- Universitätsklinikum Erlangen, Erlangen, Germany
An essential role of the intestine is to build and maintain a barrier preventing the luminal gut microbiota from invading the host. This involves two coordinated physical and immunological barriers formed by single layers of intestinal epithelial and endothelial cells, which avoid the activation of local immune responses or the systemic dissemination of microbial agents, and preserve tissue homeostasis. Accordingly, alterations of epithelial and endothelial barrier functions have been associated with gut inflammation, for example during inflammatory bowel disease (IBD). The discriminative control of nutriment uptake and sealing toward potentially pathological microorganisms requires a profound regulation of para- and transcellular permeability. On the subcellular level, the cytoskeleton exerts key regulatory functions in the maintenance of cellular barriers. Increased epithelial/endothelial permeability occurs primarily as a result of a reorganization of cytoskeletal–junctional complexes. Pro-inflammatory mediators such as cytokines can induce cytoskeletal rearrangements, causing inflammation-dependent defects in gut barrier function. In this context, small GTPases of the Rho family and large GTPases from the Dynamin superfamily appear as major cellular switches regulating the interaction between intercellular junctions and actomyosin complexes, and in turn cytoskeleton plasticity. Strikingly, some of these proteins, such as RhoA or guanylate-binding protein-1 (GBP-1) have been associated with gut inflammation and IBD. In this review, we will summarize the role of small and large GTPases for cytoskeleton plasticity and epithelial/endothelial barrier in the context of gut inflammation.
Introduction
Epithelia at mucosal surfaces represent the first barrier preventing potentially harmful environmental factors to invade the host. In the intestine, the epithelium does not only represent a simple physical obstacle against pathogen invasion but it also regulates nutrient uptake and innate immune function by avoiding the activation of mucosal immune responses (1). Thereby, maintenance of epithelial integrity is a key aspect in order to preserve homeostasis and to impair the development of inflammation in mucosal tissues (2). In addition to the epithelium, the gut–vascular barrier (GVB) has been recently described as a new anatomical structure which builds a second protective barrier preventing the microbiota to enter the bloodstream while allowing the translocation of immune cells and antigens (3). Barrier function of the epithelium as well as of the endothelium is dependent on a complex cytoskeletal organization and, in particular, on the formation of stable cell–cell junctions (4–6). These structures undergo profound changes during inflammation (7). Accordingly, increased paracellular permeability and epithelial/endothelial barrier dysfunction have been linked to the pathogenesis of chronic inflammatory disorders, such as inflammatory bowel diseases (IBDs) (2, 8, 9). IBD is defined as an idiopathic, chronic, and relapsing inflammation of the gastrointestinal tract. Two main clinical manifestations, Crohn’s disease (CD) and ulcerative colitis (UC), affect a rather young population whose quality of life is significantly reduced. Despite intensive research, the pathogenesis of IBD is not completely understood. Here, we discuss the role of small and large GTPases in the cytoskeletal rearrangements induced in intestinal epithelial and endothelial barriers during inflammation (Figure 1).
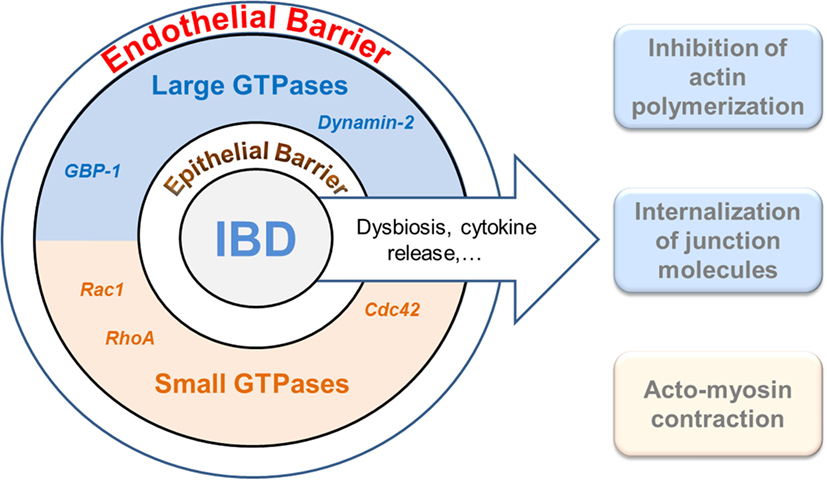
Figure 1. Interplay of GTPases and the cytoskeleton in cellular barrier defects during gut inflammation. The intestinal epithelium and the endothelium establish two coordinated physical and immunological barriers. Increased barrier permeability is pathogenetically associated with inflammatory bowel diseases (IBDs). Different members of the families of small (lower brown) and large (upper blue) GTPases have recently been shown to regulate junctional and cytoskeletal dysfunctions both in epithelial and endothelial cells and, accordingly, may play an important role in IBD. It warrants further studies to determine whether cooperative, antagonistic, or redundant functions are exerted by the different GTPases.
Intercellular Junctions in Epithelium and Endothelium
Apical junction complexes (AJC) built by tight junctions (TJs) and adherens junctions (AJs) enable the connection between adjacent cells, both in intestinal epithelium and endothelium. The AJC contribute to barrier function by controlling selective diffusion of molecules or cells, maintaining cell polarity and allowing intercellular communication (10). TJs consist of occludins, claudins, and junctional adhesion molecules (JAMs) (6, 11). AJs are composed of cadherins and nectins (12, 13). Both represent specialized zipper-like structures which enable the sealing of the paracellular space within the epithelial or endothelial layer (14). These intercellular junctions are connected to the actomyosin cytoskeleton via cytoplasmatic adaptors, such as zonula occludens (ZO) proteins, and catenins (6, 15, 16), which supports the mechanical strength of the junctions. For instance, in the resting endothelium, the cortical actin network ensures the necessary tension for the formation of stable interactions at AJs (17). AJs and TJs have been shown to influence each other’s assembly and maintenance in a reciprocal manner (18, 19). In the presence of permeability-inducing molecules, actin reorganizes into stress fibers, which increases traction forces and leads to the uncoupling of AJC from the actin cytoskeleton resulting in the formation of gaps between adjacent cells (20, 21). Contraction of a perijunctional actomyosin ring further regulates permeability in a myosin light-chain kinase- dependent manner (22). In addition, TJ and AJ molecules can be removed from the cell surface by internalization and/or by proteolytic cleavage resulting in extracellular domain shedding (18). Thus, the interaction between cytoskeleton and intercellular junctions is crucial for maintenance of epithelial/endothelial barrier function (23).
Intercellular junction composition and abundancy are tissue-dependent. Within the intestinal epithelium, TJs proteins can be categorized in three families: claudins (claudin-1, 2, 3, 4, 5, 7, and 15) (24), tight junction-associated Marvel proteins (Occludin, Marvel D3, and tricellulin) (25), and cortical thymocyte marker of the Xenopus (CTX) (JAM-A, CAR, and CLMP) (26). The composition and structure of endothelial TJs can vary according to the type of vessel or organ (27). In intestinal endothelial cells (EndoCs), TJs are composed of occludin, JAM-A, ZO-1, and cingulin, while claudin-5 was mostly associated with gut lymphatic EndoCs (3). Epithelial AJs are composed of α- and β-catenin and E-cadherin, while AJs within EndoCs are formed by VE-cadherin and β-catenin (3). The formation of VE-cadherin adhesions at AJs is the primary event regulating EndoC-cell interactions during vasculogenesis, and this depends on intracellular tension generated by the actin cytoskeleton (18).
Epithelial Barrier Regulation during Intestinal Inflammation
Epithelial integrity in the gut has to be tightly regulated. In order to build up a protective barrier against luminal content, a precise and complex cell turnover warranties the renewal of the epithelium without compromising its tightness. Stem cells at the crypt bottom proliferate and differentiate into several IECs subtypes with specialized biological functions (28). Then, most of the differentiated IECs migrate upwards to the villus tip, where aged cells die and are shed into the lumen (29, 30). During this sophisticated process, the tightness of the epithelial layer is achieved by the intimate connection between epithelial cells, which is primarily mediated by intercellular junctions connected to the actin cytoskeleton (6). Focusing on cell shedding, the maintenance of epithelial integrity is warranted by the redistribution of junctional proteins along lateral membranes in a cytoskeleton and membrane trafficking-dependent molecular mechanism (31, 32).
The complex cytoskeleton network in IECs (4, 23, 31) orchestrates key cellular and molecular events during epithelial morphogenesis and renewal (12, 33). On a cellular level, the cytoskeleton defines cell shape and polarity which are important for nutrient uptake, anchoring of IECs to the basal membrane and communication with the sub-epithelial compartment (34, 35). Cytoskeletal plasticity within IECs is relevant to maintain barrier integrity and tissue homeostasis. Accordingly, breakdown of epithelial integrity has been observed after disruption of intercellular junctions and cytoskeleton rearrangement, e.g., in the context of infection or inflammation (36–38).
Increased epithelial TJ permeability is a hallmark of tissue alterations observed in the gut of IBD patients (39–43). Although a correlation between permeability and disease activity could be shown in CD patients, for instance (44, 45), the triggering event involved in the breakdown of gut homeostasis is still a matter of controversy. Mouse studies demonstrated that deficiency of single TJ proteins is not associated with pathology due to compensatory mechanisms (46, 47), except for claudin-15 (48). By contrast, it is well accepted that inflammation-derived mediators mediate TJ dysfunction and thereby contribute to the breakdown of epithelial integrity in experimental colitis and IBD. These mediators include cytokines, such as IL-6 (49), IL-13 (50, 51), TNF (52), and type II Interferon (IFN-γ) (53–55). Then, increased intestinal permeability in IBD patients might be secondary to the release of cytokines within the gut mucosa (56, 57). These cytokines then affect paracellular permeability via myosin light-chain II-mediated contraction of the prejunctional actin ring, as shown for TNF in IBD patients (23). These observations support the assumption that epithelial integrity breakdown is indeed a consequence of inflammation.
However, recent studies in IBD patients demonstrated that flares of the disease are preceded by increased permeability, which argues for a causative role of the epithelium in the development of intestinal inflammation (41, 58–60). Interestingly, even healthy relatives (61–63) and non-inflamed gut areas in CD patients (64) showed an elevated intestinal permeability. Accordingly, new therapy strategies based on epithelial restoration led to promising results in IBD patients. For instance, therapeutically induced decrease of epithelial permeability by vitamin D (65, 66) or probiotics (67–69), IL-22-triggered mucus production (70) or maintenance of epithelial cell integrity by butyrate (71, 72), or anti-TNF antibody treatment resulted in a clinical amelioration of chronic colitis (73, 74). The remaining open question is which mechanism might regulate cytoskeleton remodeling and epithelial permeability.
Vascular Barrier Regulation during Intestinal Inflammation
The endothelium consists of a continuous monolayer of EndoCs lining the wall of blood and lymphatic vessels (75). It represents a semipermeable barrier between the bloodstream and the interstitium which regulates nutrient transport, tissue fluid homeostasis, immune cell transmigration (75), and restricts the transport of proteins in an organ-dependent manner (18). Similar to the epithelium, cell–cell junctions are crucial for the barrier role of the endothelium. The loss of EndoC-cell junctions causes a flux of proteinaceous fluid from the bloodstream into tissues, resulting in the development of edema. In addition to cell–cell junctions, coverage of the EndoC layer by pericytes is involved in the endothelial barrier function and was found to regulate permeability of the blood–brain barrier (76, 77).
The intestinal vascular endothelium represents a specialized vascular bed (3, 78). In the intestine, the capillaries are located directly underneath the epithelial layer and organized in gut–vascular units composed of EndoCs, pericytes, and enteric glial cells (3). Interestingly, the resting gut blood endothelium displays different levels of permeability depending on its localization. In the lamina propria, the endothelial permeability is increased compared to the submucosa, allowing the translocation of nutrients and antigens into the bloodstream while limiting enteric bacteria penetration (3).
During IBD, the intestine undergoes profound histological changes, including massive leukocyte infiltration, increased blood vessel density, and edema, which are all linked to vascular function (79–81). During inflammation, the vasculature is activated by inflammatory cytokines (ICs), such as TNF, interleukin-1 β (IL-1β), or IFN-γ, which leads to the expression of leukocytes adhesion molecules and fosters immune cell transmigration. In addition, neo-angiogenesis is induced and correlates with disease severity. More precisely, elevated levels of vascular endothelial growth factor (VEGF) can be found in the inflamed mucosa and in the blood during active IBD (80, 82–84) and vessel density is increased in the intestinal mucosa during IBD and in mouse model of colitis (9). However, inflammatory mediators such as ICs exhibit antiangiogenic activity and the concomitant presence of angiogenic and angiostatic molecules may disturb the physiologic regulation of angiogenesis (85–87). This might explain the disorganized intestinal vasculature observed in IBD, which is characterized by reduced vessel coverage, increased vessel leakiness, edema, and stenosis (81). Furthermore, vessel permeability strongly increases in both acute and chronic DSS-colitis mouse models compared to healthy animals (9). Interestingly, both ICs and VEGF have been shown to increase paracellular permeability of EndoC monolayers in culture (53, 88–90). In particular, high levels of IFN-γ and markers of IFN-γ-activated endothelium, such as ICAM1, VCAM1, MAdCAM, CXCL10, or guanylate-binding protein-1 (GBP-1), can be detected in the gut mucosa of mice during DSS-induced intestinal inflammation (9). In this model, neutralization of IFN-γ resulted in an increased vessel density while vessel permeability decreased (9). Hence, the vascular effects of IFN-γ during IBD might contribute to disease severity by limiting angiogenesis and increasing vessel permeability, ultimately leading to the loss of GVB function. At the molecular level, endothelial (and epithelial) cells treated with IFN-γ undergo remodeling of the actin cytoskeleton and cell–cell junctions, the latter associated with a decrease of ZO-1 expression and internalization of TJ and AJ proteins (55). Further studies are necessary to understand the exact mechanisms of barrier function regulation by IFN-γ.
Role of Large and Small GTPases in the Regulation of Cytoskeleton Remodeling during Intestinal Inflammation
Large and small GTPases are molecular switches transducing signals from the extracellular compartment to the intracellular machinery. By means of a GTP–GDP-mediated activation cycle (91), these proteins are involved in numerous biological processes, with dramatic impact on cell biology. Most functions of GTPases depend on their association with cellular membranes. The localization of the protein in close proximity to cellular membranes requires a specific posttranslational modification named prenylation. Prenylation consists of the binding of an isoprenoid at the C-terminal end of the target protein and impacts on protein physicochemical properties, subcellular localization, and function (92, 93). New findings demonstrated the important role of large and small GTPases as major cytoskeleton interacting partners and in the regulation of actomyosin dynamics and intercellular junctions (94). Changes in the GTPase activity promote actomyosin dysregulation associated with pathological conditions in several organs (95–97).
Proteins belonging to the Ras superfamily are defined as small GTPases because of their low molecular weight. The Ras superfamily of proteins consists of five families (Ras, Rho, Ran, Rab, and Arf) and more than 160 different members (98). They participate in the regulation of cell proliferation, cytoskeletal dynamics/morphology, membrane trafficking, cellular adhesion, vesicular, and nuclear transport (99–101). Besides the well-described superfamily of small GTPases, the dynamin superfamily of large GTPases represents a group of enzymes involved in pathogen resistance, budding of transport vesicles, division of organelles, cytokinesis, and cytoskeletal rearrangements (102). It comprises dynamins, Mx proteins, OPA, mitofusins, atlastins, and guanylate-binding proteins (GBPs). Large GTPases are characterized by the ability to oligomerize and harbor an oligomerization-dependent GTPase activity (102).
In the following, we will summarize the role of small and large GTPases in cytoskeleton remodeling, epithelial and endothelial integrity, and their relevance in maintenance of barrier functions in the gut.
Small GTPases
Impaired small GTPase function in the intestinal epithelium is associated with junctional and cytoskeletal dysfunctions (103–105). Numerous in vitro studies demonstrated Rho-mediated regulation of the cytoskeleton within epithelial cells (106–111); both up- and downregulation of Rho protein function can alter actomyosin contractility and in turn impair barrier function (112, 113). Actomyosin contraction due to phosphorylation of MLC2 by ROCK is involved in epithelial RhoA signaling, which is required for pathological as well as physiological epithelial cell extrusion (32, 114). The link between RhoA and intestinal inflammation was first shown in 2003, when increased RhoA activation in experimental colitis and patients suffering from IBD was identified (115). In a subsequent study, it was found that Rho-GDP dissociation inhibitor alpha expression was upregulated in CD and UC patients (116). We recently showed that IBD seems to be associated with impaired RhoA function (117). Inflamed areas in the gut of IBD patients depicted an accumulation of RhoA in the cytosol of IECs. This altered subcellular localization could presumably be a sign of RhoA dysfunction, since association to the plasma membrane is required for GTPase activation (118, 119). Furthermore, IEC-restricted lack of RhoA in mice resulted in the development of spontaneous inflammation (117). Interestingly, another recent study demonstrates that lack of Arhgap17, a RhoGTPase activating protein, causes increased epithelial permeability, not leading to spontaneous colitis but increasing the severity of DSS-induced colitis in mice (120). Taking together, RhoA can be considered as an important regulator of epithelial cytoskeleton and homeostasis in the gut. However, the mechanism and regulation of this process is still controversial. Actomyosin contraction due to phosphorylation of MLC2 by ROCK is involved in epithelial RhoA function, but whether RhoA inhibition, activation or both would modify epithelial integrity and permeability is still unclear.
Rac1 and Cdc42 also appear as attractive targets for the regulation of epithelial barrier function. In vivo genetic deletions of Cdc42 or Rac1 within IECs are associated with defects on epithelial cell proliferation and/or differentiation (121–124). Interestingly, genetic deletion of Cdc42 in mice resulted in an intestinal phenotype which resembled human microvillus inclusion disease. In the latter, cytoskeleton remodeling appears as a complementary mechanism to Paneth cell differentiation defects, leading to apical junction disorientation and increased intestinal paracellular permeability (123, 124).
Considering the relevance of regulated small GTPase function for cytoskeleton remodeling within IECs, prenylation has emerged as an attractive candidate target in epithelial restoration. Interestingly, IECs from IBD patients show decreased expression of the prenylation-catalyzing enzyme GGTase-Iβ (117). The link between GGTase-I-mediated prenylation and inflammation was confirmed by the dramatic intestinal distortion observed in mice with GGTase-Iβ-deficient IECs, which was ameliorated upon local induction of Rho activation (117). The destruction of intestinal architecture upon epithelial Pggt1b, the gene encoding for GGTase-Iβ (geranylgeranyltransferase1 beta subunit) deletion goes along with cytoskeleton remodeling, cell shedding alterations, and increased intestinal permeability. In conclusion, prenylation may represent a novel relevant pathway for maintenance of gut homeostasis and epithelial integrity. Future studies are needed in order to further elucidate the molecular mechanisms related to Rho GTPases and other targets of prenylation within the intestinal epithelium. In this context, a recent study showed that the commensal microbiota can increase intestinal epithelial permeability through the small GTPase ARF4 (125). The expression of ARF4 led to a decrease in the expression of TJ proteins by a mechanism which still has to be determined (125). These results open new perspectives for the understanding of the role of the microbiome in the regulation of intestinal barrier function and in the onset of colitis.
Similar to their function in the epithelium, small GTPases play an essential role in the regulation of the endothelial barrier function through their impact on actin dynamics (126). RhoA activation and subsequent Rock-mediated actomyosin contractility decreases endothelial barrier function upon permeability-inducing compounds, such as thrombin (127). On the other hand, Rac1 and Cdc42 signals are able to counterbalance an increase of endothelial permeability by stabilizing intercellular junctions, decreasing actin contractility, and in turn facilitating the contact between adjacent EndoCs (128, 129). A complex interplay between opposite effects from RhoA and Cdc42/Rac1 and their functional cooperation defines Rho-mediated regulation of endothelial integrity. This crosstalk between RhoA and Rac1 is of particular importance in the context of chronic inflammation. TNF is well known to induce endothelial actin cytoskeleton reorganization and intercellular gaps through a sequential activation of Cdc42, Rac and RhoA (130). In addition, novel findings demonstrated that endosomoal RhoB also controls Rac1-mediated stabilization of the endothelial barrier (131). Despite these observations, so far, little is known about the role of Rho GTPases and prenylation in EndoCs during intestinal inflammation.
Large GTPases
Among large GTPases, two molecules (dynamin-2 and GBP-1) are of particular importance in the regulation of barrier function. Dynamins are involved in transcellular and paracellular permeability (132). Both, paracellular and transcellular permeabilities are increased in the intestinal epithelium during IBD (133) and are co-regulated in the microvascular endothelium through a compensatory mechanism, involving Rac, Dynamin-2 and actin (132). In general, transcellular permeability is regulated by vesicular transcytosis, which allows the transfer through a cell of macromolecules, such as albumin, by vesicle-mediated endocytosis and exocytosis (134). During transcytosis, invaginations of the plasma membrane (caveolae) are formed and coated by clathrin and actin. Dynamin finally achieves the scission of the nascent vesicle under GTP hydrolysis (134). In addition, Dynamin-2 regulates paracellular permeability through modulation of TJs and AJs. Dynamin-2 is able to bind several AJ and TJ proteins, to link them with the actin cytoskeleton and to ensure the stability of TJs and AJs in the epithelium and the endothelium (135). Furthermore, Dynamins directly interact with actin, foster actin polymerization, and induce actin bundles formation (136). Dynamin-2 is also involved in the maintenance of the apical constriction and the recycling of E-cadherin (137, 138). Dynamin-2 plays a role in barrier maintenance during TNF-induced epithelial shedding (32) and is also involved in the maintenance of the vascular barrier function under hypoxia, by inducing the activity of eNOS (139). Hence Dynamin-2 represents an important regulator of epithelial and endothelial permeability as well as vascular homeostasis.
Members of the human GBP family are involved in immune response against intracellular pathogens and inflammation (140). GBP-1 is the best characterized protein of the seven-member family (140–143). GBP-1 expression is strongly induced by ICs, notably by IFN-γ and has been detected in the inflamed mucosa during IBD (9, 143, 144). GBP-1 has been found to mediate the inhibitory effects of IFN-γ on cell proliferation, migration, and invasion and to inhibit tumor growth and angiogenesis in vivo (85, 86, 145–148). More precisely, GBP-1 can reorganize intracellular actin cytoskeleton in epithelial, endothelial, and T-cells (149, 150). GBP-1 directly interacts with β-actin and inhibits actin stress fiber formation, while co-localizing with cortical actin (149, 151). Actin depolymerization, for instance by latrunculin, has been shown to induce Occludin internalization (152). In addition, GBP-1 was found to localize at TJs both in intestinal crypts of patients with CD and UC and in human IEC lines treated with IFN-γ (144). In this model, the silencing of GBP-1 expression led to increased apoptosis, indicating that it exerts a protective role in epithelium homeostasis (144). However, the role of GBP-1 on cell–cell permeability and junction regulation is still not well understood.
Taken together, large and small GTPases, as well as prenylation, represent novel key players for maintenance of gut homeostasis, regulating epithelial and endothelial integrity under physiological and inflammatory conditions (Figure 1). Despite the here described current knowledge in the field, some still open questions encourage the scientific community in this field to fulfill the description of the molecular mechanism behind these observations. It still remains to be determined to which extend the endothelial barrier participate to IBD pathogenesis and whether angiogenesis or endothelial activation contributes the most to the disease. On the other hand, the description of the role of other Rho GTPases, such as Rac1 or Cdcd42, for epithelial integrity; as well as molecular mechanisms regulating prenylation within IECs, should be further investigated. More detailed studies on inflammation-associated cytoskeleton remodeling within IECs and EndoCs might help in the identification of new target structures for an optimized treatment or early diagnosis of IBD.
Author Contributions
RL-P, MS, and NB-L wrote the manuscript. IA and MN were critically involved in the design of the work and the discussion of the content. All the authors approved the final manuscript.
Conflict of Interest Statement
The authors declare that the research was conducted in the absence of any commercial or financial relationships that could be construed as a potential conflict of interest.
Funding
This work was supported by grants from the German Research Foundation (DFG) (KFO257) [subproject 4], SFB 796 [subproject B9], and FOR2438 [subproject 2] to MS; BR5196/2-1 to NB-L; (KFO257) [subproject 12] to IA; the Interdisciplinary Center for Clinical Research (IZKF) of the Clinical Center Erlangen (to MS and RL-P); and the W. Lutz Stiftung (to MS).
References
1. Zhang K, Hornef MW, Dupont A. The intestinal epithelium as guardian of gut barrier integrity. Cell Microbiol (2015) 17(11):1561–9. doi:10.1111/cmi.12501
2. Pastorelli L, De Salvo C, Mercado JR, Vecchi M, Pizarro TT. Central role of the gut epithelial barrier in the pathogenesis of chronic intestinal inflammation: lessons learned from animal models and human genetics. Front Immunol (2013) 4:280. doi:10.3389/fimmu.2013.00280
3. Spadoni I, Zagato E, Bertocchi A, Paolinelli R, Hot E, Di Sabatino A, et al. A gut-vascular barrier controls the systemic dissemination of bacteria. Science (2015) 350(6262):830–4. doi:10.1126/science.aad0135
4. Koch S, Nusrat A. Dynamic regulation of epithelial cell fate and barrier function by intercellular junctions. Ann N Y Acad Sci (2009) 1165:220–7. doi:10.1111/j.1749-6632.2009.04025.x
5. Schnittler H, Taha M, Schnittler MO, Taha AA, Lindemann N, Seebach J. Actin filament dynamics and endothelial cell junctions: the Ying and Yang between stabilization and motion. Cell Tissue Res (2014) 355(3):529–43. doi:10.1007/s00441-014-1856-2
6. Niessen CM. Tight junctions/adherens junctions: basic structure and function. J Invest Dermatol (2007) 127(11):2525–32. doi:10.1038/sj.jid.5700865
7. Mehta S, Nijhuis A, Kumagai T, Lindsay J, Silver A. Defects in the adherens junction complex (E-cadherin/beta-catenin) in inflammatory bowel disease. Cell Tissue Res (2015) 360(3):749–60. doi:10.1007/s00441-014-1994-6
8. Hatoum OA, Binion DG, Otterson MF, Gutterman DD. Acquired microvascular dysfunction in inflammatory bowel disease: loss of nitric oxide-mediated vasodilation. Gastroenterology (2003) 125(1):58–69. doi:10.1016/S0016-5085(03)00699-1
9. Haep L, Britzen-Laurent N, Weber TG, Naschberger E, Schaefer A, Kremmer E, et al. Interferon gamma counteracts the angiogenic switch and induces vascular permeability in dextran sulfate sodium colitis in mice. Inflamm Bowel Dis (2015) 21(10):2360–71. doi:10.1097/MIB.0000000000000490
10. Fanning AS, Mitic LL, Anderson JM. Transmembrane proteins in the tight junction barrier. J Am Soc Nephrol (1999) 10(6):1337–45.
11. Gumbiner B. Structure, biochemistry, and assembly of epithelial tight junctions. Am J Physiol (1987) 253(6 Pt 1):C749–58.
12. Perez-Moreno M, Jamora C, Fuchs E. Sticky business: orchestrating cellular signals at adherens junctions. Cell (2003) 112(4):535–48. doi:10.1016/S0092-8674(03)00108-9
13. Gates J, Peifer M. Can 1000 reviews be wrong? Actin, alpha-catenin, and adherens junctions. Cell (2005) 123(5):769–72. doi:10.1016/j.cell.2005.11.009
14. Fries W, Belvedere A, Vetrano S. Sealing the broken barrier in IBD: intestinal permeability, epithelial cells and junctions. Curr Drug Targets (2013) 14(12):1460–70. doi:10.2174/1389450111314120011
15. Hartsock A, Nelson WJ. Adherens and tight junctions: structure, function and connections to the actin cytoskeleton. Biochim Biophys Acta (2008) 1778(3):660–9. doi:10.1016/j.bbamem.2007.07.012
16. Harris TJ, Tepass U. Adherens junctions: from molecules to morphogenesis. Nat Rev Mol Cell Biol (2010) 11(7):502–14. doi:10.1038/nrm2927
17. Prasain N, Stevens T. The actin cytoskeleton in endothelial cell phenotypes. Microvasc Res (2009) 77(1):53–63. doi:10.1016/j.mvr.2008.09.012
18. Komarova YA, Kruse K, Mehta D, Malik AB. Protein interactions at endothelial junctions and signaling mechanisms regulating endothelial permeability. Circ Res (2017) 120(1):179–206. doi:10.1161/CIRCRESAHA.116.306534
19. Edens HA, Parkos CA. Modulation of epithelial and endothelial paracellular permeability by leukocytes. Adv Drug Deliv Rev (2000) 41(3):315–28. doi:10.1016/S0169-409X(00)00049-1
20. Prasain N, Alexeyev M, Balczon R, Stevens T. Soluble adenylyl cyclase-dependent microtubule disassembly reveals a novel mechanism of endothelial cell retraction. Am J Physiol Lung Cell Mol Physiol (2009) 297(1):L73–83. doi:10.1152/ajplung.90577.2008
21. Mangold S, Wu SK, Norwood SJ, Collins BM, Hamilton NA, Thorn P, et al. Hepatocyte growth factor acutely perturbs actin filament anchorage at the epithelial zonula adherens. Curr Biol (2011) 21(6):503–7. doi:10.1016/j.cub.2011.02.018
22. Cunningham KE, Turner JR. Myosin light chain kinase: pulling the strings of epithelial tight junction function. Ann N Y Acad Sci (2012) 1258:34–42. doi:10.1111/j.1749-6632.2012.06526.x
23. Blair SA, Kane SV, Clayburgh DR, Turner JR. Epithelial myosin light chain kinase expression and activity are upregulated in inflammatory bowel disease. Lab Invest (2006) 86(2):191–201. doi:10.1038/labinvest.3700373
24. Garcia-Hernandez V, Quiros M, Nusrat A. Intestinal epithelial claudins: expression and regulation in homeostasis and inflammation. Ann N Y Acad Sci (2017) 1397(1):66–79. doi:10.1111/nyas.13360
25. Raleigh DR, Marchiando AM, Zhang Y, Shen L, Sasaki H, Wang Y, et al. Tight junction-associated MARVEL proteins marveld3, tricellulin, and occludin have distinct but overlapping functions. Mol Biol Cell (2010) 21(7):1200–13. doi:10.1091/mbc.E09-08-0734
26. Cohen CJ, Shieh JT, Pickles RJ, Okegawa T, Hsieh JT, Bergelson JM. The coxsackievirus and adenovirus receptor is a transmembrane component of the tight junction. Proc Natl Acad Sci U S A (2001) 98(26):15191–6. doi:10.1073/pnas.261452898
27. Aurrand-Lions M, Johnson-Leger C, Wong C, Du Pasquier L, Imhof BA. Heterogeneity of endothelial junctions is reflected by differential expression and specific subcellular localization of the three JAM family members. Blood (2001) 98(13):3699–707. doi:10.1182/blood.V98.13.3699
28. van der Flier LG, Clevers H. Stem cells, self-renewal, and differentiation in the intestinal epithelium. Annu Rev Physiol (2009) 71:241–60. doi:10.1146/annurev.physiol.010908.163145
29. Günther C, Neumann H, Neurath MF, Becker C. Apoptosis, necrosis and necroptosis: cell death regulation in the intestinal epithelium. Gut (2013) 62(7):1062–71. doi:10.1136/gutjnl-2011-301364
30. Watson AJ, Duckworth CA, Guan Y, Montrose MH. Mechanisms of epithelial cell shedding in the mammalian intestine and maintenance of barrier function. Ann N Y Acad Sci (2009) 1165:135–42. doi:10.1111/j.1749-6632.2009.04027.x
31. Guan Y, Watson AJ, Marchiando AM, Bradford E, Shen L, Turner JR, et al. Redistribution of the tight junction protein ZO-1 during physiological shedding of mouse intestinal epithelial cells. Am J Physiol Cell Physiol (2011) 300(6):C1404–14. doi:10.1152/ajpcell.00270.2010
32. Marchiando AM, Shen L, Graham WV, Edelblum KL, Duckworth CA, Guan Y, et al. The epithelial barrier is maintained by in vivo tight junction expansion during pathologic intestinal epithelial shedding. Gastroenterology (2011) 140(4):1208–18.e1–2. doi:10.1053/j.gastro.2011.01.004
33. Ivanov AI, Parkos CA, Nusrat A. Cytoskeletal regulation of epithelial barrier function during inflammation. Am J Pathol (2010) 177(2):512–24. doi:10.2353/ajpath.2010.100168
34. Coch RA, Leube RE. Intermediate filaments and polarization in the intestinal epithelium. Cells (2016) 5(3):E32. doi:10.3390/cells5030032
35. Crawley SW, Mooseker MS, Tyska MJ. Shaping the intestinal brush border. J Cell Biol (2014) 207(4):441–51. doi:10.1083/jcb.201407015
36. Nusrat A, von Eichel-Streiber C, Turner JR, Verkade P, Madara JL, Parkos CA. Clostridium difficile toxins disrupt epithelial barrier function by altering membrane microdomain localization of tight junction proteins. Infect Immun (2001) 69(3):1329–36. doi:10.1128/IAI.69.3.1329-1336.2001
37. Betanzos A, Javier-Reyna R, García-Rivera G, Bañuelos C, González-Mariscal L, Schnoor M, et al. The EhCPADH112 complex of Entamoeba histolytica interacts with tight junction proteins occludin and claudin-1 to produce epithelial damage. PLoS One (2013) 8(6):e65100. doi:10.1371/journal.pone.0065100
38. Shifflett DE, Clayburgh DR, Koutsouris A, Turner JR, Hecht GA. Enteropathogenic E. coli disrupts tight junction barrier function and structure in vivo. Lab Invest (2005) 85(10):1308–24. doi:10.1038/labinvest.3700330
39. Schmitz H, Barmeyer C, Fromm M, Runkel N, Foss HD, Bentzel CJ, et al. Altered tight junction structure contributes to the impaired epithelial barrier function in ulcerative colitis. Gastroenterology (1999) 116(2):301–9. doi:10.1016/S0016-5085(99)70126-5
40. Gitter AH, Wullstein F, Fromm M, Schulzke JD. Epithelial barrier defects in ulcerative colitis: characterization and quantification by electrophysiological imaging. Gastroenterology (2001) 121(6):1320–8. doi:10.1053/gast.2001.29694
41. Hollander D. Permeability in Crohn’s disease: altered barrier functions in healthy relatives? Gastroenterology (1993) 104(6):1848–51. doi:10.1016/0016-5085(93)90668-3
42. Ukabam SO, Clamp JR, Cooper BT. Abnormal small intestinal permeability to sugars in patients with Crohn’s disease of the terminal ileum and colon. Digestion (1983) 27(2):70–4. doi:10.1159/000198932
43. Mankertz J, Schulzke JD. Altered permeability in inflammatory bowel disease: pathophysiology and clinical implications. Curr Opin Gastroenterol (2007) 23(4):379–83. doi:10.1097/MOG.0b013e32816aa392
44. Yacyshyn BR, Meddings JB. CD45RO expression on circulating CD19+ B cells in Crohn’s disease correlates with intestinal permeability. Gastroenterology (1995) 108(1):132–7. doi:10.1016/0016-5085(95)90017-9
45. D’Incà R, Di Leo V, Corrao G, Martines D, D’Odorico A, Mestriner C, et al. Intestinal permeability test as a predictor of clinical course in Crohn’s disease. Am J Gastroenterol (1999) 94(10):2956–60. doi:10.1016/S0002-9270(99)00500-6
46. Khounlotham M, Kim W, Peatman E, Nava P, Medina-Contreras O, Addis C, et al. Compromised intestinal epithelial barrier induces adaptive immune compensation that protects from colitis. Immunity (2012) 37(3):563–73. doi:10.1016/j.immuni.2012.06.017
47. Pope JL, Bhat AA, Sharma A, Ahmad R, Krishnan M, Washington MK, et al. Claudin-1 regulates intestinal epithelial homeostasis through the modulation of Notch-signalling. Gut (2014) 63(4):622–34. doi:10.1136/gutjnl-2012-304241
48. Tamura A, Kitano Y, Hata M, Katsuno T, Moriwaki K, Sasaki H, et al. Megaintestine in claudin-15-deficient mice. Gastroenterology (2008) 134(2):523–34. doi:10.1053/j.gastro.2007.11.040
49. Al-Sadi R, Ye D, Boivin M, Guo S, Hashimi M, Ereifej L, et al. Interleukin-6 modulation of intestinal epithelial tight junction permeability is mediated by JNK pathway activation of claudin-2 gene. PLoS One (2014) 9(3):e85345. doi:10.1371/journal.pone.0085345
50. Heller F, Florian P, Bojarski C, Richter J, Christ M, Hillenbrand B, et al. Interleukin-13 is the key effector Th2 cytokine in ulcerative colitis that affects epithelial tight junctions, apoptosis, and cell restitution. Gastroenterology (2005) 129(2):550–64. doi:10.1053/j.gastro.2005.05.002
51. Kawashima R, Kawamura YI, Oshio T, Son A, Yamazaki M, Hagiwara T, et al. Interleukin-13 damages intestinal mucosa via TWEAK and Fn14 in mice – a pathway associated with ulcerative colitis. Gastroenterology (2011) 141(6):2119–29.e8. doi:10.1053/j.gastro.2011.08.040
52. Ma TY, Iwamoto GK, Hoa NT, Akotia V, Pedram A, Boivin MA, et al. TNF-alpha-induced increase in intestinal epithelial tight junction permeability requires NF-kappa B activation. Am J Physiol Gastrointest Liver Physiol (2004) 286(3):G367–76. doi:10.1152/ajpgi.00173.2003
53. Blum MS, Toninelli E, Anderson JM, Balda MS, Zhou J, O’Donnell L, et al. Cytoskeletal rearrangement mediates human microvascular endothelial tight junction modulation by cytokines. Am J Physiol (1997) 273(1 Pt 2):H286–94.
54. Capaldo CT, Farkas AE, Hilgarth RS, Krug SM, Wolf MF, Benedik JK, et al. Proinflammatory cytokine-induced tight junction remodeling through dynamic self-assembly of claudins. Mol Biol Cell (2014) 25(18):2710–9. doi:10.1091/mbc.E14-02-0773
55. Capaldo CT, Nusrat A. Cytokine regulation of tight junctions. Biochim Biophys Acta (2009) 1788(4):864–71. doi:10.1016/j.bbamem.2008.08.027
56. Dionne S, Hiscott J, D’Agata I, Duhaime A, Seidman EG. Quantitative PCR analysis of TNF-alpha and IL-1 beta mRNA levels in pediatric IBD mucosal biopsies. Dig Dis Sci (1997) 42(7):1557–66. doi:10.1023/A:1018895500721
57. Breese EJ, Michie CA, Nicholls SW, Murch SH, Williams CB, Domizio P, et al. Tumor necrosis factor alpha-producing cells in the intestinal mucosa of children with inflammatory bowel disease. Gastroenterology (1994) 106(6):1455–66. doi:10.1016/0016-5085(94)90398-0
58. Kiesslich R, Duckworth CA, Moussata D, Gloeckner A, Lim LG, Goetz M, et al. Local barrier dysfunction identified by confocal laser endomicroscopy predicts relapse in inflammatory bowel disease. Gut (2012) 61(8):1146–53. doi:10.1136/gutjnl-2011-300695
59. Kiesslich R, Goetz M, Angus EM, Hu Q, Guan Y, Potten C, et al. Identification of epithelial gaps in human small and large intestine by confocal endomicroscopy. Gastroenterology (2007) 133(6):1769–78. doi:10.1053/j.gastro.2007.09.011
60. Lim LG, Neumann J, Hansen T, Goetz M, Hoffman A, Neurath MF, et al. Confocal endomicroscopy identifies loss of local barrier function in the duodenum of patients with Crohn’s disease and ulcerative colitis. Inflamm Bowel Dis (2014) 20(5):892–900. doi:10.1097/MIB.0000000000000027
61. Irvine EJ, Marshall JK. Increased intestinal permeability precedes the onset of Crohn’s disease in a subject with familial risk. Gastroenterology (2000) 119(6):1740–4. doi:10.1053/gast.2000.20231
62. Peeters M, Geypens B, Claus D, Nevens H, Ghoos Y, Verbeke G, et al. Clustering of increased small intestinal permeability in families with Crohn’s disease. Gastroenterology (1997) 113(3):802–7. doi:10.1016/S0016-5085(97)70174-4
63. Katz KD, Hollander D, Vadheim CM, McElree C, Delahunty T, Dadufalza VD, et al. Intestinal permeability in patients with Crohn’s disease and their healthy relatives. Gastroenterology (1989) 97(4):927–31. doi:10.1016/0016-5085(89)91499-6
64. Söderholm JD, Olaison G, Peterson KH, Franzén LE, Lindmark T, Wirén M, et al. Augmented increase in tight junction permeability by luminal stimuli in the non-inflamed ileum of Crohn’s disease. Gut (2002) 50(3):307–13. doi:10.1136/gut.50.3.307
65. Martinesi M, Ambrosini S, Treves C, Zuegel U, Steinmeyer A, Vito A, et al. Role of vitamin D derivatives in intestinal tissue of patients with inflammatory bowel diseases. J Crohns Colitis (2014) 8(9):1062–71. doi:10.1016/j.crohns.2014.02.005
66. Zhao H, Zhang H, Wu H, Li H, Liu L, Guo J, et al. Protective role of 1,25(OH)2 vitamin D3 in the mucosal injury and epithelial barrier disruption in DSS-induced acute colitis in mice. BMC Gastroenterol (2012) 12:57. doi:10.1186/1471-230X-12-57
67. Pagnini C, Saeed R, Bamias G, Arseneau KO, Pizarro TT, Cominelli F. Probiotics promote gut health through stimulation of epithelial innate immunity. Proc Natl Acad Sci U S A (2010) 107(1):454–9. doi:10.1073/pnas.0910307107
68. Mennigen R, Nolte K, Rijcken E, Utech M, Loeffler B, Senninger N, et al. Probiotic mixture VSL#3 protects the epithelial barrier by maintaining tight junction protein expression and preventing apoptosis in a murine model of colitis. Am J Physiol Gastrointest Liver Physiol (2009) 296(5):G1140–9. doi:10.1152/ajpgi.90534.2008
69. Corridoni D, Pastorelli L, Mattioli B, Locovei S, Ishikawa D, Arseneau KO, et al. Probiotic bacteria regulate intestinal epithelial permeability in experimental ileitis by a TNF-dependent mechanism. PLoS One (2012) 7(7):e42067. doi:10.1371/journal.pone.0042067
70. Sugimoto K, Ogawa A, Mizoguchi E, Shimomura Y, Andoh A, Bhan AK, et al. IL-22 ameliorates intestinal inflammation in a mouse model of ulcerative colitis. J Clin Invest (2008) 118(2):534–44. doi:10.1172/JCI33194
71. Steinhart AH, Hiruki T, Brzezinski A, Baker JP. Treatment of left-sided ulcerative colitis with butyrate enemas: a controlled trial. Aliment Pharmacol Ther (1996) 10(5):729–36. doi:10.1046/j.1365-2036.1996.d01-509.x
72. Vernia P, Annese V, Bresci G, d’Albasio G, D’Incà R, Giaccari S, et al. Topical butyrate improves efficacy of 5-ASA in refractory distal ulcerative colitis: results of a multicentre trial. Eur J Clin Invest (2003) 33(3):244–8. doi:10.1046/j.1365-2362.2003.01130.x
73. Suenaert P, Bulteel V, Lemmens L, Noman M, Geypens B, Van Assche G, et al. Anti-tumor necrosis factor treatment restores the gut barrier in Crohn’s disease. Am J Gastroenterol (2002) 97(8):2000–4. doi:10.1111/j.1572-0241.2002.05914.x
74. Suenaert P, Bulteel V, Vermeire S, Noman M, Van Assche G, Rutgeerts P. Hyperresponsiveness of the mucosal barrier in Crohn’s disease is not tumor necrosis factor-dependent. Inflamm Bowel Dis (2005) 11(7):667–73. doi:10.1097/01.MIB.0000168371.87283.4b
76. Armulik A, Genové G, Mäe M, Nisancioglu MH, Wallgard E, Niaudet C, et al. Pericytes regulate the blood-brain barrier. Nature (2010) 468(7323):557–61. doi:10.1038/nature09522
77. Daneman R, Zhou L, Kebede AA, Barres BA. Pericytes are required for blood-brain barrier integrity during embryogenesis. Nature (2010) 468(7323):562–6. doi:10.1038/nature09513
78. Spadoni I, Pietrelli A, Pesole G, Rescigno M. Gene expression profile of endothelial cells during perturbation of the gut vascular barrier. Gut Microbes (2016) 7(6):540–8. doi:10.1080/19490976.2016.1239681
79. Zhang YZ, Li YY. Inflammatory bowel disease: pathogenesis. World J Gastroenterol (2014) 20(1):91–9. doi:10.3748/wjg.v20.i1.91
80. Danese S, Sans M, de la Motte C, Graziani C, West G, Phillips MH, et al. Angiogenesis as a novel component of inflammatory bowel disease pathogenesis. Gastroenterology (2006) 130(7):2060–73. doi:10.1053/j.gastro.2006.03.054
81. Cromer WE, Mathis JM, Granger DN, Chaitanya GV, Alexander JS. Role of the endothelium in inflammatory bowel diseases. World J Gastroenterol (2011) 17(5):578–93. doi:10.3748/wjg.v17.i5.578
82. Danese S. VEGF in inflammatory bowel disease: a master regulator of mucosal immune-driven angiogenesis. Dig Liver Dis (2008) 40(8):680–3. doi:10.1016/j.dld.2008.02.036
83. Scaldaferri F, Vetrano S, Sans M, Arena V, Straface G, Stigliano E, et al. VEGF-A links angiogenesis and inflammation in inflammatory bowel disease pathogenesis. Gastroenterology (2009) 136(2):585–95.e5. doi:10.1053/j.gastro.2008.09.064
84. Alkim C, Alkim H, Koksal AR, Boga S, Sen I. Angiogenesis in inflammatory bowel disease. Int J Inflam (2015) 2015:970890. doi:10.1155/2015/970890
85. Guenzi E, Töpolt K, Cornali E, Lubeseder-Martellato C, Jörg A, Matzen K, et al. The helical domain of GBP-1 mediates the inhibition of endothelial cell proliferation by inflammatory cytokines. EMBO J (2001) 20(20):5568–77. doi:10.1093/emboj/20.20.5568
86. Guenzi E, Töpolt K, Lubeseder-Martellato C, Jörg A, Naschberger E, Benelli R, et al. The guanylate binding protein-1 GTPase controls the invasive and angiogenic capability of endothelial cells through inhibition of MMP-1 expression. EMBO J (2003) 22(15):3772–82. doi:10.1093/emboj/cdg382
87. Ng CT, Fong LY, Sulaiman MR, Moklas MA, Yong YK, Hakim MN, et al. Interferon-gamma increases endothelial permeability by causing activation of p38 MAP kinase and actin cytoskeleton alteration. J Interferon Cytokine Res (2015) 35(7):513–22. doi:10.1089/jir.2014.0188
88. Marcus BC, Wyble CW, Hynes KL, Gewertz BL. Cytokine-induced increases in endothelial permeability occur after adhesion molecule expression. Surgery (1996) 120(2):411–6; discussion 416–7. doi:10.1016/S0039-6060(96)80317-5
89. Ozaki H, Ishii K, Horiuchi H, Arai H, Kawamoto T, Okawa K, et al. Cutting edge: combined treatment of TNF-alpha and IFN-gamma causes redistribution of junctional adhesion molecule in human endothelial cells. J Immunol (1999) 163(2):553–7.
90. Wong RK, Baldwin AL, Heimark RL. Cadherin-5 redistribution at sites of TNF-alpha and IFN-gamma-induced permeability in mesenteric venules. Am J Physiol (1999) 276(2 Pt 2):H736–48.
91. Gerwert K, Mann D, Kotting C. Common mechanisms of catalysis in small and heterotrimeric GTPases and their respective GAPs. Biol Chem (2017) 398(5–6):523–33. doi:10.1515/hsz-2016-0314
92. Gelb MH, Scholten JD, Sebolt-Leopold JS. Protein prenylation: from discovery to prospects for cancer treatment. Curr Opin Chem Biol (1998) 2(1):40–8. doi:10.1016/S1367-5931(98)80034-3
93. Harris CM, Poulter CD. Recent studies of the mechanism of protein prenylation. Nat Prod Rep (2000) 17(2):137–44. doi:10.1039/a904110i
94. Arnold TR, Stephenson RE, Miller AL. Rho GTPases and actomyosin: partners in regulating epithelial cell-cell junction structure and function. Exp Cell Res (2017) 358(1):20–30. doi:10.1016/j.yexcr.2017.03.053
95. Pleines I, Hagedorn I, Gupta S, May F, Chakarova L, van Hengel J, et al. Megakaryocyte-specific RhoA deficiency causes macrothrombocytopenia and defective platelet activation in hemostasis and thrombosis. Blood (2012) 119(4):1054–63. doi:10.1182/blood-2011-08-372193
96. Ahuja P, Perriard E, Pedrazzini T, Satoh S, Perriard JC, Ehler E. Re-expression of proteins involved in cytokinesis during cardiac hypertrophy. Exp Cell Res (2007) 313(6):1270–83. doi:10.1016/j.yexcr.2007.01.009
97. Birukova AA, Birukov KG, Adyshev D, Usatyuk P, Natarajan V, Garcia JG, et al. Involvement of microtubules and Rho pathway in TGF-beta1-induced lung vascular barrier dysfunction. J Cell Physiol (2005) 204(3):934–47. doi:10.1002/jcp.20359
98. Citalán-Madrid AF, García-Ponce A, Vargas-Robles H, Betanzos A, Schnoor M. Small GTPases of the Ras superfamily regulate intestinal epithelial homeostasis and barrier function via common and unique mechanisms. Tissue Barriers (2013) 1(5):e26938. doi:10.4161/tisb.26938
99. Crespo P, Leon J. Ras proteins in the control of the cell cycle and cell differentiation. Cell Mol Life Sci (2000) 57(11):1613–36. doi:10.1007/PL00000645
100. Heasman SJ, Ridley AJ. Mammalian Rho GTPases: new insights into their functions from in vivo studies. Nat Rev Mol Cell Biol (2008) 9(9):690–701. doi:10.1038/nrm2476
101. Itzen A, Goody RS. GTPases involved in vesicular trafficking: structures and mechanisms. Semin Cell Dev Biol (2011) 22(1):48–56. doi:10.1016/j.semcdb.2010.10.003
102. Praefcke GJ, McMahon HT. The dynamin superfamily: universal membrane tubulation and fission molecules? Nat Rev Mol Cell Biol (2004) 5(2):133–47. doi:10.1038/nrm1313
103. Quiros M, Nusrat A. RhoGTPases, actomyosin signaling and regulation of the epithelial apical junctional complex. Semin Cell Dev Biol (2014) 36:194–203. doi:10.1016/j.semcdb.2014.09.003
104. Ratheesh A, Priya R, Yap AS. Coordinating Rho and Rac: the regulation of Rho GTPase signaling and cadherin junctions. Prog Mol Biol Transl Sci (2013) 116:49–68. doi:10.1016/B978-0-12-394311-8.00003-0
105. Citi S, Guerrera D, Spadaro D, Shah J. Epithelial junctions and Rho family GTPases: the zonular signalosome. Small GTPases (2014) 5(4):1–15. doi:10.4161/21541248.2014.973760
106. Chandhoke SK, Mooseker MS. A role for myosin IXb, a motor-RhoGAP chimera, in epithelial wound healing and tight junction regulation. Mol Biol Cell (2012) 23(13):2468–80. doi:10.1091/mbc.E11-09-0803
107. Terry SJ, Zihni C, Elbediwy A, Vitiello E, Leefa Chong San IV, Balda MS, et al. Spatially restricted activation of RhoA signalling at epithelial junctions by p114RhoGEF drives junction formation and morphogenesis. Nat Cell Biol (2011) 13(2):159–66. doi:10.1038/ncb2156
108. Babbin BA, Jesaitis AJ, Ivanov AI, Kelly D, Laukoetter M, Nava P, et al. Formyl peptide receptor-1 activation enhances intestinal epithelial cell restitution through phosphatidylinositol 3-kinase-dependent activation of Rac1 and Cdc42. J Immunol (2007) 179(12):8112–21. doi:10.4049/jimmunol.179.12.8112
109. Espejo R, Rengifo-Cam W, Schaller MD, Evers BM, Sastry SK. PTP-PEST controls motility, adherens junction assembly, and Rho GTPase activity in colon cancer cells. Am J Physiol Cell Physiol (2010) 299(2):C454–63. doi:10.1152/ajpcell.00148.2010
110. Chen P, Kartha S, Bissonnette M, Hart J, Toback FG. AMP-18 facilitates assembly and stabilization of tight junctions to protect the colonic mucosal barrier. Inflamm Bowel Dis (2012) 18(9):1749–59. doi:10.1002/ibd.22886
111. Elbediwy A, Zihni C, Terry SJ, Clark P, Matter K, Balda MS. Epithelial junction formation requires confinement of Cdc42 activity by a novel SH3BP1 complex. J Cell Biol (2012) 198(4):677–93. doi:10.1083/jcb.201202094
112. Hopkins AM, Walsh SV, Verkade P, Boquet P, Nusrat A. Constitutive activation of Rho proteins by CNF-1 influences tight junction structure and epithelial barrier function. J Cell Sci (2003) 116(Pt 4):725–42. doi:10.1242/jcs.00300
113. Schlegel N, Meir M, Spindler V, Germer CT, Waschke J. Differential role of Rho GTPases in intestinal epithelial barrier regulation in vitro. J Cell Physiol (2011) 226(5):1196–203. doi:10.1002/jcp.22446
114. Eisenhoffer GT, Loftus PD, Yoshigi M, Otsuna H, Chien CB, Morcos PA, et al. Crowding induces live cell extrusion to maintain homeostatic cell numbers in epithelia. Nature (2012) 484(7395):546–9. doi:10.1038/nature10999
115. Segain JP, Raingeard de la Blétière D, Sauzeau V, Bourreille A, Hilaret G, Cario-Toumaniantz C, et al. Rho kinase blockade prevents inflammation via nuclear factor kappa B inhibition: evidence in Crohn’s disease and experimental colitis. Gastroenterology (2003) 124(5):1180–7. doi:10.1016/S0016-5085(03)00283-X
116. Shkoda A, Werner T, Daniel H, Gunckel M, Rogler G, Haller D. Differential protein expression profile in the intestinal epithelium from patients with inflammatory bowel disease. J Proteome Res (2007) 6(3):1114–25. doi:10.1021/pr060433m
117. López-Posadas R, Becker C, Günther C, Tenzer S, Amann K, Billmeier U, et al. Rho-A prenylation and signaling link epithelial homeostasis to intestinal inflammation. J Clin Invest (2016) 126(2):611–26. doi:10.1172/JCI80997
118. Benoit YD, Lussier C, Ducharme PA, Sivret S, Schnapp LM, Basora N, et al. Integrin alpha8beta1 regulates adhesion, migration and proliferation of human intestinal crypt cells via a predominant RhoA/ROCK-dependent mechanism. Biol Cell (2009) 101(12):695–708. doi:10.1042/BC20090060
119. Olofsson B. Rho guanine dissociation inhibitors: pivotal molecules in cellular signalling. Cell Signal (1999) 11(8):545–54. doi:10.1016/S0898-6568(98)00063-1
120. Lee SY, Kim H, Kim K, Lee H, Lee S, Lee D. Arhgap17, a RhoGTPase activating protein, regulates mucosal and epithelial barrier function in the mouse colon. Sci Rep (2016) 6:26923. doi:10.1038/srep26923
121. Stappenbeck TS, Gordon JI. Rac1 mutations produce aberrant epithelial differentiation in the developing and adult mouse small intestine. Development (2000) 127(12):2629–42.
122. Myant KB, Scopelliti A, Haque S, Vidal M, Sansom OJ, Cordero JB. Rac1 drives intestinal stem cell proliferation and regeneration. Cell Cycle (2013) 12(18):2973–7. doi:10.4161/cc.26031
123. Sakamori R, Das S, Yu S, Feng S, Stypulkowski E, Guan Y, et al. Cdc42 and Rab8a are critical for intestinal stem cell division, survival, and differentiation in mice. J Clin Invest (2012) 122(3):1052–65. doi:10.1172/JCI60282
124. Melendez J, Liu M, Sampson L, Akunuru S, Han X, Vallance J, et al. Cdc42 coordinates proliferation, polarity, migration, and differentiation of small intestinal epithelial cells in mice. Gastroenterology (2013) 145(4):808–19. doi:10.1053/j.gastro.2013.06.021
125. Nakata K, Sugi Y, Narabayashi H, Kobayakawa T, Nakanishi Y, Tsuda M, et al. Commensal microbiota-induced microRNA modulates intestinal epithelial permeability through a small GTPase ARF4. J Biol Chem (2017) 292(37):15426–33. doi:10.1074/jbc.M117.788596
126. Wojciak-Stothard B, Ridley AJ. Rho GTPases and the regulation of endothelial permeability. Vascul Pharmacol (2002) 39(4–5):187–99. doi:10.1016/S1537-1891(03)00008-9
127. Essler M, Amano M, Kruse HJ, Kaibuchi K, Weber PC, Aepfelbacher M. Thrombin inactivates myosin light chain phosphatase via Rho and its target Rho kinase in human endothelial cells. J Biol Chem (1998) 273(34):21867–74. doi:10.1074/jbc.273.34.21867
128. Daneshjou N, Sieracki N, van Nieuw Amerongen GP, Conway DE, Schwartz MA, Komarova YA, et al. Rac1 functions as a reversible tension modulator to stabilize VE-cadherin trans-interaction. J Cell Biol (2015) 209(1):181. doi:10.1083/jcb.20140910803202015c
129. Broman MT, Mehta D, Malik AB. Cdc42 regulates the restoration of endothelial adherens junctions and permeability. Trends Cardiovasc Med (2007) 17(5):151–6. doi:10.1016/j.tcm.2007.03.004
130. Wójciak-Stothard B, Entwistle A, Garg R, Ridley AJ. Regulation of TNF-alpha-induced reorganization of the actin cytoskeleton and cell-cell junctions by Rho, Rac, and Cdc42 in human endothelial cells. J Cell Physiol (1998) 176(1):150–65. doi:10.1002/(SICI)1097-4652(199807)176:1<150::AID-JCP17>3.0.CO;2-B
131. Marcos-Ramiro B, García-Weber D, Barroso S, Feito J, Ortega MC, Cernuda-Morollón E, et al. RhoB controls endothelial barrier recovery by inhibiting Rac1 trafficking to the cell border. J Cell Biol (2016) 213(3):385–402. doi:10.1083/jcb.201504038
132. Armstrong SM, Khajoee V, Wang C, Wang T, Tigdi J, Yin J, et al. Co-regulation of transcellular and paracellular leak across microvascular endothelium by dynamin and Rac. Am J Pathol (2012) 180(3):1308–23. doi:10.1016/j.ajpath.2011.12.002
133. Menard S, Cerf-Bensussan N, Heyman M. Multiple facets of intestinal permeability and epithelial handling of dietary antigens. Mucosal Immunol (2010) 3(3):247–59. doi:10.1038/mi.2010.5
134. Ferguson SM, De Camilli P. Dynamin, a membrane-remodelling GTPase. Nat Rev Mol Cell Biol (2012) 13(2):75–88. doi:10.1038/nrm3266
135. Lie PP, Xia W, Wang CQ, Mruk DD, Yan HH, Wong CH, et al. Dynamin II interacts with the cadherin- and occludin-based protein complexes at the blood-testis barrier in adult rat testes. J Endocrinol (2006) 191(3):571–86. doi:10.1677/joe.1.06996
136. Gu C, Yaddanapudi S, Weins A, Osborn T, Reiser J, Pollak M, et al. Direct dynamin-actin interactions regulate the actin cytoskeleton. EMBO J (2010) 29(21):3593–606. doi:10.1038/emboj.2010.249
137. Chua J, Rikhy R, Lippincott-Schwartz J. Dynamin 2 orchestrates the global actomyosin cytoskeleton for epithelial maintenance and apical constriction. Proc Natl Acad Sci U S A (2009) 106(49):20770–5. doi:10.1073/pnas.0909812106
138. Baum B, Georgiou M. Dynamics of adherens junctions in epithelial establishment, maintenance, and remodeling. J Cell Biol (2011) 192(6):907–17. doi:10.1083/jcb.201009141
139. Seerapu Hb, Subramaniam GP, Majumder S, Sinha S, Bisana S, Mahajan S, et al. Inhibition of dynamin-2 confers endothelial barrier dysfunctions by attenuating nitric oxide production. Cell Biol Int (2010) 34(7):755–61. doi:10.1042/CBI20090357
140. Britzen-Laurent N, Herrmann C, Naschberger E, Croner RS, Stürzl M. Pathophysiological role of guanylate-binding proteins in gastrointestinal diseases. World J Gastroenterol (2016) 22(28):6434–43. doi:10.3748/wjg.v22.i28.6434
141. Ghosh A, Praefcke GJ, Renault L, Wittinghofer A, Herrmann C. How guanylate-binding proteins achieve assembly-stimulated processive cleavage of GTP to GMP. Nature (2006) 440(7080):101–4. doi:10.1038/nature04510
142. Prakash B, Praefcke GJ, Renault L, Wittinghofer A, Herrmann C. Structure of human guanylate-binding protein 1 representing a unique class of GTP-binding proteins. Nature (2000) 403(6769):567–71. doi:10.1038/35000617
143. Britzen-Laurent N, Bauer M, Berton V, Fischer N, Syguda A, Reipschläger S, et al. Intracellular trafficking of guanylate-binding proteins is regulated by heterodimerization in a hierarchical manner. PLoS One (2010) 5(12):e14246. doi:10.1371/journal.pone.0014246
144. Schnoor M, Betanzos A, Weber DA, Parkos CA. Guanylate-binding protein-1 is expressed at tight junctions of intestinal epithelial cells in response to interferon-gamma and regulates barrier function through effects on apoptosis. Mucosal Immunol (2009) 2(1):33–42. doi:10.1038/mi.2008.62
145. Weinländer K, Naschberger E, Lehmann MH, Tripal P, Paster W, Stockinger H, et al. Guanylate binding protein-1 inhibits spreading and migration of endothelial cells through induction of integrin alpha4 expression. FASEB J (2008) 22(12):4168–78. doi:10.1096/fj.08-107524
146. Britzen-Laurent N, Lipnik K, Ocker M, Naschberger E, Schellerer VS, Croner RS, et al. GBP-1 acts as a tumor suppressor in colorectal cancer cells. Carcinogenesis (2013) 34(1):153–62. doi:10.1093/carcin/bgs310
147. Naschberger E, Croner RS, Merkel S, Dimmler A, Tripal P, Amann KU, et al. Angiostatic immune reaction in colorectal carcinoma: impact on survival and perspectives for antiangiogenic therapy. Int J Cancer (2008) 123(9):2120–9. doi:10.1002/ijc.23764
148. Lipnik K, Naschberger E, Gonin-Laurent N, Kodajova P, Petznek H, Rungaldier S, et al. Interferon gamma-induced human guanylate binding protein 1 inhibits mammary tumor growth in mice. Mol Med (2010) 16(5–6):177–87. doi:10.2119/molmed.2009.00172
149. Ostler N, Britzen-Laurent N, Liebl A, Naschberger E, Lochnit G, Ostler M, et al. Gamma interferon-induced guanylate binding protein 1 is a novel actin cytoskeleton remodeling factor. Mol Cell Biol (2014) 34(2):196–209. doi:10.1128/MCB.00664-13
150. Forster F, Paster W, Supper V, Schatzlmaier P, Sunzenauer S, Ostler N, et al. Guanylate binding protein 1-mediated interaction of T cell antigen receptor signaling with the cytoskeleton. J Immunol (2014) 192(2):771–81. doi:10.4049/jimmunol.1300377
151. Zou Z, Meng Z, Ma C, Liang D, Sun R, Lan K. Guanylate-binding protein 1 inhibits nuclear delivery of Kaposi’s sarcoma-associated herpesvirus virions by disrupting formation of actin filament. J Virol (2017) 91(16):e00632–17. doi:10.1128/JVI.00632-17
Keywords: epithelium, endothelium, vascular, barriers, gut, junction proteins, inflammation, inflammatory bowel disease
Citation: López-Posadas R, Stürzl M, Atreya I, Neurath MF and Britzen-Laurent N (2017) Interplay of GTPases and Cytoskeleton in Cellular Barrier Defects during Gut Inflammation. Front. Immunol. 8:1240. doi: 10.3389/fimmu.2017.01240
Received: 21 July 2017; Accepted: 19 September 2017;
Published: 05 October 2017
Edited by:
Britta Siegmund, Charité Universitätsmedizin Berlin, GermanyReviewed by:
Oliver Bachmann, Hannover Medical School, GermanyDiane Bimczok, Montana State University, United States
Copyright: © 2017 López-Posadas, Stürzl, Atreya, Neurath and Britzen-Laurent. This is an open-access article distributed under the terms of the Creative Commons Attribution License (CC BY). The use, distribution or reproduction in other forums is permitted, provided the original author(s) or licensor are credited and that the original publication in this journal is cited, in accordance with accepted academic practice. No use, distribution or reproduction is permitted which does not comply with these terms.
*Correspondence: Rocío López-Posadas, cm9jaW8ubG9wZXotcG9zYWRhc0B1ay1lcmxhbmdlbi5kZQ==;
Nathalie Britzen-Laurent, bmF0aGFsaWUuYnJpdHplbi1sYXVyZW50QHVrLWVybGFuZ2VuLmRl