- 1Swansea University Medical School, Swansea, United Kingdom
- 2Calon Cardio-Technology Ltd, Institute of Life Science, Swansea, United Kingdom
- 3Scandinavian Real Heart AB, Västerås, Sweden
- 4Department of Cardiology, Morriston Hospital, Abertawe Bro Morgannwg University Health Board, Swansea, United Kingdom
The therapeutic use of ventricular assist devices (VADs) for end-stage heart failure (HF) patients who are ineligible for transplant has increased steadily in the last decade. In parallel, improvements in VAD design have reduced device size, cost, and device-related complications. These complications include infection and thrombosis which share underpinning contribution from the inflammatory response and remain common risks from VAD implantation. An added and underappreciated difficulty in designing a VAD that supports heart function and aids the repair of damaged myocardium is that different types of HF are accompanied by different inflammatory profiles that can affect the response to the implanted device. Circulating inflammatory markers and changes in leukocyte phenotypes receive much attention as biomarkers for mortality and disease progression. However, they are seldom used to monitor progress during and outcomes from VAD therapy or during the design phase for new devices. Even the partial reversal of heart damage associated with heart failure is a desirable outcome from VAD use. Therefore, improved understanding of the interplay between VADs and the recipient's inflammatory response would potentially increase their uptake, improve patient lives, and fuel research related to other blood-contacting medical devices. Here we provide a review of what is currently known about inflammation in heart failure and how this inflammatory profile is altered in heart failure patients receiving VAD therapy.
Introduction
Heart failure (HF) is a progressive syndrome which occurs when cardiac structure or function becomes abnormal leading to failure of the heart to deliver oxygen proportionate with the requirements of metabolizing tissues (1). Symptoms of HF are fairly non-discriminating (breathlessness, fatigue, and ankle swelling) which makes early diagnosis challenging, particularly in the elderly and obese (2). HF affects at least 26 million people worldwide (3) with an expected increase in prevalence of 25% by 2030 (4). The associated healthcare costs, already estimated at $30 billion per year in the USA, are calculated to double in that period (5).
There are three categories of HF based on the measurement of left ventricular ejection fraction (LVEF). The most well-known and easiest to diagnose is heart failure with reduced ejection fraction (HFrEF, <40%). Heart failure with preserved ejection fraction (HFpEF, ≥50%) has different pathophysiological mechanisms (6) and is characterized by stiffening of the left ventricle and delayed early relaxation which contributes to elevated pressures in the left atrium (7). A newer category, heart failure with mid-range ejection fraction (HFmrEF, 40–49%), was established to encourage research into patients with different symptoms to the other two categories (8).
The progression of HF has been classified into stages A-D by the American College of Cardiology (ACC) and into stages I-IV by the New York Heart Association (NYHA) (9, 10). The stages are interchangeable and are intended to reliably identify patients for the correct treatment. The Interagency Registry for Mechanically Assisted Circulatory Support (INTERMACS) profiling system comes in during stage C/NYHA IIIb (Figure 1) to determine whether the patient is suitable for transplant or mechanical circulatory support (MCS) which includes left ventricular assist devices (VADs) (11).
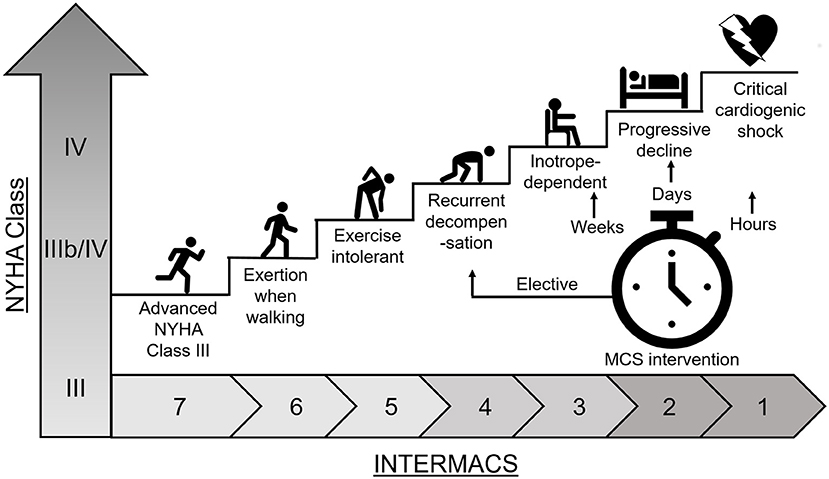
Figure 1. Heart failure disease progression. Heart failure disease progression and INTERMACS profile for determination of patient treatment with mechanical circulatory support (MCS).
Heart transplantation remains the ultimate cure for HF with approximately 7000 hearts transplanted globally per year (12), yet a vast majority of patients are classed as ineligible for transplant (13) there is a great need for implantable mechanical circulatory support such as VADs. Despite VADs being life-saving therapy, adverse effects such as gastrointestinal bleeding, infection, stroke, and device thrombosis are still significant complications (14). As the inflammatory response can contribute to these and other complications, the aim here is to review the effect of VAD treatment on the inflammatory profile of HF patients, especially as it relates to leukocytes.
Inflammation in Heart Failure
Heart failure is the result of stress to the heart due to a number of causative factors including coronary artery disease, hypertension, arrhythmias, diabetes, obesity, and age (8). The inflammatory profile of heart failure has been shown to differ depending on the ejection fraction category.
A common cause of HFrEF is myocardial infarction (2). MI-associated injury activates the innate immune system initiating a cascade of cytokines, chemokines, cell-adhesion molecules, and cell surface receptors designed to signal reparative cells to the area of injury (15). Activation of the immune system and release of inflammatory cytokines in the early stages helps maintain ventricular function (10) and is necessary to initiate repair of myocytes (15). During repair, the ventricle is remodeled which modifies size, shape, and contractility of the cardiac tissue cells (16, 17). The reduced blood flow at this time is compensated for by increasing blood volume through limiting salt release via urine. This places strain on the kidneys and the excess fluids can also affect the liver. Renal dysfunction causes derangements to metabolic and other circulating factors, which activates systemic inflammation (18). Excessive inflammation detrimentally affects repair of the heart and can culminate in cell damage and death leading to functional impairment of the myocardium through fibrosis and hypertrophy (15, 19, 20).
HFpEF on the other hand has a slightly different inflammatory profile. HFpEF can be caused by hypertension and has a bidirectional relationship with renal dysfunction (18, 21). These patients have increased blood levels of inflammatory cytokines such as tumor necrosis factor alpha (TNFα) interleukin-1 beta (IL-1β), and interleukin-6 (IL-6) (22) postulated to be linked to dysregulation of the cytokine network by regulatory T (Treg) cells (21, 23). IL-6 in particular is linked to myocardial fibrosis and increased myocardial stiffness (21).
The progression of HF can be measured through the heightened expression of inflammatory cytokines e.g., TNFα, IL-1, IL-6, IL-18, cardiotrophin-1, Fas ligand (FasL), monocyte chemoattractant protein 1 (MCP-1), IL-8, and macrophage inflammatory protein 1 alpha (MIP-1α) (21). These cytokines can be used as prognostic biomarkers (24–26) as circulating levels are directly proportional to deterioration in functional class and cardiac performance (27, 28). Increased levels of TNFα, IL-1β, IL-6, and galectin-3 (a galactose-specific lectin) levels can predict adverse outcomes (25). For example, galectin-3 is associated with inflammation and fibrosis, is a known mediator of heart failure development and progression (29) and is elevated significantly in NYHA I-IIIa vs. controls and further elevated in severe HF NYHA IIIB-IV (30). Another biomarker of HF includes N-Terminal-pro-BNP (NT-pro-BNP). Natriuretic peptides regulate volume and blood pressure homeostasis, but also have roles in the regulation of the immune response with receptors expressed on T cells, macrophages, and dendritic cells (31). Blood tests for these are available to diagnose congestive HF (32).
Inflammation in Response to Ventricular Assist Devices
VAD implantation can alleviate symptoms whilst maintaining a reasonable quality of life for patients with end-stage heart failure either awaiting or ineligible for heart transplant (10). VADs can partially reverse some of the pathological processes involved in advanced HF and restore myocardial function (33). Inflammatory biomarkers are of diagnostic value to clinicians to classify HF and monitor progression, but this has not been translated routinely for use during VAD therapy to predict positive or negative outcomes.
Given the heightened inflammatory milieu of any potential VAD recipient, as discussed above, it is critical to better understand both the impact of the VAD on the patient's inflammatory profile and to address this during the development of new VADs. These same markers could also be used to monitor patients during VAD therapy for improvement or detecting and preventing adverse effects.
Mechanisms Through Which VADs Influence Inflammation
The mechanisms through which VADs influence inflammation are yet to be fully understood. First generation VADs, such as the pneumatic VAD (PVAD; Thoratec, CA, USA), are pulsatile volume displacement pumps that mimic a physiological pulse but their size, durability, and complication rates has led to discontinuation of use. The second and third generation VADs are continuous flow pumps that have either an axial or centrifugal rotor. These pumps have the benefit of being smaller in size to ease implantation, but the continuous flow introduces high levels of non-physiological shear (34). All VAD types introduce two crucial elements that affect the immune system: high levels of shear stress and foreign materials with varying surface finishes.
Leukocytes are exposed repeatedly to the VAD at 1-min intervals (average blood flow at rest = 5 L/min) (35). Brief exposure to high shear sensitizes platelets to activation under subsequent low insult shear, so it is reasonable to presume leukocytes might be affected the same way (36). It has been suggested that shear stress activates leukocytes through structural changes rather than ligand-induced signal transduction (37). Interestingly, repeated mechanical deformation at 25 dynes/cm2 of primed neutrophils can return them to a resting state (38), but above this leads to structural disruption (39).
Leukocytes also contend with different biomaterials and varying surface finishes throughout the VAD. The surface properties of these biomaterials drive the foreign body response. This response begins with protein adsorption (i.e., albumin, fibrinogen, globulins) at the biomaterial surface creating a highly dynamic matrix which can make the surface inert, or encourage cellular activation and adhesion (40). Surface roughness is an important factor to consider as long-term continuous-flow VADs (CF-VADs) have highly polished, smooth surfaces near the rotating impeller (41) to reduce adhesion of proteins and cells (41). Some VADs, both continuous and axial flow, have sintered titanium microsphere surfaces to encourage cellular and protein adherence to form a stable, densely adherent biological lining (42). This biological lining is hypothesized to “hide” the foreign material and reduce the immune response.
The complex interactions between leukocytes and VADs, combined with the pre-existing inflammatory profile of the VAD recipient, can lead to a plethora of possible inflammatory outcomes (Figure 2). This could make their use as biomarkers difficult, but there are some inflammatory mediators that indicate the effectiveness of VAD therapy. The following section has been summarized in Table 1.
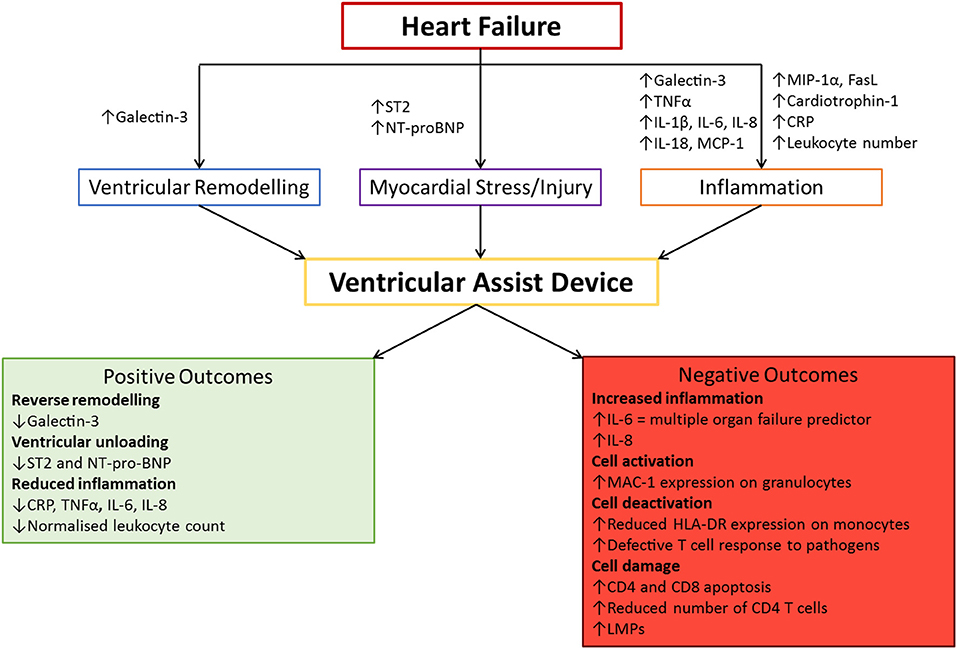
Figure 2. The association between VAD implantation and inflammation. Heart failure has its own distinct inflammatory profile. Ventricular assist device (VAD) implantation can have both positive and negative outcomes which impact the efficacy of using this treatment. These markers can be used to monitor the progression of VAD therapy and predict adverse events for early intervention purposes. CRP, C-reactive protein; IL, Interleukin; MCP-1, Monocyte Chemoattractant Protein 1; MIP-1α, Macrophage Inflammatory Protein 1 alpha; NT-proBNP, N-Terminal pro Brain Natriuretic Peptide; TNFα, Tumor Necrosis Factor alpha.
Clinical Studies of the Inflammatory Effects of VADs
Clinical studies that measure changes in circulating mediators have provided insight into how effective VAD therapy is. Biomarkers most often used include C-reactive protein (CRP), a common biomarker of systemic inflammation (43), N-terminal brain natriuretic peptide (NT-proBNP) used to establish prognosis in heart failure (44), suppressor of tumorigenicity 2 (ST2), a member of the IL-1 receptor family, that signposts cardiac remodeling and tissue fibrosis (45), and galectin-3, which is associated with higher risk of mortality (46). Inflammatory cytokines, mainly IL-6, TNFα, and IL-8, have also been studied in patients implanted with VADs and provide insight into cardiac function (21, 25).
Inflammatory and Cardiac Biomarkers
There is an initial elevation of inflammatory markers in response to VADs but this is largely due to the surgical procedure itself. Increased CRP levels are consistent with inflammation and a further increase is normal in response to the trauma of surgery (47). End-stage HF patients already have around 8-fold higher levels of circulating CRP than the typical healthy reference value of 0–5 mg/L in serum (48). VAD therapy improves the inflammatory profile of the patient with levels of CRP halved compared to pre-operative values within 60 days after implantation (33). A similar profile is seen in pediatric patients; pre-VAD CRP levels are abnormally high due to HF (~35 mg/L), are exacerbated by surgery, and begin to decrease 1 month post-implantation with levels returning to the healthy range after 5 months (49).
Circulating NT-proBNP levels decrease significantly in VAD patients between 24 h pre-implantation and >60 days post-implantation but still remain abnormally high (33). This decrease is indicative of normalizing blood volume and pressure which lessens the strain on the kidneys. However, the severity of kidney damage and its bidirectional relationship with HFpEF could be the reason NT-proBNP levels remain abnormal.
ST2 isconsidered to be a superior cardiac stress biomarker as levels are not affected by age, BMI, history of HF, anemia, or renal failure (45). Levels of ST2 decrease in patients implanted with continuous-flow VADs, but remain abnormally high >60 days post-implantation (33). This suggests cardiac improvement but not to a point where the patient is no longer at high risk of mortality. In contrast, those implanted with axial-flow VADs showed that elevated pre-operative ST2 levels were normalized 1 month post-VAD (50) suggestive of cardiac de-stress and repair. This would indicate that the axial-flow design is more favorable toward reverse remodeling.
Galectin-3 levels show a similar trend with higher levels pre-operatively in HF patients compared to healthy controls (51) and a decrease during VAD placement (33). However, galectin-3 levels become elevated again and can even exceed pre-operative levels after 6 months VAD therapy (30, 51). The reasons for this are unknown but it might be that the VAD is not supporting myocardial recovery and that galectin-3 levels identify patients at higher risk of death.
Cytokines
Circulating cytokines, especially TNFα, IL-6, and IL-8 that regulate systemic inflammation, are also of interest in HF and VADs. Elevation of these is linked to poor outcomes (52). TNFα is elevated in severe HF and can predict mortality (53). Decreases of TNFα within the myocardial tissue post-VAD implantation indicate a tissure repair response to VAD treatment although levels remain higher than in controls(54–56).
IL-6 is increased immediately after VAD implantation with a decline to below pre-operative levels after 6 weeks (57). However, patients with higher pre-operative IL-6 (such as those with HFpEF) are more susceptible to poor early outcomes (52). An increase in IL-6 post-implantation is associated with multi-organ failure (58), the main cause of death during early phase MCS (59). Blood IL-6 concentrations also differ by VAD type being higher with CF-VAD than with pulsatile (PF-VAD) flow devices (60). This is likely related to the exposure of leukocytes to non-physiological flow and high shear levels in CF-VADs leading to increased activation. As for TNFα, there isan overall reduction in IL-6 in the myocardium following VAD support (54).
IL-8 showssimilar patterns of change with an immediate increase post-implantation and a decrease to pre-operative levels by 4–6 weeks post-operatively (52, 57). There are also differences by VAD type; while pulsatile and continuous VADs both show a post-operative decline in IL-8 (61), levels had increased to greater than pre-operative levels by 9 months post-implantation with continuous flow devices (43). Again, this could be a feature of the shear stress effects on leukocyte activation.
Leukocytes
Changes in circulating leukocyte numbers and phenotype can also provide more detail about the inflammatory changes associated with VAD implantation. Pre-operatively, VAD patients have leukocyte counts at the higher end of normal range, consistent with their inflammatory profile. Overall, VAD implantation seems to be associated with a decline in peripheral blood leukocyte counts (62, 63). In patients implanted with either a PVAD, HeartMate II (HMII; Thoratec), or HVAD (HeartWare, MA, USA), these leukocyte counts initially increased significantly (post-operative day (POD) 14) then decreased significantly to below pre-op levels by POD 60 in all VAD types (62). This would suggest an improvement in the inflammatory profile.
Despite normalization of leukocyte counts, cell phenotype is changed ina VAD-dependent manner. Expression of the activation marker, macrophage antigen-1 (MAC-1), on granulocytes is a marker for systemic inflammation (64). MAC-1 was increased significantly in HMII patients at POD 14 and did not return to pre-operative levels until POD 120. For HVAD patients, an initial small increase in MAC-1 expression at POD 14 was seen with a return to pre-operative levels by POD 60. This has been linked to the higher rates of and susceptibility to infection in HMII patients compared to HVAD patients (62) likely due to design differences: HMII being an axial-flow pump and the HVAD being a centrifugal pump. Variable increase in MAC-1 expression might identify patients vulnerable to recurrent infection and inform intervention.
Additionally, there are wider immune system alterations caused by VADs. Patients implanted with pulsatile flow VADs have a selective reduction in CD4+ T cells, defective proliferative responses to stimuli such as staphylococcal enterotoxin B, and higher levels of apoptosis in CD4+ and CD8+ T cells in comparison to medically treated NYHA class IV patients (65–68). Disruption of adaptive immune cells contribute to risk of recurrent infection and sepsis. The effects of continuous-flow devices on lymphocytes are suspected to be less severe, although the data are limited. The one study we could identify showed increased apoptotic activity in CD4+ T cells up to 4 weeks post-operatively which normalized to baseline after 7 weeks (69).
Potential deactivation of monocytes through a reduced expression of HLA-DR has also been observed in VAD recipients and might be a marker of mortality. Patients in intensive care who died within the first 30 days of implantation exhibited a lower percentage of monocytes expressing HLA-DR than those who survived (70). This might be due to immunoparalysis which during the immediate support phase could hamper tissue repair for end-organ recovery (52).
Microparticles
Recent years have seen a burgeoning interest in the use of microparticles (MPs) as biomarkers of inflammatory diseases such as psoriasis, preeclampsia, pulmonary hypertension, and heart failure (71, 72). MPs are cell-derived vesicles between 0.1 and 1 μm in size that are formed from the outward blebbing of the plasma membrane due to cell stress (71). High levels of artificial mechanical stress produced by the VAD disrupts cells leading to damage, apoptosis, and stress-induced MP formation (72). MPs can be derived from platelets (PMPs), leukocytes (LMPs), and endothelial cells (EMPs) and have strong inflammatory and pro-thrombotic properties. Significantly elevated levels in VAD patients could be predictive of adverse events (73). Increased levels of LMPs have been demonstrated in VAD patients compared to historical controls and were elevated independently of leukocyte counts, suggesting that LMPs might be a marker of vascular inflammation (74). VAD type might not affect this parameter as both HMII and HVAD patients had increased LMPs (74, 75).
Summary
Overall, VADs can improve the inflammatory profile of HF patients through reducing high leukocyte counts and pro-inflammatory cytokine levels. VAD design, such as biomaterial choice and levels of shear stress, greatly affects whether inflammation and the immune system are affected positively or negatively.
Positive effects identified here includes improving pre-operative levels of inflammatory markers and cell phenotypes [e.g., quicker reduction in granulocyte activation in HVAD patients than HMII (62)]. Negative effects identified here includes monocyte deactivation, T cell apoptosis (69, 70), and higher inflammatory cytokine levels (43, 60). Future studies with newer devices of very different design to the HMII such as the HVAD and Heartmate III (HMIII) might reveal an improvement in how these VADs interact with the inflammatory response and the immune system.
Preclinical Studies for VAD Development
As discussed above, both heart failure and VAD implantation can affect the inflammatory profile of the patient. Despite this, the initial stage of VAD development focuses mainly on the effects on red blood cells through analysis of haemolysis (76). The need for total blood damage evaluation is not yet widely recognized and the effects of new VAD designs on leukocyte number and function and the inflammatory response is only slowly being included in preclinical evaluation. Studying the impact of VADs on the inflammatory response during preclinical testing would allow better understanding and improvement of the device.
However, there are no published studies of the inflammatory markers described above during in vitro VAD testing although, such approaches are being incorporated into preclinical studies in animals. In a study that involved implanting nine dogs with and six dogs without VADs, TNFα levels in blood and renal tissues did not differ after 6 h (77). Changes in leukocyte counts and activation profiles have been explored in animal preclinical studies. Cows implanted with the EVAHeart or HeartMate II had increased in monocyte tissue factor, monocyte-platelet and granulocyte-platelet aggregates in the first few days post-operatively. While levels decreased over the next 30 days they remained higher than pre-operative levels and are suggestive of increased risk of thrombosis (78). However, when the HVAD was implanted into sheep, leukocyte count remained within normal ranges (79). This shows that the impact of very different VAD designs on inflammation can be detected in vivo.
There are limitations to what can be measured during in vitro testing due to the models typically being simple blood only loops but the effects on leukocytes can be assessed. To this end, our own in vitro studies showed an increase in leukocyte-derived microparticles emerging in bovine and ovine blood circulated through the CentriMag™ (63, 80). This increase is important as the CentriMag is considered the ‘gold standard' for blood handling yet there is clearly a so far unappreciated shear-effect on leukocytes.
Summary
Heart failure is characterized by an inflammatory response, initiated by injury during myocardial infarction or the repetitive stress of hypertension on cardiac cells (15, 21, 22). Acute inflammation initially helps maintain ventricular function and support tissue repair but eventually this remodels the ventricle leading to altered function (16, 17). Chronic inflammation can lead to myocardium dysfunction due to fibrosis, hypertrophy, and cell death (15). Consequently, pro-inflammatory mediator expression can be used to map progression of HF, is proportional to functional class (21, 24–28), and can predict adverse outcomes (25). Interestingly, right ventricular failure (RVF) post-left VAD implantation is an emerging major complication. Post-operative RVF has been associated with higher pre-operative leukocyte counts and CRP levels suggesting that systemic inflammation may be contributory after LVAD implantation (81).
VADs have the potential to partially reverse the pathological processes involved in the progression of HF and restore myocardial function (33). The effectiveness of this can be monitored as a decline in biomarkers such as NT-proBNP, ST2 and the cytokines IL-6 and IL-8 although there are differences in the pattern of response for pulsatile- vs. continuous-flow devices (60, 61, 82). Irrespective of the type of device implanted, the inflammatory profile of patients pre-VAD can be linked to poor early outcomes (52).
Recent advances in VADs have led to the development of smaller pumps with better blood damage profiles compared to their predecessors. The differential effects of continuous-flow vs. pulsatile flow devices have been noted and there is a growing trend toward developing pumps with pulsatile capability, such as the HeartMate III (HMIII, Thoratec), to better maintain the natural rhythm of the body and reduce blood damage (83). VAD research is improving our knowledge of the effects of novel biomaterials and shear stress on blood. Yet, mostly for practical and ethical reasons, preclinical studies use healthy human or large animal blood (bovine/ovine/porcine) instead of blood from heart failure patients which has an inherently different inflammatory phenotype.
Evidence that VAD treatment improves these inflammatory parameters and elucidation of the associated mechanisms could benefit the design of future heart pumps for improved longevity and quality of life for patients. These changes in inflammatory markers alone could also be beneficial to clinicians in monitoring VAD therapy and early-intervention for adverse events such as sepsis.
Author Contributions
GR and IP: conception and design of the review, drafting of the manuscript, and critical revision of the manuscript. SA: drafting of the manuscript, critical revision of the manuscript. FB: critical revision of the manuscript. CT: conception and design of the review, drafting of the manuscript, critical revision of the manuscript.
Funding
GR was funded by the Swansea University Postgraduate Scholarship (50%) and Calon Cardio - Technology Ltd. (50%).
Conflict of Interest Statement
GR and SA are employees of Calon Cardio – Technology Ltd (Calon). IP is employed by Scandinavian Real Heart AB.
The remaining authors declare that the research was conducted in the absence of any commercial or financial relationships that could be construed as a potential conflict of interest.
Abbreviations
ACC, American College of Cardiology; CF, Continuous Flow; CRP, C-Reactive Protein; EMP, Endothelial-derived Microparticle; HF, Heart Failure; HFmrEF, Heart Failure with mid-range Ejection Fraction; HFpEF, Heart Failure with preserved Ejection Fraction; HFrEF, Heart Failure with reduced Ejection Fraction; HLA-DR, Human Leukocyte Antigen-D Related; HMII, HeartMate 2; HVAD, HeartWare Ventricular Assist Device; IL, Interleukin; INTERMACS, Interagency Registry for Mechanically Assisted Circulatory Support; LMP, Leukocyte-derived Microparticle; LVEF, Left Ventricular Ejection Fraction; MCP-1, Monocyte Chemotactic Protein 1; MCS, Mechanical Circulatory Support; MIP-1α, Macrophage Inflammatory Protein 1 alpha; MP, Microparticle; NT-proBNP, N-Terminal pro Brain Natriuretic Peptide; NYHA, New York Heart Association; PF, Pulsatile Flow; PMP, Platelet-derived Microparticle; POD, Post-Operative Day; PVAD, Pulsatile Ventricular Assist Device; TNFα, Tumor Necrosis Factor alpha; Treg, egulatory T cells; VAD, Ventricular Assist Device.
References
1. McMurray JJ, Adamopoulos S, Anker SD, Auricchio A, Bohm M, Dickstein K, et al. ESC guidelines for the diagnosis and treatment of acute and chronic heart failure 2012: The Task Force for the Diagnosis and Treatment of Acute and Chronic Heart Failure 2012 of the European Society of Cardiology. Developed in collaboration with the Heart Failure Association (HFA) of the ESC. Eur J Heart Fail. (2012) 14:803–69. doi: 10.1093/eurjhf/hfs105
2. Tendera M. Epidemiology, treatment, and guidelines for the treatment of heart failure in Europe. Eur Heart J Suppl. (2005) 7(suppl. J):J5–9. doi: 10.1093/eurheartj/sui056
3. Savarese G, Lund LH. Global Public Health Burden of Heart Failure. Cardiac Fail Rev. (2017) 3:7–11. doi: 10.15420/cfr.2016:25:2
4. Mazurek JA, Jessup M. Understanding Heart Failure. Heart Fail Clin. (2017) 13:1–19. doi: 10.1016/j.hfc.2016.07.001
5. Mozaffarian D, Benjamin EJ, Go AS, Arnett DK, Blaha MJ, Cushman M, et al. Heart Disease and Stroke Statistics-2016 Update: a report from the American Heart Association. Circulation. (2016) 133:e38–360. doi: 10.1161/CIR.0000000000000350
6. van Empel V, Brunner-La Rocca HP. Inflammation in HFpEF: Key or circumstantial? Int J Cardiol. (2015) 189:259–63. doi: 10.1016/j.ijcard.2015.04.110
7. Sharma K, Kass DA. Heart Failure With Preserved Ejection Fraction. Mech Clin Features Ther. (2014) 115:79–96. doi: 10.1161/CIRCRESAHA.115.302922
8. Ponikowski P. 2016 ESC Guidelines for the diagnosis and treatment of acute and chronic heart failure: the task force for the diagnosis and treatment of acute and chronic heart failure of the European Society of Cardiology (ESC). Developed with the special contribution of the Heart Failure Association (HFA) of the ESC. Eur J Heart Fail. (2016). 37:2129–200. doi: 10.1093/eurheartj/ehw128
9. Hunt SA, Baker DW, Chin MH, Cinquegrani MP, Feldmanmd AM, Francis GS, et al. ACC/AHA Guidelines for the Evaluation and Management of Chronic Heart Failure in the Adult: executive summary a report of the American College of Cardiology/American Heart Association Task Force on Practice Guidelines (Committee to Revise the 1995 Guidelines for the Evaluation and Management of Heart Failure). Circulation. (2001) 104:2996–3007. doi: 10.1161/hc4901.102568
10. Thunberg CA, Gaitan BD, Arabia FA, Cole DJ, Grigore AM. Ventricular assist devices today and tomorrow. J Cardiothorac Vasc Anesth. (2010) 24:656–80. doi: 10.1053/j.jvca.2009.11.011
11. Stevenson LW, Pagani FD, Young JB, Jessup M, Miller L, Kormos RL, et al. INTERMACS profiles of advanced heart failure: the current picture. J Heart Lung Transplant. (2009) 28:535–41. doi: 10.1016/j.healun.2009.02.015
12. Carmona M, Álvarez M, Marco J, Mahíllo B, Domínguez-Gil B, Núñez JR, et al. Global organ transplant activities in 2015. Data from the Global Observatory on Donation and Transplantation (GODT). Transplantation (2017) 101:S29. doi: 10.1097/01.tp.0000525015.43613.75
13. Daneshmand MA, Rajagopal K, Lima B, Khorram N, Blue LJ, Lodge AJ, et al. Left ventricular assist device destination therapy versus extended criteria cardiac transplant. Ann Thorac Surg. (2010) 89:1205–9. Discussion 10. doi: 10.1016/j.athoracsur.2009.12.058
14. Uriel N, Han J, Morrison KA, Nahumi N, Yuzefpolskaya M, Garan AR, et al. Device thrombosis in HeartMate II continuous-flow left ventricular assist devices: a multifactorial phenomenon. J Heart Lung Transplant. (2014) 33:51–9. doi: 10.1016/j.healun.2013.10.005
15. Briasoulis A, Androulakis E, Christophides T, Tousoulis D. The role of inflammation and cell death in the pathogenesis, progression and treatment of heart failure. Heart failure reviews. (2016) 21:169–76. doi: 10.1007/s10741-016-9533-z
16. Mill JG, Stefanon I, dos Santos L, Baldo MP. Remodeling in the ischemic heart: the stepwise progression for heart failure. Braz J Med Biol Res. (2011) 44:890–8. doi: 10.1590/S0100-879X2011007500096
17. Frangogiannis NG. The immune system and the remodeling infarcted heart: cell biological insights and therapeutic opportunities. J Cardiovasc Pharm. (2014) 63:185–95. doi: 10.1097/FJC.0000000000000003
18. ter Maaten Jozine M, Damman K, Verhaar Marianne C, Paulus Walter J, Duncker Dirk J, Cheng C, et al. Connecting heart failure with preserved ejection fraction and renal dysfunction: the role of endothelial dysfunction and inflammation. Eur J Heart Fail. (2016) 18:588–98. doi: 10.1002/ejhf.497
19. Cotton JM, Kearney MT, Shah AM. Nitric oxide and myocardial function in heart failure: friend or foe? Heart (2002) 88:564–6. doi: 10.1136/heart.88.6.564
20. Elahi MM, Naseem KM, Matata BM. Nitric oxide in blood. The nitrosative-oxidative disequilibrium hypothesis on the pathogenesis of cardiovascular disease. The FEBS J. (2007) 274:906–23. doi: 10.1111/j.1742-4658.2007.05660.x
21. Gullestad L, Ueland T, Vinge LE, Finsen A, Yndestad A, Aukrust P. Inflammatory cytokines in heart failure: mediators and markers. Cardiology (2012) 122:23–35. doi: 10.1159/000338166
22. Dinh W, Futh R, Lankisch M, Bansemir L, Nickl W, Scheffold T, et al. Cardiovascular autonomic neuropathy contributes to left ventricular diastolic dysfunction in subjects with Type 2 diabetes and impaired glucose tolerance undergoing coronary angiography. Diabetic Med. (2011) 28:311–8. doi: 10.1111/j.1464-5491.2010.03221.x
23. Yndestad A, Finsen AV, Ueland T, Husberg C, Dahl CP, Oie E, et al. The homeostatic chemokine CCL21 predicts mortality and may play a pathogenic role in heart failure. PLoS ONE (2012) 7:e33038. doi: 10.1371/journal.pone.0033038
24. Damas JK, Eiken HG, Oie E, Bjerkeli V, Yndestad A, Ueland T, et al. Myocardial expression of CC- and CXC-chemokines and their receptors in human end-stage heart failure. Cardiovasc Res. (2000) 47:778–87. doi: 10.1016/S0008-6363(00)00142-5
25. Deswal A, Petersen NJ, Feldman AM, Young JB, White BG, Mann DL. Cytokines and cytokine receptors in advanced heart failure: an analysis of the cytokine database from the Vesnarinone trial (VEST). Circulation (2001) 103:2055–9. doi: 10.1161/01.CIR.103.16.2055
26. Devaux B, Scholz D, Hirche A, Klovekorn WP, Schaper J. Upregulation of cell adhesion molecules and the presence of low grade inflammation in human chronic heart failure. Eur Heart J. (1997) 18:470–9. doi: 10.1093/oxfordjournals.eurheartj.a015268
27. Aukrust P, Ueland T, Lien E, Bendtzen K, Muller F, Andreassen AK, et al. Cytokine network in congestive heart failure secondary to ischemic or idiopathic dilated cardiomyopathy. Am J Cardiol. (1999) 83:376–82. doi: 10.1016/S0002-9149(98)00872-8
28. Torre-Amione G, Kapadia S, Benedict C, Oral H, Young JB, Mann DL. Proinflammatory cytokine levels in patients with depressed left ventricular ejection fraction: a report from the studies of left ventricular dysfunction (SOLVD). J Am Coll Cardiol. (1996) 27:1201–6. doi: 10.1016/0735-1097(95)00589-7
29. de Boer RA, Voors AA, Muntendam P, van Gilst WH, van Veldhuisen DJ. Galectin-3: a novel mediator of heart failure development and progression. Eur J Heart Fail. (2009) 11:811–7. doi: 10.1093/eurjhf/hfp097
30. Coromilas E, Que-Xu EC, Moore D, Kato TS, Wu C, Ji R, et al. Dynamics and prognostic role of galectin-3 in patients with advanced heart failure, during left ventricular assist device support and following heart transplantation. BMC Cardiovasc Disord. (2016) 16:138. doi: 10.1186/s12872-016-0298-z
31. Casserly BP, Sears EH, Gartman EJ. The role of natriuretic peptides in inflammation and immunity. Recent Pat Inflamm Allergy Drug Discov. (2010) 4:90–104. doi: 10.2174/187221310791163125
32. Pan Y, Li D, Ma J, Shan L, Wei M. NT-proBNP test with improved accuracy for the diagnosis of chronic heart failure. Medicine (2017) 96:e9181. doi: 10.1097/MD.0000000000009181
33. Ahmad T, Wang T, O'Brien EC, Samsky MD, Pura JA, Lokhnygina Y, et al. Effects of left ventricular assist device support on biomarkers of cardiovascular stress, fibrosis, fluid homeostasis, inflammation, and renal injury. JACC Heart fail. (2015) 3:30–9. doi: 10.1016/j.jchf.2014.06.013
34. Alba AC, Delgado DH. The future is here: ventricular assist devices for the failing heart. Expert Rev Cardiovasc Ther. (2009) 7:1067–77. doi: 10.1586/erc.09.86
35. Young DB. Introduction: Control of Cardiac Output. San Rafael, CA: Morgan & Claypool Life Sciences (2010).
36. Sheriff J, Bluestein D, Girdhar G, Jesty J. High-Shear Stress Sensitizes Platelets to Subsequent Low-Shear Conditions. Anna Biomed Eng. (2010) 38:1442–50. doi: 10.1007/s10439-010-9936-2
37. Niethammer P. Neutrophil mechanotransduction: a GEF to sense fluid shear stress. J Cell Biol. (2016) 215:13. doi: 10.1083/jcb.201609101
38. Ekpenyong AE, Toepfner N, Fiddler C, Herbig M, Li W, Cojoc G, et al. Mechanical deformation induces depolarization of neutrophils. Sci Adv. (2017) 3:e1602536. doi: 10.1126/sciadv.1602536
39. Komai Y, Schmid-Schonbein GW. De-activation of neutrophils in suspension by fluid shear stress: a requirement for erythrocytes. Ann Biomed Eng. (2005) 33:1375–86. doi: 10.1007/s10439-005-6768-6
40. Radley G, Pieper IL, Thornton CA. The effect of ventricular assist device-associated biomaterials on human blood leukocytes. J Biomed Mater Res Part B Appl Biomater. (2018) 106:1730–8. doi: 10.1002/jbm.b.33981
41. Linneweber J, Dohmen PM, Kertzscher U, Affeld K, Nose Y, Konertz W. The effect of surface roughness on activation of the coagulation system and platelet adhesion in rotary blood pumps. Artif Organs (2007) 31:345–51. doi: 10.1111/j.1525-1594.2007.00391.x
42. John R, Kamdar F, Liao K, Colvin–Adams M, Miller L, Joyce L, et al. Low thromboembolic risk for patients with the Heartmate II left ventricular assist device. J Thoracic Cardiovasc Surgery (2008) 136:1318–23. doi: 10.1016/j.jtcvs.2007.12.077
43. Grosman-Rimon L, Jacobs I, Tumiati LC, McDonald MA, Bar-Ziv SP, Fuks A, et al. Longitudinal assessment of inflammation in recipients of continuous-flow left ventricular assist devices. Can J Cardiol. (2015) 31:348–56. doi: 10.1016/j.cjca.2014.12.006
44. Bhalla V, Maisel AS. B-type natriuretic peptide. A biomarker for all the right reasons. Ital Heart J. (2004) 5:417–20.
45. Shah RV, Januzzi JL Jr. ST2: a novel remodeling biomarker in acute and chronic heart failure. Curr Heart Fail Rep. (2010) 7:9–14. doi: 10.1007/s11897-010-0005-9
46. Hartupee J, Mann DL. Positioning of inflammatory biomarkers in the heart failure landscape. J Cardiovasc Transl Res. (2013) 6:485–92. doi: 10.1007/s12265-013-9467-y
47. Santonocito C, De Loecker I, Donadello K, Moussa MD, Markowicz S, Gullo A, et al. C-reactive protein kinetics after major surgery. Anesth Analg. (2014) 119:624–9. doi: 10.1213/ANE.0000000000000263
48. Syeda T, Hashim AS, Rizvi HA, Hadi SM. Pre- and post-operative values of serum CRP in patients undergoing surgery for brain tumour. JPMA (2014) 64:271–4.
49. Yu X, Larsen B, Rutledge J, West L, Ross DB, Rebeyka IM, et al. The profile of the systemic inflammatory response in children undergoing ventricular assist device support. Interact CardioVasc Thorac Surg. (2012) 15:426–31. doi: 10.1093/icvts/ivs206
50. Caselli C, D'Amico A, Ragusa R, Caruso R, Prescimone T, Cabiati M, et al. IL-33/ST2 pathway and classical cytokines in end-stage heart failure patients submitted to left ventricular assist device support: a paradoxic role for inflammatory mediators? Mediat Inflamm. (2013) 2013:498703. doi: 10.1155/2013/498703
51. Lok SI, Nous FM, van Kuik J, van der Weide P, Winkens B, Kemperman H, et al. Myocardial fibrosis and pro-fibrotic markers in end-stage heart failure patients during continuous-flow left ventricular assist device support. Eur J Cardiothorac Surg. (2015) 48:407–15. doi: 10.1093/ejcts/ezu539
52. Caruso R, Botta L, Verde A, Milazzo F, Vecchi I, Trivella MG, et al. Relationship between pre-implant interleukin-6 levels, inflammatory response, and early outcome in patients supported by left ventricular assist device: a prospective study. PLoS ONE (2014) 9:e90802. doi: 10.1371/journal.pone.0090802
53. Rauchhaus M, Doehner W, Francis DP, Davos C, Kemp M, Liebenthal C, et al. Plasma cytokine parameters and mortality in patients with chronic heart failure. Circulation (2000) 102:3060–7. doi: 10.1161/01.CIR.102.25.3060
54. Bedi MS, Alvarez RJ Jr., Kubota T, Sheppard R, Kormos RL, Siegenthaler MP, et al. Myocardial Fas and cytokine expression in end-stage heart failure: impact of LVAD support. Clin Transl Sci. (2008) 1:245–8. doi: 10.1111/j.1752-8062.2008.00056.x
55. Torre-Amione G, Stetson SS, Farmer JA. Clinical implications of tumour necrosis factor α antagonism in patients with congestive heart failure. Ann Rheum Dis. (1999) 58:103–6. doi: 10.1136/ard.58.2008.i103
56. Maybaum S, Epstein S, Beniaminovitz A, Di Tullio M, Oz M, Bergmann SR, et al. Partial loading of the left ventricle during mechanical assist device support is associated with improved myocardial function, blood flow and metabolism and increased exercise capacity. J Heart Lung Transplant. (2002) 21:446–54. doi: 10.1016/S1053-2498(01)00392-8
57. Corry DC, DeLucia AI, Zhu H, Radcliffe RR, Brevetti GR, El-Khatib H, et al. Time course of cytokine release and complement activation after implantation of the heartmate left ventricular assist device. Asaio J. (1998) 44:M347–51. doi: 10.1097/00002480-199809000-00005
58. Masai T, Sawa Y, Ohtake S, Nishida T, Nishimura M, Fukushima N, et al. Hepatic dysfunction after left ventricular mechanical assist in patients with end-stage heart failure: role of inflammatory response and hepatic microcirculation. Ann Thorac Surg. (2002) 73:549–55. doi: 10.1016/S0003-4975(01)03510-X
59. Deng MC, Edwards LB, Hertz MI, Rowe AW, Keck BM, Kormos R, et al. Mechanical circulatory support device database of the International Society for Heart and Lung Transplantation: third annual report−2005. J Heart Lung Transplant. (2005) 24:1182-7. doi: 10.1016/j.healun.2005.07.002
60. Loebe M, Koster A, Sanger S, Potapov EV, Kuppe H, Noon GP, et al. Inflammatory response after implantation of a left ventricular assist device: comparison between the axial flow MicroMed DeBakey VAD and the pulsatile Novacor device. ASAIO J. (2001) 47:272–4. doi: 10.1097/00002480-200105000-00023
61. Goldstein DJ, Moazami N, Seldomridge JA, Laio H, Ashton RC Jr., Naka Y, et al. Circulatory resuscitation with left ventricular assist device support reduces interleukins 6 and 8 levels. Ann Thor Surg. (1997) 63:971–4. doi: 10.1016/S0003-4975(96)01117-4
62. Woolley JR, Teuteberg JJ, Bermudez CA, Bhama JK, Lockard KL, Kormos RL, et al. Temporal leukocyte numbers and granulocyte activation in pulsatile and rotary ventricular assist device patients. Artif Organs (2013) 38:447–55. doi: 10.1111/aor.12200
63. Pieper IL, Radley G, Christen A, Ali S, Bodger O, Thornton CA. Ovine leukocyte microparticles generated by the centrimag ventricular assist device in vitro. Artif Organs (2018) 42:E78–89. doi: 10.1111/aor.13068
64. Asimakopoulos G, Taylor KM. Effects of cardiopulmonary bypass on leukocyte and endothelial adhesion molecules. Ann Thorac Surg. (1998) 66:2135–44. doi: 10.1016/S0003-4975(98)00727-9
65. Ankersmit HJ, Edwards NM, Schuster M, John R, Kocher A, Rose EA, et al. Quantitative changes in T-cell populations after left ventricular assist device implantation: relationship to T-cell apoptosis and soluble CD95. Circulation (1999) 100(19 Suppl.):II211–5. doi: 10.1161/01.CIR.100.suppl_2.II-211
66. Ankersmit HJ, Tugulea S, Spanier T, Weinberg AD, Artrip JH, Burke EM, et al. Activation-induced T-cell death and immune dysfunction after implantation of left-ventricular assist device. Lancet (1999) 354:550–5. doi: 10.1016/S0140-6736(98)10359-8
67. Kimball PM, Flattery M, McDougan F, Kasirajan V. Cellular immunity impaired among patients on left ventricular assist device for 6 months. Ann Thorac Surg. (2008) 85:1656–61. doi: 10.1016/j.athoracsur.2008.01.050
68. Kimball P, Flattery M, Kasirajan V. T-cell response to staphylococcal enterotoxin B is reduced among heart failure patients on ventricular device support. Transplant Proc. (2006) 38:3695–6. doi: 10.1016/j.transproceed.2006.10.153
69. Ankersmit HJ, Wieselthaler G, Moser B, Gerlitz S, Roth G, Boltz-Nitulescu G, et al. Transitory immunologic response after implantation of the DeBakey VAD continuous-axial-flow pump. J Thorac Cardiovasc Surg. (2002) 123:557–61. doi: 10.1067/mtc.2002.120011
70. Kirsch M, Boval B, Damy T, Ghendouz S, Vermes E, Loisance D, et al. Importance of monocyte deactivation in determining early outcome after ventricular assist device implantation. Int J Art Organs. (2012) 35:169–76. doi: 10.5301/ijao.5000053
71. Burger D, Schock S, Thompson CS, Montezano AC, Hakim AM, Touyz RM. Microparticles: biomarkers and beyond. Clin Sci. (2013) 124:423–41. doi: 10.1042/CS20120309
72. Ivak P, Pitha J, Netuka I. Circulating microparticles as a predictor of vascular properties in patients on mechanical circulatory support; hype or hope? Physiol Res. (2016) 65: 727–35.
73. Nascimbene A, Hernandez R, George JK, Parker A, Bergeron AL, Pradhan S, et al. Association between cell-derived microparticles and adverse events in patients with nonpulsatile left ventricular assist devices. J Heart Lung Transplant. (2014) 33:470–7. doi: 10.1016/j.healun.2014.01.004
74. Diehl P, Aleker M, Helbing T, Sossong V, Beyersdorf F, Olschewski M, et al. Enhanced microparticles in ventricular assist device patients predict platelet, leukocyte and endothelial cell activation. Interact CardioVasc Thorac Surg. (2010) 11:133–7. doi: 10.1510/icvts.2010.232603
75. Sansone R, Stanske B, Keymel S, Schuler D, Horn P, Saeed D, et al. Macrovascular and microvascular function after implantation of left ventricular assist devices in end-stage heart failure: role of microparticles. J Heart Lung Transplant. (2015) 34:921–32. doi: 10.1016/j.healun.2015.03.004
76. ASTM. F1841-97: Standard Practice for Assessment of Hemolysis in Continous Flow Blood Pumps, West Conshohocken, PA (2013).
77. Yamagishi T, Oshima K, Hasegawa Y, Mohara J, Kanda T, Ishikawa S, et al. Cytokine induction by LVAD in the canine kidney. J Cardiovasc Surg. (2001) 42:759–68. doi: 10.11501/3171939
78. Snyder TA, Litwak KN, Tsukui H, Akimoto T, Kihara S, Yamazaki K, et al. Leukocyte-platelet aggregates and monocyte tissue factor expression in bovines implanted with ventricular assist devices. Artif Organs (2007) 31:126–31. doi: 10.1111/j.1525-1594.2007.00351.x
79. Tuzun E, Roberts K, Cohn WE, Sargin M, Gemmato CJ, Radovancevic B, et al. In vivo evaluation of investigation the HeartWare centrifugal ventricular assist device. Tex Heart Inst J. (2007) 34:406–11.
80. Chan CH, Pieper IL, Hambly R, Radley G, Jones A, Friedmann Y, et al. The CentriMag centrifugal blood pump as a benchmark for in vitro testing of hemocompatibility in implantable ventricular assist devices. Artif Organs (2015) 39:93–101. doi: 10.1111/aor.12351
81. Tang PC, Haft JW, Romano MA, Assi A, Hasan R, Palardy M, et al. Right ventricular failure after LVAD implantation occurs in a pro-inflammatory environment. J Heart Lung Transplant. (2018) 37:S276. doi: 10.1016/j.healun.2018.01.691
82. Ahmad F, Tufa DM, Mishra N, Jacobs R, Schmidt RE. Terminal differentiation of CD56(dim)CD16(+) natural killer cells is associated with increase in natural killer cell frequencies after antiretroviral treatment in HIV-1 infection. AIDS Res Hum Retroviruses (2015) 31:1206–12. doi: 10.1089/aid.2015.0115
Keywords: heart failure, ventricular assist devices, inflammation, cytokines, leukocytes
Citation: Radley G, Pieper IL, Ali S, Bhatti F and Thornton CA (2018) The Inflammatory Response to Ventricular Assist Devices. Front. Immunol. 9:2651. doi: 10.3389/fimmu.2018.02651
Received: 20 June 2018; Accepted: 26 October 2018;
Published: 15 November 2018.
Edited by:
Lisa Mullen, University of Sussex, United KingdomReviewed by:
Eisuke Amiya, The University of Tokyo Hospital, JapanTimothy M. Maul, Nemours Children's Hospital, United States
Copyright © 2018 Radley, Pieper, Ali, Bhatti and Thornton. This is an open-access article distributed under the terms of the Creative Commons Attribution License (CC BY). The use, distribution or reproduction in other forums is permitted, provided the original author(s) and the copyright owner(s) are credited and that the original publication in this journal is cited, in accordance with accepted academic practice. No use, distribution or reproduction is permitted which does not comply with these terms.
*Correspondence: Catherine A. Thornton, Yy5hLnRob3JudG9uQHN3YW5zZWEuYWMudWs=