- 1Department of Equine Sciences, Faculty of Veterinary Medicine, Utrecht University, Utrecht, Netherlands
- 2Department of Biochemistry & Cell Biology, Faculty of Veterinary Medicine, Utrecht University, Utrecht, Netherlands
- 3Department of Orthopaedics, University Medical Center Utrecht, Utrecht, Netherlands
The use of extracellular vesicles (EVs) as a potential therapy is currently explored for different disease areas. When it comes to the treatment of joint diseases this approach is still in its infancy. As in joint diseases both inflammation and the associated articular tissue destruction are important factors, both the immune-suppressive and the regenerative properties of EVs are potentially advantageous characteristics for future therapy. There is, however, only limited knowledge on the basic features, such as numerical profile and function, of EVs in joint articular tissues in general and their linking medium, the synovial fluid, in particular. Further insight is urgently needed in order to appreciate the full potential of EVs and to exploit these in EV-mediated therapies. Physiologic joint homeostasis is a prerequisite for proper functioning of joints and we postulate that EVs play a key role in the regulation of joint homeostasis and hence can have an important function in re-establishing disturbed joint homeostasis, and, in parallel, in the regeneration of articular tissues. In this mini-review EVs in the joint are explained from a historical perspective in both health and disease, including the potential niche for EVs in articular tissue regeneration. Furthermore, the translational potential of equine models for human joint biology is discussed. Finally, the use of MSC-derived EVs that is recently gaining ground is highlighted and recommendations are given for further EV research in this field.
Introduction
Joint diseases, with rheumatoid arthritis (RA) and osteoarthritis (OA) as most prevalent ones, represent a significant burden to human society, both in terms of loss of quality of life and as a significant part of total healthcare costs. Current demographic and societal developments—i.e., the rapid increase of life expectancy and the decreasing acceptance of disability—aggravate the problem quickly (1). In veterinary medicine, a similar situation exists, especially in horses, a species kept for its locomotor performance and in which joint disorders are, depending on equestrian discipline, invariably ranking first or second (after tendon injuries) as cause of lameness, and thus of disability to perform (2). Given the increasing burden joint diseases have on our society, new insights in joint biology and disease in both species can facilitate the development of novel therapies.
With respect to joint homeostasis, synovial joints can be envisaged as complex organs in which the articular tissues act as an entity: synovial membrane and cartilage stay in close contact via the synovial fluid (SF). Together, these tissues maintain the joint in a healthy steady state in physiologic conditions (3). As a consequence, imbalance in one of the tissues, due to trauma, infection or inflammation, will ultimately have impact on the entire joint (4, 5). Communication between tissues is of great importance to adequately stabilize these impaired conditions. Responses to undesirable situations are known to comprise production of catabolic cytokines, enzymes and inflammatory mediators (6), and it has been postulated that extracellular vesicles (EVs) can play a role as intercellular communication vehicle for these mediators and other biological signals in regulating immunologic processes to maintain joint homeostasis. In addition to a role during episodes of disease, EVs might also take part in the regulation of healthy joint homeostasis. There is thus an urgent need for comprehensive research of local and systemic EVs in healthy, as well as diseased joints before EV-based therapies, which could potentially assist in the resolution of inflammatory joint diseases and support repair of articular tissues, can be designed. We here outline the insights gained by recent research and the opportunities that lay ahead of us.
EVs: Complex and Multi-Facetted Particles
Extracellular vesicles are small, lipid-bilayer enclosed, cell-derived particles, specialized to facilitate cell-cell communication (7, 8). Their membrane contains proteins and lipids that mediate adherence to target cells, upon which active interaction takes place by several routes (Figure 1). In addition, soluble factors in the microenvironment of EVs can bind to their membrane and use EVs as shuttle vehicles for directed transport toward target cells (9, 10).
The major biogenesis routes of EVs follow generally one of two pathways (Figure 1): intraluminal vesicles (ILVs) can be produced within endosomes of the donor cells, resulting in multivesicular bodies (MVB). After subsequent fusion of MVBs with the plasma membrane, ILVs are released in the environment and are called “exosomes.” Alternatively, EVs originate directly from budding from the plasma membrane, referred to as “microvesicles” (7, 11). Often, the terms exosomes and microvesicles are used as equivalents for small and larger vesicles, respectively, but this classification is incorrect. Although exosomes are indeed generally smaller (as there is a limiting size for endosomal formation) and microvesicles are often detected as larger particles, both exosomes (~30–200 nm) and microvesicles (~50 nm−1 μm) can either be very small or relatively large (8). Vesicles shedding from apoptotic cells, including apoptotic bodies, are even more heterogeneous with diameters up to 5 μm.
The unique configuration of biologically active signaling molecules packaged into one small vesicle makes EVs highly efficient in bringing complex signals across (12, 13). Their lipid bilayer protects proteins and nucleic acids from the degradative extravesicular environment (i.e., the extracellular space), making stable transfer of proteins, rRNA, tRNA, miRNA, lncRNA, and (mitochondrial) DNA possible, over short and longer distances (14, 15). Also, specialized enzymes can be carried by EVs, which hence provide a tool to activate precursor molecules in the EV or in the recipient cell (13).
Extracellular vesicles typically have specific protein and lipid signatures of the cells of origin (16). In general, the EV membrane is composed of a bilayer of phospholipids, interspersed with glyco(sphingo)lipids, cholesterol, sphingomyelin, prostaglandins, integrins, tetraspanins, cell adhesion molecules and growth factor receptors (10, 15, 17). These molecules facilitate adhesion and/or fusion with recipient cells and may serve in ligand-receptor signaling. The EV membrane can also contain membrane transport proteins (e.g., sodium-dependent inorganic phosphate transporters) (18) and ion channels (e.g., annexins function as Ca2+ channels in matrix vesicles) (19). These characteristics enable EVs to act as sites of active processing of (signaling) molecules, in addition to being shuttling vehicles for passive transport of biologically active factors.
All cell types tested up to date can produce EVs. Production and release are tightly regulated processes which may vary between physiological and pathologic conditions (10, 20). In addition, the stimulation of cells by external stimuli can drastically change the EV-production rate and EV content or composition (21, 22). The pool of EVs found in biological fluids represents vesicles from the various cell types which are in direct contact with the fluid, or from infiltrating pathogens that also shed EVs (23, 24). In some cases, EVs can even cross epithelial and endothelial barriers, such as the blood brain barrier (25). Thus, the EV pool in body fluids reflects the systemic activity of the body as a whole or of specific organs and can be used as a monitoring tool (“liquid biopsy”) for active disease processes (26–28).
Cartilage Matrix Vesicles: The Ancestors of EV Research
The discovery of matrix vesicles is historically important for the general recognition that cell-derived vesicles may be functional, instead of only representing cell debris. Matrix vesicles, for the first time described in independent parallel research by Bonucci and Anderson in 1967 (29–31) are a specialized type of EVs with diameters of 30–500 nm, known for their function in endochondral ossification, the process during which fetal growth cartilage is converted into bone. This process takes place in the hypertrophic zone of the epiphyseal growth plates and in the ossification front under the articular surface. Here, matrix vesicles are formed by budding from the plasma membrane of maturing chondrocytes and osteoblasts. These vesicles subsequently collect calcium and phosphorus in their lumina and increase the concentration of phosphate through the action of alkaline phosphatase, which facilitates mineralization of the tissue (32, 33) (Figure 2). Very uniquely, this process takes place in a polarized fashion: vesicles pinch off from the lateral side of growth plate chondrocytes and from the osteoid-facing surface of osteoblasts, in the longitudinal direction of the bone (30, 36).
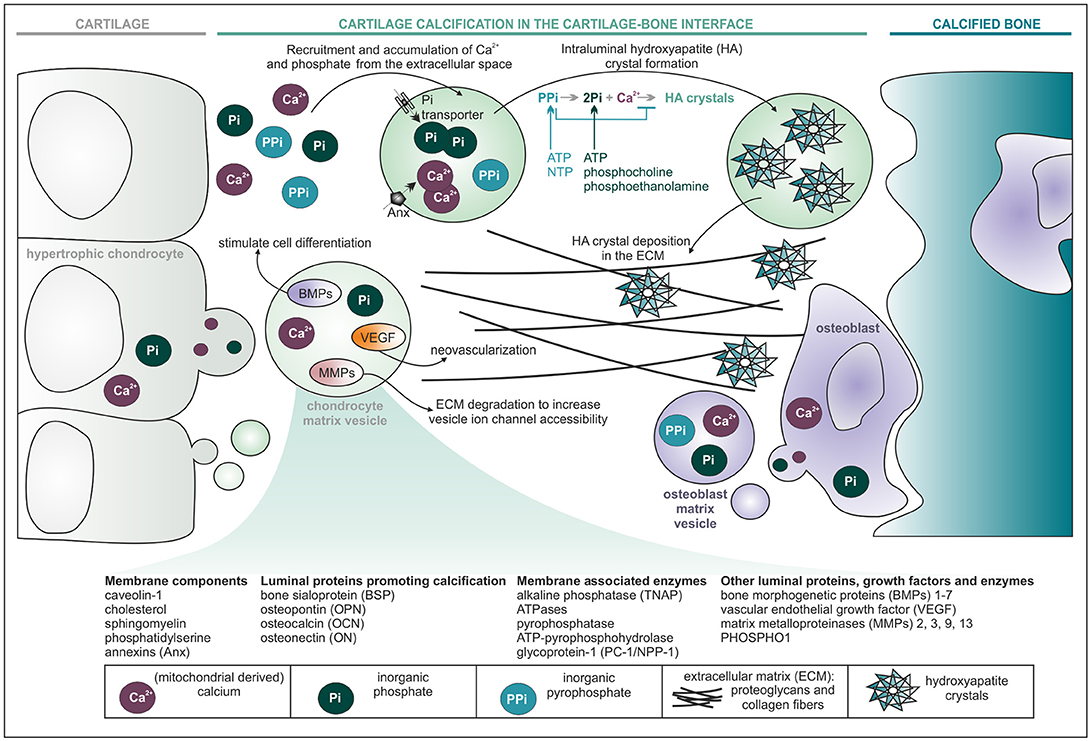
Figure 2. Matrix vesicles start cartilage calcification during endochondral ossification. Vesicles originating from maturing (hypertrophic) chondrocytes and osteoblasts accumulate calcium and phosphate ions in their lumen for the formation of hydroxyapatite (HA) crystals. Deposition of HA crystals in the extracellular matrix (ECM), together with calcification promoting proteins, leads to complete transformation of cartilage into bone. It is hypothesized that other factors found in matrix vesicles, such as BMPs, VEGF, and MMPs, could be involved in chondrocyte and osteoblast differentiation, neovascularisation and ECM degradation, respectively (34, 35). The electrophoretic profile of matrix vesicles is characterized by mineralisation promoting enzymes (TNAP, ATPases, etc.) that hydrolyze adenosine triphosphate (ATP) and nucleoside triphosphate (NTP) into inorganic pyrophosphate (PPi) and PPi into inorganic phosphate (Pi), thereby increasing concentrations of Pi and decreasing concentrations of PPi in the vesicle lumen and its surrounding matrix (36, 37). Keeping PPi concentrations low at sites of active mineralisation is critical, since PPi is the most important physiologic suppressor of hydroxyapatite crystal deposition (38). To further increase the pool of Pi in the vesicle lumen, the enzyme PHOSPHO1 is suggested to hydrolyse luminal phosphoethanolamine and phosphocholine (derived from membrane phospholipids) in order to produce Pi (37, 39, 40). Active phosphate transporters in the vesicle membrane facilitate further influx of Pi from the ECM (18).
The cues for the very precisely timed matrix vesicle biogenesis during endochondral ossification have not yet been completely elucidated, but an increase in intracellular Ca2+ concentration has been shown to induce matrix vesicle production in growth plate-derived chondrocytes in vitro (41). Furthermore, matrix vesicle production has been suggested to be the result of a specific form of programmed cell death by which hypertrophic chondrocytes are cleared from the growth plate and replaced by osteoblasts, leading to events of vesiculation (42).
Dysregulation of this matrix vesicle induced calcification of tissue is a feature of several joint diseases (43). In OA for example, prematurely differentiated chondrocytes are thought to release increased amounts of alkaline phosphatase and BMP-loaded matrix vesicles into the ECM, which may stimulate formation of osteosclerosis in the subchondral bone and osteophyte formation (33).
In addition to bone matrix vesicles, it is highly likely that other EV types play a role in the development of the musculoskeletal system, although direct evidence is lacking thus far. Skeletogenesis and synovial joint formation are highly orchestrated processes regulated by at least two important signaling pathways, Wnt and Hedgehog (44). These pathways steer chondrogenesis, osteoblast development and angiogenesis in concert with other regulatory factors that are expressed in the developing cartilage and perichondrium, such as BMPs, fibroblast growth factors (FGFs), TGFβ, and VEGF (45–47). These factors also have a role in homeostasis of the mature joint and all of them have been related to EVs, or found to be involved in (the regulation of) EV production and function (48, 49). It is, for example, known for Wnt signaling molecules that these are expressed on EVs derived from both Drosophila and human cells (50), indicating an evolutionary conserved process. The same holds true for Notch signaling. Notch modulates endochondral ossification (51), is required for articular cartilage and joint maintenance (52), and has been reported in multiple studies to be regulated in an EV-dependent manner (53, 54). The investigation of the role of these EVs during joint development is hence a new interesting avenue for joint biology research with potential benefits for regenerative medicine of the joint.
EVs: Regulators in Inflammatory Joint Disease?
So far, the knowledge about SF-derived EVs and their role in articular (patho)physiology is limited to a number of descriptive investigations that have revealed the presence of EVs in SF and a small number of elegant studies pointing out specific characteristics of EVs in human joint disease (55–72).
Joint diseases are in most cases associated with (chronic) inflammation (73). In addition to the high concentrations of cytokines, chemokines, catabolic enzymes and inflammatory mediators that can be measured in the SF (74), also EVs are present in substantial amounts in SF of patients with RA and OA (55, 56, 63, 75, 76). So far, in these samples EVs have been detected that originated from synovial fibroblasts (77), platelets (60), erythrocytes (55), neutrophils (64), monocytes and T-cells (63, 78). Apart from being produced by activated synoviocytes or by infiltrating immune cells, which are a hallmark of joint inflammation, EVs in SF can be derived from blood plasma of which SF is an ultra-filtrate. Finally, chondrocytes could also be a possible EV source, but chondrocyte-derived EVs have as far as we know not been detected in SF.
Although the exact mode of action of EVs in inflammatory joint diseases still has to be elucidated, several general mechanisms that are related to inflammation have been suggested (79, 80). These include the recognition of pathogen-derived EVs by immune cells, EV-mediated shuttling of inflammatory cytokines, lipid mediators, receptors and miRNA, and the ability of EVs to carry proteolytic enzymes that cause tissue destruction and further propagation of inflammation (8, 80–82). Also, a role is claimed for EVs in autoimmune diseases, such as RA (83, 84). Interestingly, whilst most studies so far have suggested a pro-inflammatory function for SF-derived EVs, a recent study has suggested that neutrophil-derived EVs from RA SF have a protective phenotype (64, 85). Probably the most interesting and urgent question at this moment is which role EVs take in different types of joint inflammation and at different timings during the disease process. Findings from these studies will not only bring opportunities for using EVs as potential biomarkers for early detection and categorization of joint inflammation, but also guide future development of (EV-mediated) therapeutics, targeting inflammation-inducing EV pathways or suppressing inflammation, locally in the joint or at a systemic level.
EVs for Articular Tissue Regeneration
Cartilage and bone destruction in joint disorders is essentially irreversible and usually worsens progressively during the course of the disease. The lack of repair capacity of mature articular cartilage is notorious and has been signaled as early as the mid-1700s by William Hunter in his famous publication on cartilage structure and cartilage diseases (86). This problem has not yet been solved and the quest for innovative strategies for cartilage repair is more intense than ever, driven by societal and demographic stressors. An important development in this quest is the introduction of scaffolds constructed from biomaterials that serve as artificial matrix for the repair of (osteo)chondral defects (87). Such scaffolds can be generated using 3-dimensional (3D)-bioprinting technology (88, 89) and they can be seeded with a combination of cells and growth factors of interest (90). A comparable approach is the use of fibrin matrices containing chondrons and mesenchymal stem cells (MSCs), which currently shows promising results in a clinical trial of patients with cartilage defects (91). It recently became clear that secreted EVs are the important driving force of the bioactive capacity of these treatments. Importantly, also the bioactivity of de-cellularized ECM-derived constructs is associated with the presence of residual EVs with regenerative effects, and ECM-derived EVs are currently considered as vehicle for the functionalisation of bioscaffolds (92).
The potential of EVs for (supporting) articular tissue regeneration has already stimulated the development of biodegradable EV-like microparticles (referred to as microspheres) for controlled delivery in the joint. For example, transforming growth factor beta-1, bone morphogenetic protein 2 and insulin-like growth factor 1 have been incorporated successfully into microspheres (93). Several of these bioactive molecules have also been found as cargo of EVs (34, 94). Hence, the use of nanovesicle-mediated delivery is expected to be more efficient than using the soluble form of the proteins, which are usually prone to fast degradation after injection. In addition since several miRNAs can support chondrogenesis and decrease inflammation, incorporation of miRNAs into artificial vesicles is an interesting option to assist in regenerative strategies. Currently, several possibilities are being explored for EV-loaded scaffolds (95–98).
Mesenchymal Stem Cell EVs for Joint Repair
Mesenchymal stem cells are seen as a promising cellular source in cartilage tissue engineering (99). The supposed mechanism through paracrine signaling is supported by several studies showing that conditioned medium from MSCs alone (100, 101), or even only the culture medium EV-fraction (102), is sufficient to induce beneficial effects to harmed tissue or to prevent tissue damage. This indicates that soluble biomolecules and possibly EVs are the main effectors in the MSC-driven regeneration cascade—not the capacity of these cells to differentiate into several lineages, as was thought for long. In 2016 we and others suggested the potential use of MSC-derived EVs as an off-the-shelf autologous regenerative treatment for tissue repair in the joint (79) and in the years that followed the first studies on this topic have been performed and showed that MSC-derived EVs indeed were able to promote osteochondral regeneration in vivo (97, 103) and cartilage regeneration in vitro (98). Recently, also the protective effect of MSC-derived EVs against bone and cartilage degradation in OA has been demonstrated (104).
In addition to MSCs, synovial membrane-derived cells with similar pluripotent characteristics as MSCs can sometimes be detected in SF or isolated from the synovial lining and their use for treatment of cartilage defects has already shown promising results (105–108). Also, specific chondrogenic progenitor cells have been found in articular cartilage and are seen as a potentially good cell source for cartilage repair (109, 110). These cells are therefore interesting EV donors for treatment of cartilage damage. Although their natural low abundance in the joint may be not sufficient for endogenous repair of cartilage defects, in vitro expansion of these cells and collection of the EVs they produce would allow for intra-articular administration of high concentrations of biologically active EVs.
Finally, lasting repair of joint defects can only be successful if the disturbed joint homeostasis is targeted in parallel. When joint disease presents with auto-immunity or (chronic) inflammation, immune downregulation can be achieved by using MSC-derived EVs (104). Together with the immunotherapeutic potential of other EV types (111) from different cellular sources, EV-mediated restoration of joint homeostasis can be effectuated, which is, as said, a prerequisite for durable joint repair.
Equine Model for ORTHOPEDIC EV Research
The use of animal models is under debate and efforts are made to develop alternatives by using advanced (bio)technology and in silico modeling. Currently, there is consensus that animal models are still necessary for various purposes, which includes setting up translational studies focussing on EVs. The choice of the animal model herein is critical. The overall role and functionality of EVs is most likely conserved in the system biology of most mammals or even vertebrates (112), albeit specific EV functions can be dependent on external cues, e.g., dietary and environmental factors (113). Larger animals are more convenient than the classic laboratory species with regard to sample size for the recovery of sufficient EVs and repeated harvesting is easier, but these larger animal species come with the disadvantage that many research tools, especially antibodies and genomic sequences, are not (yet) available.
For orthopedic research the horse is one of the optimal models because of the strong similarities between equine and human joints with respect to cartilage thickness and cellular and biochemical composition of the cartilage extracellular matrix (114). For this reason, results from fundamental studies on EVs in the equine joint can—with certain caution—be extrapolated to the human situation. Since the horse is a target species in itself with a clear clinical need for improved care for joint disorders, also equine medicine will benefit from the results of these studies.
So far, the horse has been used for studying joint biology on the EV level with the purpose of unraveling fundamental EV-mediated processes (115). Possibly in the near future, the horse can also serve as animal model for testing EV-mediated treatment of joint disease, for example by using (inducible) synovitis as a model for human arthritis (116–120) or for the assessment of repair of cartilage and bone lesions with EV-mediated therapies.
Conclusion
The interpretation of data from the relatively few studies performed so far, both for the analysis of EVs from SF and for the testing of EV-inspired drug delivery systems and EV-mediated therapies in joint disease and articular tissue repair, is hampered by the lack of consistent and standardized isolation and processing protocols (79). This is now recognized within the EV community and the International Society for Extracellular Vesicles (ISEV), and the development of experimental guidelines and requirements for standardized sample processing and EV isolation has become a high priority area in EV-research. These activities will also fuel the elucidation of the role of EVs in articular homeostasis and pathology. From this, the step toward potential use of EVs as biomarkers and even targeting or modification of specific EVs for therapeutic applications may come into reach. Given the observed anti-inflammatory and immune modulatory activity of certain EV subsets, the application of EVs for modulation of joint inflammation may be the first EV-application in joint diseases. The potential use of EVs as (decisive) stimulators of the regeneration of articular tissues in general and of hyaline cartilage in particular seems further away, as the roles of EVs in these processes are still elusive.
Author Contributions
JB drafted the manuscript and the figures. JM, CvdL, PvW, and MW edited the manuscript and made substantial direct and intellectual contribution to the work. All authors approved the manuscript before submission.
Funding
This work was supported by a grant from the Dutch government to the Netherlands Institute for Regenerative Medicine (NIRM, grant No. FES0908), the Dutch Arthritis Foundation (grants No. LLP-12 and LLP-22) and the European Research Council (grant No. 647426 [3D-JOINT]).
Conflict of Interest Statement
The authors declare that the research was conducted in the absence of any commercial or financial relationships that could be construed as a potential conflict of interest.
References
1. Lunenfeld B, Stratton P. The clinical consequences of an ageing world and preventive strategies. Best Pract Res Clin Obstet Gynaecol. (2013) 27:643–59. doi: 10.1016/j.bpobgyn.2013.02.005
2. Caron J. Osteoarthritis. In: Ross M, Dyson S, editors. Diagnosis and Management of Lameness in the Horse. Philadelphia, PA: Elsevier Saunders (2011). p. 655–68.
3. Hui AY, McCarty WJ, Masuda K, Firestein GS, Sah RL. A systems biology approach to synovial joint lubrication in health, injury, and disease. Wiley Interdiscip Rev Syst Biol Med. (2012) 4:15–37. doi: 10.1002/wsbm.157
4. de Grauw JC. Molecular monitoring of equine joint homeostasis. Vet Q. (2011) 31:77–86. doi: 10.1080/01652176.2011.565546
5. Goldring MB, Marcu KB. Cartilage homeostasis in health and rheumatic diseases. Arthritis Res Ther. (2009) 11:224. doi: 10.1186/ar2592
6. Wojdasiewicz P, Poniatowski LA, Szukiewicz D. The role of inflammatory and anti-inflammatory cytokines in the pathogenesis of osteoarthritis. Mediators Inflamm. (2014) 2014:561459. doi: 10.1155/2014/561459
7. Camussi G, Deregibus MC, Bruno S, Cantaluppi V, Biancone L. Exosomes/microvesicles as a mechanism of cell-to-cell communication. Kidney Int. (2010) 78:838–48. doi: 10.1038/ki.2010.278
8. Thery C, Ostrowski M, Segura E. Membrane vesicles as conveyors of immune responses. Nat Rev Immunol. (2009) 9:581–93. doi: 10.1038/nri2567
9. Mulcahy LA, Pink RC, Carter DR. Routes and mechanisms of extracellular vesicle uptake. J Extracell Vesic. (2014) 3. doi: 10.3402/jev.v3.24641
10. Yanez-Mo M, Siljander PR, Andreu Z, Zavec AB, Borras FE, Buzas EI, et al. Biological properties of extracellular vesicles and their physiological functions. J Extracell Vesic. (2015) 4:27066. doi: 10.3402/jev.v4.27066
11. van Niel G, D'Angelo G, Raposo G. Shedding light on the cell biology of extracellular vesicles. Nat Rev Mol Cell Biol. (2018) 19:213–28. doi: 10.1038/nrm.2017.125
12. Subra C, Grand D, Laulagnier K, Stella A, Lambeau G, Paillasse M, et al. Exosomes account for vesicle-mediated transcellular transport of activatable phospholipases and prostaglandins. J Lipid Res. (2010) 51:2105–20. doi: 10.1194/jlr.M003657
13. Record M, Carayon K, Poirot M, Silvente-Poirot S. Exosomes as new vesicular lipid transporters involved in cell-cell communication and various pathophysiologies. Biochim Biophys Acta (2014) 1841:108–20. doi: 10.1016/j.bbalip.2013.10.004
14. Valadi H, Ekstrom K, Bossios A, Sjostrand M, Lee JJ, Lotvall JO. Exosome-mediated transfer of mRNAs and microRNAs is a novel mechanism of genetic exchange between cells. Nat Cell Biol. (2007) 9:654–9. doi: 10.1038/ncb1596
15. Yoon YJ, Kim OY, Gho YS. Extracellular vesicles as emerging intercellular communicasomes. BMB Rep. (2014) 47:531–9. doi: 10.5483/BMBRep.2014.47.10.164
16. Subra C, Laulagnier K, Perret B, Record M. Exosome lipidomics unravels lipid sorting at the level of multivesicular bodies. Biochimie (2007) 89:205–12. doi: 10.1016/j.biochi.2006.10.014
17. Llorente A, Skotland T, Sylvanne T, Kauhanen D, Rog T, Orlowski A, et al. Molecular lipidomics of exosomes released by PC-3 prostate cancer cells. Biochim Biophys Acta (2013) 1831:1302–9. doi: 10.1016/j.bbalip.2013.04.011
18. Solomon DH, Browning JA, Wilkins RJ. Inorganic phosphate transport in matrix vesicles from bovine articular cartilage. Acta Physiol. (2007) 190:119–25. doi: 10.1111/j.1748-1716.2007.01670.x
19. Kirsch T, Harrison G, Golub EE, Nah HD. The roles of annexins and types II and X collagen in matrix vesicle-mediated mineralization of growth plate cartilage. J Biol Chem. (2000) 275:35577–83. doi: 10.1074/jbc.M005648200
20. De Toro J, Herschlik L, Waldner C, Mongini C. Emerging roles of exosomes in normal and pathological conditions: new insights for diagnosis and therapeutic applications. Front Immunol. (2015) 6:203. doi: 10.3389/fimmu.2015.00203
21. Nolte-'t Hoen EN, Wauben MH. Immune cell-derived vesicles: modulators and mediators of inflammation. Curr Pharm Des. (2012) 18:2357–68. doi: 10.2174/138161212800166013
22. van der Vlist EJ, Arkesteijn GJ, van de Lest CH, Stoorvogel W, Nolte-'t Hoen EN, Wauben MH. CD4(+) T cell activation promotes the differential release of distinct populations of nanosized vesicles. J Extracell Vesic. (2012) 1. doi: 10.3402/jev.v1i0.18364
23. Kim JH, Lee J, Park J, Gho YS. Gram-negative and Gram-positive bacterial extracellular vesicles. Semin Cell Dev Biol. (2015) 40:97–104. doi: 10.1016/j.semcdb.2015.02.006
24. Szempruch AJ, Dennison L, Kieft R, Harrington JM, Hajduk SL. Sending a message: extracellular vesicles of pathogenic protozoan parasites. Nat Rev Microbiol. (2016) 14:669–75. doi: 10.1038/nrmicro.2016.110
25. Alvarez-Erviti L, Seow Y, Yin H, Betts C, Lakhal S, Wood MJ. Delivery of siRNA to the mouse brain by systemic injection of targeted exosomes. Nat Biotechnol. (2011) 29:341–5. doi: 10.1038/nbt.1807
26. Boukouris S, Mathivanan S. Exosomes in bodily fluids are a highly stable resource of disease biomarkers. Proteomics Clin Appl. (2015) 9:358–67. doi: 10.1002/prca.201400114
27. Verma M, Lam TK, Hebert E, Divi RL. Extracellular vesicles: potential applications in cancer diagnosis, prognosis, and epidemiology. BMC Clin Pathol. (2015) 15:6. doi: 10.1186/s12907-015-0005-5
28. Gamez-Valero A, Lozano-Ramos SI, Bancu I, Lauzurica-Valdemoros R, Borras FE. Urinary extracellular vesicles as source of biomarkers in kidney diseases. Front Immunol. (2015) 6:6. doi: 10.3389/fimmu.2015.00006
29. Anderson HC. Electron microscopic studies of induced cartilage development and calcification. J Cell Biol. (1967) 35:81–101.
30. Anderson HC. Vesicles associated with calcification in the matrix of epiphyseal cartilage. J Cell Biol. (1969) 41:59–72.
32. Hessle L, Johnson KA, Anderson HC, Narisawa S, Sali A, Goding JW, et al. Tissue-nonspecific alkaline phosphatase and plasma cell membrane glycoprotein-1 are central antagonistic regulators of bone mineralization. Proc Natl Acad Sci USA. (2002) 99:9445–9. doi: 10.1073/pnas.142063399
33. Anderson HC. Matrix vesicles and calcification. Curr Rheumatol Rep. (2003) 5:222–6. doi: 10.1007/s11926-003-0071-z
34. Nahar NN, Missana LR, Garimella R, Tague SE, Anderson HC. Matrix vesicles are carriers of bone morphogenetic proteins (BMPs), vascular endothelial growth factor (VEGF), and noncollagenous matrix proteins. J Bone Miner Metab. (2008) 26:514–9. doi: 10.1007/s00774-008-0859-z
35. Cui L, Houston DA, Farquharson C, MacRae VE. Characterisation of matrix vesicles in skeletal and soft tissue mineralisation. Bone (2016) 87:147–58. doi: 10.1016/j.bone.2016.04.007
37. Stewart AJ, Roberts SJ, Seawright E, Davey MG, Fleming RH, Farquharson C. The presence of PHOSPHO1 in matrix vesicles and its developmental expression prior to skeletal mineralization. Bone (2006) 39:1000–7. doi: 10.1016/j.bone.2006.05.014
38. Johnson KA, Hessle L, Vaingankar S, Wennberg C, Mauro S, Narisawa S, et al. Osteoblast tissue-nonspecific alkaline phosphatase antagonizes and regulates PC-1. Am J Physiol Regul Integr Comp Physiol. (2000) 279:R1365–77. doi: 10.1152/ajpregu.2000.279.4.R1365
39. Roberts S, Narisawa S, Harmey D, Millan JL, Farquharson C. Functional involvement of PHOSPHO1 in matrix vesicle-mediated skeletal mineralization. J Bone Miner Res. (2007) 22:617–27. doi: 10.1359/jbmr.070108
40. Roberts SJ, Stewart AJ, Sadler PJ, Farquharson C. Human PHOSPHO1 exhibits high specific phosphoethanolamine and phosphocholine phosphatase activities. Biochem J. (2004) 382:59–65. doi: 10.1042/BJ20040511
41. Iannotti JP, Naidu S, Noguchi Y, Hunt RM, Brighton CT. Growth plate matrix vesicle biogenesis. The role of intracellular calcium. Clin Orthop Relat Res. (1994) 306:222–9.
42. Roach HI, Clarke NM. Physiological cell death of chondrocytes in vivo is not confined to apoptosis. New observations on the mammalian growth plate. J Bone Joint Surg Br. (2000) 82:601–13. doi: 10.1302/0301-620X.82B4.9846
43. Morhayim J, Baroncelli M, van Leeuwen JP. Extracellular vesicles: specialized bone messengers. Arch Biochem Biophys. (2014) 561:38–45. doi: 10.1016/j.abb.2014.05.011
44. Lefebvre V, Bhattaram P. Vertebrate skeletogenesis. Curr Top Dev Biol. (2010) 90:291–317. doi: 10.1016/S0070-2153(10)90008-2
45. Colnot C. Cellular and molecular interactions regulating skeletogenesis. J Cell Biochem. (2005) 95:688–97. doi: 10.1002/jcb.20449
46. Kobayashi T, Lyons KM, McMahon AP, Kronenberg HM. BMP signaling stimulates cellular differentiation at multiple steps during cartilage development. Proc Natl Acad Sci USA. (2005) 102:18023–7. doi: 10.1073/pnas.0503617102
47. Mackie EJ, Ahmed YA, Tatarczuch L, Chen K-, Mirams M. Endochondral ossification: How cartilage is converted into bone in the developing skeleton. Int J Biochem Cell Biol. (2008) 40:46–62. doi: 10.1016/j.biocel.2007.06.009
48. Corrigan L, Redhai S, Leiblich A, Fan SJ, Perera SM, Patel R, et al. BMP-regulated exosomes from Drosophila male reproductive glands reprogram female behavior. J Cell Biol. (2014) 206:671–88. doi: 10.1083/jcb.201401072
49. Wendler F, Bota-Rabassedas N, Franch-Marro X. Cancer becomes wasteful: emerging roles of exosomes(dagger) in cell-fate determination. J Extracell Vesic. (2013) 2. doi: 10.3402/jev.v2i0.22390
50. Gross JC, Chaudhary V, Bartscherer K, Boutros M. Active Wnt proteins are secreted on exosomes. Nat Cell Biol. (2012) 14:1036–45. doi: 10.1038/ncb2574
51. Hosaka Y, Saito T, Sugita S, Hikata T, Kobayashi H, Fukai A, et al. Notch signaling in chondrocytes modulates endochondral ossification and osteoarthritis development. Proc Natl Acad Sci USA. (2013) 110:1875–80. doi: 10.1073/pnas.1207458110
52. Mirando AJ, Liu Z, Moore T, Lang A, Kohn A, Osinski AM, et al. RBP-Jkappa-dependent Notch signaling is required for murine articular cartilage and joint maintenance. Arthritis Rheum. (2013) 65:2623–33. doi: 10.1002/art.38076
53. Ristorcelli E, Beraud E, Mathieu S, Lombardo D, Verine A. Essential role of Notch signaling in apoptosis of human pancreatic tumoral cells mediated by exosomal nanoparticles. Int J Cancer (2009) 125:1016–26. doi: 10.1002/ijc.24375
54. Sheldon H, Heikamp E, Turley H, Dragovic R, Thomas P, Oon CE, et al. New mechanism for Notch signaling to endothelium at a distance by Delta-like 4 incorporation into exosomes. Blood (2010) 116:2385–94. doi: 10.1182/blood-2009-08-239228
55. Berckmans RJ, Nieuwland R, Tak PP, Boing AN, Romijn FP, Kraan MC, et al. Cell-derived microparticles in synovial fluid from inflamed arthritic joints support coagulation exclusively via a factor VII-dependent mechanism. Arthritis Rheum. (2002) 46:2857–66. doi: 10.1002/art.10587
56. Berckmans RJ, Nieuwland R, Kraan MC, Schaap MC, Pots D, Smeets TJ, et al. Synovial microparticles from arthritic patients modulate chemokine and cytokine release by synoviocytes. Arthritis Res Ther. (2005) 7:R536–44. doi: 10.1186/ar1706
57. Biro E, Nieuwland R, Tak PP, Pronk LM, Schaap MC, Sturk A, et al. Activated complement components and complement activator molecules on the surface of cell-derived microparticles in patients with rheumatoid arthritis and healthy individuals. Ann Rheum Dis. (2007) 66:1085–92. doi: 10.1136/ard.2006.061309
58. Boilard E, Nigrovic PA, Larabee K, Watts GF, Coblyn JS, Weinblatt ME, et al. Platelets amplify inflammation in arthritis via collagen-dependent microparticle production. Science (2010) 327:580–3. doi: 10.1126/science.1181928
59. Cloutier N, Tan S, Boudreau LH, Cramb C, Subbaiah R, Lahey L, et al. The exposure of autoantigens by microparticles underlies the formation of potent inflammatory components: the microparticle-associated immune complexes. EMBO Mol Med. (2013) 5:235–49. doi: 10.1002/emmm.201201846
60. Duchez AC, Boudreau LH, Naika GS, Bollinger J, Belleannee C, Cloutier N, et al. Platelet microparticles are internalized in neutrophils via the concerted activity of 12-lipoxygenase and secreted phospholipase A2-IIA. Proc Natl Acad Sci USA. (2015) 112:E3564–73. doi: 10.1073/pnas.1507905112
61. Fourcade O, Simon MF, Viode C, Rugani N, Leballe F, Ragab A, et al. Secretory phospholipase A2 generates the novel lipid mediator lysophosphatidic acid in membrane microvesicles shed from activated cells. Cell (1995) 80:919–27.
62. Gyorgy B, Modos K, Pallinger E, Paloczi K, Pasztoi M, Misjak P, et al. Detection and isolation of cell-derived microparticles are compromised by protein complexes resulting from shared biophysical parameters. Blood (2011) 117:e39–48. doi: 10.1182/blood-2010-09-307595
63. Gyorgy B, Szabo TG, Turiak L, Wright M, Herczeg P, Ledeczi Z, et al. Improved flow cytometric assessment reveals distinct microvesicle (cell-derived microparticle) signatures in joint diseases. PLoS ONE (2012) 7:e49726. doi: 10.1371/journal.pone.0049726
64. Headland SE, Jones HR, Norling LV, Kim A, Souza PR, Corsiero E, et al. Neutrophil-derived microvesicles enter cartilage and protect the joint in inflammatory arthritis. Sci Transl Med. (2015) 7:315ra190. doi: 10.1126/scitranslmed.aac5608
65. Junkar I, Sustar V, Frank M, Jansa V, Zavec A, Rozman B, et al. Blood and synovial microparticles as revealed by atomic force and scanning electron microscope. Open Autoimmun J. (2009) 1:50–8. doi: 10.2174/1876894600901010050
66. Martinez-Lorenzo MJ, Anel A, Saez-Gutierrez B, Royo-Canas M, Bosque A, Alava MA, et al. Rheumatoid synovial fluid T cells are sensitive to APO2L/TRAIL. Clin Immunol. (2007) 122:28–40. doi: 10.1016/j.clim.2006.07.007
67. Matei CI, Boulocher C, Boule C, Schramme M, Viguier E, Roger T, et al. Ultrastructural analysis of healthy synovial fluids in three mammalian species. Microsc Microanal. (2014) 20:903–11. doi: 10.1017/S1431927614000415
68. Messer L, Alsaleh G, Freyssinet J, Zobairi F, Leray I, Gottenberg J, et al. Microparticle-induced release of B-lymphocyte regulators by rheumatoid synoviocytes. Arthritis Res Ther. (2009) 11:R40. doi: 10.1186/ar2648
69. Pasztoi M, Sodar B, Misjak P, Paloczi K, Kittel A, Toth K, et al. The recently identified hexosaminidase D enzyme substantially contributes to the elevated hexosaminidase activity in rheumatoid arthritis. Immunol Lett. (2013) 149:71–6. doi: 10.1016/j.imlet.2012.10.012.
70. Skriner K, Adolph K, Jungblut PR, Burmester GR. Association of citrullinated proteins with synovial exosomes. Arthritis Rheum. (2006) 54:3809–14. doi: 10.1002/art.22276
71. Mustonen AM, Nieminen P, Joukainen A, Jaroma A, Kaariainen T, Kroger H, et al. First in vivo detection and characterization of hyaluronan-coated extracellular vesicles in human synovial fluid. J Orthop Res. (2016) 34:1960–8. doi: 10.1002/jor.23212
72. Reich N, Beyer C, Gelse K, Akhmetshina A, Dees C, Zwerina J, et al. Microparticles stimulate angiogenesis by inducing ELR(+) CXC-chemokines in synovial fibroblasts. J Cell Mol Med. (2011) 15:756–62. doi: 10.1111/j.1582-4934.2010.01051.x
73. Attur MG, Dave M, Akamatsu M, Katoh M, Amin AR. Osteoarthritis or osteoarthrosis: the definition of inflammation becomes a semantic issue in the genomic era of molecular medicine. Osteoarthritis Cartilage (2002) 10:1–4. doi: 10.1053/joca.2001.0488
74. Rahmati M, Mobasheri A, Mozafari M. Inflammatory mediators in osteoarthritis: a critical review of the state-of-the-art, current prospects, and future challenges. Bone (2016) 85:81–90. doi: 10.1016/j.bone.2016.01.019
75. Domenis R, Zanutel R, Caponnetto F, Toffoletto B, Cifu A, Pistis C, et al. Characterization of the proinflammatory profile of synovial fluid-derived exosomes of patients with osteoarthritis. Mediators Inflamm. (2017) 2017:4814987. doi: 10.1155/2017/4814987
76. Kolhe R, Hunter M, Liu S, Jadeja RN, Pundkar C, Mondal AK, et al. Gender-specific differential expression of exosomal miRNA in synovial fluid of patients with osteoarthritis. Sci Rep. (2017) 7:2029. doi: 10.1038/s41598-017-01905-y
77. Kato T, Miyaki S, Ishitobi H, Nakamura Y, Nakasa T, Lotz MK, et al. Exosomes from IL-1beta stimulated synovial fibroblasts induce osteoarthritic changes in articular chondrocytes. Arthritis Res Ther. (2014) 16:R163. doi: 10.1186/ar4679
78. Jungel A, Distler O, Schulze-Horsel U, Huber LC, Ha HR, Simmen B, et al. Microparticles stimulate the synthesis of prostaglandin E(2) via induction of cyclooxygenase 2 and microsomal prostaglandin E synthase 1. Arthritis Rheum. (2007) 56:3564–74. doi: 10.1002/art.22980
79. Malda J, Boere J, van de Lest CH, van Weeren P, Wauben MH. Extracellular vesicles–new tool for joint repair and regeneration. Nat Rev Rheumatol. (2016) 12:243–9. doi: 10.1038/nrrheum.2015.170
80. Buzas EI, Gyorgy B, Nagy G, Falus A, Gay S. Emerging role of extracellular vesicles in inflammatory diseases. Nat Rev Rheumatol. (2014) 10:356–64. doi: 10.1038/nrrheum.2014.19
81. Distler JH, Pisetsky DS, Huber LC, Kalden JR, Gay S, Distler O. Microparticles as regulators of inflammation. Novel players of cellular crosstalk in the rheumatic diseases. Arthritis Rheum. (2005) 52:3337–48. doi: 10.1002/art.21350
82. Greisen SR, Yan Y, Hansen AS, Veno MT, Nyengaard JR, Moestrup SK, et al. Extracellular vesicles transfer the receptor programmed death-1 in rheumatoid arthritis. Front Immunol. (2017) 8:851. doi: 10.3389/fimmu.2017.00851
83. Turpin D, Truchetet ME, Faustin B, Augusto JF, Contin-Bordes C, Brisson A, et al. Role of extracellular vesicles in autoimmune diseases. Autoimmun Rev. (2016) 15:174–83. doi: 10.1016/j.autrev.2015.11.004
84. Fu H, Hu D, Zhang L, Tang P. Role of extracellular vesicles in rheumatoid arthritis. Mol Immunol. (2018) 93:125–32. doi: 10.1016/j.molimm.2017.11.016
85. Rhys HI, Dell'Accio F, Pitzalis C, Moore A, Norling LV, Perretti M. Neutrophil microvesicles from healthy control and rheumatoid arthritis patients prevent the inflammatory activation of macrophages. EBioMed. (2018) 29:60–9. doi: 10.1016/j.ebiom.2018.02.003
86. Hunter W. Of the structure and disease of articular cartilages. Philos Trans R Soc Lond. (1742):514–21.
87. Smith BD, Grande DA. The current state of scaffolds for musculoskeletal regenerative applications. Nat Rev Rheumatol. (2015) 11:213–22. doi: 10.1038/nrrheum.2015.27.
88. Malda J, Visser J, Melchels FP, Jungst T, Hennink WE, Dhert WJ, et al. 25th anniversary article: engineering hydrogels for biofabrication. Adv Mater. (2013) 25:5011–28. doi: 10.1002/adma.201302042
89. Visser J, Peters B, Burger TJ, Boomstra J, Dhert WJ, Melchels FP, et al. Biofabrication of multi-material anatomically shaped tissue constructs. Biofabrication (2013) 5:035007. doi: 10.1088/1758-5082/5/3/035007
90. Makris EA, Gomoll AH, Malizos KN, Hu JC, Athanasiou KA. Repair and tissue engineering techniques for articular cartilage. Nat Rev Rheumatol. (2015) 11:21–34. doi: 10.1038/nrrheum.2014.157
91. de Windt TS, Vonk LA, Slaper-Cortenbach IC, van den Broek MP, Nizak R, van Rijen MH, et al. Allogeneic Mesenchymal stem cells stimulate cartilage regeneration and are safe for single-stage cartilage repair in humans upon mixture with recycled autologous chondrons. Stem Cells (2017) 35:256–64. doi: 10.1002/stem.2475
92. Huleihel L, Hussey GS, Naranjo JD, Zhang L, Dziki JL, Turner NJ, et al. Matrix-bound nanovesicles within ECM bioscaffolds. Sci Adv. (2016) 2:e1600502. doi: 10.1126/sciadv.1600502
93. Lam J, Lu S, Kasper FK, Mikos AG. Strategies for controlled delivery of biologics for cartilage repair. Adv Drug Deliv Rev. (2015) 84:123–34. doi: 10.1016/j.addr.2014.06.006
94. Proia P, Schiera G, Mineo M, Ingrassia AM, Santoro G, Savettieri G, et al. Astrocytes shed extracellular vesicles that contain fibroblast growth factor-2 and vascular endothelial growth factor. Int J Mol Med. (2008) 21:63–7. doi: 10.3892/ijmm.21.1.63
95. Zhang J, Liu X, Li H, Chen C, Hu B, Niu X, et al. Exosomes/tricalcium phosphate combination scaffolds can enhance bone regeneration by activating the PI3K/Akt signaling pathway. Stem Cell Res Ther. (2016) 7:136. doi: 10.1186/s13287-016-0391-3
96. Toh WS, Lai RC, Hui JH, Lim SK. MSC exosome as a cell-free MSC therapy for cartilage regeneration: Implications for osteoarthritis treatment. Semin Cell Dev Biol. (2017) 67:56–64. doi: 10.1016/j.semcdb.2016.11.008
97. Zhang S, Chu WC, Lai RC, Lim SK, Hui JH, Toh WS. Exosomes derived from human embryonic mesenchymal stem cells promote osteochondral regeneration. Osteoarthritis Cartilage (2016) 24:2135–40. doi: 10.1016/j.joca.2016.06.022
98. Vonk LA, van Dooremalen SFJ, Liv N, Klumperman J, Coffer PJ, Saris DBF, et al. Mesenchymal stromal/stem cell-derived extracellular vesicles promote human cartilage regeneration in vitro. Theranostics (2018) 8:906–20. doi: 10.7150/thno.20746
99. Savkovic V, Li H, Seon JK, Hacker M, Franz S, Simon JC. Mesenchymal stem cells in cartilage regeneration. Curr Stem Cell Res Ther. (2014) 9:469–88. doi: 10.2174/1574888X09666140709111444
100. Gnecchi M, He H, Liang OD, Melo LG, Morello F, Mu H, et al. Paracrine action accounts for marked protection of ischemic heart by Akt-modified mesenchymal stem cells. Nat Med. (2005) 11:367–8. doi: 10.1038/nm0405-367
101. van Buul GM, Villafuertes E, Bos PK, Waarsing JH, Kops N, Narcisi R, et al. Mesenchymal stem cells secrete factors that inhibit inflammatory processes in short-term osteoarthritic synovium and cartilage explant culture. Osteoarthritis Cartilage (2012) 20:1186–96. doi: 10.1016/j.joca.2012.06.003
102. Lai RC, Arslan F, Lee MM, Sze NS, Choo A, Chen TS, et al. Exosome secreted by MSC reduces myocardial ischemia/reperfusion injury. Stem Cell Res. (2010) 4:214–22. doi: 10.1016/j.scr.2009.12.003
103. Zhang S, Chuah SJ, Lai RC, Hui JHP, Lim SK, Toh WS. MSC exosomes mediate cartilage repair by enhancing proliferation, attenuating apoptosis and modulating immune reactivity. Biomaterials (2018) 156:16–27. doi: 10.1016/j.biomaterials.2017.11.028
104. Cosenza S, Ruiz M, Toupet K, Jorgensen C, Noel D. Mesenchymal stem cells derived exosomes and microparticles protect cartilage and bone from degradation in osteoarthritis. Sci Rep. (2017) 7:16214. doi: 10.1038/s41598-017-15376-8
105. Gullo F, De Bari C. Prospective purification of a subpopulation of human synovial mesenchymal stem cells with enhanced chondro-osteogenic potency. Rheumatology (2013) 52:1758–68. doi: 10.1093/rheumatology/ket205
106. Lee JC, Min HJ, Park HJ, Lee S, Seong SC, Lee MC. Synovial membrane-derived mesenchymal stem cells supported by platelet-rich plasma can repair osteochondral defects in a rabbit model. Arthroscopy (2013) 29:1034–46. doi: 10.1016/j.arthro.2013.02.026
107. Murata D, Miyakoshi D, Hatazoe T, Miura N, Tokunaga S, Fujiki M, et al. Multipotency of equine mesenchymal stem cells derived from synovial fluid. Vet J. (2014) 202:53–61. doi: 10.1016/j.tvjl.2014.07.029
108. Tao SC, Yuan T, Zhang YL, Yin WJ, Guo SC, Zhang CQ. Exosomes derived from miR-140-5p-overexpressing human synovial mesenchymal stem cells enhance cartilage tissue regeneration and prevent osteoarthritis of the knee in a rat model. Theranostics (2017) 7:180–95. doi: 10.7150/thno.17133
109. Jiang Y, Tuan RS. Origin and function of cartilage stem/progenitor cells in osteoarthritis. Nat Rev Rheumatol. (2015) 11:206–12. doi: 10.1038/nrrheum.2014.200
110. McCarthy HE, Bara JJ, Brakspear K, Singhrao SK, Archer CW. The comparison of equine articular cartilage progenitor cells and bone marrow-derived stromal cells as potential cell sources for cartilage repair in the horse. Vet J. (2012) 192:345–51. doi: 10.1016/j.tvjl.2011.08.036
111. Zhang B, Yin Y, Lai RC, Lim SK. Immunotherapeutic potential of extracellular vesicles. Front Immunol. (2014) 5:518. doi: 10.3389/fimmu.2014.00518
112. Vyas N, Walvekar A, Tate D, Lakshmanan V, Bansal D, Lo Cicero A, et al. Vertebrate Hedgehog is secreted on two types of extracellular vesicles with different signaling properties. Sci Rep. (2014) 4:7357. doi: 10.1038/srep07357
113. Neven KY, Nawrot TS, Bollati V. Extracellular Vesicles: How the external and internal environment can shape cell-to-cell communication. Curr Environ Health Rep. (2017) 4:30–7. doi: 10.1007/s40572-017-0130-7
114. Malda J, Benders KE, Klein TJ, de Grauw JC, Kik MJ, Hutmacher DW, et al. Comparative study of depth-dependent characteristics of equine and human osteochondral tissue from the medial and lateral femoral condyles. Osteoarthritis Cartilage (2012) 20:1147–51. doi: 10.1016/j.joca.2012.06.005
115. Boere J, van de Lest CH, Libregts SF, Arkesteijn GJ, Geerts WJ, Nolte-'t Hoen EN, et al. Synovial fluid pretreatment with hyaluronidase facilitates isolation of CD44+ extracellular vesicles. J Extracell Vesic. (2016) 5:31751. doi: 10.3402/jev.v5.31751
116. de Grauw JC, van de Lest CH, Brama PA, Rambags BP, van Weeren PR. In vivo effects of meloxicam on inflammatory mediators, MMP activity and cartilage biomarkers in equine joints with acute synovitis. Equine Vet J. (2009) 41:693–9. doi: 10.2746/042516409X436286
117. Ross TN, Kisiday JD, Hess T, McIlwraith CW. Evaluation of the inflammatory response in experimentally induced synovitis in the horse: a comparison of recombinant equine interleukin 1 beta and lipopolysaccharide. Osteoarthritis Cartilage (2012) 20:1583–90. doi: 10.1016/j.joca.2012.08.008
118. Palmer JL, Bertone AL. Experimentally-induced synovitis as a model for acute synovitis in the horse. Equine Vet J. (1994) 26:492–5.
119. de Grauw JC, van de Lest CH, van Weeren PR. Inflammatory mediators and cartilage biomarkers in synovial fluid after a single inflammatory insult: a longitudinal experimental study. Arthritis Res Ther. (2009) 11:R35. doi: 10.1186/ar2640
Keywords: extracellular vesicle, joint, inflammation, immune suppression, cartilage, therapy, regeneration, joint homeostasis
Citation: Boere J, Malda J, van de Lest CHA, van Weeren PR and Wauben MHM (2018) Extracellular Vesicles in Joint Disease and Therapy. Front. Immunol. 9:2575. doi: 10.3389/fimmu.2018.02575
Received: 29 May 2018; Accepted: 18 October 2018;
Published: 12 November 2018.
Edited by:
Denise Doolan, James Cook University, AustraliaReviewed by:
Estrella Mariel Levy, Consejo Nacional de Investigaciones Científicas y Técnicas (CONICET), ArgentinaRae Ritchie, Cook Biotech, United States
Copyright © 2018 Boere, Malda, van de Lest, van Weeren and Wauben. This is an open-access article distributed under the terms of the Creative Commons Attribution License (CC BY). The use, distribution or reproduction in other forums is permitted, provided the original author(s) and the copyright owner(s) are credited and that the original publication in this journal is cited, in accordance with accepted academic practice. No use, distribution or reproduction is permitted which does not comply with these terms.
*Correspondence: Marca H. M. Wauben, bS5oLm0ud2F1YmVuQHV1Lm5s
†These authors have contributed equally to this work and share co-senior authorship