- 1Laboratory of Experimental Feto-Maternal Medicine, Department of Obstetrics and Fetal Medicine, University Medical Center Hamburg-Eppendorf, Hamburg, Germany
- 2Developmental Neurophysiology, Institute of Neuroanatomy, University Medical Center Hamburg-Eppendorf, Hamburg, Germany
- 3Institute of Medical Psychology, Berlin Institute of Health, Charité-Universitätsmedizin Berlin, Corporate Member of Freie Universität Berlin, Humboldt-Universität zu Berlin, Berlin, Germany
- 4Development, Health, and Disease Research Program, University of California, Irvine, Orange, CA, United States
Milestones of brain development in mammals are completed before birth, which provide the prerequisite for cognitive and intellectual performances of the offspring. Prenatal challenges, such as maternal stress experience or infections, have been linked to impaired cognitive development, poor intellectual performances as well as neurodevelopmental and psychiatric disorders in the offspring later in life. Fetal microglial cells may be the target of such challenges and could be functionally modified by maternal markers. Maternal markers can cross the placenta and reach the fetus, a phenomenon commonly referred to as “vertical transfer.” These maternal markers include hormones, such as glucocorticoids, and also maternal immune cells and cytokines, all of which can be altered in response to prenatal challenges. Whilst it is difficult to discriminate between the maternal or fetal origin of glucocorticoids and cytokines in the offspring, immune cells of maternal origin—although low in frequency—can be clearly set apart from offspring's cells in the fetal and adult brain. To date, insights into the functional role of these cells are limited, but it is emergingly recognized that these maternal microchimeric cells may affect fetal brain development, as well as post-natal cognitive performances and behavior. Moreover, the inheritance of vertically transferred cells across generations has been proposed, yielding to the presence of a microchiome in individuals. Hence, it will be one of the scientific challenges in the field of neuroimmunology to identify the functional role of maternal microchimeric cells as well as the brain microchiome. Maternal microchimeric cells, along with hormones and cytokines, may induce epigenetic changes in the fetal brain. Recent data underpin that brain development in response to prenatal stress challenges can be altered across several generations, independent of a genetic predisposition, supporting an epigenetic inheritance. We here discuss how fetal brain development and offspring's cognitive functions later in life is modulated in the turnstile of prenatal challenges by introducing novel and recently emerging pathway, involving maternal hormones and immune markers.
Introduction
The vertical transmission of maternal immune and endocrine markers is increasingly recognized to modulate fetal neurodevelopment and future mental health of the offspring. Emerging evidence arising from observational studies in humans reveals that prenatal environmental challenges such as maternal distress perception and infections are associated with an impaired fetal neurodevelopment and increased risk for neurological or psychiatric disorders later in life (1–6). Insights into the underlying mechanisms and pathogenesis of prenatally programmed poor mental health are increasingly emerging. It is well known that neurodevelopment results from the interaction of genetic, epigenetic and environmental factors, through which proliferation, migration of neural progenitor cells and establishment of neuronal circuits are modulated. Disruptions of these neurodevelopmental pathways may subsequently affect future brain function, as reflected by cognitive and intellectual impairment and increased the risk for neurodevelopmental and psychiatric disorders later in life (7).
Here, we compile the currently available evidence arising from observational studies supporting the concept of a developmental origin of brain disorders. We further outline cornerstones of brain development in mice and humans and discuss the effect of prenatal challenges, primarily maternal distress and infections, on maternal immune-endocrine adaptation to pregnancy. Lastly, we introduce novel concepts on how an altered maternal immune-endocrine adaptation to pregnancy can impact on offspring's brain development and subsequent mental health.
Developmental Origin of Neurological Dysfunctions and Psychiatric Disorders
Pregnancy is characterized by significant adaptational processes of the maternal immune and endocrine system in order to ensure its progression until term, which is required for adequate fetal development. These adaptational processes are highly responsive and vulnerable to challenges, such as high maternal stress perception or maternal infections. In this context, chronic stress states (e.g., depression or anxiety) affect approx. 10–15% of pregnant women worldwide (8). Moreover, negative life events may pose a significant threat to maternal wellbeing during pregnancy. Table 1 provides a comprehensive overview of published evidence largely arising from observational studies that reveal a significant association between various types of maternal distress perception to which the mother was exposed to at specific gestational periods and the risk for psychiatric disorders in the offspring later in life, i.e., during childhood or adolescence. Disorders observed in the offspring include autism spectrum disorder (ASD) (9), depressive symptoms (10–12), anxiety, borderline personality disorder, eating disorders (23) and attention-deficit/hyperactivity disorder (ADHD) (3, 14–20, 22, 45). Interestingly, whilst a sex-specific risk is well known for psychiatric disorders, only very few studies paid attention whether prenatal stress perception skews the risk for such disorders in a sex-specific way. One study describes a sex-bias for ADHD upon prenatal stress, mirrored by a higher risk in daughters (20). Moreover, the timing of the prenatal challenge may be pivotal, as the risk for neurodevelopmental diseases appears to be differentially affected by the trimester of exposure (Table 1). In fact, surges of maternal IL-6 levels during the third trimester—which may result from distress or infections—showed a strongest impact on working memory performance in children. These behavioral changes were associated with alterations of brain regions tightly associated with working memory, as identified by functional MRI (46).
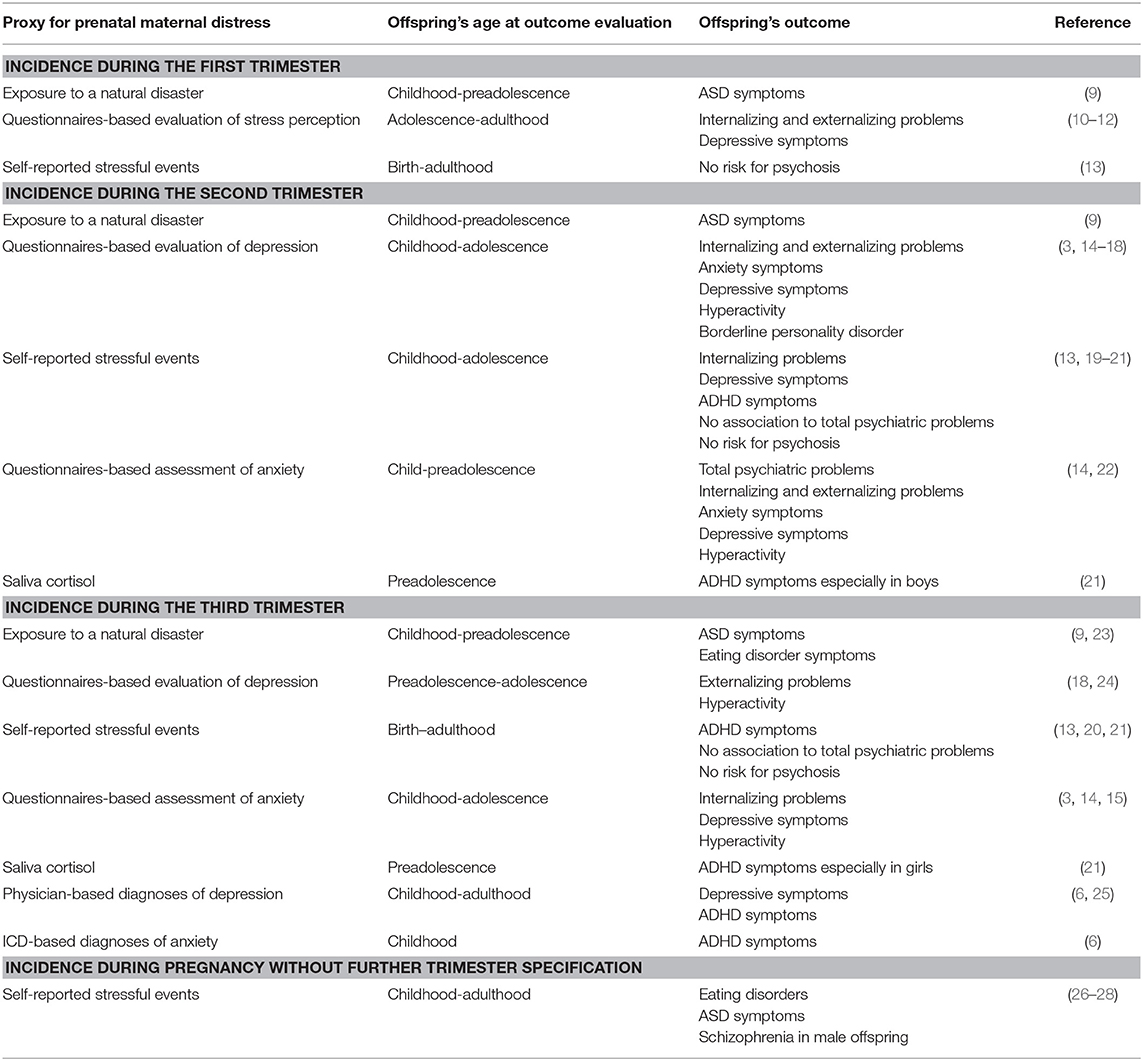
Table 1. Summary of human studies examining the effect of prenatal maternal distress on offspring's mental health.
Besides high stress perception, maternal infection during pregnancy can interfere with fetal neurodevelopment and increase the risk for neurological dysfunctions and psychiatric disorders in the offspring (Table 2). Here, most of the studies focus on the distinct pathogens that have led to maternal infection during pregnancy (1, 35, 37, 42, 43). For example, maternal infection with influenza A or B virus has been associated with an increased risk for developing schizophrenia, although findings between studies are highly ambiguous and hence, hotly debated. Some studies report that influenza infection during the first trimester may trigger the risk for schizophrenia, whilst such effect could not be confirmed in studies focusing on infection at mid to late pregnancy (29–31, 47). The latter includes observations arising from Scandinavian registry analyses, where the query for prenatal influenza infection was solidly based on International Classification of Diseases (ICD)-coded diagnoses (48, 49). A recent meta-analysis confirms that evidence is insufficient to support gestational influenza as a risk factor for schizophrenia and bipolar disorder in the offspring (50). Besides these viral infections, also bacterial infections during pregnancy have been linked to an increased risk for schizophrenia in the offspring in adulthood (36, 44). For instance, 13% of all children surviving the maternal listeria monocytogenes infection were suffering from meningitis in early childhood (38) and this in return is significantly associated with developing schizophrenia and psychotic episodes later in life (39).
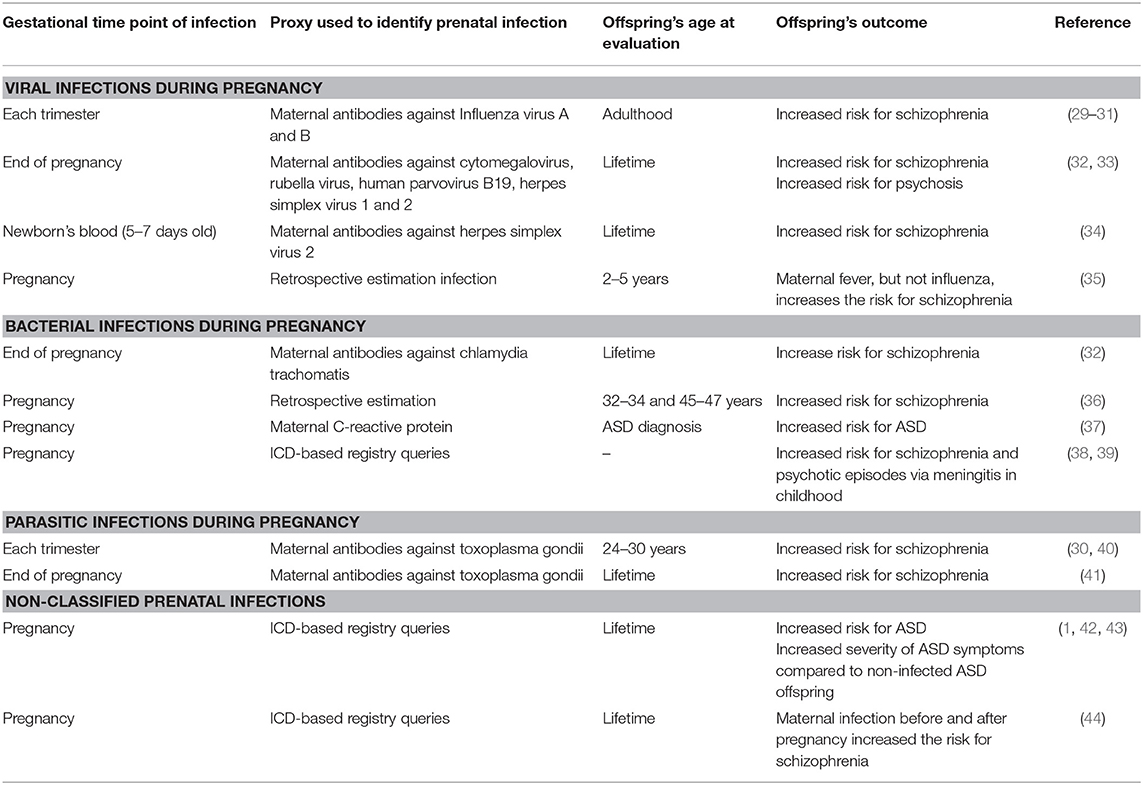
Table 2. Overview of studies examining the effects prenatal infection and related maternal immune activation on offspring's mental health in human.
Milestones of Brain Development in Mice and Humans
Given that some of the observational studies summarized above highlight that the time point at which challenges occur prenatally may be crucial to trigger changes in fetal neurodevelopment, we here provide a brief summary of key aspects of fetal brain development that commence or are completed at distinct periods of gestation. We also include the brain development of mice, as mouse models have become pivotal in understanding how prenatal challenges affect neurodevelopment and brain functions later in life, as outlined in the subsequent paragraphs.
In humans and mice, brain development shows similar developmental processes (Figure 1). Noteworthy, sex-specific differences occur as a result of a faster cerebral maturation in girls (55), which leaves boys at higher risk for challenges-induced disruptions due to the greater window of vulnerability. Some developmental steps continue after birth in mice, which are already completed at birth in humans. This reduces the time window in mice during which vertically transferred maternal biological mediators can impact on offspring's brain development, a confounder which should be considered when discussing the biological relevance of findings on prenatal challenges in mice. Hence, when evaluating the impact of maternal markers on fetal development in mice, research endeavor should focus on milestones that are completed prior to birth, such as neurulation, neuronal migration and microglia invasion, as well as synaptogenesis and neurogenesis, the latter being largely completed at birth.
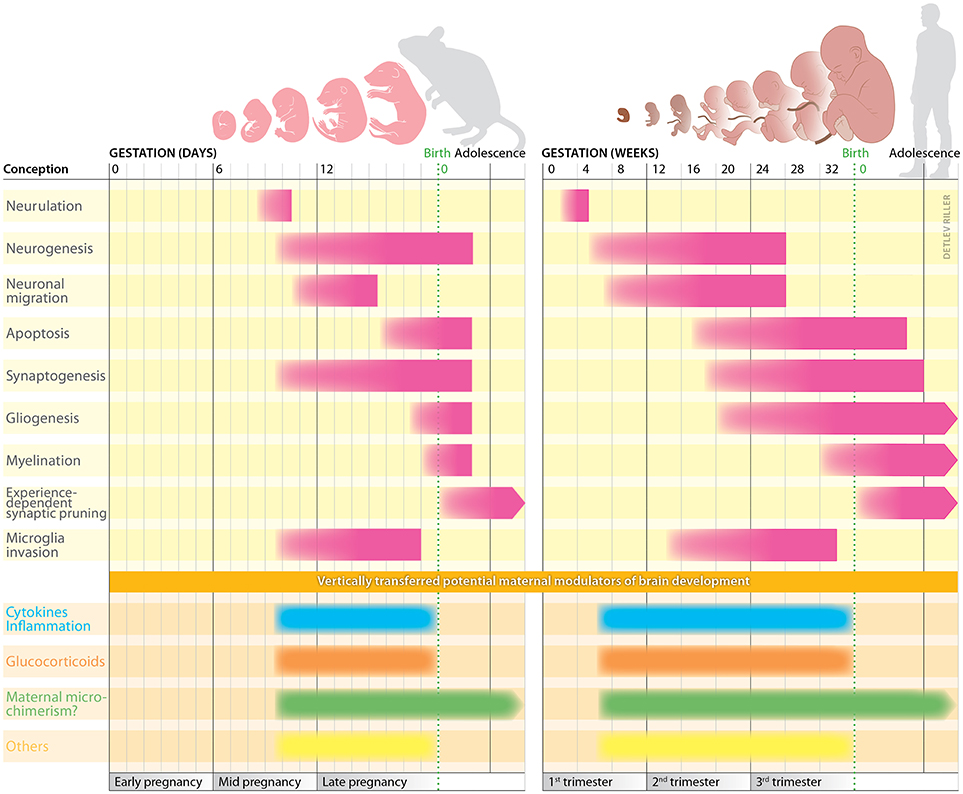
Figure 1. Milestones of brain development in mice and humans. In both species, brain development commences with neurulation, a process creating the neural tube. This provides the prerequisite for the subsequent production of neuron from neural stem cells, a process defined as neurogenesis. Early during human development, at gestation week 4, the anterior part of the neural tube begins to form into distinct regions. The forebrain, midbrain and hindbrain are defined as the anterior part; the spinal cord is located at the posterior part. Two weeks later, the neural tube can be clearly divided into the brain regions that are present at birth. Some of the previously produced neurons now start to migrate to distinct brain regions, a process that continues until approx. week 26. Earlier by week 11, the cerebrum has developed—more rapidly than other structures—and largely covers the entire brain, except cerebellum and medulla oblongata. Due to its progressive development within the cranium, the cerebrum is forced to convolve itself resulting in gyri and sulci (51). During the second trimester of pregnancy, several processes start to define brain connectivity. These include synaptogenesis, gliogenesis, and apoptosis. Simultaneously, microglial cells invasion begins. By mid-third trimester, the fine-tuning of neuronal connectivity starts with proliferation of myelin sheaths throughout the neurons of the central nervous system (52). Shortly after birth, the previously established neuronal connections are reduced based on neuronal activity, meaning a reduction of neuronal connections to the ones often used. Since murine gestation is much shorter compared to human pregnancy, some developmental steps continue to proceed after birth. In mice, neural development begins during mid-pregnancy, followed by neurulation and formation of the neural tube (53). Subsequently, production of neurons, their migration and the formation of synapses occur almost simultaneously. Also, yolk sac-derived microglial cells invade the fetus starting on day 9 of pregnancy (54). The developmental milestones underlying brain development in mice and humans are highly susceptible to challenges and can be modulated by maternal markers vertically transferred during pregnancy. Hereby, the time point and intensity of the challenges clearly determine the impact and damage they may cause.
Effect of Prenatal Challenges on Maternal Immune-Endocrine Adaptation to Pregnancy
In mice and humans, healthy brain development is crucially dependent on an endocrine and immunological homeostasis of the mother-fetus dyad. Here, the balance between pro- and anti-inflammatory cytokines is crucial, as neurogenesis, migration, differentiation and apoptosis are well known to be responsive to cytokine challenges (Table 3). Besides cytokines, chemokines are also important in modulating neurodevelopment and the risk for psychiatric diseases. Since these interactions have been addressed in recent reviews (80–82), we refrain from including them here. Similarly, glucocorticoids, which are initially largely maternally derived during gestation in the fetus, can interfere with fetal brain development in mice and humans. Moreover, additional factors such as maternal immune cells can affect fetal brain development. Prenatal stimuli and challenges, including maternal stress perception and infection, have been show to interfere with the endocrine and immunological hemostasis in the mother. This includes the activation of the sympathetic nervous system and the hypothalamus-pituitary-adrenal (HPA) axis, subsequently leading to an excess secretion and availability of free, biologically active glucocorticoids (83, 84). Pregnancy itself is considered to be a state of “hypercortisolism,” which is an essential requirement to meet the maternal demand for increased metabolism and energy generation. The fetus is also critically dependent on the transfer of maternal glucocorticoids, which is controlled for by placental enzymes such as 11ß-Hydroxysteroid-Dehydrogenase (11ß-HSD)-1 and -2 (83, 84). Maternal glucocorticoids ensure structural and functional development of fetal organs, as the fetus is not capable of producing glucocorticoids until late in development. However, elevated glucocorticoid concentrations in the context of maternal stress may negatively impact on fetal brain development (83).
Besides the effect of prenatal challenges on maternal glucocorticoid levels, the maternal immune response may also be skewed toward inflammation in the context of stress in human pregnancy or infection (85, 86). Similarly in mice, maternal stress challenges have been shown to increase levels of pro-inflammatory cytokines in dams (87) and decrease tolerogenic markers such as CD4+ regulatory T cells (88). Similarly, prenatal infection e.g., with influenza A virus in mice leads to an increased type 1 response, along with an increased production of pro-inflammatory cytokines, compared to non-infected pregnant mice (89). Equally, the use of “danger signals” such as lipopolysaccharide to induce an inflammatory response in pregnant mice resulted in a collapse of immune tolerance toward the fetus (90).
Maternal cytokines, glucocorticoids as well as maternal immune cells can cross the placenta. Whilst it is difficult to determine if cytokines are maternally derived or produced by the fetus, maternal immune cells can be clearly identified in the offspring. These maternal microchimeric cells can persist in the offspring long after birth (91). Hence, opposed to the vertical transfer of maternal cytokines and glucocorticoids, maternal microchimeric cells may be capable to continuously modulate brain function in the offspring even after birth.
Impact of Altered Maternal Immune-Endocrine Adaptation to Pregnancy and Offspring's Brain Development and Mental Health
The vertical transfer of maternal immune and endocrine markers is increasingly recognized to modulate fetal neurodevelopment and future mental health of the offspring. As mentioned above, the impact of cytokines on fetal brain development has been well studied and it is widely accepted that milestones of physiological fetal brain development are modulated by cytokines (Table 3). Hence, exposure to an imbalanced cytokine response during fetal life may disturb fetal brain development, thereby increasing the risk for neurodevelopmental disorders (92).
In mice, fetal exposure to an altered, pro-inflammatory maternal cytokine response can affect brain morphology, mirrored by e.g., an increased pyramidal cell density or reduced neurogenesis (93–96). Similarly, prenatal maternal treatment with the immunostimulant polyinosinic:polycytidylic acid (poly I:C) to mirror some effects of a viral infection has been shown to result in reduced axonal size, myelin thickness and cortical volume of the hippocampus and amygdala in rodent offspring (97, 98). A general maternal immune activation during pregnancy has also been shown to cause presynaptic deficits in hippocampus (99), pro-inflammatory activation in hippocampal microglia (100) or an increase of microglial cell frequencies in the fetal brain (101). Hence, a wealth of studies has shown that maternal immune activation during pregnancy adversely affects fetal brain development on multiple levels. Yet, it is difficult to comprehensively pinpoint distinct pathways, as the studies performed to date have been rather diverse with regard to the species, the gestational time point or the cause of maternal immune activation (102). In humans, a great deal of research focused on the role of maternal levels of interleukin-6 as a proxy for a prenatal inflammatory challenge. Key observations include that maternal IL-6 levels affects offspring's structural and functional connectivity already at the time of birth (103), delaying the development of sensory and cognitive processing (46, 104).
Similar to cytokines, fetal exposure to elevated concentrations of maternal glucocorticoids has been proposed to exert long-lasting, partly sex-specific effects on offspring's brain morphology and function in rodents but also in humans. This includes decreased dendritic morphology and neuronal volume in hippocampi of both sexes in mice, whereas an increased dendritic branching has been detected only in females (105). Another study using mouse models reports an increased spine density, dendritic length and other morphological features in pyramidal neurons of prenatally stressed males, which was decreased in females (106).
In humans, elevated maternal cortisol concentrations in early pregnancy have been associated with larger amygdala volumes, an altered neural connectivity, along with affective symptoms and internalizing problems in girls (107). Interestingly, moderately elevated maternal cortisol levels in late pregnancy could be associated with greater cortical thickness primarily in frontal regions and enhanced cognitive performance in children (108). These findings point to the importance of considering the moderating role of sex and timing of exposure to glucocorticoids during pregnancy.
Since the experimental or study designs differ with regard to stress paradigms/glucocorticoids applications, time point of prenatal interventions or postnatal analyses, species and read-out parameter (109–111), it is not yet possible to comprehensively summarize the outcome of these studies beyond the statement that prenatal stress and related glucocorticoid surges induce sex- and brain area-specific differences in neuronal complexity and neurogenesis. This may subsequently lead to altered cognitive functions later in life.
Microglial cells, which are the resident macrophages of the CNS, have also been extensively studied in response to prenatal stress or prenatal glucocorticoid application. Due to their phagocytic phenotype, microglia have a functional role in remodeling, shaping and pruning of synapses (112). And indeed, prenatal corticosterone application increased the microglial density in the embryonic brain overall and promoted their amoeboid phenotype (113). Interestingly, this result was supported by isolating and culturing P1-2 microglial cells, showing that they are more likely to acquire an amoeboid phenotype (114). At postnatal age, stress increased the total glia cell number in the hippocampus of female but not male offspring (115). One study showed an increased number of microglia cells in the dentate gyrus, suggesting an adverse effect on neurogenesis and simultaneously no changes in the CA1 of the hippocampus (116, 117). The early stress exposure seems to support phagocytic microglia function in the early brain and potentially stimulate their activity throughout early murine lifetime. Their role as a potential mediator between prenatal stress and early neurobiological changes remains vague, especially in other important brain areas such like the PFC.
The barrier between periphery and the brain is an essential interface for communication between both compartments. Even though the blood brain barrier is supposed to be well established during early development, its function in stress and cytokine-related diseases is poorly investigated. However, its permeability is a key component in how maternal markers may influence the offspring's brain development. This topic is excellently described in an comprehensive review elsewhere [see review, (118)] and thus not explained here.
In Figure 2, we depict key effects of cytokines/inflammation and glucocorticoids on microglia cells and neurons in the prefrontal cortex, hippocampus and amygdala of the fetal brain. We deliberately focused on these specific brain regions due to their specific relevance for the mental health problems that have been described in the context of maternal stress and infection (122).
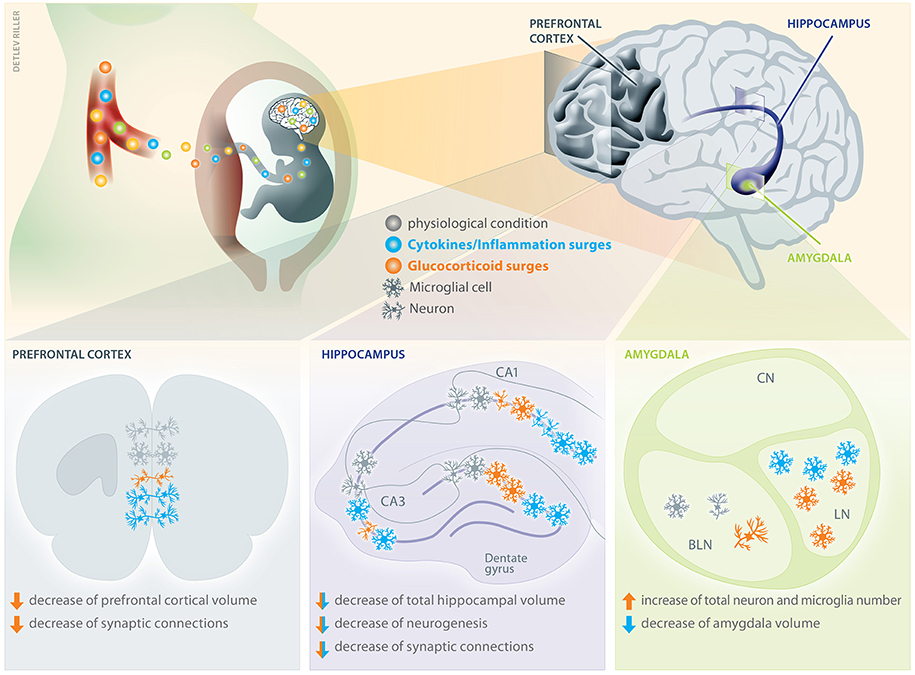
Figure 2. Consequences of cytokine and glucocorticoid surges on distinct areas of the offspring's brain. Maternal cytokines and glucocorticoids can transplacentally cross into the fetus and differentially affect offspring's brain development by interfering with e.g., cell differentiation, axonal growth, and synaptic connectivity. The brain regions depicted here are of pivotal relevance for mental health due to their involvement in cognitive functions. Compared to physiological conditions, prenatal surges of maternal cytokines increase the number of neuronal connections in a subdivision of the prefrontal cortex, whereas glucocorticoids decreases them (105, 119). Additionally, prenatal glucocorticoid exposure decreases prefrontal cortical volume. In the hippocampus, an increase of cytokines is known to increase the number of microglial cells in the corn ammonis area 1 (CA1), whilst simultaneously reducing dendritic arborizations and neuronal complexity (111). Similarly, in the CA3 and dentate gyrus (DG) of the hippocampus, the microglia density increases after cytokine exposure. Also glucocorticoid surges deteriorate the neuronal complexity in CA1 and CA3 (109, 110) and have been shown to increases the number of microglia in DG. Both, cytokines and glucocorticoids can decrease total hippocampal volume, neurogenesis and synaptic connections. Prenatal cytokine surges can also decrease the amygdala volume, whereas glucocorticoids have been shown to increase the number of neurons and microglia. There is no evidence that the central nucleus (CN) of the amygdala is affected, but an increased microglia density after prenatal cytokine and glucocorticoid exposure has been detected in the lateral nucleus (LN) (120, 121). Contrarily, an increased number of neuronal connections and microglia was detectable upon glucocorticoid challenge in the basolateral nucleus (BLN).
Besides the effect of glucocorticoids and cytokines on neurogenesis, synaptogenesis, axon growth etc., these mediators can also exert indirect effects on these fundamental processes of fetal brain development, i.e., by altering the concentration or availability of neurotransmitters. The primary excitatory neurochemical in the central nervous system, glutamate, which acts through different types of metabotropic glutamate receptors (mGluR) has been investigated in a number of studies (123–125). Indeed, a variant of the Kozak sequence of exon 1 of the mGluR 3 could be associated with e.g., bipolar affective disorder (126). Moreover, a reduced expression of mGluR receptors in the hippocampus has been observed in response to prenatal stress (127–129). Brain functions such as mood, satiety, sleep, body temperature and nociception are also critically dependent on the serotonergic system, and prenatal challenges have been shown to interfere with the rate of serotonin synthesis (130), the number of serotonin positive cells (131) and its receptor density (132) in the offspring's brain. Further, the cholinergic system is important in regulating brain function (e.g., learning and short-term memory) (133) that has been shown to be impacted in the offspring in the context of maternal stress and infection during pregnancy. Some evidence from rodent models support the notion that prenatal stress challenges interfere with the release of cholinergic factors in the offspring, such as acetylcholine (134), whereby changes of behavior have not been evaluated in this study.
Introducing a Novel Pathway in the Developmental Origin of Neurocognitive Functions and Psychiatric Disorders: the Impact of Maternal Microchimeric Cells
Maternal microchimeric cells, which are vertically transferred from mother to fetus during pregnancy and—at least in part—during lactation (135–137), can be detected in the offspring's brain during fetal and adult life. Hence, maternal microchimeric cells have the potential to modulate brain development and the risk for neurodevelopmental disorders. To date, no insights are available on the role of maternal microchimeric cells in brain development and their potential ability to tailor the nervous system individually. Moreover, brain structures where maternal microchimeric cells may abundantly populate have not yet been identified. However, since maternal microchimeric cells have been identified as maternal immune cells of the innate and adaptive immune response, they hold the strong potential to shape neurons by acquiring a phagocytic phenotype, akin to offspring's microglial cells. Future studies should aim at elucidating the functional role of maternal microchimeric cells on the developing brain and to understand whether they may modulate the risk for brain-related disorders.
Given the plethora of mediators that may be functionally involved in shaping brain development and subsequent function, whilst being altered upon maternal stress, infection and other prenatal conditions (138), it is not surprising that we are far from fully understanding the developmental origin of neurocognitive functions and brain disorders. Also, it seems unlikely that single mediators determine a clear-cut “good or bad” outcome. It is more likely that the mediators we here proposed act synergistically in modulating brain development and subsequent function with an advantageous or disadvantageous outcome. Hypothetically, this synergistic cross talk could involve the expression of glucocorticoid receptor on maternal microchimeric cells or the release of cytokines from maternal microchimeric cells entering the fetal brain. The longevity of such cells would surpass the short-term effect that could result from the potential transplacental transfer of cytokines itself, as cytokines are rapidly metabolized.
Functional Impact of Vertically Transferred Maternal Markers on the Developing Brain
In response the environmental challenges, altered levels of maternal markers that cross the placental barrier may affect the developing brain by inducing epigenetic alterations of somatic cells (139–142) Persistent epigenetic differences triggered by the prenatal exposure to stress challenges in humans include increased and decreased methylation of insulin-like growth factor 2 or the glucocorticoid receptor gene (NR3C1) in brain cells, depending on the timing of exposure (143, 144). Prenatal distress has been associated with hyper- as well as demethylation of specific regulatory sites in key genes involved in stress processing, such as the glucocorticoid receptor (144–146). Similarly, findings arising from mouse models on maternal immune activation during pregnancy include the observations of a hypoacetlyation of e.g., genes modulating neuronal development, synaptic transmission and immune signaling in the cortex region in exposed offspring (147), as well as sex-specific DNA hypomethylation in the hypothalamus of females (148), specifically affecting the promoter region of methyl CpG-binding protein 2, which is associated with neurodevelopmental disorders (149). Interestingly, prenatal immune activation in mice could be linked to hypermethylation of glutamic acid decarboxylase 1 and 2 in the brain (150), associated with altered behavior.
Strikingly, mouse models have revealed that alterations of brain function can be passed on to the next generations (151), suggesting that underlying epigenetic alterations triggered by prenatal challenges may be intergenerationally inherited. This notion could provide an explanation for the increasing incidence of behavioral disorders (152). Moreover, it implies that exposure of the mother to environmental challenges during pregnancy may not only directly interfere with fetal brain development, but also affects fetal primordial germ cells (153), which may subsequently interfere with brain development in the generation of grandchildren. Primordial germ cells undergo sequential epigenetic events, which are distinct from fetal somatic cells, hereby preserving the plasticity required for the generation of gametes (154–157). Once the offspring reaches adulthood and such oocytes are fertilized, the resulting zygote again undergoes significant epigenetic reprogramming, which includes the demethylation of the maternal and paternal genome, followed by a genome-wide de novo methylation (158).
Animal data indicate the possibility of transmission of behavioral traits mediated by epigenetic modifications through the maternal, as well as paternal germ line (159–162), implying the generation of oocytes and sperm may be equally affected. Intriguingly, how epigenetic changes induced by environmental challenges can be maintained throughout the multiple epigenetic reprogramming events physiologically occurring during reprogramming of primordial germ cells and the zygote is still largely elusive. Insights from mouse studies provide a first glimpse, as they reveal that certain regions of the genome, i.e., differentially methylated regions, are resistant to zygotic reprogramming (158). However, future research is required to identify pathways of intergeneration epigenetic inheritance of altered brain function in the offspring, aiming also to differentiate between de novo acquired epigenetic alterations from those inherited through the germ line. Besides such intergenerational inheritance of epigenetic marks, the possibility of transgenerational inheritance of epigenetic changes to one further generation of descent, the great-grand generation, has been considered. The primordial germ cells of the forth generation would not have been directly exposed to the environmental challenges or mediators released by the great-grandmother during pregnancy. However, to date, convincing evidence of transgenerational inheritance of epigenetic marks is only available from botany research using plants, whilst confirmation in mammals is somewhat elusive (163).
Besides such epigenetic pathways, the brain as target tissue for prenatal challenges may be affected in its electrical synchrony, which is defined as the coordinated oscillatory activity and neural firing rate between connected brain areas. These links are a prerequisite to execute cognitive tasks (164, 165). Interestingly, prenatal exposure to maternal inflammation or stress impairs oscillatory synchronicity (166), which commenced already during developmental stages in a mouse model of neuropsychiatric disorders (167–169) and affected spatial memory tasks (170, 171).
Outlook
Higher cognitive functions such as planning, self-regulation, memory, learning, and emotional processes result from a complex, tailored, and precisely shaped large-scale communication of neuronal networks (172). These neuronal networks begins to develop prenatally and disturbances of such developing neural systems during pregnancy can disrupt brain development via the vertical transfer of maternal markers, such as cytokines, glucocorticoids or microchimeric cells of the maternal immune system. Subsequently, the risk for mental disorders and diseases can increase in the offspring (Figure 3). As most of the studies are correlative, future research should aim to investigate causalities between maternal factors and children's health outcome. Clearly, adverse postnatal childhood experiences can further aggravate such cognitive and behavioral dysfunctions (173–178) and thus, should be considered in experimental designs and observational studies.
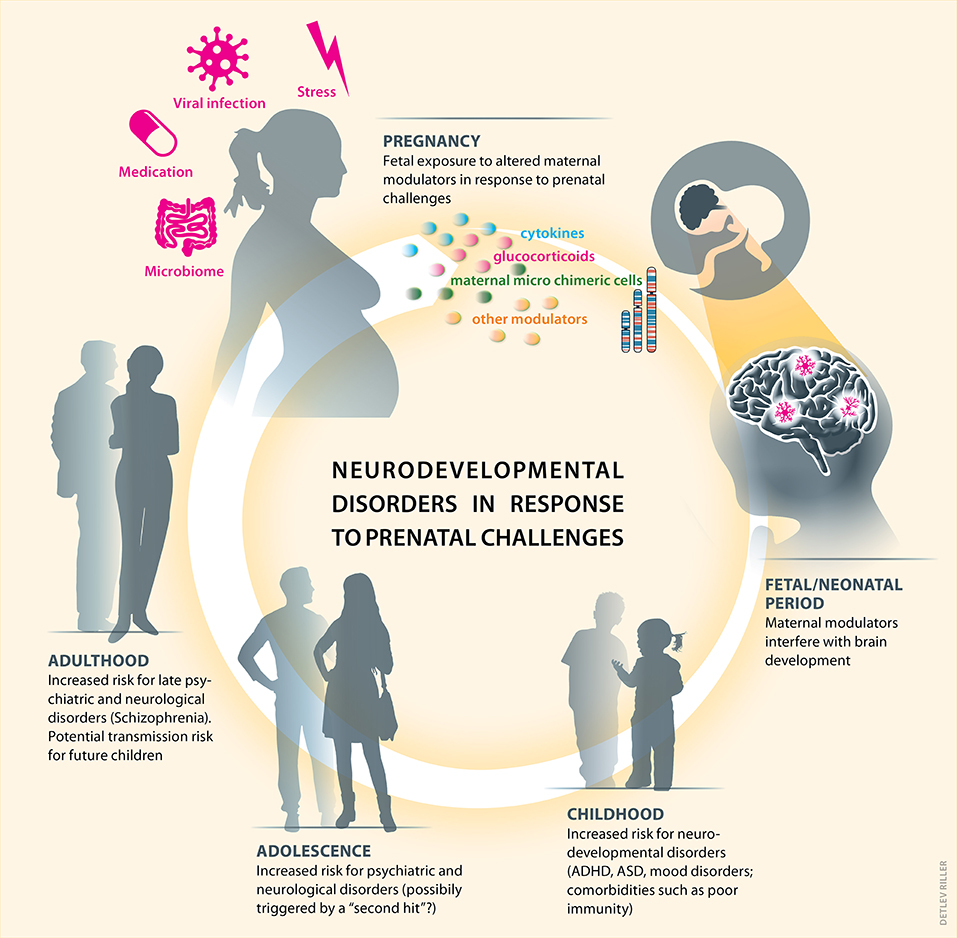
Figure 3. Prenatal challenges and related alterations of immune and endocrine markers can prime postnatal neurodevelopmental disorders. Maternal well-being and health can be challenged during pregnancy, e.g., by distress or infection. This subsequently leads to increased cytokines and glucocorticoids levels and potentially to altered frequencies or phenotypes of maternal microchimeric cells in the offspring. Upon entering the fetal brain, such vertically transferred maternal modulators can significantly interfere with physiologically occurring brain development. A combination of genetic susceptibility and disturbed brain development can subsequently increase the risk for neurodevelopmental disorders in childhood. Subsequent postnatal environmental challenges —drug abuse, trauma, infection, others—may perpetuate such prenatally triggered risk for neurodevelopmental disorders, psychiatric and neurological diseases during adolescence and adulthood, which can also be passed on to the next generation.
Author Contributions
SS and PA developed the structure of the review article, SS provided the first draft, which was amended by CB on aspects including prenatal cytokines and epigenetic pathways, by IH-O by insights on brain development and by PA on issues related to maternal immune adaptation to pregnancy. All authors have been involved in the interpretation of published evidence, critically revised the manuscript and gave their final approval of the version to be published.
Conflict of Interest Statement
The authors declare that the research was conducted in the absence of any commercial or financial relationships that could be construed as a potential conflict of interest.
Acknowledgments
Writing of this review and reference to the authors' own work were made possible through funding by the Deutsche Forschungsgemeinschaft (KFO296, AR232/26-2 to PA and SFB 936 B5, SPP 1665 Ha4466/12-1 to IH-O) and ERC Consolidator Grant 681577 to IH-O and R01 MH-105538, 5UG3OD023349 and ERC Starting grant 639766 to CB.
References
1. Christian MA, Samms-Vaughan M, Lee M, Bressler J, Hessabi M, Grove ML, et al. Maternal exposures associated with autism spectrum disorder in jamaican children. J Autism Dev Disord. (2018) 48:2766–78. doi: 10.1007/s10803-018-3537-6
2. Dimitrova N, Turpin H, Borghini A, Harari MM, Urben S, Müller-Nix C, Perinatal stress moderates the link between early and later emotional skills in very preterm-born children: an 11-year-long longitudinal study. Early Hum Dev. (2018) 121:8–14. doi: 10.1016/j.earlhumdev.2018.04.015
3. Glynn LM, Howland MA, Sandman CA, Davis EP, Phelan M, Baram TZ, et al. Prenatal maternal mood patterns predict child temperament and adolescent mental health. J Affect Disord. (2018) 228:83–90. doi: 10.1016/j.jad.2017.11.065
4. Laplante DP, Hart KJ, O'Hara MW, Brunet A, and King S. Prenatal maternal stress is associated with toddler cognitive functioning: the Iowa flood study. Early Hum Dev. (2018) 116:84–92. doi: 10.1016/j.earlhumdev.2017.11.012
5. Nolvi S, Pesonen H, Bridgett DJ, Korja R, Kataja EL, Karlsson H, et al. Infant sex moderates the effects of maternal pre-and postnatal stress on executive functioning at 8 months of age. Infancy (2018) 23:194–210. doi: 10.1111/infa.12206
6. Vizzini L, Popovic M, Zugna D, Vitiello B, Trevisan M, Pizzi C, et al. Maternal anxiety, depression and sleep disorders before and during pregnancy, and preschool ADHD symptoms in the NINFEA birth cohort study. Epidemiol Psychiatric Sci. 2018:1–11. doi: 10.1017/S2045796018000185
7. Bale TL, Baram TZ, Brown AS, Goldstein JM, Insel TR, McCarthy MM, et al. Early life programming and neurodevelopmental disorders. Biol Psychiatry (2010) 68:314–9. doi: 10.1016/j.biopsych.2010.05.028
8. Fisher J, Cabral de Mello M, Patel V, Rahman A, Tran T, Holton S, et al. Prevalence and determinants of common perinatal mental disorders in women in low- and lower-middle-income countries: a systematic review. Bull World Health Organ. (2012) 90:139G−49G. doi: 10.2471/BLT.11.091850
9. Walder DJ, Laplante DP, Sousa-Pires A, Veru F, Brunet A, and King S. Prenatal maternal stress predicts autism traits in 6 year-old children: Project Ice Storm. Psychiatry Res. (2014) 219:353–60. doi: 10.1016/j.psychres.2014.04.034
10. Betts KS, Williams GM, Najman JM, Alati R. Maternal depressive, anxious, and stress symptoms during pregnancy predict internalizing problems in adolescence. Depress Anxiety (2014) 31:9–18. doi: 10.1002/da.22210
11. Betts KS, Williams GM, Najman JM, Alati R. The relationship between maternal depressive, anxious, and stress symptoms during pregnancy and adult offspring behavioral and emotional problems. Depress Anxiety (2015) 32:82–90. doi: 10.1002/da.22272
12. Kingsbury M, Weeks M, MacKinnon N, Evans J, Mahedy L, Dykxhoorn J, et al. Stressful life events during pregnancy and offspring depression: evidence from a prospective cohort study. J Am Acad Child Adolesc Psychiatry (2016) 55:709–716e2. doi: 10.1016/j.jaac.2016.05.014
13. Abel KM, Heuvelman HP, Jorgensen L, Magnusson C, Wicks S, Susser E, et al. Severe bereavement stress during the prenatal and childhood periods and risk of psychosis in later life: population based cohort study. BMJ (2014) 348:f7679. doi: 10.1136/bmj.f7679
14. O'Donnell KJ, Glover V, Holbrook JD, O'Connor TG. Maternal prenatal anxiety and child brain-derived neurotrophic factor (BDNF) genotype: effects on internalizing symptoms from 4 to 15 years of age. Dev Psychopathol. (2014). 26(4 Pt 2):1255–66. doi: 10.1017/S095457941400100X
15. O'Donnell KJ, Glover V, Barker ED, O'Connor TG. The persisting effect of maternal mood in pregnancy on childhood psychopathology. Dev Psychopathol. (2014) 26:393–403. doi: 10.1017/S0954579414000029
16. Capron LE, Glover V, Pearson RM, Evans J, O'Connor TG, Stein A, et al. Associations of maternal and paternal antenatal mood with offspring anxiety disorder at age 18 years. J Affect Disord. (2015) 187:20–6. doi: 10.1016/j.jad.2015.08.012
17. Winsper C, Wolke D, Lereya T. Prospective associations between prenatal adversities and borderline personality disorder at 11-12 years. Psychol Med. (2015) 45:1025–37. doi: 10.1017/S0033291714002128
18. Leis JA, Heron J, Stuart EA, and Mendelson T. Associations between maternal mental health and child emotional and behavioral problems: does prenatal mental health matter? J Abnormal Child Psychol. (2014). 42:161–71. doi: 10.1007/s10802-013-9766-4
19. Tearne JE, Allen KL, Herbison CE, Lawrence D, Whitehouse AJ, Sawyer MG, et al. The association between prenatal environment and children's mental health trajectories from 2 to 14 years. Eur Child Adolesc Psychiatry (2015) 24:1015–24. doi: 10.1007/s00787-014-0651-7
20. Zhu P, Hao JH, Tao RX, Huang K, Jiang XM, Zhu YD, et al. Sex-specific and time-dependent effects of prenatal stress on the early behavioral symptoms of ADHD: a longitudinal study in China. Eur Child Adolesc Psychiatry (2015) 24:1139–47. doi: 10.1007/s00787-015-0701-9
21. Isaksson J, Lindblad F, Valladares E, Hogberg U. High maternal cortisol levels during pregnancy are associated with more psychiatric symptoms in offspring at age of nine - A prospective study from Nicaragua. J Psychiatr Res. (2015) 71:97–102. doi: 10.1016/j.jpsychires.2015.09.016
22. Pickles A, Sharp H, Hellier J, and Hill J. Prenatal anxiety, maternal stroking in infancy, and symptoms of emotional and behavioral disorders at 3.5 years. Eur Child Adolesc Psychiatry (2017) 26:325–34. doi: 10.1007/s00787-016-0886-6
23. St-Hilaire A, Steiger H, Liu A, Laplante DP, Thaler L, Magill T, et al. A prospective study of effects of prenatal maternal stress on later eating-disorder manifestations in affected offspring: preliminary indications based on the Project Ice Storm cohort. Int J Eat Disord. (2015) 48:512–6. doi: 10.1002/eat.22391
24. Korhonen M, Luoma I, Salmelin R, Tamminen T. Maternal depressive symptoms: associations with adolescents' internalizing and externalizing problems and social competence. Nord J Psychiatry (2014) 68:323–32. doi: 10.3109/08039488.2013.838804
25. Plant DT, Pariante CM, Sharp D, Pawlby S. Maternal depression during pregnancy and offspring depression in adulthood: role of child maltreatment. Br J Psychiatry (2015) 207:213–20. doi: 10.1192/bjp.bp.114.156620
26. Fineberg AM, Ellman LM, Schaefer CA, Maxwell SD, Shen L, H Chaudhury N, et al. Fetal exposure to maternal stress and risk for schizophrenia spectrum disorders among offspring: differential influences of fetal sex. Psychiatry Res. (2016). 236:91–7. doi: 10.1016/j.psychres.2015.12.026
27. Rijlaarsdam J, van IMH, Verhulst FC, Jaddoe VW, Felix JF, Tiemeier H, et al. Prenatal stress exposure, oxytocin receptor gene (OXTR) methylation, and child autistic traits: the moderating role of OXTR rs53576 genotype. Autism Res. (2017) 10:430–8. doi: 10.1002/aur.1681
28. Su X, Xu B, Liang H, Olsen J, Yuan W, Cnattingius S, et al. Prenatal maternal bereavement and risk of eating disorders in infants and toddlers: a population-based cohort study. BMC Psychiatry (2015) 15:229. doi: 10.1186/s12888-015-0612-9
29. Brown AS, Begg MD, Gravenstein S, Schaefer CA, Wyatt RJ, Bresnahan M, et al. Serologic evidence of prenatal influenza in the etiology of schizophrenia. Arch Gen Psychiatry (2004) 61:774–80. doi: 10.1001/archpsyc.61.8.774
30. Brown AS, Vinogradov S, Kremen WS, Poole JH, Deicken RF, Penner JD, et al. Prenatal exposure to maternal infection and executive dysfunction in adult schizophrenia. Am J Psychiatry (2009) 166:683–90. doi: 10.1176/appi.ajp.2008.08010089
31. Ellman LM, Yolken RH, Buka SL, Torrey EF, Cannon TD. Cognitive functioning prior to the onset of psychosis: the role of fetal exposure to serologically determined influenza infection. Biol Psychiatry (2009) 65:1040–7. doi: 10.1016/j.biopsych.2008.12.015
32. Buka SL, Tsuang MT, Torrey EF, Klebanoff MA, Bernstein D, Yolken RH. Maternal infections and subsequent psychosis among offspring. Arch Gen Psychiatry (2001) 58:1032–7. doi: 10.1001/archpsyc.58.11.1032
33. Buka SL, Cannon TD, Torrey EF, Yolken RH, Collaborative Study Group on the Perinatal Origins of Severe Psychiatric D. Maternal exposure to herpes simplex virus and risk of psychosis among adult offspring. Biol Psychiatry (2008) 63:809–15. doi: 10.1016/j.biopsych.2007.09.022
34. Mortensen PB, Pedersen CB, Hougaard DM, Norgaard-Petersen B, Mors O, Borglum AD, et al. A Danish national birth cohort study of maternal HSV-2 antibodies as a risk factor for schizophrenia in their offspring. Schizophr Res. (2010) 122:257–63. doi: 10.1016/j.schres.2010.06.010
35. Zerbo O, Iosif AM, Walker C, Ozonoff S, Hansen RL, Hertz-Picciotto I. Is maternal influenza or fever during pregnancy associated with autism or developmental delays? Results from the CHARGE (CHildhood Autism Risks from Genetics and Environment) study. J Autism Dev Disord. (2013). 43:25–33. doi: 10.1007/s10803-012-1540-x
36. Sørensen HJ, Mortensen EL, Reinisch JM, Mednick SA. Association between prenatal exposure to bacterial infection and risk of schizophrenia. Schizophrenia Bull. (2008) 35:631–7. doi: 10.1093/schbul/sbn121
37. Brown AS, Sourander A, Hinkka-Yli-Salomaki S, McKeague IW, Sundvall J, Surcel HM. Elevated maternal C-reactive protein and autism in a national birth cohort. Mol Psychiatry (2014) 19:259–64. doi: 10.1038/mp.2012.197
38. Smith B, Kemp M, Ethelberg S, Schiellerup P, Bruun BG, Gerner-Smidt P, et al. Listeria monocytogenes: maternal-foetal infections in Denmark 1994-2005. Scand J Infect Dis. (2009). 41:21–5. doi: 10.1080/00365540802468094
39. Abrahao AL, Focaccia R, Gattaz WF. Childhood meningitis increases the risk for adult schizophrenia. World J Biol Psychiatry (2005). 6(Suppl 2):44–8. doi: 10.1080/15622970510030063
40. Brown AS, Schaefer CA, Quesenberry CP Jr, Liu L, Babulas VP, Susser ES. Maternal exposure to toxoplasmosis and risk of schizophrenia in adult offspring. Am J Psychiatry (2005) 162:767–73. doi: 10.1176/appi.ajp.162.4.767
41. Xiao J, Buka SL, Cannon TD, Suzuki Y, Viscidi RP, Torrey EF, et al. Serological pattern consistent with infection with type I Toxoplasma gondii in mothers and risk of psychosis among adult offspring. Microbes Infect. (2009) 11:1011–8. doi: 10.1016/j.micinf.2009.07.007
42. Atladottir HO, Thorsen P, Ostergaard L, Schendel DE, Lemcke S, Abdallah M, et al. Maternal infection requiring hospitalization during pregnancy and autism spectrum disorders. J Autism Dev Disord. (2010) 40:1423–30. doi: 10.1007/s10803-010-1006-y
43. Patel S, Masi A, Dale RC, Whitehouse AJO, Pokorski I, Alvares GA, et al. Social impairments in autism spectrum disorder are related to maternal immune history profile. Mol Psychiatry (2017). doi: 10.1038/mp.2017.201. [Epub ahead of print].
44. Nielsen PR, Laursen TM, Mortensen PB. Association between parental hospital-treated infection and the risk of schizophrenia in adolescence and early adulthood. Schizophrenia Bull. (2011) 39:230–7. doi: 10.1093/schbul/sbr149
45. Polanczyk G, de Lima MS, Horta BL, Biederman J, Rohde LA. The worldwide prevalence of ADHD: a systematic review and metaregression analysis. Am J Psychiatry (2007) 164:942–8. doi: 10.1176/ajp.2007.164.6.942
46. Rudolph MD, Graham AM, Feczko E, Miranda-Dominguez O, Rasmussen JM, Nardos R, et al. Maternal IL-6 during pregnancy can be estimated from newborn brain connectivity and predicts future working memory in offspring. Nat Neurosci. (2018) 21:765–72. doi: 10.1038/s41593-018-0128-y
47. Brown AS, Hooton J, Schaefer CA, Zhang H, Petkova E, Babulas V, et al. Elevated maternal interleukin-8 levels and risk of schizophrenia in adult offspring. Am J Psychiatry (2004) 161:889–95. doi: 10.1176/appi.ajp.161.5.889
48. Blomstrom A, Karlsson H, Gardner R, Jorgensen L, Magnusson C, Dalman C. Associations between maternal infection during pregnancy, childhood infections, and the risk of subsequent psychotic disorder–a Swedish cohort study of nearly 2 million individuals. Schizophr Bull. (2016) 42:125–33. doi: 10.1093/schbul/sbv112
49. Selten JP, Frissen A, Lensvelt-Mulders G, Morgan VA. Schizophrenia and 1957 pandemic of influenza: meta-analysis. Schizophr Bull. (2010) 36:219–28. doi: 10.1093/schbul/sbp147
50. Selten JP, Termorshuizen F. The serological evidence for maternal influenza as risk factor for psychosis in offspring is insufficient: critical review and meta-analysis. Schizophr Res. (2017) 183:2–9. doi: 10.1016/j.schres.2016.11.006
51. Andersen SL. Trajectories of brain development: point of vulnerability or window of opportunity? Neurosci Biobehav Rev. (2003) 27:3–18. doi: 10.1016/S0149-7634(03)00005-8
52. Knuesel I, Chicha L, Britschgi M, Schobel SA, Bodmer M, Hellings JA, et al. Maternal immune activation and abnormal brain development across CNS disorders. Nat Rev Neurol. (2014) 10:643–60. doi: 10.1038/nrneurol.2014.187
53. Molnár Z, Price DJ. Brain development. In: Baldock R, Bard J, Davidson DR, Morriss-Kay G, editors. Kaufman's Atlas of Mouse Development Supplement. Amsterdam: Academic Press (2015). p. 239–52.
54. Ginhoux F, Greter M, Leboeuf M, Nandi S, See P, Gokhan S, et al. Fate mapping analysis reveals that adult microglia derive from primitive macrophages. Science (2010) 330:841–5. doi: 10.1126/science.1194637
55. Taylor DC, Differential rates of cerebral maturation between sexes and between hemispheres. Evidence from epilepsy. Lancet (1969) 2:140–2. doi: 10.1016/S0140-6736(69)92445-3
56. Ajmone-Cat MA, Cacci E, Ragazzoni Y, Minghetti L, Biagioni S. Pro-gliogenic effect of IL-1alpha in the differentiation of embryonic neural precursor cells in vitro. J Neurochem. (2010) 113:1060–72. doi: 10.1111/j.1471-4159.2010.06670.x
57. Ling ZD, Potter ED, Lipton JW, Carvey PM. Differentiation of mesencephalic progenitor cells into dopaminergic neurons by cytokines. Exp Neurol. (1998) 149:411–23. doi: 10.1006/exnr.1998.6715
58. Monje ML, Toda H, Palmer TD. Inflammatory blockade restores adult hippocampal neurogenesis. Science (2003) 302:1760–5. doi: 10.1126/science.1088417
59. Koo JW, Duman RS. IL-1beta is an essential mediator of the antineurogenic and anhedonic effects of stress. Proc Natl Acad Sci USA. (2008) 105:751–6. doi: 10.1073/pnas.0708092105
60. Crampton SJ, Collins LM, Toulouse A, Nolan YM, O'Keeffe GW. Exposure of foetal neural progenitor cells to IL-1beta impairs their proliferation and alters their differentiation-a role for maternal inflammation? J Neurochem. (2012) 120:964–73. doi: 10.1111/j.1471-4159.2011.07634.x
61. Wang X, Fu S, Wang Y, Yu P, Hu J, Gu W, et al. Interleukin-1beta mediates proliferation and differentiation of multipotent neural precursor cells through the activation of SAPK/JNK pathway. Mol Cell Neurosci. (2007) 36:343–54. doi: 10.1016/j.mcn.2007.07.005
62. Chucair-Elliott AJ, Conrady C, Zheng M, Kroll CM, Lane TE, Carr DJ. Microglia-induced IL-6 protects against neuronal loss following HSV-1 infection of neural progenitor cells. Glia (2014) 62:1418–34. doi: 10.1002/glia.22689
63. Yang P, Wen H, Ou S, Cui J, Fan D. IL-6 promotes regeneration and functional recovery after cortical spinal tract injury by reactivating intrinsic growth program of neurons and enhancing synapse formation. Exp Neurol. (2012) 236:19–27. doi: 10.1016/j.expneurol.2012.03.019
64. Oh J, McCloskey MA, Blong CC, Bendickson L, Nilsen-Hamilton M, Sakaguchi DS. Astrocyte-derived interleukin-6 promotes specific neuronal differentiation of neural progenitor cells from adult hippocampus. J Neurosci Res. (2010) 88:2798–809. doi: 10.1002/jnr.22447
65. Iosif RE, Ekdahl CT, Ahlenius H, Pronk CJ, Bonde S, Kokaia Z, et al. Tumor necrosis factor receptor 1 is a negative regulator of progenitor proliferation in adult hippocampal neurogenesis. J Neurosci. (2006) 26:9703–12. doi: 10.1523/JNEUROSCI.2723-06.2006
66. Bruce AJ, Boling W, Kindy MS, Peschon J, Kraemer PJ, Carpenter MK, et al. Altered neuronal and microglial responses to excitotoxic and ischemic brain injury in mice lacking TNF receptors. Nat Med. (1996) 2:788–94. doi: 10.1038/nm0796-788
67. Sarder M, Abe K, Saito H, Nishiyama N. Comparative effect of IL-2 and IL-6 on morphology of cultured hippocampal neurons from fetal rat brain. Brain Res. (1996) 715:9–16. doi: 10.1016/0006-8993(95)01291-5
68. Beck RD Jr, Wasserfall C, Ha GK, Cushman JD, Huang Z, Atkinson MA, et al. Changes in hippocampal IL-15, related cytokines, and neurogenesis in IL-2 deficient mice. Brain Res. (2005) 1041:223–30. doi: 10.1016/j.brainres.2005.02.010
69. Butovsky O, Ziv Y, Schwartz A, Landa G, Talpalar AE, Pluchino S, et al. Microglia activated by IL-4 or IFN-gamma differentially induce neurogenesis and oligodendrogenesis from adult stem/progenitor cells. Mol Cell Neurosci. (2006) 31:149–60. doi: 10.1016/j.mcn.2005.10.006
70. Yang J, Jiang Z, Fitzgerald DC, Ma C, Yu S, Li H, et al. Adult neural stem cells expressing IL-10 confer potent immunomodulation and remyelination in experimental autoimmune encephalitis. J Clin Invest. (2009) 119:3678–91. doi: 10.1172/JCI37914
71. Molina-Holgado E, Vela JM, Arevalo-Martin A, Guaza C. LPS/IFN-gamma cytotoxicity in oligodendroglial cells: role of nitric oxide and protection by the anti-inflammatory cytokine IL-10. Eur J Neurosci. (2001) 13:493–502. doi: 10.1046/j.0953-816x.2000.01412.x
72. Guan Y, Jiang Z, Ciric B, Rostami AM, Zhang GX. Upregulation of chemokine receptor expression by IL-10/IL-4 in adult neural stem cells. Exp Mol Pathol. (2008) 85:232–6. doi: 10.1016/j.yexmp.2008.07.003
73. Buckwalter MS, Yamane M, Coleman BS, Ormerod BK, Chin JT, Palmer T, et al. Chronically increased transforming growth factor-beta1 strongly inhibits hippocampal neurogenesis in aged mice. Am J Pathol. (2006) 169:154–64. doi: 10.2353/ajpath.2006.051272
74. Ikeda K, Iwasaki Y, Shiojima T, Kinoshita M. Neuroprotective effect of various cytokines on developing spinal motoneurons following axotomy. J Neurol Sci. (1996) 135:109–13. doi: 10.1016/0022-510X(95)00263-2
75. Bauer S, Patterson PH. Leukemia inhibitory factor promotes neural stem cell self-renewal in the adult brain. J Neurosci. (2006) 26:12089–99. doi: 10.1523/JNEUROSCI.3047-06.2006
76. Deverman BE, Patterson PH. Cytokines and CNS development. Neuron (2009) 64:61–78. doi: 10.1016/j.neuron.2009.09.002
77. Li Z, Li K, Zhu L, Kan Q, Yan Y, Kumar P, et al. Inhibitory effect of IL-17 on neural stem cell proliferation and neural cell differentiation. BMC Immunol. (2013) 14:20. doi: 10.1186/1471-2172-14-20
78. Liu Q, Xin W, He P, Turner D, Yin J, Gan Y, et al. Interleukin-17 inhibits adult hippocampal neurogenesis. Sci Rep. (2014) 4:7554. doi: 10.1038/srep07554
79. Wong H, Hoeffer C. Maternal IL-17A in autism. Exp Neurol. (2018) 299(Pt A):228–40. doi: 10.1016/j.expneurol.2017.04.010
80. Guyon A. CXCL12 chemokine and its receptors as major players in the interactions between immune and nervous systems. Front Cell Neurosci. (2014) 8:65. doi: 10.3389/fncel.2014.00065
81. Stuart MJ, Singhal G, Baune BT. Systematic review of the neurobiological relevance of chemokines to psychiatric disorders. Front Cell Neurosci. (2015) 9:357. doi: 10.3389/fncel.2015.00357
82. Tran PB, Miller RJ. Chemokine receptors: signposts to brain development and disease. Nat Rev Neurosci. (2003) 4:444–55. doi: 10.1038/nrn1116
83. Solano ME, Holmes MC, Mittelstadt PR, Chapman KE, Tolosa E. Antenatal endogenous and exogenous glucocorticoids and their impact on immune ontogeny and long-term immunity. Semin Immunopathol. (2016) 38:739–63. doi: 10.1007/s00281-016-0575-z
84. Sandman CA, Davis EP, Buss C, Glynn LM. Prenatal programming of human neurological function. Int J Pept. (2011) 2011:837596. doi: 10.1155/2011/837596
85. Arck PC, Rose M, Hertwig K, Hagen E, Hildebrandt M, Klapp BF. Stress and immune mediators in miscarriage. Hum Reprod. (2001) 16:1505–11. doi: 10.1093/humrep/16.7.1505
86. Christian LM, Glaser R, Porter K, Iams JD. Stress-induced inflammatory responses in women: effects of race and pregnancy. Psychosom Med. (2013) 75:658–69. doi: 10.1097/PSY.0b013e31829bbc89
87. Solano ME, Kowal MK, O'Rourke GE, Horst AK, Modest K, Plosch T, et al. Progesterone and HMOX-1 promote fetal growth by CD8+ T cell modulation. J Clin Invest. (2015) 125:1726–38. doi: 10.1172/JCI68140
88. Blois SM, Ilarregui JM, Tometten M, Garcia M, Orsal AS, Cordo-Russo R, et al. A pivotal role for galectin-1 in fetomaternal tolerance. Nat Med. (2007) 13:1450–7. doi: 10.1038/nm1680
89. Engels G, Hierweger AM, Hoffmann J, Thieme R, Thiele S, Bertram S, et al. Pregnancy-related immune adaptation promotes the emergence of highly virulent H1N1 influenza virus strains in allogenically pregnant mice. Cell Host Microbe (2017) 21:321–33. doi: 10.1016/j.chom.2017.02.020
90. Friebe A, Douglas AJ, Solano E, Blois SM, Hagen E, Klapp BF, et al. Neutralization of LPS or blockage of TLR4 signaling prevents stress-triggered fetal loss in murine pregnancy. J Mol Med. (2011) 89:689–99. doi: 10.1007/s00109-011-0743-5
91. Kinder JM, Stelzer IA, Arck PC, Way SS. Immunological implications of pregnancy-induced microchimerism. Nat Rev Immunol. (2017) 17:483–94. doi: 10.1038/nri.2017.38
92. Bauer S, Kerr BJ, Patterson PH. The neuropoietic cytokine family in development, plasticity, disease and injury. Nat Rev Neurosci. (2007) 8:221–32. doi: 10.1038/nrn2054
93. Fatemi SH, Earle J, Kanodia R, Kist D, Emamian ES, Patterson PH, et al. Prenatal viral infection leads to pyramidal cell atrophy and macrocephaly in adulthood: implications for genesis of autism and schizophrenia. Cell Mol Neurobiol. (2002) 22:25–33. doi: 10.1023/A:1015337611258
94. Baharnoori M, Brake WG, Srivastava LK. Prenatal immune challenge induces developmental changes in the morphology of pyramidal neurons of the prefrontal cortex and hippocampus in rats. Schizophr Res. (2009) 107:99–109. doi: 10.1016/j.schres.2008.10.003
95. Cui K, Ashdown H, Luheshi GN, Boksa P. Effects of prenatal immune activation on hippocampal neurogenesis in the rat. Schizophr Res. (2009) 113:288–97. doi: 10.1016/j.schres.2009.05.003
96. Meyer U, Nyffeler M, Engler A, Urwyler A, Schedlowski M, Knuesel I, et al. The time of prenatal immune challenge determines the specificity of inflammation-mediated brain and behavioral pathology. J Neurosci. (2006) 26:4752–62. doi: 10.1523/JNEUROSCI.0099-06.2006
97. Makinodan M, Tatsumi K, Manabe T, Yamauchi T, Makinodan E, Matsuyoshi H, et al. Maternal immune activation in mice delays myelination and axonal development in the hippocampus of the offspring. J Neurosci Res. (2008) 86:2190–200. doi: 10.1002/jnr.21673
98. Fernandez de Cossio L, Guzman A, van der Veldt S, Luheshi GN. Prenatal infection leads to ASD-like behavior and altered synaptic pruning in the mouse offspring. Brain Behav Immun. (2017) 63:88–98. doi: 10.1016/j.bbi.2016.09.028
99. Giovanoli S, Weber-Stadlbauer U, Schedlowski M, Meyer U, Engler H. Prenatal immune activation causes hippocampal synaptic deficits in the absence of overt microglia anomalies. Brain Behav Immun. (2016) 55:25–38. doi: 10.1016/j.bbi.2015.09.015
100. Schaafsma W, Basterra LB, Jacobs S, Brouwer N, Meerlo P, Schaafsma A, et al. Maternal inflammation induces immune activation of fetal microglia and leads to disrupted microglia immune responses, behavior, and learning performance in adulthood. Neurobiol Dis. (2017) 106:291–300. doi: 10.1016/j.nbd.2017.07.017
101. Hui CW, St-Pierre A, El Hajj H, Remy Y, Hebert SS, Luheshi GN, et al. Prenatal immune challenge in mice leads to partly sex-dependent behavioral, microglial, and molecular abnormalities associated with schizophrenia. Front Mol Neurosci. (2018) 11:13. doi: 10.3389/fnmol.2018.00013
102. Bilbo SD, Schwarz JM. Early-life programming of later-life brain and behavior: a critical role for the immune system. Front Behav Neurosci. (2009) 3:14. doi: 10.3389/neuro.08.014.2009
103. Graham AM, Rasmussen JM, Rudolph MD, Heim CM, Gilmore JH, Styner M, et al. Maternal systemic interleukin-6 during pregnancy is associated with newborn amygdala phenotypes and subsequent behavior at 2 years of age. Biol Psychiatry (2018) 83:109–19. doi: 10.1016/j.biopsych.2017.05.027
104. Graham AM, Buss C, Rasmussen JM, Rudolph MD, Demeter DV, Gilmore JH, et al. Implications of newborn amygdala connectivity for fear and cognitive development at 6-months-of-age. Dev Cogn Neurosci. (2016) 18:12–25. doi: 10.1016/j.dcn.2015.09.006
105. Mychasiuk R, Gibb R, Kolb B. Prenatal stress alters dendritic morphology and synaptic connectivity in the prefrontal cortex and hippocampus of developing offspring. Synapse (2012) 66:308–14. doi: 10.1002/syn.21512
106. Bock J, Murmu MS, Biala Y, Weinstock M, Braun K. Prenatal stress and neonatal handling induce sex-specific changes in dendritic complexity and dendritic spine density in hippocampal subregions of prepubertal rats. Neuroscience (2011) 193:34–43. doi: 10.1016/j.neuroscience.2011.07.048
107. Buss C, Davis EP, Shahbaba B, Pruessner JC, Head K, Sandman CA. Maternal cortisol over the course of pregnancy and subsequent child amygdala and hippocampus volumes and affective problems. Proc Natl Acad Sci USA. (2012) 109:E1312-9. doi: 10.1073/pnas.1201295109
108. Davis EP, Head K, Buss C, Sandman CA. Prenatal maternal cortisol concentrations predict neurodevelopment in middle childhood. Psychoneuroendocrinology (2017) 75:56–63. doi: 10.1016/j.psyneuen.2016.10.005
109. Lemaire V, Koehl M, Le Moal M, Abrous DN. Prenatal stress produces learning deficits associated with an inhibition of neurogenesis in the hippocampus. Proc Natl Acad Sci USA. (2000) 97:11032–7. doi: 10.1073/pnas.97.20.11032
110. Jia N, Yang K, Sun Q, Cai Q, Li H, Cheng D, et al. Prenatal stress causes dendritic atrophy of pyramidal neurons in hippocampal CA3 region by glutamate in offspring rats. Dev Neurobiol. (2010) 70:114–25. doi: 10.1002/dneu.20766
111. Hayashi A, Nagaoka M, Yamada K, Ichitani Y, Miake Y, Okado N. Maternal stress induces synaptic loss and developmental disabilities of offspring. Int J Dev Neurosci. (1998) 16:209–16. doi: 10.1016/S0736-5748(98)00028-8
112. Schafer DP, Lehrman EK, Kautzman AG, Koyama R, Mardinly AR, Yamasaki R, et al. Microglia sculpt postnatal neural circuits in an activity and complement-dependent manner. Neuron (2012) 74:691–705. doi: 10.1016/j.neuron.2012.03.026
113. Bittle J, Stevens HE. The role of glucocorticoid, interleukin-1beta, and antioxidants in prenatal stress effects on embryonic microglia. J Neuroinflammation (2018) 15:44. doi: 10.1186/s12974-018-1079-7
114. Slusarczyk J, Trojan E, Glombik K, Budziszewska B, Kubera M, Lason W, et al. Prenatal stress is a vulnerability factor for altered morphology and biological activity of microglia cells. Front Cell Neurosci. (2015) 9:82. doi: 10.3389/fncel.2015.00082
115. Behan AT, van den Hove DL, Mueller L, Jetten MJ, Steinbusch HW, Cotter DR, et al. Evidence of female-specific glial deficits in the hippocampus in a mouse model of prenatal stress. Eur Neuropsychopharmacol. (2011) 21:71–9. doi: 10.1016/j.euroneuro.2010.07.004
116. Diz-Chaves Y, Astiz M, Bellini MJ, Garcia-Segura LM. Prenatal stress increases the expression of proinflammatory cytokines and exacerbates the inflammatory response to LPS in the hippocampal formation of adult male mice. Brain Behav Immun. (2013) 28:196–206. doi: 10.1016/j.bbi.2012.11.013
117. Diz-Chaves Y, Pernia O, Carrero P, Garcia-Segura LM. Prenatal stress causes alterations in the morphology of microglia and the inflammatory response of the hippocampus of adult female mice. J Neuroinflammation (2012) 9:71. doi: 10.1186/1742-2094-9-71
118. Stolp HB, Liddelow SA, Sá-Pereira I, Dziegielewska KM, Saunders NR. Immune responses at brain barriers and implications for brain development and neurological function in later life. Front Integr Neurosci. (2013) 7:61. doi: 10.3389/fnint.2013.00061
119. Murmu MS, Salomon S, Biala Y, Weinstock M, Braun K, Bock J. Changes of spine density and dendritic complexity in the prefrontal cortex in offspring of mothers exposed to stress during pregnancy. Eur J Neurosci. (2006) 24:1477–87. doi: 10.1111/j.1460-9568.2006.05024.x
120. Salm AK, Pavelko M, Krouse EM, Webster W, Kraszpulski M, Birkle DL. Lateral amygdaloid nucleus expansion in adult rats is associated with exposure to prenatal stress. Brain Res Dev Brain Res. (2004) 148:159–67. doi: 10.1016/j.devbrainres.2003.11.005
121. Ancatén González C, Gutiérrez-Rojas C, Bustamante Valdés C. Maternal exercise reverses morphologic changes in amygdala neurons produced by prenatal stress. Neurology Psychiatry Brain Res. (2017) 24:36–42. doi: 10.1016/j.npbr.2017.04.004
122. McEwen BS, Nasca C, Gray JD. Stress effects on neuronal structure: hippocampus, amygdala, and prefrontal cortex. Neuropsychopharmacology (2016) 41:3–23. doi: 10.1038/npp.2015.171
123. Shigemoto R, Kinoshita A, Wada E, Nomura S, Ohishi H, Takada M, et al. Differential presynaptic localization of metabotropic glutamate receptor subtypes in the rat hippocampus. J Neurosci. (1997) 17:7503–22. doi: 10.1523/JNEUROSCI.17-19-07503.1997
124. Skeberdis VA, Lan J, Opitz T, Zheng X, Bennett MV, Zukin RS. mGluR1-mediated potentiation of NMDA receptors involves a rise in intracellular calcium and activation of protein kinaseC. Neuropharmacology C (2001) 40:856–65. doi: 10.1016/S0028-3908(01)00005-3
125. Ambrosini A, Bresciani L, Fracchia S, Brunello N, Racagni G. Metabotropic glutamate receptors negatively coupled to adenylate cyclase inhibit N-methyl-D-aspartate receptor activity and prevent neurotoxicity in mesencephalic neurons in vitro. Mol Pharmacol. (1995) 47:1057–64.
126. Kandaswamy R, McQuillin A, Sharp SI, Fiorentino A, Anjorin A, Blizard RA, et al. Genetic association, mutation screening, and functional analysis of a Kozak sequence variant in the metabotropic glutamate receptor 3 gene in bipolar disorder. JAMA Psychiatry (2013) 70:591–8. doi: 10.1001/jamapsychiatry.2013.38
127. Laloux C, Mairesse J, Van Camp G, Giovine A, Branchi I, Bouret S, et al. Anxiety-like behaviour and associated neurochemical and endocrinological alterations in male pups exposed to prenatal stress. Psychoneuroendocrinology (2012) 37:1646–58. doi: 10.1016/j.psyneuen.2012.02.010
128. Zuena AR, Mairesse J, Casolini P, Cinque C, Alema GS, Morley-Fletcher S, et al. Prenatal restraint stress generates two distinct behavioral and neurochemical profiles in male and female rats. PLoS ONE (2008) 3:e2170. doi: 10.1371/journal.pone.0002170
129. Yaka R, Salomon S, Matzner H, Weinstock M. Effect of varied gestational stress on acquisition of spatial memory, hippocampal LTP and synaptic proteins in juvenile male rats. Behav Brain Res. (2007) 179:126–32. doi: 10.1016/j.bbr.2007.01.018
130. Muneoka K, Mikuni M, Ogawa T, Kitera K, Kamei K, Takigawa M, et al. Prenatal dexamethasone exposure alters brain monoamine metabolism and adrenocortical response in rat offspring. Am J Physiol. (1997) 273(5 Pt 2):R1669–75. doi: 10.1152/ajpregu.1997.273.5.R1669
131. Van den Hove DL, Leibold NK, Strackx E, Martinez-Claros M, Lesch KP, Steinbusch HW, et al. Prenatal stress and subsequent exposure to chronic mild stress in rats; interdependent effects on emotional behavior and the serotonergic system. Eur Neuropsychopharmacol. (2014) 24:595–607. doi: 10.1016/j.euroneuro.2013.09.006
132. Ramboz S, Oosting R, Amara DA, Kung HF, Blier P, Mendelsohn M, et al. Serotonin receptor 1A knockout: an animal model of anxiety-related disorder. Proc Natl Acad Sci USA. (1998) 95:14476–81. doi: 10.1073/pnas.95.24.14476
133. Giocomo LM, Hasselmo ME. Nicotinic modulation of glutamatergic synaptic transmission in region CA3 of the hippocampus. Eur J Neurosci. (2005) 22:1349–56. doi: 10.1111/j.1460-9568.2005.04316.x
134. Day JC, Koehl M, Deroche V, Le Moal M, Maccari S. Prenatal stress enhances stress- and corticotropin-releasing factor-induced stimulation of hippocampal acetylcholine release in adult rats. J Neurosci. (1998) 18:1886–92. doi: 10.1523/JNEUROSCI.18-05-01886.1998
135. Nelson JL. The otherness of self: microchimerism in health and disease. Trends Immunol. (2012) 33:421–7. doi: 10.1016/j.it.2012.03.002
136. Vernochet C, Caucheteux SM, Kanellopoulos-Langevin C. Bi-directional cell trafficking between mother and fetus in mouse placenta. Placenta (2007) 28:639–49. doi: 10.1016/j.placenta.2006.10.006
137. Stelzer IA, Thiele K, Solano ME. Maternal microchimerism: lessons learned from murine models. J Reprod Immunol. (2015) 108:12–25. doi: 10.1016/j.jri.2014.12.007
138. Buss C, Entringer S, Wadhwa PD. Fetal programming of brain development: intrauterine stress and susceptibility to psychopathology. Sci Signal. (2012) 5(245):pt7. doi: 10.1126/scisignal.2003406
139. Palma-Gudiel H, Córdova-Palomera A, Leza JC, Fananás L. Glucocorticoid receptor gene (NR3C1) methylation processes as mediators of early adversity in stress-related disorders causality: a critical review. Neurosci Biobehav Rev. (2015) 55:520–35. doi: 10.1016/j.neubiorev.2015.05.016
140. Bale TL. Epigenetic and transgenerational reprogramming of brain development. Nat Rev Neurosci. (2015) 16:332. doi: 10.1038/nrn3818
142. Teh AL, Pan H, Chen L, Ong ML, Dogra S, Wong J, et al. The effect of genotype and in utero environment on inter-individual variation in neonate DNA methylomes. Genome Res. (2014) 24:1064–74. doi: 10.1101/gr.171439.113
143. Heijmans BT, Tobi EW, Stein AD, Putter H, Blauw GJ, Susser ES, et al. Persistent epigenetic differences associated with prenatal exposure to famine in humans. Proc Natl Acad Sci USA. (2008) 105:17046–9. doi: 10.1073/pnas.0806560105
144. Oberlander TF, Weinberg J, Papsdorf M, Grunau R, Misri S, Devlin AM. Prenatal exposure to maternal depression, neonatal methylation of human glucocorticoid receptor gene (NR3C1) and infant cortisol stress responses. Epigenetics (2008) 3:97–106. doi: 10.4161/epi.3.2.6034
145. Braithwaite EC, Kundakovic M, Ramchandani PG, Murphy SE, Champagne FA. Maternal prenatal depressive symptoms predict infant NR3C1 1F and BDNF IV DNA methylation. Epigenetics (2015) 10:408–17. doi: 10.1080/15592294.2015.1039221
146. Radtke KM, Ruf M, Gunter HM, Dohrmann K, Schauer M, Meyer A, et al. Transgenerational impact of intimate partner violence on methylation in the promoter of the glucocorticoid receptor. Transl Psychiatry (2011) 1:e21. doi: 10.1038/tp.2011.21
147. Tang B, Jia H, Kast RJ, Thomas EA. Epigenetic changes at gene promoters in response to immune activation in utero. Brain Behav Immun. (2013) 30:168–75. doi: 10.1016/j.bbi.2013.01.086
148. Basil P, Li Q, Dempster EL, Mill J, Sham PC, Wong CC, et al. Prenatal maternal immune activation causes epigenetic differences in adolescent mouse brain. Transl Psychiatry (2014) 4:e434. doi: 10.1038/tp.2014.80
149. Chahrour M, Jung SY, Shaw C, Zhou X, Wong ST, Qin J, et al. MeCP2, a key contributor to neurological disease, activates and represses transcription. Science (2008) 320:1224–9. doi: 10.1126/science.1153252
150. Labouesse MA, Dong E, Grayson DR, Guidotti A, Meyer U. Maternal immune activation induces GAD1 and GAD2 promoter remodeling in the offspring prefrontal cortex. Epigenetics (2015) 10:1143–55. doi: 10.1080/15592294.2015.1114202
151. Bohacek J, Gapp K, Saab BJ, Mansuy IM. Transgenerational epigenetic effects on brain functions. Biol Psychiatry (2013) 73:313–20. doi: 10.1016/j.biopsych.2012.08.019
152. Buss C, Entringer S, Moog NK, Toepfer P, Fair DA, Simhan HN, et al. Intergenerational transmission of maternal childhood maltreatment exposure: implications for fetal brain development. J Am Acad Child Adolesc Psychiatry (2017) 56:373–82. doi: 10.1016/j.jaac.2017.03.001
153. Skinner MK. Role of epigenetics in developmental biology and transgenerational inheritance. Birth Defects Res C Embryo Today (2011) 93:51–5. doi: 10.1002/bdrc.20199
154. Cowley M, Oakey RJ. Resetting for the next generation. Mol Cell. (2012) 48:819–21. doi: 10.1016/j.molcel.2012.12.007
155. Hackett JA, Zylicz JJ, Surani MA. Parallel mechanisms of epigenetic reprogramming in the germline. Trends Genet. (2012) 28:164–74. doi: 10.1016/j.tig.2012.01.005
156. Seisenberger S, Andrews S, Krueger F, Arand J, Walter J, Santos F, et al. The dynamics of genome-wide DNA methylation reprogramming in mouse primordial germ cells. Mol Cell. (2012) 48:849–62. doi: 10.1016/j.molcel.2012.11.001
157. Smallwood SA, Tomizawa S, Krueger F, Ruf N, Carli N, Segonds-Pichon A, et al. Dynamic CpG island methylation landscape in oocytes and preimplantation embryos. Nat Genet. (2011) 43:811–4. doi: 10.1038/ng.864
158. Smith ZD, Chan MM, Mikkelsen TS, Gu H, Gnirke A, Regev A, et al. A unique regulatory phase of DNA methylation in the early mammalian embryo. Nature (2012) 84:339–44. doi: 10.1038/nature10960
159. Gapp K, Jawaid A, Sarkies P, Bohacek J, Pelczar P, Prados J, et al. Implication of sperm RNAs in transgenerational inheritance of the effects of early trauma in mice. Nat Neurosci. (2014) 17:667–9. doi: 10.1038/nn.3695
160. Franklin TB, Russig H, Weiss IC, Graff J, Linder N, Michalon A, et al. Epigenetic transmission of the impact of early stress across generations. Biol Psychiatry (2010) 68:408–15. doi: 10.1016/j.biopsych.2010.05.036
161. Pang S, Curran SP. Longevity and the long arm of epigenetics: acquired parental marks influence lifespan across several generations. Bioessays (2012) 34:652–4. doi: 10.1002/bies.201200046
162. Rodgers AB, Morgan CP, Leu NA, Bale TL. Transgenerational epigenetic programming via sperm microRNA recapitulates effects of paternal stress. Proc Natl Acad Sci USA. (2015) 112:13699–704. doi: 10.1073/pnas.1508347112
163. Heard E, Martienssen RA. Transgenerational epigenetic inheritance: myths and mechanisms. Cell (2014) 157:95–109. doi: 10.1016/j.cell.2014.02.045
164. Cenquizca LA, Swanson LW. Spatial organization of direct hippocampal field CA1 axonal projections to the rest of the cerebral cortex. Brain Res Rev. (2007) 56:1–26. doi: 10.1016/j.brainresrev.2007.05.002
165. Jones MW, Wilson MA. Theta rhythms coordinate hippocampal-prefrontal interactions in a spatial memory task. PLoS Biol. (2005) 3:e402. doi: 10.1371/journal.pbio.0030402
166. Dickerson DD, Wolff AR, Bilkey DK. Abnormal long-range neural synchrony in a maternal immune activation animal model of schizophrenia. J Neurosci. (2010) 30:12424–31. doi: 10.1523/JNEUROSCI.3046-10.2010
167. Bitzenhofer SH, Sieben K, Siebert KD, Spehr M, Hanganu-Opatz IL. Oscillatory activity in developing prefrontal networks results from theta-gamma-modulated synaptic inputs. Cell Rep. (2015) 11:486–97. doi: 10.1016/j.celrep.2015.03.031
168. Zheng C, Quan M, Zhang T. Decreased thalamo-cortical connectivity by alteration of neural information flow in theta oscillation in depression-model rats. J Comput Neurosci. (2012) 33:547–58. doi: 10.1007/s10827-012-0400-1
169. Quan M, Zheng C, Zhang N, Han D, Tian Y, Zhang T, et al. Impairments of behavior, information flow between thalamus and cortex, and prefrontal cortical synaptic plasticity in an animal model of depression. Brain Res Bull. (2011) 85:109–16. doi: 10.1016/j.brainresbull.2011.03.002
170. Oliveira JF, Dias NS, Correia M, Gama-Pereira F, Sardinha VM, Lima A, et al. Chronic stress disrupts neural coherence between cortico-limbic structures. Front Neural Circuits (2013) 7:10. doi: 10.3389/fncir.2013.00010
171. Jacinto LR, Reis JS, Dias NS, Cerqueira JJ, Correia JH, Sousa N. Stress affects theta activity in limbic networks and impairs novelty-induced exploration and familiarization. Front Behav Neurosci. (2013) 7:127. doi: 10.3389/fnbeh.2013.00127
172. Kirischuk S, Sinning A, Blanquie O, Yang JW, Luhmann HJ, Kilb W. Modulation of neocortical development by early neuronal activity: physiology and pathophysiology. Front Cell Neurosci. (2017) 11:379. doi: 10.3389/fncel.2017.00379
173. Biedermann SV, Meliss S, Simmons C, Nothling J, Suliman S, Seedat S. Sexual abuse but not posttraumatic stress disorder is associated with neurocognitive deficits in South African traumatized adolescents. Child Abuse Negl. (2018) 80:257–67. doi: 10.1016/j.chiabu.2018.04.003
174. Teicher MH, Anderson CM, Ohashi K, Khan A, McGreenery CE, Bolger EA, et al. Differential effects of childhood neglect and abuse during sensitive exposure periods on male and female hippocampus. Neuroimage (2018) 169:443–52. doi: 10.1016/j.neuroimage.2017.12.055
175. Anda RF, Felitti VJ, Bremner JD, Walker JD, Whitfield C, Perry BD, et al. The enduring effects of abuse and related adverse experiences in childhood. A convergence of evidence from neurobiology and epidemiology. Eur Arch Psychiatry Clin Neurosci. (2006) 256:174–86. doi: 10.1007/s00406-005-0624-4
176. Danese A, Moffitt TE, Harrington H, Milne BJ, Polanczyk G, Pariante CM, et al. Adverse childhood experiences and adult risk factors for age-related disease: depression, inflammation, and clustering of metabolic risk markers. Arch Pediatr Adolesc Med. (2009) 163:1135–43. doi: 10.1001/archpediatrics.2009.214
177. Dube SR, Felitti VJ, Dong M, Giles WH, Anda RF. The impact of adverse childhood experiences on health problems: evidence from four birth cohorts dating back to 1900. Prev Med. (2003) 37:268–77. doi: 10.1016/S0091-7435(03)00123-3
Keywords: pregnancy, fetal brain development, prenatal infection, maternal distress, maternal microchimeric cells, cytokines, glucocorticoids (GC), epigenetic aberrations
Citation: Schepanski S, Buss C, Hanganu-Opatz IL and Arck PC (2018) Prenatal Immune and Endocrine Modulators of Offspring's Brain Development and Cognitive Functions Later in Life. Front. Immunol. 9:2186. doi: 10.3389/fimmu.2018.02186
Received: 23 July 2018; Accepted: 04 September 2018;
Published: 26 September 2018.
Edited by:
Julia Szekeres-Bartho, University of Pécs, HungaryReviewed by:
Gerard Chaouat, INSERM U976 Immunologie, Dermatologie, Oncologie, FranceRaj Raghupathy, Kuwait University, Kuwait
Copyright © 2018 Schepanski, Buss, Hanganu-Opatz and Arck. This is an open-access article distributed under the terms of the Creative Commons Attribution License (CC BY). The use, distribution or reproduction in other forums is permitted, provided the original author(s) and the copyright owner(s) are credited and that the original publication in this journal is cited, in accordance with accepted academic practice. No use, distribution or reproduction is permitted which does not comply with these terms.
*Correspondence: Petra C. Arck, cC5hcmNrQHVrZS5kZQ==
†These authors share senior authorship