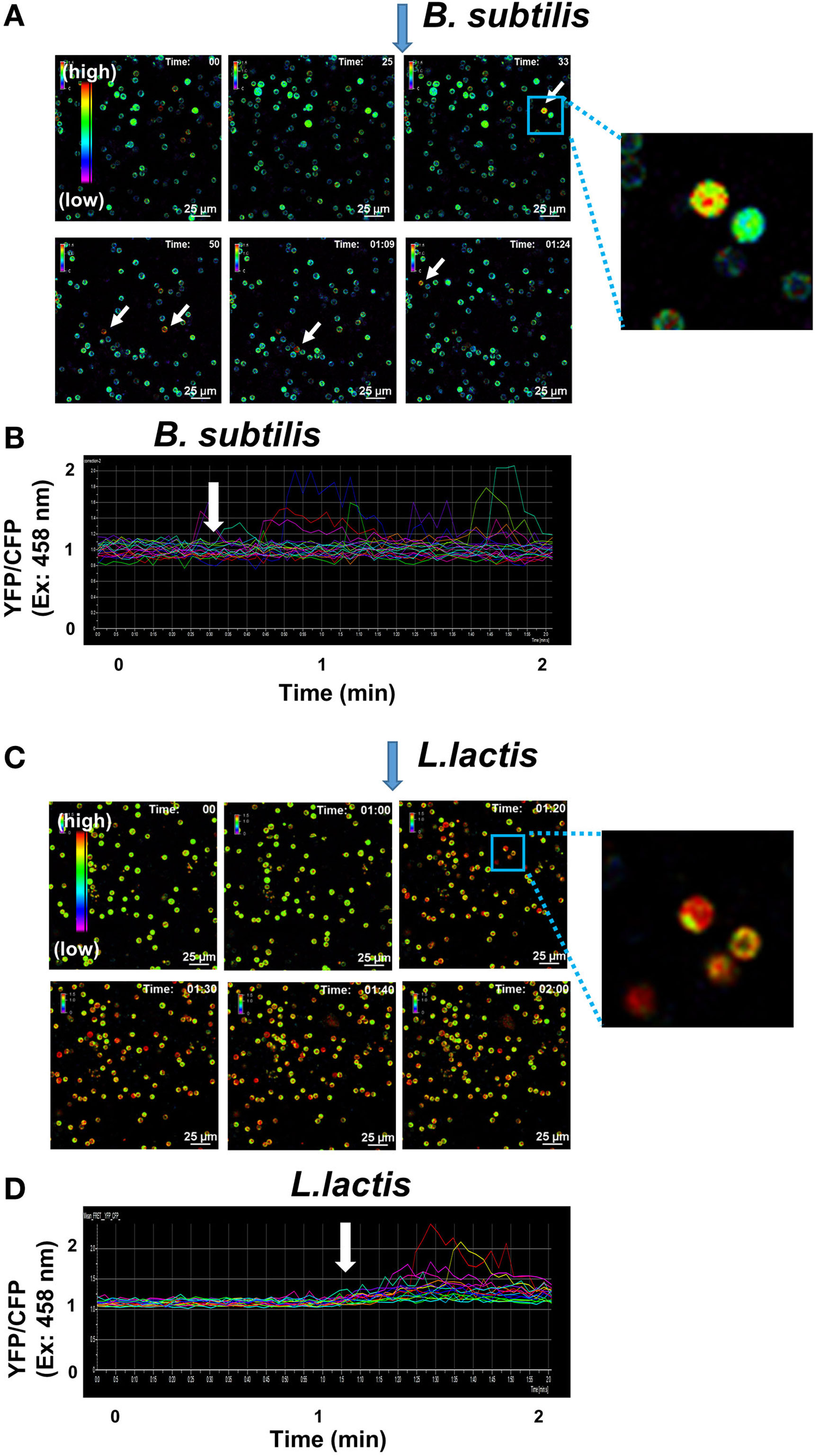
Figure 5. Ca2+ signaling images mediated by probiotics in B cells in vitro. (A) Representative Ca2+ signaling images in spleen B cells form CD19-Cre/YC3.60 mice. Ratiometric images [YFP/cyan fluorescent protein (CFP) at excitation of 458 nm] are shown. Ten microliters of Bacillus subtilis natto in PBS (109 cells/ml) were added to the cell culture (0.2 ml) at the indicated time point. A rainbow parameter indicates relative Ca2+ concentration. (B) The time course for fluorescence intensities of YFP/CFP on excitation at 458 nm in the cells (n = 15). Scale bar, 25 µm; frame = 56. (C) Representative Ca2+ signaling images in spleen B cells of CD19-Cre/YC3.60 expression in mice. Ratiometric images (YFP/CFP at excitation of 458 nm) are shown. Ten microliters of Lactococcus lactis in PBS (109 cells/ml) were added to the cell culture at the indicated time point. A rainbow parameter indicates relative Ca2+ concentration. (D) The time course for fluorescence intensities of YFP/CFP on excitation at 458 nm in the cells (n = 15). Scale bar, 25 µm; frame = 61. Shown are representative results of three experiments.
Discussion
Here, we establish a real-time visualization system of Ca2+ signaling in small IECs in vivo to monitor food signals. By using this system, we show that B. subtilis natto triggers Ca2+ signaling in IECs and that L. lactis evokes Ca2+ signaling in gut tissue under germ-free conditions but not under SPF conditions. These results suggest that gut microbiota regulate their responses against orally administered probiotics.
In this study, we successfully visualize probiotic-mediated Ca2+ signaling in IECs based on the 5D (x, y, z, time, and Ca2+) live imaging system in YC3.60 mice (25). A calcium biosensor YC3.60 is a double-chromophore indicator that employs FRET between CFP and YFP mutants (Venus) (26). Ca2+ signaling can be monitored by measuring YFP/CFP when CFP is excited. Motion-induced artifacts or unequal biosensor expression are corrected by an internal control in the denominator of the ratio. Thus, the ratiometric sensor YC3.60 is suitable for in vivo imaging of the gut, which exhibits vigorous motion with peristalsis in addition to beating and breathing. Moreover, 5D live imaging of tissues enables time-lapse monitoring of dynamic Ca2+ signaling in single cells, cell–cell interactions, and other segments of interests. Response of these tissues is quantified and integrated over the desired time span as shown in Figures 2B, 3C,F and 4B.
Probiotic B. subtilis natto, but not L. lactis, triggers Ca2+ signaling in the gut epithelium in SPF mice, although both of them are capable of stimulating spleen B cells from mice with the same microbial conditions. Since L. lactis C60 stimulates IECs in the absence of gut microbiota in germ-free mice, the gut microbiota may shape the responsiveness of IECs against LAB.
Intestinal epithelial cells express a series of pattern recognition receptors (PRRs) including TLRs, nucleotide-binding sites, leucine-rich repeat-containing receptors, and retinoic acid-inducible gene-I-like receptors (36). Bacterial components stimulate these PRRs and regulate IRFs, NF-κB, and/or the MAP kinase signaling pathway. One study showed that IECs from germ-free mice show a reduction in TLR expression (37). Another study reported that immunobiotic strains regulate the expression and activity of TLRs in IECs (38). These findings suggest that microbial conditions shape the homeostatic regulation of functional PRRs. Since Lactococcus is similar to Enterococcus, a major member of small intestinal commensal LAB, probably IECs, at least in part, is hyporesponsive to this symbiotic genus of bacteria. We hypothesize that due to this hyporesponsiveness, further stimulation with L. lactis does not induce visible Ca2+ signaling despite the expression of PRRs on the IECs. As reported, probiotic strains tolerate IECs (38, 39); such causal relationships may also explain the frequent and sporadic Ca2+ signaling observed in IECs of germ-free mice under steady-state conditions (Figure 4). The molecular mechanisms underlying these observations, however, remain unclear.
We find that IECs exhibit sporadic transient Ca2+ signaling, although we do not know the precise mechanism of this action. The signals appear to be more striking in the germ-free mice than in the SPF mice, suggesting that these signals are mediated by the gut microbiota or endocrine systems (40).
B cells also express PRRs and are directly stimulated by microbial components (41). Although L. lactis C60 fails to induce Ca2+ signaling in IECs under SPF conditions, it strongly induces Ca2+ signaling in spleen B cells from SPF mice. In contrast, B. subtilis natto induces Ca2+ signaling in both IECs in vivo and B cells in vitro. It is not known whether the different reactivity against two types of probiotics can be attributed to the difference in the methods between the intravital and in vitro assay, to the skewed influence of stimuli including commensal flora and diet on local and systemic immune cells, or to the outcome of strain difference of probiotics. Additional studies are required to evaluate these results further.
Understanding the function of IECs is important to evaluate immune responses, since stimulated IECs produce cytokines and/or chemokines (11, 23). Goblet cells and M cells deliver antigens to dendritic cells (42, 43). Beneficial probiotic signaling may be transferred to immune cells through these mechanisms, resulting in the regulation of immune tolerance or response. In this study, we show that an intravital imaging system using YC3.60 mice allows for the detection of real-time activation of IECs by probiotics. This system is proven here to be a powerful method for not only clarifying the effects of probiotics on epithelial cell–immune cell communication with stoichiometries but also detecting a subtle disorder before pathological onset (25) and developing preventive and therapeutic strategies with probiotics.
Ethics Statement
YC3.60 mice were maintained in our animal facility under SPF conditions in accordance with the guidelines of the Tokyo Medical and Dental University for animal care. IECs of anesthetized mice were imaged. Small intestinal tracts were surgically opened, immobilized on a microscope stage, and maintained. Then, images were obtained by a confocal laser microscopy. These procedures have been approved by the Committee of the Tokyo Medical and Dental University for animal care. Germ-free BALB/cA mice used as foster mothers were bred at the Laboratory of Veterinary Public Health, the University of Tokyo. All the germ-free animals were kept in flexible vinyl isolators in a room with 24°C, relative humidity of 60% and 12-h periods of light and dark and fed CMF-pelleted diet (Oriental Yeast Co., Tokyo, Japan) sterilized by γ-irradiation at dose of 50 kGy. For the generation of germ-free ubiquitous YC3.60 expression mice, in vitro fertilization and caesarean operation were performed as described below. Female ubiquitous YC3.60 expression mice were superovulated by intraperitoneal injection of 7.5 IU eCG followed by 7.5 IU hCG at an interval of 48 h. Eggs were collected from sacrificed female mice and fertilized with sperm of male ubiquitous YC3.60 expression mice in HTF medium (ARK Resource, Kumamoto, Japan). After over night culture in KSOM medium (ARK Resource), two-cell embryos were transferred into oviduct of pseudopregnant female ICR mice. Estimated delivery date was controlled by subcutaneous injection of Progehorrmon (Mochida Pharmaceutical Co., Ltd., Tokyo, Japan). The surrogate mothers were sacrificed at the fetal age of 21st day by cervical dislocation and “uterine package” was aseptically removed with clamps at the top of each uterine horn and the base of the uterus close to the cervix. The “uterine package” was introduced into isolator for operation through germicidal trap with 2% peracetic acid solution kept at 37°C. Then, “uterine package” was cut open with scissors and pups were taken out. The pups were stimulated breathing while cleaning them with dry gauze. After the pups started breathing normally, pups were transferred to the isolator with foster mothers. The germ-free status was check once a month. These procedures have been approved by the Committee for Care of Laboratory Animals in the Graduate School of Agricultural and Life Sciences at the University of Tokyo. There is no additional consideration.
Author Contributions
KH, NT, YA, NO, AM, SY, HK, and TA designed the research; TA and NT wrote the manuscript; KH, SK, YA, TU, NH, TK, YW, HK-N, SY, and TA performed the experiments, analyzed the data, and prepared the figures.
Conflict of Interest Statement
The authors declare that the research was conducted in the absence of any commercial or financial relationships that could be construed as a potential conflict of interest.
Acknowledgments
The authors are grateful to Dr. K. Rajewsky (MDC) for the CD19-Cre mice, Dr. M. Okabe (Osaka University) for the CAG-Cre mice, and Drs. K. Nishitani and M. Nishikawa (Takano Foods Co., Ltd.) for a B. subtilis strain TTCC12.
Funding
This work was supported in part by grants from the Ministry of Education, Culture, Sports, Science and Technology of Japan, the Futaba Electronics Memorial Foundation, the All Japan Coffee Association, the Mishima Kaiun Memorial, the Yamada Foundation (to TA), the Joint Usage/Research Program of Medical Research Institute, Tokyo Medical and Dental University (to SY, HK, AM, and NT), a Grant-in-Aid from Strategic International Collaborative Research Program (SICORP), the fund from Japan Society for the Promotion Science 15H04504 (JSPS), a Grant-in-Aid from Cross-ministerial Strategic Innovation Promotion Program (SIP), the Research Program on Hepatitis from Japan Agency for Medical Research and Development, AMED, Yakult Bio-Science Foundation, Takano Bio-Science Foundation, and the Canon Foundation (to NT).
Supplementary Material
The Supplementary Material for this article can be found online at http://journal.frontiersin.org/article/10.3389/fimmu.2016.00601/full#supplementary-material.
Video S1. Intravital Ca2+ signaling images mediated by Bacillus subtilis natto in the intestinal tract of a mouse under ubiquitous YC3.60 expression and specific pathogen-free conditions. B. subtilis natto in PBS (109 cells/ml) in PBS was added at the time point of 1 min 30 s. A rainbow parameter indicates relative Ca2+ concentration. The time course for fluorescence intensity of yellow fluorescent protein/cyan fluorescent protein on excitation at 458 nm was performed. Scale bar, 50 µm; frame = 151.
Video S2. Intravital Ca2+ signaling images mediated by Lactococcus lactis in the intestinal tract of a ubiquitous YC3.60 expression mouse under germ-free conditions. L. lactis in PBS (109 cells/ml) was added at the time point of 1 min 50 s. A rainbow parameter indicates relative Ca2+ concentration. The time course for fluorescence intensity of yellow fluorescent protein/cyan fluorescent protein on excitation at 458 nm was performed. Scale bar, 25 µm; frame = 145.
References
1. Mitsuoka T. Development of functional foods. Biosci Microbiota Food Health (2014) 33(3):117–28. doi: 10.12938/bmfh.33.117
2. van Baarlen P, Wells JM, Kleerebezem M. Regulation of intestinal homeostasis and immunity with probiotic lactobacilli. Trends Immunol (2013) 34(5):208–15. doi:10.1016/j.it.2013.01.005
3. Tsuji NM, Kosaka A. Oral tolerance: intestinal homeostasis and antigen-specific regulatory T cells. Trends Immunol (2008) 29(11):532–40. doi:10.1016/j.it.2008.09.002
4. Tsuji NM, Yan H, Watanabe Y. Gut to systemic immune-homeostasis mediated by innate signals. Nihon Rinsho Meneki Gakkai Kaishi (2015) 38(6):448–56. doi:10.2177/jsci.38.448
5. Yan H, Kakuta S, Nishihara M, Sugi M, Adachi Y, Ohno N, et al. Kjellmaniella crassifolia Miyabe (Gagome) extract modulates intestinal and systemic immune responses. Biosci Biotechnol Biochem (2011) 75(11):2178–83. doi:10.1271/bbb.110473
6. Yan H, Ohno N, Tsuji NM. The role of C-type lectin receptors in immune homeostasis. Int Immunopharmacol (2013) 16(3):353–7. doi:10.1016/j.intimp.2013.04.013
7. Hao WL, Lee YK. Microflora of the gastrointestinal tract: a review. Methods Mol Biol (2004) 268:491–502. doi:10.1385/1-59259-766-1:491
8. Suzuki K, Meek B, Doi Y, Muramatsu M, Chiba T, Honjo T, et al. Aberrant expansion of segmented filamentous bacteria in IgA-deficient gut. Proc Natl Acad Sci U S A (2004) 101(7):1981–6. doi:10.1073/pnas.0307317101
9. Mitsuoka T. Establishment of intestinal bacteriology. Biosci Microbiota Food Health (2014) 33(3):99–116. doi:10.12938/bmfh.33.99
10. Shida K, Nanno M. Probiotics and immunology: separating the wheat from the chaff. Trends Immunol (2008) 29(11):565–73. doi:10.1016/j.it.2008.07.011
11. Cerf-Bensussan N, Gaboriau-Routhiau V. The immune system and the gut microbiota: friends or foes? Nat Rev Immunol (2010) 10(10):735–44. doi:10.1038/nri2850
12. Kawashima T, Kosaka A, Yan H, Guo Z, Uchiyama R, Fukui R, et al. Double-stranded RNA of intestinal commensal but not pathogenic bacteria triggers production of protective interferon-beta. Immunity (2013) 38(6):1187–97. doi:10.1016/j.immuni.2013.02.024
13. Corr SC, Gahan CG, Hill C. Impact of selected Lactobacillus and Bifidobacterium species on Listeria monocytogenes infection and the mucosal immune response. FEMS Immunol Med Microbiol (2007) 50(3):380–8. doi:10.1111/j.1574-695X.2007.00264.x
14. Kawashima T, Hayashi K, Kosaka A, Kawashima M, Igarashi T, Tsutsui H, et al. Lactobacillus plantarum strain YU from fermented foods activates Th1 and protective immune responses. Int Immunopharmacol (2011) 11(12):2017–24. doi:10.1016/j.intimp.2011.08.013
15. Chiba E, Tomosada Y, Vizoso-Pinto MG, Salva S, Takahashi T, Tsukida K, et al. Immunobiotic Lactobacillus rhamnosus improves resistance of infant mice against respiratory syncytial virus infection. Int Immunopharmacol (2013) 17(2):373–82. doi:10.1016/j.intimp.2013.06.024
16. Kosaka A, Yan H, Ohashi S, Gotoh Y, Sato A, Tsutsui H, et al. Lactococcus lactis subsp. cremoris FC triggers IFN-gamma production from NK and T cells via IL-12 and IL-18. Int Immunopharmacol (2012) 14(4):729–33. doi:10.1016/j.intimp.2012.10.007
17. Tohno M, Shimosato T, Moue M, Aso H, Watanabe K, Kawai Y, et al. Toll-like receptor 2 and 9 are expressed and functional in gut-associated lymphoid tissues of presuckling newborn swine. Vet Res (2006) 37(6):791–812. doi:10.1051/vetres:2006036
18. Kimoto H, Kurisaki J, Tsuji NM, Ohmomo S, Okamoto T. Lactococci as probiotic strains: adhesion to human enterocyte-like Caco-2 cells and tolerance to low pH and bile. Lett Appl Microbiol (1999) 29(5):313–6. doi:10.1046/j.1365-2672.1999.00627.x
19. Thomas CM, Versalovic J. Probiotics-host communication: modulation of signaling pathways in the intestine. Gut Microbes (2010) 1(3):148–63. doi:10.4161/gmic.1.3.11712
20. Scharenberg AM, Humphries LA, Rawlings DJ. Calcium signalling and cell-fate choice in B cells. Nat Rev Immunol (2007) 7(10):778–89. doi:10.1038/nri2172
21. Oh-hora M, Rao A. Calcium signaling in lymphocytes. Curr Opin Immunol (2008) 20(3):250–8. doi:10.1016/j.coi.2008.04.004
22. Kurosaki T, Shinohara H, Baba Y. B cell signaling and fate decision. Annu Rev Immunol (2010) 28:21–55. doi:10.1146/annurev.immunol.021908.132541
23. Feske S. Calcium signalling in lymphocyte activation and disease. Nat Rev Immunol (2007) 7(9):690–702. doi:10.1038/nri2152
24. Engelke M, Engels N, Dittmann K, Stork B, Wienands J. Ca(2+) signaling in antigen receptor-activated B lymphocytes. Immunol Rev (2007) 218:235–46. doi:10.1111/j.1600-065X.2007.00539.x
25. Yoshikawa S, Usami T, Kikuta J, Ishii M, Sasano T, Sugiyama K, et al. Intravital imaging of Ca(2+) signals in lymphocytes of Ca(2+) biosensor transgenic mice: indication of autoimmune diseases before the pathological onset. Sci Rep (2016) 6:18738. doi:10.1038/srep18738
26. Nagai T, Yamada S, Tominaga T, Ichikawa M, Miyawaki A. Expanded dynamic range of fluorescent indicators for Ca(2+) by circularly permuted yellow fluorescent proteins. Proc Natl Acad Sci U S A (2004) 101(29):10554–9. doi:10.1073/pnas.0400417101
27. Rickert RC, Roes J, Rajewsky K. B lymphocyte-specific, Cre-mediated mutagenesis in mice. Nucleic Acids Res (1997) 25(6):1317–8. doi:10.1093/nar/25.6.1317
28. Matsumura H, Hasuwa H, Inoue N, Ikawa M, Okabe M. Lineage-specific cell disruption in living mice by Cre-mediated expression of diphtheria toxin A chain. Biochem Biophys Res Commun (2004) 321(2):275–9. doi:10.1016/j.bbrc.2004.06.139
29. Tsuji MN, Kimoto H. Lactic acid bacteria and their cellular components inducing immunoregulatory function, and method of obtaining the same. EP 1538198 A2 (2005).
30. Gerbe F, Legraverend C, Jay P. The intestinal epithelium tuft cells: specification and function. Cell Mol Life Sci (2012) 69(17):2907–17. doi:10.1007/s00018-012-0984-7
31. Howitt MR, Lavoie S, Michaud M, Blum AM, Tran SV, Weinstock JV, et al. Tuft cells, taste-chemosensory cells, orchestrate parasite type 2 immunity in the gut. Science (2016) 351(6279):1329–33. doi:10.1126/science.aaf1648
32. Hosoi T, Ametani A, Kiuchi K, Kaminogawa S. Improved growth and viability of lactobacilli in the presence of Bacillus subtilis (natto), catalase, or subtilisin. Can J Microbiol (2000) 46(10):892–7. doi:10.1139/cjm-46-10-892
33. Kim S, Yang JY, Lee K, Oh KH, Gi M, Kim JM, et al. Bacillus subtilis-specific poly-gamma-glutamic acid regulates development pathways of naive CD4(+) T cells through antigen-presenting cell-dependent and -independent mechanisms. Int Immunol (2009) 21(8):977–90. doi:10.1093/intimm/dxp065
34. Ozawa N, Shimojo N, Suzuki Y, Ochiai S, Nakano T, Morita Y, et al. Maternal intake of natto, a Japan’s traditional fermented soybean food, during pregnancy and the risk of eczema in Japanese babies. Allergol Int (2014) 63(2):261–6. doi:10.2332/allergolint.13-OA-0613
35. Rescigno M. Dendritic cell-epithelial cell crosstalk in the gut. Immunol Rev (2014) 260(1):118–28. doi:10.1111/imr.12181
36. Lavelle EC, Murphy C, O’Neill LA, Creagh EM. The role of TLRs, NLRs, and RLRs in mucosal innate immunity and homeostasis. Mucosal Immunol (2010) 3(1):17–28. doi:10.1038/mi.2009.124
37. Lundin A, Bok CM, Aronsson L, Bjorkholm B, Gustafsson JA, Pott S, et al. Gut flora, toll-like receptors and nuclear receptors: a tripartite communication that tunes innate immunity in large intestine. Cell Microbiol (2008) 10(5):1093–103. doi:10.1111/j.1462-5822.2007.01108.x
38. Hosoya S, Villena J, Chiba E, Shimazu T, Suda Y, Aso H, et al. Advanced application of porcine intestinal epithelial cells for the selection of immunobiotics modulating toll-like receptor 3-mediated inflammation. J Microbiol Immunol Infect (2013) 46(6):474–81. doi:10.1016/j.jmii.2012.04.005
39. Shimazu T, Villena J, Tohno M, Fujie H, Hosoya S, Shimosato T, et al. Immunobiotic Lactobacillus jensenii elicits anti-inflammatory activity in porcine intestinal epithelial cells by modulating negative regulators of the Toll-like receptor signaling pathway. Infect Immun (2012) 80(1):276–88. doi:10.1128/IAI.05729-11
40. El Aidy S, van den Bogert B, Kleerebezem M. The small intestine microbiota, nutritional modulation and relevance for health. Curr Opin Biotechnol (2015) 32:14–20. doi:10.1016/j.copbio.2014.09.005
41. Michallet MC, Rota G, Maslowski K, Guarda G. Innate receptors for adaptive immunity. Curr Opin Microbiol (2013) 16(3):296–302. doi:10.1016/j.mib.2013.04.003
42. Honda K, Littman DR. The microbiome in infectious disease and inflammation. Annu Rev Immunol (2012) 30:759–95. doi:10.1146/annurev-immunol-020711-074937
Keywords: probiotic, Ca2+ signaling, intestinal epithelial cell, intravital imaging, Lactococcus, Bacillus subtilis, small intestine, germ-free mouse
Citation: Adachi T, Kakuta S, Aihara Y, Kamiya T, Watanabe Y, Osakabe N, Hazato N, Miyawaki A, Yoshikawa S, Usami T, Karasuyama H, Kimoto-Nira H, Hirayama K and Tsuji NM (2016) Visualization of Probiotic-Mediated Ca2+ Signaling in Intestinal Epithelial Cells In Vivo. Front. Immunol. 7:601. doi: 10.3389/fimmu.2016.00601
Received: 05 October 2016; Accepted: 30 November 2016;
Published: 16 December 2016
Edited by:
Haruki Kitazawa, Tohoku University, JapanCopyright: © 2016 Adachi, Kakuta, Aihara, Kamiya, Watanabe, Osakabe, Hazato, Miyawaki, Yoshikawa, Usami, Karasuyama, Kimoto-Nira, Hirayama and Tsuji. This is an open-access article distributed under the terms of the Creative Commons Attribution License (CC BY). The use, distribution or reproduction in other forums is permitted, provided the original author(s) or licensor are credited and that the original publication in this journal is cited, in accordance with accepted academic practice. No use, distribution or reproduction is permitted which does not comply with these terms.
*Correspondence: Takahiro Adachi, tadachi.imm@mri.tmd.ac.jp;
Kazuhiro Hirayama, akazu@mail.ecc.u-tokyo.ac.jp;
Noriko M. Tsuji, nm-tsuji@aist.go.jp