- Division of Immunology and Allergy, Department of Medicine Solna, Karolinska Institutet, Karolinska University Hospital Solna, Stockholm, Sweden
Emerging viruses have become increasingly important with recurrent epidemics. Influenza A virus (IAV), a respiratory virus displaying continuous re-emergence, contributes significantly to global morbidity and mortality, especially in young children, immunocompromised, and elderly people. IAV infection is typically confined to the airways and the virus replicates in respiratory epithelial cells but can also infect resident immune cells. Clearance of infection requires virus-specific adaptive immune responses that depend on early and efficient innate immune responses against IAV. Mononuclear phagocytes (MNPs), comprising monocytes, dendritic cells, and macrophages, have common but also unique features. In addition to being professional antigen-presenting cells, MNPs mediate leukocyte recruitment, sense and phagocytose pathogens, regulate inflammation, and shape immune responses. The immune protection mediated by MNPs can be compromised during IAV infection when the cells are also targeted by the virus, leading to impaired cytokine responses and altered interactions with other immune cells. Furthermore, it is becoming increasingly clear that immune cells differ depending on their anatomical location and that it is important to study them where they are expected to exert their function. Defining tissue-resident MNP distribution, phenotype, and function during acute and convalescent human IAV infection can offer valuable insights into understanding how MNPs maintain the fine balance required to protect against infections that the cells are themselves susceptible to. In this review, we delineate the role of MNPs in the human respiratory tract during IAV infection both in mediating immune protection and as targets of the virus.
Introduction
Emerging viruses including influenza viruses, contribute significantly to human morbidity and mortality. Influenza is one of the oldest diseases known to mankind, with historical reports of influenza outbreaks dating as far back as 1173 (1). Still, influenza viruses are considered emerging/re-emerging viruses due to their capacity to dramatically change and cause epidemics with high mortality rate (2–5).
There are two forms of influenza: seasonal and pandemic. Seasonal influenza epidemics are caused by influenza A and B viruses and seasonal strains undergo mutations referred to as antigenic drift. For influenza A viruses (IAVs) antigenic drift is typically more pronounced each season, while it is more gradual for influenza B (6–9). Seasonal influenza epidemics contribute heavily to global disease burden and to deaths associated with lower respiratory tract (LRT) infections. 3–5 million cases of severe illness and 290–650,000 deaths annually are estimated, especially in young children, immunocompromised, and elderly people (10–13). The clinical picture of IAV infection is broad, ranging from mild/no symptoms, to viral pneumonia, severe respiratory failure, or acute respiratory distress syndrome. IAV infection results in increased susceptibility to secondary bacterial infections, which also contribute to mortality (14–16). In addition, circulating IAV strains can, at unpredictable intervals, cause influenza pandemics when the virus undergoes more dramatic genetic changes known as antigenic shift. Four pandemics have occurred in the past century: the 1918 Spanish flu, the 1957 Asian flu, the 1968 Hong Kong flu, and the 2009 Swine flu. Influenza pandemics are usually characterized by higher mortality than seasonal epidemics, often in age groups that are not typically at risk for influenza infections (17–23).
The nature and severity of influenza disease are influenced by the properties of the virus, host genetics, pre-existing immunity, and the immune response generated to varying extents—their relative contributions remaining incompletely understood (24–30). Highly pathogenic strains, like the Spanish flu, induce massive immune responses, suggesting that too potent antiviral immune responses are pathogenic rather than protective and that immunopathology is central in influenza (19, 31–37). Still, robust immune responses against IAV are required to control and clear infection (38–40). Mononuclear phagocytes (MNPs)— monocytes, dendritic cells (DCs), and macrophages (Mϕ)—are important in IAV infection as they are capable of limiting virus release; sensing and phagocytosing pathogens; clearing virus and apoptotic cells; releasing cytokines to mediate inflammation; directing leukocyte traffic via chemokine release; processing and presenting viral antigens; and finally activating naïve T cells (41–49).
The distribution and function of immune cells, including MNPs, differ between anatomical compartments (50–53). However, the exact nature of MNP involvement in human IAV infection remains largely unclear. Sampling the human respiratory tract in patients during ongoing infection poses significant challenges of accessibility and risk of causing further injury to the mucosal barrier. Defining human respiratory MNP distribution, phenotype, and function during IAV infection can therefore offer valuable insights into understanding how the immune system maintains the fine balance required to protect against infections. In this review, we will summarize insights on the role of MNPs in the human respiratory tract during IAV infection both in mediating immune protection and as targets of the virus.
Human Respiratory MNPs
The human respiratory tract encompasses a large mucosal surface with the densest vasculature of all organ systems, that is constantly exposed to the external environment with every inhalation (54, 55). MNPs are positioned along the respiratory tree, in anticipation of exposure to foreign material and respiratory pathogens. MNPs are dually tasked with both promoting inflammation and maintaining tolerance, without disrupting the mucosal barrier that separates the air-filled alveolar spaces from sterile blood in the capillaries (56). A detailed understanding of the distribution and function of respiratory MNPs from the nasal cavities to the alveoli is essential, yet currently incomplete, largely due to the challenges of accessing these tissues in humans. However, recent studies have generated important insight in this area and Mϕs, monocytes, monocyte-derived DCs (mo-DCs), and bona fide DC subsets have been identified from healthy human respiratory tissues. Figure 1 summarizes the current understanding of the phenotype and distribution of human MNP subsets in respiratory tissues at steady state, as reported (41, 51, 52, 57–62).
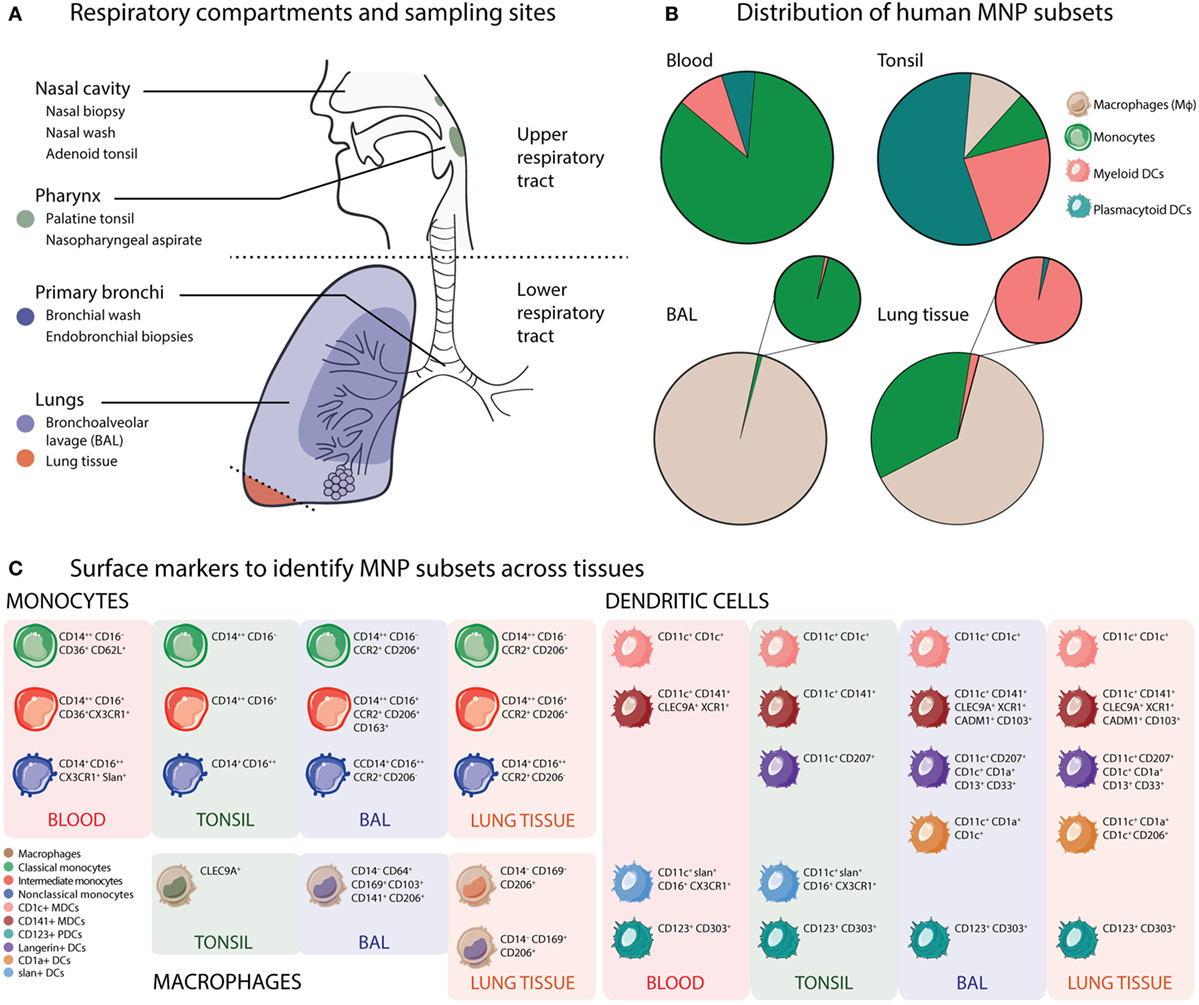
Figure 1. Mononuclear phagocyte (MNP) phenotype and distribution vary across human respiratory compartments. (A) Respiratory compartments and sampling sites. In the human upper respiratory tract, the initial site of influenza A virus infection, immune cells including macrophage (Mϕ), monocyte, and dendritic cell (DC) subsets from the nasal cavity and sinuses can be collected with nasal biopsies or nasal wash sampling. Along with pharyngeal palatine tonsils (and tubal and lingual tonsils), the adenoids form the Waldeyer’s ring, an anatomical structure comprising a ring of lymphoid tissue guarding the pharynx. In the lower respiratory tract, bronchoscopy allows sampling of discrete regions of the airways and lungs. Bronchial washes can be used to sample the cells lining the bronchi and bronchioles. Endobronchial biopsies can also be obtained from the mucosal tissue of the bronchial walls. Bronchoalveolar lavages (BALs) sample the most distal airways and alveolar sacs. Finally, lung resection samples allow sampling of lung parenchyma and tissue-resident immune cells. (B) Distribution of human MNP subsets. Pie charts illustrate broadly pooled data from 21 published studies on human MNP subset distribution in blood, tonsils, BAL, and lung tissue to demonstrate the differential distribution of MNPs across anatomical compartments reported from many research groups (51, 52, 57–61, 63–76). As different studies utilize different strategies to specifically define MNPs, the pie charts show groups of cells typically including several subsets of cells: Mϕs (beige), monocytes (green), myeloid DCs (MDCs) (coral), and plasmacytoid DCs (PDCs) (teal). (C) Surface markers to identify MNP subsets across human tissues. The various MNP subsets across tissues can be identified using flow cytometry from HLA-DR+ leukocytes that do not express lineage (T cells, B cells, NK cells, and granulocytes) markers. Apart from CD123+ PDCs, the MNP subsets express different levels of the myeloid marker CD11c. Mϕs have been studied in detail in both BAL and lung tissue, where CD169 expression distinguished alveolar from interstitial Mϕs. Monocyte subsets can be identified from most tissues based on relative expression of CD14 and CD16, as first defined in blood. The major MDC subsets are defined by expression of CD1c or CD141. The extended MDC subsets are now distinguished by expression of CD207 (langerin), CD1a, or slan (51, 52, 57–61, 63–76).
Alveolar macrophages (AMϕs) are the most abundant phagocytes of the human lungs, responsible for internalizing inhaled pathogens and antigens, and comprising 95% of cells sampled via bronchoalveolar lavage (BAL) (51, 58, 60, 77). Interstitial macrophages, a functionally distinct population of Mϕs residing in lung parenchymal tissue, are less accessible and thus less well studied (63, 78). Similar to monocytes in blood, respiratory monocytes have been characterized as classical monocytes (CMs: CD14+ CD16−), intermediate monocytes (IM: CD14+ CD16+), and non-classical monocytes (NCMs: CD14− CD16+) (51, 52, 58, 64–66). IMs are more frequent in the airways, as opposed to blood, where CMs are in abundance; while NCMs seem to be the rarest monocyte subset (51, 58–60). CMs are the first cells to migrate out of blood to infiltrate sites of inflammation, release chemokines to attract other leukocytes; and can differentiate into mo-DCs and Mϕs (67, 68). IMs represent a population of differentiating monocytes that have been reported to expand during inflammation and/or infection (79–82). NCMs have been attributed with patrolling functions, debris removal, promoting wound healing (64, 81), and to some extent, TLR3 mediated type I interferon (IFN) production (69). Mo-DCs are an interesting subset that transiently arises in tissues from (primarily classical) monocytes recruited to the site of inflammation (46). In comparison to monocytes, DCs are rare in blood, and rarer still in the airways. Subsets of CD11c-expressing myeloid DCs (MDCs); CD1c+ MDCs, CD141+ MDCs, and more recently, langerin+ MDCs (with variable CD1a expression), as well as CD123+ plasmacytoid DCs (PDCs) have been described in the human respiratory tract (51, 57–60, 70–72). MDCs are excellent antigen-presenting cells, CD141+ MDCs specialize in cross presentation via MHC I; and PDCs excel at type I IFN-mediated antiviral protection.
In the human respiratory system, the upper respiratory tract (URT) is comprised of the nasal cavity, sinuses, and the pharynx (Figure 1A). The LRT including the trachea, bronchi, bronchioles, and alveoli, is typically divided into the proximal conducting zone and the distal respiratory zone (Figure 1A) (83). The LRT accounts for a larger cumulative surface area and consequently higher likelihood of pathogen–immune cell interactions. However, it is the URT that is initially involved in prevention of pathogen entry (83). MNP distribution in the URT, especially at steady state, also remains poorly characterized. Recent studies have shown Mϕs, CMs, MDCs, and PDCs in the nasal cavities (84, 85); CMs in the sinuses; CMs, MDCs, and PDCs in the nasopharynx (43, 44); CD1c+ MDCs in nasal tissue (86); and Mϕs, CMs, and several DC subsets (PDC, CD1c+, CD141+, CD207+, slan+, Axl+, and CD4+) have been described in human tonsils (73–76). What is evident, however, is that the relative distribution of MNP subsets at steady state varies greatly across the different compartments of the respiratory tract (51, 87). For example, in blood, monocytes greatly outnumber all other MNP subsets, whereas in tonsils, PDCs are the most abundant MNP subset. In BAL, AMϕs make up almost 95% of all cells, but IMs are more frequent than DCs. In lung tissues, both alveolar and interstitial Mϕs can be found at different frequencies. Monocytes and MDCs are also present at greater frequencies than PDCs (Figure 1B). The immunological map of the human respiratory tree is becoming more detailed (Figure 1C), enabling a better understanding of how the respiratory immune system changes during disease including respiratory viral infections like IAV.
MNPs: Innate Immune Responders in IAV Infection
Respiratory MNPs function as mucosal sentinels and come into play rapidly after onset of IAV infection. Monocytes and DCs resident in the nasopharyngeal mucosa can rapidly sense the presence of IAV and elicit an early response featuring a predominance of monocyte-recruiting chemokines like CCL2, CCL17, CX3CL1, and MCP3 (45, 88, 89). Mϕs, that are abundant in the LRT, are less likely to be involved in uncomplicated human IAV infections, when the virus typically remains localized in the URT. However, when the virus spreads lower toward the lungs, not uncommon among pandemic IAV strains, Mϕs are likely central in the innate immune response.
The diverse functional capacity of monocytes translates into their involvement in several aspects of immunity to IAV, as depicted in Figure 2. Monocytes rapidly infiltrate the URT following IAV infection where increased nasal CM numbers and cytokine (MCP3, IFNα2, and CCL17) levels can predict disease severity (43–45). Similarly, in patients infected with the pandemic A/CA/07/09 (pH1N1) strain, high numbers of CD14+, TNF-producing monocytes were reported in blood, that positively correlated with disease severity in young, otherwise healthy adults (88, 90). In addition, exposure to IAV also drives differentiation of monocytes into mo-DCs in vitro (91). Studies on human IAV infections demonstrate causal association between CCR2-dependent lung monocyte and mo-DC recruitment and IAV-induced mortality in an NOS-2-dependent manner (91–95).
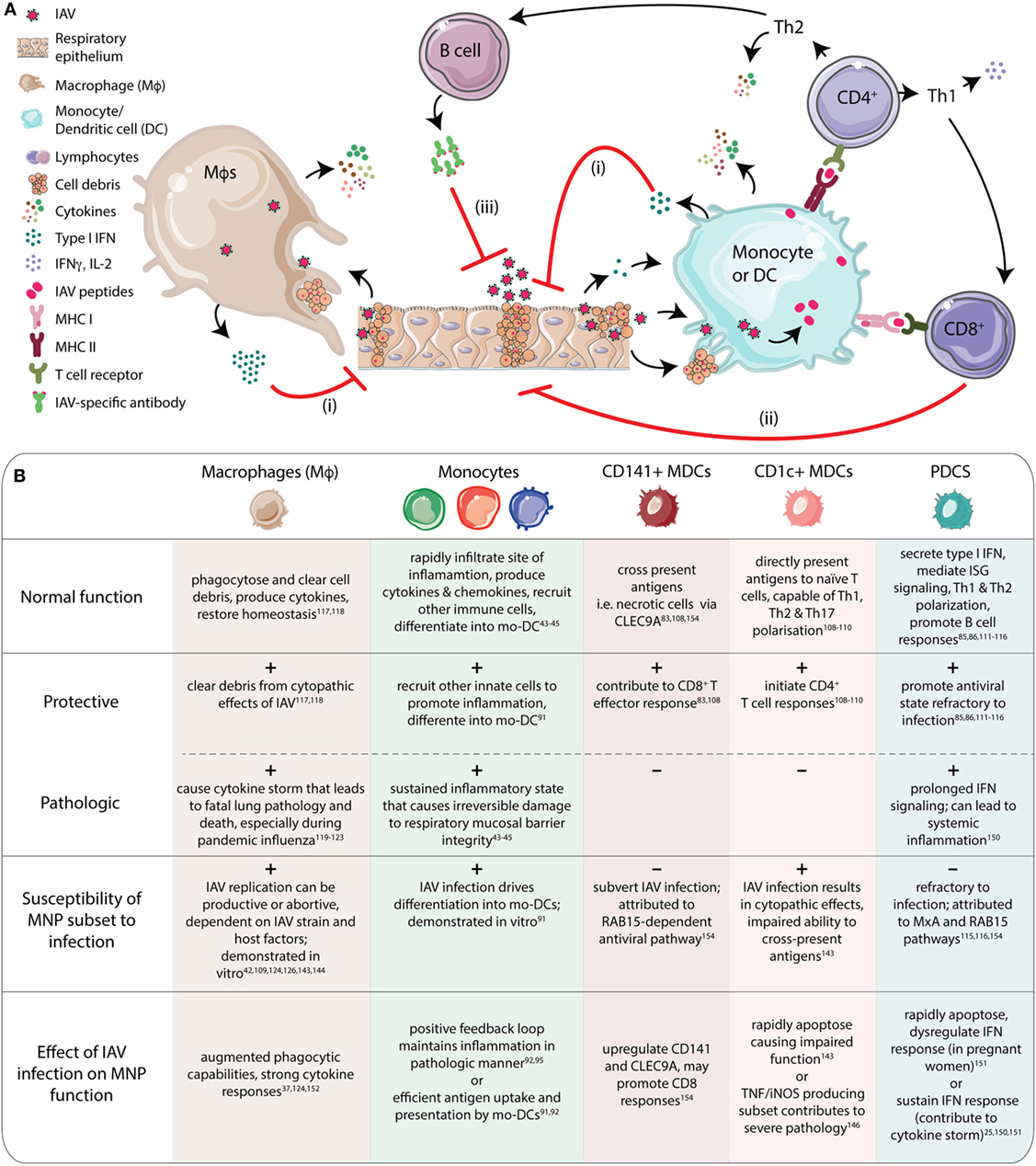
Figure 2. Human mononuclear phagocytes (MNPs) play a multitude of roles to mediate immune protection during influenza A virus (IAV) infection. (A) MNP subsets have many overlapping functions. Macrophages (Mϕs) clear up cell debris and release cytokines. Monocytes and dendritic cells (DCs) can also release cytokines and present antigens to initiate adaptive responses. (i) Following IAV infection of respiratory epithelium, Mϕs, monocytes, and DCs respond to the virus and cell debris, launching potent cytokine responses (TNFα, IL-6, IL-12p40, and IL-10), including interferon (IFN)α. Induction of interferon-stimulated genes (ISGs) promotes an antiviral state in bystander cells, protecting them from infection. (ii) The antigens taken up by monocytes/DCs are processed and presented via MHC I and II to CD8+ and CD4+ T cells, respectively. Antigen-specific CD8+ T cells perform effector functions via cytotoxic granule- and FasL-mediated caspase-dependent apoptosis. (iii) CD4+ T cells mature into subsets with specific functions. Th1 cells primarily produce IFNγ, IL-2, and TNFβ; and aid CD8+ T cell proliferation. Th2 cells on the other hand, produce IL-4, IL-5, and IL-13 and assist B cells, especially during antibody class switching, promoting production of neutralizing antibodies. Induction of broadly neutralizing antibodies against all strains of influenza virus remains a challenge in the field of influenza immunology (45, 57, 75, 76, 96–106). (B) The table summarizes the individual functions of MNP subsets that can protect against IAV infection, but also contribute to pathology. Most MNP subsets are susceptible to IAV infection, as demonstrated by in vitro studies. As a consequence of IAV infection, MNP function can be directly affected, prompting them to respond in a protective or pathologic fashion (25, 37, 42–45, 73, 75, 76, 91, 102–105, 107–127).
Mucosal tissue-resident DCs in peripheral tissues like the respiratory tract sense and take up antigens. They then migrate to draining lymph nodes to present processed antigen to T cells. Antigen-specific, clonally expanded T cells migrate back to the site of infection to control and clear infection (128–131) (Figure 2). This process is critical to restoration of homeostasis as well as for induction of potent adaptive immune responses. Murine models have elegantly demonstrated DC function during IAV infection (96–100, 132). What remains to be described is the exact role of human DC subsets. During pediatric IAV infection, MDCs and PDCs mobilize to the nasopharynx while DC numbers are reduced in blood (43, 44). The potential redistribution of DC subsets remains to be characterized in adults as well as over the course of infection. The different DC subsets each likely perform individualized tasks during IAV infection. CD1c+ MDCs are the most abundant MDCs in the airways (51, 58, 60), and are excellent at pathogen recognition (101, 133), inducing expansive T helper responses (107–109); and cytokine secretion (101). CD141+ MDCs possess superior MHC I cross-presenting abilities that can aid IAV clearance by CD8+ T cells (73, 107). TLR3 mediated cytokine production (TNF, IL-6, IL-12, and IFN-β) and importantly, type III IFN production by CD141+ MDCs, assist in enhanced innate MNP protection against IAV (57). PDCs mediate type I IFN-dependent antiviral protection that is beneficial during IAV infection. In addition to transcriptional activation of many IFN-stimulated genes (ISGs), PDCs also promote both T and B cell responses (75, 76, 102–104, 110–112).
Macrophages contribute during IAV infection by clearing cell debris, chemokine and cytokine production to modulate inflammation, recruitment of other MNPs, and to restore subsequent tissue homeostasis (Figure 2A) (105, 113). AMϕs are of particular importance when the infection reaches the LRT, where the AMϕs are in vast abundance. Severe influenza with LRT pathology is often accompanied by AMϕ involvement (114–118). Unhindered AMϕ-associated cytokinemia can result in devastating consequences for patients, ranging from delayed recovery to fatal lung pathology (116). Several factors control the extent of Mϕ involvement, two of the most likely contributors being IAV subtype/strain and Mϕ phenotype (90, 105, 113, 114, 118–120, 134) (Figure 1C). For example, Mϕ cytokine production differs across H5N1 and pandemic/seasonal H1N1 strains (119). The protective and pathologic roles of MNP subsets during IAV infection have also been summarized in Figure 2B.
Murine models of IAV infection have extensively characterized the role of MNPs in antiviral protection (92, 100, 132, 135–137). A potent immune response to human IAV infection is also likely dependent on synergy between the different MNP subsets and their functions (53, 138–141). However, MNP susceptibility to IAV infection can easily upset the balance, impacting both virus clearance and return to homeostasis.
MNPs and Respiratory Epithelium (RE): Mucosal Barriers and Targets of IAV Infection
During IAV infection, the virus is largely confined to the airways, where the RE is primarily targeted (13, 106, 142–147). The RE and MNPs represent an interesting functional dichotomy—both are targets of the virus and also capable of immune functions to limit infection (25). The epithelial tight junctions constitute a mechanical barrier against the exterior and secrete antiviral molecules. The RE senses IAV via TLRs and RIG-I; with RIG-I signaling concentrated at the tight junctions, resulting in type I and type III interferon-mediated antiviral protection (106). Chemokines secreted from the RE aid neutrophil and MNP recruitment to the site of infection, enhancing innate protection. While potently responding to IAV, the RE is also highly susceptible to the cytopathic effects of IAV infection (Figure 2A). Loss of mucosal barrier integrity promotes bacterial adherence, contributing to secondary bacterial infections and lung pathology often associated with severe IAV infection (118, 147–149).
Mononuclear phagocytes are well located in the human respiratory mucosa to be targeted by the virus upon entry (135), and the endocytic and migratory properties of MNPs are likely favorable to viral infection and dissemination (120, 134). In vitro IAV infection of human Mϕs and DCs has been shown to result in productive infection with release of infectious particles (119–121) but has also been reported to result in abortive infection (42, 108, 121, 122), the contrast being discussed in great detail in Ref. (42). Which of these alternatives prevail in clinical cases, and what host factors determine their own fate, are questions that are yet to be answered. In addition, the negative implications of IAV infection, from an immunological perspective, they may be more pronounced for MNPs than for epithelial cells as MNPs are central in establishing a protective, specific immune response.
Consequences of IAV Infection of MNPs
Mononuclear phagocyte susceptibility to IAV infection can impair their many functions. For example, MDCs are crucial for T cell activation but they are also readily susceptible to IAV infection, impairing their ability to present antigens via both the direct presentation and cross presentation pathways (46, 121, 150). Most seasonal and low-pathogenic IAV strains infect respiratory human Mϕs and DCs but replication is typically abortive and therefore skews in favor of host defense (120). However, highly pathogenic strains of IAV can overcome this barrier and productively infect Mϕs and DCs, which in turn can impact viral amplification, dissemination, as well as pathogenicity and immunogenicity (123). Primary human monocytes exposed to H5N1 or highly pathogenic avian influenza strains in vitro exhibit a reduced antiviral response, as a consequence of impaired NF-κB signaling (91, 114, 115). In a murine model of IAV infection, CCR2+ inflammatory monocytes accumulate in lungs (92, 94). Impaired virus clearance by MNPs triggers IFN-mediated recruitment of CCR2+ monocytes inflammatory in a positive-feedback loop, resulting in severe lung pathology (92) (Figure 2B).
Impaired MNP responses have also been observed in IAV patients. Peripheral blood monocytes and to some extent PDCs, exhibit attenuated IFN responses indicating dysregulation at a systemic level, in particular in infants and the elderly, two of the largest risk groups for severe influenza disease (151–153). Human PDCs that potently produce large amounts of type I IFN, in response even to low doses of IAV, can rapidly undergo apoptosis when exposed to high doses of the virus (25, 124). Possibly related to that, it has been reported that pregnant women, a risk group for influenza, have fewer PDCs in circulation that are also less efficient at IFN production, which could contribute to more severe IAV disease during pregnancy (125) (Figure 2B).
As undesirable as depressed MNP function is, excessive activation of MNPs can also be equally dangerous, by contributing to IAV-induced immune pathology leading to fatal respiratory distress. Human monocyte-derived pro-inflammatory Mϕs exposed to IAV in vitro exhibit augmented phagocytic capability and strong cytokine responses (119). While this can encourage adaptive responses, it also contributes to the cytokine storm that is a hallmark of severe influenza disease (37, 126). Prolonged IFN signaling can also destroy alveolar epithelium and contribute to development of secondary bacterial infections, the most common complication associated with influenza infections (93). TNF/iNOS-producing DCs, a subset of inflammatory DCs, accumulate in the LRT and promote CD8+ T cell responses in an IAV mouse model, but are also positively correlated with higher lethality (123). However, in vitro, human CD8+ T cells can rapidly induce monocyte differentiation into tip-DCs that in turn prime naïve CD4+ T cells and promote protective Th1 responses (154) (Figure 2B).
Not all respiratory MNP–IAV interactions have adverse implications. Virus-induced human in vitro mo-DCs express both CLEC9A and CD141, as do blood CD141+ MDCs. But uniquely, mo-DCs express CD141 on the cell surface and CLEC9A intracellularly (91). CD141+ DCs can efficiently prime and drive CD8+ T cell proliferation, while CLEC9A is linked to antigen uptake. CD141+ MDCs also subvert IAV infection by resisting virus entry in a RAB-15 dependent manner, instead relying on uptake of apoptotic virus-infected CD1c+ MDCs (and other cells) as a source of antigens (127) (Figure 2B). Virus-induced CD141+ DCs also exhibit type I IFN secretion and upregulate ISGs (tetherin, viperin, and IFITM3) and RIG-I/MDA5, suggesting an important protective role for them during infection; despite poor expression of co-stimulatory molecules (CD40, CD86, and HLA-DR), weaker pro-inflammatory cytokine expression, and impaired ability to activate naïve CD4+ T cells (46). Induction of CD141+ DCs could therefore be employed in vaccination/therapeutic strategies. To summarize, while IAV infection of MNP compromises some aspects of innate protection, biological redundancy due to the overlapping functions of MNP subsets can likely prevent loss of essential immune responses.
Concluding Remarks
Respiratory MNPs are important in the immune responses to IAV infection. At the same time, MNP susceptibility to IAV infection poses an interesting immunological challenge. Several key questions still remain to be further addressed to understand this dichotomy better. Does compromised MNP function result in altered innate immune responses? Do altered innate immune responses subsequently impair efficient induction of adaptive responses, ultimately contributing to increased host morbidity and mortality? If on the other hand, robust, unchecked innate responses lead to prolonged inflammation, causing irreparable damage to the host, is there a commonality in host responses across the various demographics affected by influenza? To answer these questions, and delineate the role of respiratory MNPs in human IAV infection, it will be critical to detail the function of the different MNP subsets—for example, functional assessment of sorted cells from the respiratory system and performing RNA sequencing or epigenetic analyses. Prospective studies of human IAV patients where detailed analyses of tissue samples can be correlated to clinical parameters are likely required to fully understand how MNPs contribute to disease severity.
Author Contributions
SV and MY performed the literature review. SV designed the figures. SV, MY, and AS-S organized and wrote the manuscript. SV and AS-S edited the manuscript.
Conflict of Interest Statement
The authors declare that the research was conducted in the absence of any commercial or financial relationships that could be construed as a potential conflict of interest.
The handling Editor declared a shared affiliation, though no other collaboration, with the authors.
Funding
This work was supported by grants to AS-S from the following sources: the Swedish Research Council, the Swedish Foundation for Strategic Research, the Swedish Heart-Lung Foundation, and Karolinska Institutet.
Abbreviations
AMϕ, alveolar macrophage; ARDS, acute respiratory distress syndrome; BAL, bronchoalveolar lavage; CM, classical monocyte; DC, dendritic cell; IAV, influenza A virus; IFN, interferon; IM, intermediate monocyte; IMϕ, interstitial macrophage; ISG, interferon-stimulated gene; LRT, lower respiratory tract; MDC, myeloid dendritic cell; MNP, mononuclear phagocyte; Mo-DC, monocyte-derived dendritic cell; Mϕ, macrophage; NCM, non-classical monocyte; PDC, plasmacytoid dendritic cell; RE, respiratory epithelium; tipDC, TNF/iNOS-producing dendritic cell; URT, upper respiratory tract.
References
1. Hirsch A. Handbook of Geographical and Historical Pathology. London: The New Sydenham Society (1883).
2. Potter CW. A history of influenza. J App Microbiol. (2001) 91:572–9. doi:10.1046/j.1365-2672.2001.01492.x
3. Dunn JJ, Miller MB. Emerging respiratory viruses other than influenza. Clin Lab Med (2014) 34(2):409–30. doi:10.1016/j.cll.2014.02.011
4. Hui DS, Zumla A. Emerging respiratory tract viral infections. Curr Opin Pulm Med (2015) 21(3):284–92. doi:10.1097/MCP.0000000000000153
5. Gao GF. From “A”IV to “Z”IKV: attacks from emerging and re-emerging pathogens. Cell (2018) 172(6):1157–9. doi:10.1016/j.cell.2018.02.025
6. Bedford T, Suchard MA, Lemey P, Dudas G, Gregory V, Hay AJ, et al. Integrating influenza antigenic dynamics with molecular evolution. Elife (2014) 3:e01914. doi:10.7554/eLife.01914
7. Drake JW. Rates of spontaneous mutation among RNA viruses. Proc Natl Acad Sci U S A (1993) 90:4171–5. doi:10.1073/pnas.90.9.4171
8. Drake JW, Holland JJ. Mutation rates among RNA viruses. Proc Natl Acad Sci U S A (1999) 96(24):13910–3. doi:10.1073/pnas.96.24.13910
9. Chen H, Deng Q, Ng SH, Lee RT, Maurer-Stroh S, Zhai W. Dynamic convergent evolution drives the passage adaptation across 48 years’ history of H3N2 influenza evolution. Mol Biol Evol (2016) 33(12):3133–43. doi:10.1093/molbev/msw190
10. WHO. The Top 10 Causes of Death – Fact Sheet No. 310 2014. Geneva: WHO (2017). Available from: http://www.who.int/news-room/fact-sheets/detail/the-top-10-causes-of-death (Accessed: March 14, 2018).
11. Russell CA, Kasson PM, Donis RO, Riley S, Dunbar J, Rambaut A, et al. Improving pandemic influenza risk assessment. Elife (2014) 3:e03883. doi:10.7554/eLife.03883
12. Petrova VN, Russell CA. The evolution of seasonal influenza viruses. Nat Rev Microbiol (2018) 16(1):47–60. doi:10.1038/nrmicro.2017.118
13. Wootton S, Aguilera EA, Wanger A, Jewell A, Patel K, Murphy JR, et al. Detection of NH1N1 influenza virus in nonrespiratory sites among children. Pediatr Infect Dis J (2014) 33(1):95–6. doi:10.1097/INF.0b013e3182a09de7
14. Zhu L, Liu L, Zhang Y, Pu L, Liu J, Li X, et al. High level of neutrophil extracellular traps correlates with poor prognosis of severe influenza A infection. J Infect Dis (2018) 217(3):428–37. doi:10.1093/infdis/jix475
15. Wang K, Lai C, Li T, Wang C, Wang W, Ni B, et al. Basic fibroblast growth factor protects against influenza A virus-induced acute lung injury by recruiting neutrophils. J Mol Cell Biol (2017) 1–13. doi:10.1093/jmcb/mjx047
16. Buttignol M, Pires-Neto RC, Rossi ESRC, Albino MB, Dolhnikoff M, Mauad T. Airway and parenchyma immune cells in influenza A(H1N1)pdm09 viral and non-viral diffuse alveolar damage. Respir Res (2017) 18(1):147. doi:10.1186/s12931-017-0630-x
17. Iuliano AD, Roguski KM, Chang HH, Muscatello DJ, Palekar R, Tempia S, et al. Estimates of global seasonal influenza-associated respiratory mortality: a modelling study. Lancet (2018) 391(10127):1285–300. doi:10.1016/S0140-6736(17)33293-2
18. Summers JA, Stanley J, Baker MG, Wilson N. Risk factors for death from pandemic influenza in 1918-1919: a case-control study. Influenza Other Respir Viruses (2014) 8(3):329–38. doi:10.1111/irv.12228
19. Gagnon A, Miller MS, Hallman SA, Bourbeau R, Herring DA, Earn DJ, et al. Age-specific mortality during the 1918 influenza pandemic: unravelling the mystery of high young adult mortality. PLoS One (2013) 8(8):e69586. doi:10.1371/journal.pone.0069586
20. Ma J, Dushoff J, Earn DJ. Age-specific mortality risk from pandemic influenza. J Theor Biol (2011) 288:29–34. doi:10.1016/j.jtbi.2011.08.003
21. Viboud C, Simonsen L, Fuentes R, Flores J, Miller MA, Chowell G. Global mortality impact of the 1957-1959 influenza pandemic. J Infect Dis (2016) 213(5):738–45. doi:10.1093/infdis/jiv534
22. Taubenberger JK, Morens DM. The pathology of influenza virus infections. Annu Rev Pathol (2008) 3:499–522. doi:10.1146/annurev.pathmechdis.3.121806.154316
23. Taubenberger JK, Reid AH, Krafft AE, Bijwaard KE, Fanning TG. Initial genetic characterization of the 1918 “Spanish” influenza virus. Science (1997) 275:1793–6. doi:10.1126/science.275.5307.1793
24. Connors TJ, Ravindranath TM, Bickham KL, Gordon CL, Zhang F, Levin B, et al. Airway CD8(+) T cells are associated with lung injury during infant viral respiratory tract infection. Am J Respir Cell Mol Biol (2016) 54(6):822–30. doi:10.1165/rcmb.2015-0297OC
25. Gerlach RL, Camp JV, Chu YK, Jonsson CB. Early host responses of seasonal and pandemic influenza A viruses in primary well-differentiated human lung epithelial cells. PLoS One (2013) 8(11):e78912. doi:10.1371/journal.pone.0078912
26. Gerlach T, Hensen L, Matrosovich T, Bergmann J, Winkler M, Peteranderl C, et al. pH optimum of hemagglutinin-mediated membrane fusion determines sensitivity of influenza A viruses to the interferon-induced antiviral state and IFITMs. J Virol (2017) 91(11):1–16. doi:10.1128/JVI.00246-17
27. Kroetz DN, Allen RM, Schaller MA, Cavallaro C, Ito T, Kunkel SL. Type I interferon induced epigenetic regulation of macrophages suppresses innate and adaptive immunity in acute respiratory viral infection. PLoS Pathog (2015) 11(12):e1005338. doi:10.1371/journal.ppat.1005338
28. Travanty E, Zhou B, Zhang H, Di YP, Alcorn JF, Wentworth DE, et al. Differential susceptibilities of human lung primary cells to H1N1 influenza viruses. J Virol (2015) 89(23):11935–44. doi:10.1128/JVI.01792-15
29. Yang Y, Tang H. Aberrant coagulation causes a hyper-inflammatory response in severe influenza pneumonia. Cell Mol Immunol (2016) 13(4):432–42. doi:10.1038/cmi.2016.1
30. Peiris JS, Hui KP, Yen HL. Host response to influenza virus: protection versus immunopathology. Curr Opin Immunol (2010) 22(4):475–81. doi:10.1016/j.coi.2010.06.003
31. Kamal RP, Alymova IV, York IA. Evolution and virulence of influenza A virus protein PB1-F2. Int J Mol Sci (2018) 19(1):1–15. doi:10.3390/ijms19010096
32. Mok BW, Liu H, Chen P, Liu S, Lau SY, Huang X, et al. The role of nuclear NS1 protein in highly pathogenic H5N1 influenza viruses. Microbes Infect (2017) 19(12):587–96. doi:10.1016/j.micinf.2017.08.011
33. Shanks GD, Brundage JF. Pathogenic responses among young adults during the 1918 influenza pandemic. Emerg Infect Dis (2012) 18(2):201–7. doi:10.3201/eid1802.102042
34. Loo YM, Gale M Jr. Fatal immunity and the 1918 virus. Nature (2007) 445:267–8. doi:10.1038/445267a
35. Kobasa D, Jones SM, Shinya K, Kash JC, Copps J, Ebihara H, et al. Aberrant innate immune response in lethal infection of macaques with the 1918 influenza virus. Nature (2007) 445(7125):319–23. doi:10.1038/nature05495
36. Bertoletti A, Sette A, Chisari FV, Penna A, Levrero M, De Carli M, et al. Natural variants of cytotoxic epitopes are T-cell receptor antagonists for antiviral cytotoxic T cells. Nature (1994) 369:407–10. doi:10.1038/369407a0
37. Berdal JE, Mollnes TE, Waehre T, Olstad OK, Halvorsen B, Ueland T, et al. Excessive innate immune response and mutant D222G/N in severe A (H1N1) pandemic influenza. J Infect (2011) 63(4):308–16. doi:10.1016/j.jinf.2011.07.004
38. Sridhar S, Begom S, Bermingham A, Hoschler K, Adamson W, Carman W, et al. Cellular immune correlates of protection against symptomatic pandemic influenza. Nat Med (2013) 19(10):1305–12. doi:10.1038/nm.3350
39. van de Sandt CE, Hillaire ML, Geelhoed-Mieras MM, Osterhaus AD, Fouchier RA, Rimmelzwaan GF. Human influenza A virus-specific CD8+ T-cell response is long-lived. J Infect Dis (2015) 212(1):81–5. doi:10.1093/infdis/jiv018
40. Reichman RC, Pons VG, Murphy BR, Caplan EA, Dolin R. Cell-mediated cytotoxicity following influenza infection and vaccination in humans. J Med Virol (1979) 4:1–14. doi:10.1002/jmv.1890040102
41. Baharom FRG, Blomberg A, Smed-Sörensen A. Human lung mononuclear phagocytes in health and disease. Front Immunol (2017) 8:499. doi:10.3389/fimmu.2017.00499
42. Short KR, Brooks AG, Reading PC, Londrigan SL. The fate of influenza A virus after infection of human macrophages and dendritic cells. J Gen Virol (2012) 93(Pt 11):2315–25. doi:10.1099/vir.0.045021-0
43. Gill MA, Long K, Kwon T, Muniz L, Mejias A, Connolly J, et al. Differential recruitment of dendritic cells and monocytes to respiratory mucosal sites in children with influenza virus or respiratory syncytial virus infection. J Infect Dis (2008) 198(11):1667–76. doi:10.1086/593018
44. Gill MA, Palucka KA, Barton T, Ghaffar F, Jafri H, Banchereau J, et al. Mobilization of plasmacytoid and myeloid dendritic cells to mucosal sites in children with respiratory syncytial virus and other viral respiratory infections. J Infect Dis (2005) 2005(191):1105–15. doi:10.1086/428589
45. Oshansky CM, Gartland AJ, Wong SS, Jeevan T, Wang D, Roddam PL, et al. Mucosal immune responses predict clinical outcomes during influenza infection independently of age and viral load. Am J Respir Crit Care Med (2014) 189(4):449–62. doi:10.1164/rccm.201309-1616OC
46. Cao W, Taylor AK, Biber RE, Davis WG, Kim JH, Reber AJ, et al. Rapid differentiation of monocytes into type I IFN-producing myeloid dendritic cells as an antiviral strategy against influenza virus infection. J Immunol (2012) 189(5):2257–65. doi:10.4049/jimmunol.1200168
47. Diao H, Cui G, Wei Y, Chen J, Zuo J, Cao H, et al. Severe H7N9 infection is associated with decreased antigen-presenting capacity of CD14+ cells. PLoS One (2014) 9(3):e92823. doi:10.1371/journal.pone.0092823
48. Lichtner M, Mastroianni CM, Rossi R, Russo G, Belvisi V, Marocco R, et al. Severe and persistent depletion of circulating plasmacytoid dendritic cells in patients with 2009 pandemic H1N1 infection. PLoS One (2011) 6(5):e19872. doi:10.1371/journal.pone.0019872
49. Soloff AC, Weirback HK, Ross TM, Barratt-Boyes SM. Plasmacytoid dendritic cell depletion leads to an enhanced mononuclear phagocyte response in lungs of mice with lethal influenza virus infection. Comp Immunol Microbiol Infect Dis (2012) 35(4):309–17. doi:10.1016/j.cimid.2012.01.012
50. Guilliams M, Lambrecht BN, Hammad H. Division of labor between lung dendritic cells and macrophages in the defense against pulmonary infections. Mucosal Immunol (2013) 6(3):464–73. doi:10.1038/mi.2013.14
51. Baharom F, Thomas S, Rankin G, Lepzien R, Pourazar J, Behndig AF, et al. Dendritic cells and monocytes with distinct inflammatory responses reside in lung mucosa of healthy humans. J Immunol (2016) 196(11):4498–509. doi:10.4049/jimmunol.1600071
52. Baharom F, Rankin G, Scholz S, Pourazar J, Ahlm C, Blomberg A, et al. Human lung dendritic cells: spatial distribution and phenotypic identification in endobronchial biopsies using immunohistochemistry and flow cytometry. J Vis Exp (2017) 119:e55222. doi:10.3791/55222
53. Pulendran B, Tang H, Denning TL. Division of labor, plasticity, and crosstalk between dendritic cell subsets. Curr Opin Immunol (2008) 20(1):61–7. doi:10.1016/j.coi.2007.10.009
54. Gehr P, Bachofen M, Weibel E. The normal human lung – ultrastructure and morphometric estimation of diffusion capacity. Respir Physiol (1978) 32:121–40. doi:10.1016/0034-5687(78)90104-4
55. Hamm H, Fabel H, Bartsch W. The surfactant system of the adult lung – physiology and clinical perspectives. Clin Investig (1992) 70:637–57. doi:10.1007/BF00180279
56. Wonderlich ER, Swan ZD, Bissel SJ, Hartman AL, Carney JP, O’Malley KJ, et al. Widespread virus replication in alveoli drives acute respiratory distress syndrome in aerosolized H5N1 influenza infection of macaques. J Immunol (2017) 198(4):1616–26. doi:10.4049/jimmunol.1601770
57. Granot T, Senda T, Carpenter DJ, Matsuoka N, Weiner J, Gordon CL, et al. Dendritic cells display subset and tissue-specific maturation dynamics over human life. Immunity (2017) 46(3):504–15. doi:10.1016/j.immuni.2017.02.019
58. Desch AN, Gibbings SL, Goyal R, Kolde R, Bednarek J, Bruno T, et al. Flow cytometric analysis of mononuclear phagocytes in nondiseased human lung and lung-draining lymph nodes. Am J Respir Crit Care Med (2016) 193(6):614–26. doi:10.1164/rccm.201507-1376OC
59. Patel VI, Booth JL, Duggan ES, Cate S, White VL, Hutchings D, et al. Transcriptional classification and functional characterization of human airway macrophage and dendritic cell subsets. J Immunol (2017) 198(3):1183–201. doi:10.4049/jimmunol.1600777
60. Yu YR, Hotten DF, Malakhau Y, Volker E, Ghio AJ, Noble PW, et al. Flow cytometric analysis of myeloid cells in human blood, bronchoalveolar lavage, and lung tissues. Am J Respir Cell Mol Biol (2016) 54(1):13–24. doi:10.1165/rcmb.2015-0146OC
61. Patel VI, Metcalf JP. Identification and characterization of human dendritic cell subsets in the steady state: a review of our current knowledge. J Investig Med (2016) 64(4):833–47. doi:10.1136/jim-2016-000072
62. Iwasaki A. Mucosal dendritic cells. Annu Rev Immunol (2007) 25:381–418. doi:10.1146/annurev.immunol.25.022106.141634
63. Fathi M, Johansson A, Lundborg M, Orre L, Skold CM, Camner P. Functional and morphological differences between human alveolar and interstitial macrophages. Exp Mol Pathol (2001) 70(2):77–82. doi:10.1006/exmp.2000.2344
64. Thomas GD, Hamers AAJ, Nakao C, Marcovecchio P, Taylor AM, McSkimming C, et al. Human blood monocyte subsets: a new gating strategy defined using cell surface markers identified by mass cytometry. Arterioscler Thromb Vasc Biol (2017) 37(8):1548–58. doi:10.1161/ATVBAHA.117.309145
65. Ziegler-Heitbrock L. Blood monocytes and their subsets: established features and open questions. Front Immunol (2015) 6:423. doi:10.3389/fimmu.2015.00423
66. Abeles RD, McPhail MJ, Sowter D, Antoniades CG, Vergis N, Vijay GK, et al. CD14, CD16 and HLA-DR reliably identifies human monocytes and their subsets in the context of pathologically reduced HLA-DR expression by CD14(hi)/CD16(neg) monocytes: expansion of CD14(hi)/CD16(pos) and contraction of CD14(lo)/CD16(pos) monocytes in acute liver failure. Cytometry A (2012) 81(10):823–34. doi:10.1002/cyto.a.22104
67. Serbina NV, Pamer EG. Monocyte emigration from bone marrow during bacterial infection requires signals mediated by chemokine receptor CCR2. Nat Immunol (2006) 7(3):311–7. doi:10.1038/ni1309
68. Patel AA, Zhang Y, Fullerton JN, Boelen L, Rongvaux A, Maini AA, et al. The fate and lifespan of human monocyte subsets in steady state and systemic inflammation. J Exp Med (2017) 214(7):1913–23. doi:10.1084/jem.20170355
69. Boyette LB, Macedo C, Hadi K, Elinoff BD, Walters JT, Ramaswami B, et al. Phenotype, function, and differentiation potential of human monocyte subsets. PLoS One (2017) 12(4):e0176460. doi:10.1371/journal.pone.0176460
70. Alcantara-Hernandez M, Leylek R, Wagar LE, Engleman EG, Keler T, Marinkovich MP, et al. High-dimensional phenotypic mapping of human dendritic cells reveals interindividual variation and tissue specialization. Immunity (2017) 47(6):1037–50.e6. doi:10.1016/j.immuni.2017.11.001
71. See P, Dutertre CA, Chen J, Gunther P, McGovern N, Irac SE, et al. Mapping the human DC lineage through the integration of high-dimensional techniques. Science (2017) 356(6342):1–13. doi:10.1126/science.aag3009
72. Villani AC, Satija R, Reynolds G, Sarkizova S, Shekhar K, Fletcher J, et al. Single-cell RNA-seq reveals new types of human blood dendritic cells, monocytes, and progenitors. Science (2017) 356(6335):1–12. doi:10.1126/science.aah4573
73. Jongbloed SL, Kassianos AJ, McDonald KJ, Clark GJ, Ju X, Angel CE, et al. Human CD141+ (BDCA-3)+ dendritic cells (DCs) represent a unique myeloid DC subset that cross-presents necrotic cell antigens. J Exp Med (2010) 207(6):1247–60. doi:10.1084/jem.20092140
74. De Monte AO, Olivieri CV, Vitale S, Bailleux S, Castillo L, Giordanengo V, et al. CD1c-related DCs that express CD207: Langerin, but are distinguishable from Langerhans cells, are consistently present in human tonsils. Front Immunol (2016) 7:197. doi:10.3389/fimmu.2016.00197
75. Segura E, Durand M, Amigorena S. Similar antigen cross-presentation capacity and phagocytic functions in all freshly isolated human lymphoid organ-resident dendritic cells. J Exp Med (2013) 210(5):1035–47. doi:10.1084/jem.20121103
76. Segura E, Valladeau-Guilemond J, Donnadieu MH, Sastre-Garau X, Soumelis V, Amigorena S. Characterization of resident and migratory dendritic cells in human lymph nodes. J Exp Med (2012) 209(4):653–60. doi:10.1084/jem.20111457
77. Pinilla-Vera M, Xiong Z, Zhao Y, Zhao J, Donahoe MP, Barge S, et al. Full spectrum of LPS activation in alveolar macrophages of healthy volunteers by whole transcriptomic profiling. PLoS One (2016) 11(7):e0159329. doi:10.1371/journal.pone.0159329
78. Liegeois M, Legrand C, Desmet CJ, Marichal T, Bureau F. The interstitial macrophage: a long-neglected piece in the puzzle of lung immunity. Cell Immunol (2018). doi:10.1016/j.cellimm.2018.02.001
79. Duan M, Hibbs ML, Chen W. The contributions of lung macrophage and monocyte heterogeneity to influenza pathogenesis. Immunol Cell Biol (2017) 95(3):225–35. doi:10.1038/icb.2016.97
80. Shi C, Pamer EG. Monocyte recruitment during infection and inflammation. Nat Rev Immunol (2011) 11(11):762–74. doi:10.1038/nri3070
81. Lee J, Tam H, Adler L, Ilstad-Minnihan A, Macaubas C, Mellins ED. The MHC class II antigen presentation pathway in human monocytes differs by subset and is regulated by cytokines. PLoS One (2017) 12(8):e0183594. doi:10.1371/journal.pone.0183594
82. Kwissa M, Nakaya HI, Onlamoon N, Wrammert J, Villinger F, Perng GC, et al. Dengue virus infection induces expansion of a CD14(+)CD16(+) monocyte population that stimulates plasmablast differentiation. Cell Host Microbe (2014) 16(1):115–27. doi:10.1016/j.chom.2014.06.001
83. Standring S, Borley NR, Collins P, Crossman AR, Gatzoulis MA, Healy JC, et al. Gray’s Anatomy The Anatomical Basis of Clinical Practice. 40th ed. Standring S, editor. London, United Kingdom: Elsevier (2008).
84. Jochems SP, Piddock K, Rylance J, Adler H, Carniel BF, Collins A, et al. Novel analysis of immune cells from nasal microbiopsy demonstrates reliable, reproducible data for immune populations, and superior cytokine detection compared to nasal wash. PLoS One (2017) 12(1):e0169805. doi:10.1371/journal.pone.0169805
85. Juliusson S, Bachert C, Klementsson H, Karlsson G, Pipkorn U. Macrophages on the nasal mucosal surface in provoked and naturally occurring allergic rhinitis. Acta Otolaryngol (2009) 111(5):946–53. doi:10.3109/00016489109138435
86. Lee H, Ruane D, Law K, Ho Y, Garg A, Rahman A, et al. Phenotype and function of nasal dendritic cells. Mucosal Immunol (2015) 8(5):1083–98. doi:10.1038/mi.2014.135
87. Kopf M, Schneider C, Nobs SP. The development and function of lung-resident macrophages and dendritic cells. Nat Immunol (2015) 16(1):36–44. doi:10.1038/ni.3052
88. Cole SL, Ho LP. Contribution of innate immune cells to pathogenesis of severe influenza virus infection. Clin Sci (Lond) (2017) 131(4):269–83. doi:10.1042/CS20160484
89. Sprenger H, Meyer RG, Kaufmann A, Bussfeld D, Rischkowsky E, Gemsa D. Selective induction of monocyte and not neutrophil-attracting chemokines after influenza A virus infection. J Exp Med (1996) 184:1191–6. doi:10.1084/jem.184.3.1191
90. Cole SL, Dunning J, Kok WL, Benam KH, Benlahrech A, Repapi E, et al. M1-like monocytes are a major immunological determinant of severity in previously healthy adults with life-threatening influenza. JCI Insight (2017) 2(7):e91868. doi:10.1172/jci.insight.91868
91. Hou W, Gibbs JS, Xiuju L, Brooke CB, Roy D, Modlin RL, et al. Viral infection triggers rapid differentiation of human blood monocytes into dendritic cells. Blood (2012) 119(13):3128–31. doi:10.1182/blood-2011-09-379479
92. Lin KL, Suzuki Y, Nakano H, Ramsburg E, Gunn MD. CCR2+ monocyte-derived dendritic cells and exudate macrophages produce influenza-induced pulmonary immune pathology and mortality. J Immunol (2008) 180(4):2562–72. doi:10.4049/jimmunol.180.4.2562
93. Lin SJ, Lee YC. Effect of influenza A infection on maturation and function of neonatal monocyte-derived dendritic cells. Viral Immunol (2014) 27(6):277–84. doi:10.1089/vim.2014.0007
94. Dawson TC, Beck MA, Kuziel WA, Henderson F, Maeda N. Contrasting effects of CCR5 and CCR2 deficiency in the pulmonary inflammatory response to influenza A virus. Am J Pathol (2000) 156(6):1951–9. doi:10.1016/S0002-9440(10)65068-7
95. Herold S, Steinmueller M, von Wulffen W, Cakarova L, Pinto R, Pleschka S, et al. Lung epithelial apoptosis in influenza virus pneumonia: the role of macrophage-expressed TNF-related apoptosis-inducing ligand. J Exp Med (2008) 205(13):3065–77. doi:10.1084/jem.20080201
96. McGill J, Van Rooijen N, Legge KL. Protective influenza-specific CD8 T cell responses require interactions with dendritic cells in the lungs. J Exp Med (2008) 205(7):1635–46. doi:10.1084/jem.20080314
97. Helft J, Manicassamy B, Guermonprez P, Hashimoto D, Silvin A, Agudo J, et al. Cross-presenting CD103+ dendritic cells are protected from influenza virus infection. J Clin Invest (2012) 122(11):4037–47. doi:10.1172/JCI60659
98. Lawrence CW, Braciale TJ. Activation, differentiation, and migration of naive virus-specific CD8+ T cells during pulmonary influenza virus infection. J Immunol (2004) 173(2):1209–18. doi:10.4049/jimmunol.173.2.1209
99. Lawrence CW, Ream RM, Braciale TJ. Frequency, specificity, and sites of expansion of CD8+ T cells during primary pulmonary influenza virus infection. J Immunol (2005) 174(9):5332–40. doi:10.4049/jimmunol.174.9.5332
100. Yu CI, Becker C, Wang Y, Marches F, Helft J, Leboeuf M, et al. Human CD1c+ dendritic cells drive the differentiation of CD103+ CD8+ mucosal effector T cells via the cytokine TGF-beta. Immunity (2013) 38(4):818–30. doi:10.1016/j.immuni.2013.03.004
101. Hemont C, Neel A, Heslan M, Braudeau C, Josien R. Human blood mDC subsets exhibit distinct TLR repertoire and responsiveness. J Leukoc Biol (2013) 93(4):599–609. doi:10.1189/jlb.0912452
102. Kool M, Geurtsvankessel C, Muskens F, Madeira FB, van Nimwegen M, Kuipers H, et al. Facilitated antigen uptake and timed exposure to TLR ligands dictate the antigen-presenting potential of plasmacytoid DCs. J Leukoc Biol (2011) 90(6):1177–90. doi:10.1189/jlb.0610342
103. Matsui T, Connolly JE, Michnevitz M, Chaussabel D, Yu CI, Glaser C, et al. CD2 distinguishes two subsets of human plasmacytoid dendritic cells with distinct phenotype and functions. J Immunol (2009) 182(11):6815–23. doi:10.4049/jimmunol.0802008
104. Haller K. Human MxA protein – an interferon-induced dynamin-like GTPase with broad antiviral activity. J Interferon Cytokine Res (2011) 31(1):79–87. doi:10.1089/jir.2010.0076
105. Sakabe S, Iwatsuki-Horimoto K, Takano R, Nidom CA, Le M, Nagamura-Inoue T, et al. Cytokine production by primary human macrophages infected with highly pathogenic H5N1 or pandemic H1N1 2009 influenza viruses. J Gen Virol (2011) 92(Pt 6):1428–34. doi:10.1099/vir.0.030346-0
106. Short KR, Kasper J, van der Aa S, Andeweg AC, Zaaraoui-Boutahar F, Goeijenbier M, et al. Influenza virus damages the alveolar barrier by disrupting epithelial cell tight junctions. Eur Respir J (2016) 47(3):954–66. doi:10.1183/13993003.01282-2015
107. Schlitzer A, McGovern N, Teo P, Zelante T, Atarashi K, Low D, et al. IRF4 transcription factor-dependent CD11b+ dendritic cells in human and mouse control mucosal IL-17 cytokine responses. Immunity (2013) 38(5):970–83. doi:10.1016/j.immuni.2013.04.011
108. Faith A, McDonald J, Peek E, Richards D, Caulfield J, Chevretton E, et al. Functional plasticity of human respiratory tract dendritic cells: GM-CSF enhances T(H)2 development. J Allergy Clin Immunol (2005) 116(5):1136–43. doi:10.1016/j.jaci.2005.08.002
109. Bogiatzi SI, Guillot-Delost M, Cappuccio A, Bichet JC, Chouchane-Mlik O, Donnadieu MH, et al. Multiple-checkpoint inhibition of thymic stromal lymphopoietin-induced TH2 response by TH17-related cytokines. J Allergy Clin Immunol (2012) 130(1):233–40.e5. doi:10.1016/j.jaci.2012.04.038
110. Di Pucchio T, Chatterjee B, Smed-Sorensen A, Clayton S, Palazzo A, Montes M, et al. Direct proteasome-independent cross-presentation of viral antigen by plasmacytoid dendritic cells on major histocompatibility complex class I. Nat Immunol (2008) 9(5):551–7. doi:10.1038/ni.1602
111. Fonteneau JF, Gilliet M, Larsson M, Dasilva I, Munz C, Liu YJ, et al. Activation of influenza virus-specific CD4+ and CD8+ T cells: a new role for plasmacytoid dendritic cells in adaptive immunity. Blood (2003) 101(9):3520–6. doi:10.1182/blood-2002-10-3063
112. Haller O, Gao S, von der Malsburg A, Daumke O, Kochs G. Dynamin-like MxA GTPase: structural insights into oligomerization and implications for antiviral activity. J Biol Chem (2010) 285(37):28419–24. doi:10.1074/jbc.R110.145839
113. Wang J, Nikrad MP, Travanty EA, Zhou B, Phang T, Gao B, et al. Innate immune response of human alveolar macrophages during influenza A infection. PLoS One (2012) 7(3):e29879. doi:10.1371/journal.pone.0029879
114. Friesenhagen J, Boergeling Y, Hrincius E, Ludwig S, Roth J, Viemann D. Highly pathogenic avian influenza viruses inhibit effective immune responses of human blood-derived macrophages. J Leukoc Biol (2012) 92(1):11–20. doi:10.1189/jlb.0911479
115. Friesenhagen J, Viemann D, Börgeling Y, Schmolke M, Spiekermann C, Kirschnek S, et al. Highly pathogenic influenza viruses inhibit inflammatory response in monocytes via activation of Rar-related orphan receptor RORa. J Innate Immun (2013) 5:505–18. doi:10.1159/000346706
116. Kuiken T, van den Brand J, van Riel D, Pantin-Jackwood M, Swayne DE. Comparative pathology of select agent influenza a virus infections. Vet Pathol (2010) 47(5):893–914. doi:10.1177/0300985810378651
117. Guarner J, Falcon-Escobedo R. Comparison of the pathology caused by H1N1, H5N1, and H3N2 influenza viruses. Arch Med Res (2009) 40(8):655–61. doi:10.1016/j.arcmed.2009.10.001
118. Nakajima N, Sato Y, Katano H, Hasegawa H, Kumasaka T, Hata S, et al. Histopathological and immunohistochemical findings of 20 autopsy cases with 2009 H1N1 virus infection. Mod Pathol (2012) 25(1):1–13. doi:10.1038/modpathol.2011.125
119. Hoeve MA, Nash AA, Jackson D, Randall RE, Dransfield I. Influenza virus A infection of human monocyte and macrophage subpopulations reveals increased susceptibility associated with cell differentiation. PLoS One (2012) 7(1):e29443. doi:10.1371/journal.pone.0029443
120. Yu WC, Chan RW, Wang J, Travanty EA, Nicholls JM, Peiris JS, et al. Viral replication and innate host responses in primary human alveolar epithelial cells and alveolar macrophages infected with influenza H5N1 and H1N1 viruses. J Virol (2011) 85(14):6844–55. doi:10.1128/JVI.02200-10
121. Smed-Sorensen A, Chalouni C, Chatterjee B, Cohn L, Blattmann P, Nakamura N, et al. Influenza A virus infection of human primary dendritic cells impairs their ability to cross-present antigen to CD8 T cells. PLoS Pathog (2012) 8(3):e1002572. doi:10.1371/journal.ppat.1002572
122. Baharom F, Thomas OS, Lepzien R, Mellman I, Chalouni C, Smed-Sorensen A. Visualization of early influenza A virus trafficking in human dendritic cells using STED microscopy. PLoS One (2017) 12(6):e0177920. doi:10.1371/journal.pone.0177920
123. Aldridge JRJ, Moseley CE, Boltz DA, Negovetich NJ, Reynolds C, Franks J, et al. TNF/iNOS-producing dendritic cells are the necessary evil of lethal influenza virus infection. Proc Natl Acad Sci U S A (2009) 106(13):5306–11. doi:10.1073/pnas.0900655106
124. Thomas JM, Pos Z, Reinboth J, Wang RY, Wang E, Frank GM, et al. Differential responses of plasmacytoid dendritic cells to influenza virus and distinct viral pathogens. J Virol (2014) 88(18):10758–66. doi:10.1128/JVI.01501-14
125. Vanders RL, Gibson PG, Murphy VE, Wark PA. Plasmacytoid dendritic cells and CD8 T cells from pregnant women show altered phenotype and function following H1N1/09 infection. J Infect Dis (2013) 208(7):1062–70. doi:10.1093/infdis/jit296
126. Wu W, Booth JL, Duggan ES, Wu S, Patel KB, Coggeshall KM, et al. Innate immune response to H3N2 and H1N1 influenza virus infection in a human lung organ culture model. Virology (2010) 396(2):178–88. doi:10.1016/j.virol.2009.10.016
127. Silvin A, Yu CI, Lahaye X, Imperatore F, Brault J-B, Cardinaud S, et al. Constitutive resistance to viral infection in human CD141+ dendritic cells. Sci Immunol (2017) 2(13):1–12. doi:10.1126/sciimmunol.aai8071
128. Banchereau J, Briere F, Caux C, Davoust J, Lebecque S, Liu YJ, et al. Immunobiology of dendritic cells. Annu Rev Immunol (2000) 18:767–811. doi:10.1146/annurev.immunol.18.1.767
129. Merad M, Sathe P, Helft J, Miller J, Mortha A. The dendritic cell lineage: ontogeny and function of dendritic cells and their subsets in the steady state and the inflamed setting. Annu Rev Immunol (2013) 31:563–604. doi:10.1146/annurev-immunol-020711-074950
130. Pulendran B. The varieties of immunological experience: of pathogens, stress, and dendritic cells. Annu Rev Immunol (2015) 33:563–606. doi:10.1146/annurev-immunol-020711-075049
131. Randolph GJ, Angeli V, Swartz MA. Dendritic-cell trafficking to lymph nodes through lymphatic vessels. Nat Rev Immunol (2005) 5(8):617–28. doi:10.1038/nri1670
132. Waithman J, Mintern JD. Dendritic cells and influenza A virus infection. Virulence (2012) 3(7):603–8. doi:10.4161/viru.21864
133. Clark GJ, Kupresanin F, Fromm PD, Ju X, Muusers L, Silveira PA, et al. New insights into the phenotype of human dendritic cell populations. Clin Transl Immunol (2016) 5(1):e61. doi:10.1038/cti.2015.40
134. Perrone LA, Plowden JK, Garcia-Sastre A, Katz JM, Tumpey TM. H5N1 and 1918 pandemic influenza virus infection results in early and excessive infiltration of macrophages and neutrophils in the lungs of mice. PLoS Pathog (2008) 4(8):e1000115. doi:10.1371/journal.ppat.1000115
135. Idoyaga J, Fiorese C, Zbytnuik L, Lubkin A, Miller J, Malissen B, et al. Specialized role of migratory dendritic cells in peripheral tolerance induction. J Clin Invest (2013) 123(2):844–54. doi:10.1172/JCI65260
136. GeurtsvanKessel CH, Bergen IM, Muskens F, Boon L, Hoogsteden HC, Osterhaus AD, et al. Both conventional and interferon killer dendritic cells have antigen-presenting capacity during influenza virus infection. PLoS One (2009) 4(9):e7187. doi:10.1371/journal.pone.0007187
137. Cella M. Plasmacytoid monocytes migrate to inflamed lymph nodes and produce large amounts of type I interferon. Nat Med (1999) 5(8):919–23. doi:10.1038/11360
138. Pulendran B, Maddur MS. Innate immune sensing and response to influenza. Curr Top Microbiol Immunol (2015) 386:23–71. doi:10.1007/82_2014_405
139. Chen X, Liu S, Goraya MU, Maarouf M, Huang S, Chen JL. Host immune response to influenza A virus infection. Front Immunol (2018) 9(320):1–13. doi:10.3389/fimmu.2018.00320
140. Ascough S, Paterson S, Chiu C. Induction and subversion of human protective immunity – contrasting influenza and respiratory syncytial virus. Front Immunol (2018) 9(323):1–23. doi:10.3389/fimmu.2018.00323
141. Miyauchi K. Helper T cell responses to respiratory viruses in the lung: development, virus suppression, and pathogenesis. Viral Immunol (2017) 30(6):421–30. doi:10.1089/vim.2017.0018
142. Gan H, Hao Q, Idell S, Tang H. Transcription factor Runx3 is induced by influenza A virus and double-strand RNA and mediates airway epithelial cell apoptosis. Sci Rep (2015) 5:17916. doi:10.1038/srep17916
143. Kim S, Kim MJ, Park DY, Chung HJ, Kim CH, Yoon JH, et al. Mitochondrial reactive oxygen species modulate innate immune response to influenza A virus in human nasal epithelium. Antiviral Res (2015) 119:78–83. doi:10.1016/j.antiviral.2015.04.011
144. McAuley JL, Corcilius L, Tan HX, Payne RJ, McGuckin MA, Brown LE. The cell surface mucin MUC1 limits the severity of influenza A virus infection. Mucosal Immunol (2017) 10(6):1581–93. doi:10.1038/mi.2017.16
145. Shin N, Pyo CW, Jung KI, Choi SY. Influenza A virus PB1-F2 is involved in regulation of cellular redox state in alveolar epithelial cells. Biochem Biophys Res Commun (2015) 459(4):699–705. doi:10.1016/j.bbrc.2015.03.010
146. Oughton M, Dascal A, Laporta D, Charest H, Afilalo M, Miller M. Evidence of viremia in 2 cases of severe pandemic influenza A H1N1/09. Diagn Microbiol Infect Dis (2011) 70(2):213–7. doi:10.1016/j.diagmicrobio.2010.12.013
147. Sobata R, Matsumoto C, Igarashi M, Uchida S, Momose S, Hino S, et al. No viremia of pandemic (H1N1) 2009 was demonstrated in blood donors who had donated blood during the probable incubation period. Transfusion (2011) 51(9):1949–56. doi:10.1111/j.1537-2995.2011.03109.x
148. Jamieson AM, Yu S, Annicelli CH, Medzhitov R. Influenza virus-induced glucocorticoids compromise innate host defense against a secondary bacterial infection. Cell Host Microbe (2010) 7(2):103–14. doi:10.1016/j.chom.2010.01.010
149. Nita-Lazar M, Banerjee A, Feng C, Amin MN, Frieman MB, Chen WH, et al. Desialylation of airway epithelial cells during influenza virus infection enhances pneumococcal adhesion via galectin binding. Mol Immunol (2015) 65(1):1–16. doi:10.1016/j.molimm.2014.12.010
150. Baharom F, Thomas S, Bieder A, Hellmer M, Volz J, Sandgren KJ, et al. Protection of human myeloid dendritic cell subsets against influenza A virus infection is differentially regulated upon TLR stimulation. J Immunol (2015) 194(9):4422–30. doi:10.4049/jimmunol.1402671
151. Levy O, Zarember KA, Roy RM, Cywes C, Godowski PJ, Wessels MR. Selective impairment of TLR-mediated innate immunity in human newborns: neonatal blood plasma reduces monocyte TNF-α induction by bacterial lipopeptides, lipopolysaccharide, and imiquimod, but preserves the response to R-848. J Immunol (2004) 173(7):4627–34. doi:10.4049/jimmunol.173.7.4627
152. Marr N, Wang TI, Kam SH, Hu YS, Sharma AA, Lam A, et al. Attenuation of respiratory syncytial virus-induced and RIG-I-dependent type I IFN responses in human neonates and very young children. J Immunol (2014) 192(3):948–57. doi:10.4049/jimmunol.1302007
153. Molony RD, Nguyen JT, Kong Y, Montgomery RR, Shaw AC, Iwasaki A. Aging impairs both primary and secondary RIG-I signaling for interferon induction in human monocytes. Sci Signal (2017) 10(509):1–11. doi:10.1126/scisignalaan2392
Keywords: emerging, virus, influenza, respiratory, monocyte, dendritic cell, macrophage
Citation: Vangeti S, Yu M and Smed-Sörensen A (2018) Respiratory Mononuclear Phagocytes in Human Influenza A Virus Infection: Their Role in Immune Protection and As Targets of the Virus. Front. Immunol. 9:1521. doi: 10.3389/fimmu.2018.01521
Received: 30 March 2018; Accepted: 19 June 2018;
Published: 03 July 2018
Edited by:
Francesca Chiodi, Karolinska Institutet (KI), SwedenReviewed by:
Elisa Vicenzi, San Raffaele Hospital (IRCCS), ItalyNicolas Ruffin, Institut Curie, France
Copyright: © 2018 Vangeti, Yu and Smed-Sörensen. This is an open-access article distributed under the terms of the Creative Commons Attribution License (CC BY). The use, distribution or reproduction in other forums is permitted, provided the original author(s) and the copyright owner are credited and that the original publication in this journal is cited, in accordance with accepted academic practice. No use, distribution or reproduction is permitted which does not comply with these terms.
*Correspondence: Anna Smed-Sörensen, anna.smed.sorensen@ki.se