- 1Department of Biosystems Science and Engineering, ETH Zurich, Basel, Switzerland
- 2Novartis Institutes for BioMedical Research, Basel, Switzerland
High-throughput sequencing of immunoglobulin (Ig) repertoires (Ig-seq) is a powerful method for quantitatively interrogating B cell receptor sequence diversity. When applied to human repertoires, Ig-seq provides insight into fundamental immunological questions, and can be implemented in diagnostic and drug discovery projects. However, a major challenge in Ig-seq is ensuring accuracy, as library preparation protocols and sequencing platforms can introduce substantial errors and bias that compromise immunological interpretation. Here, we have established an approach for performing highly accurate human Ig-seq by combining synthetic standards with a comprehensive error and bias correction pipeline. First, we designed a set of 85 synthetic antibody heavy-chain standards (in vitro transcribed RNA) to assess correction workflow fidelity. Next, we adapted a library preparation protocol that incorporates unique molecular identifiers (UIDs) for error and bias correction which, when applied to the synthetic standards, resulted in highly accurate data. Finally, we performed Ig-seq on purified human circulating B cell subsets (naïve and memory), combined with a cellular replicate sampling strategy. This strategy enabled robust and reliable estimation of key repertoire features such as clonotype diversity, germline segment, and isotype subclass usage, and somatic hypermutation. We anticipate that our standards and error and bias correction pipeline will become a valuable tool for researchers to validate and improve accuracy in human Ig-seq studies, thus leading to potentially new insights and applications in human antibody repertoire profiling.
Introduction
Adaptive immune responses are governed by cooperative interactions between B and T lymphocytes upon antigen recognition. A hallmark of these cells is the somatic generation of clonally unique antigen receptors during primary lymphocyte differentiation. In particular, B cell antigen receptors (BCRs, and their analogous secreted form, antibodies) are generated upon rearrangement of incomplete, germline-encoded variable (V), diversity (D, heavy-chain only), and joining (J) gene segments. Unique V(D)J recombination events in differentiating B cells create a highly complex repertoire of receptors [generally interchangeably referred to as BCR, antibody, or immunoglobulin (Ig) repertoires], which is shaped upon antigen experience to produce the more targeted, high-affinity memory BCR network. In-depth and accurate characterization of these repertoires provides valuable insight into the generation and maintenance of immunocompetence, which can be used to monitor changes in immune status, and to identify potentially reactive clones for therapeutic or other uses. Due to rapid technological advances, high-throughput sequencing of Ig genes (Ig-seq) has become a major approach to cataloguing the diversity of antibody repertoires (1–3). Ig-seq applied to human B cells has potential in a variety of applications (4), particularly in antibody drug discovery (5–7), profiling for vaccine development (8, 9), and biomarker-based diagnostics (10, 11). Additionally, Ig-seq is enabling a more comprehensive understanding of basic human immunobiology, for example by ascertaining B cell clonal distribution and repertoire complexity across physiological compartments in health and disease (12, 13).
A major challenge when performing Ig-seq is the production of accurate and high-quality datasets. Several current library preparation protocols are based on target enrichment from genomic DNA or mRNA (14). For example, the conversion of mRNA (more commonly used due to transcript abundance and isotype/subclass identification) into antibody sequencing libraries relies on a number of reagents and amplification steps (e.g., multiplex primer sets, PCR), which potentially introduce errors and bias. Due to the highly polymorphic nature of repertoires, especially from affinity-matured memory B cells and plasma cells, it is essential to determine if such technical noise occurs at non-negligible rates, as this could alter quantitation of critical repertoire features such as clonal frequencies, germline gene usage, and somatic hypermutation (SHM) (14, 15). One way to address this is by implementing synthetic control standards, for which the sequence and abundance is known prior to sequencing, thus providing a means to assess quality and accuracy (16). Several examples of Ig-seq standards have already been presented; Shugay et al. sequenced libraries prepared from a small polyclonal pool of B and T lymphocyte cell lines, and observed nearly 5% erroneous reads, resulting in approximately 100 false-positive variants per clone (17). Recently, Khan et al. developed a set of synthetic RNA (in vitro transcribed) spike-in standards based on mouse antibody sequences, which were used to show that a substantial amount of errors and bias are introduced during multiplex-PCR library preparation and sequencing (18).
Various experimental and computational workflows exist to mitigate the effects of errors and bias in Ig-seq. One of the most advanced and powerful strategies is to incorporate random, unique molecular identifiers (UIDs, also commonly referred to as UMIs or molecular barcodes) during library preparation. Following sequencing, error correction can be performed by clustering reads that share the same UID to form a consensus; reads sharing the same UID are assumed to be derived from the same original mRNA/cDNA molecule (19). Furthermore, by counting the number of UIDs (instead of total reads), one can correct for cDNA abundance bias (20, 21). Several iterations of UID-tagging have been developed for Ig-seq, such as UID-labeling during first- and second-strand cDNA synthesis (22), UID addition during reverse transcription (RT) template switching (23), and so-called “tagmentation” of UID-labeled amplicons (24). Recently, we developed an innovative strategy to add UIDs both during first-strand cDNA synthesis as well as multiplex-PCR amplification; this protocol, known as molecular amplification fingerprinting (MAF), results in comprehensive error and bias correction of mouse antibody repertoires (18).
Here, we describe an experimental–computational approach for producing highly accurate human Ig-seq data. We first designed a comprehensive set of synthetic standards based on human antibody heavy-chain variable (IGHV) sequences: a total of 85 in vitro transcribed RNA standards, each with a unique complementarity determining region 3 (CDR3) sequence and covering nearly the entire set of productive human Ig heavy-chain (IgH) germline (IGHV) gene segments. We used these synthetic standards to quantify the impact of errors and bias introduced during multiplex-PCR library preparation, and the robustness with which our previously developed method for UID addition by MAF could correct these artifact sequences. Finally, we implemented MAF-based error and bias correction on human B cell subsets (naïve and memory), which enabled us to make accurate clonal diversity estimates and quantify divergent repertoire features across B cell compartments.
Results
Design of a Comprehensive Set of Human Synthetic Standards
Our previously established murine synthetic antibody standard set contains 16 unique clones (CDR3s) covering 7 IGHV-gene segments (out of more than 140 annotated murine IGHV-gene segments) (18). For our human standards, we developed a more comprehensive set consisting of 85 clones encompassing nearly the entire germline IGHV repertoire. The most commonly used repository for human germline segments is the International ImMunoGenetTics Database (IMGT), which has annotated 61 IGHV alleles as functional or having an open reading frame (25). After filtering out paralogs and selecting only gene segments that have been found in productive rearrangements (2), we used 48 IGHV-gene segments to develop our standard collection (Table S1 in Supplementary Material). Each synthetic gene contained the following elements (5′–3′): (i) a conserved non-coding region, (ii) an ATG start codon and leader peptide sequence spliced to its respective IGHV-gene segment, (iii) a synthetic CDR3 sequence, (iv) a germline IgH J (IGHJ) gene segment, (v) a non-coding synthetic sequence identifier (for the separation of standards from biological sequences), (vi) partial segments of the constant region from isotypes IgM, IgG, and IgA (Figure 1A). This flexible design allows amplification of synthetic controls with a variety of PCR primer sets. Notably, for control experiments, all standards can be amplified by a single forward primer (targeting the conserved 5′ non-coding region) and a single reverse primer (targeting one of the isotype constant regions). Since IGHV gene segment usage has been reported to be non-uniform (2, 26), we selected the most abundant segments for use in multiple standards, adding synthetic point mutations to otherwise redundant IGHV sequences to mimic SHM (Figure 1B; Table S1 in Supplementary Material).
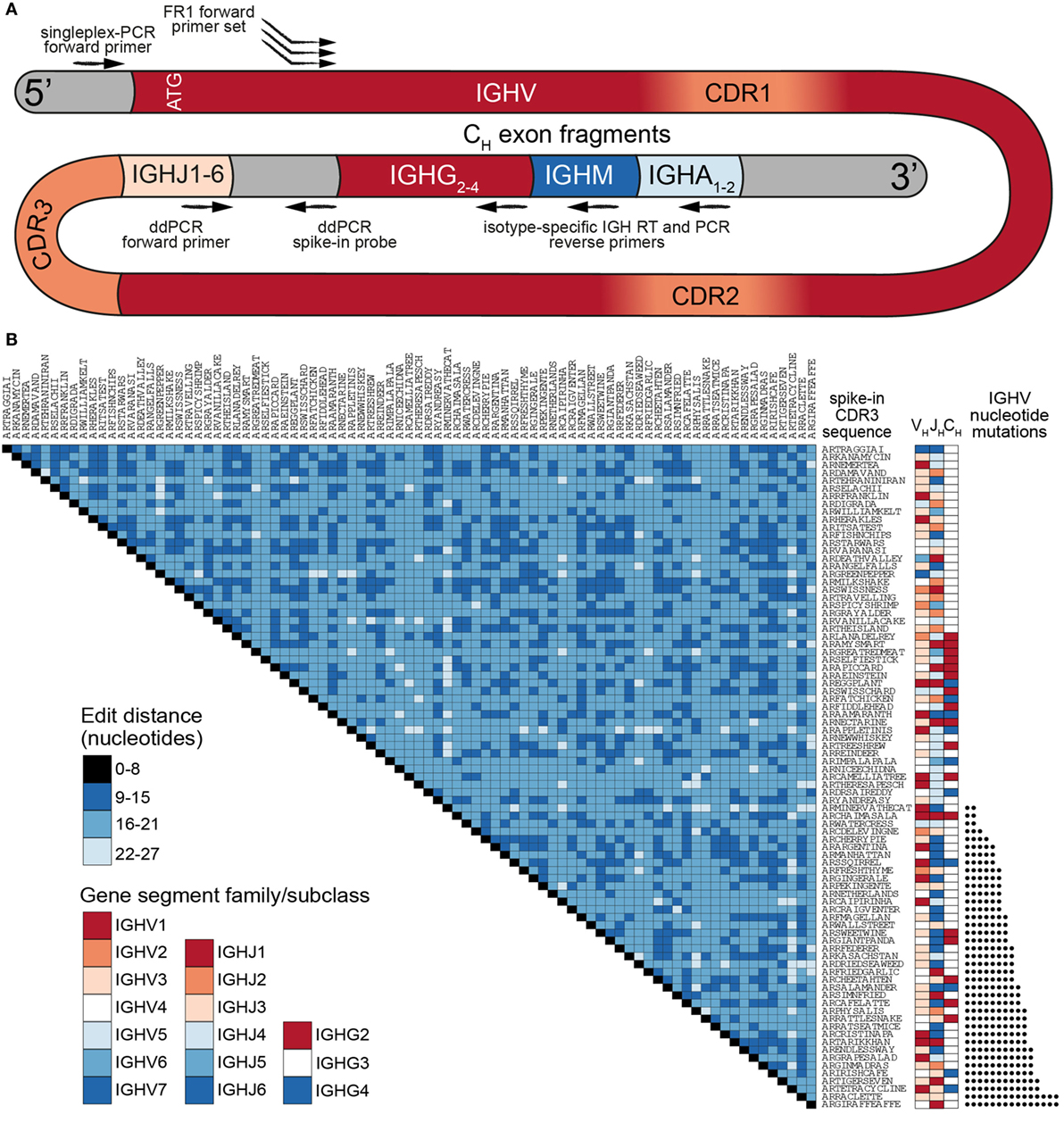
Figure 1. A comprehensive set of human synthetic spike-in standards for Ig-seq. (A) Schematic showing the prototypical spike-in with the following regions (5′–3′): a conserved non-coding region, ATG start codon, IGHV region with framework region 1 (FR1) specific for multiplex-PCR, IGHJ regions, non-coding synthetic sequence identifier [specific for digital droplet PCR (ddPCR) probes], and downstream heavy-chain constant domain sequences (IGHG, IGHM, IGHA) containing primer-binding sites used for cDNA synthesis. Each spike-in contains a complete VDJ open reading frame, including nucleotides upstream of the ATG start codon, and downstream constant domain sequences containing primer-binding sites used for sample cDNA synthesis. (B) Pairwise comparisons based on a.a. Levenshtein edit distance of all 85 standard complementarity determining region 3 (CDR3) sequences. The germline IGHV and IGHJ segment family usage and IgG subclasses are denoted. 39 spike-ins contain rationally designed nt somatic hypermutation (SHM) (black circles) across the IGHV regions.
All standards carry a unique CDR3 sequence, which visually aids the analysis of sequencing results. Furthermore, all clones were designed to be resilient against sequencing and PCR errors: at least 9 specific nucleotide (nt) deletions, insertions, and/or mutations are required to turn one CDR3 nt sequence into another (Figure 1B). For our experiments, synthetic standard genes were in vitro transcribed to RNA and subsequently reverse transcribed to cDNA. We quantitated individual cDNA molecules via digital droplet PCR (ddPCR) and capillary electrophoresis. Standards were then pooled in a non-uniform concentration distribution and maintained as a master stock (Table S1 in Supplementary Material).
Human Ig-Seq Library Preparation Using the MAF Protocol
We adapted our previously described library preparation protocol for murine antibody repertoires to be compatible with human Ig-seq (18). This protocol is based on targeted conversion of RNA to first-strand cDNA, followed by two PCR amplification steps (27, 28), the first of which uses a multiplex forward primer set targeting the IGHV framework region 1 (FR1). Each step also incorporates successive fragments of Illumina sequencing adapters (IA), such that the final product of the workflow is fully compatible with the Illumina sequencing platform (Figure 2A).
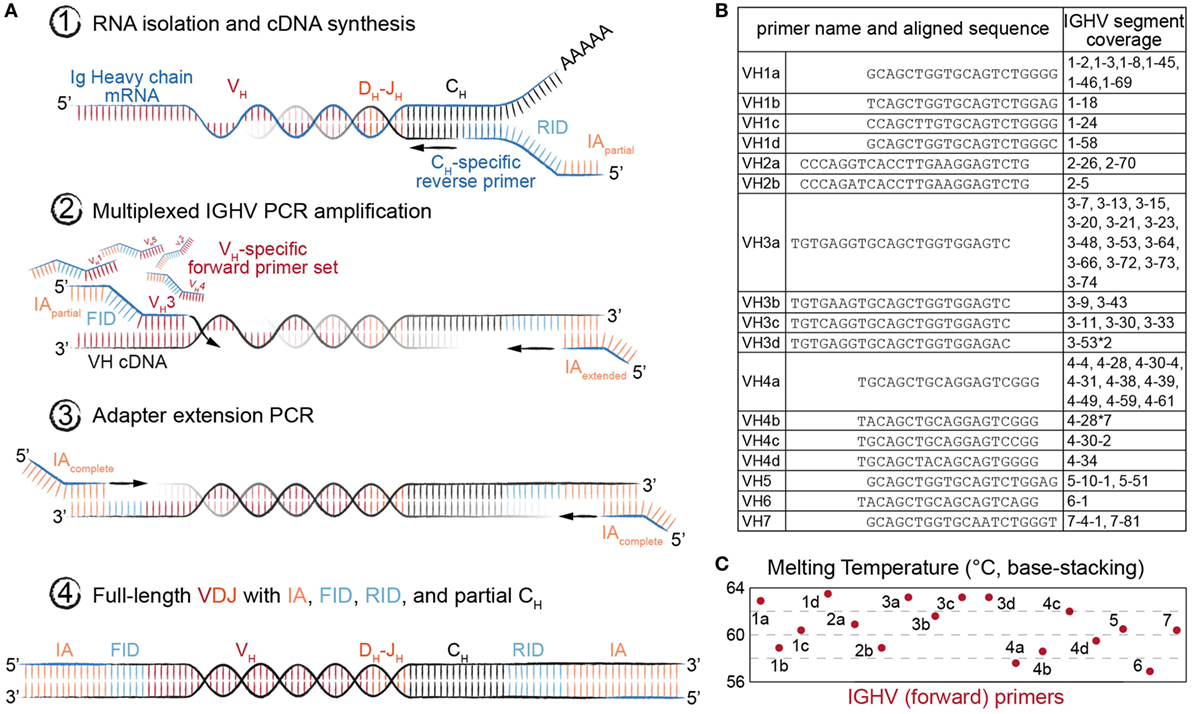
Figure 2. Library preparation of immunoglobulin (Ig) heavy-chain genes for high-throughput sequencing (Ig-seq) using molecular amplification fingerprinting (MAF). (A) In step 1, reverse transcription (RT) is performed to generate first-strand cDNA with a gene-specific (IgM or IgG) primer, which includes a unique reverse molecular identifier (RID) and partial Illumina adapter (IA) region. This results in single-molecule labeling of each cDNA with an RID. In step 2, several cycles of multiplex-PCR are performed using a forward primer set with gene-specific regions targeting heavy-chain variable (VH) framework region 1 (FR1), with overhang regions comprised of a forward unique molecular identifier (FID) and partial IA. In step 3, singleplex-PCR is used to extend the partial IAs. The result (Step 4) is the generation of antibody amplicons with FID, RIDs, and full IA ready for Ig-seq and subsequent MAF-based error and bias correction. (B) List of oligonucleotides sequences annealing to the VH FR1 used in multiplex-PCR (Step 2) of the MAF library preparation protocol. The nearest germline IGHV segment(s) likely to be amplified by the respective primer are listed in the rightmost column. (C) The estimated melting temperature distribution of the VH FR1 forward primer set.
Importantly, our library preparation protocol used primers incorporating random-nucleotide UIDs, thus enabling MAF-based error and bias correction. A reverse-UID (RID) with theoretical diversity up to 2 × 107 unique sequences is included in the RT primer (between the Ig constant region-specific and partial IA regions), and a forward-UID (FID with additional diversity of approximately 7 × 105 unique sequences) is present on the forward multiplex primer set (between the FR1-specific and partial IA regions) used in the first PCR reaction. Such high diversity among RIDs is necessary in order to prevent tagging of multiple cDNA molecules with the same barcode. Our multiplex forward primer set was designed to target all IMGT-annotated IGHV gene segments (Figure 2B). To compromise between maintaining identical amplicon length across gene segment families and creating thermodynamically equivalent oligonucleotides, we placed the primers at or near the beginning of each FR1, resulting in a melting temperature range of 57–63°C (Figure 2C). This range and the accompanying (unavoidable) variability in GC content have been shown to differentially impact amplification efficiencies, which leads to a biased representation of segment usage frequencies in Ig-seq data. Our workflow aimed to solve this problem in two ways: first, since the RID labels cDNA at the single-molecule level, we are able to resolve the number of molecules by counting the number of RIDs instead of raw reads. Second, by using the FIDs on our forward primers, we can further normalize our molecular count, since Ig genes preferentially amplified by the primer set should show a higher ratio of FIDs to RIDs. Additionally, the RIDs can be used to correct for errors introduced during PCR and the sequencing process itself by grouping sequencing reads based on their RID, then correcting diverging nt positions by generating a consensus sequence (majority voting scheme). This is especially useful in Ig-seq when attempting to distinguish true SHM variants from erroneous sequences.
Combining Standards With MAF to Correct Errors and Bias in Ig-Seq
To evaluate the extent of errors and bias present in human Ig-seq data, standards were mixed with cDNA prepared from circulating purified human B cells. In total, we sequenced 28 independently prepared libraries and annotated them with a custom aligner (18, 29). Prior to alignment, reads were either kept as uncorrected (raw) reads or were corrected using our MAF pipeline that takes into account RID and FID information. In this way, we could directly compare the number of erroneous sequences produced in uncorrected vs. MAF-corrected datasets. Clonal assignment of uncorrected reads produced many erroneous CDR3 amino acid (a.a.) variants (sequences with at least 1 a.a. difference from the nearest standard control sequence); for example, in a dataset with 100,000 aligned reads, there was a median value of 23 errors per clone (Figure 3A). The number of erroneous variants produced showed a clear correlation with the individual abundance of each clone within the master stock (r = 0.89). When taking the entire VDJ nt sequence into account, an even greater number of erroneous variants was observed (≥1 nt difference from the standard sequence) (Figure 3B). We observed a median value of 118 erroneous nt variants per standard (per 100,000 aligned sequences). Again, the number of erroneous variants exhibited a clear correlation with clone abundance (r = 0.90). However, we did not observe any significant trend linking IGHV family to the error rate (F-Test on full and reduced linear model, p = 0.083).
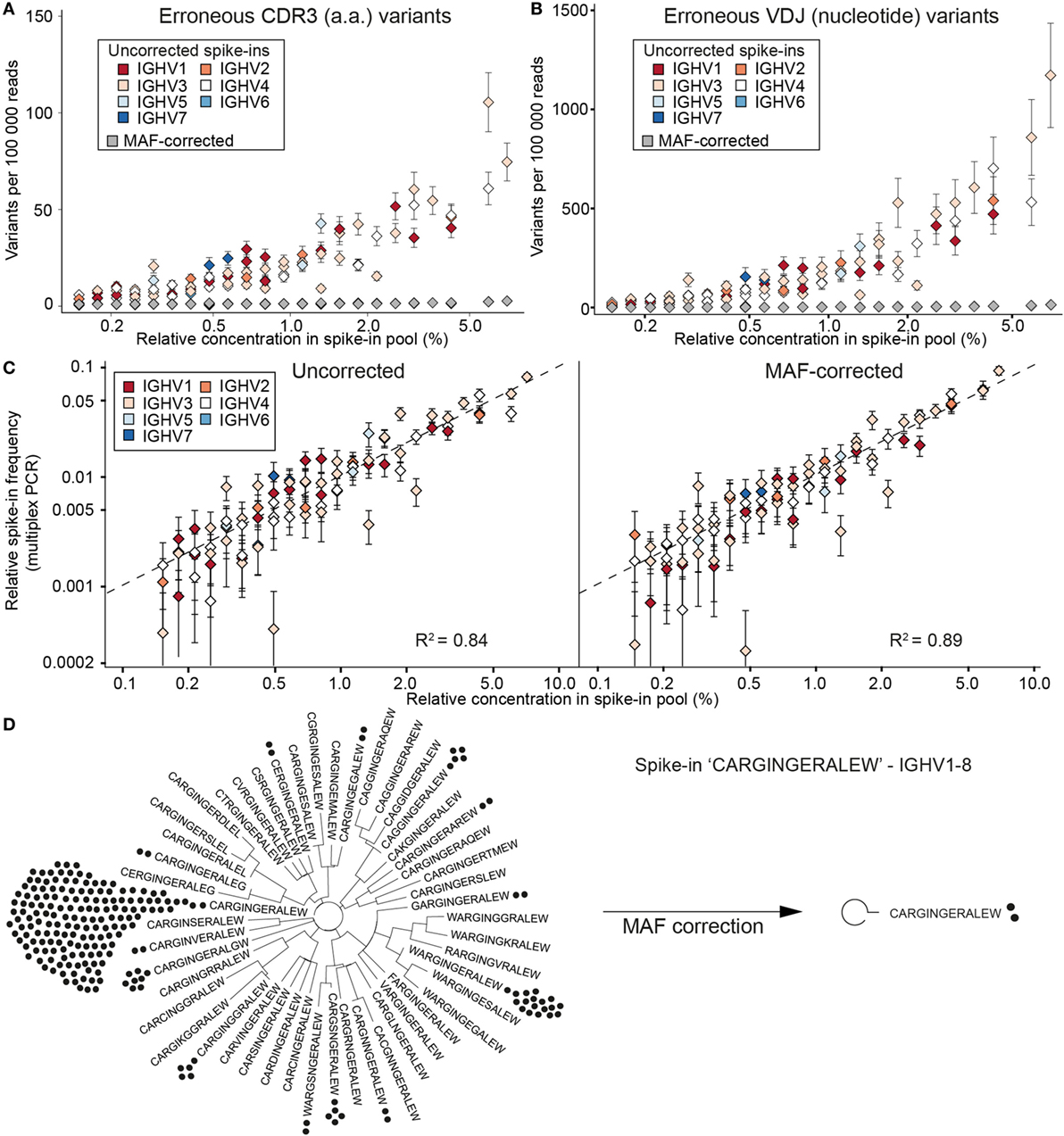
Figure 3. Synthetic standards used to evaluate error and bias correction of Ig-seq data by molecular amplification fingerprinting (MAF). (A) The number of erroneous complementarity determining region 3 (CDR3) sequences (≤1 a.a. difference from the correct CDR3) per 100,000 reads is plotted against the relative concentration of each standard in a master stock. Color-coded diamonds, germline IGHV segment family of the respective standard and number of erroneous variants in uncorrected (raw) data; gray diamonds, number of variants remaining after MAF error correction. (B) The number of erroneous VDJ variants derived from each standard was calculated by finding all variants that carried the correct CDR3 a.a. sequence, but differed by ≤1 nt across the entire VDJ region. Colored diamonds, uncorrected data; gray diamonds, variants remaining after MAF error correction. (C) Sequencing bias introduced by multiplex-PCR using the framework region 1 (FR1) primer set was assessed by plotting the measured frequencies of each standard against its relative concentration in the master stock. Dashed line represents a bias-free ideal scenario (R2 = 1). Left and right plots show observed frequencies pre- and post-MAF bias correction, respectively. (D) Phylogenetic trees visualizing the CDR3 a.a. variants present for a selected standard with the CDR3 a.a. sequence CARGINGERALEW using IGHV1-8 and IGHJ1. Prior to error correction, 39 erroneous CDR3 a.a. variants (branches) and 218 VDJ nt variants (black circles) were observed. Following MAF error correction, only the original correct CDR3 a.a. (two VDJ nt variants) remain. Ig-seq datasets for panels (A–C) consisted of ≈3 × 105 preprocessed full-length antibody reads from the synthetic spike-in only samples; dataset IgG1_D1 was used for panel (D) (see Table S3 in Supplementary Material).
After performing error correction with our MAF pipeline, there was a dramatic reduction of CDR3 and VDJ errors: we observed a median value of 0 and 1 error per clone, respectively. Across all datasets, we removed an average of 94.2% CDR3 a.a. and 97.4% VDJ nt erroneous variants (Figures 3A,B). For example, prior to error correction, the standard “CARGINGERALEW” (from dataset Donor 1, IgG aliquot 1, see Table S3 in Supplementary Material) displayed 39 additional CDR3 a.a. variants and 217 additional VDJ nt variants (Figure 3D). After error correction, we retain only the correct CDR3 a.a. sequence and one additional (erroneous) nt variant. With our current filtering criteria (see Methods), we observed 16 instances in which we removed a standard control sequence that was present in the raw data. However, in the vast majority of cases, we kept the standard when it was observed in the raw data (2,321 instances). In only 43 instances, a standard sequence was either too low in abundance or too frequently mutated to be annotated in either the raw or error-corrected datasets.
In order to assess potential bias introduced by library preparation, we prepared control libraries containing only the pool of synthetic standards (from the master stock). The libraries were generated in the same manner as the described MAF protocol, with the exception that in the first PCR step, instead of using a multiplex forward primer set, a single forward primer targeting the conserved 5′ non-coding region (singleplex-PCR) was used. Ig-seq on these samples allowed us to establish a baseline for pipetting accuracy by comparing the obtained standard frequencies from the singleplex-PCR against the expected frequencies based on our pooling scheme: this yielded an R2 of 0.88 and average mean squared error (MSE) of 0.29 ± 0.02% (Figure S1A in Supplementary Material). These values indicate that only small systematic deviations occurred, most likely due to minor pipetting error. Next, we compared standard frequencies (expected relative concentration) with frequencies generated in our previous multiplex-PCR libraries, both with and without MAF correction (Figure 3C). On uncorrected data, the multiplex-PCR libraries achieved an R2 of 0.84 with an average MSE of 0.34 ± 0.06%, which is significantly worse than the value obtained by singleplex-PCR (Student’s t-test p = 0.008). After error and bias correction on these same datasets, the correlation improved to an R2 of 0.89 and an average MSE of 0.28 ± 0.08%. While MAF-corrected MSE values show no significant difference to the singleplex-PCR libraries (Student’s t-test p = 0.49), they do highlight a significant improvement over the uncorrected data (paired Student’s t-test, p = 0.0007).
Impact of MAF Error Correction on Human B Cell Repertoires
Next, we analyzed the impact of MAF on the BCR repertoires of B cells isolated from the peripheral blood of three healthy donors. We used flow cytometry sorting to isolate CD27− IgM+ (naïve) and CD27+ IgG+ (memory) B cell populations (Figure 4A). Naïve cells were identified using a gating strategy to select IgM-expressing cells after eliminating transitional B cells (which express high levels of CD24 and CD38); although this strategy excludes a significant portion of naïve, mature B cells, it guarantees a high degree of purity based on empirical observations. Furthermore, this subset was not a limiting factor in our cross-population analysis, for which the goal was to obtain approximately equal numbers of cells from each fraction. Across all donors, the fraction of CD19+ peripheral blood B cells was 16–29% for CD27− IgM+ and 5–9% for CD27+ IgG+. Importantly, each donor population was split into four to five separate aliquots containing 200,000 cells each (cellular replicates) prior to cell lysis. Total RNA was extracted and RT for cDNA synthesis was performed independently in order to prevent the mixing of transcripts across cellular replicates. The cDNA of synthetic standards (from the master stock) was then mixed with the B cell cDNA, and corresponding molecular quantities were measured by ddPCR (Figure 4B; Table S3 in Supplementary Material). All cDNA libraries were then processed into libraries using the MAF protocol (Figure 2A) and subjected to Ig-seq.
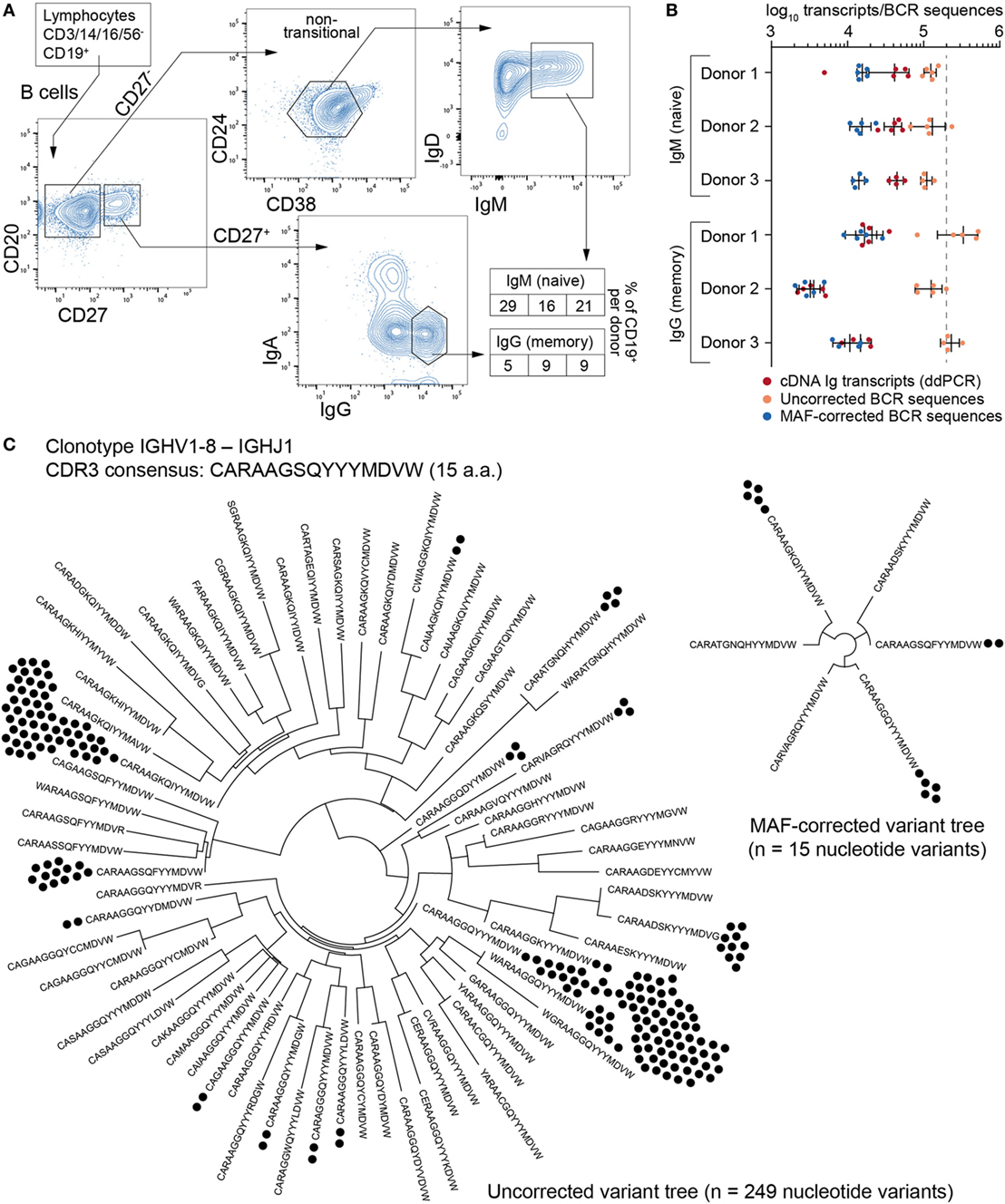
Figure 4. Ig-seq analysis of human naïve (CD27−IgM+) and memory (CD27+IgG+) B cells. (A) The flow cytometric workflow for isolating CD27−IgM+ and CD27+IgG+ B cells from peripheral blood. Boxed-in values indicate the frequency of each sorted subset as a percentage of the total B cell (CD19+) population from each of three donors (1–3 from left to right). (B) Experimental and Ig-seq based quantitation of antibody diversity; points represent cDNA molecule counts [using digital droplet PCR (ddPCR)] or unique reads [before and after molecular amplification fingerprinting (MAF) error correction] from cellular replicates (with mean and standard deviation shown) isolated from each donor and B cell subset. Unique read counts were based on the VDJ nt seqeunce. Dashed line represents the number of B cells isolated per cellular replicate (2 × 105 cells). (C) Phylogenetic trees illustrating complementarity determining region 3 (CDR3) a.a. and nt variants present for the selected clonotype with the consensus CDR3 sequence CARAAGSQYYYMDVW and IGHV1-8 to IGHJ1 recombination. Prior to error correction, 70 erroneous CDR3 a.a. variants and 249 VDJ nt variants (black circles) were observed. Following MAF error correction, only 6 CDR3 a.a. and 15 VDJ nt variants remain. The Ig-seq data sets used in (B) are described in Table S3 in Supplementary Material; IgG1_D1 was used for the tree in panel (C).
A simple global analysis of Ig-seq data revealed that diversity measurements were dramatically exaggerated, as the number of unique antibody sequence variants obtained from the raw, uncorrected data often exceeded both the number of cells and the number of total cDNA transcripts in a given aliquot. Following error correction by MAF, the variant count returned to ranges that are physically possible, thereby highlighting the importance of proper error correction and quality control when globally determining repertoire diversity (Figure 4B). We further examined the influence of erroneous variants on CDR3 clonotype analysis. In order to identify clonotypes, we used complete linkage hierarchical clustering (30) based on sequences sharing the following features: identical IGHV and IGHJ gene segment usage, identical CDR3 length, and a CDR3 a.a. similarity of at least 80% to one other sequence in the given clonotype. These criteria, previously established for clonotype clustering (30), are selected to be sufficiently stringent to retain synthetic standard sequences (as well as presumably unique naïve BCRs) as single, individual clonotypes, while being generous enough to identify clonally related sequences among antigen experienced, expanded memory B cells. Experimentally, using more or less rigorous conditions shifts reported numbers of unique clonotypes but does not dramatically influence the overall picture with respect to naïve and memory B cell subsets (Figure S2 in Supplementary Material). When performing such an analysis on uncorrected data, clonotypes contained an artificially high number of distinct clones (Figure 4C, left tree). Here, the IgG-derived clonotype with the consensus CDR3 of “CARAAGSQYYMDVW” (from the same sample and IGHV-gene segment used by the standard shown in Figure 3D) contains 249 unique nt variants and 70 unique a.a. sequences. After MAF-based error correction, only 15 nt variants and 6 distinct CDR3 a.a. sequences remained. It is worthy to note that although both the standard sequence (Figure 3D) and the biological clonotype (Figure 4C) had a large number of CDR3 a.a. variants in uncorrected data, after error correction only memory B cell clonotypes retained multiple a.a. variants, indicating these are true variants generated in vivo by SHM. This general trend of each IgG memory B cell-derived clonotype to contain more variants relative to antigen inexperienced IgM-expressing B cells was clear across our biological data sets (Figure 5A).
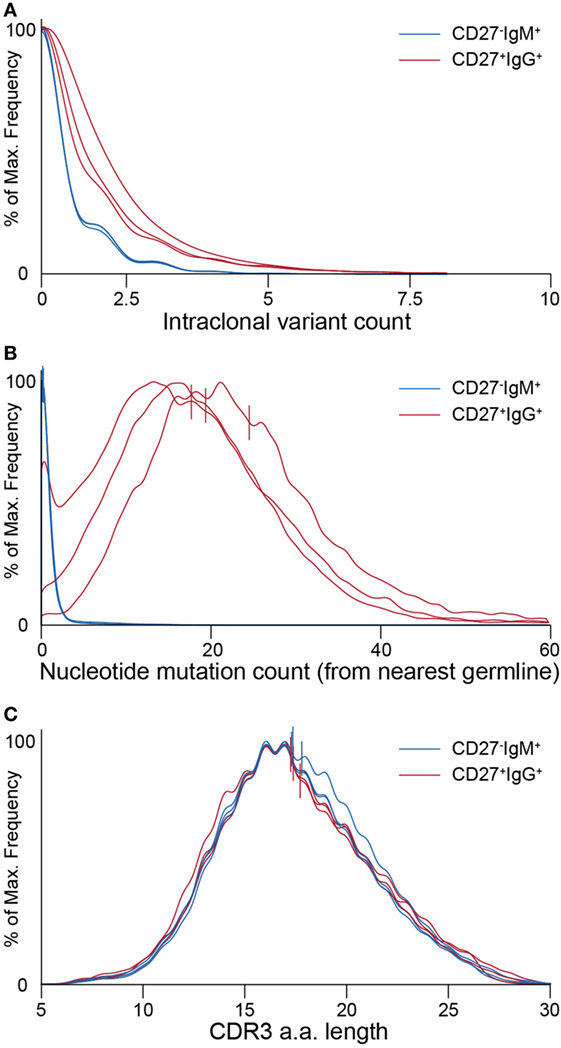
Figure 5. Ig-seq analysis of molecular features highlight global differences between naïve (CD27−IgM+) and memory (CD27+IgG+) B cells. (A) Clonotype size (calculated as the total number of variants within a clonotype) for naïve IgM (blue lines) and memory IgG (red lines) B cells. Each pair of lines represents a single donor. (B) Graph showing the distribution of average somatic hypermutation (SHM) frequencies for VDJ nt variants per clonotype. The CD27−IgM+ B cell repertoires (blue lines) have a median SHM value of 0, whereas only a small fraction of clonotypes (approximately 8%) contain an average of one or more mutations. CD27+IgG+ repertoires (red lines) have a median of 20 to 24 SHM per nt variant within each clonotype. (C) Complementarity determining region 3 (CDR3) a.a. length distribution across clonotypes from naïve (blue lines) and memory (red lines) B cell subsets. Vertical lines in panels (B,C) denote mean values.
Clonal Diversity Measurements of Human B Cell Repertoires After Error and Bias Correction
After establishing the value of performing MAF error correction on biological repertoires, we next focused on determining the clonotype diversity present in each B cell sample. First, we determined the overlap of clonotypes present in each cellular replicate (Figure 6A). Notably, we observed an overlap of several clonotypes in the CD27−IgM+ subset; this was unexpected given that this subset should be highly enriched for naïve B cells, which by definition are not antigen experienced or clonally expanded, and should, therefore, be mostly unique (not present in multiple replicates). For each donor in the CD27−IgM+ subset, 1–2% of all clonotypes were present in at least one other cellular replicate. In the CD27+IgG+ subset, clonotypes shared between at least two cellular replicates were nearly tenfold more frequent (12–15%), which was expected given that this population is comprised of antigen experienced, clonally expanded memory B cells. Another observation discordant with the expected naïve B cell properties of the CD27−IgM+ subset was that overlapping clonotypes (in donors 1 and 3) were significantly more likely to have acquired mutations (Figure 6B), which are not a typical feature of naïve B cells. In comparison, over 90% of all CD27+IgG+ (overlapping and non-overlapping) clonotypes possessed at least one SHM, an expected observation for a memory subset.
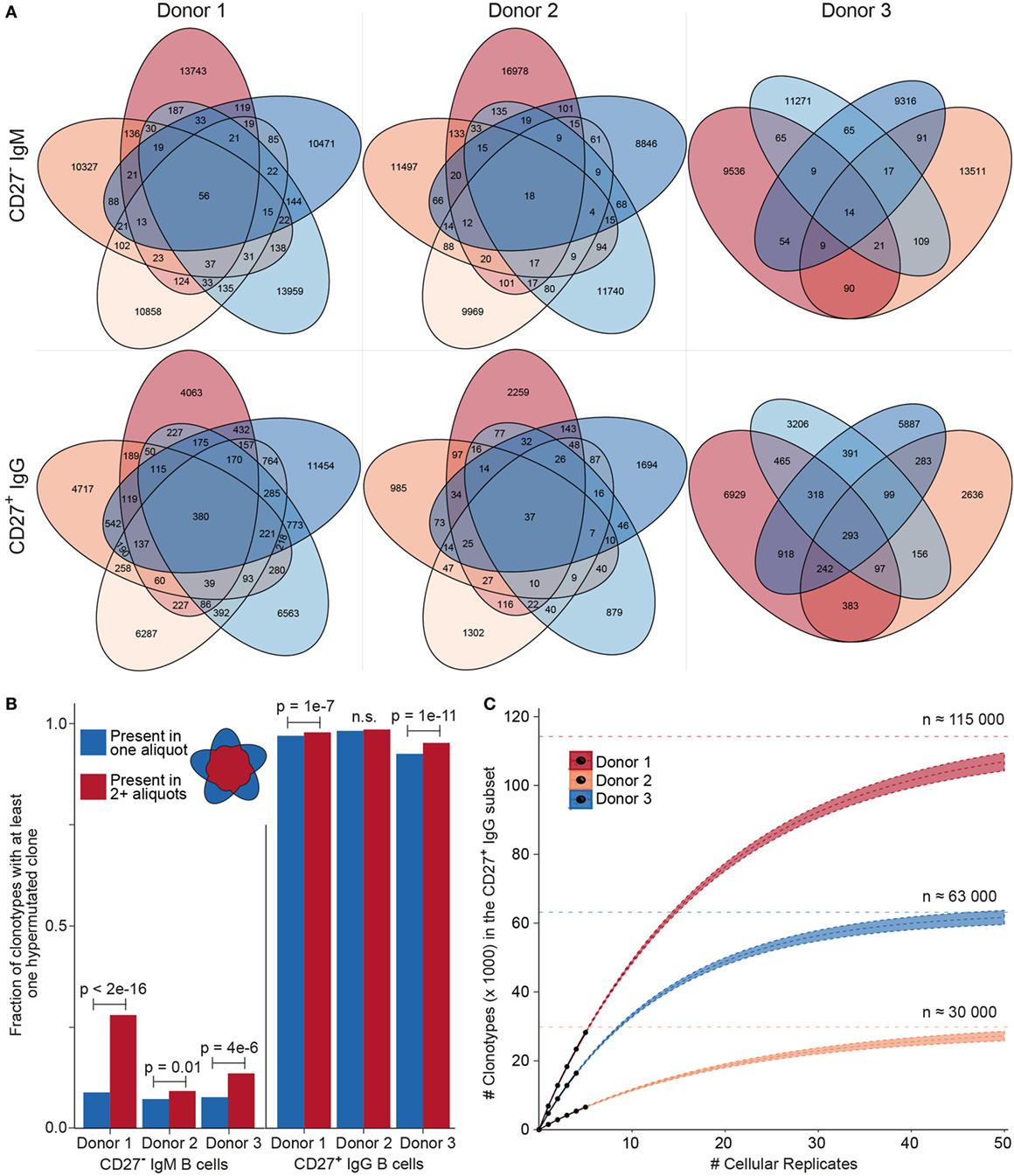
Figure 6. Clonotype diversity analysis across cellular replicates of naïve (CD27−IgM+) and memory (CD27+IgG+) B cells. (A) Venn diagrams show the presence of clonotypes [80% complementarity determining region 3 (CDR3) a.a. similarity to least one clone in the cluster, same CDR3 a.a. length, same IGHV, and IGHJ gene segment usage] across cellular replicates (2 × 105 cells each) from each donor B cell subset. (B) Bar graph showing the fraction of clonotypes containing at least one variant with at least one nt somatic hypermutation (SHM). Blue and red bars indicate clonotypes identified either in only one aliquot (unique) or in several aliquots (shared), respectively. The p-values represent significance using Fisher’s exact test. (C) Species accumulation curves for CD27+IgG+ B cells: the number of newly discovered clonotypes from each additional cellular replicate (black circles) is plotted. Extrapolating the observed overlap provides an estimate for the total number of distinct clonotypes (Chao2 estimator: D1 = 113,794 ± 1,701; D2 = 29,609 ± 958; D3 = 63,172 ± 1,176) and the approximate amount of cellular replicates needed to discover all clonotypes present in the peripheral blood CD27+ IgG+ population.
The high amount of overlap within the CD27+IgG+ B cell replicates of each donor allowed us to use established population diversity estimation techniques to calculate clonal diversity (31). Rarefaction curves were generated and estimates were extrapolated as a function of real and predicted cellular replicates (Figure 6C). The asymptote was determined by the standard form of the Chao2 estimator and yielded the following values for clonotype numbers: donor 1 = 113,794 ± 1,701, donor 2 = 29,609 ± 958, and donor 3 = 63,172 ± 1,176. Since the 95% confidence intervals for the three donors did not overlap, we could also infer that the size of each donor’s repertoire at the collection time point was significantly different. This analysis indicates that we would need to sample at least tenfold more cellular replicates in order to observe >90% of all clonotypes; however, the first five samples analyzed here were sufficient to observe >25% of the clonotypic memory repertoire. We also generated rarefaction and extrapolation curves rescaled to the RID count (Figures S3A,B in Supplementary Material). In the case of the CD27−IgM+ repertoire data, while asymptotic curves could be generated, a diversity estimation is impractical. This is because plotting the observed numbers of newly discovered clonotypes for each additional RID and donor shows that the number of newly discovered clonotypes in the CD27−IgM+ dataset continues to grow over the observed range, whereas the number of new clonotypes starts to converge at approximately 20,000 RIDs for CD27+IgG+ repertoires (Figure S3C in Supplementary Material).
Divergent Features of CD27−IgM+ and CD27+IgG+ Repertoires
After pooling all clonotypes (expanded and unique to a single cellular replicate) for each donor, we globally characterized sequences of the naïve CD27−IgM+ and memory CD27+IgG+ subsets. First, we determined the SHM count (nt) of each clone with respect to its nearest germline IGHV and IGHJ gene segment sequence. The median values of SHM for the CD27−IgM+ repertoires were 0, which was to be expected for a naïve B cell subset. In contrast, the median values for CD27+IgG+ repertoires were 20–24 mutations per clone (Figure 5B). It is widely appreciated that human heavy chain CDR3 sequences are much longer than their murine counterparts, which we also observed here, with a slight (but consistent across donors) variation between naïve and memory B cells (Figure 5C). Interestingly, the IGHV-gene segment family usage correlated with B cell subset. The CD27+IgG+ repertoires across all donors were relatively enriched for IGHV1 and IGHV3 gene segment family members, whereas the relative share of the IGHV4 gene segment family was larger in the CD27−IgM+ repertoires (Figure 7A). We validated these observations quantitatively using linear discriminant analysis (LDA) fitted on the centered log ratio-transformed frequencies of each cellular replicate (Figure S4 in Supplementary Material). The LDA classifier was fit on different splits of the data (based on two of the donors) and used to predict a holdout set (based on the remaining donor). This showed that the fitted classifier in each instance is highly predictive of the remaining aliquots and that prediction is robustly driven by the relative abundance of IGHV4 segment family usage in the CD27−IgM+ repertoires and the IGHV1, 2, and 3 families in the CD27+IgG+ repertoires (Figures S4A–C in Supplementary Material). Next, we utilized LDA to perform dimensionality reduction of all one-dimensional axis; again, the most important components were the relative abundance of IGHV3 and IGHV4 gene segment families (Figure S4D in Supplementary Material).
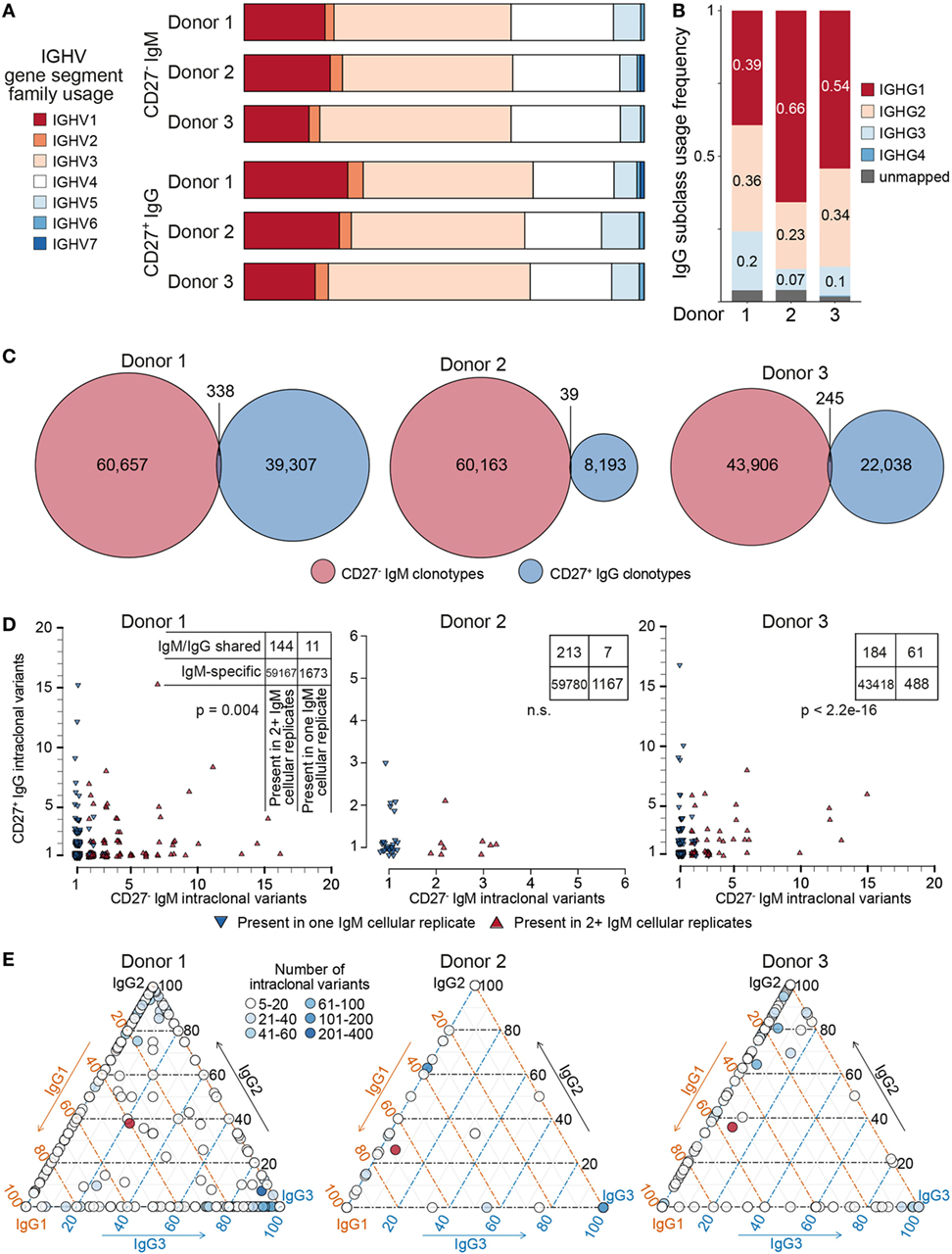
Figure 7. Genetic features of naïve and IgG memory BCR repertoires. (A) Bar graph depicting IGHV-gene segment usage sorted by family (color-coded) across donors and B cell subset; bars are normalized to the total number of clonotypes within each group. (B) IgG subclass usage in CD27+IgG+ donor repertoires. IgG4 sequences (dark blue bars) were virtually absent among three donors; a small fraction of sequences could not be unambiguously mapped based on the sequencing read (gray bars). (C) Venn diagrams showing the overlap of clonotypes (80% CDR-H3 amino acid similarity to least one clone in the cluster, same complementarity determining region 3 (CDR3) a.a. length, same IGHV and IGHJ gene segment usage) between the naïve (CD27−IgM+) and memory (CD27+IgG+) BCR heavy-chain repertoire in each of three donors. (D) Each plot shows the clonal composition of each shared clonotype [from panel (C)] in terms of its IgG and IgM intraclonal variants. Red triangles indicate clonotypes found in multiple IgM cellular aliquots; blue triangles show clonotypes which could only be found in one IgM cellular aliquot. The total number of clonotypes found are depicted in the corresponding contingency table. Fisher’s exact test was used to quantitatively analyze enrichment of expanded IgM clonotypes in the shared IgM/IgG subset. (E) Ternary plots comprised of three axes representing the IgG1, IgG2, and IgG3 isotype subclasses. The relative subclass composition of intraclonal variants per IgG clonotype (each represented by a circle colored according to the number of variants belonging to that clonotype) is depicted by the position of the circle within the triagonal space. Red circles represent the average subclass composition for all IgG variants of each donor as shown in panel (B).
Next, we leveraged the ability of our reverse primer to distinguish among IgG subclasses (Figure 7B). The majority of sequences mapped either to IgG1 (39–66%), IgG2 (23–36%), or IgG3 (1–7%), whereas IgG4 sequences were extremely rare, observed solely in donor 3 (0.3%). Finally, we compared the CD27−IgM+ and CD27+IgG+ repertoires of each donor to determine the clonotype overlap of each B cell subset and isotype. Strikingly, the observed overlap was very small, with only 338 shared clonotypes for donor 1, 39 shared clonotypes for donor 2, and 245 clonotypes for donor 3 (Figure 7C). In each donor, these represented less than 0.5% of identified clonotypes. Closer examination revealed that clonotypes shared between the CD27−IgM+ and CD27+IgG+ subsets were also significantly enriched for intraclonal variants (SHM in CDR3) in only one of the two populations (Figure 7D; Figure S5 in Supplementary Material). Furthermore, we could see that clonotypes with multiple IgM variants were also shared specifically among IgM cellular replicates (Figure 7D, contingency tables). This intraclonal variant bias was not limited to isotype: IgG clonotypes with ≥5 intraclonal variants also exhibited subclass composition skewing toward the IgG1/2 or IgG1/3 axis, but rarely at proportions similar to the overall IgG subclass distribution (Figure 7E).
Discussion
Ig-seq has become an essential tool for quantitative analysis of antibody repertoire diversity and distribution. However, similar to other areas of high-throughput sequencing, Ig-seq also suffers from technical errors and bias; thus, standardized experimental and analytical methods that increase the validity of immunological interpretations must be developed. Here, we have designed a comprehensive set of synthetic standards which, when combined with UID-labeling and MAF-based error and bias correction, produces highly accurate antibody repertoire data. By applying this approach to human B cell subsets, we gain unique insights into repertoire features such as clonal diversity, germline gene usage, SHM, and clonal history.
These synthetic standards, and the associated increase in analytical fidelity, enable quantitative interrogation of several accuracy-related features in Ig-seq. Critical to our hypothesis at the outset, raw uncorrected data indeed contains many erroneous variants, found both within the clonotype-defining CDR3 and across the entire VDJ region (Figures 3A,B). The number of false-positive variants correlated with the abundance of each standard; this is of particular concern because high frequency, clonally expanded B cells are often correlated with antigen specificity and thus important for biological interpretations (32). However, when applying our MAF error correction protocol, we were able to remove nearly all (94–97%); erroneous CDR3 and VDJ variants this correction was robust even for high-frequency standards where the number of erroneous variants was especially high. Errors not removed by MAF could potentially be addressed with more stringent filtering criteria (e.g., read number cutoffs); however, this may come at the cost of reducing overall dataset size and loss of legitimate intraclonal variants in biological samples.
Another aspect we quantified with our standard pool was the impact of multiplex primer sets, which has been shown to introduce substantial bias during library preparation (18, 33). By designing our standards with a 5′ conserved singleplex region (Figure 1A), we were able to directly compare Ig-seq data from libraries (on the same master stock) prepared by singleplex-PCR vs. multiplex-PCR. Our newly designed FR1-targeting multiplex primer set (Figures 2B,C) demonstrated a relatively strong correlation with singleplex-PCR data (R2 = 0.84) (Figure 3C). However, by performing multiple MAF error and bias correction steps, the correlation was improved by an additional 7% (Figure 3D). The remaining variability does not appear to be restricted to a particular IGHV-gene family, indicating there is little systematic bias with respect to homologous sequences within the standard pool. MAF, therefore, represents an essential step in eliminating technical artifacts from human Ig-seq workflows, as it is able to generate data that closely mirrors that of the original sample. In future applications, these synthetic standards could be a critical asset for evaluating newly designed primer sets, library preparation protocols, or implementing new error and bias correction pipelines.
Having established a comprehensive set of synthetic standards and a validated error and bias correction pipeline, we were able to perform several analyses on human B cell repertoires with greater confidence in the accuracy and quantitative resolution of the Ig-seq data. A simple approach to estimating repertoire diversity is to calculate the number of unique antibody sequences as a fraction of total transcript (cDNA) input. However, performing this analysis on our samples suggests that the CD27+IgG+ memory B cell compartment is significantly more diverse than the naïve CD27−IgM+ B cells (82% vs. 35% unique nt variants, respectively, averaged across donors and cellular aliquots, Student’s t-test p < 10−4). This is potentially due to sample size variability with respect to the number of transcripts and our ability to oversample smaller libraries. Critically, when using bulk-sorted cells with UID-labeling, it is not possible to discriminate between transcript copies that are identical because they came from the same lysed cell, and those which are identical because they represent two distinct, clonally related B cells. Thus, by biological subsampling through cellular replicates, we ensured that clonotypes observed in multiple samples must come from distinct, clonally related B cells, thereby providing an effective solution for estimating clonal diversity.
Applying computational approaches from ecology (31) to our biological subsampling strategy, we attempted to estimate the number of unique clonotypes in a given antibody repertoire. The CD27− IgM+ B-cell subset did not show substantial clonotype overlap across cellular aliquots (Figure 6A). As it is commonly assumed (and typically the case as shown in Figure 5A) that each newly generated B cell is a unique clone, the size of the naïve repertoire in the human peripheral blood would be equal to the total number of naïve B cells, in the range of 10–30 million. While it is improbable to sample this subset in its entirety, and its diversity is also too high to estimate based on the cell numbers obtained here, our observations are consistent with this model, since each additional cellular replicate produced overwhelmingly unique sequences. One donor did show an unexpected presence of overlapping sequences (shared clonotypes) across IgM cellular replicates (Figure 6A, donor 1); these clonotype sequences were significantly enriched for SHM (Figure 6B), suggesting the possible presence of an antigen-experienced B cell subset within the CD27−IgM+ population, and highlighting the need for improved characterization of the heterogeneity within circulating human B cell subsets. In the CD27+IgG+ B cell subset, we observed substantial overlap across cellular replicates, which was expected given that memory-enriched B cells would have experienced antigen and undergone clonal expansion. By extrapolating theoretical unique clonotypes with each subsequent cellular aliquot, we predict the size of the peripheral CD27+IgG+ B-cell clonotype repertoire to be on the order of 105 (Figure 6C). Indeed, rough estimates of the number of antigen-specific clonotypes generated by a single immune response [≈100, a number in line with what has been described regarding serum antibody clonotypes (34)] and the number of structurally distinct pathogens against which an individual has mounted a response [≈1,000, a generous estimate given work showing that worldwide, individuals have on average a serological history against less than 100 viral species (35)] suggest that a memory repertoire of this size could reasonably protect against latent infection and/or subsequent antigen encounter.
Many features of the heavy-chain repertoires profiled here were similar for both naïve and memory B cells. For example, CDR3 amino acid lengths were not significantly longer for naïve BCR sequences, although small differences of approximately one amino acid have previously been reported (1). However, we did observe a clear shift in IGHV segment family usage from the naïve to the memory BCR repertoire (Figure 7A). Consistently observing this reshaping in three independent healthy donors, and comparing to our standard controls to exclude the possibility of biased amplification, we can conclude that it is a genuine phenomenon. Relatively more abundant IGHV1 and less abundant IGHV4 segment usage in IgG memory B cells has been previously observed in one three-donor cohort (1) but not in another which pooled sequences from both class-switched and IgM-memory cells (36), underscoring the importance of experimental design and accurate bias correction in antibody repertoire analysis.
Our Ig-seq workflow also allowed us to unambiguously assign IgG antibody sequences to their appropriate subclass, offering further insight into patterns of class-switch recombination present in memory-enriched B cells. While plasma cell-secreted IgG proteins in human serum are present at ratios of approximately 14:8:1:1 [for IgG1:2:3:4, respectively (37)], CD27+IgG+ B cells showed a distribution of approximately 5:3:1 for IgG1:2:3, with a nearly complete absence of IgG4 (Figure 7B). Cole et al. similarly observed a lack of IgG4 heavy-chains in a single donor but described an enrichment of IgG2 relative to IgG1 and IgG3 (24). The abundance of IgG3+ B cells relative to its concentration in the serum seen here indicates IgG3 may play a more important role in maintaining the reactive memory response compared to the protective memory response provided by serum IgG. Notably, the IgG3 locus is the most proximal, and thereby the most plastic of the human IgG subclasses; that is, an IgG3+ B cell still retains the capacity to class-switch to any of the remaining three IgG subclasses, whereas IgG1, IgG2, and IgG4 cannot return to any of the previous states. Similar to these findings, a flow cytometry-based investigation has also found healthy human donors to have low frequencies of IgG4-expressing circulating memory B cells (38).
With new daily production and relatively rapid turnover of naïve B cells, it was not unexpected to see little overlap of clonotypes between the intradonor CD27−IgM+ and CD27+IgG+ populations (Figure 7C). An interesting finding was that for clonotypes present in both B cell subsets, intraclonal variation was largely restricted to one of the two isotypes. Assuming that clonotype overlap among CD27−IgM+ cellular replicates represents the presence of antigen experienced, clonally expanded B cells, this suggests that the antigen specificity of antibody variable domains may to some extent be influenced by the downstream constant regions, which has been observed functionally for small cohorts of human and murine IgG and IgA antibodies (39). Notably, we also observe similar clonal restriction within IgG clonotypes with respect to heavy-chain subclass (Figure 7E). This may be driven by the type of antigen and the nature of the elicited immune response, or governed by physical constraints as we suggest for the differences between the IgM and IgG repertoires. A larger-scale functional study, including IgM sequences, could provide crucial support for this model, which would shed new light on the role of Ig isotypes and subclasses on B cells in the post-antigen encounter setting.
Methods
Preparation of Spike-In Master Stocks
The spike-in standards were ordered from GeneArt (Invitrogen) in the form of plasmids. Each spike-in sequence contained a T7 promoter for in vitro transcription. Approximately 1.5 µg of each plasmid was digested with 10 U of EcoRV-HF (New England BioLabs) and purified with DNA-binding magnetic beads (SPRI-select, Beckman Coulter). Approximately, 1 µg of the digested plasmid was then used for in vitro transcription (MEGAscript T7 Transcription Kit, ThermoFisher Scientific). RNA was purified by lithium-chloride precipitation, eluted (TE with 1 U/μl RiboLock) and aliquoted. The final concentration was then determined with the TapeStation (Agilent Technologies).
The spike-in RNA obtained this way was reverse transcribed with the Maxima Reverse Transcriptase kit (Thermofisher Scientific). 500 ng mRNA was mixed with 20 pmol of IgM reverse primer and 3 µl dNTP-mix (10 mM each) and was then filled up to 14.5 µl with water. The reaction-mix was incubated at 65°C for 5 min. 4 µl of 5× RT-buffer, 0.5 µl (20 U) RiboLock, and 1 µl Maxima reverse transcriptase (200 U) were then added. The resulting reaction-mix was incubated for 35 min at 55°C, followed by a termination step at 85°C for 5 min. 2.5 µl of RNase A (Thermofisher Scientific) was added and the mix was again incubated at 60°C for 30 min. The resulting cDNA was purified with SPRI-Select magnetic beads and eluted in nuclease-free water. The concentration of each cDNA reaction was determined afterward with the Fragment Analyzer and pooled according to Table S1 in Supplementary Material. The exact concentration of the pooled spike-ins was determined by ddPCR with dilutions of the pool ranging from 10−3 to 10−6. The measured spike-in pool was afterward diluted to a final storage concentration of 250,000 transcripts per microliter.
Transcript Quantitation by ddPCR
Quantifiying cDNA and PCR products by ddPCR was conducted for all measurements in the following way: A dilution series with 3 or 4 points was prepared. Droplets were generated with BioRad’s droplet generator using 12.25 µl of ddPCR Supermix (BioRad) combined with 10 µl of the diluted sample, 25 pmol of the biological ddPCR probe (SF_21), 25 pmol of the spike-in specific ddPCR probe (TAK_499), 22.5 pmol of the forward (SF_63) and reverse ddPCR primer (TAK_522) and 55 µl of droplet generation oil (BioRad). Droplets were then transferred to a 96-well reaction plate, which was heat sealed with easy pierce foil (VWR International). Then a PCR reaction was performed using the following conditions: 95°C for 10 min; 45 cycles of 94°C for 30 s, 53°C for 30 s, 64°C for 1 min; 98°C for 10 min; and holding at 4°C. After the PCR step, every 96-well plate was read using BioRad’s droplet reader.
B-Cell Isolation, Sorting, and Lysis
Peripheral blood leukocyte-enriched fractions (“buffy coats”) were received from the Bern (Switzerland) blood donation center (project reference: P_223) after obtaining written informed consent from healthy human donors in accordance with the Declaration of Helsinki. Blood samples were diluted 1:3 with sterile PBS and overlaid on Ficoll-Paque PLUS (GE Healthcare) using LeukoSep conical centrifuge tubes (Greiner Bio-One). Peripheral blood mononuclear cells (PBMCs) were harvested after separation for 30 min at 400 × g without braking. Successive centrifugation steps were performed to wash the mononuclear cell fraction and remove residual neutrophils and granulocytes. Total B cells were isolated from PBMCs by negative selection with the EasySep Human B Cell Enrichment Kit (STEMCELL Technologies) according to the manufacturer’s instructions. The following fluorescently labeled antibodies were used to stain the enriched B-cell fraction prior to sorting by flow cytometry: anti-CD3-APC/Cy7 (clone HIT3a BioLegend #300318), anti-CD14-APC/Cy7 (clone HCD14 BioLegend #325620), anti-CD16-APC/Cy7 (clone 3G8 BioLegend #302018), anti-CD19-BV785 (clone HIB19, BioLegend #302240), anti-CD20-BV650 (clone 2H7, BioLegend #302336), anti-CD27-V450 (clone M-T271, BD Horizon #560448), anti-CD24-BV510 (clone ML5, BioLegend #311126), anti-CD38-PC5 (clone LS198-4-3, Beckman Coulter #A07780), anti-IgD-PEcy7 (clone IA6-2, BioLegend #348210), anti-IgG-Alexa Fluor 647 (Jackson ImmunoResearch #109-606-003) anti-IgA-Alexa Fluor 488 (Jackson ImmunoResearch #109-549-011), and anti-IgM-PE (clone SA-DA4, eBioscience #12-9998). Cell sorting was performed on a BD FACS Aria III following the gating strategy depicted in Figure 4A. After sorting, isolated fractions were centrifuged 5 min at 300 × g, the supernatants were aspirated, and the cell pellets were re-suspended in 1 ml PBS. Recovered cells were hand-counted using a Neubauer hemocytometer, and aliquots containing equal numbers of cells were prepared from the cellular suspension. These aliquots were centrifuged, and the supernatant aspirated. Cell pellets were lysed directly in 200 µl TRI Reagent (Sigma), allowed to dissociate for 5 min at room temperature, then frozen on dry ice prior to storage at −80°C.
RNA Isolation From Sorted B Cell Populations
Immediately prior to use, phase lock gel tubes were pelleted at 12,000–16,000× g in a microcentrifuge for 20–30 s. Each TRIzol aliquot was then thawed on ice. After thawing and an incubation time of 5 min at room temperature, 1 ml of the TRIzol homogenate was transferred to the phase lock tube. 0.2 ml chloroform was added and the tube was shaken vigorously by hand (~15 s). After an incubation time of 3 min, the phase lock tube was centrifuged at 12,000 × g during 15 min at 4°C. The resulting upper aqueous phase was transferred to a fresh Eppendorf tube, an equal volume of 70% ethanol was added and the solution was purified on a PureLink RNA column according to the manufacturer’s instructions (Life Technologies). Finally, RNA was eluted in 25 µl nuclease-free water.
Library Preparation and Next-Generation Sequencing on Illumina’s MiSeq
First-strand cDNA synthesis was carried out using Maxima reverse transcriptase (Life Technologies). The protocol for one reaction is as follows: A 29-µl reaction-mix was prepared using up to 2 µg of RNA together with 40 pmol of the respective gene-specific reverse primer [for IgG sequences, 5′-RID-GTTCTGGGAAGTAGTCCTTGACCAG-3′ (IgH_10r); for IgM, 5′-RID-ACGAGGGGGAAAAGGGTTGG-3′ (CH1_1r)], 2 µl dNTP (10 mM each) and the required amount of nuclease-free water. This mix is incubated for 5 min at 65°C. A master mix of 8 µl 5× RT-buffer, 1 µl of (20 U) RiboLock, and 2 µl of Maxima reverse transcriptase is then prepared and added to the reaction-mix. Finally, the mix is incubated for 30 min at 50°C and the reaction is terminated by incubating at 85°C for 5 min.
The obtained cDNA is then cleaned with a left-sided SPRI-Select bead clean-up (0.8×) according to the manufacturer’s instructions (Beckman coulter) and subsequently measured by ddPCR.
Up to 135,000 cDNA transcripts were then pooled together with 12,500 spike-in transcripts. Multiplex-PCR was performed using an equimolar pool of the forward primer mix (Figure 2B) and the reverse primer (TAK_423) targeting the overhang introduced during cDNA synthesis. Due to low cDNA yields, the first PCR was carried out for 20 cycles and the following cycling protocol: 2 min at 95°C; 20 cycles of 98°C for 20 s; 60°C for 50; 72°C for 1 min; and 72°C and then holding at 4°C. PCR reactions were prepared using 15 µl of Kapa HiFi HotStart ReadyMix (KAPA Biosystems), 50 pmol of the forward mix and the reverse primer and 9 µl of the cDNA mix. After the first PCR, we again performed a left-sided bead clean-up (0.8×) and measured the PCR product concentration using ddPCR. We use 800,000 transcripts from PCR 1 as input into the adapter extension PCR. For this PCR 25 µl of Kapa HiFI Hotstart ReadyMix was combined with 25 pmol of the forward primer (TAK_424) and 25 pmol of the index primer (TAK_531) as well as the diluted PCR product. Finally, the reaction volume was adjusted to 50 µl by the addition of nuclease-free water. Thermocycling was performed as follows: 95°C for 5 min; 23 cycles of 98°C for 20 s, 65°C for 15 s, 72°C for 15 s; 72°C for 5 min; and 4°C indefinitely. Following second-step adapter extension PCR, reactions were cleaned using a double-sided SPRIselect bead clean-up process (0.5×–0.8×), with an additional ethanol wash and elution in TE buffer.
Libraries were then quantified by capillary electrophoresis (Fragment analyzer, Agilent). After quantitation, libraries were pooled accordingly and sequenced on a MiSeq System (Illumina) with the paired-end 2× 300 bp kit.
Bioinformatic Pipeline
Paired-end fastq files were merged using PandaSeq (40). Afterward, sequences were filtered for quality and length using the FASTX toolkit (http://hannonlab.cshl.edu/fastx_toolkit/). After the quality trim, sequences were processed with a custom Python script that performed error correction by consensus building on our sequences and RIDs. In order to utilize as many sequencing reads as possible, we required UIDs to have at least three reads, but did not remove sequences that only had one UID group mapping to them. VDJ annotation and frequency calculation was then performed by our in-house aligner (18), which was updated with the human reference database downloaded from IMGT. The complete error correction and alignment pipeline is available at https://gitlab.ethz.ch/reddy/MAF.
Statistical Analysis
All statistical and computational analyses following the alignment step were performed in R.
Details about specific tests that were used can be found in the results section and in the figure legends. Scripts are available upon request.
Data Availability
In adherence to the data sharing recommendations of the AIRR community (41), our data are publically available in the following repositories: BioProject, BioSample, SRA, and GenBank and can be accessed under the accession number PRJNA430091 (BioProject). The exact data processing steps, including software tools and version numbers, can be found on zenodo.org under the following 10.5281/zenodo.1201416.
Likewise, the designed spike-in sequences are also stored on GenBank (accession numbers MG785894-MG785978).
Author Contributions
JL, SF, ET, and SR designed experiments. VC performed B-cell enrichment, sorting, and mRNA extraction. MI and SF prepared IgH libraries. JL designed primer sequences. JL and AZ designed antibody spike-ins. EM, AZ, SM, and MI conducted preliminary experiments and data analysis. SF was responsible for the bioinformatics pipeline.
Conflict of Interest Statement
The authors declare that the research was conducted in the absence of any commercial or financial relationships that could be construed as a potential conflict of interest.
Acknowledgments
The authors would like to acknowledge the Genomics Facility Basel of ETH Zurich for Illumina sequencing support, in particular E. Burcklen, K. Eschbach, and C. Beisel. The authors also want to thank H. Ruscheweyh for bioinformatic code support.
Funding
This was work was funded by Swiss National Science Foundation SystemsX.ch AntibodyX RTD project (to SR); the professorship of SR is made possible by the generous endowment of the S. Leslie Misrock Foundation.
Supplementary Material
The Supplementary Material for this article can be found online at https://www.frontiersin.org/articles/10.3389/fimmu.2018.01401/full#supplementary-material.
References
1. Wu Y-C, Kipling D, Leong HS, Martin V, Ademokun AA, Dunn-Walters DK. High-throughput immunoglobulin repertoire analysis distinguishes between human IgM memory and switched memory B-cell populations. Blood (2010) 116(7):1070–8. doi:10.1182/blood-2010-03-275859
2. Arnaout R, Lee W, Cahill P, Honan T, Sparrow T, Weiand M, et al. High-resolution description of antibody heavy-chain repertoires in humans. PLoS One (2011) 6(8):e22365. doi:10.1371/journal.pone.0022365
3. DeKosky BJ, Kojima T, Rodin A, Charab W, Ippolito GC, Ellington AD, et al. In-depth determination and analysis of the human paired heavy- and light-chain antibody repertoire. Nat Med (2015) 21(1):86–91. doi:10.1038/nm.3743
4. Robinson WH. Sequencing the functional antibody repertoire – diagnostic and therapeutic discovery. Nat Rev Rheumatol (2015) 11(3):171–82. doi:10.1038/nrrheum.2014.220
5. Williams LD, Ofek G, Schätzle S, McDaniel JR, Lu X, Nicely NI, et al. Potent and broad HIV-neutralizing antibodies in memory B cells and plasma. Sci Immunol (2017) 2(7):eaal2200. doi:10.1126/sciimmunol.aal2200
6. Doria-Rose NA, Schramm CA, Gorman J, Moore PL, Bhiman JN, DeKosky BJ, et al. Developmental pathway for potent V1V2-directed HIV-neutralizing antibodies. Nature (2014) 509(7498):55–62. doi:10.1038/nature13036
7. Zhu J, Ofek G, Yang Y, Zhang B, Louder MK, Lu G, et al. Mining the antibodyome for HIV-1-neutralizing antibodies with next-generation sequencing and phylogenetic pairing of heavy/light chains. Proc Natl Acad Sci U S A (2013) 110(16):6470–5. doi:10.1073/pnas.1219320110
8. Lavinder JJ, Wine Y, Giesecke C, Ippolito GC, Horton AP, Lungu OI, et al. Identification and characterization of the constituent human serum antibodies elicited by vaccination. Proc Natl Acad Sci U S A (2014) 111(6):2259–64. doi:10.1073/pnas.1317793111
9. Jiang N, He J, Weinstein JA, Penland L, Sasaki S, He X-S, et al. Lineage structure of the human antibody repertoire in response to influenza vaccination. Sci Transl Med (2013) 5(171):171ra19. doi:10.1126/scitranslmed.3004794
10. Roskin KM, Simchoni N, Liu Y, Lee J-Y, Seo K, Hoh RA, et al. IgH sequences in common variable immune deficiency reveal altered B cell development and selection. Sci Transl Med (2015) 7(302):302ra135. doi:10.1126/scitranslmed.aab1216
11. Palanichamy A, Apeltsin L, Kuo TC, Sirota M, Wang S, Pitts SJ, et al. Immunoglobulin class-switched B cells form an active immune axis between CNS and periphery in multiple sclerosis. Sci Transl Med (2014) 6(248):248ra106. doi:10.1126/scitranslmed.3008930
12. Meng W, Zhang B, Schwartz GW, Rosenfeld AM, Ren D, Thome JJC, et al. An atlas of B-cell clonal distribution in the human body. Nat Biotechnol (2017) 35(9):879–84. doi:10.1038/nbt.3942
13. Lee YN, Frugoni F, Dobbs K, Tirosh I, Du L, Ververs FA, et al. Characterization of T and B cell repertoire diversity in patients with RAG deficiency. Sci Immunol (2016) 1(6):eaah6109. doi:10.1126/sciimmunol.aah6109
14. Friedensohn S, Khan TA, Reddy ST. Advanced methodologies in high-throughput sequencing of immune repertoires. Trends Biotechnol (2017) 35(3):203–14. doi:10.1016/j.tibtech.2016.09.010
15. Georgiou G, Ippolito GC, Beausang J, Busse CE, Wardemann H, Quake SR. The promise and challenge of high-throughput sequencing of the antibody repertoire. Nat Biotechnol (2014) 32(2):158–68. doi:10.1038/nbt.2782
16. Jiang L, Schlesinger F, Davis CA, Zhang Y, Li R, Salit M, et al. Synthetic spike-in standards for RNA-seq experiments. Genome Res (2011) 21(9):1543–51. doi:10.1101/gr.121095.111
17. Shugay M, Britanova OV, Merzlyak EM, Turchaninova MA, Mamedov IZ, Tuganbaev TR, et al. Towards error-free profiling of immune repertoires. Nat Methods (2014) 11(6):653–5. doi:10.1038/nmeth.2960
18. Khan TA, Friedensohn S, Gorter de Vries AR, Straszewski J, Ruscheweyh H-J, Reddy ST. Accurate and predictive antibody repertoire profiling by molecular amplification fingerprinting. Sci Adv (2016) 2(3):e1501371. doi:10.1126/sciadv.1501371
19. Kinde I, Wu J, Papadopoulos N, Kinzler KW, Vogelstein B. Detection and quantification of rare mutations with massively parallel sequencing. Proc Natl Acad Sci U S A (2011) 108(23):9530–5. doi:10.1073/pnas.1105422108
20. Shiroguchi K, Jia TZ, Sims PA, Xie XS. Digital RNA sequencing minimizes sequence-dependent bias and amplification noise with optimized single-molecule barcodes. Proc Natl Acad Sci U S A (2012) 109(4):1347–52. doi:10.1073/pnas.1118018109
21. Kivioja T, Vähärautio A, Karlsson K, Bonke M, Enge M, Linnarsson S, et al. Counting absolute numbers of molecules using unique molecular identifiers. Nat Methods (2011) 9(1):72–4. doi:10.1038/nmeth.1778
22. Vollmers C, Sit RV, Weinstein JA, Dekker CL, Quake SR. Genetic measurement of memory B-cell recall using antibody repertoire sequencing. Proc Natl Acad Sci U S A (2013) 110(33):13463–8. doi:10.1073/pnas.1312146110
23. Turchaninova MA, Davydov A, Britanova OV, Shugay M, Bikos V, Egorov ES, et al. High-quality full-length immunoglobulin profiling with unique molecular barcoding. Nat Protoc (2016) 11(9):1599–616. doi:10.1038/nprot.2016.093
24. Cole C, Volden R, Dharmadhikari S, Scelfo-Dalbey C, Vollmers C. Highly accurate sequencing of full-length immune repertoire amplicons using Tn5-enabled and molecular identifier-guided amplicon assembly. J Immunology (2016) 196(6):2902–7. doi:10.4049/jimmunol.1502563
25. Lefranc M-P, Giudicelli V, Duroux P, Jabado-Michaloud J, Folch G, Aouinti S, et al. IMGT®, the international ImMunoGeneTics information system® 25 years on. Nucleic Acids Res (2015) 43(Database issue):D413–22. doi:10.1093/nar/gku1056
26. Wang C, Liu Y, Xu LT, Jackson KJL, Roskin KM, Pham TD, et al. Effects of aging, cytomegalovirus infection, and EBV infection on human B cell repertoires. J Immunol (2014) 192(2):603–11. doi:10.4049/jimmunol.1301384
27. Menzel U, Greiff V, Khan TA, Haessler U, Hellmann I, Friedensohn S, et al. Comprehensive evaluation and optimization of amplicon library preparation methods for high-throughput antibody sequencing. PLoS One (2014) 9(5):e96727. doi:10.1371/journal.pone.0096727
28. Greiff V, Menzel U, Haessler U, Cook SC, Friedensohn S, Khan TA, et al. Quantitative assessment of the robustness of next-generation sequencing of antibody variable gene repertoires from immunized mice. BMC Immunol (2014) 15(1):40. doi:10.1186/s12865-014-0040-5
29. Laserson U, Vigneault F, Gadala-Maria D, Yaari G, Uduman M, Vander Heiden JA, et al. High-resolution antibody dynamics of vaccine-induced immuneresponses. Proc Natl Acad Sci U S A (2014) 111(13):4928–33. doi:10.1073/pnas.1323862111
30. Greiff V, Miho E, Menzel U, Reddy ST. Bioinformatic and statistical analysis of adaptive immune repertoires. Trends Immunol (2015) 36(11):738–49. doi:10.1016/j.it.2015.09.006
31. Colwell RK, Chao A, Gotelli NJ, Lin SY, Mao CX, Chazdon RL, et al. Models and estimators linking individual-based and sample-based rarefaction, extrapolation and comparison of assemblages. J Plant Ecol (2012) 5(1):3–21. doi:10.1093/jpe/rtr044
32. Reddy ST, Ge X, Miklos AE, Hughes RA, Kang SH, Hoi KH, et al. Monoclonal antibodies isolated without screening by analyzing the variable-gene repertoire of plasma cells. Nat Biotechnol (2010) 28(9):965–9. doi:10.1038/nbt.1673
33. Carlson CS, Emerson RO, Sherwood AM, Desmarais C, Chung M-W, Parsons JM, et al. Using synthetic templates to design an unbiased multiplex PCR assay. Nat Commun (2013) 4:2680. doi:10.1038/ncomms3680
34. Lee J, Boutz DR, Chromikova V, Joyce MG, Vollmers C, Leung K, et al. Molecular-level analysis of the serum antibody repertoire in young adults before and after seasonal influenza vaccination. Nat Med (2016) 22(12):1456–64. doi:10.1038/nm.4224
35. Xu GJ, Kula T, Xu Q, Li MZ, Vernon SD, Ndung’u T, et al. Viral immunology. Comprehensive serological profiling of human populations using a synthetic human virome. Science (2015) 348(6239):aaa0698. doi:10.1126/science.aaa0698
36. DeWitt WS, Lindau P, Snyder TM, Sherwood AM, Vignali M, Carlson CS, et al. A public database of memory and naive B-cell receptor sequences. PLoS One (2016) 11(8):e0160853. doi:10.1371/journal.pone.0160853
37. Vidarsson G, Dekkers G, Rispens T. IgG subclasses and allotypes: from structure to effector functions. Front Immunol (2014) 5(16):520. doi:10.3389/fimmu.2014.00520
38. Heeringa JJ, Karim AF, van Laar JAM, Verdijk RM, Paridaens D, van Hagen PM, et al. Expansion of blood IgG4+ B, TH2, and regulatory T cells in patients with IgG4-related disease. J Allergy Clin Immunol (2018) 141(5):1831–43 e10. doi:10.1016/j.jaci.2017.07.024
39. Torres M, Casadevall A. The immunoglobulin constant region contributes to affinity and specificity. Trends Immunol (2008) 29(2):91–7. doi:10.1016/j.it.2007.11.004
40. Masella AP, Bartram AK, Truszkowski JM, Brown DG, Neufeld JD. PANDAseq: paired-end assembler for illumina sequences. BMC Bioinformatics (2012) 13(1):31. doi:10.1186/1471-2105-13-31
Keywords: antibody repertoire, next-generation sequencing, unique molecular identifiers, B cells, bioinformatics, systems immunology
Citation: Friedensohn S, Lindner JM, Cornacchione V, Iazeolla M, Miho E, Zingg A, Meng S, Traggiai E and Reddy ST (2018) Synthetic Standards Combined With Error and Bias Correction Improve the Accuracy and Quantitative Resolution of Antibody Repertoire Sequencing in Human Naïve and Memory B Cells. Front. Immunol. 9:1401. doi: 10.3389/fimmu.2018.01401
Received: 19 March 2018; Accepted: 06 June 2018;
Published: 20 June 2018
Edited by:
Harry W. Schroeder, University of Alabama at Birmingham, United StatesReviewed by:
Paolo Casali, University of Texas Health Science Center San Antonio, United StatesClaude-Agnes Reynaud, Institut National de la Santé et de la Recherche Médicale (INSERM), France
Copyright: © 2018 Friedensohn, Lindner, Cornacchione, Iazeolla, Miho, Zingg, Meng, Traggiai and Reddy. This is an open-access article distributed under the terms of the Creative Commons Attribution License (CC BY). The use, distribution or reproduction in other forums is permitted, provided the original author(s) and the copyright owner are credited and that the original publication in this journal is cited, in accordance with accepted academic practice. No use, distribution or reproduction is permitted which does not comply with these terms.
*Correspondence: Elisabetta Traggiai, elisabetta.traggiai@novartis.com;
Sai T. Reddy, sai.reddy@ethz.ch
†These authors have contributed equally to this work.