- 1Univ. Lille, U1019 – UMR 8204 – CIIL – Centre d’Infection et d’Immunité de Lille, Lille, France
- 2Centre National de la Recherche Scientifique, UMR 8204, Lille, France
- 3Institut National de la Santé et de la Recherche Médicale U1019, Lille, France
- 4Hospitalier Universitaire de Lille, Lille, France
- 5Institut Pasteur de Lille, Lille, France
- 6Translational Nanobiomaterials and Imaging, Department of Radiology, Leiden University Medical Center, Leiden, Netherlands
Type I natural killer T (NKT) cells have gained considerable interest in anticancer immune therapy over the last decade. This “innate-like” T lymphocyte subset has the unique ability to recognize foreign and self-derived glycolipid antigens in association with the CD1d molecule expressed by antigen-presenting cells. An important property of these cells is to bridge innate and acquired immune responses. The adjuvant function of NKT cells might be exploited in the clinics. In this review, we discuss the approaches currently being used to target NKT cells for cancer therapy. In particular, we highlight ongoing strategies utilizing NKT cell-based nanovaccines to optimize immune therapy.
Introduction
Invariant or type I natural killer T cells (referred as NKT cells) represent a highly conserved subset of non-conventional T lymphocytes endowed with a remarkably broad range of immune effector and regulatory functions. These cells recognize foreign and self-derived glycolipid antigens presented by the monomorphic MHC/HLA class I-like molecule CD1d expressed by antigen-presenting cells, including dendritic cells (DCs) [for reviews, Ref. (1–5)]. NKT cells express on their surface a semi-invariant T cell receptor (TCR) composed by a unique TCR-α chain paired with a restricted number of β-chains. Rapidly after natural activation (inflammation, infection), NKT cells produce huge amounts of cytokines including T helper (Th)1-like (INF-γ), Th2-like (IL-4), Th17-like (IL-17, IL-22), and regulatory (IL-10) cytokines. This flexibility depends on the mode of stimulation, on the location and on the NKT cell subset challenged. Of note, NKT cells can be activated by direct TCR triggering and also via cytokines, without TCR engagement (5). With their ability to swiftly release cytokines, NKT cells have also the potential to lyse cellular targets following TCR recognition of lipid antigens (6). This property is important in immune surveillance against tumor cells and could be exploited for immune-based therapy. The role of NKT cells in various pathologies including cancer, infection, acute, and chronic inflammation and autoimmune diseases has been evidenced in experimental models and in humans (5). Along with their natural (beneficial or detrimental) role in pathological settings, NKT cells can also be manipulated by means of specific CD1d-restricted ligands. For instance, exposure of antigen-presenting cells to α-galactosylceramide (α-GalCer) triggers potent innate and acquired immune responses. Of particular interest is the exquisite capacity of NKT cells to promote DC maturation and, as a consequence, to trigger potent T and B cell responses (7). This unique property, and given that the CD1d/NKT axis is conserved in humans (with no HLA restriction), could be used in clinical situations, including cancer. There is a strong interest to exploit the adjuvant effects of α-GalCer or related glycolipid derivatives to develop more efficient NKT cell-based vaccines (8–10). We herein review the effects of α-GalCer in preclinical and clinical studies and discuss ongoing and future strategies that aim to optimize NKT cell-based antitumor therapy with a particular focus on nanovector delivery systems. These systems, particularly those allowing encapsulation of tumor antigens and α-GalCer derivatives (adjuvant), might realize maximal therapeutic benefit with minimal toxicity.
Free α-GalCer in Antitumor Therapy: from Preclinical Studies to Clinical Development
Alpha-GalCer is a marine sponge-derived glycosphingolipid originally discovered in a screen for antitumor compounds (11, 12). This seminal discovery has led to the development of synthetic α-GalCer derivatives as a family of powerful glycolipid agonists for NKT cells in order to promote protective immune responses against infections and cancers (13–15). α-GalCer triggers a mixed response by NKT cells including the production of IFN-γ, a cytokine important in tumor immune surveillance and inhibition of angiogenesis. Different agonists with Th1-promoting functions (which appear to be more adapted for anticancer therapies) have been described (13, 16). Preclinical studies have highlighted the potent antitumor effect of α-GalCer and α-GalCer derivatives against solid tumors (sarcoma, melanoma and colon, prostate, and lung carcinoma) and hematological malignancies (lymphoma) (12, 17–21). Mechanisms involved include early production of IFN-γ by NKT cells and NK cells and secretion of IL-12 by DCs (20). This success has led to clinical trials in patients with advanced lung cancer. Free soluble α-GalCer was used. Unfortunately, no or low clinical benefits were reported among patients (22–24). These disappointing results might be due to the lower number of NKT cells in patients relative to healthy individuals and/or to their diminished (but reversible) activation threshold capacity (22–32). Hence, one concern in NKT cell-based therapy is the diminished NKT cell count and/or function, although this cannot be generalized to all advanced cancer patients. Various means of circumventing this potential drawback are being developed including infusion of autologous ex vivo-expanded NKT cells. This approach can lead to clinically relevant antitumor responses (33–39). In vivo transfer of NKT cells expressing chimeric antigen receptor in order to redirect their cytotoxicity against tumor cells has also been explored in preclinical studies. This approach may provide potent antitumor activity (40, 41). Moreover, the reprogramming of NKT cells to induced pluripotent stem cells and their subsequent re-differentiation into more functional NKT cells (compared with the parental cells) is opening up new avenues in this field (42, 43). Another reason that might explain disappointing clinical data relates to the uncontrolled delivery of α-GalCer, which might lead to suboptimal primary and secondary activation of NKT cells. This later issue prompted researchers to inoculate α-GalCer in a vectorized (cellular or acellular systems) form in order to better control the delivery of the active principle and to generate more efficient innate and acquired immune-based antitumor responses.
Vectorization of α-GalCer in Cellular Systems
Cellular systems in which α-GalCer is incorporated can act as potent (NKT cell-based) cellular adjuvants. As described below, these cellular systems include DCs, non-antigen presenting cells, and cancer cells. Studies in mice have demonstrated that α-GalCer loaded in DCs has a higher ability to activate NKT cells and to trigger antitumor responses relative to α-GalCer injected in a free (non-vectorized) form (18, 44). In the same line, adoptive transfer of α-GalCer-loaded autologous peripheral blood mononuclear cells or DCs induced clinical benefits in some patients (lung cancer and head and neck cancer), an effect that correlates with IFN-γ production (23, 33, 34, 36, 45–49). Of note, adoptive transfer of autologous NKT cells along with α-GalCer-pulsed mononuclear cells or DCs led to encouraging clinical results in term of prolonged median overall survival time (35, 36, 50). This effect was associated with a significant infiltration of NKT cells into the tumor (36) Hence, this combination therapy led to significant clinical efficacy, although technical and economic issues still persist.
Taniguchi’s group was the first to exploit artificial adjuvant vectors (aAVCs) to enhance NKT cell-based antitumor responses (8). This system can induce both innate and long-term memory CD8+ T cell responses against cancer. For instance, inoculation (single dose) of allogeneic fibroblasts (used as a vector cell) into which tumor antigen mRNA and CD1d with α-GalCer were introduced led to a long-lasting antitumor response (51–54). The same group has designed a human aAVC consisting of embryonic kidney cells transfected with the human melanoma MART-1 antigen and CD1d and pulsed with α-GalCer. This cellular system promoted antitumor response in humanized mice (55). Mechanistically, it is likely that allogeneic cells are selectively taken up by DCs and that the subsequent cross-presentation of tumor antigens to CD8+ T cells and α-GalCer to NKT cell is critical in the promotion of strong and long-lasting tumor-specific cytotoxic CD8+ T lymphocytes (CTL) responses.
Tumor cells are rich sources of tumor antigens. However, due to the low immunogenicity of tumor antigens, combined adjuvants are requisite in order to develop cancer vaccines. Shimizu and collaborators were the first to evaluate the capacity of α-GalCer-pulsed tumor cells (melanoma) to act as a cellular adjuvant (56). Numerous studies have validated the efficacy of this strategy in therapeutical settings in the mouse system (solid tumor and hematological malignancies) (57–66). Mechanistically, inoculated α-GalCer-pulsed tumor cells are selectively taken up by DCs (as for aAVC), which have a unique capacity to cross-present antigens from dying cells. It is also possible that the killing of CD1d-expressing tumor cells by activated NKT cells leads to the release of tumor antigens and to their subsequent cross-presentation by DCs. Whatever the mechanism, it is likely that the presentation of both α-GalCer and tumor antigens by the same DC is critical in the development of the protective tumor-specific CTL-based antitumor response. Whether this strategy could be exploited in the human setting to harness cancer progression and recurrence, without inducing autoimmunity, is still unknown. Cooperative action of toll-like receptor (TLR) ligands and iNKT cells on DC function is a well-recognized phenomenon (67). Of interest, relative to inoculation of α-GalCer-loaded tumor cells alone, coadministration of α-GalCer-loaded tumor cells and TLR9 agonists augments the antitumor response (66).
Introduction of α-GalCer and tumor antigens in antigen-presenting cells has also been attempted in preclinical models. DCs expressing the mammary tumor-associated antigen Her-2 and pulsed with α-GalCer trigger potent antitumor responses (68). The use of different models of tumors revealed that this strategy was effective both in prophylactic and therapeutic settings (69). Of interest, vaccination with DCs transduced with OVA (used here as a model tumor antigen) plus CCL21, a chemokine that attracts both T cells and NKT cells, protects against OVA-expressing tumors (70). Finally, human embryonic stem cell-derived DCs genetically engineered to express CD1d can prime CD8+ T cells against tumor antigens (71). The potential benefit of this latter strategy in cancer immunotherapy is being studied. In conclusion, cell-based vaccines to optimize α-GalCer activity in vivo are promising although technical, logistical, and financial difficulties might limit the development of such vaccines.
Vectorization of α-GalCer in Acellular Systems
Definition of Nanovectors
Development of nanovectors (<1 μm) holds great potential for cancer immunotherapy, including antitumor vaccines (72–74). The interest of using nanosized carriers able to incorporate α-GalCer (with or without tumor antigen) to optimize NKT cell-based anticancer therapy has recently emerged. Encapsulation of α-GalCer into nanovectors might offer several advantages relative to soluble α-GalCer. This includes preferential internalization by antigen-presenting cells (due to the size), slower and sustained release of α-GalCer in CD1d-containing endosomes, and minimal side effects (due to the lower amount required for a similar biological effect). Moreover, compared to cell-based vectorization, nanovectors are less invasive and costly (no adoptive transfer). Nanovectors offer the unique opportunity to deliver both adjuvant (including α-GalCer) and tumor antigens to the same antigen-presenting cells, especially DCs (75–77). Nanovectors represent an interesting class of delivery vehicles able to induce potent and long-lasting immune responses (78, 79). Surprisingly enough, few studies have exploited this unique property to enhance the antitumor functions of NKT cells.
Nanovectors include a multiple range of particulate systems including (among others) virus-like particles, dendrimers, silica microspheres, micelles, nanogels, nanoemulsions, liposomes, carbon nanotubes, metallic nanoparticles, and polymeric nanoparticles, which include nanospheres and nanocapsules (Table 1 and not shown). The physical properties as well as the advantages and drawbacks of nanovectors are presented in Table 1. For vaccine development, a major goal is to target DCs. Uptake of nanovectors by DCs depends on several physicochemical properties including the size, shape, surface charge, hydrophobicity, and hydrophilicity of nanovectors. To target more selectively DCs, it is possible to arm nanovectors with ligands or antibodies on their surface. Among the different delivery systems for antigen encapsulation in vaccines, particularly for cancer therapy, polymeric nanoparticles have many advantages including low toxicity, high biodegradability, amenability to controlled release of the bioactive agents (antigen and adjuvant), preservation of their stability, and potential for surface functionalization (79, 80). Currently, there is a long list of polymers used to produce nanovectors including plasma albumin, chitosan, polyethyleneimine, polylactic acid, and polylactic-coglycolic acid (PLGA). PLGA is one of the most successful biocompatible and biodegradable polymers (approved for in vivo use by the United States Food and Drug Administration). PLGA-based nanoparticle systems are particularly interesting since they allow high antigen density, incorporation of different classes of molecules including proteins and lipids, ability to reach MHC I pathway after uptake by DCs, and slow release kinetics delivery (75, 81–85). Attempts have been made to incorporate α-GalCer in nanosized vectors, with or without tumor antigens (Table 2). Here, we detail the effect of vectorized α-GalCer in innate and acquired immune-based antitumor responses.
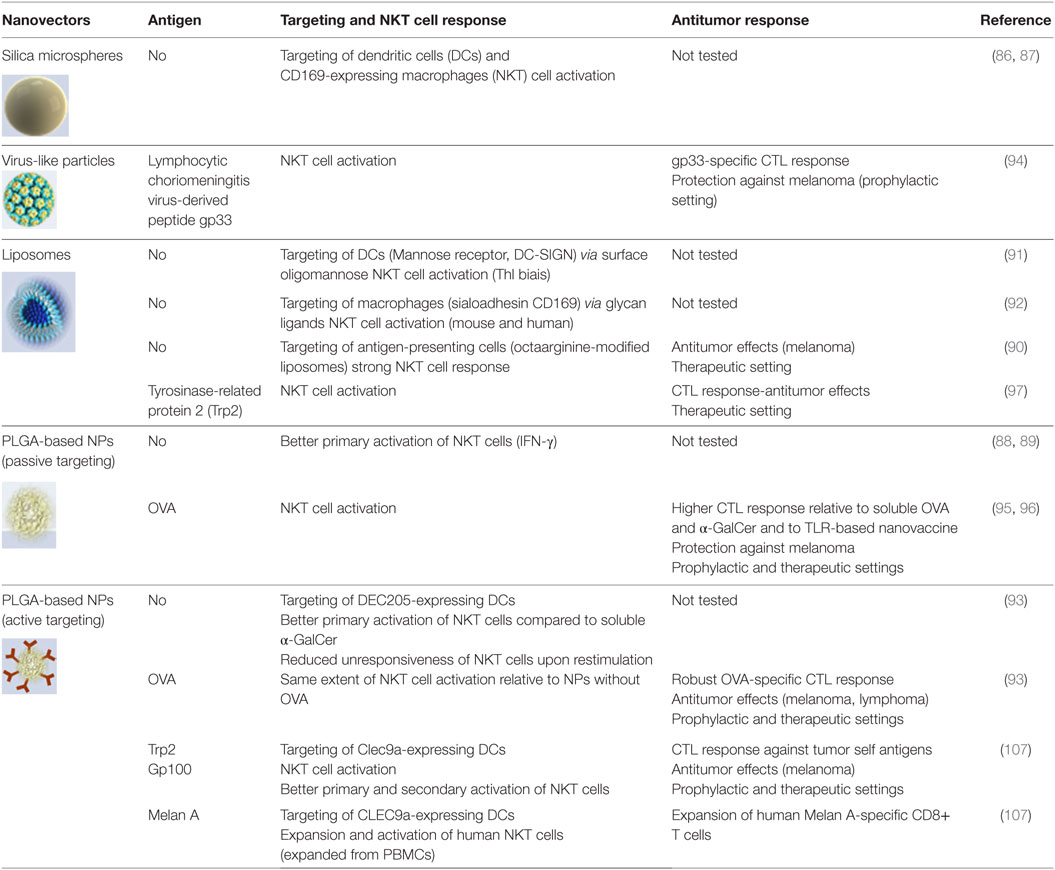
Table 2. Utilization of α-GalCer-encapsulated nanovectors to promote NKT cell activation and antitumor responses.
Vectorization of α-GalCer without Tumor Antigen
Preclinical studies suggest that α-GalCer vectorized in nanovectors is of potential interest. This relies mainly on passive (untargeted) and active (targeted) delivery of α-GalCer to antigen-presenting cells. For instance, silica microspheres coated with lipid bilayers plus α-GalCer target mouse CD169-expressing macrophages and DCs, both cell types being critical in the primary activation of NKT cells (86, 87). Others and we have demonstrated that PLGA-based nanoparticles are internalized by DCs to promote NKT cell activation (88, 89). Another study has shown that α-GalCer incorporated in octaarginine-modified liposomes are passively taken up by antigen-presenting cells and strongly activate NKT cells. This leads to therapeutic protection against B16F10 lung metastases (90). In order to optimize the targeting of α-GalCer to antigen-presenting cells, nanovectors can be armed with ligands or antibodies that bind to specific markers. For instance, liposomes decorated with oligomannose that binds to mannose receptor and DC-SIGN target DCs in vivo and potently stimulate NKT cells toward a Th1 direction (91). Encapsulating α-GalCer in liposomes bearing on their surface glycans specific for the sialoadhesin CD169 strongly activates NKT cells in vivo (92). Our recent data demonstrate that, relative to non-vectorized α-GalCer, α-GalCer incorporated into antibody-armed PLGA nanoparticles that target DCs increases NKT cell-based innate immune responses (93).
Vectorization of α-GalCer and Tumor Antigens
Passive (Untargeted) Delivery
Very few studies have been devoted so far to study the potential benefit of encapsulating α-GalCer and tumor antigens in nanosized vectors. A pioneer study from McKee and colleagues analyzed the consequences of α-GalCer and antigen co-encapsulation in antitumor responses (94). In this work, α-GalCer and the gp33 peptide derived from lymphocytic choriomeningitis virus (used as a model antigen) were incorporated into virus-like particles. This composite particle system induced a 10-fold more active gp33-specific CTL response, compared to free α-GalCer and gp33, and prophylactically protected against gp33-expressing melanoma. Mechanistically, it is likely that α-GalCer and gp33 are delivered in the endosomal compartment of antigen-presenting cells to load to CD1d and MHC Class I, respectively, thus favoring cross-presentation by DCs. Dölen and collaborators have recently demonstrated that encapsulating α-GalCer and OVA in PLGA-based nanoparticles is efficient to trigger antitumor responses (95). Of interest was the observation that the response was superior compared to TLR agonist and OVA co-encapsulation. More recently, using a similar strategy, Li and colleagues showed that the immune responses triggered by α-GalCer and OVA encapsulated in PLGA nanoparticles was longer compared to that induced by its soluble counterparts (96). Of note, both intranasal and intraperitoneal injection of nanovaccine triggered robust antigen-specific CD8+ T cell response. Of interest, Neumann and colleagues investigated the effect of α-GalCer and tumor antigens co-delivery on antitumor responses using a cationic liposome (97). The self-antigen tyrosinase-related protein 2 (Trp2) was used. The authors found that the liposomal formulation elicits potent antigen-specific CTL response and prevents tumor progression in a therapeutic setting. Collectively, encapsulation of α-GalCer and tumor antigens in nanovectors, including liposomes and PLGA NPs (Table 2), elicits antitumor responses in experimental models. In these settings (passive delivery), DCs and probably other antigen-presenting cells are critically important.
Active (Targeted) Delivery in DCs
Our work was the first to investigate the consequences of active α-GalCer and tumor antigen delivery to DCs by means of multifunctional nanovectors. In light of the literature showing the unique ability of cross-priming DCs (CD8α+ DCs and BDCA3+ DCs in the mouse and human system, respectively) to initiate and maintain CTL responses (98–101), we decided to target this DC subset. Moreover, we and others showed that CD8α+ DCs are very potent to stimulate primary and secondary NKT cell activation (93, 102). Finally, the fact that NKT cells can substitute “classical” CD4+ Th cells to license the DCs for cross-priming represents another reason explaining our devised strategy (103). Since cross-priming DCs express specific markers on their surface, we armed PLGA-based nanoparticles with antibodies in order to target these cells in vivo. Although DEC205 is not entirely specific for cross-priming CD8α+ DCs, nanoparticles armed with anti-DEC205 antibodies and carrying both α-GalCer and OVA successfully led to antigen cross-presentation and to potent antitumor responses (93). Of interest, this strategy also led to a long lasting antigen-specific antibody response. The C-type lectin Clec9a (also known as DNGR1) is almost exclusively expressed by cross-priming mouse and human DCs and is known to confer potent CTL responses (104–106). Our recent data indicate that PLGA-based nanoparticles armed with anti-Clec9a antibodies and incorporating both α-GalCer and OVA can confer protection against OVA-expressing tumors (lymphoma) (107). We also investigated whether our vectorization/targeting strategy might break tolerance to tumor self-antigens, an important challenge for optimal antitumor therapy [for reviews see Ref. (108–112)]. Indeed, co-incorporation of α-GalCer and tumor melanoma-derived self-antigens (including Trp2) triggered a potent CD8+ T cell-mediated antitumor response (107). Hence, our vaccine strategy, probably by enhancing DC/NKT cell/naive CD8+ T cell interactions (Figure 1), abrogates self-tolerance and promotes effective antitumor CTL responses. Signals incorporated by DCs are critical to shape the functions of naive T lymphocytes, including CD8+ T cells. Because maturation processes of DCs due to direct innate sensor (such as TLR) signaling might be different to those triggered by NKT cells, it would be interesting to compare the efficacy of TLR-based and NKT cell-based targeted nanovaccines in cancer therapies (Figure 2). Co-administration of soluble α-GalCer and TLR agonists with antigen was shown to enhance the CD8+ T cell response with augmented effect on tumor progression, relative to antigen mixed with an adjuvant alone (113). Therefore, encapsulation of both TLR ligands and α-GalCer derivatives in antibody-armed nanovectors might additively or synergistically enhance the responses, a hypothesis that needs further investigations.
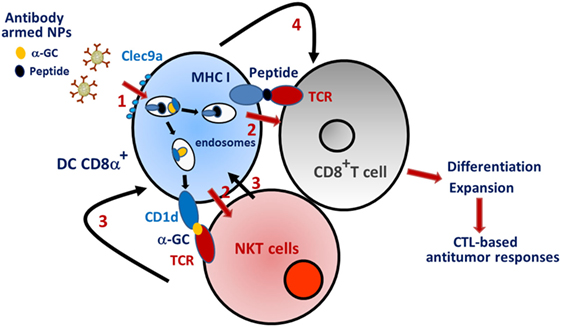
Figure 1. Schematic “ménage à trois” between CD8α+ DC, natural killer T (NKT) cells, and naive CD8+ T cells. (1) Anti-Clec9a-armed nanoparticles that carry α-GalCer and tumor antigen are taken up by CD8α+ DC via the endocytic receptor Clec9a. (2) The active components are delivered in the endosomes and presented via MHC class I (peptide) and CD1d (α-GalCer) to naïve CD8+ T cells and NKT cells, respectively. (3) In response to TCR triggering, NKT cells activate the maturation of CD8α+ dendritic cells (DCs) through cytokines and costimulatory (CD40) molecules. (4) Mature DCs transmit signals to naïve CD8+ T cells, which, in turn, differentiate into CTLs. (5) CTLs destroy tumor cells. Note that α-GalCer is acquired and presented by DCs that are also actively engaged in presenting peptides to T cells. This scheme does not consider reciprocal interactions between NKT cells and CD8+ T cells.
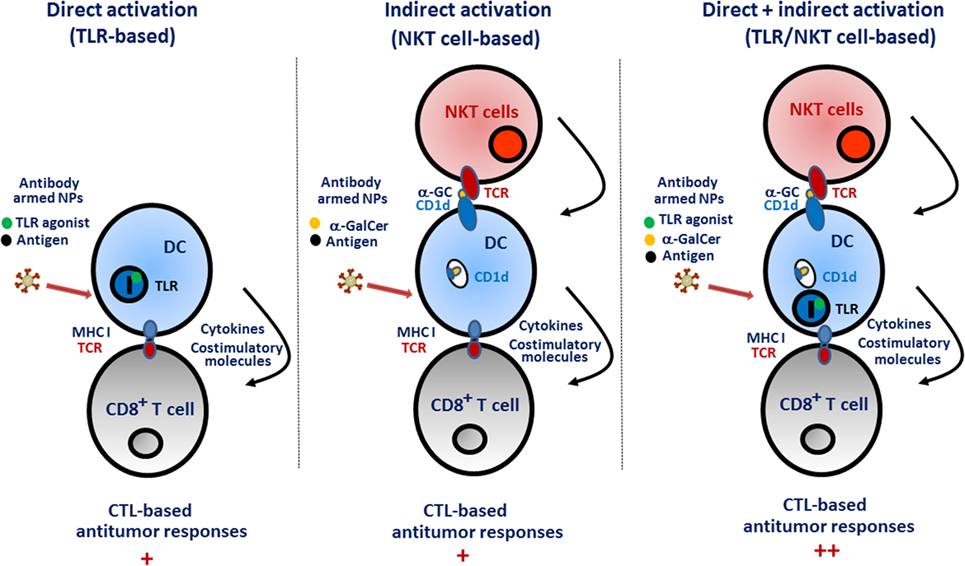
Figure 2. Promotion of CD8+ T cell responses upon direct [toll-like receptor (TLR)-based] and/or indirect [natural killer T (NKT) cell based] dendritic cell (DC) activation. Direct activation. Nanoparticles bearing TLR agonists are internalized by DCs (i.e., those that excel in cross-presentation) and activate endosomal TLRs (such as TLR3, TLR7/8, or TLR9). This rapidly leads to DC maturation and to the production of inflammatory cytokines and costimulatory molecules that in turn promote the differentiation and expansion of naïve CD8+ T cells. Indirect activation. In this setting, the delivery of α-GalCer in DCs leads to the exposition of the glycolipid on the cell surface in association with the CD1d molecule (at this stage, the DC is still immature). TCR triggering in NKT cells leads to the release of cytokines and to the expression of costimulatory (CD40) molecules culminating in DC maturation. In turn, mature DCs activate naïve CD8+ T cells. Direct and indirect activation. One may suppose that the two effects are additive, or even synergistic, to promote optimal CD8+ T cell responses that control tumor progression.
Concluding Remarks and Future Perspectives
Growing evidences demonstrate that α-GalCer (or α-GalCer derivatives) might be successfully used in cancer therapy. However, innovative strategies to better manipulate the adjuvant properties and the antitumor potentials of NKT cells are required. Among them, optimization of delivery systems that contain α-GalCer and tumor antigens to optimally activate NKT cell-based immune responses remains an important goal. Cell-based vaccines that promote strong and long-lasting CTL responses offer an interesting immunotherapeutic strategy for the future although concerns still exist (cost, invasive procedure). Nanovectors that passively or actively target (cross-priming) DCs are also of clinical interest. Future studies will aim to enhance the efficacy of delivery systems in order to improve cell targeting and to optimize the delivery of the active principles (α-GalCer and tumor antigens) in the right cellular compartment. Such development will require the use of more sophisticated nanovectors to improve surgical strikes and possibly the targeting of other (DC expressed) specific molecules. Complementary approaches including strategies that boost the number/function of NKT cells in patients (transfer of functional NKT cells) and/or that aim to control immune suppression (e.g., check point blockers, immunomodulatory drugs) are of interest. Moreover, combination of NKT cell and TLR agonists might amplify the strength and the quality of the immune response in patients. An important area for future research is the development of humanized mouse models to accurately replicate the NKT cell response in humans. It is likely that, in a near future, the use of nanovector-based medicine will optimize antitumor responses for the sake of cancer patients, in combination with conventional immunotherapy.
Author Contributions
All authors listed have made a substantial, direct, and intellectual contribution to the work and approved it for publication.
Conflict of Interest Statement
The authors declare that the research was conducted in the absence of any commercial or financial relationships that could be construed as a potential conflict of interest.
Acknowledgments
We apologize to colleagues whose works could not be cited due to space constraints.
Funding
This work was supported by the INSERM, the CNRS, the University of Lille Nord de France, the Pasteur Institute of Lille, and the Institut National du Cancer (INCa, projets libres) under reference R08046EE/RPT08003EEA and R13071EE/RPT13001EEA.
Abbreviations
NKT, natural killer T; DCs, dendritic cells; TCR, T cell receptor; α-GalCer, α-galactosylceramide; CAR, chimeric antigen receptor; aAVC, artificial adjuvant vector; PLGA, polylactic-coglycolic acid; CTL, cytotoxic CD8+ T lymphocyte; TLR, toll-like receptor; Trp2, tyrosinase-related protein 2.
References
1. Taniguchi M, Harada M, Kojo S, Nakayama T, Wakao H. The regulatory role of Valpha14 NKT cells in innate and acquired immune response. Annu Rev Immunol (2003) 21:483–513. doi:10.1146/annurev.immunol.21.120601.141057
2. Bendelac A, Savage PB, Teyton L. The biology of NKT cells. Annu Rev Immunol (2007) 25:297–336. doi:10.1146/annurev.immunol.25.022106.141711
3. Berzins SP, Smyth MJ, Baxter AG. Presumed guilty: natural killer T cell defects and human disease. Nat Rev Immunol (2011) 11:131–42. doi:10.1038/nri2904
4. Rossjohn J, Pellicci DG, Patel O, Gapin L, Godfrey DI. Recognition of CD1d-restricted antigens by natural killer T cells. Nat Rev Immunol (2012) 12:845–57. doi:10.1038/nri3328
5. Brennan PJ, Brigl M, Brenner MB. Invariant natural killer T cells: an innate activation scheme linked to diverse effector functions. Nat Rev Immunol (2013) 13:101–17. doi:10.1038/nri3369
6. Metelitsa LS. Anti-tumor potential of type-I NKT cells against CD1d-positive and CD1d-negative tumors in humans. Clin Immunol (2011) 140:119–29. doi:10.1016/j.clim.2010.10.005
7. Hermans IF, Silk JD, Gileadi U, Salio M, Mathew B, Ritter G, et al. NKT cells enhance CD4+ and CD8+ T cell responses to soluble antigen in vivo through direct interaction with dendritic cells. J Immunol (2003) 171:5140–7. doi:10.4049/jimmunol.171.10.5140
8. Fujii S-I, Shimizu K, Okamoto Y, Kunii N, Nakayama T, Motohashi S, et al. NKT cells as an ideal anti-tumor immunotherapeutic. Front Immunol (2013) 4:409. doi:10.3389/fimmu.2013.00409
9. Cerundolo V, Silk JD, Masri SH, Salio M. Harnessing invariant NKT cells in vaccination strategies. Nat Rev Immunol (2009) 9:28–38. doi:10.1038/nri2451
10. Mattarollo SR, Smyth MJ. NKT cell adjuvants in therapeutic vaccines against hematological cancers. Oncoimmunology (2013) 2:e22615. doi:10.4161/onci.22615
11. Kobayashi E, Motoki K, Uchida T, Fukushima H, Koezuka Y. KRN7000, a novel immunomodulator, and its antitumor activities. Oncol Res (1995) 7:529–34.
12. Kawano T, Cui J, Koezuka Y, Toura I, Kaneko Y, Motoki K, et al. CD1d-restricted and TCR-mediated activation of valpha14 NKT cells by glycosylceramides. Science (1997) 278:1626–9. doi:10.1126/science.278.5343.1626
13. Carreño LJ, Saavedra-Ávila NA, Porcelli SA. Synthetic glycolipid activators of natural killer T cells as immunotherapeutic agents. Clin Transl Immunology (2016) 5:e69. doi:10.1038/cti.2016.14
14. Kharkwal SS, Arora P, Porcelli SA. Glycolipid activators of invariant NKT cells as vaccine adjuvants. Immunogenetics (2016) 68:597–610. doi:10.1007/s00251-016-0925-y
15. East JE, Kennedy AJ, Webb TJ. Raising the roof: the preferential pharmacological stimulation of Th1 and th2 responses mediated by NKT cells. Med Res Rev (2014) 34:45–76. doi:10.1002/med.21276
16. Li X, Fujio M, Imamura M, Wu D, Vasan S, Wong C-H, et al. Design of a potent CD1d-binding NKT cell ligand as a vaccine adjuvant. Proc Natl Acad Sci U S A (2010) 107:13010–5. doi:10.1073/pnas.1006662107
17. Nakagawa R, Motoki K, Ueno H, Iijima R, Nakamura H, Kobayashi E, et al. Treatment of hepatic metastasis of the colon26 adenocarcinoma with an alpha-galactosylceramide, KRN7000. Cancer Res (1998) 58:1202–7.
18. Toura I, Kawano T, Akutsu Y, Nakayama T, Ochiai T, Taniguchi M. Cutting edge: inhibition of experimental tumor metastasis by dendritic cells pulsed with alpha-galactosylceramide. J Immunol (1999) 163:2387–91.
19. Shin T, Nakayama T, Akutsu Y, Motohashi S, Shibata Y, Harada M, et al. Inhibition of tumor metastasis by adoptive transfer of IL-12-activated Valpha14 NKT cells. Int J Cancer (2001) 91:523–8. doi:10.1002/1097-0215(20010215)91:4<523::AID-IJC1087>3.0.CO;2-L
20. Smyth MJ, Crowe NY, Pellicci DG, Kyparissoudis K, Kelly JM, Takeda K, et al. Sequential production of interferon-gamma by NK1.1(+) T cells and natural killer cells is essential for the antimetastatic effect of alpha-galactosylceramide. Blood (2002) 99:1259–66. doi:10.1182/blood.V99.4.1259
21. Nowak M, Arredouani MS, Tun-Kyi A, Schmidt-Wolf I, Sanda MG, Balk SP, et al. Defective NKT cell activation by CD1d+ TRAMP prostate tumor cells is corrected by interleukin-12 with α-galactosylceramide. PLoS One (2010) 5:e11311. doi:10.1371/journal.pone.0011311
22. Giaccone G, Punt CJ, Ando Y, Ruijter R, Nishi N, Peters M, et al. A phase I study of the natural killer T-cell ligand alpha-galactosylceramide (KRN7000) in patients with solid tumors. Clin Cancer Res (2002) 8:3702–9. doi:10.1155/2007/89017
23. Schneiders FL, Scheper RJ, von Blomberg BME, Woltman AM, Janssen HLA, van den Eertwegh AJM, et al. Clinical experience with α-galactosylceramide (KRN7000) in patients with advanced cancer and chronic hepatitis B/C infection. Clin Immunol (2011) 140:130–41. doi:10.1016/j.clim.2010.11.010
24. Exley MA, Nakayama T. NKT-cell-based immunotherapies in clinical trials. Clin Immunol (2011) 140:117–8. doi:10.1016/j.clim.2011.04.015
25. Tahir SM, Cheng O, Shaulov A, Koezuka Y, Bubley GJ, Wilson SB, et al. Loss of IFN-gamma production by invariant NK T cells in advanced cancer. J Immunol (2001) 167:4046–50. doi:10.4049/jimmunol.167.7.4046
26. Yanagisawa K, Seino K, Ishikawa Y, Nozue M, Todoroki T, Fukao K. Impaired proliferative response of V alpha 24 NKT cells from cancer patients against alpha-galactosylceramide. J Immunol (2002) 168:6494–9. doi:10.4049/jimmunol.168.12.6494
27. Dhodapkar MV, Geller MD, Chang DH, Shimizu K, Fujii S-I, Dhodapkar KM, et al. A reversible defect in natural killer T cell function characterizes the progression of premalignant to malignant multiple myeloma. J Exp Med (2003) 197:1667–76. doi:10.1084/jem.20021650
28. Fujii S, Shimizu K, Klimek V, Geller MD, Nimer SD, Dhodapkar MV. Severe and selective deficiency of interferon-gamma-producing invariant natural killer T cells in patients with myelodysplastic syndromes. Br J Haematol (2003) 122:617–22. doi:10.1046/j.1365-2141.2003.04465.x
29. Molling JW, Kölgen W, van der Vliet HJJ, Boomsma MF, Kruizenga H, Smorenburg CH, et al. Peripheral blood IFN-gamma-secreting Valpha24+Vbeta11+ NKT cell numbers are decreased in cancer patients independent of tumor type or tumor load. Int J Cancer (2005) 116:87–93. doi:10.1002/ijc.20998
30. Molling JW, Langius JAE, Langendijk JA, Leemans CR, Bontkes HJ, van der Vliet HJJ, et al. Low levels of circulating invariant natural killer T cells predict poor clinical outcome in patients with head and neck squamous cell carcinoma. J Clin Oncol (2007) 25:862–8. doi:10.1200/JCO.2006.08.5787
31. Konishi J, Yamazaki K, Yokouchi H, Shinagawa N, Iwabuchi K, Nishimura M. The characteristics of human NKT cells in lung cancer – CD1d independent cytotoxicity against lung cancer cells by NKT cells and decreased human NKT cell response in lung cancer patients. Hum Immunol (2004) 65:1377–88. doi:10.1016/j.humimm.2004.09.003
32. Gorini F, Azzimonti L, Delfanti G, Scarfò L, Scielzo C, Bertilaccio MT, et al. Invariant NKT cells contribute to chronic lymphocytic leukemia surveillance and prognosis. Blood (2017) 129:3440–51. doi:10.1182/blood-2016-11-751065
33. Chang DH, Osman K, Connolly J, Kukreja A, Krasovsky J, Pack M, et al. Sustained expansion of NKT cells and antigen-specific T cells after injection of alpha-galactosyl-ceramide loaded mature dendritic cells in cancer patients. J Exp Med (2005) 201:1503–17. doi:10.1084/jem.20042592
34. Motohashi S, Ishikawa A, Ishikawa E, Otsuji M, Iizasa T, Hanaoka H, et al. A phase I study of in vitro expanded natural killer T cells in patients with advanced and recurrent non-small cell lung cancer. Clin Cancer Res (2006) 12:6079–86. doi:10.1158/1078-0432.CCR-06-0114
35. Kunii N, Horiguchi S, Motohashi S, Yamamoto H, Ueno N, Yamamoto S, et al. Combination therapy of in vitro-expanded natural killer T cells and alpha-galactosylceramide-pulsed antigen-presenting cells in patients with recurrent head and neck carcinoma. Cancer Sci (2009) 100:1092–8. doi:10.1111/j.1349-7006.2009.01135.x
36. Yamasaki K, Horiguchi S, Kurosaki M, Kunii N, Nagato K, Hanaoka H, et al. Induction of NKT cell-specific immune responses in cancer tissues after NKT cell-targeted adoptive immunotherapy. Clin Immunol (2011) 138:255–65. doi:10.1016/j.clim.2010.11.014
37. de Lalla C, Rinaldi A, Montagna D, Azzimonti L, Bernardo ME, Sangalli LM, et al. Invariant NKT cell reconstitution in pediatric leukemia patients given HLA-haploidentical stem cell transplantation defines distinct CD4+ and CD4- subset dynamics and correlates with remission state. J Immunol (2011) 186:4490–9. doi:10.4049/jimmunol.1003748
38. Casorati G, de Lalla C, Dellabona P. Invariant natural killer T cells reconstitution and the control of leukemia relapse in pediatric haploidentical hematopoietic stem cell transplantation. Oncoimmunology (2012) 1:355–7. doi:10.4161/onci.18399
39. Exley MA, Friedlander P, Alatrakchi N, Vriend L, Yue SC, Sasada T, et al. Adoptive transfer of invariant NKT cells as immunotherapy for advanced melanoma: a phase 1 clinical trial. Clin Cancer Res (2017) 25:3510–19. doi:10.1158/1078-0432.CCR-16-0600
40. Heczey A, Liu D, Tian G, Courtney AN, Wei J, Marinova E, et al. Invariant NKT cells with chimeric antigen receptor provide a novel platform for safe and effective cancer immunotherapy. Blood (2014) 124:2824–33. doi:10.1182/blood-2013-11-541235
41. Tian G, Courtney AN, Jena B, Heczey A, Liu D, Marinova E, et al. CD62L+ NKT cells have prolonged persistence and antitumor activity in vivo. J Clin Invest (2016) 126:2341–55. doi:10.1172/JCI83476
42. Kitayama S, Zhang R, Liu T-Y, Ueda N, Iriguchi S, Yasui Y, et al. Cellular adjuvant properties, direct cytotoxicity of re-differentiated Vα24 invariant NKT-like cells from human induced pluripotent stem cells. Stem Cell Reports (2016) 6:213–27. doi:10.1016/j.stemcr.2016.01.005
43. Yamada D, Iyoda T, Vizcardo R, Shimizu K, Sato Y, Endo TA, et al. Efficient regeneration of human Vα24(+) invariant natural killer T cells and their anti-tumor activity in vivo. Stem Cells (2016) 34:2852–60. doi:10.1002/stem.2465
44. Nishimura T, Kitamura H, Iwakabe K, Yahata T, Ohta A, Sato M, et al. The interface between innate and acquired immunity: glycolipid antigen presentation by CD1d-expressing dendritic cells to NKT cells induces the differentiation of antigen-specific cytotoxic T lymphocytes. Int Immunol (2000) 12:987–94. doi:10.1093/intimm/12.7.987
45. Nieda M, Okai M, Tazbirkova A, Lin H, Yamaura A, Ide K, et al. Therapeutic activation of Valpha24+Vbeta11+ NKT cells in human subjects results in highly coordinated secondary activation of acquired and innate immunity. Blood (2004) 103:383–9. doi:10.1182/blood-2003-04-1155
46. Ishikawa A, Motohashi S, Ishikawa E, Fuchida H, Higashino K, Otsuji M, et al. A phase I study of alpha-galactosylceramide (KRN7000)-pulsed dendritic cells in patients with advanced and recurrent non-small cell lung cancer. Clin Cancer Res (2005) 11:1910–7. doi:10.1158/1078-0432.CCR-04-1453
47. Motohashi S, Nagato K, Kunii N, Yamamoto H, Yamasaki K, Okita K, et al. A phase I-II study of alpha-galactosylceramide-pulsed IL-2/GM-CSF-cultured peripheral blood mononuclear cells in patients with advanced and recurrent non-small cell lung cancer. J Immunol (2009) 182:2492–501. doi:10.4049/jimmunol.0800126
48. Nicol AJ, Tazbirkova A, Nieda M. Comparison of clinical and immunological effects of intravenous and intradermal administration of α-galactosylceramide (KRN7000)-pulsed dendritic cells. Clin Cancer Res (2011) 17:5140–51. doi:10.1158/1078-0432.CCR-10-3105
49. Nagato K, Motohashi S, Ishibashi F, Okita K, Yamasaki K, Moriya Y, et al. Accumulation of activated invariant natural killer T cells in the tumor microenvironment after α-galactosylceramide-pulsed antigen presenting cells. J Clin Immunol (2012) 32:1071–81. doi:10.1007/s10875-012-9697-9
50. Dellabona P, Casorati G, de Lalla C, Montagna D, Locatelli F. On the use of donor-derived iNKT cells for adoptive immunotherapy to prevent leukemia recurrence in pediatric recipients of HLA haploidentical HSCT for hematological malignancies. Clin Immunol (2011) 140:152–9. doi:10.1016/j.clim.2010.11.015
51. Fujii S, Goto A, Shimizu K. Antigen mRNA-transfected, allogeneic fibroblasts loaded with NKT-cell ligand confer antitumor immunity. Blood (2009) 113:4262–72. doi:10.1182/blood-2008-08-176446
52. Shimizu K, Asakura M, Shinga J, Sato Y, Kitahara S, Hoshino K, et al. Invariant NKT cells induce plasmacytoid dendritic cell (DC) cross-talk with conventional DCs for efficient memory CD8+ T cell induction. J Immunol (2013) 190:5609–19. doi:10.4049/jimmunol.1300033
53. Fujii S-I, Shimizu K. Immunotherapy with artificial adjuvant vector cells: harnessing both arms of the immune response. Oncoimmunology (2013) 2:e23432. doi:10.4161/onci.23432
54. Shimizu K, Yamasaki S, Shinga J, Sato Y, Watanabe T, Ohara O, et al. Systemic DC activation modulates the tumor microenvironment and shapes the long-lived tumor-specific memory mediated by CD8+ T cells. Cancer Res (2016) 76:3756–66. doi:10.1158/0008-5472.CAN-15-3219
55. Shimizu K, Mizuno T, Shinga J, Asakura M, Kakimi K, Ishii Y, et al. Vaccination with antigen-transfected, NKT cell ligand-loaded, human cells elicits robust in situ immune responses by dendritic cells. Cancer Res (2013) 73:62–73. doi:10.1158/0008-5472.CAN-12-0759
56. Shimizu K, Kurosawa Y, Taniguchi M, Steinman RM, Fujii S-I. Cross-presentation of glycolipid from tumor cells loaded with alpha-galactosylceramide leads to potent and long-lived T cell mediated immunity via dendritic cells. J Exp Med (2007) 204:2641–53. doi:10.1084/jem.20070458
57. Shimizu K, Goto A, Fukui M, Taniguchi M, Fujii S. Tumor cells loaded with alpha-galactosylceramide induce innate NKT and NK cell-dependent resistance to tumor implantation in mice. J Immunol (2007) 178:2853–61. doi:10.4049/jimmunol.178.5.2853
58. Chung Y, Qin H, Kang C-Y, Kim S, Kwak LW, Dong C. An NKT-mediated autologous vaccine generates CD4 T-cell dependent potent antilymphoma immunity. Blood (2007) 110:2013–9. doi:10.1182/blood-2006-12-061309
59. Choi YS, Hoory T, Monie A, Wu A, Connolly D, Hung C-F. alpha-Galactosylceramide enhances the protective and therapeutic effects of tumor cell based vaccines for ovarian tumors. Vaccine (2008) 26:5855–63. doi:10.1016/j.vaccine.2008.08.027
60. Mattarollo SR, West AC, Steegh K, Duret H, Paget C, Martin B, et al. NKT cell adjuvant-based tumor vaccine for treatment of myc oncogene-driven mouse B-cell lymphoma. Blood (2012) 120:3019–29. doi:10.1182/blood-2012-04-426643
61. Hunn MK, Farrand KJ, Broadley KWR, Weinkove R, Ferguson P, Miller RJ, et al. Vaccination with irradiated tumor cells pulsed with an adjuvant that stimulates NKT cells is an effective treatment for glioma. Clin Cancer Res (2012) 18:6446–59. doi:10.1158/1078-0432.CCR-12-0704
62. Mattarollo SR, Steegh K, Li M, Duret H, Foong Ngiow S, Smyth MJ. Transient Foxp3(+) regulatory T-cell depletion enhances therapeutic anticancer vaccination targeting the immune-stimulatory properties of NKT cells. Immunol Cell Biol (2013) 91:105–14. doi:10.1038/icb.2012.58
63. Hong S, Lee H, Jung K, Lee SM, Lee S-J, Jun HJ, et al. Tumor cells loaded with α-galactosylceramide promote therapeutic NKT-dependent anti-tumor immunity in multiple myeloma. Immunol Lett (2013) 156:132–9. doi:10.1016/j.imlet.2013.10.002
64. Hunn MK, Hermans IF. Exploiting invariant NKT cells to promote T-cell responses to cancer vaccines. Oncoimmunology (2013) 2:e23789. doi:10.4161/onci.23789
65. Gibbins JD, Ancelet LR, Weinkove R, Compton BJ, Painter GF, Petersen TR, et al. An autologous leukemia cell vaccine prevents murine acute leukemia relapse after cytarabine treatment. Blood (2014) 124:2953–63. doi:10.1182/blood-2014-04-568956
66. Dong T, Yi T, Yang M, Lin S, Li W, Xu X, et al. Co-operation of α-galactosylceramide-loaded tumour cells and TLR9 agonists induce potent anti-tumour responses in a murine colon cancer model. Biochem J (2016) 473:7–19. doi:10.1042/BJ20150129
67. Hermans IF, Silk JD, Gileadi U, Masri SH, Shepherd D, Farrand KJ, et al. Dendritic cell function can be modulated through cooperative actions of TLR ligands and invariant NKT cells. J Immunol (2007) 178:2721–9. doi:10.4049/jimmunol.178.5.2721
68. Ko H-J, Kim Y-J, Kim Y-S, Chang W-S, Ko S-Y, Chang S-Y, et al. A combination of chemoimmunotherapies can efficiently break self-tolerance and induce antitumor immunity in a tolerogenic murine tumor model. Cancer Res (2007) 67:7477–86. doi:10.1158/0008-5472.CAN-06-4639
69. Fukushima S, Hirata S, Motomura Y, Fukuma D, Matsunaga Y, Ikuta Y, et al. Multiple antigen-targeted immunotherapy with alpha-galactosylceramide-loaded and genetically engineered dendritic cells derived from embryonic stem cells. J Immunother (2009) 32:219–31. doi:10.1097/CJI.0b013e318194b63b
70. Matsuyoshi H, Hirata S, Yoshitake Y, Motomura Y, Fukuma D, Kurisaki A, et al. Therapeutic effect of alpha-galactosylceramide-loaded dendritic cells genetically engineered to express SLC/CCL21 along with tumor antigen against peritoneally disseminated tumor cells. Cancer Sci (2005) 96:889–96. doi:10.1111/j.1349-7006.2005.00123.x
71. Zeng J, Shahbazi M, Wu C, Toh HC, Wang S. Enhancing immunostimulatory function of human embryonic stem cell-derived dendritic cells by CD1d overexpression. J Immunol (2012) 188:4297–304. doi:10.4049/jimmunol.1102343
72. Craparo EF, Bondì ML. Application of polymeric nanoparticles in immunotherapy. Curr Opin Allergy Clin Immunol (2012) 12:658–64. doi:10.1097/ACI.0b013e3283588c57
73. Smith DM, Simon JK, Baker JR. Applications of nanotechnology for immunology. Nat Rev Immunol (2013) 13:592–605. doi:10.1038/nri3488
74. Serda RE. Particle platforms for cancer immunotherapy. Int J Nanomedicine (2013) 8:1683–96. doi:10.2147/IJN.S31756
75. Cruz LJ, Tacken PJ, Fokkink R, Joosten B, Stuart MC, Albericio F, et al. Targeted PLGA nano- but not microparticles specifically deliver antigen to human dendritic cells via DC-SIGN in vitro. J Control Release (2010) 144:118–26. doi:10.1016/j.jconrel.2010.02.013
76. Ghotbi Z, Haddadi A, Hamdy S, Hung RW, Samuel J, Lavasanifar A. Active targeting of dendritic cells with mannan-decorated PLGA nanoparticles. J Drug Target (2011) 19:281–92. doi:10.3109/1061186X.2010.499463
77. Waeckerle-Men Y, Groettrup M. PLGA microspheres for improved antigen delivery to dendritic cells as cellular vaccines. Adv Drug Deliv Rev (2005) 57:475–82. doi:10.1016/j.addr.2004.09.007
78. Bachmann MF, Jennings GT. Vaccine delivery: a matter of size, geometry, kinetics and molecular patterns. Nat Rev Immunol (2010) 10:787–96. doi:10.1038/nri2868
79. Park Y-M, Lee SJ, Kim YS, Lee MH, Cha GS, Jung ID, et al. Nanoparticle-based vaccine delivery for cancer immunotherapy. Immune Netw (2013) 13:177. doi:10.4110/in.2013.13.5.177
80. Alyautdin R, Khalin I, Nafeeza MI, Haron MH, Kuznetsov D. Nanoscale drug delivery systems and the blood-brain barrier. Int J Nanomedicine (2014) 9:795–811. doi:10.2147/IJN.S52236
81. Fahmy TM, Demento SL, Caplan MJ, Mellman I, Saltzman WM. Design opportunities for actively targeted nanoparticle vaccines. Nanomedicine (Lond) (2008) 3:343–55. doi:10.2217/17435889.3.3.343
82. Mundargi RC, Babu VR, Rangaswamy V, Patel P, Aminabhavi TM. Nano/micro technologies for delivering macromolecular therapeutics using poly(D,L-lactide-co-glycolide) and its derivatives. J Control Release (2008) 125:193–209. doi:10.1016/j.jconrel.2007.09.013
83. Lü J-M, Wang X, Marin-Muller C, Wang H, Lin PH, Yao Q, et al. Current advances in research and clinical applications of PLGA-based nanotechnology. Expert Rev Mol Diagn (2009) 9:325–41. doi:10.1586/erm.09.15
84. Lee Y-R, Lee Y-H, Im S-A, Kim K, Lee C-K. Formulation and characterization of antigen-loaded PLGA nanoparticles for efficient cross-priming of the antigen. Immune Netw (2011) 11:163–8. doi:10.4110/in.2011.11.3.163
85. Silva AL, Soema PC, Slütter B, Ossendorp F, Jiskoot W. PLGA particulate delivery systems for subunit vaccines: linking particle properties to immunogenicity. Hum Vaccin Immunother (2016) 12:1056–69. doi:10.1080/21645515.2015.1117714
86. Barral P, Polzella P, Bruckbauer A, van Rooijen N, Besra GS, Cerundolo V, et al. CD169(+) macrophages present lipid antigens to mediate early activation of iNKT cells in lymph nodes. Nat Immunol (2010) 11:303–12. doi:10.1038/ni.1853
87. Barral P, Sánchez-Niño MD, van Rooijen N, Cerundolo V, Batista FD. The location of splenic NKT cells favours their rapid activation by blood-borne antigen. EMBO J (2012) 31:2378–90. doi:10.1038/emboj.2012.87
88. Thapa P, Zhang G, Xia C, Gelbard A, Overwijk WW, Liu C, et al. Nanoparticle formulated alpha-galactosylceramide activates NKT cells without inducing anergy. Vaccine (2009) 27:3484–8. doi:10.1016/j.vaccine.2009.01.047
89. Macho Fernandez E, Chang J, Fontaine J, Bialecki E, Rodriguez F, Werkmeister E, et al. Activation of invariant Natural Killer T lymphocytes in response to the α-galactosylceramide analogue KRN7000 encapsulated in PLGA-based nanoparticles and microparticles. Int J Pharm (2012) 423:45–54. doi:10.1016/j.ijpharm.2011.04.068
90. Nakamura T, Yamazaki D, Yamauchi J, Harashima H. The nanoparticulation by octaarginine-modified liposome improves α-galactosylceramide-mediated antitumor therapy via systemic administration. J Control Release (2013) 171:216–24. doi:10.1016/j.jconrel.2013.07.004
91. Ishii M, Kojima N. Effective stimulation of invariant natural killer T cells by oligomannose-coated liposomes. Int Immunopharmacol (2013) 15:685–92. doi:10.1016/j.intimp.2013.03.009
92. Kawasaki N, Vela JL, Nycholat CM, Rademacher C, Khurana A, van Rooijen N, et al. Targeted delivery of lipid antigen to macrophages via the CD169/sialoadhesin endocytic pathway induces robust invariant natural killer T cell activation. Proc Natl Acad Sci U S A (2013) 110:7826–31. doi:10.1073/pnas.1219888110
93. Macho-Fernandez E, Cruz LJ, Ghinnagow R, Fontaine J, Bialecki E, Frisch B, et al. Targeted delivery of α-galactosylceramide to CD8α+ dendritic cells optimizes type I NKT cell-based antitumor responses. J Immunol (2014) 193:961–9. doi:10.4049/jimmunol.1303029
94. McKee SJ, Young VL, Clow F, Hayman CM, Baird MA, Hermans IF, et al. Virus-like particles and α-galactosylceramide form a self-adjuvanting composite particle that elicits anti-tumor responses. J Control Release (2012) 159:338–45. doi:10.1016/j.jconrel.2012.02.015
95. Dölen Y, Kreutz M, Gileadi U, Tel J, Vasaturo A, van Dinther EAW, et al. Co-delivery of PLGA encapsulated invariant NKT cell agonist with antigenic protein induce strong T cell-mediated antitumor immune responses. Oncoimmunology (2015) 5:e1068493. doi:10.1080/2162402X.2015.1068493
96. Li B, Siuta M, Bright V, Koktysh D, Matlock BK, Dumas ME, et al. Improved proliferation of antigen-specific cytolytic T lymphocytes using a multimodal nanovaccine. Int J Nanomedicine (2016) 11:6103–21. doi:10.2147/IJN.S112432
97. Neumann S, Young K, Compton B, Anderson R, Painter G, Hook S. Synthetic TRP2 long-peptide and α-galactosylceramide formulated into cationic liposomes elicit CD8+ T-cell responses and prevent tumour progression. Vaccine (2015) 33:5838–44. doi:10.1016/j.vaccine.2015.08.083
98. Crozat K, Guiton R, Contreras V, Feuillet V, Dutertre C-A, Ventre E, et al. The XC chemokine receptor 1 is a conserved selective marker of mammalian cells homologous to mouse CD8alpha+ dendritic cells. J Exp Med (2010) 207:1283–92. doi:10.1084/jem.20100223
99. Bachem A, Güttler S, Hartung E, Ebstein F, Schaefer M, Tannert A, et al. Superior antigen cross-presentation and XCR1 expression define human CD11c+CD141+ cells as homologues of mouse CD8+ dendritic cells. J Exp Med (2010) 207:1273–81. doi:10.1084/jem.20100348
100. Poulin LF, Salio M, Griessinger E, Anjos-Afonso F, Craciun L, Chen J-L, et al. Characterization of human DNGR-1+ BDCA3+ leukocytes as putative equivalents of mouse CD8alpha+ dendritic cells. J Exp Med (2010) 207:1261–71. doi:10.1084/jem.20092618
101. Jongbloed SL, Kassianos AJ, McDonald KJ, Clark GJ, Ju X, Angel CE, et al. Human CD141+ (BDCA-3)+ dendritic cells (DCs) represent a unique myeloid DC subset that cross-presents necrotic cell antigens. J Exp Med (2010) 207:1247–60. doi:10.1084/jem.20092140
102. Arora P, Baena A, Yu KOA, Saini NK, Kharkwal SS, Goldberg MF, et al. A single subset of dendritic cells controls the cytokine bias of natural killer T cell responses to diverse glycolipid antigens. Immunity (2014) 40:105–16. doi:10.1016/j.immuni.2013.12.004
103. Semmling V, Lukacs-Kornek V, Thaiss CA, Quast T, Hochheiser K, Panzer U, et al. Alternative cross-priming through CCL17-CCR4-mediated attraction of CTLs toward NKT cell-licensed DCs. Nat Immunol (2010) 11:313–20. doi:10.1038/ni.1848
104. Sancho D, Mourão-Sá D, Joffre OP, Schulz O, Rogers NC, Pennington DJ, et al. Tumor therapy in mice via antigen targeting to a novel, DC-restricted C-type lectin. J Clin Invest (2008) 118:2098–110. doi:10.1172/JCI34584
105. Picco G, Beatson R, Taylor-Papadimitriou J, Burchell JM. Targeting DNGR-1 (CLEC9A) with antibody/MUC1 peptide conjugates as a vaccine for carcinomas. Eur J Immunol (2014) 44:1947–55. doi:10.1002/eji.201344076
106. Tullett KM, Lahoud MH, Radford KJ. Harnessing human cross-presenting CLEC9A(+)XCR1(+) dendritic cells for immunotherapy. Front Immunol (2014) 5:239. doi:10.3389/fimmu.2014.00239
107. Ghinnagow R, De Meester J, Cruz LJ, Aspord C, Corgnac S, Macho-Fernandez E, et al. Co-delivery of the NKT agonist α-galactosylceramide and tumor antigens to cross-priming dendritic cells breaks tolerance to self-antigens and promotes antitumor responses. Oncoimmunology (in press).
108. Klebanoff CA, Acquavella N, Yu Z, Restifo NP. Therapeutic cancer vaccines: are we there yet? Immunol Rev (2011) 239:27–44. doi:10.1111/j.1600-065X.2010.00979.x
109. Andersen BM, Ohlfest JR. Increasing the efficacy of tumor cell vaccines by enhancing cross priming. Cancer Lett (2012) 325:155–64. doi:10.1016/j.canlet.2012.07.012
110. Palucka K, Banchereau J. Dendritic-cell-based therapeutic cancer vaccines. Immunity (2013) 39:38–48. doi:10.1016/j.immuni.2013.07.004
111. Chen DS, Mellman I. Oncology meets immunology: the cancer-immunity cycle. Immunity (2013) 39:1–10. doi:10.1016/j.immuni.2013.07.012
112. Pol J, Bloy N, Buqué A, Eggermont A, Cremer I, Sautès-Fridman C, et al. Trial watch: peptide-based anticancer vaccines. Oncoimmunology (2015) 4:e974411. doi:10.4161/2162402X.2014.974411
113. Coelho-Dos-Reis JG, Huang J, Tsao T, Pereira FV, Funakoshi R, Nakajima H, et al. Co-administration of α-GalCer analog and TLR4 agonist induces robust CD8(+) T-cell responses to PyCS protein and WT-1 antigen and activates memory-like effector NKT cells. Clin Immunol (2016) 168:6–15. doi:10.1016/j.clim.2016.04.014
Keywords: natural killer T cells, adjuvant, α-galactosylceramide, CTL response, nanovaccines, dendritic cells, cancer
Citation: Ghinnagow R, Cruz LJ, Macho-Fernandez E, Faveeuw C and Trottein F (2017) Enhancement of Adjuvant Functions of Natural Killer T Cells Using Nanovector Delivery Systems: Application in Anticancer Immune Therapy. Front. Immunol. 8:879. doi: 10.3389/fimmu.2017.00879
Received: 01 June 2017; Accepted: 10 July 2017;
Published: 27 July 2017
Edited by:
Paolo Dellabona, San Raffaele Hospital (IRCCS), ItalyReviewed by:
Tonya J. Webb, University of Maryland, Baltimore, United StatesAlessandro Poggi, IRCCS AOU San Martino IST, Italy
Moriya Tsuji, Aaron Diamond AIDS Research Center, United States
Copyright: © 2017 Ghinnagow, Cruz, Macho-Fernandez, Faveeuw and Trottein. This is an open-access article distributed under the terms of the Creative Commons Attribution License (CC BY). The use, distribution or reproduction in other forums is permitted, provided the original author(s) or licensor are credited and that the original publication in this journal is cited, in accordance with accepted academic practice. No use, distribution or reproduction is permitted which does not comply with these terms.
*Correspondence: François Trottein, ZnJhbmNvaXMudHJvdHRlaW5AcGFzdGV1ci1saWxsZS5mcg==