- 1Walter-Brendel-Centre for Experimental Medicine, University Hospital, LMU Munich, Planegg Martinsried, Germany
- 2Biomedical Center, LMU Munich, Planegg Martinsried, Germany
- 3Immunobiology Laboratory, The Francis Crick Institute, London, United Kingdom
- 4Technische Universität München, Klinikum Rechts der Isar, Institut für Klinische Chemie und Pathobiochemie, Munich, Germany
- 5Core Facility Bioinformatics, Biomedical Center (BMC), LMU Munich, Planegg Martinsried, Germany
Conventional dendritic cells (cDCs) are versatile activators of immune responses that develop as part of the myeloid lineage downstream of hematopoietic stem cells. We have recently shown that in mice precursors of cDCs, but not of other leukocytes, are marked by expression of DNGR-1/CLEC9A. To genetically deplete DNGR-1-expressing cDC precursors and their progeny, we crossed Clec9a-Cre mice to Rosa-lox-STOP-lox-diphtheria toxin (DTA) mice. These mice develop signs of age-dependent myeloproliferative disease, as has been observed in other DC-deficient mouse models. However, despite efficient depletion of cDC progenitors in these mice, cells with phenotypic characteristics of cDCs populate the spleen. These cells are functionally and transcriptionally similar to cDCs in wild type control mice but show somatic rearrangements of Ig-heavy chain genes, characteristic of lymphoid origin cells. Our studies reveal a previously unappreciated developmental heterogeneity of cDCs and suggest that the lymphoid lineage can generate cells with features of cDCs when myeloid cDC progenitors are impaired.
Introduction
Conventional dendritic cells (cDCs) are remarkable activators of adaptive immune responses with a superior capacity to capture, process, and present antigens to T cells (1–4). Through the production of cytokines, cDCs further direct effector responses and play essential roles in immune homeostasis and innate immunity (5–10). This versatility of cDCs in immune responses is regulated in part through the functional specialization of cDC subpopulations. cDCs exist as two main developmentally distinct subsets that are found across lymphoid and non-lymphoid organs. cDC1 requires the transcription factors Batf3, IRF8, and ID2 for their development and are marked by expression of XCR1 and DNGR-1 (a.k.a. CLEC9A) across tissues and species (1, 11, 12). They are exceptional activators of CD8 T cells owing to their superior capacity to take up dead cells and cross-present antigens (1–4). Through the production of IL-12, cDC1 promotes type-I immune responses hallmarked by expression of Th1 cytokines (1, 5). In contrast, the differentiation and function of the cDC2 subset is controlled by the transcription factors IRF4, KLF4, RelB, and ZEB2 (1, 11–14), although ZEB2 may predominantly control cDC2 differentiation in inflammatory conditions (15). Phenotypically cDC2 can be distinguished from cDC1 by expression of CD11b, CD172a, and CLEC4A4 (DCIR2), as well as expression of IRF4 and concomitant lack of IRF8 (1, 11, 12, 16). cDC2 appears to play a more prominent role in CD4 T-cell activation and the promotion of Th2 and Th17 responses, particularly at mucosal surfaces (17, 18).
Although the existence of cDCs as independent cell type has been a matter of intense debate, lineage tracing and other studies in mice have established that cDCs develop as distinct hematopoietic cell lineage (12, 19, 20). Single-cell analyses have recently suggested that myeloid lineage decisions take place early in hematopoiesis but the production of lymphoid and myeloid lineages is thought to branch after the lymphoid-primed multipotent progenitor (LMPP) stage (20–24). Downstream of LMPP, in a stepwise differentiation process, common myeloid progenitors give rise to a lineage (lin) negative CD115+CD117hiCD135+ fraction of bone marrow that generates monocytes, plasmacytoid DCs (pDCs), and cDCs and has therefore been termed macrophage and DC progenitor (MDP) (25, 26). Whether MDPs are a true bi-potent developmental intermediate for cDCs/pDCs and monocytes is controversial (27). MDPs further give rise to common monocyte progenitors that exclusively generate monocytes (28) and a lin−CD115+CD117lowCD135+ fraction of cells termed common DC progenitors (CDP) that lose monocyte potential but generate cDCs and pDCs (29, 30). Within CDPs the expression of the C-type lectin receptor DNGR-1 (encoded by the Clec9a gene) distinguishes cells with exclusive cDC potential (19). CDPs further differentiate into pre-cDCs, which also express DNGR-1 (19) and travel via blood to peripheral organs, where their terminal differentiation into cDC1 and cDC2 takes place in response to environmental cues (31–33). Some pre-cDCs exist as pre-committed subsets that can be already distinguished in bone marrow based on the expression of specific surface markers and transcription factors (32, 34, 35). The developmental steps of cDC differentiation appear conserved, as equivalent progenitors and transcriptional requirements for cDC differentiation have been identified in humans (12, 36–40).
By crossing mice expressing Cre recombinase (Cre) under the control of the endogenous Clec9a promoter to Rosa26-lox-STOP-lox-yellow fluorescent protein (YFP) reporter mice, we were able to demonstrate that Clec9a expression history faithfully traces the cDC lineage, including the main cDC1 and cDC2 subsets, but no other myeloid and lymphoid lineages in steady state as well as during inflammation (19). In this model, any cell-expressing Cre becomes irreversibly labeled with YFP, thereby allowing us to trace DNGR-1-expressing CDP and pre-cDC irrespective of continuous DNGR-1 expression (19). One exception to faithful tracing of the cDC lineage is pDCs, which do not arise from DNGR-1-expressing cDC progenitors but express low levels of DNGR-1 in their differentiated form (19). In pDCs Clec9a expression history, therefore, is not necessarily a measure of cell origin (19). The same applies to cDC1, which express high levels of DNGR-1 and could become labeled with YFP because they express Cre in their differentiated form (19). However, cDC1 are CDP-derived (1, 11) and arise from DNGR-1-expressing cDC progenitors upon adoptive transfer, confirming their classification as cDCs (19). Despite these limitations, Clec9a-Cre mice offer a powerful means to identify cDCs as descendants from Clec9a-expressing cDC progenitors and to trace the cDC lineage, particularly the cDC2 subtype, which lacks DNGR-1 in its differentiated form.
Although we have reached a consensus that cDCs arise as part of the myeloid lineage, several studies have suggested a lymphoid path to cDC development. Purified lymphoid progenitors can differentiate into cells resembling cDC1 and cDC2 after adoptive transfer and in vitro (41–47). This process appears to be driven by the same lineage promoting transcription factors that control cDC development from myeloid progenitors, such as IRF8 (46, 48). Additionally, B-cell receptor gene rearrangements at the IgH locus, indicative of a lymphoid past, can be found in populations of thymic cDCs, some splenic cDC1, and pDCs (49–52). Nevertheless, fate-mapping studies in steady-state mice have excluded a prominent contribution of lymphoid progenitors to the steady-state cDC pool (53, 54) and have confirmed a binary branching of lymphoid and myeloid lineages downstream of hematopoietic stem cells (21). However, whether lymphoid progenitors can serve as an alternative path to DC poiesis in conditions of inflammation or when myeloid cDC progenitors are absent has not been investigated. Because cDCs generated in vitro from purified human lymphoid or myeloid progenitors are indistinguishable by gene expression analysis (42), addressing this question requires faithful ontogeny-based fate-mapping models.
Here, we investigated cDC development in mice in conditions in which cDC progenitors are impaired. We crossed Clec9a-Cre mice to Rosa26-lox-STOP-lox-diphtheria toxin (DTA) reporter mice (55) (Clec9aCreRosaDTA) to constitutively deplete Clec9a-expressing cDC progenitors and their progeny. We found that Clec9aCreRosaDTA mice lack cDC progenitors and cDC1 but not cDC2. We show that in the absence of cDC progenitors, cells with features of cDC2 arise via an alternative developmental path. These cells show similarities to bona fide cDC2 in terms of transcriptional profile and inflammatory cytokine production but exhibit evidence of Ig receptor rearrangements, indicating a lymphoid origin. Thus, our data suggest a previously unrecognized role for lymphoid progenitors as an alternative source of cDC2, when the conventional myeloid path of cDC development is blocked.
Materials and Methods
Mice
Clec9a-Cre (19), Rosa26-lox-STOP-lox-EYFP (56), Rosa26-lox-STOP-lox-DTA (55), Rosa26-lox-STOP-lox-DTR (57), C57BL/6J, and B6.SJL mice were bred at Cancer Research UK, at ENVIGO or the Biomedical Center in specific pathogen-free conditions. All animal experiments were performed in accordance with national and institutional guidelines for animal care and approved by the Francis Crick Institute Animal Ethics Committee, the UK Home Office, or the Regierung of Oberbayern.
Cell Isolation
Spleen and lymph nodes were cut into small pieces and digested with Collagenase IV (200 U/mL; Worthington) and DNase I (0.2 mg/mL Roche) in RPMI for 30 min at 37°C. Cells were strained through 70-µm cell strainers (BD Biosciences), washed with FACS buffer [PBS, 1% fetal calf serum (FCS), 2.5-mM EDTA, 0.02% sodium azide] and incubated for 2 min in red blood cell lysis buffer (Sigma). Cells were then washed and resuspended in FACS buffer. Bone marrow was isolated by flushing one femur with FACS buffer onto a cell strainer. Erythrocytes were lysed as above. Colonic single-cell suspensions were prepared as published (19).
Cell Culture
CD11c+ cells from spleen were enriched by positive selection using magnetic beads and LS-columns (Miltenyi), cultured in complete RPMI (10% FCS, penicillin/streptomycin, non-essential amino acids, sodium pyruvate, l-glutamine, 0.025-mM β-mercaptoethanol), and stimulated with LPS (100 ng/mL) or CpG (0.5 µg/mL) for 2 h before addition of brefeldin A (5 µg/mL) for an additional 4 h.
ELISA
FLT3L and G-CSF were measured with DuoSet mFLT3L (DY427) and Quantikine® ELISA kits (both R&D Systems) according to the manufacturer’s recommendations. Other cytokines were measured by Legendplex (BioLegend).
Flow Cytometry
Data were collected on a LSR Fortessa (BD Biosciences) and analyzed with FlowJo software (Tree Star, Inc.). Cell sorting was performed on an Aria III Fusion (BD Biosciences). Antibodies used in this study can be found in Table S2 in Supplementary Material. For intracellular cytokine staining cells were fixed with 2% paraformaldehyde (15 min, room temperature), then washed in FACS buffer with 0.05% saponin and stained in 0.5% saponin. IRF8 and IRF4 were stained in Foxp3 transcription factor staining set (eBioscience-00-5523-00) and Zbtb46 in transcription factor buffer from BDBioscience-562574. Dead cells were identified with Dapi or live/dead fixable violet (Invitrogen) or zombie UV dye (Biolegend).
Microarray Analysis
CD11c+ cells were enriched using magnetic beads and LS columns (Miltenyi Biotec). Cells were then sorted as CD11c+MHCII+CD11b+CD24−CD64−Gr-1− cells from Clec9acre/creRosaDTA mice or as YFP+CD11c+MHCII+CD11b+CD24−CD64−Gr-1− cells from Clec9acre/creRosaYFP mice. Total RNA was isolated using Qiagen RNeasy Micro Kit and prepared for hybridization on Affymetrix Mouse Gene 1.0 ST Arrays. We processed microarray data with R/bioconductor (R version 3.4.2) using the annotation package “mogene10sttranscriptcluster.db” version 8.7.0. The Robust Multichip Average (RMA) algorithm was used to extract raw expression values (library “oligo,” version 1.42.0 and “pd.mogene.1.0.st.v1” version 3.14.1). We subsequently removed probe sets with zero variance and kept the ones with a median log2 expression level higher than 4.5 in at least one condition. Many-to-one probe-sets-to-gene relationships were resolved by retaining per gene only the probe set with the highest interquartile range of expression levels across the samples. External data were merged and batch corrected using ComBat (library “sva,” version 3.26.0). Principle component analysis (PCA) was performed on 50% of the genes defined by highest variance across all samples. Heatmaps were generated using function pheatmap (library pheatmap, version 1.0.8) including gene-based scaling and clustering.
DJ-Rearrangement Polymerase Chain Reaction (PCR)
Genomic DNA was extracted by phenol chloroform extraction and 20-ng DNA were used per reaction. PCR for the IgH locus (Dfl16 and Dsp2 D gene families) (58) was split in two reactions for Germline (J3 & Mu0) and DJ-rearrangement (DH L & J3).
DHL-GGAATTCG(AorC)TTTTTGT(CorG)AAGGGATCTACTACTGTG;
Mu0-CCGCATGCCAAGGCTAGCCTGAAAGATTACC; J3-GTCTAGATTCTCACAAGAGTCCGATAGACCCTGG. IgH DJ-rearrangements for the DHQ52 element were assessed by sequential PCRs with the following primers:
PCR-1: DHQ52-1-CACAGAGAATTCTCCATAGTTGATAGCTCAG;DHQ52-2GCCTCAGAATTCCTGTGGTCTCTGACTGGT; PCR-2: JH4-1-AGGCTCTGAGATCCCTAGACAG; JH4-2- GGGTCTAGACTCTCAGCCGGCTCCCTCAGGG as previously described (51).
Diphtheria Toxin (DT)-Mediated Cell Ablation
Mice were injected intraperitoneally with 25 ng per gram body weight DT (SIGMA). Spleens were analyzed 24 h later.
Statistical Analysis
Statistical testing was performed using two-sided, unpaired Welch t-tests in GraphPad Prism 6 software (GraphPad, La Jolla, CA, USA). A p < 0.05 was considered significant.
Results
Clec9aCre/CreRosaDTA Mice Develop Myeloproliferative Disease
To generate mice lacking progeny derived from cDC-restricted progenitors, we crossed Clec9a-Cre mice to Rosa26-lox-STOP-lox-DTA reporter mice to induce apoptotic cell death in Cre-expressing cells and their progeny (55). We have previously shown that the penetrance of Cre-mediated recombination is increased in homozygous Clec9a-Cre mice without affecting specificity (19). For most experiments, we therefore generated mice homozygous for the Clec9a-Cre locus that were either heterozygous or homozygous for the Rosa26-lox-STOP-lox-DTA allele (henceforth referred to as Clec9aCre/CreRosaDTA mice). Clec9aCre/CreRosaDTA mice were born at Mendelian ratio and developed normally (not shown). With age, spleens from Clec9aCre/CreRosaDTA mice appeared larger (not shown) and exhibited significantly increased cellularity compared to spleens from littermate controls (Clec9a+/+RosaDTA or Clec9aCreRosa+/+ mice, henceforth referred to as controls, Figure 1A). Despite prominent splenomegaly in mice 10 weeks or older, no difference in bone-marrow cellularity was observed (Figure 1A). DC deficiency is associated with myeloproliferation leading to systemic neutrophilia and monocytosis (59–62). In 5-week-old mice, we observed no differences in the frequency and number of Ly-6C+CD11b+ myeloid cells but in mice 10 weeks or older Ly-6C+CD11b+ cells were significantly increased (Figures 1B,C). This age-dependent increase in Ly-6C+CD11b+ cells encompassed both neutrophils and monocytes, whereas red pulp macrophages were not affected (Figures S1A–C in Supplementary Material). Myeloproliferation in DC-deficient mice is thought to be secondary to dysregulation of growth factors, such as FLT3L, G-CSF, or GM-CSF (59, 61). Clec9aCre/CreRosaDTA mice displayed increased serum levels of FLT3L, with a slight increase evident even before the onset of overt splenomegaly in 6-week-old mice (Figure 1D). Systemic levels of other cytokines implicated in neutrophil and monocyte homeostasis were unaffected (Figure S1D in Supplementary Material). Of note, we could not detect GM-CSF in serum of control or Clec9aCre/CreRosaDTA mice (unpublished observation). Therefore, Clec9aCre/CreRosaDTA mice develop splenomegaly and exhibit increased levels of FLT3L, reminiscent of the myeloproliferative disease reported in other cDC-deficient animal models (59, 61–63).
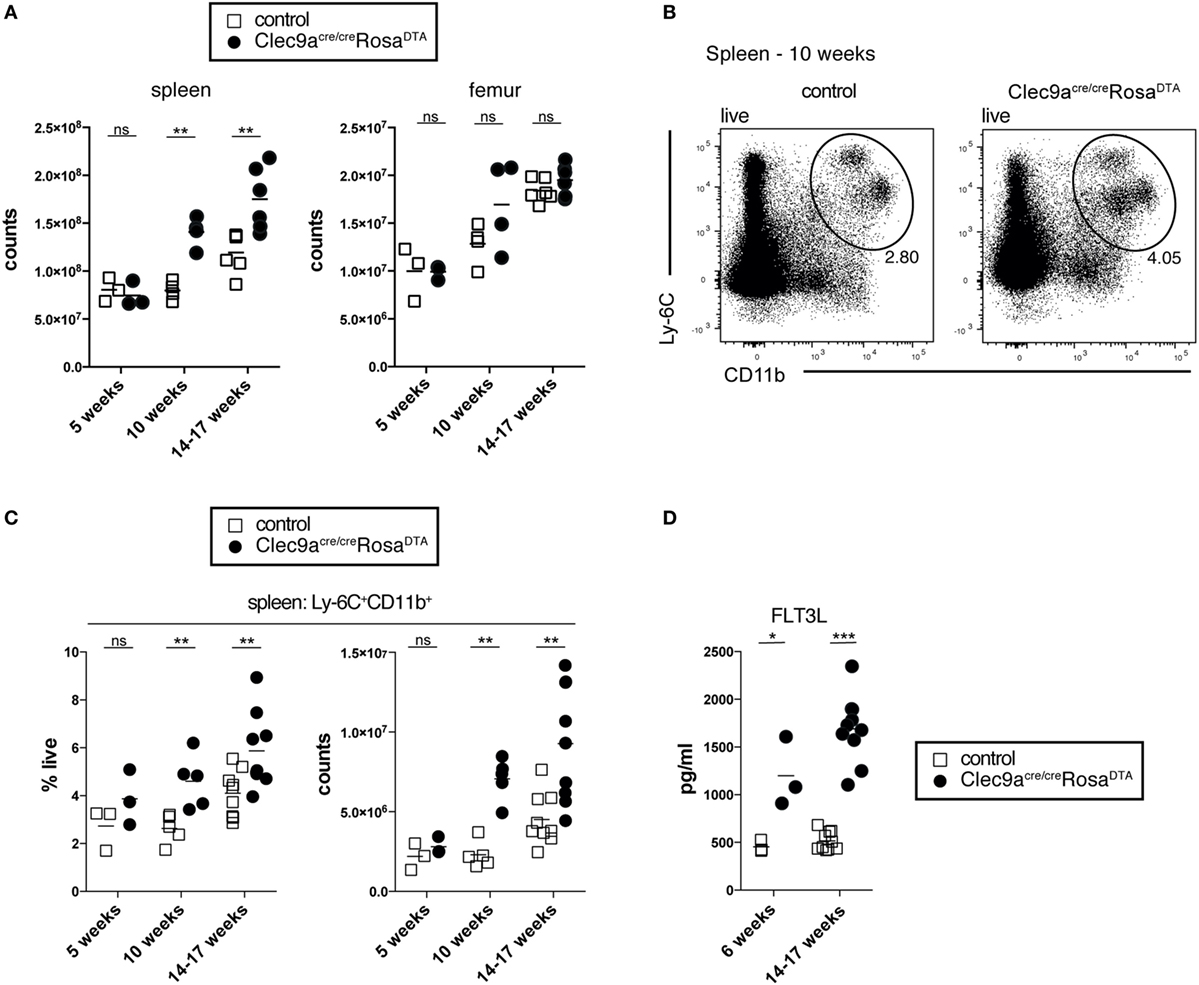
Figure 1. Clec9acre/creRosaDTA mice showing signs of age-dependent myeloproliferation. (A) The total number of leukocytes per spleen and femur from control and Clec9acre/creRosaDTA mice of the indicated ages is shown. (B,C) Ly-6C+CD11b+ cells were identified by flow cytometry in spleen from control and Clec9acre/creRosaDTA mice. (B) Representative gating strategy of Ly-6C+CD11b+ cells in 10-week-old mice. Plots are gated on DAPI− live cells. (C) Frequency and total counts of Ly-6C+CD11b+ cells in mice of the indicated ages and genotypes. (D) Serum was collected from control and Clec9acre/creRosaDTA mice at 6 and 14–17 weeks of age and the concentrations of FLT3L were determined by ELISA. Each symbol represents one mouse. ns, not significant; * p < 0.05, ** p < 0.001, and *** p < 0.0001.
Loss of cDC1 but Not cDC2 in Clec9aCre/CreRosaDTA Mice
To analyze the efficiency of cDC depletion in Clec9aCre/CreRosaDTA mice, we focused on steady-state spleen, where cDCs can reliably be identified as CD11c+MHCII+ cells and the two main cDC1 and cDC2 subsets can be distinguished as CD24+ and CD11b+ cells, respectively (Figure 2A) (12). At 10 weeks of age Clec9aCre/CreRosaDTA mice showed a twofold reduction in the frequency of splenic cDCs compared to controls. Due to the increased organ cellularity, however, there was no effect on the absolute number of splenic CD11c+MHCII+ cells (Figures 2A,B). The same was observed in older Clec9aCre/CreRosaDTA mice (14–17 weeks; Figure 2B). In 5-week-old mice that do not exhibit increased spleen cellularity, a reduction of splenic CD11c+MHCII+ cells was apparent in cell counts (Figure 2B), yet cell depletion was surprisingly inefficient (about 3.5-fold). When we further separated CD11c+MHCII+ cells into cDC1 and cDC2 using the surface markers CD24 and CD11b (Figure 2A), we found that cDC1 were completely depleted in spleens from Clec9aCre/CreRosaDTA mice at all ages examined (Figure 2C and unpublished observations). In contrast, CD11b+ cDC2 showed a significant but small reduction (Figure 2C) only in 5-week-old Clec9aCre/CreRosaDTA mice and their frequency and absolute number was not reduced in mice 10 weeks or older (Figure 2C). This could be seen in other lymphoid organs, as well as in mice heterozygous for Cre expression, as the lymph nodes of Clec9a+/CreRosaDTA mice also lacked cDC1 but not resident cDC2 or migratory CD103+CD11b+ and CD103- CD11b+ cDC2 (Figure S2F in Supplementary Material). Finally, these findings could be extended to non-lymphoid organs as cDC1 but not CD103+CD11b+ or CD103− CD11b+ cDC2 were absent from colon of Clec9aCre/CreRosaDTA mice (Figure S2G in Supplementary Material).
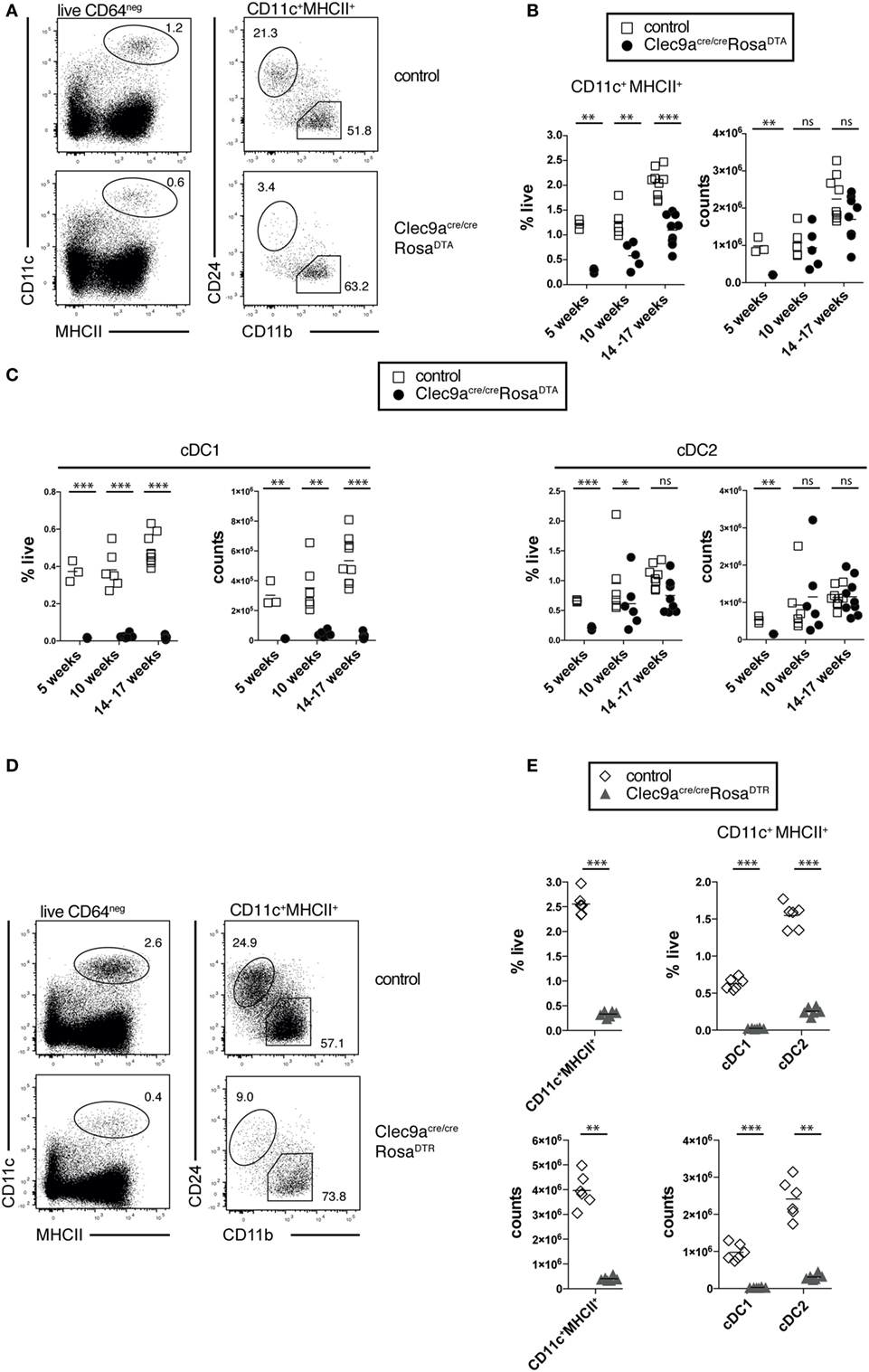
Figure 2. Loss of cDC1 but not cDC2 in spleen from Clec9acre/creRosaDTA mice. (A–C) CD11c+MHCII+ conventional dendritic cells (cDCs) were identified by flow cytometry in spleen from Clec9acre/creRosaDTA and control mice and further analyzed for CD24 and CD11b expression, to identify cDC1 and cDC2, respectively. (A) Representative gating strategy to identify CD11c+MHCII+ cDCs, CD24+ cDC1, and CD11b+ cDC2. (B) Frequency and total counts of CD11c+MHCII+ cells in spleen from control and Clec9acre/creRosaDTA mice of the indicated ages. (C) Frequency and total counts of CD24+ cDC1 and CD11b+ cDC2 in mice of the indicated ages. (D,E) Clec9acre/creRosaDTR and control mice were injected i.p. with DT and 24 h later spleens were analyzed by flow cytometry to identify CD11c+MHCII+ cells, as well CD24+ cDC1 and CD11b+ cDC2 (D). (E) The frequency and counts of total cDCs and cDC subsets identified as in (D). Each symbol represents one mouse. ns, not significant; * p < 0.05, ** p < 0.001, and *** p < 0.0001.
The lack of cDC2 depletion was surprising, as this population shows near complete fluorescent reporter labeling in Clec9aCre/CreRosaYFP mice (19) and can be depleted with DT in Clec9a-Cre mice crossed to Rosa26-lox-STOP-lox-diphtheria toxin receptor mice (DTR; Clec9aCre/CreRosaDTR) (64). We confirmed that a single injection of DT was sufficient to efficiently deplete pre-cDCs, cDC1, and cDC2 in Clec9aCre/CreRosaDTR mice (Figures 2D,E; Figures S2A,B in Supplementary Material). Of note, 24 h after DT injection no increase in splenic neutrophils or monocytes was observed (Figure S2C in Supplementary Material), suggesting that Clec9aCre/CreRosaDTR mice do not develop acute monocytosis and neutrophilia (62). Therefore, Clec9a-Cre mice serve as an efficient model to transiently deplete cDC1 and cDC2 when crossed to RosaDTR mice (64) but depletion of cDC2 is not seen when crossed to RosaDTA mice.
Fully differentiated pDCs express low levels of DNGR-1 although they do not arise from DNGR-1-expressing CDPs (19). Splenic pDC numbers were not altered in Clec9aCre/CreRosaDTA mice (Figure S2D in Supplementary Material). However, pDCs that developed in Clec9a+/CreRosaDTA mice almost completely lacked DNGR-1 (Figure S2E in Supplementary Material). These data suggest that DNGR-1-expressing pDCs are depleted and replaced with DNGR-1 negative pDCs. We conclude that constitutive depletion of DNGR-1-expressing cells in Clec9aCre/CreRosaDTA adult mice leads to a complete loss of cDC1 but not cDC2 or pDCs.
Efficient Depletion of Pre-cDCs in Bone Marrow and Spleen of Clec9aCre/CreRosaDTA Mice
We next investigated whether cDC progenitors were depleted in Clec9aCre/CreRosaDTA mice. As expected, Clec9aCre/CreRosaDTA mice showed no reduction in MDPs lin− CD11c−CD115+CD135+CD117hi compared to control mice (Figures 3A,B). In contrast, CDPs (lin−CD11c−CD115+CD135+CD117low/−) were significantly reduced in Clec9aCre/CreRosaDTA mice (Figures 3A,B). High serum levels of FLT3L in Clec9aCre/CreRosaDTA mice could have led to downregulation of CD135 in MDPs or CDPs, causing them to be missed in our gating. This was not the case because bone marrow lin−CD11c−CD115+CD117hi and lin−CD11c−CD115+CD117low/− cells from control and Clec9aCre/CreRosaDTA mice expressed similar levels of CD135 (Figure 3C). Pre-cDCs, identified as lin−CD11c+CD135+CD172alow cells, were also nearly absent in bone marrow of Clec9aCre/CreRosaDTA mice when compared to controls (Figures 3A,B) and this was true when pre-cDC were also identified irrespective of the CD135 marker as the lin−CD11c+ fraction of bone marrow (Figure S3A in Supplementary Material). Similarly, in spleen, lin−CD11c+CD43+CD135+CD172alow pre-cDCs were virtually absent in Clec9aCre/CreRosaDTA mice, as well as heterozygous Clec9a+/CreRosaDTA mice (Figures 3D,E; Figure S3C in Supplementary Material). Although CD43+CD135− cells were found in the splenic pre-cDC gate, those cells expressed reduced levels of CD11c, indicating that they were contaminants rather than bona fide pre-cDCs (Figures 3D–F; Figures S3B,C in Supplementary Material). A more efficient depletion of pre-cDCs compared with CDPs is to be expected because Cre-mediated DNA rearrangement in pre-cDCs is higher than in CDPs, reflecting the developmental hierarchy of DNGR-1 expression in rapidly cycling progenitors (19). Thus, our data indicate efficient depletion of cDC progenitors in bone marrow and spleen of Clec9aCre/CreRosaDTA mice and suggest that cells resembling cDC2 in Clec9aCre/CreRosaDTA mice arise independently of the classical CDP and pre-cDC differentiation pathway.
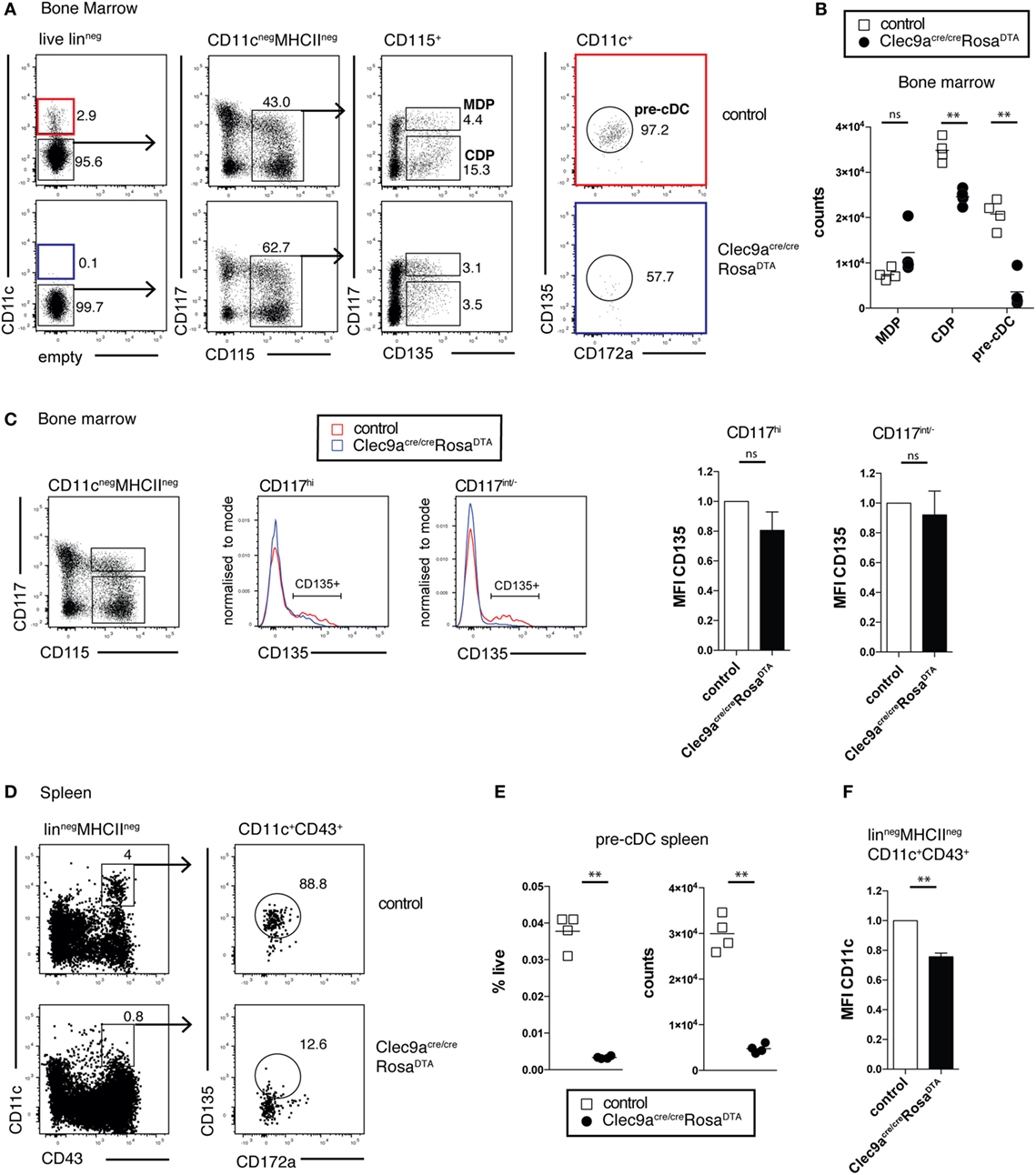
Figure 3. Efficient depletion of conventional dendritic cell (cDC) progenitors in bone marrow and spleen. (A,B) cDC progenitors were identified by flow cytometry in the bone marrow of 10-week-old control and Clec9acre/creRosaDTA mice. (A) Live lineage negative (lin−; CD3, CD4, CD8, CD11b, MHCII, Ter119, NK1.1, and B220) cells were gated and further analyzed for CD11c, CD117, CD115, CD172a, and CD135 expression. Macrophage and DC progenitor (MDPs) were identified as CD11c−CD115+CD135+CD117hi cells, common DC progenitors (CDPs) as CD11c−CD115+CD135+CD117int/− cells, and pre-cDCs as CD11c+CD172aintCD135+ cells. (B) The number of MDP, CDP, and pre-cDC per femur is shown. (C) Lin−CD115+ CD117hi and lin−CD115+CD117int/− bone-marrow cells from control and Clec9acre/creRosaDTA mice were gated and further analyzed for CD135 expression. Mean fluorescence intensity (MFI) (n = 4) of the CD135+ cell fraction, as gated in the histogram overlays, is shown. It was normalized to MFI on the same population from control mice, which is set as 1. (D–F) Pre-cDCs in spleen from control and Clec9acre/creRosaDTA mice 10 weeks of age were identified as lin−CD11c+CD43+CD172aintCD135+ cells by flow cytometry (D). (E) The frequency and number of pre-cDCs per spleen is shown. (F) CD11c MFI on splenic lin−MCHII−CD11c+CD43+ cells from Clec9acre/creRosaDTA mice is shown. It was normalized to MFI on the same population from control mice, which is set as 1 (n = 4). Each symbol represents one mouse. ns, not significant; * p < 0.05, ** p < 0.001, and *** p < 0.0001.
cDC2 From Clec9aCre/CreRosaDTA Mice Phenotypically and Functionally Resemble cDCs
Phenotypic analysis revealed that cDC2 from Clec9aCre/CreRosaDTA mice showed no alterations in surface expression of the cDC markers CD172a, MHCII, CLEC4A4, and ESAM, although the expression of CD4 was slightly lower on cDC2 from Clec9aCre/CreRosaDTA mice compared to controls (Figure 4A). cDC2 from Clec9aCre/CreRosaDTA mice further showed normal expression of lineage defining transcription factors ZBTB46, IRF4 and IRF8 (Figure 4A). cDC2 from control and Clec9aCre/CreRosaDTA mice responded similarly to stimulation with the TLR ligands lipopolysaccharide (LPS) and CpG oligonucleotides (Figure 4B). Interestingly, upon LPS treatment fewer cDC2 from Clec9aCre/CreRosaDTA mice than from control mice produced TNF, whereas this was not the case for IL-12 (Figure 4B). Reduced TNF production after LPS stimulation was already present in cDC2 from young Clec9aCre/CreRosaDTA mice that did not yet display myeloproliferation (Figure 4C) indicating that it was not merely a functional adaptation to the myeloproliferative environment. We conclude that cDC2 from Clec9aCre/CreRosaDTA mice are broadly similar to cDC2 from control mice, other than a slight impairment in the production of TNF after LPS stimulation.
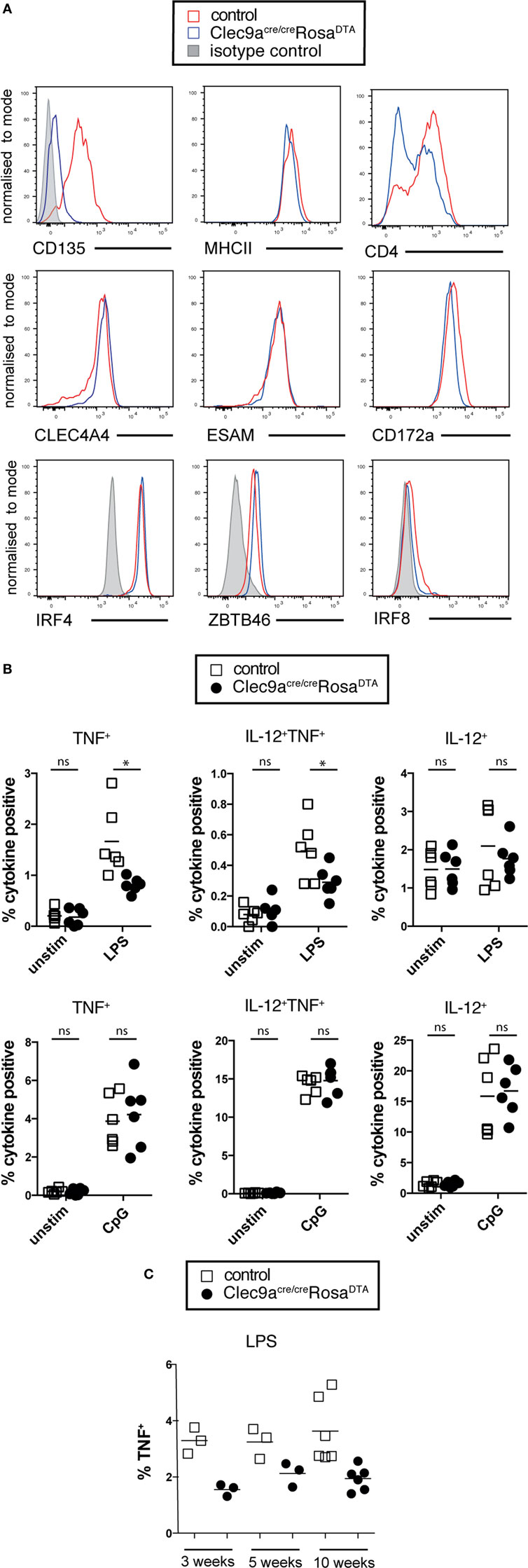
Figure 4. cDC2 from Clec9aCre/CreRosaDTA mice phenotypically and functionally resemble cDCs. (A) Splenic CD11c+MHCII+CD11b+ cells from control (red) and Clec9acre/creRosaDTA (blue) mice were analyzed for the expression of indicated surface markers or transcription factors (lower line) by flow cytometry. Gray traces represent staining with isotype matched control antibody. (B,C) CD11c+ cells from control and Clec9acre/creRosaDTA mice of the indicated ages were enriched using magnetic beads, stimulated with lipopolysaccharide (LPS) (100 ng/mL) or CpG (0.5 µg/mL) for 6 h followed by intracellular cytokine staining. CD11c+MHCII+CD11b+ cDC2 were identified by flow cytometry and TNF and IL-12 production was analyzed. (B) The frequency of cytokine positive cDC2 is shown. (C) Frequency of TNF producing cDC2 from mice of the indicated ages stimulated with LPS. Each data point represents one mouse. Data are compiled from two independent experiments. * p < 0.05.
cDC2 From Clec9aCre/CreRosaDTA Mice Are Transcriptionally Similar to Bona Fide cDC2 but Exhibit Somatic Rearrangements of Lymphoid Receptor Genes
We next performed whole transcriptome profiling by microarray of cDC2 from Clec9aCre/CreRosaDTA mice. To this end, we sorted splenic CD11c+MHCII+CD11b+ cDC2 from Clec9aCre/CreRosaDTA mice (CD11b+ DTA) and compared their mRNA profile to that of cDC2 sorted as YFP+CD11c+MHCII+CD11b+ cells from Clec9aCre/CreRosaYFP mice (YFP+; Figure 5; Figure S3 and Table S1 in Supplementary Material). We chose this experimental strategy to ensure comparison to bona fide cDC2 arising from Clec9a-expressing myeloid cDC progenitors. Our analysis revealed surprisingly few differences in gene expression, indicating that cDC2 from Clec9aCre/CreRosaDTA mice are very similar to YFP+ cDC2. Interestingly, among the 50 most significantly differentially expressed genes, only 11 showed more than a twofold difference and we found CD135 as a top hit (Table S1 in Supplementary Material). The expression of CD135 was about twofold lower in cDC2 from Clec9aCre/CreRosaDTA mice than in YFP+ cDC2 (Table S1 in Supplementary Material), raising the possibility that expression of the lineage defining marker CD135 may be dysregulated at the mRNA level. Consistent with that notion, staining for CD135 was reduced on cDC2 from Clec9aCre/CreRosaDTA mice (Figure 4A). However, incubation of cDCs with FLT3L inhibited CD135 staining intensity, even in conditions in which endocytosis should be blocked (Figure S3D in Supplementary Material), suggesting that, when occupied by FLT3L, CD135 may no longer be freely available for antibody binding. Therefore, decreased CD135 staining on cDC2 from Clec9aCre/CreRosaDTA mice may reflect the fact that the receptor is engaged by FLT3L, as well as a possible reduction in gene expression. We next used cDC and macrophage core gene signatures (65) and performed unsupervised hierarchical clustering of cDC2 from Clec9aCre/CreRosaDTA mice and YFP+ cDC2 in the context of published datasets for cDC and macrophage populations from the Immgen database. When compared in this manner cDC2 from Clec9aCre/CreRosaDTA mice and YFP+ cDC2 showed greater similarity to splenic cDC2 than to cDC1 or red pulp macrophages (Figures 5A,B; Figure S3 in Supplementary Material). cDC2 from Clec9aCre/CreRosaDTA mice did not display a migratory cDC signature (Figure S4 in Supplementary Material), suggesting that they are not cDC2 that have aberrantly migrated from the periphery.
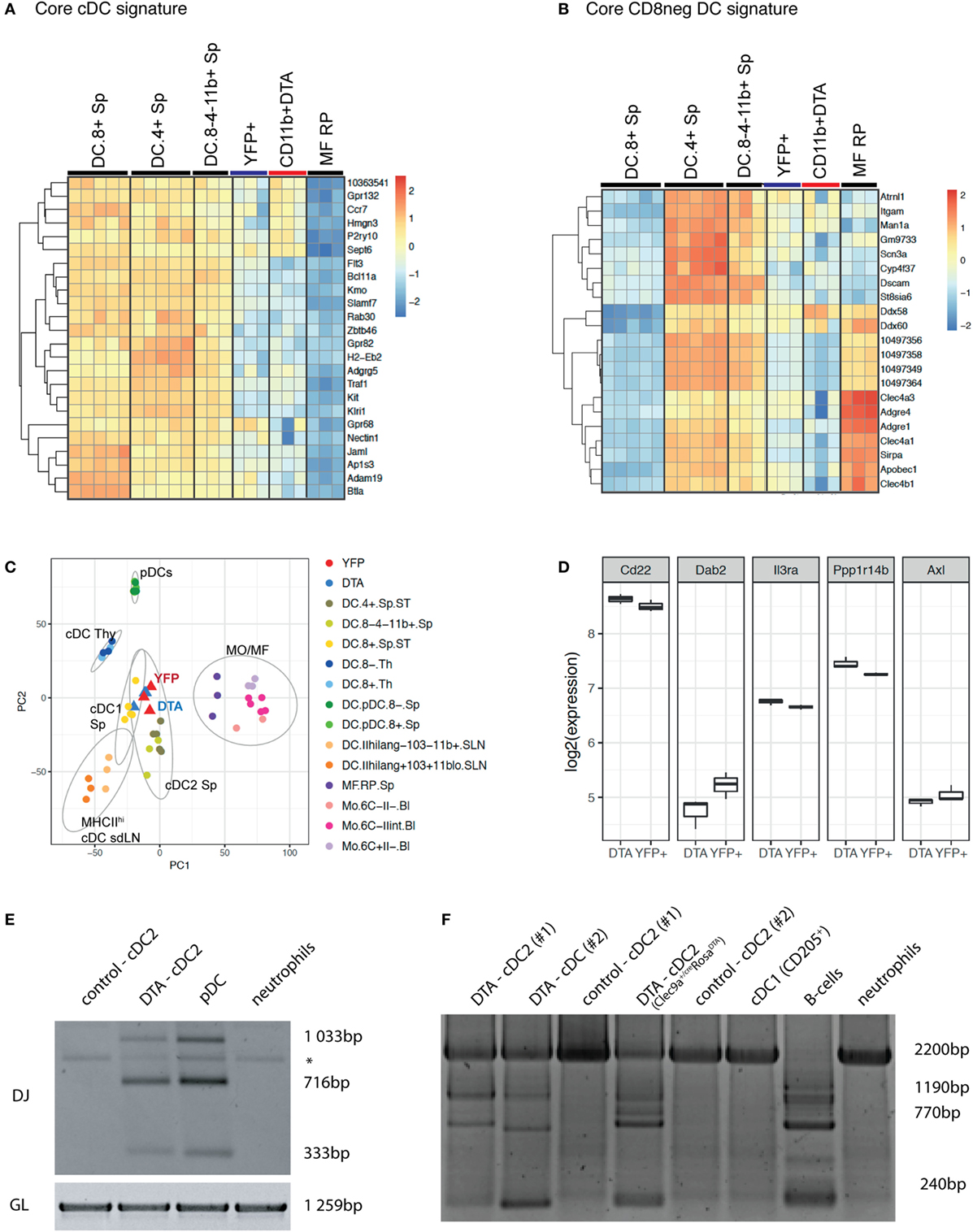
Figure 5. cDC2 from Clec9aCre/CreRosaDTA mice are transcriptionally similar to bona fide cDC2 but exhibit somatic rearrangements of lymphoid receptor genes. Splenic CD11c+MHCII+CD11b+ cDC2 from Clec9acre/creRosaDTA mice (CD11b+ DTA) and CD11c+MHCII+CD11b+YFP+ cDC2 (YFP+) from Clec9acre/creRosaYFP mice were sorted and subjected to microarray analysis. (A,B) Population clustering and heat map display of the relative expression values for core conventional dendritic cells (cDC) signature genes (A) and core CD8neg cDC signature genes (B) comparing cDC2 from Clec9acre/creRosaDTA mice and YFP+ cDC2 to the indicated murine cDC and macrophage populations from the Immgen database. (C) Principle component analysis (PCA) was performed on 50% of the genes defined by highest variance across all samples. Each dot of the same color represents a replicate sample. For each cluster, normal confidence ellipses are indicated. (D) Box plots of the log2 expression values of the indicated genes in cDC2 from Clec9acre/creRosaDTA mice and YFP+ cDC2. (E,F) CD11c+MHCII+CD11b+ cDC2 from control and Clec9acre/creRosaDTA mice were sorted and genomic DNA was isolated. (E) Polymerase chain reaction (PCR) was performed using primers for the germline (GL) locus and primer mixtures homologous for regions of the Dfl16 and Dsp2 D gene families for detecting D–J rearrangements of the IgH chain. DJ rearrangements in pDCs (SiglecH+B220+) and neutrophils (Ly-6G+) are shown as control. * indicates an unspecific band. (F) Genomic PCR was performed using primers for the GL locus and primer sets for the DHQ52 element for detecting D–J rearrangements of the IgH chain. DJ rearrangements in pDCs (SiglecH+B220+), CD205+ cDC1, and neutrophils (Ly-6G+) are shown as control. Each lane in E and F represents independent replicates from different mice.
We next performed principal component analysis (PCA) in the context of published datasets for splenic cDCs, thymic cDCs, migratory cDCs, splenic pDCs, macrophages, and monocytes. Notably, cDC2 from Clec9aCre/CreRosaDTA mice were most closely related to YFP+ cDC2 and splenic cDC2 and clustered far away from monocytes/macrophages, thymic cDCs, or pDCs (Figure 5C). In humans, a subset of cDC2 has been identified that appears to be related to pDCs (66). These cells can be distinguished from cDC2 and pDCs by expression of signature genes, including Axl, Pp1rb, Dab2, CD22, and IL3Ra (66). However, we found no enrichment of the Axl gene signature in cDC2 from Clec9aCre/CreRosaDTA mice compared with YFP+ cDC2 (Figure 5D). Taken together, these data demonstrate that cDC2 from Clec9aCre/CreRosaDTA mice do not show a comprehensive loss of cDC identity and resemble cDC2 from wild type mice.
CLPs can give rise to cDCs in vivo and in vitro under certain conditions (41–48) and pDCs in normal mice can originate from myeloid or lymphoid progenitors that appear phenotypically indistinguishable except for a history of RAG expression in those originating from the latter (50, 67–69). We therefore sorted CD11b+CD11c+MHCII+ cells from control and Clec9aCre/CreRosaDTA mice (Figures 5E,F; Figures S5A–C in Supplementary Material) and performed PCR analysis for Ig gene rearrangements indicative of historical RAG activity. Sorted pDCs from control mice exhibited prominent Ig gene rearrangements (Figures 5E,F; Figure S5C in Supplementary Material), as reported (49, 50), serving as a positive control. Strikingly, cDC2 from Clec9aCre/CreRosaDTA mice also showed clear evidence of Ig gene rearrangements, which were completely absent in cDC2 from control mice (Figures 5E,F; Figure S5C in Supplementary Material). In summary, cDC2 that arise in the absence of Clec9a-expressing cDC progenitors are transcriptionally similar to bona fide cDC2 but they exhibit RAG gene expression history in the form of D–J somatic rearrangements, indicating a putative lymphoid origin.
Discussion
Although the existence of a lymphoid path to cDC development in mice has been suggested (43, 47, 49, 50, 70), in steady-state cDCs arise primarily from myeloid progenitors (53). Here, we report the generation of Clec9aCre/CreRosaDTA mice as a model to constitutively deplete Clec9a-expressing cDC progenitors and their progeny. We show that in the absence of these myeloid-derived cDC progenitors, cells that are phenotypically and transcriptionally similar to bona fide cDC2 develop in spleen. These cells show RAG gene expression history in the form of D-J rearrangements at the IgH locus indicating a relation to the lymphoid branch of hematopoiesis. Therefore, our study suggests that lymphopoiesis can contribute to the generation of cDC-like cells in a situation in which myeloid cDC progenitors are impaired. This observation opens an interesting conundrum, namely, whether such cells should be considered part of the cDC lineage. We and others have proposed that mononuclear phagocytes should predominantly be defined on the basis of their ontogeny (12, 71, 72). However, environmental imprinting appears to be a major identity determining criterion for mononuclear phagocytes (73–77). Therefore, an ontogenetic view to cell definition may be less tenable if cell populations with different origins turn out to be identical by all measures examined. Because cDC2 in Clec9aCre/CreRosaDTA mice are not CDP-derived but show lymphoid gene expression history, we suggest to refer to these cells as lymphoid DC2 for the time being (12). Although we find strong similarities between cDC2 and lymphoid DC2, additional studies are necessary to further define the transcriptional and growth factor requirements, as well as functional properties of lymphoid DC2 to firmly establish their lineage affiliation and determine whether these cells should be considered a new type of mononuclear phagocyte (12).
As cDC differentiation from precursors is thought to be homeostatically regulated by the size of the mature cDC pool (78, 79), we cannot formally exclude at this point that some CDPs escape deletion in Clec9aCre/CreRosaDTA mice to preferentially expand and contribute to the cDC2 population. However, our data demonstrate that at least part of the cDC2 pool in Clec9aCre/CreRosaDTA mice derives from a cell type that has undergone Ig gene rearrangement and that pool is therefore qualitatively distinct from CDP-derived cDC2. Whether these ontogenetically distinct cDC2s arise from early lymphoid-committed progenitors, such as CLPs, is an attractive possibility that will need to be explored. However, it is worth mentioning that some myeloid progenitors reportedly express RAG1 and can generate pDCs with D-J rearrangements in vitro (50). Such RAG1-expressing myeloid progenitors, which would presumably lack DNGR-1, could contribute to cDC2 generation in Clec9aCre/CreRosaDTA mice, in which case our designation of these cells as “lymphoid cDC2” would be incorrect. Finally, it is possible that pDCs, some of which have a lymphoid past (49, 50), could convert to lymphoid cDC2 in the FLT3L-rich environment of Clec9aCre/CreRosaDTA mice, although this is difficult to reconcile with the fact that we failed to find any pDC-specific transcripts in lymphoid DC2 (Figure 5 and unpublished observations). Another intriguing observation is that lymphoid DC2 display decreased surface staining for CD135. Gene expression analysis suggests that CD135 may be dysregulated at the RNA level (Figure 5). However, it is equally possible that CD135 could be downregulated upon continuous receptor engagement by FLT3L (80) or may no longer be available for antibody binding (Figure S3D in Supplementary Material). As FLT3L-deficient mice lack all cDCs (33, 81), it is unlikely that lymphoid DC2 arise independently of FLT3L. It will be important to determine whether different growth factors may preferentially promote the differentiation of lymphoid and myeloid progenitors into cDCs.
The fact that lymphoid DC2 are strikingly similar to bona fide cDC2 of myeloid origin in terms of transcriptional profile is in agreement with data demonstrating that in vitro generated human myeloid-derived cDC1 are indistinguishable from ones derived from human lymphoid-committed progenitors (42). These data suggest a primary role for the environment in the functional imprinting of cDCs, similar to observations made for macrophages and monocytes (73–76). Despite functional similarities between lymphoid DC2 and cDC2 in vitro, these cells respond somewhat differently to the TLR ligand LPS. These data raise the possibility that lymphoid DC2 are not fully functionally equivalent to cDC2, which needs to be addressed experimentally in more detail. In this context, it is noteworthy that Clec9aCre/CreRosaDTA mice develop signs of systemic myeloproliferation, which is typical of mice lacking both cDC1 and cDC2 but has not been reported in mice lacking only the cDC1 subset (82, 83).
It will be important to determine whether additional situations exist where a lymphoid path may contribute to generating or replacing bona fide cDC2, either to ensure functional redundancy or to mediate specific immune functions. Notably, RAG-expressing immune-restricted progenitors contribute to embryonic myelopoiesis in the fetal liver and, although this occurs before the onset of definitive hematopoiesis (84), it indicates that lymphoid and myeloid lineage decisions are not always binary. During emergency hematopoiesis distinct monocyte progenitors have been suggested to respond to inflammatory stimuli on a per need basis, yielding a situation-adapted repertoire of inflammatory monocytes (85). It is intriguing to speculate that, in a similar manner, lymphoid and myeloid progenitors may be differentially triggered in certain conditions of inflammation to generate lymphoid DC2 and cDC2, respectively.
Ethics Statement
All animal experiments were performed in accordance with national and institutional guidelines for animal care and approved by the Francis Crick Institute Animal Ethics Committee, the UK Home Office, or the Regierung of Oberbayern.
Author Contributions
JS and BS planned and performed experiments. JB, NP, SR, MP, DP, NR, and SJK performed experiments. TS performed Microarray analysis. JS, BS, and CRS wrote the manuscript. BS and CRS designed the study.
Conflict of Interest Statement
The authors declare that the research was conducted in the absence of any commercial or financial relationships that could be construed as a potential conflict of interest.
Acknowledgments
We thank members of the Schraml lab for helpful discussions and critical reading of the manuscript. We also thank members of the Brocker and Krug laboratories for technical help. This work was supported by the German Research Foundation Emmy Noether Grant: Schr 1444/1-1 (to BS) and SFB914- project A11 (to BS), by The Francis Crick Institute (to CRS), which receives core funding from Cancer Research UK (FC001136), the UK Medical Research Council (FC001136), and the Wellcome Trust (FC001136), by an ERC Starting Grant awarded to BS (ERC-2016-STG-715182), and an ERC Advanced Investigator grant awarded to CRS (2010-AdG-268670).
Supplementary Material
The Supplementary Material for this article can be found online at https://www.frontiersin.org/articles/10.3389/fimmu.2018.00699/full#supplementary-material.
References
1. Merad M, Sathe P, Helft J, Miller J, Mortha A. The dendritic cell lineage: ontogeny and function of dendritic cells and their subsets in the steady state and the inflamed setting. Annu Rev Immunol (2013) 31:563–604. doi:10.1146/annurev-immunol-020711-074950
2. Steinman RM, Idoyaga J. Features of the dendritic cell lineage. Immunol Rev (2010) 234:5–17. doi:10.1111/j.0105-2896.2009.00888.x
3. Schlitzer A, Ginhoux F. Organization of the mouse and human DC network. Curr Opin Immunol (2014) 26:90–9. doi:10.1016/j.coi.2013.11.002
4. Durai V, Murphy KM. Functions of murine dendritic cells. Immunity (2016) 45:719–36. doi:10.1016/j.immuni.2016.10.010
5. Briseño CG, Murphy TL, Murphy KM. Complementary diversification of dendritic cells and innate lymphoid cells. Curr Opin Immunol (2014) 29:69–78. doi:10.1016/j.coi.2014.04.006
6. Satpathy AT, Briseño CG, Briseño GC, Lee JS, Ng D, Manieri NA, et al. Notch2-dependent classical dendritic cells orchestrate intestinal immunity to attaching-and-effacing bacterial pathogens. Nat Immunol (2013) 14:937–48. doi:10.1038/ni.2679
7. Kinnebrew MA, Buffie CG, Diehl GE, Zenewicz LA, Leiner I, Hohl TM, et al. Interleukin 23 production by intestinal CD103(+)CD11b(+) dendritic cells in response to bacterial flagellin enhances mucosal innate immune defense. Immunity (2012) 36:276–87. doi:10.1016/j.immuni.2011.12.011
8. Arora P, Baena A, Yu KO, Saini NK, Kharkwal SS, Goldberg MF, et al. A single subset of dendritic cells controls the cytokine bias of natural killer T cell responses to diverse glycolipid antigens. Immunity (2014) 40:105–16. doi:10.1016/j.immuni.2013.12.004
9. Loschko J, Schreiber HA, Rieke GJ, Esterházy D, Meredith MM, Pedicord VA, et al. Absence of MHC class II on cDCs results in microbial-dependent intestinal inflammation. J Exp Med (2016) 213:517–34. doi:10.1084/jem.20160062
10. Naik S, Bouladoux N, Linehan JL, Han S-J, Harrison OJ, Wilhelm C, et al. Commensal-dendritic-cell interaction specifies a unique protective skin immune signature. Nature (2015) 520:104–8. doi:10.1038/nature14052
11. Murphy TL, Grajales-Reyes GE, Wu X, Tussiwand R, Briseño CG, Iwata A, et al. Transcriptional control of dendritic cell development. Annu Rev Immunol (2015) 34:93–119. doi:10.1146/annurev-immunol-032713-120204
12. Guilliams M, Ginhoux F, Jakubzick C, Naik SH, Onai N, Schraml BU, et al. Dendritic cells, monocytes and macrophages: a unified nomenclature based on ontogeny. Nat Rev Immunol (2014) 14:571–8. doi:10.1038/nri3712
13. Tussiwand R, Everts B, Grajales-Reyes GE, Kretzer NM, Iwata A, Bagaitkar J, et al. Klf4 expression in conventional dendritic cells is required for T helper 2 cell responses. Immunity (2015) 42:916–28. doi:10.1016/j.immuni.2015.04.017
14. Scott CL, Soen B, Martens L, Skrypek N, Saelens W, Taminau J, et al. The transcription factor Zeb2 regulates development of conventional and plasmacytoid DCs by repressing Id2. J Exp Med (2016) 213(6):897–911. doi:10.1084/jem.20151715
15. Wu X, Briseño CG, Grajales-Reyes GE, Haldar M, Iwata A, Kretzer NM, et al. Transcription factor Zeb2 regulates commitment to plasmacytoid dendritic cell and monocyte fate. Proc Natl Acad Sci U S A (2016) 113:14775–80. doi:10.1073/pnas.1611408114
16. Guilliams M, Dutertre C-A, Scott CL, McGovern N, Sichien D, Chakarov S, et al. Unsupervised high-dimensional analysis aligns dendritic cells across tissues and species. Immunity (2016) 45:669–84. doi:10.1016/j.immuni.2016.08.015
17. Schlitzer A, McGovern N, Teo P, Zelante T, Atarashi K, Low D, et al. IRF4 transcription factor-dependent CD11b+ dendritic cells in human and mouse control mucosal IL-17 cytokine responses. Immunity (2013) 38:970–83. doi:10.1016/j.immuni.2013.04.011
18. Persson EK, Uronen-Hansson H, Semmrich M, Rivollier A, Hägerbrand K, Marsal J, et al. IRF4 transcription-factor-dependent CD103(+)CD11b(+) dendritic cells drive mucosal T helper 17 cell differentiation. Immunity (2013) 38:958–69. doi:10.1016/j.immuni.2013.03.009
19. Schraml BU, van Blijswijk J, Zelenay S, Whitney PG, Filby A, Acton SE, et al. Genetic tracing via DNGR-1 expression history defines dendritic cells as a hematopoietic lineage. Cell (2013) 154:843–58. doi:10.1016/j.cell.2013.07.014
20. Naik SH, Perié L, Swart E, Gerlach C, van Rooij N, de Boer RJ, et al. Diverse and heritable lineage imprinting of early haematopoietic progenitors. Nature (2013) 496:229–32. doi:10.1038/nature12013
21. Pei W, Feyerabend TB, Rössler J, Wang X, Postrach D, Busch K, et al. Polylox barcoding reveals haematopoietic stem cell fates realized in vivo. Nature (2017) 548:456–60. doi:10.1038/nature23653
22. Paul F, Arkin Y, Giladi A, Jaitin DA, Kenigsberg E, Keren-Shaul H, et al. Transcriptional heterogeneity and lineage commitment in myeloid progenitors. Cell (2015) 163:1663–77. doi:10.1016/j.cell.2015.11.013
23. Perié L, Duffy KR, Kok L, de Boer RJ, Schumacher TN. The branching point in erythro-myeloid differentiation. Cell (2015) 163:1655–62. doi:10.1016/j.cell.2015.11.059
24. Schultze JL, Beyer M. Myelopoiesis reloaded: single-cell transcriptomics leads the way. Immunity (2016) 44:18–20. doi:10.1016/j.immuni.2015.12.019
25. Fogg DK, Sibon C, Miled C, Jung S, Aucouturier P, Littman DR, et al. A clonogenic bone marrow progenitor specific for macrophages and dendritic cells. Science (2006) 311:83–7. doi:10.1126/science.1117729
26. Auffray C, Fogg DK, Narni-Mancinelli E, Senechal B, Trouillet C, Saederup N, et al. CX3CR1+ CD115+ CD135+ common macrophage/DC precursors and the role of CX3CR1 in their response to inflammation. J Exp Med (2009) 206:595–606. doi:10.1084/jem.20081385
27. Sathe P, Metcalf D, Vremec D, Naik SH, Langdon WY, Huntington ND, et al. Lymphoid tissue and plasmacytoid dendritic cells and macrophages do not share a common macrophage-dendritic cell-restricted progenitor. Immunity (2014) 41:104–15. doi:10.1016/j.immuni.2014.05.020
28. Hettinger J, Richards DM, Hansson J, Barra MM, Joschko A-C, Krijgsveld J, et al. Origin of monocytes and macrophages in a committed progenitor. Nat Immunol (2013) 14:821–30. doi:10.1038/ni.2638
29. Onai N, Obata-Onai A, Schmid MA, Ohteki T, Jarrossay D, Manz MG. Identification of clonogenic common Flt3+M-CSFR+ plasmacytoid and conventional dendritic cell progenitors in mouse bone marrow. Nat Immunol (2007) 8:1207–16. doi:10.1038/ni1518
30. Naik SH, Sathe P, Park H-Y, Metcalf D, Proietto AI, Dakic A, et al. Development of plasmacytoid and conventional dendritic cell subtypes from single precursor cells derived in vitro and in vivo. Nat Immunol (2007) 8:1217–26. doi:10.1038/ni1522
31. Liu K, Victora GD, Schwickert TA, Guermonprez P, Meredith MM, Yao K, et al. In vivo analysis of dendritic cell development and homeostasis. Science (2009) 324:392–7. doi:10.1126/science.1170540
32. Naik SH, Metcalf D, Van Nieuwenhuijze A, Wicks I, Wu L, O’Keeffe M, et al. Intrasplenic steady-state dendritic cell precursors that are distinct from monocytes. Nat Immunol (2006) 7:663–71. doi:10.1038/ni1340
33. Ginhoux F, Liu K, Helft J, Bogunovic M, Greter M, Hashimoto D, et al. The origin and development of nonlymphoid tissue CD103+ DCs. J Exp Med (2009) 206:3115–30. doi:10.1084/jem.20091756
34. Grajales-Reyes GE, Iwata A, Albring J, Wu X, Tussiwand R, Kc W, et al. Batf3 maintains autoactivation of Irf8 for commitment of a CD8α(+) conventional DC clonogenic progenitor. Nat Immunol (2015) 16:708–17. doi:10.1038/ni.3197
35. Schlitzer A, Sivakamasundari V, Chen J, Sumatoh HRB, Schreuder J, Lum J, et al. Identification of cDC1- and cDC2-committed DC progenitors reveals early lineage priming at the common DC progenitor stage in the bone marrow. Nat Immunol (2015) 16:718–28. doi:10.1038/ni.3200
36. Breton G, Lee J, Zhou YJ, Schreiber JJ, Keler T, Puhr S, et al. Circulating precursors of human CD1c+ and CD141+ dendritic cells. J Exp Med (2015) 212:401–13. doi:10.1084/jem.20141441
37. Breton G, Zheng S, Valieris R, Tojal da Silva I, Satija R, Nussenzweig MC. Human dendritic cells (DCs) are derived from distinct circulating precursors that are precommitted to become CD1c +or CD141 +DCs. J Exp Med (2016) 213:2861–70. doi:10.1084/jem.20161135
38. Lee J, Breton G, Oliveira TYK, Zhou YJ, Aljoufi A, Puhr S, et al. Restricted dendritic cell and monocyte progenitors in human cord blood and bone marrow. J Exp Med (2015) 212:385–99. doi:10.1084/jem.20141442
39. Poulin LF, Reyal Y, Uronen-Hansson H, Schraml BU, Sancho D, Murphy KM, et al. DNGR-1 is a specific and universal marker of mouse and human Batf3-dependent dendritic cells in lymphoid and nonlymphoid tissues. Blood (2012) 119:6052–62. doi:10.1182/blood-2012-01-406967
40. Collin M, Bigley V, Haniffa M, Hambleton S. Human dendritic cell deficiency: the missing ID? Nat Rev Immunol (2011) 11:575–83. doi:10.1038/nri3046
41. Manz MG, Traver D, Miyamoto T, Weissman IL, Akashi K. Dendritic cell potentials of early lymphoid and myeloid progenitors. Blood (2001) 97:3333–41. doi:10.1182/blood.V97.11.3333
42. Helft J, Anjos-Afonso F, Van der Veen AG, Chakravarty P, Bonnet D, Reis e Sousa C. Dendritic cell lineage potential in human early hematopoietic progenitors. Cell Rep (2017) 20:529–37. doi:10.1016/j.celrep.2017.06.075
43. Izon D, Rudd K, DeMuth W, Pear WS, Clendenin C, Lindsley RC, et al. A common pathway for dendritic cell and early B cell development. J Immunol (2001) 167:1387–92. doi:10.4049/jimmunol.167.3.1387
44. Ardavin C, Wu L, Li CL, Shortman K. Thymic dendritic cells and T cells develop simultaneously in the thymus from a common precursor population. Nature (1993) 362:761–3. doi:10.1038/362761a0
45. Welner RS, Esplin BL, Garrett KP, Pelayo R, Luche H, Fehling HJ, et al. Asynchronous RAG-1 expression during B lymphopoiesis. J Immunol (2009) 183:7768–77. doi:10.4049/jimmunol.0902333
46. Becker AM, Michael DG, Satpathy AT, Sciammas R, Singh H, Bhattacharya D. IRF-8 extinguishes neutrophil production and promotes dendritic cell lineage commitment in both myeloid and lymphoid mouse progenitors. Blood (2012) 119:2003–12. doi:10.1182/blood-2011-06-364976
47. Wu L, D’Amico A, Hochrein H, O’Keeffe M, Shortman K, Lucas K. Development of thymic and splenic dendritic cell populations from different hemopoietic precursors. Blood (2001) 98:3376–82. doi:10.1182/blood.V98.12.3376
48. Lee J, Zhou YJ, Ma W, Zhang W, Aljoufi A, Luh T, et al. Lineage specification of human dendritic cells is marked by IRF8 expression in hematopoietic stem cells and multipotent progenitors. Nat Immunol (2017) 18:877–88. doi:10.1038/ni.3789
49. Corcoran L, Ferrero I, Vremec D, Lucas K, Waithman J, O’Keeffe M, et al. The lymphoid past of mouse plasmacytoid cells and thymic dendritic cells. J Immunol (2003) 170:4926–32. doi:10.4049/jimmunol.170.10.4926
50. Sathe P, Vremec D, Wu L, Corcoran L, Shortman K. Convergent differentiation: myeloid and lymphoid pathways to murine plasmacytoid dendritic cells. Blood (2013) 121:11–9. doi:10.1182/blood-2012-02-413336
51. Bar-On L, Birnberg T, Lewis KL, Edelson BT, Bruder D, Hildner K, et al. CX3CR1+ CD8alpha+ dendritic cells are a steady-state population related to plasmacytoid dendritic cells. Proc Natl Acad Sci U S A (2010) 107:14745–50. doi:10.1073/pnas.1001562107
52. Shigematsu H, Reizis B, Iwasaki H, Mizuno S-I, Hu D, Traver D, et al. Plasmacytoid dendritic cells activate lymphoid-specific genetic programs irrespective of their cellular origin. Immunity (2004) 21:43–53. doi:10.1016/j.immuni.2004.06.011
53. Schlenner SM, Madan V, Busch K, Tietz A, Läufle C, Costa C, et al. Fate mapping reveals separate origins of T cells and myeloid lineages in the thymus. Immunity (2010) 32:426–36. doi:10.1016/j.immuni.2010.03.005
54. Luche H, Ardouin L, Teo P, See P, Henri S, Merad M, et al. The earliest intrathymic precursors of CD8α(+) thymic dendritic cells correspond to myeloid-type double-negative 1c cells. Eur J Immunol (2011) 41:2165–75. doi:10.1002/eji.201141728
55. Ivanova A, Signore M, Caro N, Greene NDE, Copp AJ, Martinez-Barbera JP. In vivo genetic ablation by Cre-mediated expression of diphtheria toxin fragment A. Genesis (2005) 43:129–35. doi:10.1002/gene.20162
56. Srinivas S, Watanabe T, Lin CS, William CM, Tanabe Y, Jessell TM, et al. Cre reporter strains produced by targeted insertion of EYFP and ECFP into the ROSA26 locus. BMC Dev Biol (2001) 1:4. doi:10.1186/1471-213X-1-4
57. Buch T, Heppner FL, Tertilt C, Heinen TJAJ, Kremer M, Wunderlich FT, et al. A Cre-inducible diphtheria toxin receptor mediates cell lineage ablation after toxin administration. Nat Methods (2005) 2:419–26. doi:10.1038/nmeth762
58. Schlissel MS, Corcoran LM, Baltimore D. Virus-transformed pre-B cells show ordered activation but not inactivation of immunoglobulin gene rearrangement and transcription. J Exp Med (1991) 173:711–20. doi:10.1084/jem.173.3.711
59. Birnberg T, Bar-On L, Sapoznikov A, Caton ML, Cervantes-Barragán L, Makia D, et al. Lack of conventional dendritic cells is compatible with normal development and T cell homeostasis, but causes myeloid proliferative syndrome. Immunity (2008) 29:986–97. doi:10.1016/j.immuni.2008.10.012
60. Sichien D, Scott CL, Martens L, Vanderkerken M, Van Gassen S, Plantinga M, et al. IRF8 transcription factor controls survival and function of terminally differentiated conventional and plasmacytoid dendritic cells, respectively. Immunity (2016) 45:626–40. doi:10.1016/j.immuni.2016.08.013
61. Jiao J, Dragomir A-C, Kocabayoglu P, Rahman AH, Chow A, Hashimoto D, et al. Central role of conventional dendritic cells in regulation of bone marrow release and survival of neutrophils. J Immunol (2014) 192:3374–82. doi:10.4049/jimmunol.1300237
62. Tittel AP, Heuser C, Ohliger C, Llanto C, Yona S, Hämmerling GJ, et al. Functionally relevant neutrophilia in CD11c diphtheria toxin receptor transgenic mice. Nat Methods (2012) 9:385–90. doi:10.1038/nmeth.1905
63. Holtschke T, Löhler J, Kanno Y, Fehr T, Giese N, Rosenbauer F, et al. Immunodeficiency and chronic myelogenous leukemia-like syndrome in mice with a targeted mutation of the ICSBP gene. Cell (1996) 87:307–17. doi:10.1016/S0092-8674(00)81348-3
64. van Blijswijk J, Schraml BU, Rogers NC, Whitney PG, Zelenay S, Acton SE, et al. Altered lymph node composition in diphtheria toxin receptor-based mouse models to ablate dendritic cells. J Immunol (2015) 194:307–15. doi:10.4049/jimmunol.1401999
65. Miller JC, Brown BD, Shay T, Gautier EL, Jojic V, Cohain A, et al. Deciphering the transcriptional network of the dendritic cell lineage. Nat Immunol (2012) 13:888–99. doi:10.1038/ni.2370
66. Villani A-C, Satija R, Reynolds G, Sarkizova S, Shekhar K, Fletcher J, et al. Single-cell RNA-seq reveals new types of human blood dendritic cells, monocytes, and progenitors. Science (2017) 356:eaah4573. doi:10.1126/science.aah4573
67. Shortman K, Sathe P, Vremec D, Naik S, O’Keeffe M. Plasmacytoid dendritic cell development. Adv Immunol (2013) 120:105–26. doi:10.1016/B978-0-12-417028-5.00004-1
68. Reizis B. Regulation of plasmacytoid dendritic cell development. Curr Opin Immunol (2010) 22:206–11. doi:10.1016/j.coi.2010.01.005
69. Pelayo R, Hirose J, Huang J, Garrett KP, Delogu A, Busslinger M, et al. Derivation of 2 categories of plasmacytoid dendritic cells in murine bone marrow. Blood (2005) 105:4407–15. doi:10.1182/blood-2004-07-2529
70. Vremec D, Shortman K. What’s in a name? Some early and current issues in dendritic cell nomenclature. Front Immunol (2015) 6:267. doi:10.3389/fimmu.2015.00267
71. Schraml BU, Reis e Sousa C. Defining dendritic cells. Curr Opin Immunol (2015) 32:13–20. doi:10.1016/j.coi.2014.11.001
72. Ginhoux F, Guilliams M, Naik S. Dendritic cell and macrophage nomenclature and classification. Front Immunol (2016). doi:10.3389/978-2-88919-918-1
73. Lavin Y, Winter D, Blecher-Gonen R, David E, Keren-Shaul H, Merad M, et al. Tissue-resident macrophage enhancer landscapes are shaped by the local microenvironment. Cell (2014) 159:1312–26. doi:10.1016/j.cell.2014.11.018
74. Gosselin D, Link VM, Romanoski CE, Fonseca GJ, Eichenfield DZ, Spann NJ, et al. Environment drives selection and function of enhancers controlling tissue-specific macrophage identities. Cell (2014) 159:1327–40. doi:10.1016/j.cell.2014.11.023
75. Gosselin D, Skola D, Coufal NG, Holtman IR, Schlachetzki JCM, Sajti E, et al. An environment-dependent transcriptional network specifies human microglia identity. Science (2017) 1617:eaal3222. doi:10.1126/science.aal3222
76. van de Laar L, Saelens W, De Prijck S, Martens L, Scott CL, Van Isterdael G, et al. Yolk sac macrophages, fetal liver, and adult monocytes can colonize an empty niche and develop into functional tissue-resident macrophages. Immunity (2016) 44:1–27. doi:10.1016/j.immuni.2016.02.017
77. Gibbings SL, Goyal R, Desch AN, Leach SM, Prabagar M, Atif SM, et al. Transcriptome analysis highlights the conserved difference between embryonic and postnatal-derived alveolar macrophages. Blood (2015) 126:1357–66. doi:10.1182/blood-2015-01-624809
78. Hochweller K, Miloud T, Striegler J, Naik S, Hämmerling GJ, Garbi N. Homeostasis of dendritic cells in lymphoid organs is controlled by regulation of their precursors via a feedback loop. Blood (2009) 114:4411–21. doi:10.1182/blood-2008-11-188045
79. Kabashima K, Banks TA, Ansel KM, Lu TT, Ware CF, Cyster JG. Intrinsic lymphotoxin-beta receptor requirement for homeostasis of lymphoid tissue dendritic cells. Immunity (2005) 22:439–50. doi:10.1016/j.immuni.2005.02.007
80. Tussiwand R, Onai N, Mazzucchelli L, Manz MG. Inhibition of natural type I IFN-producing and dendritic cell development by a small molecule receptor tyrosine kinase inhibitor with Flt3 affinity. J Immunol (2005) 175:3674–80. doi:10.4049/jimmunol.175.6.3674
81. McKenna HJ, Stocking KL, Miller RE, Brasel K, De Smedt T, Maraskovsky E, et al. Mice lacking flt3 ligand have deficient hematopoiesis affecting hematopoietic progenitor cells, dendritic cells, and natural killer cells. Blood (2000) 95:3489–97.
82. Hildner K, Edelson BT, Purtha WE, Diamond M, Matsushita H, Kohyama M, et al. Batf3 deficiency reveals a critical role for CD8alpha+ dendritic cells in cytotoxic T cell immunity. Science (2008) 322:1097–100. doi:10.1126/science.1164206
83. Ohta T, Sugiyama M, Hemmi H, Yamazaki C, Okura S, Sasaki I, et al. Crucial roles of XCR1-expressing dendritic cells and the XCR1- XCL1 chemokine axis in intestinal immune homeostasis. Sci Rep (2016) 6:1–11. doi:10.1038/srep23505
84. Böiers C, Carrelha J, Lutteropp M, Luc S, Green JCA, Azzoni E, et al. Lymphomyeloid contribution of an immune-restricted progenitor emerging prior to definitive hematopoietic stem cells. Cell Stem Cell (2013) 13:535–48. doi:10.1016/j.stem.2013.08.012
Keywords: dendritic cell, development, CLEC9A/DNGR-1, DC depletion, myelopoiesis, lymphopoiesis, fate mapping
Citation: Salvermoser J, van Blijswijk J, Papaioannou NE, Rambichler S, Pasztoi M, Pakalniškytė D, Rogers NC, Keppler SJ, Straub T, Reis e Sousa C and Schraml BU (2018) Clec9a-Mediated Ablation of Conventional Dendritic Cells Suggests a Lymphoid Path to Generating Dendritic Cells In Vivo. Front. Immunol. 9:699. doi: 10.3389/fimmu.2018.00699
Received: 07 February 2018; Accepted: 21 March 2018;
Published: 16 April 2018
Edited by:
Simon Yona, University College London, United KingdomReviewed by:
Charlotte Scott, VIB-UGent Center for Inflammation Research (IRC), BelgiumDawn Shuiping Lin, Walter and Eliza Hall Institute of Medical Research, Australia
Ken Shortman, Walter and Eliza Hall Institute of Medical Research, Australia
Copyright: © 2018 Salvermoser, van Blijswijk, Papaioannou, Rambichler, Pasztoi, Pakalniškytė, Rogers, Keppler, Straub, Reis e Sousa and Schraml. This is an open-access article distributed under the terms of the Creative Commons Attribution License (CC BY). The use, distribution or reproduction in other forums is permitted, provided the original author(s) and the copyright owner are credited and that the original publication in this journal is cited, in accordance with accepted academic practice. No use, distribution or reproduction is permitted which does not comply with these terms.
*Correspondence: Caetano Reis e Sousa, Y2FldGFub0Bjcmljay5hYy51aw==;
Barbara U. Schraml, YmFyYmFyYS5zY2hyYW1sQG1lZC51bmktbXVlbmNoZW4uZGU=
†These authors have contributed equally to this work.