- 1Centre de Recherche en Transplantation et Immunologie UMR1064, INSERM, Université de Nantes, Nantes, France
- 2Institut de Transplantation Urologie Néphrologie (ITUN), CHU Nantes, Nantes, France
- 3Etablissement Français du sang, Nantes, France
- 4CRCINA, INSERM, Université d’Angers, Université de Nantes, Nantes, France
- 5LabEx Transplantex, Université de Strasbourg, France
- 6Nephrology Dialysis Renal Transplantation Center, Assistance Publique des Hôpitaux de Marseille, Hospital de la Conception, UMR 1076, Vascular Research Center of Marseille, INSERM, Aix-Marseille University, Marseille, France
- 7Établissement Français du Sang Alpes Méditerranée, Marseille, France
- 8ADES UMR 7268, CNRS, EFS, Aix-Marseille Université, Marseille, France
- 9CIC Biotherapy, CHU Nantes, Nantes, France
The role of natural killer (NK) cells in organ transplantation is controversial. This study aims to decipher their role in kidney transplant tolerance in humans. Previous studies highlighted several modulated genes involved in NK cell biology in blood from spontaneously operationally tolerant patients (TOLs; drug-free kidney-transplanted recipients with stable graft function). We performed a phenotypic, functional, and genetic characterization of NK cells from these patients compared to kidney-transplanted patients with stable graft function under immunosuppression and healthy volunteers (HVs). Both operationally TOLs and stable patients harbored defective expression of the NKp46 activator receptor and lytic molecules perforin and granzyme compared to HVs. Surprisingly, NK cells from operationally TOLs also displayed decreased expression of the CD16 activating marker (in the CD56Dim NK cell subset). This decrease was associated with impairment of their functional capacities upon stimulation, as shown by lower interferon gamma (IFNγ) production and CD107a membranous expression in a reverse antibody-dependent cellular cytotoxicity (ADCC) assay, spontaneous lysis assays, and lower target cell lysis in the 51Cr release assay compared to HVs. Conversely, despite impaired K562 cell lysis in the 51Cr release assay, patients with stable graft function harbored a normal reverse ADCC and even increased amounts of IFNγ+ NK cells in the spontaneous lysis assay. Altogether, the strong impairment of the phenotype and functional cytotoxic capacities of NK cells in operationally TOLs may accord with the establishment of a pro-tolerogenic environment, despite remaining highly activated after transplantation in patients with stable graft function.
Introduction
Natural killer (NK) lymphoid cells are major components of innate immunity and serve as the first barrier against microbial infection and tumor development (1, 2). NK cells are cytolytic effector cells with two major modes of action. The first is direct lysis of human leukocyte antigen (HLA) class I-deficient target cells, a mode of action also called “spontaneous lysis.” Recognition of self-HLA class I molecules by inhibitory receptors known as killer cell immunoglobulin-like receptors (KIRs) present on NK cells inhibits their cytotoxicity and maintains self-recognition. In the case of the “missing self,” the absence of HLA class I molecules on target cells (i.e., tumor cells) prevents the inhibitory signal from triggering NK cell cytotoxicity (3). The second mode of action of NK cells is lysis induced by the interaction between the Fc receptor CD16 and the Fc fragment of an antibody (Ab) that recognizes foreign antigens on target cells (i.e., infected cells), an action also called antibody-dependent cellular cytotoxicity (ADCC) (4). In both cases, the lytic function of NK cells is dependent on cytolytic molecules, principally granzyme and perforin, and their activation leads to the production of various inflammatory cytokines, including interferon gamma (IFNγ).
In contrast to what was thought few decades ago (5, 6), it has now been clearly established that NK cells play a role in allograft rejections (7–9). The absence of self-major histocompatibility complex class I molecules on an allograft or the activation of the Fc receptor by donor-specific Abs induce NK cell activation, leading to direct cell lysis of the allograft and secretion of pro-inflammatory molecules that promote adaptive immunity (10–14). However, NK cells are two-faced. In addition to playing a role in allograft rejection, they are also involved in transplant tolerance according to studies in animal models (15–18). Allograft tolerance in solid organ transplantation [i.e., allograft acceptance in absence of immunosuppression (IS)] has been achieved in small animal models (19, 20), but translation to non-human primates and humans remains challenging. In clinical settings, protocols for tolerance induction have been attempted in kidney transplantation, mainly chimerism-based tolerance strategies (21), with some success (22–24). Tolerance has also been observed as a result of IS interruption due to non-compliance or medical decision [especially posttransplant lymphoproliferative disorders (PTLDs)] (24, 25). So-called spontaneously operationally tolerant kidney recipients display stable, good graft function for years. They are not immunosuppressed, they respond to immunological challenge (24, 26), and they do not harbor opportunistic infections (24, 25). From a clinical point of view, these patients do not differ from patients with stable graft function under standard IS (24, 25, 27).
This state of “spontaneous” tolerance is more frequently observed in liver transplantation in experimental models (28–30) and in humans, with no less than 80% recipients being tolerant after 10 years of transplantation (31). Interestingly, whereas mechanisms involved in the two situations appear to be different (31–36), compelling data in kidney and liver tolerant recipients have allowed identification of 63 NK-related genes that are specifically regulated in operationally tolerant kidney transplanted patients (32, 34). In the present study, we analyzed the NK cell phenotype and functional capacities from a cohort of patients operationally tolerant to a kidney graft [tolerant patient (TOL)] and compared the results to those from kidney transplanted patients with stable graft function [stable patient (STA)] under IS and healthy volunteers (HVs). We performed a deep phenotyping analysis of NK cells enriched by genotypic analysis of the KIR, perforin, and CD16 genes. In addition, we analyzed the capacity of NK cells to produce IFNγ and to degranulate and lyse target cells, using different assays mimicking the ADCC and spontaneous lysis. No differences were observed in the KIR, perforin, and CD16 genotypes between the three cohorts, but we found a dramatic decrease of perforin+ and NKp46+ NK cell frequencies, which was in turn associated with a decreased frequency of NK cells expressing the CD16 activator receptors in TOLs. These phenotypic changes are associated with broad impairment of both reverse ADCC and spontaneous lysis of NK cells from TOL, with a profound decrease of IFNγ+ and CD107a+ NK cells and chromium release in cytotoxicity assays. Conversely, despite impaired K562 cell lysis in the 51Cr release assay, we observed a normal reverse ADCC and an increased IFNγ+ and CD107a+ NK cells in the spontaneous lysis assay in STA recipients. These data highlight a defective NK cell profile in TOL recipients that may contribute to establishing a favorable microenvironment.
Materials and Methods
Operationally Tolerant Kidney Transplant Patients, STAs, and HVs
Healthy volunteer donors were recruited at the Blood Transfusion Center (EFS, Nantes, France). All subjects gave written informed consent in accordance with the Declaration of Helsinki. HVs were enrolled by the Etablissement Français du Sang (EFS, Nantes, France) within the context of a research contract. A convention has been signed between our laboratory (CRTI—INSERM UMR 1064) and the blood bank (Etablissement Français du Sang Pays de La Loire) and approval of an ethical committee was thus not necessary. The University Hospital Ethical Committee and the Committee for the Protection of Patients from Biological Risks approved the study for patients. The biological samples and data are gathered in accordance with French Law, more specifically with “Bioethical law” of August 6, 2004, Act no. 78-17 of January 6, 1978, on data processing, data, files, and individual liberties, with the European regulation: Directive 2004/23/EC of European Parliament and of the council of March 31, 2004 on setting, standards of quality and safety of donation, procurement, testing, processing, preservation, storage, and distribution of human tissue and cells, and with Directive 95/46/EC on the protection of individuals with regard to the processing of personal data and on the free movement of such data. PBMCs were isolated from blood by gradient centrifugation on Ficoll (Lymphoprep, Axis-Shield, PoC AS, Oslo, Norway). TOLs were defined as patients with stable kidney graft function (creatinemia <150 mmol/L and proteinuria <1 g/24 h) in the absence of IS for at least 1 year, and STAs were characterized by same functional criteria whereas under IS. Demographic and clinical data are presented in Tables 1 and 2. PBMCs of TOL used for the study were frozen cells coming from the DIVAT (Données Informatisées et Validées en Transplantation) biocoll (http://www.divat.fr/biocollection and CNIL number n°891735).
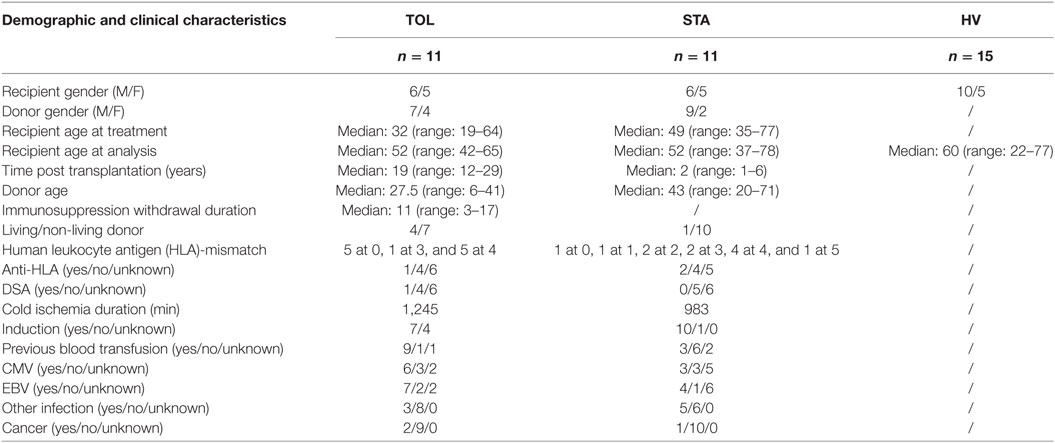
Table 1. Demographic and clinical characteristics from tolerant patient (TOL), stable patient (STA), and healthy volunteer (HV) used for phenotypic analysis and functional assay.
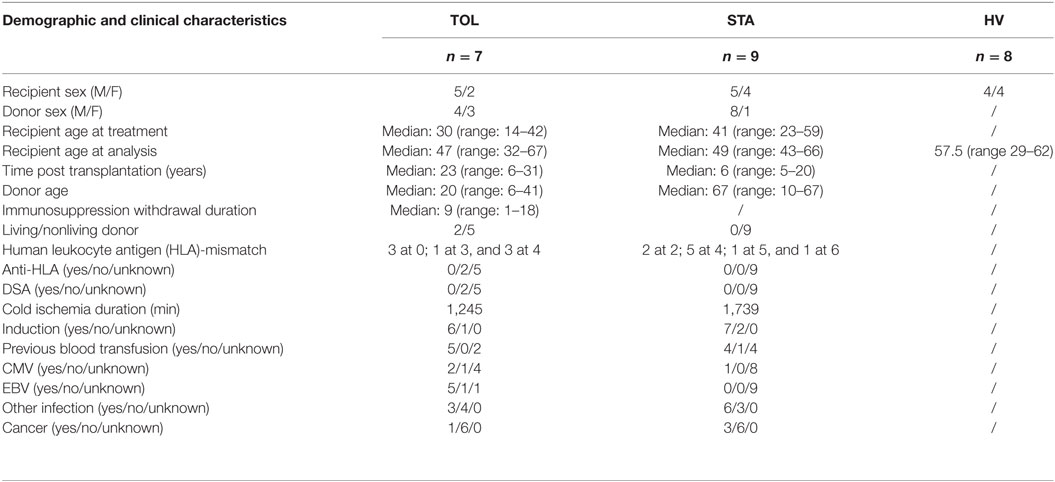
Table 2. Demographic and clinical characteristics from tolerant patient (TOL), stable patient (STA), and healthy volunteers (HV) used for 51Cr release assay.
Cell Lines Culture
The P815 murine, the HLA class I-deficient 721.221 (221) and the K562 cell lines were cultured in RPMI 1640 medium (Life Technologies, Paisley, UK) containing glutamine (Life Technologies) and penicillin-streptomycin (LifeTechnologies). The P815 cell line medium was supplemented with 10% human serum (EFS, Nantes) and the 221 and K562 cell lines medium was supplemented with 10% Fetal Bovin Serum (Life Technologies).
KIR and HLA Genotyping
Genomic DNA was extracted using a classical salting-out method (37). All DNAs were typed for KIR2DL1, 2DL2, 2DL3, 2DL4, 2DL5A/B, 3DL1, 3DL2, 3DL3, 2DS1, 2DS2, 2DS3, 2DS4/1D, 2DS5, and 3DS1, using a KIR multiplex polymerase chain reaction (PCR)-SSP method as previously described (38). Twenty-seven KIR locus-specific primers kindly provided by Pr David Senitzer (City of Hope, Duarte, CA, USA) for research purposesand split into four (G1, G2, G3, and G4) KIR primer-pair groups were used. The first G1 group amplify KIR2DL1, 2DS3, 2DL4, and 2DL2 loci; the second G2 group amplify 3DL1, 2DL3, 2DS2, and 3DS1 loci; the third G3 group amplify 2DS1, 3DL2, and 2DL5 loci; and the last G4 group amplify 2DS5, 3DL3, and 2DS4/1D loci. PCR amplifications were performed in 10 mL of reaction solution, including AmpliTaq Gold enzyme (0.75 U final, ThermoFischer Scientific, Villebon sur Yvette, France) and its bufferMgCl2 (2.25 mM final, ThermoFischer Scientific), dNTP mixtures (0.25 mM final each, ThermoFischer Scientific), G1/G2/G3/G4 primers (0.1–1.8 mM final), and DNA template (150 ng final). The multiplex PCR-SSP for KIR genotyping basic PCR protocol consists of one hold cycle at 93°C, 15 min followed by 32 cycles at 93°C, 20 s; 65°C, 30 s; 72°C, 30 s; and a final extension of 72°C, 5 min. PCR amplifications were performedeither in aGeneAmp 9700 (AppliedBiosystems/ThermoFischer Scientific), a T100 (Biorad, Les Ulis France) or a C1000 (Biorad) thermal cycler. The KIR PCR products were separated by electrophoresis on a homemade 3% agarose gel (Metaphor®, Ozyme, France) in 1X TBE (Sigma-Aldrich, Saint Quentin, France) buffer. KIR genes were identified depending on the length of each specific KIR amplification obtained in the four KIR primer-pair groups. High-resolution typing for HLA-A, HLA-B, and HLA-C loci (HLA laboratory, EFS Nantes, France) was carried out on samples by a Sequence Based Typing kit (Abbott Molecular Park, IL, USA). KIR genotypes were determined based on the presence or absence of activating KIR, KIR AA genotype presenting only KIR2DS4 as activating KIR, and KIR B+ genotype presenting several activating KIR. KIR ligand (i.e., A3/A11, Bw4, C1, and C2) were defined based on allelic HLA class I typing.
Phenotype and Functional Assays by Flow Cytometry
Peripheral blood mononuclear cells were stained with Abs against CD3 (SK7), CD56 (NCAM16.2), CD16 (NKP15), CD8 (HIT8a), CD161 (DX12), ILT2 (GH1/75), CD57 (HNK-1), CD226 (DNAM-1) (DX11), NKp46 (9E2), granzyme A (CB9), perforin (γG9), NKG2D (1D11), CD4 (RPA-T4), CD8 (HIT8a), γ2TCR (B6), CD38 (HB7) (BD Biosciences), NKG2C (134591) (R&D Systems), NKp44 (Z231), NKp30 (Z25), NKG2A (Z199), KIR2DL1/S1 (EB6), KIR2DL2/3/2DS2 (GL183), KIR3DL1/S1 (Z27), HLA-DR (Immu357), KIR2DS4 (FES172), pan γδTCR (IMMU510) (Beckman Coulter, Fullerton, CA, USA), KIR2DL1 (143211) (R&D Systems), CD14 (RMO-52) (EFS, Rennes), KIR2DL2/3/2DS2 (4A8) (39), KIR2DL3/S2 (1F12) (39), and HLA-A,-B,-C (W6/32) (Biolegend). PBMCs were preincubated with anti-CD107a (H4A3; BD Biosciences, San Jose, CA, USA). NK cell degranulation was assessed after incubation for 5 h with media (negative control), with 721.221(221) (E: T ratio of 1:1), or with P815 cell line after a preincubation with CD16 specific mAb or IgG control at 10 µg/mL. For the last 4 h of incubation, the cells were treated with brefeldin A (Sigma) at 10 mg/mL to allow the intracellular accumulation of IFNγ. The cells were stained and permeabilized before intracellular IFNγ staining with PE-anti-human IFNγ (B27, BD Biosciences). Flow cytometry was performed using a FACSCalibur apparatus with CellQuest software (BD Biosciences) and analyzed using FlowJo 7.6.1 software (Tree Star, Ashland, OR, USA). The assay of PBMC incubation with P815 cell line and CD16 specific Ab was used to mimic the ADCC and interaction of the anti-CD16 Ab with the CD16 of NK cells, leading to their activation, IFNγ production and degranulation, which was measured by CD107a surface expression. Stimulation assay of PBMCs with the 221 cell line mimicked the direct NK cell activity, which was assessed by analyzing IFNγ production and CD107a surface expression.
EVOS® Fluorescence Cell Imaging
Natural killer cells were enriched after T-cell depletion of PBMCs using a CD3-specific Ab (BD Biosciences, San Jose, CA, USA) and murine IgG-coupled magnetic Dynabeads according to the manufacturer’s instructions (Dynal, Oslo, Norway). Enriched NK cells were activated with the 221 cell line as previously describes in the functional assay section. NK cells were stained with anti-NKp46 (9E2, BD Biosciences) and fixed with PFA 4%. Cells were then permeabilized in a PBS 0.1% saponin solution, and perforin intracellular expression was measured using anti-Perforin (Dg9, BD Biosciences) after 1 h incubation at room temperature. Samples were mounted with the ProLong® Gold reagent (Invitrogen). After 48 h, cells were imaged using a ×60/0.075 NA oil immersion lens and acquired using phase contrast and fluorescence imaging by EVOS® fluorescence microscope (ThermoFisher Scientific). Two light cubes were combined to identify NKp46-Alexa fluor 647 (RFP) and perforin-Alexa fluor 568 (Cy5) expressions.
Perforin Gene Sequencing
Genomic DNA was analyzed to identify potential mutation on the perforin gene. Genomic DNA was isolated from peripheral blood using the standard phenol–chloroform protocol. We used the protocol described by Molleran Lee et al. (40) Briefly, exons 2 and 3 of the perforin gene were amplified by PCR using the following primers: for exon 2, 5′-CCCTTCCATGTGCCCTGATAATC-3′ and 5′-AAGCAGCCTCCAAGTTTGATTG-3′, and for exon 3, 5′-CCAGTCCTAGTTCTGCCCACTTAC-3′ and 5′-GAACCCCTTCAGTCCAAGCATAC-3′. For amplification, we used 500 ng of gDNA in 1× PCR buffer, 1.5 mmol/L of MgCl2, 0.2 mM of each dNTP, 0.4 µmol of each primer (forward and reverse), and 2.5 U of Taq pol (Invitrogen). Reaction conditions were 3 min at 95°C; 30 cycles of 45 s at 95°C, 30 s at 60°C, and 1 min 45 s at 72°C; and a last step of 10 min at 72°C. For the sequencing of the PCR product, we used the same primers as for DNA amplification for exon 2, and for exon 3 we used the same forward primer and two other reverse primers, one to better analyzed the 3′ end, 5′-TTGGTCTAATGGGAATACGAAG-3′ and one for the internal exon 3, 5′-CCATCACACCTCCATTAACGA-3′. DNA PCR products were sequenced using ABI BigDye terminator reactions and run on AB3730 capillary sequencer.
51Cr Release Assay
Cytotoxicity assay was performed in triplicate in a standard chromium release assay. K562 cells were labeled with 100 μCi Na51CrO4 (NEZ030, Perkin Elmer, Courtaboeuf, France) for 1 h at 37°C, and 1 × 103 target cells were mixed with PBMCs at various effector/target ratios (100:1, 25:1, and 6.25:1). After 4 h at 37°C, 25 µL aliquots of supernatants were each mixed with 100 µL of scintillation liquid (OptiphaseSupermix, Wallack, United Kingdom) for measurement of radioactive content on a beta plate counter (Microbeta Jet 1450, PerkinElmer). The percentage of target cell lysis was calculated according to the following formula: [(experimental release − spontaneous release)/(maximum release − spontaneous release)] × 100. Maximum and spontaneous releases were, respectively, determined by adding 0.1% Triton X-100 or RPMI 1640 10% FBS on 51Cr-labeled K562 cells.
Statistical Analysis
Statistical analyses were performed with Prism-6 software (GraphPad Software). The non-parametric Kruskal–Wallis test was used for comparisons of multiple groups followed by Dunn’s post-test to compare all paired of columns. Continuous non-parametric variables are expressed as medians (min and max). Non-parametric Spearman test was used for correlation analysis. Significance was defined as p less than 0.05. *p < 0.05, **p < 0.01, ***p < 0.001, and ****p < 0.0001.
Results
Circulating NK Cells from TOL Harbor a Defect in Expression of NKp46+ and CD16+ Activating Receptors and Perforin and Granzyme A Cytotoxic Molecules
We performed an exhaustive analysis of the phenotype of NK cells from TOL, STA, and HV. All analyses were conducted in CD3−CD56+ cells divided into two CD56Bright (major producer of cytokines by NK) and CD56Dim (mostly responsible of cytotoxicity by NK) subsets as shown in Figure 1A. This phenotypic analysis included activation receptors (NKG2D, NKG2C, NKp30, Nkp44, NKp46, 2B4, DNAM-1, CD16, CD161); inhibitory receptors (ILT2, KIR2DL1/S1, KIR2DL2/3/S2, NKG2A); and maturation (CD57), activation (CD38), and cytotoxic markers (perforin and granzyme A). Both TOL and STA displayed normal NK cell frequency compared to HV (Figure 1B) and we did not observe any significant difference the expression level of CD56. Interestingly, TOL had a significant decrease in the percentage of NKp46+CD56Dim NK cells (p < 0.001 in TOL vs HV) associated with decreased expression of NKp46 compared with HV and STA (Figures 1C,D) (Table S1 in Supplementary Material). This defect is associated with a decrease of CD16+ NK cells in TOL (Figures 1C,D) (median and range are given in Table S1 in Supplementary Material). Granzyme and perforin are major instrumental molecules for cytotoxic NK cell activity. In steady-state conditions, CD56Bright and CD56Dim NK cells from TOL and STA displayed a significantly lower frequency of perforin+ NK cells associated with decreased expression per se in their granules (median and range are given in Table S1 in Supplementary Material) (Figures 2A,B) compared to HV. This pattern was associated with lower expression of granzyme A in CD56Bright and CD56Dim NK cell subsets (TOL vs HV, p < 0.05) (Figures 2C,D) (median and range are given in Table S1 in Supplementary Material). Altogether, these data suggest that despite a normal peripheral frequency, NK cells, particularly the CD56Dim subset, from TOL have an impoverished profile, with decreased expression of major NK cell activator receptors. At steady state, NK cells from transplanted patients had dramatic lower levels of perforin and NK cells from TOL in particular displayed significantly lower levels of granzyme A but less significant than perforin.
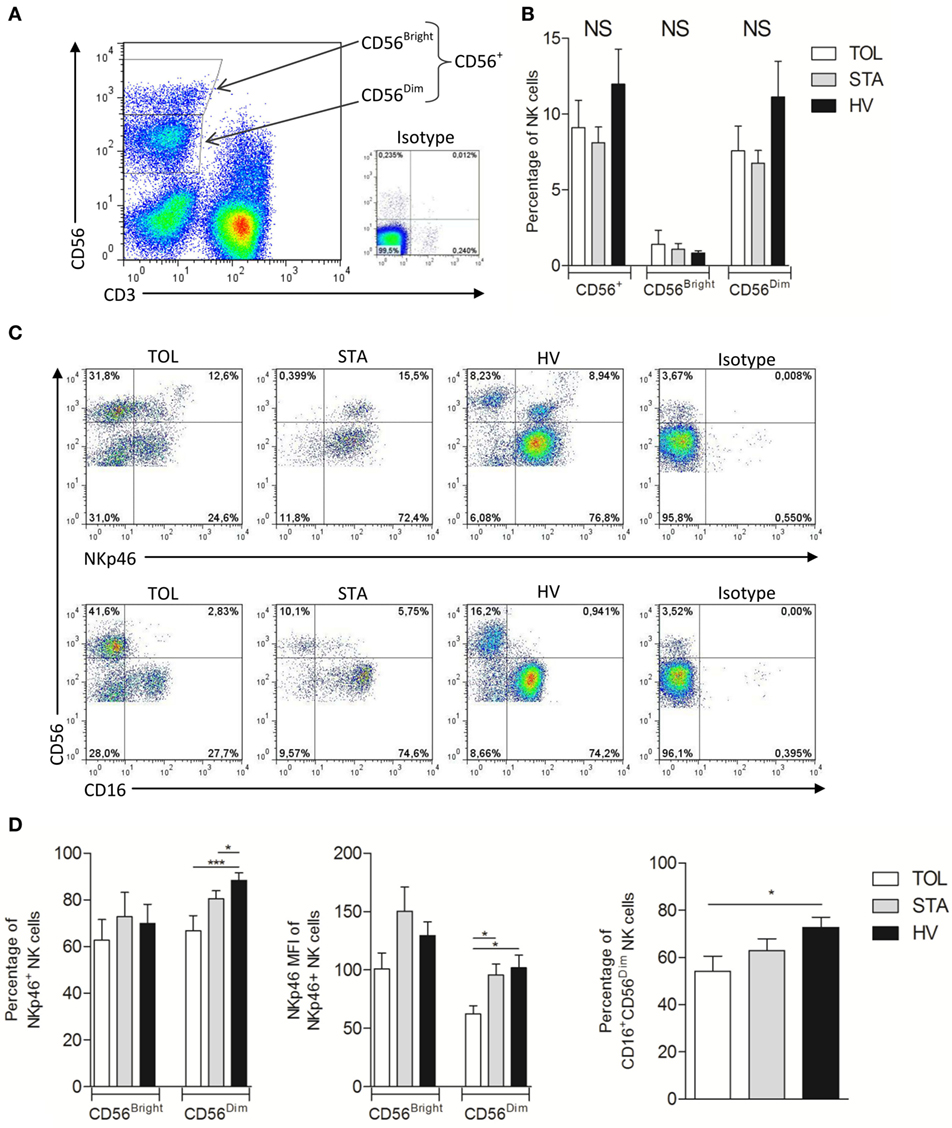
Figure 1. (A) Dotplot representation of the gates performed depending on CD3 and CD56 expression to divide natural killer (NK) cells into CD56Bright and CD56Dim subsets. Cells on the dotplot are lymphocytes gated regarding the morphology based on the SSC and FSC. Isotype controls are shown in the little dotplot. (B) Mean percentage with SEM of CD56+, CD56Bright, and CD56Dim NK cells in tolerant patient (TOL) (white bar) (n = 11), stable patient (STA) (gray bar) (n = 11), and healthy volunteer (HV) (black bar) (n = 15). (C) Representative dotplot of NKp46 (up) and CD16 (down) expression in NK cells in TOL (left), STA (middle), HV (right), and isotype controls (far right). (D) Mean percentage with SEM of NKp46+ NK cells (left) and mean of MFI with SEM of NKp46 (middle) among CD56Bright or CD56Dim NK cells and mean percentage with SEM of CD16+CD56Dim NK cells (right) in TOL (white bar) (n = 11), STA (gray bar) (n = 11), and HV (black bar) (n = 15). Significance was defined as *p < 0.05, **p < 0.01, ***p < 0.001, and ****p < 0.0001.
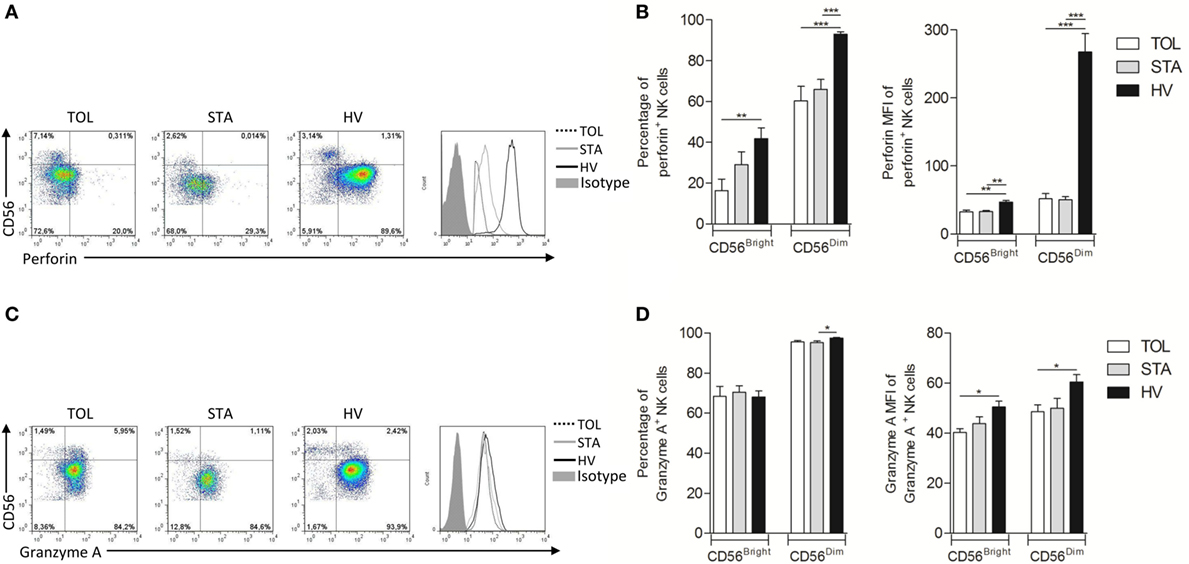
Figure 2. (A) Representative dotplot of perforin expression in tolerant patient (TOL) (left), STA (middle), and healthy volunteers (HV) (right); and histogram of perforin expression in CD56Dim natural killer (NK) cells in TOL (dotted line), STA (gray line), HV (black line), and isotype control in the filled histogram. (B) Mean percentage with SEM of perforin+ NK cells and mean of MFI with SEM of perforin in NK cells in TOL (white bar) (n = 11), STA (gray bar) (n = 12), and HV (black bar) (n = 15) among CD56Bright or CD56Dim. (C) Representative dotplot of granzyme A expression in TOL (left), STA (middle), and HV (right); and histogram of granzyme A expression in CD56Dim NK cells in TOL (dotted line), STA (gray line), HV (black line), and isotype control in the filled histogram. (D) Mean percentage with SEM of granzyme A+ NK cells and mean of MFI with SEM of granzyme A in NK cells in TOL (white bar) (n = 11), STA (gray bar) (n = 12) and HV (Black bar) (n = 15) among CD56Bright or CD56Dim. (Median and range are given in Table S1 in Supplementary Material.). Significance was defined as *p < 0.05, **p < 0.01, ***p < 0.001, and ****p < 0.0001.
NK Cells from TOL Harbor a Cytotoxic Functional Impairment
Because phenotypic analysis of NK cells from transplanted patients revealed important modifications with regard to activation of receptors and cytotoxic molecules, particularly for TOLs, we analyzed the functional capacities of these cells to determine if these phenotypic defects affect their function. Three different and complementary functional assays were performed to analyze the cytotoxic capacities of NK cells. The first was a functional assay based on 51Cr release by an HLA-Class I-negative K562 cell line following lysis by NK cells to analyze the “self-missing” process in the functional cytotoxic capacities of NK cells. We found a significant decrease in 51Cr released by K562 when cells are in contact with NK cells from TOL and STA compared to HV (Figure 3A). No difference was observed between TOL and STA, which suggested the same level of self-missing NK cell reactivity regardless of effector/target cell ratios (100:1, 25:1, and 6.25:1) (Figure 3A). We then used a second functional assay, in which peripheral blood mononuclear cells (PBMCs) were cultured with the 221 cell line lacking HLA class I expression. With this assay, we analyzed the direct lysis activity of NK cells by measuring IFNγ production, which indicated the activation status of NK cells and CD107a cell surface expression, which in turn reflected their degranulation process capacities. We found that IFNγ+CD56Dim and CD107a+CD56Dim NK cells were significantly decreased in TOL compared to STA and HV (p < 0.01 and p < 0.05, respectively, for IFNγ+, Table S2 in Supplementary Material and Figures 3B,C), whereas both IFNγ+CD56Dim, CD107a+CD56Dim NK cells and IFNγ+CD56bright, CD107a+CD56bright NK cells were increased in STA (Figures 3B,C). Interestingly, we observed a correlation between the percentage of IFNγ+ NK cells and the CD107a+ NK cells in the spontaneous lysis assay, suggesting that the cells that produced less IFNγ also had impairment in their degranulation process (Figure 3D). Moreover, we also observed a correlation between the expression of the NKp46 activating receptor and the level of NK cells IFNγ+CD107a+CD56Dim, which suggests that the NK cells impairment in the lysis process was due to the lower level of the activating receptor (Figure 3D). Finally, we performed a third test mimicking the lysis of target cells by ADCC in which we again measured IFNγ production and CD107a cell surface expression upon activation via CD16. In accordance with previous results, TOL had a decrease in IFNγ+CD56Dim NK cells and CD107a+CD56Dim NK cells (P < 0.05 in TOL vs HV for IFNγ) after anti-CD16 mAb stimulation, suggesting a deficit of activation and degranulation compared with NK cells from HV and STA (Table S2 in Supplementary Material; Figures 3E,F). As in the previous assay, we observed a correlation between IFNγ+ NK cells and CD107a+ NK cells, linking low cytokine production and a reduction in the degranulation process (Figure 3G). We also observed a correlation between the percentage of CD16+ NK cells and the percentage of IFNγ+CD107a+CD56Dim NK cells (Figure 3G), suggesting that the lower percentage of CD16+ NK cells in TOL is partly responsible for the reversed ADCC impairment. Interestingly, analysis of IFNγ+CD107a+CD56Dim NK cells after spontaneous lysis and reverse ADCC revealed a strong correlation between the two functional tests (r = 0.7087, p < 0.0001) (Figure S1 in Supplementary Material), meaning that patients who have impaired spontaneous lysis also have impaired reverse ADCC. Finally, we used an Invasive EVOS® fluorescence cell imaging microscopic analysis, in which enriched NK cells from TOL, STA, or HV were cocultured for 5 h with the 221 cell line to measure intracellular perforin expression and NKp46 cell surface expression. We confirmed the very low level of perforin and NKp46 cell surface expression in NK cells from TOL after stimulation. By contrast, both were expressed in STA and HV (Figure 4). Altogether, the three complementary functional assays showed that NK cells from TOL display dramatically lower cytotoxicity through ADCC and mostly through the direct lysis and harbor a defect in IFNγ, perforin cells, and perforin/granzyme secretion at steady state and upon stimulation.
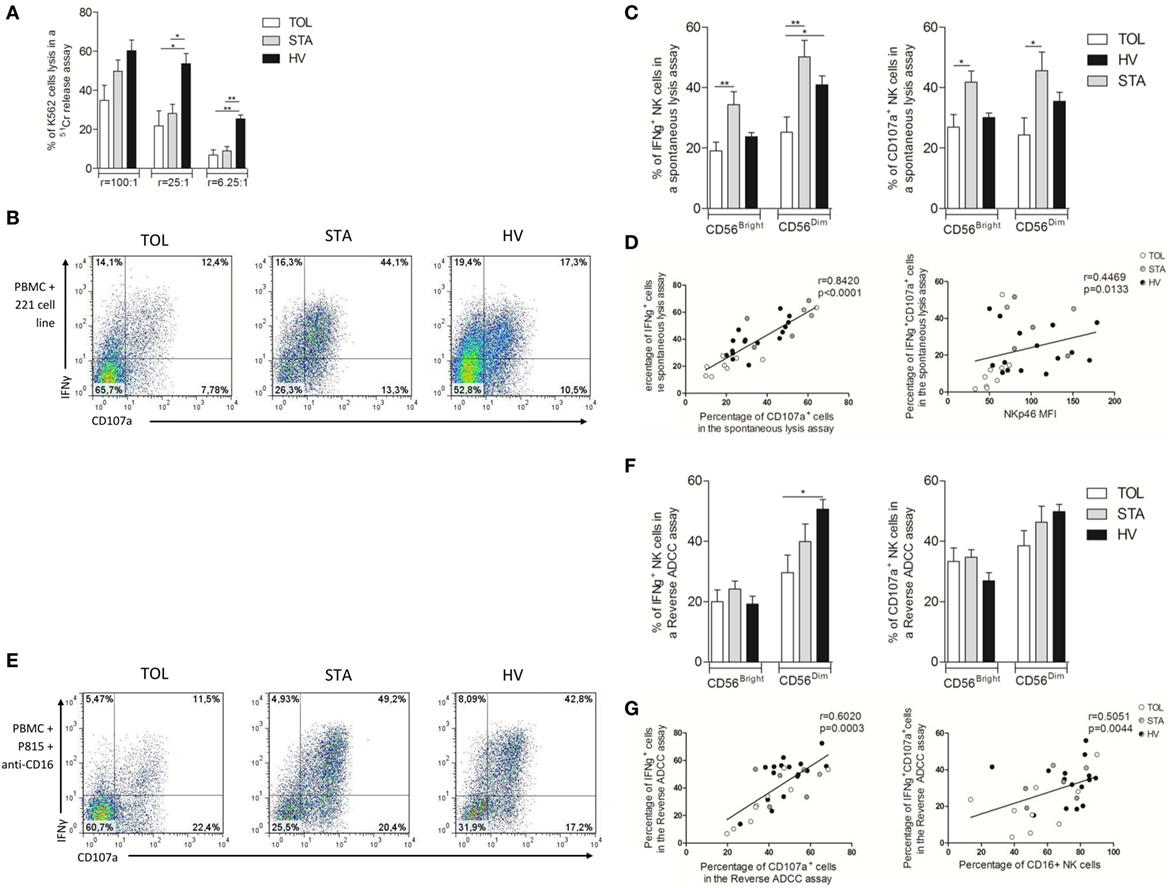
Figure 3. (A) Mean percentage with SEM of K562 lysis based on 51Cr release. Peripheral blood mononuclear cells (PBMCs) from tolerant patient (TOL), stable patient (STA), or healthy volunteer (HV) were cocultured with K562, and 51Cr release by K562 following lysis by PBMCs was evaluated. Three different PBMC:K562 ratios were analyzed: 100:1, 25:1, and 6.25:1. TOL (n = 7) are represented by white bars, STA (n = 9) by gray bars, and HV (n = 8) by black bars. (Median and range are given in Table S2 in Supplementary Material.) (B) Representative dot plots illustrating CD107a membranous expression and IFNγ production by CD56Dim NK cells from one representative TOL, STA, and HV in a spontaneous lysis functional assay. (C) Mean percentage with SEM of IFNγ+ NK cells and the mean percentage with SEM of CD107a+ NK cells among CD56Bright or CD56Dim NK cells from TOL (white bars) (n = 9), STA (gray bars) (n = 7), and HV (black bars) (n = 15) following 5 h of coculture with 221 cell lines. (D) Correlation analysis between the percentage of IFNγ+ NK cells and the percentage of CD107a+ NK cells in the spontaneous lysis assay and correlation between the percentage of IFNγ+CD107a+ NK cells and the level of NKp46 (MFI) (r = Spearman correlation coefficient) (TOL = white dot, STA = gray dot, and HV = black dot). (Median and range are given in Table S2 in Supplementary Material.) (E) Representative dot plots illustrating CD107a membranous expression and IFNγ production by CD56Dim NK cells from one representative TOL, STA, and HV in a reverse antibody-dependent cellular cytotoxicity (ADCC) assay. (Median and range are given in Table S2 in Supplementary Material.). (F) Mean percentage with SEM of IFNγ+ NK cells and the mean percentage with SEM of CD107a+ NK cells among CD56Bright or CD56Dim NK cells from TOL (white bars) (n = 9), STA (gray bars) (n = 7), and HV (black bars) (n = 15) following 5 h of coculture with P815 cell lines and anti-CD16. (G) Correlation analysis between the percentage of IFNγ+ NK cells and the percentage of CD107a+ NK cells in the reverse ADCC assay and correlation between the percentage of IFNγ+CD107a+ NK cells and the percentage of CD16+ NK cells (r = Spearman correlation coefficient) (TOL = white dot, STA = gray dot, and HV = black dot). Significance was defined as *p < 0.05, **p < 0.01, ***p < 0.001, and ****p < 0.0001.
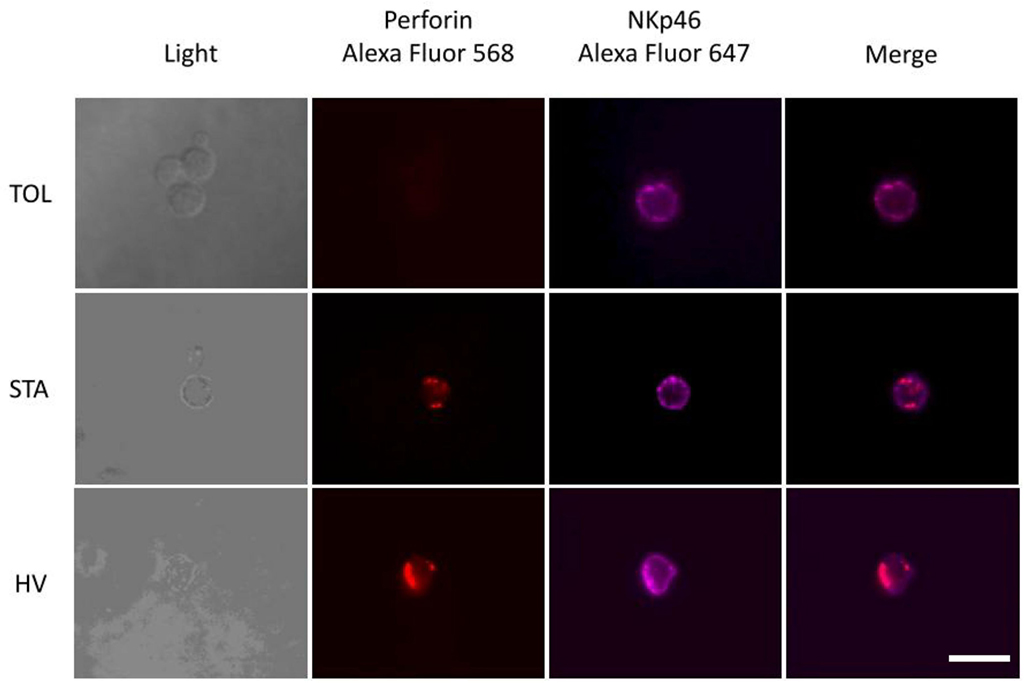
Figure 4. Microscopic analysis of perforin and NKp46 expression. Perforin and NKp46 expression were analyzed following 5 h coculture of peripheral blood mononuclear cells (PBMC) with 221 cell lines. Images were captured by using EVOS systems with light (Left) and fluorescent detectors perforin (AF568), NKp46 (Cy5), or an overlay of all fluorescent channels (Right). Examples are shown for one tolerant patient (TOL) (top), one stable patient (STA) (middle), and one healthy volunteer (HV) (bottom). NK cells were activated following the spontaneous lysis assay protocol and then labeled with anti-perforin-Alexa Fluor 568 and anti-NKp46-Alexa Fluor 647.
NK Cell Function Impairment in TOL Is Not Associated with Modification of Their KIR Genetic Profile
Killer cell immunoglobulin-like receptors are a family of receptors present on NK cells, which define their response according to their inhibitory and/or activating profiles. Because some genes are known to be differentially expressed at the transcriptional level in NK cells from TOL (27, 32, 34), we wondered if their KIR profile might also be associated with genetic modification. We analyzed both their KIR gene content and KIR gene frequencies by a multiplex PCR-SSP method (Table 3). Representative electrophoresis results of KIR multiplex PCR-SSP products and the corresponding KIR amplification patterns are shown in Figure 5A. KIR gene frequencies in TOLs were very similar to those in HV, with the only exception being a decreased frequency of the KIR2DS5 gene in TOL (p = 0.046) (Figure 5B; Table 3), representing an activating KIR receptor for which the ligand is still unknown. No difference was observed for inhibitory KIR genes (Figure 5B). As for HV, TOL KIR genotyping mainly showed the AB KIR gene (Figure 5C). Flow cytometry analysis of activating and inhibitory KIR expression in NK cells did not highlight any difference of mean fluorescence intensity (MFI) or cell frequency between TOL and HV (data not shown). Altogether, these data indicate that NK cell function impairment in TOL is not associated with modification of the KIR genetic profile.
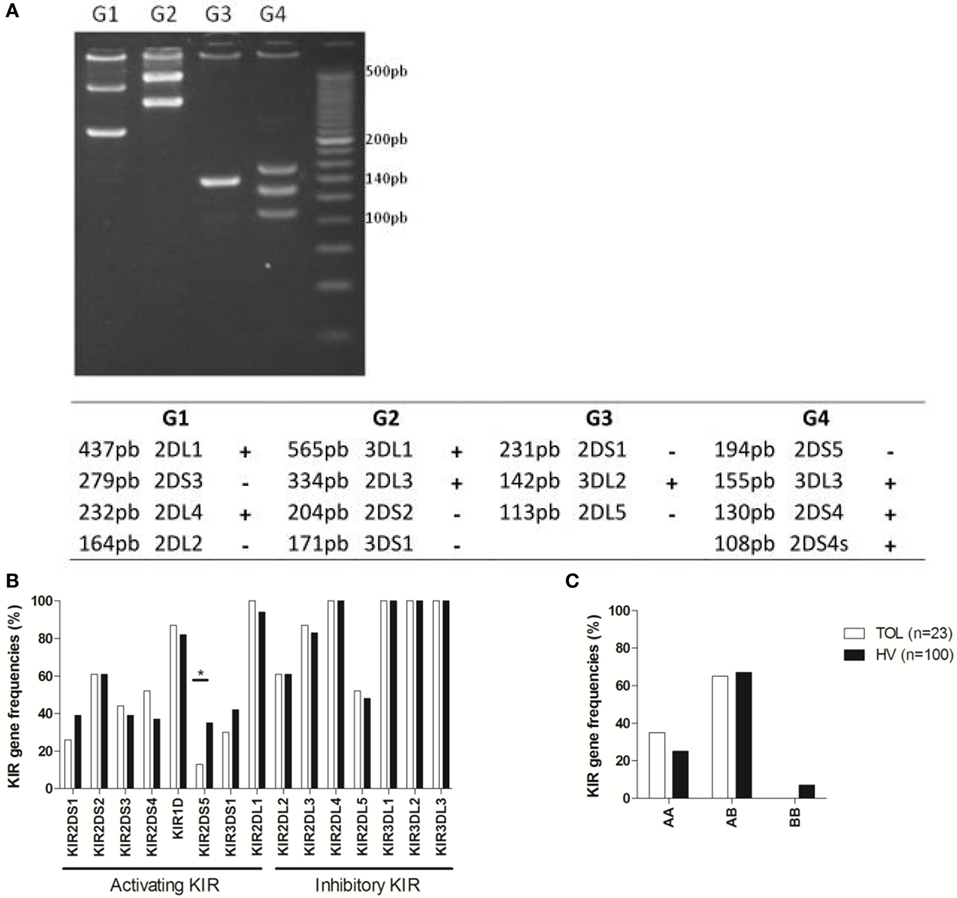
Figure 5. Representative 3% agarose gel electrophoresis of KIR multiplex polymerase chain reaction-SSP products and corresponding KIR amplification patterns and KIR profile in kidney transplanted patients. (A) The figure represents the presence (+) or absence (−) of each KIR gene in one representative tolerant patient (TOL) with the four groups of primers (G1, G2, G3, and G4) allowing the detection of all functional KIR genes. KIR primer-pair groups provide short amplicons from 108 to 565 bp. (B) Representation of the KIR genotype frequencies TOL (n = 23) and healthy volunteer (HV) (n = 100). (C) KIR genotype AA, AB, and BB frequencies in TOL (n = 23) and HV (n = 100). Significance was defined as *p < 0.05, **p < 0.01, ***p < 0.001, and ****p < 0.0001.
TOL Do Not Harbor a Specific Perforin Genomic DNA Mutation Profile or CD16 Polymorphism
Defective perforin has been described in immune pathologies due to mutations in the perforin gene (41). Because of the strong decrease of perforin expression by NK cells from TOL at steady state and upon stimulation, we analyzed the perforin gene mutation levels following the amplification of exons 2 and 3 of the perforin gene in TOL, STA, and HV genomic DNA sequences. Classical and non-pathological mutations are present in the three groups at the same frequencies (C272 > T, C822 > T, C900 > T) (Table 4), suggesting no modification of the perforin genomic DNA phenotype in TOL that could be associated with their functional NK cell defect. Finally, because a CD16 polymorphism could influence ADCC as well as CD16 cell surface expression (42), we explored CD16 polymorphism in our patients. However, we found no particular differences among the three groups (data not shown).
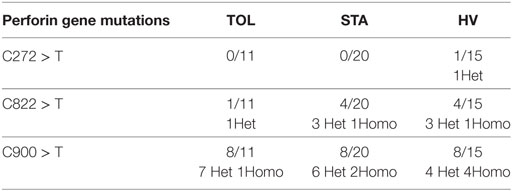
Table 4. Mutations observed in the perforin gene from tolerant patient (TOL), stable patient (STA), and healthy volunteer (HV).
Discussion
The role of NK cells in organ transplantation is controversial. Although early studies suggested that they are not implicated in mice lacking B or T cells that retain NK cells and do not reject their graft (5), NK cells have also been identified in chronic allograft vasculopathy lesions (14, 43, 44) in Ab-mediated rejection (45–47) and rejection of bone marrow allografts in humans (48). We previously reported a specific transcriptional profile in the blood of patients tolerating a kidney graft that showed modulated expression of NK cell genes (32, 34). In the present study, we investigated their profile by analyzing the cells’ exhaustive activated/inhibitory phenotype, their functional cytotoxic capacities, KIR and CD16 genotypes, and extent of perforin gene mutation. The NK cell profile from TOL was compared with NK cells from STA and HV. This double comparison has two purposes. First, the absence of IS in the TOL and HV groups may not interfere with NK cell biology and function, and second, the comparison between TOL and STA allowed integrating the impact of the graft on NK cells and IS treatment on NK cells (49–51).
TOL patients harbor normal NK cell peripheral frequency and number (CD3−CD56+, CD56Dim, and CD56Bright NK cell) in accordance with physiological levels of molecules associated with NK cell differentiation [CD57 (52), CD161 (53), NKG2A, KIR molecules (54)] (not shown). However, these patients harbor a defective profile of activation with a decreased frequency of activating KIR2DS5 gene and NK cells that express NKp46 and CD16, suggesting that their activation is impaired. In comparison, STA also displayed a decreased frequency of NKp46+ NK cells, but they had normal CD16 expression. The lower expression level of these molecules, which play an important role in effector functions of NK cells, including both cell cytotoxicity and cytokine release (55–62), strongly suggests a defect in the functional activity of NK cells in TOL.
Natural killer cells activity is regulated by activating or inhibitory receptors and in accordance with their unique phenotype, we observed a strong impairment of the function of NK cells from TOL. Specifically, there was a profound decrease of IFNγ+ and CD107a+ NK cells in both ADCC and spontaneous lysis and a decrease of 51Cr release, which is according with decreased levels of the activating receptors, NKp46 and CD16. In association with the prominent defect in lysing K562 target cells and producing IFNγ upon stimulation, NK cells from TOL dramatically lacked intracellular perforin and harbored lower expression of granzyme A. By contrast, whereas NK cells from STA also had lower 51Cr release, they displayed a normal ADCC and even had higher spontaneous lysis compared to HV.
A key question is why NK cells from TOL patients express lower amounts of these molecules. Although their levels of expression may vary with age (63), age was not a confounding factor in this study (Table 1). The lower CD16 expression does not correspond with any particular CD16 polymorphism in TOL that could explain this lower ADCC activity. Similarly, whereas KIR polymorphism is associated with various infections, autoimmune diseases, and cancers and has a major role in the structure and the function of NK cells (64), genetic analysis did not reveal any major differential KIR expression on the surface of NK cells from TOL that could explain their impairment. Finally, the defect of perforin of NK from TOL is not due to an abnormal mutation level of the perforin gene, which is not surprising given the absence of associated related pathologies in these patients (41). We suggest that this low perforin level is due to transduction or translation regulation via external factors that could also act on granzyme A, CD16, and NKp46. Surprisingly, we also found a lack of intracellular perforin in STA at steady state despite normal ADCC and spontaneous lysis. Since it is known that IS dramatically influences NK cell function (49–51), we hypothesize an effect of IS. Indeed, after stimulation and culture in IS-free medium (Invasive EVOS® fluorescence cell imaging microscopic analysis) when perforin production is no longer inhibited by the treatment, we clearly confirm the absence of perforin only in NK cells from TOL, whereas it is strongly expressed in STA. The high level of IFNγ+ NK cells and the recovery of perforin expression in NK cells following the spontaneous lysis assay strongly suggests that the impairment of NK cell cytotoxicity in STA patients in the 51Cr assay is not IFNγ or perforin dependent and implicates other pathways.
It has been shown that NKp46+ and CD16+ receptors could be downregulated upon stimulation (65–67). Indeed, in acute myeloid leukemia (AML) it is known that AML cells induce NK cells abnormalities, including the CD16 and NKp46 activating receptor downregulation but also the apoptosis (68, 69). In addition, low expression of NKp46 has been reported as an “evasion mechanism” associated with low cell activity in cancer (70), mainly following chronic stimulation (71), or described as an “exhausted” profile in chronically infected patients (72, 73), patients with cancer (Kaposi sarcoma, PTLD) (74, 75), a mechanism involved in peripheral tolerance. In the same way, chronic exposure to allo-reactive donor antigens from the transplant strongly stimulates NK cells and induces downregulation of these receptors. We hypothesize that the low NKp46 expression likely participates in a “pro-tolerant milieu” as previously reported (76–78), with establishment of a non-deleterious environment or “active shut-down process” to avoid excessive response in these patients following environmental events.
Thus, in the last decade, the status of NK cells has gone from “detrimental role” to “no role” and then to “beneficial role” in the field of transplantation. In animal models as in humans, NK cells have, thus, been shown to have beneficial effects and may be potent regulators of immunity. They delay allograft rejection by downregulating homeostatic CD8+ T-cell proliferation by competing for IL15 (15, 79), and they are able to inhibit clonal expansion of antigen-stimulated T cells (15, 80, 81) in addition to killing dendritic cells (17, 18, 82). Thus, NK cells clearly influence graft outcome and one challenge is to reconcile their role with their potential tolerizing or facilitating role in transplantation. Finally, NK cells are engaged in crosstalk with different immune cells, such as monocytes (83, 84), B cells (85), and Treg cells (86, 87) via mechanisms involving TGFβ (86) or contact dependency (88). In a same way, NK cell degenerative functions are mediated by IL21 derived from autoreactive CD4+ T cells (89). Moreover, a link between NK cells and increased induction of Tregs has been clearly established (90, 91) in correlation with higher TGFβ level in inflammatory situations (92). This general “regulatory” profile fits with the NK cell profile of TOL that correlates with increased frequency of Treg cells with higher immunosuppressive properties (93), higher numbers of granzyme B regulatory B cells (94), and regulation by IL21 secretion by CD4+ T cells (94) in a TGFβ environment (95). Finally, these data agree with recent data showing a clear link between NK cytotoxicity and DSA since these patients rarely develop donor-specific Ab (24, 27).
We hypothesize that this “favorable” or “shut-down” environment contribute pro-tolerogenic conditions, where each cell may play a role. How these cells interact, orchestrate, and/or control each other and their capacity to maintain homeostatic environment remain to explore. Despite this profound defect NK cells, which constitute the first line of innate and adaptive defense against infection and tumors, TOL do not experience more infections than healthy individuals and are able to respond normally to stimulation such as vaccination (25). This suggests that this profile does not affect their innate immunity, and it is likely strongly regulated to maintain “healthy homeostasis” in these patients. This study paves the way for dissecting more deeply the interplay of these immune cells. These observations may have critical implications for the discovery of new effective tolerance strategies to monitor NK cells in transplantation.
Ethics Statement
Healthy volunteer (HV) donors were recruited at the Blood Transfusion Center (EFS, Nantes, France). All subjects gave written informed consent in accordance with the Declaration of Helsinki. HVs were enrolled by the Etablissement Français du Sang (EFS, Nantes, France) within the context of a research contract. A convention has been signed between our laboratory (CRTI—INSERM UMR 1064) and the blood bank (Etablissement Français du Sang Pays de La Loire) and approval of an ethical committee was, thus, not necessary. The University Hospital Ethical Committee and the Committee for the Protection of Patients from Biological Risks approved the study for patients. The biological samples and data are gathered in accordance with French Law, more specifically with “Bioethical law” of August 6, 2004, Act no. 78-17 of January 6, 1978, on data processing, data, files, and individual liberties, with the European regulation: Directive 2004/23/EC of European Parliament and of the council of March 31, 2004 on setting, standards of quality and safety of donation, procurement, testing, processing, preservation, storage, and distribution of human tissue and cells, and with Directive 95/46/EC on the protection of individuals with regard to the processing of personal data and on the free movement of such data.
Author Contributions
SB and CR designed the study; ED, GD, RO, J-PJ, and CP acquired data; MC, PG, MG, and J-PS collected and provided important samples; ED, RD, KG, ND, PP, CP, SC, NG, CR, and SB analyzed and interpreted data; ED, CR, and SB wrote the manuscript; and all authors approved the final version of the manuscript.
Conflict of Interest Statement
The authors declare that the research was conducted in the absence of any commercial or financial relationships that could be construed as a potential conflict of interest.
Acknowledgments
We are most grateful to the Genomics platform of Nantes (Bio-genouest Genomics) core facility for its technical support. We thank the biological resource center for bio-banking (CHU Nantes, Hôtel-Dieu, Centre de resources biologiques (CRB), Nantes, F-44093, France (BRIF: BB-0033-00040)). We thank Pr David Senitzer, City of Hope National Cancer Center, Duarte USA for KIR primers provided. This work was realized in the context of the “Fondation CENTAURE” (RTRS), which supports a French research network in transplantation, and in the context of the Lab-EX IGO program supported by the National Research Agency via the investment of the future program ANR-11-LABX-0016-01. This work was also supported by the FP7 VISICORT project, which has received funding from the European Union’s Seventh Framework Programme for research, technological development and demonstration under grant agreement 602470. This research was also supported by INSERM, DHU, and IHU-CESTI institutes receiving financial support from the French Government managed by the National Research Agency (investment into the future program ANR-10-IBHU-005), Nantes Metropole, and the Pays de la Loire Region. This work was also financially supported by the EtablissementFrançais du Sang (EFS)/Pays de la Loire and by grants from the International Research Group on unrelated HEmatopoietic stem cell Transplantation (IRGHET), Agence de Biomédecine and LeucémieEspoirAtlantiqueFamille (LEAF).
Supplementary Material
The Supplementary Material for this article can be found online at http://www.frontiersin.org/article/10.3389/fimmu.2017.01721/full#supplementary-material.
Abbreviations
51Cr, chromium 51; ADCC, antibody-dependent cellular cytotoxicity; APC, antigen-presenting cell; CNI, calcineurin inhibitor; DSA, donor-specific antibody; HLA, human leukocyte antigen; HV, healthy volunteer; IFNγ, interferon gamma; IS, immunosuppression; KIR, killer cell immunoglobulin-like receptors; MFI, mean fluorescence intensity; MHC, major histocompatibility complex; NK, natural killer; PBMC, peripheral blood mononuclear cell; PCR, polymerase chain reaction; PTLD, posttransplant lymphoproliferative disorder; STA, stable patient; SSP, sequence specific primer; TOL, tolerant patient; Tregs, regulatory T cells.
References
1. Lee SH, Miyagi T, Biron CA. Keeping NK cells in highly regulated antiviral warfare. Trends Immunol (2007) 28(6):252–9. doi:10.1016/j.it.2007.04.001
2. Smyth MJ, Hayakawa Y, Takeda K, Yagita H. New aspects of natural-killer-cell surveillance and therapy of cancer. Nat Rev Cancer (2002) 2(11):850–61. doi:10.1038/nrc928
3. Ljunggren HG, Karre K. In search of the ‘missing self’: MHC molecules and NK cell recognition. Immunol Today (1990) 11(7):237–44. doi:10.1016/0167-5699(90)90097-S
4. Renard V, Cambiaggi A, Vely F, Blery M, Olcese L, Olivero S, et al. Transduction of cytotoxic signals in natural killer cells: a general model of fine tuning between activatory and inhibitory pathways in lymphocytes. Immunol Rev (1997) 155:205–21. doi:10.1111/j.1600-065X.1997.tb00953.x
5. Heidecke CD, Araujo JL, Kupiec-Weglinski JW, Abbud-Filho M, Araneda D, Stadler J, et al. Lack of evidence for an active role for natural killer cells in acute rejection of organ allografts. Transplantation (1985) 40(4):441–4. doi:10.1097/00007890-198510000-00020
6. Markus PM, van den Brink M, Cai X, Harnaha J, Palomba L, Hiserodt JC, et al. Effect of selective depletion of natural killer cells on allograft rejection. Transplant Proc (1991) 23(1 Pt 1):178–9.
7. Kitchens WH, Uehara S, Chase CM, Colvin RB, Russell PS, Madsen JC. The changing role of natural killer cells in solid organ rejection and tolerance. Transplantation (2006) 81(6):811–7. doi:10.1097/01.tp.0000202844.33794.0e
8. van der Touw W, Bromberg JS. Natural killer cells and the immune response in solid organ transplantation. Am J Transplant (2010) 10(6):1354–8. doi:10.1111/j.1600-6143.2010.03086.x
9. Rajalingam R. The impact of HLA class I-specific killer cell immunoglobulin-like receptors on antibody-dependent natural killer cell-mediated cytotoxicity and organ allograft rejection. Front Immunol (2016) 7:585. doi:10.3389/fimmu.2016.00585
10. Hadad U, Martinez O, Krams SM. NK cells after transplantation: friend or foe. Immunol Res (2014) 58(2–3):259–67. doi:10.1007/s12026-014-8493-4
11. Kummer JA, Wever PC, Kamp AM, ten Berge IJ, Hack CE, Weening JJ. Expression of granzyme A and B proteins by cytotoxic lymphocytes involved in acute renal allograft rejection. Kidney Int (1995) 47(1):70–7. doi:10.1038/ki.1995.8
12. Vivier E, Raulet DH, Moretta A, Caligiuri MA, Zitvogel L, Lanier LL, et al. Innate or adaptive immunity? The example of natural killer cells. Science (2011) 331(6013):44–9. doi:10.1126/science.1198687
13. Narni-Mancinelli E, Jaeger BN, Bernat C, Fenis A, Kung S, De Gassart A, et al. Tuning of natural killer cell reactivity by NKp46 and Helios calibrates T cell responses. Science (2012) 335(6066):344–8. doi:10.1126/science.1215621
14. Hirohashi T, Chase CM, Della Pelle P, Sebastian D, Alessandrini A, Madsen JC, et al. A novel pathway of chronic allograft rejection mediated by NK cells and alloantibody. Am J Transplant (2012) 12(2):313–21. doi:10.1111/j.1600-6143.2011.03836.x
15. Zecher D, Li Q, Oberbarnscheidt MH, Demetris AJ, Shlomchik WD, Rothstein DM, et al. NK cells delay allograft rejection in lymphopenic hosts by downregulating the homeostatic proliferation of CD8+ T cells. J Immunol (2010) 184(12):6649–57. doi:10.4049/jimmunol.0903729
16. Beilke JN, Kuhl NR, Van Kaer L, Gill RG. NK cells promote islet allograft tolerance via a perforin-dependent mechanism. Nat Med (2005) 11(10):1059–65. doi:10.1038/nm1296
17. Yu G, Xu X, Vu MD, Kilpatrick ED, Li XC. NK cells promote transplant tolerance by killing donor antigen-presenting cells. J Exp Med (2006) 203(8):1851–8. doi:10.1084/jem.20060603
18. Laffont S, Seillet C, Ortaldo J, Coudert JD, Guery JC. Natural killer cells recruited into lymph nodes inhibit alloreactive T-cell activation through perforin-mediated killing of donor allogeneic dendritic cells. Blood (2008) 112(3):661–71. doi:10.1182/blood-2007-10-120089
19. Owen RD, Wood HR, Foord AG, Sturgeon P, Baldwin LG. Evidence for actively acquired tolerance to Rh antigens. Proc Natl Acad Sci U S A (1954) 40(6):420–4. doi:10.1073/pnas.40.6.420
20. Billingham RE, Brent L, Medawar PB. Actively acquired tolerance of foreign cells. Nature (1953) 172(4379):603–6. doi:10.1038/172603a0
21. Sykes M, Sachs DH. Mixed allogeneic chimerism as an approach to transplantation tolerance. Immunol Today (1988) 9(1):23–7. doi:10.1016/0167-5699(88)91352-7
22. Scandling JD, Busque S, Dejbakhsh-Jones S, Benike C, Millan MT, Shizuru JA, et al. Tolerance and chimerism after renal and hematopoietic-cell transplantation. N Engl J Med (2008) 358(4):362–8. doi:10.1056/NEJMoa074191
23. Kawai T, Cosimi AB, Spitzer TR, Tolkoff-Rubin N, Suthanthiran M, Saidman SL, et al. HLA-mismatched renal transplantation without maintenance immunosuppression. N Engl J Med (2008) 358(4):353–61. doi:10.1056/NEJMoa071074
24. Massart A, Pallier A, Pascual J, Viklicky O, Budde K, Spasovski G, et al. The DESCARTES-Nantes survey of kidney transplant recipients displaying clinical operational tolerance identifies 35 new tolerant patients and 34 almost tolerant patients. Nephrol Dial Transplant (2016) 31(6):1002–13. doi:10.1093/ndt/gfv437
25. Roussey-Kesler G, Giral M, Moreau A, Subra JF, Legendre C, Noël C, et al. Clinical operational tolerance after kidney transplantation. Am J Transplant (2006) 6(4):736–46. doi:10.1111/j.1600-6143.2006.01280.x
26. Ballet C, Roussey-Kesler G, Aubin JT, Brouard S, Giral M, Miqueu P, et al. Humoral and cellular responses to influenza vaccination in human recipients naturally tolerant to a kidney allograft. Am J Transplant (2006) 6(11):2796–801. doi:10.1111/j.1600-6143.2006.01533.x
27. Brouard S, Pallier A, Renaudin K, Foucher Y, Danger R, Devys A, et al. The natural history of clinical operational tolerance after kidney transplantation through twenty-seven cases. Am J Transplant (2012) 12(12):3296–307. doi:10.1111/j.1600-6143.2012.04249.x
28. Calne RY, Sells RA, Pena JR, Davis DR, Millard PR, Herbertson BM, et al. Induction of immunological tolerance by porcine liver allografts. Nature (1969) 223(5205):472–6. doi:10.1038/223472a0
29. Qian S, Demetris AJ, Murase N, Rao AS, Fung JJ, Starzl TE. Murine liver allograft transplantation: tolerance and donor cell chimerism. Hepatology (1994) 19(4):916–24. doi:10.1016/0270-9139(94)90292-5
30. Kamada N, Shinomiya T. Clonal deletion as the mechanism of abrogation of immunological memory following liver grafting in rats. Immunology (1985) 55(1):85–90.
31. Martínez-Llordella M, Puig-Pey I, Orlando G, Ramoni M, Tisone G, Rimola A, et al. Multiparameter immune profiling of operational tolerance in liver transplantation. Am J Transplant (2007) 7(2):309–19. doi:10.1111/j.1600-6143.2006.01621.x
32. Lozano JJ, Pallier A, Martinez-Llordella M, Danger R, López M, Giral M, et al. Comparison of transcriptional and blood cell-phenotypic markers between operationally tolerant liver and kidney recipients. Am J Transplant (2011) 11(9):1916–26. doi:10.1111/j.1600-6143.2011.03638.x
33. Newell KA, Asare A, Kirk AD, Gisler TD, Bourcier K, Suthanthiran M, et al. Identification of a B cell signature associated with renal transplant tolerance in humans. J Clin Invest (2010) 120(6):1836–47. doi:10.1172/JCI39933
34. Sagoo P, Perucha E, Sawitzki B, Tomiuk S, Stephens DA, Miqueu P, et al. Development of a cross-platform biomarker signature to detect renal transplant tolerance in humans. J Clin Invest (2010) 120(6):1848–61. doi:10.1172/JCI39922
35. Baron D, Ramstein G, Chesneau M, Echasseriau Y, Pallier A, Paul C, et al. A common gene signature across multiple studies relate biomarkers and functional regulation in tolerance to renal allograft. Kidney Int (2015) 87(5):984–95. doi:10.1038/ki.2014.395
36. Pallier A, Hillion S, Danger R, Giral M, Racapé M, Degauque N, et al. Patients with drug-free long-term graft function display increased numbers of peripheral B cells with a memory and inhibitory phenotype. Kidney Int (2010) 78(5):503–13. doi:10.1038/ki.2010.162
37. Miller SA, Dykes DD, Polesky HF. A simple salting out procedure for extracting DNA from human nucleated cells. Nucleic Acids Res (1988) 16(3):1215. doi:10.1093/nar/16.3.1215
38. Sun JY, Gaidulis L, Miller MM, Goto RM, Rodriguez R, Forman SJ, et al. Development of a multiplex PCR-SSP method for killer-cell immunoglobulin-like receptor genotyping. Tissue Antigens (2004) 64(4):462–8. doi:10.1111/j.1399-0039.2004.00303.x
39. David G, Morvan M, Gagne K, Kerdudou N, Willem C, Devys A, et al. Discrimination between the main activating and inhibitory killer cell immunoglobulin-like receptor positive natural killer cell subsets using newly characterized monoclonal antibodies. Immunology (2009) 128(2):172–84. doi:10.1111/j.1365-2567.2009.03085.x
40. Molleran Lee S, Villanueva J, Sumegi J, Zhang K, Kogawa K, Davis J, et al. Characterisation of diverse PRF1 mutations leading to decreased natural killer cell activity in North American families with haemophagocytic lymphohistiocytosis. J Med Genet (2004) 41(2):137–44. doi:10.1136/jmg.2003.011528
41. Voskoboinik I, Trapani JA. Perforinopathy: a spectrum of human immune disease caused by defective perforin delivery or function. Front Immunol (2013) 4:441. doi:10.3389/fimmu.2013.00441
42. Oboshi W, Watanabe T, Matsuyama Y, Kobara A, Yukimasa N, Ueno I, et al. The influence of NK cell-mediated ADCC: structure and expression of the CD16 molecule differ among FcgammaRIIIa-V158F genotypes in healthy Japanese subjects. Hum Immunol (2016) 77(2):165–71. doi:10.1016/j.humimm.2015.11.001
43. Uehara S, Chase CM, Colvin RB, Russell PS, Madsen JC. Further evidence that NK cells may contribute to the development of cardiac allograft vasculopathy. Transplant Proc (2005) 37(1):70–1. doi:10.1016/j.transproceed.2005.01.052
44. Uehara S, Chase CM, Kitchens WH, Rose HS, Colvin RB, Russell PS, et al. NK cells can trigger allograft vasculopathy: the role of hybrid resistance in solid organ allografts. J Immunol (2005) 175(5):3424–30. doi:10.4049/jimmunol.175.5.3424
45. Hidalgo LG, Sellares J, Sis B, Mengel M, Chang J, Halloran PF. Interpreting NK cell transcripts versus T cell transcripts in renal transplant biopsies. Am J Transplant (2012) 12(5):1180–91. doi:10.1111/j.1600-6143.2011.03970.x
46. Hidalgo LG, Sis B, Sellares J, Campbell PM, Mengel M, Einecke G, et al. NK cell transcripts and NK cells in kidney biopsies from patients with donor-specific antibodies: evidence for NK cell involvement in antibody-mediated rejection. Am J Transplant (2010) 10(8):1812–22. doi:10.1111/j.1600-6143.2010.03201.x
47. Legris T, Picard C, Todorova D, Lyonnet L, Laporte C, Dumoulin C, et al. Antibody-dependent NK cell activation is associated with late kidney allograft dysfunction and the complement-independent alloreactive potential of donor-specific antibodies. Front Immunol (2016) 7:288. doi:10.3389/fimmu.2016.00288
48. Murphy WJ, Kumar V, Bennett M. Acute rejection of murine bone marrow allografts by natural killer cells and T cells. Differences in kinetics and target antigens recognized. J Exp Med (1987) 166(5):1499–509. doi:10.1084/jem.166.5.1499
49. Neudoerfl C, Mueller BJ, Blume C, Daemen K, Stevanovic-Meyer M, Keil J, et al. The peripheral NK cell repertoire after kidney transplantation is modulated by different immunosuppressive drugs. Front Immunol (2013) 4:46. doi:10.3389/fimmu.2013.00046
50. Meehan AC, Mifsud NA, Nguyen TH, Levvey BJ, Snell GI, Kotsimbos TC, et al. Impact of commonly used transplant immunosuppressive drugs on human NK cell function is dependent upon stimulation condition. PLoS One (2013) 8(3):e60144. doi:10.1371/journal.pone.0060144
51. Hoffmann U, Neudorfl C, Daemen K, Keil J, Stevanovic-Meyer M, Lehner F, et al. NK cells of kidney transplant recipients display an activated phenotype that is influenced by immunosuppression and pathological staging. PLoS One (2015) 10(7):e0132484. doi:10.1371/journal.pone.0132484
52. Bjorkstrom NK, Riese P, Heuts F, Andersson S, Fauriat C, Ivarsson MA, et al. Expression patterns of NKG2A, KIR, and CD57 define a process of CD56dim NK-cell differentiation uncoupled from NK-cell education. Blood (2010) 116(19):3853–64. doi:10.1182/blood-2010-04-281675
53. Freud AG, Yokohama A, Becknell B, Lee MT, Mao HC, Ferketich AK, et al. Evidence for discrete stages of human natural killer cell differentiation in vivo. J Exp Med (2006) 203(4):1033–43. doi:10.1084/jem.20052507
54. Beziat V, Descours B, Parizot C, Debre P, Vieillard V. NK cell terminal differentiation: correlated stepwise decrease of NKG2A and acquisition of KIRs. PLoS One (2010) 5(8):e11966. doi:10.1371/journal.pone.0011966
55. Della Chiesa M, Romeo E, Falco M, Balsamo M, Augugliaro R, Moretta L, et al. Evidence that the KIR2DS5 gene codes for a surface receptor triggering natural killer cell function. Eur J Immunol (2008) 38(8):2284–9. doi:10.1002/eji.200838434
56. Pessino A, Sivori S, Bottino C, Malaspina A, Morelli L, Moretta L, et al. Molecular cloning of NKp46: a novel member of the immunoglobulin superfamily involved in triggering of natural cytotoxicity. J Exp Med (1998) 188(5):953–60. doi:10.1084/jem.188.5.953
57. Sivori S, Pende D, Bottino C, Marcenaro E, Pessino A, Biassoni R, et al. NKp46 is the major triggering receptor involved in the natural cytotoxicity of fresh or cultured human NK cells. Correlation between surface density of NKp46 and natural cytotoxicity against autologous, allogeneic or xenogeneic target cells. Eur J Immunol (1999) 29(5):1656–66. doi:10.1002/(SICI)1521-4141(199905)29:05<1656::AID-IMMU1656>3.0.CO;2-1
58. Sivori S, Vitale M, Morelli L, Sanseverino L, Augugliaro R, Bottino C, et al. p46, a novel natural killer cell-specific surface molecule that mediates cell activation. J Exp Med (1997) 186(7):1129–36. doi:10.1084/jem.186.7.1129
59. Pende D, Parolini S, Pessino A, Sivori S, Augugliaro R, Morelli L, et al. Identification and molecular characterization of NKp30, a novel triggering receptor involved in natural cytotoxicity mediated by human natural killer cells. J Exp Med (1999) 190(10):1505–16. doi:10.1084/jem.190.10.1505
60. Lakshmikanth T, Burke S, Ali TH, Kimpfler S, Ursini F, Ruggeri L, et al. NCRs and DNAM-1 mediate NK cell recognition and lysis of human and mouse melanoma cell lines in vitro and in vivo. J Clin Invest (2009) 119(5):1251–63. doi:10.1172/JCI36022
61. Shibuya A, Campbell D, Hannum C, Yssel H, Franz-Bacon K, McClanahan T, et al. DNAM-1, a novel adhesion molecule involved in the cytolytic function of T lymphocytes. Immunity (1996) 4(6):573–81. doi:10.1016/S1074-7613(00)70060-4
62. Zhang Z, Wu N, Lu Y, Davidson D, Colonna M, Veillette A. DNAM-1 controls NK cell activation via an ITT-like motif. J Exp Med (2015) 212(12):2165–82. doi:10.1084/jem.20150792
63. Sanchez-Correa B, Campos C, Pera A, Bergua JM, Arcos MJ, Banas H, et al. Natural killer cell immunosenescence in acute myeloid leukaemia patients: new targets for immunotherapeutic strategies? Cancer Immunol Immunother (2016) 65(4):453–63. doi:10.1007/s00262-015-1720-6
64. Kulkarni S, Martin MP, Carrington M. The Yin and Yang of HLA and KIR in human disease. Semin Immunol (2008) 20(6):343–52. doi:10.1016/j.smim.2008.06.003
65. Cavalcanti M, Jewett A, Bonavida B. Irreversible cancer cell-induced functional anergy and apoptosis in resting and activated NK cells. Int J Oncol (1999) 14(2):361–6.
66. Zhou Q, Gil-Krzewska A, Peruzzi G, Borrego F. Matrix metalloproteinases inhibition promotes the polyfunctionality of human natural killer cells in therapeutic antibody-based anti-tumour immunotherapy. Clin Exp Immunol (2013) 173(1):131–9. doi:10.1111/cei.12095
67. Jost S, Reardon J, Peterson E, Poole D, Bosch R, Alter G, et al. Expansion of 2B4+ natural killer (NK) cells and decrease in NKp46+ NK cells in response to influenza. Immunology (2011) 132(4):516–26. doi:10.1111/j.1365-2567.2010.03394.x
68. Arriga R, Caratelli S, Coppola A, Spagnoli GC, Venditti A, Amadori S, et al. Enhancement of anti-leukemia activity of NK cells in vitro and in vivo by inhibition of leukemia cell-induced NK cell damage. Oncotarget (2016) 7(2):2070–9. doi:10.18632/oncotarget.6529
69. Costello RT, Sivori S, Marcenaro E, Lafage-Pochitaloff M, Mozziconacci MJ, Reviron D, et al. Defective expression and function of natural killer cell-triggering receptors in patients with acute myeloid leukemia. Blood (2002) 99(10):3661–7. doi:10.1182/blood.V99.10.3661
70. Garcia-Iglesias T, Del Toro-Arreola A, Albarran-Somoza B, Del Toro-Arreola S, Sanchez-Hernandez PE, Ramirez-Duenas MG, et al. Low NKp30, NKp46 and NKG2D expression and reduced cytotoxic activity on NK cells in cervical cancer and precursor lesions. BMC Cancer (2009) 9:186. doi:10.1186/1471-2407-9-186
71. Parsons MS, Tang CC, Jegaskanda S, Center RJ, Brooks AG, Stratov I, et al. Anti-HIV antibody-dependent activation of NK cells impairs NKp46 expression. J Immunol (2014) 192(1):308–15. doi:10.4049/jimmunol.1301247
72. Nattermann J, Feldmann G, Ahlenstiel G, Langhans B, Sauerbruch T, Spengler U. Surface expression and cytolytic function of natural killer cell receptors is altered in chronic hepatitis C. Gut (2006) 55(6):869–77. doi:10.1136/gut.2005.076463
73. Della Chiesa M, Pesce S, Muccio L, Carlomagno S, Sivori S, Moretta A, et al. Features of memory-like and PD-1(+) human NK cell subsets. Front Immunol (2016) 7:351. doi:10.3389/fimmu.2016.00351
74. Beldi-Ferchiou A, Lambert M, Dogniaux S, Vely F, Vivier E, Olive D, et al. PD-1 mediates functional exhaustion of activated NK cells in patients with Kaposi sarcoma. Oncotarget (2016) 7(45):72961–77. doi:10.18632/oncotarget.12150
75. Wiesmayr S, Webber SA, Macedo C, Popescu I, Smith L, Luce J, et al. Decreased NKp46 and NKG2D and elevated PD-1 are associated with altered NK-cell function in pediatric transplant patients with PTLD. Eur J Immunol (2012) 42(2):541–50. doi:10.1002/eji.201141832
76. Durr S, Kindler V. Implication of indolamine 2,3 dioxygenase in the tolerance toward fetuses, tumors, and allografts. J Leukoc Biol (2013) 93(5):681–7. doi:10.1189/jlb.0712347
77. Brandacher G, Margreiter R, Fuchs D. Clinical relevance of indoleamine 2,3-dioxygenase for alloimmunity and transplantation. Curr Opin Organ Transplant (2008) 13(1):10–5. doi:10.1097/MOT.0b013e3282f3df26
78. Della Chiesa M, Carlomagno S, Frumento G, Balsamo M, Cantoni C, Conte R, et al. The tryptophan catabolite L-kynurenine inhibits the surface expression of NKp46- and NKG2D-activating receptors and regulates NK-cell function. Blood (2006) 108(13):4118–25. doi:10.1182/blood-2006-03-006700
79. Kroemer A, Xiao X, Degauque N, Edtinger K, Wei H, Demirci G, et al. The innate NK cells, allograft rejection, and a key role for IL-15. J Immunol (2008) 180(12):7818–26. doi:10.4049/jimmunol.180.12.7818
80. Deniz G, Erten G, Kücüksezer UC, Kocacik D, Karagiannidis C, Aktas E, et al. Regulatory NK cells suppress antigen-specific T cell responses. J Immunol (2008) 180(2):850–7. doi:10.4049/jimmunol.180.2.850
81. Maroof A, Beattie L, Zubairi S, Svensson M, Stager S, Kaye PM. Posttranscriptional regulation of II10 gene expression allows natural killer cells to express immunoregulatory function. Immunity (2008) 29(2):295–305. doi:10.1016/j.immuni.2008.06.012
82. Garrod KR, Liu FC, Forrest LE, Parker I, Kang SM, Cahalan MD. NK cell patrolling and elimination of donor-derived dendritic cells favor indirect alloreactivity. J Immunol (2010) 184(5):2329–36. doi:10.4049/jimmunol.0902748
83. Serti E, Werner JM, Chattergoon M, Cox AL, Lohmann V, Rehermann B. Monocytes activate natural killer cells via inflammasome-induced interleukin 18 in response to hepatitis C virus replication. Gastroenterology (2014) 147(1):209–20.e3. doi:10.1053/j.gastro.2014.03.046
84. Lenac Rovis T, Kucan Brlic P, Kaynan N, Juranic Lisnic V, Brizic I, Jordan S, et al. Inflammatory monocytes and NK cells play a crucial role in DNAM-1-dependent control of cytomegalovirus infection. J Exp Med (2016) 213(9):1835–50. doi:10.1084/jem.20151899
85. Blanca IR, Bere EW, Young HA, Ortaldo JR. Human B cell activation by autologous NK cells is regulated by CD40-CD40 ligand interaction: role of memory B cells and CD5+ B cells. J Immunol (2001) 167(11):6132–9. doi:10.4049/jimmunol.167.11.6132
86. Ghiringhelli F, Ménard C, Terme M, Flament C, Taieb J, Chaput N, et al. CD4+CD25+ regulatory T cells inhibit natural killer cell functions in a transforming growth factor-beta-dependent manner. J Exp Med (2005) 202(8):1075–85. doi:10.1084/jem.20051511
87. Zimmer J, Andres E, Hentges F. NK cells and Treg cells: a fascinating dance cheek to cheek. Eur J Immunol (2008) 38(11):2942–5. doi:10.1002/eji.200838813
88. Vivier E, Tomasello E, Baratin M, Walzer T, Ugolini S. Functions of natural killer cells. Nat Immunol (2008) 9(5):503–10. doi:10.1038/ni1582
89. Liu R, Van Kaer L, La Cava A, Price M, Campagnolo DI, Collins M, et al. Autoreactive T cells mediate NK cell degeneration in autoimmune disease. J Immunol (2006) 176(9):5247–54. doi:10.4049/jimmunol.176.9.5247
90. Vacca P, Cantoni C, Vitale M, Prato C, Canegallo F, Fenoglio D, et al. Crosstalk between decidual NK and CD14+ myelomonocytic cells results in induction of Tregs and immunosuppression. Proc Natl Acad Sci U S A (2010) 107(26):11918–23. doi:10.1073/pnas.1001749107
91. Pedroza-Pacheco I, Madrigal A, Saudemont A. Interaction between natural killer cells and regulatory T cells: perspectives for immunotherapy. Cell Mol Immunol (2013) 10(3):222–9. doi:10.1038/cmi.2013.2
92. Regateiro FS, Howie D, Cobbold SP, Waldmann H. TGF-beta in transplantation tolerance. Curr Opin Immunol (2011) 23(5):660–9. doi:10.1016/j.coi.2011.07.003
93. Braza F, Dugast E, Panov I, Paul C, Vogt K, Pallier A, et al. Central role of CD45RA- Foxp3hi memory regulatory T cells in clinical kidney transplantation tolerance. J Am Soc Nephrol (2015) 26(8):1795–805. doi:10.1681/ASN.2014050480
94. Chesneau M, Michel L, Dugast E, Chenouard A, Baron D, Pallier A, et al. Tolerant kidney transplant patients produce B cells with regulatory properties. J Am Soc Nephrol (2015) 26(10):2588–98. doi:10.1681/ASN.2014040404
Keywords: natural killer, cytotoxicity, tolerance, kidney, transplantation
Citation: Dugast E, David G, Oger R, Danger R, Judor J-P, Gagne K, Chesneau M, Degauque N, Soulillou J-P, Paul P, Picard C, Guerif P, Conchon S, Giral M, Gervois N, Retière C and Brouard S (2017) Broad Impairment of Natural Killer Cells From Operationally Tolerant Kidney Transplanted Patients. Front. Immunol. 8:1721. doi: 10.3389/fimmu.2017.01721
Received: 18 September 2017; Accepted: 21 November 2017;
Published: 11 December 2017
Edited by:
Aurore Saudemont, GlaxoSmithKline, United KingdomReviewed by:
Giuseppe Sconocchia, Istituto di Farmacologia Traslazionale (CNR), ItalyMyra Coppage, University of Rochester Medical Center, United States
Copyright: © 2017 Dugast, David, Oger, Danger, Judor, Gagne, Chesneau, Degauque, Soulillou, Paul, Picard, Guerif, Conchon, Giral, Gervois, Retière and Brouard. This is an open-access article distributed under the terms of the Creative Commons Attribution License (CC BY). The use, distribution or reproduction in other forums is permitted, provided the original author(s) and the copyright owner are credited and that the original publication in this journal is cited, in accordance with accepted academic practice. No use, distribution or reproduction is permitted which does not comply with these terms.
*Correspondence: Sophie Brouard, c29waGllLmJyb3VhcmRAdW5pdi1uYW50ZXMuZnI=
†These authors have contributed equally to this work.