- Department of Microbiology and Molecular Genetics, University of Pittsburgh School of Medicine, Pittsburgh, PA, United States
A majority of the morbidity and mortality associated with the genetic disease Cystic Fibrosis (CF) is due to lung disease resulting from chronic respiratory infections. The CF airways become chronically colonized with bacteria in childhood, and over time commensal lung microbes are displaced by bacterial pathogens, leading to a decrease in microbial diversity that correlates with declining patient health. Infection with the pathogen Pseudomonas aeruginosa is a major predictor of morbidity and mortality in CF, with CF individuals often becoming chronically colonized with P. aeruginosa in early adulthood and thereafter having an increased risk of hospitalization. Progression of CF respiratory disease is also influenced by infection with respiratory viruses. Children and adults with CF experience frequent respiratory viral infections with respiratory syncytial virus (RSV), rhinovirus, influenza, parainfluenza, and adenovirus, with RSV and influenza infection linked to the greatest decreases in lung function. Along with directly causing severe respiratory symptoms in CF populations, the impact of respiratory virus infections may be more far-reaching, indirectly promoting bacterial persistence and pathogenesis in the CF respiratory tract. Acquisition of P. aeruginosa in CF patients correlates with seasonal respiratory virus infections, and CF patients colonized with P. aeruginosa experience increased severe exacerbations and declines in lung function during respiratory viral co-infection. In light of such observations, efforts to better understand the impact of viral-bacterial co-infections in the CF airways have been a focus of clinical and basic research in recent years. This review summarizes what has been learned about the interactions between viruses and bacteria in the CF upper and lower respiratory tract and how co-infections impact the health of individuals with CF.
Factors Promoting Respiratory Infections in Cystic Fibrosis
Cystic fibrosis (CF) is a lethal genetic disease caused by mutations in the Cystic Fibrosis Transmembrane Conductance Regulator (CFTR) gene (1) that result in dysfunction of the CFTR anion channel (2). To date, close to 2,000 individual mutations in the CFTR gene have been identified (3), and these mutations are further sub-divided into five classes based on how they lead to defective production of CFTR protein, resulting in deficiencies in protein folding, intracellular trafficking, and/or gating reviewed in-depth by Rowntree and Harris (4). While CFTR mutations affect most cell types and all mucosal surfaces in the body, manifesting in different types of disease, respiratory disease remains the most heavily-studied pathology of CF. Chronic respiratory infections and the resulting robust but ineffective inflammatory response, culminating in respiratory failure, are the primary causes of death in CF patients.
In the CF respiratory tract, numerous factors resulting from dysfunctional CFTR combine to create an environment that promotes chronic bacterial and recurring viral infections. A dysfunctional CFTR alters the osmolarity of the airway surface liquid (ASL) layer, resulting in dehydrated ASL, and facilitating the buildup of a thick mucus layer. Diminished ASL hydration and thick mucus at the airway epithelial surface leads to failure of mucociliary clearance in the CF respiratory tract due to collapse of airway cilia, thereby preventing ciliary beat that normally clears debris, and infectious agents from the lungs. This allows microorganisms to repeatedly infect, eliciting robust inflammatory responses dominated by elevated proinflammatory cytokines, and continued accumulation of neutrophils in the CF airway (5). However, these inflammatory responses are ineffective at clearing pathogens in the CF lung, instead creating a hyperinflammatory cycle that leads to host tissue damage, respiratory failure, lung transplant or eventually, death (6). Additionally, the dysregulated conductance of bicarbonate anions by the CFTR channel in CF results in improper mucus formation and an altered ASL pH, which impacts the function of secreted antimicrobial peptides, disrupting a first line of defense against invading bacterial pathogens (7–9). Together, these deficiencies in CF respiratory tract physiology prevent efficient clearance of pathogens from the airways, allowing for the establishment of a robust community of microbes.
The CF Respiratory Microbiome
Identification of Bacterial Species in the CF Microbiome
The microbial community in the CF lung is complex, and lung health is affected by the presence and interactions of bacteria, fungi, and respiratory viruses (10, 11). Identification of bacterial species in the CF airways has traditionally relied on culture of bacteria by clinical microbiology laboratories from expectorated sputum samples, respiratory swabs, or samples obtained through bronchoscopy (referred to as culture-dependent methods) (12). Advances in next-generation sequencing have made it possible to identify populations of bacteria residing in the airways without culturing through the isolation of genomic DNA from CF patient samples and sequencing of the gene encoding the bacterial 16S ribosomal subunit. The 16S ribosomal subunit contains variable regions whose sequences can be assigned to bacteria at the species level (termed culture-independent methods) (13). Because culture-dependent methods require knowledge of which bacterial species to target for identification and how to isolate them, known bacterial pathogens were the main species identified from CF respiratory samples prior to culture-independent methods. With the advent of culture-independent methods, it became appreciated that in addition to traditional pathogens, many other bacterial species often associated with the oral cavity or upper respiratory tract, and considered commensal or colonizing organisms were present in the CF lung at high abundance (14). These newly recognized populations included many species of anaerobic bacteria, which previously were not identified, as clinical laboratories did not use culture methods that would allow anaerobic growth (15). It remains a debated issue in the field as to whether all bacterial species identified via culture-independent methods are truly established in the CF lower airways, or if presence of these species is due to contamination of samples by oral or upper respiratory tract microbes during the collection process (11, 16).
Commensal Microbiome Members
Focusing on bacterial members of the CF microbiome, from culture-independent studies we find that before chronic infections are established by pathogens, the CF lung is colonized by several genera of commensal bacteria. Studies have identified core bacterial airway microbiome members as belonging to the genera Streptococcus, Prevotella, Veillonella, Rothia, Granulicatella, Gemella, and Fusobacterium (14, 17, 18). Many factors can impact individual patients' respiratory microbiomes, most notably age and antibiotic exposures. Studies of longitudinal samples collected from patients over time have found distinct bacterial community profiles exist for younger vs. older CF patients, with pediatric CF patients possessing a greater abundance of core/commensal bacterial species, and a more diverse bacterial microbiome than CF adults (19). It is thought that these core species are displaced over time as patients age and pathogens are introduced and become established in the airways (10, 18). Multiple studies have found that a higher diversity of species in the lung correlates with better patient outcomes, and decreased diversity correlates with an over-abundance of CF pathogens and declines in patient health (10, 17, 18). Like microbiomes associated with other organ systems in healthy adults, the microbiome in the CF airways has ultimately been found to change minimally upon exposure to antibiotics, exhibiting an altered community structure or decrease in overall abundance during treatment but rebounding to the original community structure after treatment ends (20, 21). Roles for interspecies interactions occurring between commensal and pathogenic bacteria in the CF airways are just beginning to be elucidated. Species of commensal streptococci have been found to have direct protective effects toward the lung by inhibiting pathogens such as P. aeruginosa (22–24). We have only recently begun to recognize the complexity of the microbial ecology in the CF airways and how dynamics of microbial communities, and not solely presence of pathogens, can contribute to disease outcomes (25). It is likely that many as yet unknown interspecies interactions exist that impact bacterial populations in the CF airways at a given time, and future studies will pinpoint specific mechanisms mediating bacterial crosstalk that may be targeted to alter the abundance of distinct species.
Bacterial Respiratory Pathogens in CF
A number of bacterial species have been identified as major respiratory pathogens in CF, including Staphylococcus aureus, Haemophilus influenzae, P. aeruginosa, and Burkholderia complex (3, 26). S. aureus is the most frequently isolated bacterial pathogen in CF pediatric populations, whereas P. aeruginosa becomes established as CF patients age (3). S. aureus is regarded as a commensal species of the nares and upper respiratory tract but is recognized as a pathogen when identified in other body sites, such as the lower airways, and is cultured from over 70% of CF patients (3). P. aeruginosa is the dominant pathogen in end-stage CF lung disease, and chronic infection with P. aeruginosa is correlated with more severe reductions in pulmonary function measures (27) and mortality in CF patients. Methicillin-resistant (MRSA) and methicillin-sensitive (MSSA) S. aureus and P. aeruginosa that display enhanced antibiotic resistance during chronic infection pose significant challenges to treatment efforts (28). Non-traditional bacterial pathogens, including Achromobacter xylosoxidans, Stenotrophomonas maltophilia, and non-tuberculosis Mycobacterium (NTM), also contribute to respiratory infections in CF patients and have been associated with worsening lung function (26, 29). A mechanism common to most CF bacterial pathogens for evasion of host immune defenses in the CF lung, despite its hyperinflammatory state, is growth in bacterial aggregates or biofilms. During the transition from acute to chronic infection, P. aeruginosa, S. aureus, and other bacterial pathogens exhibit altered metabolism, decreased growth rate and up-regulated expression of antibiotic resistance genes, and these changes, together with increased production of polymeric matrix materials, protect organisms within biofilms from the hostile environment in the CF lung (30–32).
Respiratory Viruses in CF
Acute Respiratory Viral Infections in CF
Pediatric and adult CF patients experience frequent acute respiratory virus infections. Specific respiratory viruses responsible for infections are identified when patients present with symptoms indicative of a viral infection, leading physicians to take a viral swab from which genetic material is extracted for PCR, and a viral panel is performed consisting of primer sets specific to common viral culprits. The true incidence of viral infections is likely under-reported for several reasons, including infrequent use of viral swabs and incomplete PCR panels to detect viral infections, as well as the fact that not all patients present with symptoms during a viral infection (33, 34). The most commonly identified viral pathogens in CF populations are respiratory syncytial virus (RSV), human rhinovirus (RV), Influenza types A and B, and parainfluenza, all belonging to families of RNA viruses (34–36). It has been reported that close to 40% of children with CF are hospitalized at some point for severe respiratory infections, and of these hospitalizations, respiratory viruses were identified in 50% of patients, with RSV predominating (33). While in non-CF populations RSV is thought to be almost exclusively a pediatric pathogen, RSV infections are frequent in both adult and pediatric CF patients, and can result in severe symptoms. RSV infection may result in upper respiratory disease, including rhinitis, cough, fever, and acute otitis media, or progress to the lower respiratory tract, resulting in bronchiolitis or pneumonia in children, and exacerbate existing chronic airway disease in adults (37). RSV infection is especially aggressive in young infants with CF, leading to significant respiratory morbidity (38).
Links Between Viral Infections and Exacerbations
CF patients frequently experience periods of rapidly worsening respiratory symptoms, termed pulmonary exacerbations (39). Pulmonary exacerbations are typically defined by a decrease in lung function or increases in patient symptoms, however symptoms and severity of exacerbations vary from patient to patient and can be triggered by a multitude of causes (40). Exacerbations are often treated by initiating courses of additional antibiotics, increasing airway clearance therapies, or hospitalization in severe cases (41). Many clinical studies have now linked viral infections with pulmonary exacerbations (33, 35, 36, 42). Respiratory viral infections account for at least 40% of pulmonary exacerbations of CF adults (38, 43) and are linked to pulmonary function decline, antibiotic use, prolonged hospitalizations, and increased respiratory symptoms in CF patients (44–46). Respiratory viruses most frequently cultured during periods of exacerbation include the major viral pathogens appreciated in CF: influenza A and B, RSV, and RV (47–50).
Severity of Viral Infections in CF
CF patients are known to be pre-disposed to chronic bacterial infections, and several groups have examined whether CF disease also leads to more severe respiratory viral infections. In vitro studies evaluating CF vs. non-CF primary human bronchial epithelial cells in culture found that RV replication was increased in CF cells (51). Enhancement of viral infection could be attributed to a diminished innate antiviral response in CF cells, which showed weaker induction of interferon and expression of some interferon-stimulated genes, as compared to non-CF controls (52). A clinical study evaluating severity of RV infections in CF children compared to non-CF pediatric patients with asthma, non-CF bronchiectasis or healthy controls found CF patients had a higher prevalence of RV, and higher viral load in bronchoalveolar lavage (BAL), both when patients were stable and at even higher levels during pulmonary exacerbations (53). Higher RV load correlated with worse lung function scores in CF children, and RV infection in CF resulted in lower levels of inflammatory markers than in non-CF children, again indicating a dysregulated innate immune response in CF patients could be responsible for increased severity of viral infections (53). A longitudinal study reported that RV was identified more frequently in CF children than non-CF subjects, and RV infections in CF children persisted longer (54). These studies suggest inherent properties of CF airway cells may make the CF airway epithelium more prone to viral infection, and together with what is known regarding links between airway physiology and bacterial respiratory infection in CF, these factors could have important implications in cases of viral-bacterial co-infections.
Viral-Bacterial Co-Infections in CF
CF patients are commonly chronically infected with bacterial pathogens and maintain a high abundance of microbes in the respiratory tract, including pathogens, and commensal organisms. These same patient populations also experience frequent acute respiratory viral infections. There are numerous ways in which infection with a viral pathogen can alter the host response, impacting previously existing chronic bacterial infections and microbial communities, potentiating secondary bacterial infections, and/or permitting the acquisition of new bacterial species in the airways (summarized in Figure 1). In this section, we evaluate insights from clinical studies of CF patient populations and mechanistic in vitro studies that inform us of viral-bacterial interactions occurring in CF during co-infections.
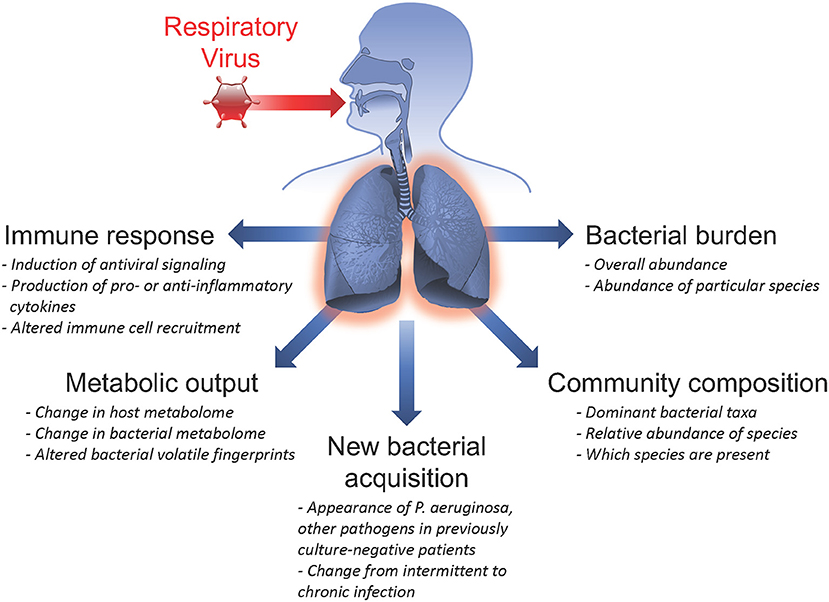
Figure 1. Summary of respiratory virus infection outcomes impacting viral-bacterial interactions in Cystic Fibrosis. Acute respiratory viral infections are known to affect subsequent infection with bacterial pathogens and influence pre-existing chronic bacterial infections in individuals with Cystic Fibrosis. Some ways in which respiratory viruses have been shown to impact bacterial infections include: inducing the host immune response; altering metabolic output of both host and infecting bacteria; causing new bacterial acquisition in patients who were previously culture-negative; altering bacterial community composition by shifting relative abundance of specific bacterial species; and inducing fluctuations in total bacterial burden.
Impact of Virus Infection on the CF Microbiome
Temporal changes in microbiome composition could result from a variety of disturbances that alter the environment in the CF airways, including initiation of antimicrobial therapies, mechanical or airway clearance treatments, or an altered host response. Respiratory viral infections can promote the onset of respiratory symptoms, as well as trigger the innate antiviral response in the CF airway epithelium, resulting in induction of antiviral signaling, and inflammation. From non-CF studies, respiratory viral infection is known to skew the immune status of the respiratory tract to be predisposed to secondary bacterial infection, which has been most studied for influenza (55). The altered host immune status following viral infection reduces antibacterial effector functions, like phagocyte recruitment (56), antimicrobial peptide production (57, 58), and protective adaptive immune responses (59–61), increasing susceptibility to bacterial infections. Specifically, interferon-mediated antiviral responses following influenza infection in mice (62) and vaccination with live attenuated influenza in humans (63) have been shown to shift the composition of the upper respiratory microbiome and increase the potential for emergence of S. aureus infections. An altered immune status resulting from respiratory viral infection likely also alters the microbial composition of the CF airways, potentially leading to shifts in bacterial populations comprising the microbiome and promoting infection by specific bacterial pathogens.
How respiratory viral infections impact the CF airway microbiome can be evaluated by observing changes in overall bacterial burden (or bacterial load), community composition, or dominant taxa. In non-viral-associated pulmonary exacerbations, the overall bacterial burden in the CF airways rarely changes in the time leading up to an exacerbation or during exacerbations (64–66). Studies evaluating bacterial burden during acute respiratory viral-associated pulmonary exacerbations have produced conflicting results. In one prospective study of CF adults, P. aeruginosa density in sputum was not found to increase during exacerbation, compared to patients' stable states in either the presence or absence of a respiratory virus co-infection (67). A similar study design by another group found the opposite to be true: in adult CF patients evaluated, a significantly higher load of P. aeruginosa was observed during respiratory-virus associated exacerbations (48). An observational study of CF children found P. aeruginosa density was not significantly different between patients experiencing viral- or non-viral-associated exacerbations (47). Additional prospective studies with larger patient cohorts are needed to gain a more definitive understanding of the effects of virus co-infection on overall bacterial burden and burden of specific organisms, like P. aeruginosa, in pediatric and adult CF populations.
While the relationship between respiratory virus co-infection and bacterial burden remains unclear, more efforts have been made toward investigating associations between viral infection and culture of specific bacterial pathogens from the CF airways. Findings from multiple studies indicate approximately 15–25% of CF patients undergoing a respiratory viral infection also culture positive for a known CF bacterial pathogen (68–70). Previously uncolonized CF patients have been reported to undergo new acquisition of P. aeruginosa following seasonal respiratory virus infections (44). Regarding roles for specific respiratory viruses, both RSV and RV are linked in clinical studies to development of P. aeruginosa co-infections and conversion from intermittent to chronic P. aeruginosa colonization in CF patients (33, 35, 43, 44, 71). Similarly, other known bacterial respiratory pathogens, including H. influenzae, Moraxella catarrhalis, and Streptococcus pneumoniae, were cultured more frequently from CF patients experiencing a RV co-infection (68). A separate pediatric study also found that in a cohort of CF children experiencing viral-bacterial co-infections, RV and S. aureus were co-cultured more frequently than any other viral-bacterial pair (69). Together, these studies present strong clinical evidence for association of respiratory viral co-infection with presence of bacterial pathogens in the airways and suggest associations between specific species of viruses and bacteria co-cultured that may vary with age.
Toward Mechanisms Underlying Viral-Bacterial Interactions
Mechanistic studies evaluating the outcomes of viral-bacterial co-infections have been made possible through the availability of isogenic immortalized CF airway epithelial cell lines (72) and access to well-differentiated primary airway epithelial cells cultured from CF lung tissue following lung transplant (73). In cell culture experiments by our group and others, simultaneous inoculation of CF and non-CF airway cells with RSV and P. aeruginosa increased adherence of both mucoid and non-mucoid P. aeruginosa strains (74, 75). This effect could be blocked by pre-treating non-polarized airway cells with heparin prior to inoculation with RSV and P. aeruginosa (75). A recent study from our group found no significant difference in P. aeruginosa adherence to polarized CF airway cells with a preceding RSV infection (24–72 h) compared to control CF cells; however, co-infection with RSV, RV, and adenovirus each promoted the growth of P. aeruginosa biofilms (74). Treatment of CF airway cells with exogenous type I or type III interferon prior to P. aeruginosa inoculation also stimulated biofilm growth, suggesting P. aeruginosa benefits from the innate antiviral response in CF airway cells. Together, these studies suggest physical binding of RSV to P. aeruginosa or the CF airway cell surface may facilitate initial adherence of P. aeruginosa to the epithelium, whereas progression of viral infections and activation of interferon-stimulated innate antiviral signaling pathways may play a role in promoting chronic P. aeruginosa growth in biofilms. New work from our group finds co-infection with RSV and RV also promotes S. aureus biofilm growth on CF airway cells through as yet unidentified mechanisms (76).
Bacteria depend largely on nutrients and metabolites supplied by the host during infection, and virus co-infection has been shown to alter nutritional availability, thereby influencing bacterial responses. Iron is known to be a key nutrient required for growth and pathogenesis of many pathogens (see the Frontiers Research Topic, “Role of Iron in Bacterial Pathogenesis”). The host normally sequesters iron and other essential metals from invading microbes through a process termed nutritional immunity (77), but these mechanisms have been found to be dysregulated during virus infection. In CF airway cell studies, it was discovered that RSV infection promoted increased secretion of iron-bound host transferrin protein, which stimulated P. aeruginosa biofilm growth (74). Lipocalin-2, a host antimicrobial protein that sequesters iron (78), was found to be reduced during influenza A infection through virus-mediated suppression of NF-kB activation and IL-1B expression, exacerbating S. aureus acute pneumonia in mice (58). Other potential nutrient sources in the airways, such as surfactant proteins (79–81), and mucins (82–84), are known to change during virus co-infections, and there is evidence that CF bacterial pathogens can utilize these nutrient sources (85–88), but specific links between these nutrient shifts and viral-bacterial co-infections CF have not yet been confirmed.
Conversely, bacterial interactions with the CF airway epithelium can also alter subsequent virus infection. In one study, pre-infection of CF human bronchial cells with P. aeruginosa was found to diminish the interferon response to RV infection and resulted in a higher RV load than RV infection alone (89). As CF cells showed increased generation of reactive oxygen species (ROS) at baseline compared to non-CF cells, treatment of CF cells with antioxidants prior to P. aeruginosa infection helped to restore the IFN response, and it was observed that while RV infection alone acted through PI-3 kinase to induce Akt phosphorylation, this was prevented by pre-infection of CF airway cells with P. aeruginosa. A later study evaluating the effects of P. aeruginosa secreted factors on primary CF and non-CF airway cells observed no effect of pre-treatment on RV load or antiviral gene expression; however, pre-exposure of cells to P. aeruginosa secreted factors did potentiate IL-8 production upon subsequent RV infection (90). Another secreted P. aeruginosa protein, Cif, also potentiated virus infections in CF airway epithelial cells by preventing MHC class I antigen presentation and CD8 T cell-mediated clearance of influenza A-infected cells (91). Taken together, these findings suggest a complex interplay between bacterial pathogens, respiratory viruses, and the innate immune response in the CF airway epithelium, where an appropriate immune response to one pathogen may alter secondary infection by another pathogen. The effects of virus co-infection on many prominent CF bacterial pathogens has yet to be evaluated, and relationships between respiratory viruses and bacteria in multi-species polymicrobial infections representative of the complex communities existing in the CF airways is also an underexplored area.
Impact of Therapeutics on Co-Infections
Antimicrobial Treatments for Viral and Bacterial Infections
As we've observed with the host immune response, attempts to clear one type of pathogen may have unintended effects on other microbes in the CF airways. The same may be true for cases of antiviral or antibacterial treatments administered to CF patients. It is appreciated that despite intense antibiotic therapy and even with alternating antibiotic courses, chronic infections with bacterial pathogens established as biofilms in the CF airways resist clearance through multiple mechanisms reviewed in Høiby et al. (92) and Lambert (93). Several therapies are now shown to impact both viral and bacterial pathogens, potentially leading to new therapeutic options for polymicrobial infections. We recently reported that an engineered antimicrobial peptide therapy, WLBU2, reduced both bacterial biofilm, and RSV titers in a mixed infection model in vitro (94). In addition, members of the macrolide class of antibiotics, including erythromycin, azithromycin, and bafilomycin, which are known to effect antibacterial activity by binding to bacterial ribosomal subunits to inhibit protein synthesis (95) were also found to have anti-inflammatory effects. By blocking production of the pro-inflammatory cytokines IL-6 and IL-8, macrolide antibiotics reduced neutrophil recruitment to sites of injury, and infection (96). In non-CF bronchial epithelial cells, azithromycin (97), bafilomycin (98), and clarithromycin (99) treatments were found to reduce RV replication by increasing induction of interferon-stimulated antiviral genes, demonstrating that in addition to its anti-inflammatory, and anti-bacterial properties, azithromycin has anti-viral activity. In CF airway cells, azithromycin also reduced RV replication and increased RV-induced expression of interferon and interferon-stimulated antiviral genes; however, azithromycin did not prevent induction of IL-6 or IL-8 during RV infection, suggesting that the anti-inflammatory effects of azithromycin are diminished during a virus infection (51). Administration of azithromycin as an antiviral or anti-inflammatory agent could provide a potential therapeutic option for CF patients, yet as macrolide resistance is known to be widespread in clinical isolates from chronic airway infections (100), it is important to keep in mind the broader effects antibiotic administration may have on the CF microbiome.
As viral infections can lead to severe respiratory morbidity and are linked to exacerbations in CF populations, there is a demand for effective anti-viral therapies, especially for major CF pathogens like RV and RSV for which no successful vaccine exists. RSV immunotherapy was shown to be effective at preventing lower respiratory tract infections and reducing symptom severity in high-risk infants, and young children (101). A humanized monocolonal antibody treatment for RSV, palivizumab, was developed (102) and prophylactic treatment with palivizumab significantly reduced hospitalizations (103), and incidences of respiratory-related illness (104) in CF children compared to untreated control groups. While potentially effective at preventing RSV infection, palivizumab prophylaxis is costly and has shown limited benefits for populations that do not regularly have high incidence of RSV-related hospitalizations (105), leading some to propose that anti-RSV therapy would be best-reserved for treatment during infections, not as prophylaxis, or for fall, and winter seasons when probability of virus-related illnesses and hospitalizations typically increases (106). As it has been observed that virus co-infection promotes P. aeruginosa colonization, a secondary benefit of antiviral therapies could be a delay in acquisition of bacterial pathogens in CF children. However, a recent study found that prophylactic treatment of CF infants with palivizumab to prevent RSV infection did not delay acquisition of either P. aeruginosa or S. aureus (107). A separate retrospective study found that although palivizumab reduced RSV-related hospitalizations and overall P. aeruginosa chronic colonization rates did not differ between treatment and control groups, the time to first P. aeruginosa isolate was significantly earlier in palivizumab-treated CF children (108). Many factors could have affected these outcomes, including patients' genetics, environmental exposures, and differences in clinical care quality and access. Broader studies evaluating the impact of palivizumab on the CF microbiome, including changes in abundance of commensal and pathogenic bacterial species, could shed light on how anti-viral therapies affect viral-bacterial-host interactions.
CFTR Modulators and Impact on Infections
In the field of CF research and patient care, there is great excitement surrounding the promise of CFTR modulating drugs that improve mutated CFTR rescue to the cell surface (correctors) or modulate activity of dysfunctional CFTR protein channels (potentiators). Three drugs have undergone clinical trials and are now options for CF patients with specific CFTR mutations: the potentiator ivacaftor and correctors lumacaftor and tezacaftor (109, 110). Trials showed CF patients treated with CFTR modulators had improved lung function and decreased rates of pulmonary exacerbations, hospitalization, and IV antibiotic use, and the first studies on how these treatments impact respiratory microbiology in CF patients are now becoming available. During clinical trials, CF patients receiving ivacaftor, and lumacaftor were still found to experience adverse events, including upper respiratory infections (usually attributed to acute respiratory viral infection), at rates similar to placebo groups (111–113), and CF patients experiencing acute upper or lower respiratory infections have also been excluded from trials on the basis that this could confound results, although this practice may limit knowledge of the impact of these drugs during infection (113).
In vitro studies of ivacaftor, whose structure resembles that of quinolone antibiotics, found that ivacaftor has dose-dependent antibacterial activity against S. aureus and S. pneumoniae clinical isolates, and synergy of ivacaftor and the anti-Gram positive antibiotic vancomycin was observed (114). Ivacaftor was also observed to have a milder antimicrobial effect toward P. aeruginosa that was improved in combination with the anti-pseudomonal antibiotic ciprofloxacin. These results suggest that in addition to the intended ability of CFTR modulators to improve CFTR production and function, these treatments could have the added benefit of helping to reduce certain bacterial populations in the CF airways.
Studies evaluating changes in the airway microbiome of CF patients undergoing CFTR modulator therapies can begin to inform us if the above observed properties of CFTR modulators translate to the clinic. A small study using quantitative PCR and 16S sequencing to evaluate the microbiome of three CF children undergoing ivacaftor treatment found no significance in overall bacterial burden or bacterial genera represented prior to and following treatment, and individual patients' microbiomes pre- and post-treatment were found to be more similar than treated vs. non-treated microbiomes across all patients (115). Recent work evaluating CF adults showed that ivacaftor treatment reduced P. aeruginosa and overall bacterial density in sputum samples, yet despite immediate effects, the same clonal isolates of P. aeruginosa sampled prior to treatment persisted in the airways of CF patients after ivacaftor (116). Inflammatory markers in patient sputum were found to be decreased in patients through mass spectrometry analysis of sputum proteins, and a separate study unexpectedly found that ivacaftor treatment dampened the interferon-gamma response and impaired monocyte recruitment, effects that modulate immune responses in the respiratory tract and could potentially influence disease outcome (117). Trials conducted in adult populations, many of whom are already chronically colonized with P. aeruginosa, do not allow for evaluation of the potential impact of CFTR modulators in preventing P. aeruginosa acquisition. Initiating new studies in younger patient populations following modulator treatment and following patients that begin treatment culturing P. aeruginosa-negative for longer periods of time post-treatment will be important to address the question of whether CFTR modulators impact P. aeruginosa acquisition. No specific effects of CFTR modulators on viral-bacterial co-infections have been reported to date, and valuable information could be gained from testing for the presence of specific respiratory viruses in patients undergoing modulator therapy, along with measuring changes in bacterial microbiome constituents. The above described effects of CFTR modulators on specific bacterial pathogens and the host immune response suggest co-infections will likely be impacted by such therapies and warrant further study.
New Techniques for Evaluation of Viral-Bacterial Co-Infections
Traditional means of diagnosing viral and bacterial infections through specific PCR panels or culture-based techniques, respectively, have provided the majority of our current knowledge regarding which microbes comprise the CF respiratory microbiome and can be considered pathogens in the CF airways. However, as previously mentioned, these methods of identification are limited, as each requires prior knowledge of which viruses to screen for or which bacteria to culture, and therefore we may be underestimating the number of species that exist in the airway environment and the impact they have on CF respiratory disease. The recent trend toward 16S studies has identified additional members of the bacterial microbial community, and the application of new techniques such as metagenomics, metatranscriptomics, and metabolomics to the study of the CF microbiome may begin to reveal previously unknown roles for new microbial species and offer unprecedented insight into their functions in the CF respiratory tract.
Metagenomics and Metatranscriptomics
Metagenomic studies evaluating the total genomic content of samples have great potential to identify a broader range of viruses and other microbes in CF, in addition to bacterial species. Such evaluations are technically challenging from a computational standpoint, and to date, few metagenomic studies have been published applying this technique to the study of viromes in CF populations. While RNA viruses have been the most appreciated viruses in causing acute respiratory infections in CF, a metagenomic study evaluating DNA viruses identified genomes of herpesviruses, and retroviruses in CF sputum and found overall eukaryotic viral diversity was low in both CF and non-CF individuals (118). The majority of viral diversity in airway microbial communities was found to be derived from populations of bacteriophage, with CF phage communities being more similar to one another than non-CF phage, and indicative of the dominant bacterial species residing in the CF airways that comprise the host range of the phage (118, 119). Although phage are not traditionally thought of as viruses that impact human health, recent work has shown that phage affect P. aeruginosa biofilm assembly and promote survival of bacteria in biofilms by enhancing adhesion and tolerance to antibiotics (120), and the role of phage in promoting a healthy microbiome in other organ systems, namely the gastrointestinal tract, has been a rapidly growing area of research outside of CF (121, 122). Going further, functional genomic analyses that consider the predicted functions of all genes present in the total DNA from a sample predicted the viromes of CF patients had a separate set of core metabolic functions compared to healthy subjects (118), with enrichment of genes for metabolizing aromatic amino acids. This suggests the host organisms of these phage have a specialized metabolism specific to CF disease, and the genes carried by phage represent factors necessary for survival in the CF airways.
Similar to metagenomics, metatranscriptomics is the analysis of the total RNA content of a sample and can thus account for changes in expression of host and microbial genes. To date, limited metatranscriptomics studies have been performed evaluating both host and pathogen gene expression in the same sample. In a mouse model of acute P. aeruginosa infection, metatranscriptomics revealed genes related to P. aeruginosa outer membrane vesicle production, and iron uptake and utilization were significantly upregulated, indicating the importance of iron-mediated regulation and scavenging in vivo (123). In infected mice, expression of pro-inflammatory cytokines and chemokines associated with toll-like receptor signaling were induced. Recent dual-RNA sequencing experiments from our group show polarized CF airway cells and S. aureus exhibit altered transcriptional profiles during co-culture in the presence of RSV co-infection (76). In CF cells, differences in innate immune and inflammatory signaling were observed in the presence of RSV, S. aureus or viral-bacterial co-infection, and S. aureus exhibited an altered metabolic transcriptional profile during virus co-infection, with upregulation of genes for cofactor biosynthesis and amino acid utilization, perhaps reflecting altered availability of protein substrates in the airway surface liquid of virus-infected CF cells. The use of next-generation sequencing approaches is expanding, and these techniques as applied to CF in future studies will no doubt expand our view of viruses and other underappreciated microbes that exist in the CF airways.
Metabolomics
Supplementing metagenomic studies that identify which microbial species are present in the airways, metabolomics studies can account for microbial and host-derived metabolites and proteins. For studies of biological samples, liquid chromatography-mass spectrometry (LC-MS) is often used to identify small molecules, and liquid chromatography-tandem mass spectrometry (LC-MS/MS) can be applied to identify sequences of individual peptides for proteomic analysis in complex samples (124).
A novel application of MS techniques is analysis of volatile compounds found in breath to diagnose infections. Volatile compounds produced during microbial metabolism can provide species-specific signatures based on knowledge of individual species' metabolic capabilities from genome sequences, and variation in volatile compound production can be an indicator of compounds available for use in the environment. Early studies in CF utilized the knowledge that P. aeruginosa produces cyanide, and using cyanide as a biomarker, selected ion flow mass spectrometry (SIFT-MS) was able to identify the presence of P. aeruginosa in the airways by sampling the breath of infected CF patients (125, 126). Later studies showed that beyond identifying presence of a specific molecule, groups of P. aeruginosa-colonized CF patients could be differentiated from non-colonized CF patients based on overall volatile breath profiles (127). Using genomic data available for other CF-related bacterial species to predict organisms capable of producing specific volatile compounds, high levels of acetaldehyde, ethanol, and methanol in CF subjects' breath were linked to Lactococcus, Escherichia and Rothia species, respectively (128), confirming that metabolic predictions based on genetic sequence could translate to positive identification in patient samples for species other than P. aeruginosa.
Translating breath detection to bench studies, a volatile fingerprint could be identified for CF airway cells co-cultured with P. aeruginosa (129). Evaluating RSV-P. aeruginosa co-infections in CF airway cells, different levels of volatile compounds were found to be produced during co-infection rather than infection with either P. aeruginosa or RSV alone, and predictive models were able to discriminate P. aeruginosa-infected cells, but not cells undergoing only RSV infection (129). Breath diagnosis could prove to be a quick, non-invasive, and culture-independent means for diagnosing the presence of CF pathogens, and with additional knowledge of the links between overall metabolic state and respiratory function, breath testing could serve as an indicator of a stable or exacerbating CF airway environment. The prospect of using breath analysis to identify a virus co-infection is intriguing, and in vitro studies require further investigation and translation into CF patients undergoing respiratory viral infections to confirm specific volatile signatures for co-infections.
In summary, the individual roles of bacterial and viral infections in CF respiratory disease have long been appreciated, and independently, viral infections, and chronic bacterial infections are known to influence pulmonary exacerbations and progression of respiratory function decline. Now, advances in sequencing technology have facilitated our understanding of the complexity of the microbial communities in the CF airways, bringing to light new interactions between bacteria and viruses in the airways that suggest microbial population dynamics and interplay between microbes and the host, and not just the presence of known pathogens, could be the true drivers of CF respiratory disease. While metabolomics has not yet been used to evaluate differences in metabolite profiles in CF patients with respiratory viral infections or viral-bacterial co-infections, the technology used in the above described studies provide an exciting window into potential host- and bacterial-associated changes in metabolism that likely accompany co-infections. Expanding the use of such new technologies in clinical and basic CF research will undoubtedly allow us to better understand these microbe-microbe and microbe-host interactions, improving our ability to more accurately diagnose and treat respiratory infections in CF patients and informing us of underlying mechanisms of microbial pathogenesis in the CF respiratory tract.
Author Contributions
MK and JB conceptualized and wrote the manuscript.
Funding
This work was provided by NIH R01HL123771, NIH R61HL137077, and CFF BOMBER14G0 to JB, and CFF KIEDRO1F5, CFF KIEDRO17F0, and NIH T32AI049820 to MK.
Conflict of Interest statement
The authors declare that the research was conducted in the absence of any commercial or financial relationships that could be construed as a potential conflict of interest.
References
1. Rommens JM, Iannuzzi MC, Kerem B, Drumm ML, Melmer G, Dean M, et al. Identification of the cystic fibrosis gene: chromosome walking and jumping. Science (1989) 245:1059–65. doi: 10.1126/science.2772657
2. Welsh MJ, Smith AE. Molecular mechanisms of CFTR chloride channel dysfunction in cystic fibrosis. Cell (1993) 73:1251–4. doi: 10.1016/0092-8674(93)90353-R
4. Rowntree RK, Harris A. The phenotypic consequences of CFTR mutations. Ann Hum Genet. (2003) 67:471–85. doi: 10.1046/j.1469-1809.2003.00028.x
5. Nichols D, Chmiel J, Berger M. Chronic inflammation in the cystic fibrosis lung: alterations in inter- and intracellular signaling. Clin Rev Allergy Immunol. (2008) 34:146–62. doi: 10.1007/s12016-007-8039-9
6. Gifford AM, Chalmers JD. The role of neutrophils in cystic fibrosis. Curr Opin Hematol. (2014) 21:16–22. doi: 10.1097/MOH.0000000000000009
7. Pezzulo AA, Tang XX, Hoegger MJ, Alaiwa MHA, Ramachandran S, Moninger TO, et al. Reduced airway surface pH impairs bacterial killing in the porcine cystic fibrosis lung. Nature (2012) 487:109–13. doi: 10.1038/nature11130
8. Quinton PM. Role of epithelial HCO3? transport in mucin secretion: lessons from cystic fibrosis. Am J Physiol Cell Physiol. (2010) 299:C1222–33. doi: 10.1152/ajpcell.00362.2010
9. Singh PK, Tack BF, McCray PB, Welsh MJ. Synergistic and additive killing by antimicrobial factors found in human airway surface liquid. Am J Physiol Lung Cell Mol Physiol. (2000) 279:L799–805. doi: 10.1152/ajplung.2000.279.5.L799
10. Nguyen LDN, Viscogliosi E, Delhaes L. The lung mycobiome: an emerging field of the human respiratory microbiome. Front Microbiol. (2015) 6:89. doi: 10.3389/fmicb.2015.00089
11. Surette MG. The cystic fibrosis lung microbiome. Ann Am Thorac Soc. (2014) 11 (Suppl. 1):S61–5. doi: 10.1513/AnnalsATS.201306-159MG
12. Recommendations of the Clinical Subcommittee of the Medical/Scientific Advisory Committee of the Canadian Cystic Fibrosis Foundation. Microbiological processing of respiratory specimens from patients with cystic fibrosis. Can J Infect Dis. (1993) 4:166–9. doi: 10.1155/1993/989086
13. Muyzer G, de Waal EC, Uitterlinden AG. Profiling of complex microbial populations by denaturing gradient gel electrophoresis analysis of polymerase chain reaction-amplified genes coding for 16S rRNA. Appli Environ Microbiol. (1993) 59:695–700.
14. Mahboubi MA, Carmody LA, Foster BK, Kalikin LM, VanDevanter DR, LiPuma JJ. Culture-based and culture-independent bacteriologic analysis of cystic fibrosis respiratory specimens. J Clin Microbiol. (2016) 54:613–9. doi: 10.1128/JCM.02299-15
15. Sibley CD, Grinwis ME, Field TR, Eshaghurshan CS, Faria MM, Dowd SE, et al. Culture enriched molecular profiling of the cystic fibrosis airway microbiome. PLoS ONE (2011) 6:e22702. doi: 10.1371/journal.pone.0022702
16. Zemanick ET, Sagel SD, Harris JK. The airway microbiome in cystic fibrosis and implications for treatment. Curr Opin Pediatr. (2011) 23:319–24. doi: 10.1097/MOP.0b013e32834604f2
17. Coburn B, Wang PW, Diaz Caballero J, Clark ST, Brahma V, Donaldson S, et al. Lung microbiota across age and disease stage in cystic fibrosis. Sci Rep. (2015) 5:10241. doi: 10.1038/srep10241
18. Filkins LM, Hampton TH, Gifford AH, Gross MJ, Hogan DA, Sogin ML, et al. Prevalence of streptococci and increased polymicrobial diversity associated with cystic fibrosis patient stability. J Bacteriol. (2012) 194:4709–17. doi: 10.1128/JB.00566-12
19. Cox MJ, Allgaier M, Taylor B, Baek MS, Huang YJ, Daly RA, et al. Airway microbiota and pathogen abundance in age-stratified cystic fibrosis patients. PLoS ONE (2010) 5:e11044. doi: 10.1371/journal.pone.0011044
20. Fodor AA, Klem ER, Gilpin DF, Elborn JS, Boucher RC, Tunney MM, et al. The adult cystic fibrosis airway microbiota is stable over time and infection type, and highly resilient to antibiotic treatment of exacerbations. PLoS ONE (2012) 7:e45001. doi: 10.1371/journal.pone.0045001
21. Smith DJ, Badrick AC, Zakrzewski M, Krause L, Bell SC, Anderson GJ, et al. Pyrosequencing reveals transient cystic fibrosis lung microbiome changes with intravenous antibiotics. Eur Respir J. (2014) 44:922–30. doi: 10.1183/09031936.00203013
22. Scoffield JA, Wu H. Oral streptococci and nitrite-mediated interference of Pseudomonas aeruginosa. Infect Immun. (2015) 83:101–7. doi: 10.1128/IAI.02396-14
23. Scoffield JA, Wu H. Nitrite reductase is critical for Pseudomonas aeruginosa survival during co-infection with the oral commensal Streptococcus parasanguinis. Microbiology (2016) 162:376–83. doi: 10.1099/mic.0.000226
24. Scoffield JA, Duan D, Zhu F, Wu H. A commensal streptococcus hijacks a Pseudomonas aeruginosa exopolysaccharide to promote biofilm formation. PLoS Pathog. (2017) 13:e1006300. doi: 10.1371/journal.ppat.1006300
25. Conrad D, Haynes M, Salamon P, Rainey PB, Youle M, Rohwer F. Cystic fibrosis therapy: a community ecology perspective. Am J Respir Cell Mol Biol. (2013) 48:150–6. doi: 10.1165/rcmb.2012-0059PS
26. Sherrard LJ, Tunney MM, Elborn JS. Antimicrobial resistance in the respiratory microbiota of people with cystic fibrosis. Lancet (2014) 384:703–13. doi: 10.1016/S0140-6736(14)61137-5
27. Kerem E, Viviani L, Zolin A, MacNeill S, Hatziagorou E, Ellemunter H, et al. Factors associated with FEV1 decline in cystic fibrosis: analysis of the data of the ECFS patient registry. Eur Respir J. (2013) 43:125–33. doi: 10.1183/09031936.00166412
28. Goss CH, Muhlebach MS. Review: Staphylococcus aureus and MRSA in cystic fibrosis. J Cyst Fibros. (2011) 10:298–306. doi: 10.1016/j.jcf.2011.06.002
29. Dalbøge CS, Hansen CR, Pressler T, Høiby N, Johansen HK. Chronic pulmonary infection with Stenotrophomonas maltophilia and lung function in patients with cystic fibrosis. J Cyst Fibros. (2011) 10:318–25. doi: 10.1016/j.jcf.2011.03.006
30. Costerton JW. Cystic fibrosis pathogenesis and the role of biofilms in persistent infection. Trends Microbiol. (2001) 9:50–2. doi: 10.1016/S0966-842X(00)01918-1
31. Heijerman H. Infection and inflammation in cystic fibrosis: a short review. J Cyst Fibros. (2005) 4 (Suppl. 2):3–5. doi: 10.1016/j.jcf.2005.05.005
32. Lyczak JB, Cannon CL, Pier GB. Lung infections associated with cystic fibrosis. Clin Microbiol Rev. (2002) 15:194–222. doi: 10.1128/CMR.15.2.194-222.2002
33. Armstrong D, Grimwood K, Carlin JB, Carzino R, Hull J, Olinsky A, et al. Severe viral respiratory infections in infants with cystic fibrosis. Pediatr Pulmonol. (1998) 26:371–9.
34. Wat D, Doull I. Respiratory virus infections in cystic fibrosis. Paediatr Respir Rev. (2003) 4:172–7. doi: 10.1016/S1526-0542(03)00059-9
35. Collinson J, Nicholson KG, Cancio E, Ashman J, Ireland DC, Hammersley V, et al. Effects of upper respiratory tract infections in patients with cystic fibrosis. Thorax (1996) 51:1115–22. doi: 10.1136/thx.51.11.1115
36. Smyth AR, Smyth RL, Tong CY, Hart CA, Heaf DP. Effect of respiratory virus infections including rhinovirus on clinical status in cystic fibrosis. Arch Dis Child. (1995) 73:117–20. doi: 10.1136/adc.73.2.117
37. Simoes EA. Respiratory syncytial virus infection. Lancet (1999) 354:847–52. doi: 10.1016/S0140-6736(99)80040-3
38. Abman SH, Ogle JW, Butler-Simon N, Rumack CM, Accurso FJ. Role of respiratory syncytial virus in early hospitalizations for respiratory distress of young infants with cystic fibrosis. J Pediatr. (1988) 113:826–30. doi: 10.1016/S0022-3476(88)80008-8
39. Rosenfeld M, Emerson J, Williams-Warren J, Pepe M, Smith A, Montgomery AB, et al. Defining a pulmonary exacerbation in cystic fibrosis. J Pediatr. (2001) 139:359–65. doi: 10.1067/mpd.2001.117288
40. Goss CH, Burns JL. Exacerbations in cystic fibrosis. 1: Epidemiology and pathogenesis. Thorax (2007) 62:360–7. doi: 10.1136/thx.2006.060889
41. Smyth A, Elborn JS. Exacerbations in cystic fibrosis: 3 management. Thorax (2008) 63:180–4. doi: 10.1136/thx.2006.060905
42. Hiatt PW, Grace SC, Kozinetz CA, Raboudi SH, Treece DG, Taber LH, et al. Effects of viral lower respiratory tract infection on lung function in infants with cystic fibrosis. Pediatrics (1999) 103:619–26. doi: 10.1542/peds.103.3.619
43. Wang EE, Prober CG, Manson B, Corey M, Levison H. Association of respiratory viral infections with pulmonary deterioration in patients with cystic fibrosis. N Engl J Med. (1984) 311:1653–8. doi: 10.1056/NEJM198412273112602
44. Johansen HK, Høiby N. Seasonal onset of initial colonisation and chronic infection with Pseudomonas aeruginosa in patients with cystic fibrosis in Denmark. Thorax (1992) 47:109–11. doi: 10.1136/thx.47.2.109
45. van Ewijk BE, van der Zalm MM, Wolfs TFW, van der Ent CK. Viral respiratory infections in cystic fibrosis. J Cyst Fibros. (2005) 4:31–6. doi: 10.1016/j.jcf.2005.05.011
46. van Ewijk BE, van der Zalm MM, Wolfs TFW, Fleer A, Kimpen JLL, Wilbrink B, et al. Prevalence and impact of respiratory viral infections in young children with cystic fibrosis: prospective cohort study. Pediatrics (2008) 122:1171–6. doi: 10.1542/peds.2007-3139
47. Asner S, Waters V, Solomon M, Yau Y, Richardson SE, Grasemann H, et al. Role of respiratory viruses in pulmonary exacerbations in children with cystic fibrosis. J Cyst Fibros. (2012) 11:433–9. doi: 10.1016/j.jcf.2012.04.006
48. Wark PAB, Tooze M, Cheese L, Whitehead B, Gibson PG, Wark KF, et al. Viral infections trigger exacerbations of cystic fibrosis in adults and children. Eur Respir J. (2012) 40:510–2. doi: 10.1183/09031936.00202311
49. Flight WG, Bright-Thomas RJ, Tilston P, Mutton KJ, Guiver M, Morris J, et al. Incidence and clinical impact of respiratory viruses in adults with cystic fibrosis. Thorax (2014) 69:247–53. doi: 10.1136/thoraxjnl-2013-204000
50. Goffard A, Lambert V, Salleron J, Herwegh S, Engelmann I, Pinel C, et al. Virus and cystic fibrosis: rhinoviruses are associated with exacerbations in adult patients. J Clin Virol. (2014) 60:147–53. doi: 10.1016/j.jcv.2014.02.005
51. Schögler A, Kopf BS, Edwards MR, Johnston SL, Casaulta C, Kieninger E, et al. Novel antiviral properties of azithromycin in cystic fibrosis airway epithelial cells. Eur Respir J. (2015) 45:428–39. doi: 10.1183/09031936.00102014
52. Schögler A, Stokes AB, Casaulta C, Regamey N, Edwards MR, Johnston SL, et al. Interferon response of the cystic fibrosis bronchial epithelium to major and minor group rhinovirus infection. J Cyst Fibros. (2016) 15:332–9. doi: 10.1016/j.jcf.2015.10.013
53. Kieninger E, Singer F, Tapparel C, Alves MP, Latzin P, Tan H-L, et al. High rhinovirus burden in lower airways of children with cystic fibrosis. Chest (2013) 143:782–90. doi: 10.1378/chest.12-0954
54. Dijkema JS, van Ewijk BE, Wilbrink B, Wolfs TFW, Kimpen JLL, van der Ent CK. Frequency and duration of rhinovirus infections in children with cystic fibrosis and healthy controls: a longitudinal cohort study. Pediatr Infect Dis J. (2016) 35:379–83. doi: 10.1097/INF.0000000000001014
55. Rynda-Apple A, Robinson KM, Alcorn JF. Influenza and bacterial super-infection: illuminating the immunologic mechanisms of disease. Infect Immun. (2015) 83:3764–70. doi: 10.1128/IAI.00298-15
56. Shahangian A, Chow EK, Tian X, Kang JR, Ghaffari A, Liu SY, et al. Type I IFNs mediate development of postinfluenza bacterial pneumonia in mice. J Clin Invest. (2009) 119:1910–20. doi: 10.1172/JCI35412
57. Lee B, Robinson KM, McHugh KJ, Scheller EV, Mandalapu S, Chen C, et al. Influenza-induced type I interferon enhances susceptibility to gram-negative and gram-positive bacterial pneumonia in mice. Am J Physiol Lung Cell Mol Physiol. (2015) 309:L158–67. doi: 10.1152/ajplung.00338.2014
58. Robinson KM, Choi S-M, McHugh KJ, Mandalapu S, Enelow RI, Kolls JK, et al. Influenza A exacerbates Staphylococcus aureus pneumonia by attenuating IL-1β production in mice. J Immunol. (2013) 191:5153–9. doi: 10.4049/jimmunol.1301237
59. Kudva A, Scheller EV, Robinson KM, Crowe CR, Choi S-M, Slight SR, et al. Influenza A inhibits Th17-mediated host defense against bacterial pneumonia in mice. J Immunol. (2011) 186:1666–74. doi: 10.4049/jimmunol.1002194
60. McCullers JA. The co-pathogenesis of influenza viruses with bacteria in the lung. Nat Rev Microbiol. (2014) 12:252–62. doi: 10.1038/nrmicro3231
61. Sun K, Metzger DW. Inhibition of pulmonary antibacterial defense by interferon-gamma during recovery from influenza infection. Nat Med. (2008) 14:558–64. doi: 10.1038/nm1765
62. Planet PJ, Parker D, Cohen TS, Smith H, Leon JD, Ryan C, et al. Lambda interferon restructures the nasal microbiome and increases susceptibility to Staphylococcus aureus superinfection. mBio (2016) 7:e01939–15. doi: 10.1128/mBio.01939-15
63. Tarabichi Y, Li K, Hu S, Nguyen C, Wang X, Elashoff D, et al. The administration of intranasal live attenuated influenza vaccine induces changes in the nasal microbiota and nasal epithelium gene expression profiles. Microbiome (2015) 3:74. doi: 10.1186/s40168-015-0133-2
64. Carmody LA, Zhao J, Schloss PD, Petrosino JF, Murray S, Young VB, et al. Changes in cystic fibrosis airway microbiota at pulmonary exacerbation. Ann Am Thorac Soc. (2013) 10:179–87. doi: 10.1513/AnnalsATS.201211-107OC
65. Price KE, Hampton TH, Gifford AH, Dolben EL, Hogan DA, Morrison HG, et al. Unique microbial communities persist in individual cystic fibrosis patients throughout a clinical exacerbation. Microbiome (2013) 1:27. doi: 10.1186/2049-2618-1-27
66. Stressmann FA, Rogers GB, Marsh P, Lilley AK, Daniels TWV, Carroll MP, et al. Does bacterial density in cystic fibrosis sputum increase prior to pulmonary exacerbation? J Cyst Fibros. (2011) 10:357–65. doi: 10.1016/j.jcf.2011.05.002
67. Chin M, De Zoysa M, Slinger R, Gaudet E, Vandemheen KL, Chan F, et al. Acute effects of viral respiratory tract infections on sputum bacterial density during CF pulmonary exacerbations. J Cyst Fibros. (2015) 14:482–9. doi: 10.1016/j.jcf.2014.11.009
68. Esther CR, Lin FC, Kerr A, Miller MB, Gilligan PH. Respiratory viruses are associated with common respiratory pathogens in cystic fibrosis. Pediatr Pulmonol. (2014) 49:926–31. doi: 10.1002/ppul.22917
69. Miró-Cañís S, Capilla-Rubio S, Marzo-Checa L, Fontanals-Aymerich D, Sanfeliu-Sala I, Espasa-Soley M, et al. Multiplex PCR reveals that viruses are more frequent than bacteria in children with cystic fibrosis. J Clin Virol. (2017) 86:1–4. doi: 10.1016/j.jcv.2016.11.004
70. Wat D, Gelder C, Hibbitts S, Cafferty F, Bowler I, Pierrepoint M, et al. The role of respiratory viruses in cystic fibrosis. J Cyst Fibros. (2008) 7:320–8. doi: 10.1016/j.jcf.2007.12.002
71. Petersen NT, Høiby N, Mordhorst CH, Lind K, Flensborg EW, Bruun B. Respiratory infections in cystic fibrosis patients caused by virus, chlamydia and mycoplasma–possible synergism with Pseudomonas aeruginosa. Acta Paediatr Scand. (1981) 70:623–8. doi: 10.1111/j.1651-2227.1981.tb05757.x
72. Gruenert DC, Willems M, Cassiman JJ, Frizzell RA. Established cell lines used in cystic fibrosis research. J Cyst Fibros. (2004) 3 (Suppl 2):191–6. doi: 10.1016/j.jcf.2004.05.040
73. Randell SH, Fulcher ML, O'Neal W, Olsen JC. Primary epithelial cell models for cystic fibrosis research. Methods Mol Biol. (2011) 742:285–310. doi: 10.1007/978-1-61779-120-8_18
74. Hendricks MR, Lashua LP, Fischer DK, Flitter BA, Eichinger KM, Durbin JE, et al. Respiratory syncytial virus infection enhances Pseudomonas aeruginosa biofilm growth through dysregulation of nutritional immunity. Proc Natl Acad Sci USA. (2016) 113:1642–7. doi: 10.1073/pnas.1516979113
75. van Ewijk BE, Wolfs TFW, Aerts PC, Van Kessel KPM, Fleer A, Kimpen JLL, et al. RSV mediates Pseudomonas aeruginosa binding to cystic fibrosis and normal epithelial cells. Pediatr Res. (2007) 61:398–403. doi: 10.1203/pdr.0b013e3180332d1c
76. Kiedrowski MR, Gaston JR, Kocak BR, Coburn SL, Lee S, Pilewski JM, et al. Staphylococcus aureus biofilm growth on Cystic Fibrosis airway epithelial cells is enhanced during respiratory syncytial virus co-infection. mSphere (2018) 3:e00341–18. doi: 10.1128/mSphere.00341-18
77. Hood MI, Skaar EP. Nutritional immunity: transition metals at the pathogen-host interface. Nat Rev Microbiol. (2012) 10:525–37. doi: 10.1038/nrmicro2836
78. Flo TH, Smith KD, Sato S, Rodriguez DJ, Holmes MA, Strong RK, et al. Lipocalin 2 mediates an innate immune response to bacterial infection by sequestrating iron. Nature (2004) 432:917–21. doi: 10.1038/nature03104
79. Bruce SR, Atkins CL, Colasurdo GN, Alcorn JL. Respiratory syncytial virus infection alters surfactant protein A expression in human pulmonary epithelial cells by reducing translation efficiency. Am J Physiol Lung Cell Mol Physiol. (2009) 297:L559–67. doi: 10.1152/ajplung.90507.2008
80. Griese M. Respiratory syncytial virus and pulmonary surfactant. Viral Immunol. (2002) 15:357–63. doi: 10.1089/08828240260066279
81. Numata M, Chu HW, Dakhama A, Voelker DR. Pulmonary surfactant phosphatidylglycerol inhibits respiratory syncytial virus-induced inflammation and infection. Proc Natl Acad Sci USA. (2010) 107:320–5. doi: 10.1073/pnas.0909361107
82. Siegel SJ, Roche AM, Weiser JN. Influenza promotes pneumococcal growth during coinfection by providing host sialylated substrates as a nutrient source. Cell Host Microbe (2014) 16:55–67. doi: 10.1016/j.chom.2014.06.005
83. Baños-Lara MDR, Piao B, Guerrero-Plata A. Differential mucin expression by respiratory syncytial virus and human metapneumovirus infection in human epithelial cells. Mediators Inflamm. (2015) 2015:347292. doi: 10.1155/2015/347292
84. Currier MG, Lee S, Stobart CC, Hotard AL, Villenave R, Meng J, et al. EGFR interacts with the fusion protein of respiratory syncytial virus strain 2–20 and mediates infection and mucin expression. PLoS Pathog (2016) 12:e1005622. doi: 10.1371/journal.ppat.1005622
85. Aristoteli LP, Willcox MDP. Mucin degradation mechanisms by distinct Pseudomonas aeruginosa isolates in vitro. Infect Immun. (2003) 71:5565–75. doi: 10.1128/IAI.71.10.5565-5575.2003
86. Flynn JM, Niccum D, Dunitz JM, Hunter RC. Evidence and role for bacterial mucin degradation in cystic fibrosis airway disease. PLoS Pathog. (2016) 12:e1005846. doi: 10.1371/journal.ppat.1005846
87. Son MS, Matthews WJ, Kang Y, Nguyen DT, Hoang TT. In vivo evidence of Pseudomonas aeruginosa nutrient acquisition and pathogenesis in the lungs of cystic fibrosis patients. Infect Immun. (2007). 75:5313–24. doi: 10.1128/IAI.01807-06
88. Sun Z, Kang Y, Norris MH, Troyer RM, Son MS, Schweizer HP, et al. Blocking phosphatidylcholine utilization in Pseudomonas aeruginosa, via mutagenesis of fatty acid, glycerol and choline degradation pathways, confirms the importance of this nutrient source in vivo. PLoS ONE (2014) 9:e103778. doi: 10.1371/journal.pone.0103778
89. Chattoraj SS, Ganesan S, Faris A, Comstock A, Lee WM, Sajjan US. Pseudomonas aeruginosa suppresses interferon response to rhinovirus infection in Cystic fibrosis, but not in normal bronchial epithelial cells. Infect Immun. (2011) 79:4131–45. doi: 10.1128/IAI.05120-11
90. Dauletbaev N, Das M, Cammisano M, Chen H, Singh S, Kooi C, et al. Rhinovirus load is high despite preserved interferon-β response in cystic fibrosis bronchial epithelial cells. PLoS ONE (2015) 10:e0143129. doi: 10.1371/journal.pone.0143129
91. Bomberger JM, Ely KH, Bangia N, Ye S, Green KA, Green WR, et al. Pseudomonas aeruginosa Cif protein enhances the ubiquitination and proteasomal degradation of the transporter associated with antigen processing (TAP) and reduces major histocompatibility complex (MHC) class I antigen presentation. J Biol Chem. (2014) 289:152–62. doi: 10.1074/jbc.M113.459271
92. Høiby N, Bjarnsholt T, Givskov M, Molin S, Ciofu O. Antibiotic resistance of bacterial biofilms. Int J Antimicrob Agents (2010) 35:322–32. doi: 10.1016/j.ijantimicag.2009.12.011
93. Lambert PA. Mechanisms of antibiotic resistance in Pseudomonas aeruginosa. J R Soc Med. (2002) 95 (Suppl. 41):22–6.
94. Melvin JA, Lashua LP, Kiedrowski MR, Yang G, Deslouches B, Montelaro RC, et al. Simultaneous antibiofilm and antiviral activities of an engineered antimicrobial peptide during virus-bacterium coinfection. mSphere (2016) 1:e00083–16. doi: 10.1128/mSphere.00083-16
95. Champney WS, Burdine R. Macrolide antibiotics inhibit 50S ribosomal subunit assembly in Bacillus subtilis and Staphylococcus aureus. Antimicrob Agents Chemother. (1995) 39:2141–4. doi: 10.1128/AAC.39.9.2141
96. Amsden GW. Anti-inflammatory effects of macrolides–an underappreciated benefit in the treatment of community-acquired respiratory tract infections and chronic inflammatory pulmonary conditions? J Antimicrob Chemother. (2005) 55:10–21. doi: 10.1093/jac/dkh519
97. Gielen V, Johnston SL, Edwards MR. Azithromycin induces anti-viral responses in bronchial epithelial cells. Eur Respir J. (2010) 36:646–54. doi: 10.1183/09031936.00095809
98. Suzuki T, Yamaya M, Sekizawa K, Hosoda M, Yamada N, Ishizuka S, et al. Bafilomycin A1 inhibits rhinovirus infection in human airway epithelium: effects on endosome and ICAM-1. Am J Physiol Lung Cell Mol Physiol. (2001) 280:L1115–27. doi: 10.1152/ajplung.2001.280.6.L1115
99. Jang YJ, Kwon H-J, Lee B-J. Effect of clarithromycin on rhinovirus-16 infection in A549 cells. Eur Respir J. (2006) 27:12–9. doi: 10.1183/09031936.06.00008005
100. Phaff SJ, Tiddens HAWM, Verbrugh HA, Ott A. Macrolide resistance of Staphylococcus aureus and Haemophilus species associated with long-term azithromycin use in cystic fibrosis. J Antimicrob Chemother. (2006) 57:741–6. doi: 10.1093/jac/dkl014
101. Groothuis JR, Simoes EA, Levin MJ, Hall CB, Long CE, Rodriguez WJ, et al. Prophylactic administration of respiratory syncytial virus immune globulin to high-risk infants and young children. The respiratory syncytial virus immune globulin study group. N Engl J Med. (1993) 329:1524–30. doi: 10.1056/NEJM199311183292102
102. Null DM, Bimle C, Weisman LE, Johnson K, Steichen J, Singh S, et al. Palivizumab, a humanized respiratory syncytial virus monoclonal antibody, reduces hospitalization from respiratory syncytial virus infection in high-risk infants. Pediatrics (1998) 102:531–7. doi: 10.1542/peds.102.3.531
103. Giebels K, Marcotte JE, Podoba J, Rousseau C, Denis MH, Fauvel V, et al. Prophylaxis against respiratory syncytial virus in young children with cystic fibrosis. Pediatr Pulmonol. (2008) 43:169–74. doi: 10.1002/ppul.20751
104. Bjornson C, Chan P, Li A, Paes B, Lanctôt KL, Mitchell I. Palivizumab prophylaxis for respiratory syncytial virus in infants with cystic fibrosis: is there a need? Eur J Clin Microbiol Infect Dis. (2018) 37:1113–8. doi: 10.1007/s10096-018-3225-7
105. Linnane B, Kiernan MG, O'Connell NH, Kearse L, Dunne CP. Anti-RSV prophylaxis efficacy for infants and young children with cystic fibrosis in Ireland. Multidiscip Respir Med. (2015) 10:32. doi: 10.1186/s40248-015-0029-9
106. McGirr AA, Schwartz KL, Allen U, Solomon M, Sander B. The cost-effectiveness of palivizumab in infants with cystic fibrosis in the Canadian setting: a decision analysis model Hum Vaccin Immunother. (2017) 13:599–606. doi: 10.1080/21645515.2016.1235670
107. Buchs C, Dalphin M-L, Sanchez S, Perceval M, Coutier L, Mainguy C, et al. Palivizumab prophylaxis in infants with cystic fibrosis does not delay first isolation of Pseudomonas aeruginosa or Staphylococcus aureus. Eur J Pediatr. (2017) 176:891–7. doi: 10.1007/s00431-017-2926-8
108. Groves HE, Jenkins L, Macfarlane M, Reid A, Lynn F, Shields MD. Efficacy and long-term outcomes of palivizumab prophylaxis to prevent respiratory syncytial virus infection in infants with cystic fibrosis in Northern Ireland. Pediatr Pulmonol. (2016) 51:379–85. doi: 10.1002/ppul.23376
109. Wainwright CE, Elborn JS, Ramsey BW, Marigowda G, Huang X, Cipolli M, et al. Lumacaftor-ivacaftor in patients with cystic fibrosis homozygous for Phe508del CFTR. N Engl J Med. (2015) 373:220–31. doi: 10.1056/NEJMoa1409547
110. Taylor-Cousar JL, Munck A, McKone EF, van der Ent CK, Moeller A, Simard C, et al. Tezacaftor-ivacaftor in patients with cystic fibrosis homozygous for Phe508del. N Engl J Med. (2017) 377:2013–23. doi: 10.1056/NEJMoa1709846
111. Boyle MP, Bell SC, Konstan MW, McColley SA, Rowe SM, Rietschel E, et al. A CFTR corrector (lumacaftor) and a CFTR potentiator (ivacaftor) for treatment of patients with cystic fibrosis who have a phe508del CFTR mutation: a phase 2 randomised controlled trial. Lancet Respir Med. (2014) 2:527–38. doi: 10.1016/S2213-2600(14)70132-8
112. Clancy JP, Rowe SM, Accurso FJ, Aitken ML, Amin RS, Ashlock MA, et al. Results of a phase IIa study of VX-809, an investigational CFTR corrector compound, in subjects with cystic fibrosis homozygous for the F508del-CFTR mutation. Thorax (2012) 67:12–8. doi: 10.1136/thoraxjnl-2011-200393
113. Ratjen F, Hug C, Marigowda G, Tian S, Huang X, Stanojevic S, et al. Efficacy and safety of lumacaftor and ivacaftor in patients aged 6-11 years with cystic fibrosis homozygous for F508del-CFTR: a randomised, placebo-controlled phase 3 trial. Lancet Respir Med. (2017) 5:557–67. doi: 10.1016/S2213-2600(17)30215-1
114. Reznikov LR, Abou Alaiwa MH, Dohrn CL, Gansemer ND, Diekema DJ, Stoltz DA, et al. Antibacterial properties of the CFTR potentiator ivacaftor. J Cyst Fibros. (2014) 13:515–9. doi: 10.1016/j.jcf.2014.02.004
115. Bernarde C, Keravec M, Mounier J, Gouriou S, Rault G, Férec C, et al. Impact of the CFTR-potentiator ivacaftor on airway microbiota in cystic fibrosis patients carrying a G551D Mutation. PLoS ONE (2015) 10:e0124124. doi: 10.1371/journal.pone.0124124
116. Hisert KB, Heltshe SL, Pope C, Jorth P, Wu X, Edwards RM, et al. Restoring cystic fibrosis transmembrane conductance regulator function reduces airway bacteria and inflammation in people with cystic fibrosis and chronic lung infections. Am J Respir Crit Care Med. (2017) 195:1617–28. doi: 10.1164/rccm.201609-1954OC
117. Hisert KB, Schoenfelt KQ, Cooke G, Grogan B, Launspach JL, Gallagher CG, et al. Ivacaftor-induced proteomic changes suggest monocyte defects may contribute to the pathogenesis of cystic fibrosis. Am J Respir Cell Mol Biol. (2016) 54:594–7. doi: 10.1165/rcmb.2015-0322LE
118. Willner D, Furlan M, Haynes M, Schmieder R, Angly FE, Silva J, et al. Metagenomic analysis of respiratory tract DNA viral communities in cystic fibrosis and non-cystic fibrosis individuals. PLoS ONE (2009) 4:e7370. doi: 10.1371/journal.pone.0007370
119. Lim YW, Schmieder R, Haynes M, Willner D, Furlan M, Youle M, et al. Metagenomics and metatranscriptomics: windows on CF-associated viral and microbial communities. J Cyst Fibros. (2013) 12:154–64. doi: 10.1016/j.jcf.2012.07.009
120. Secor PR, Sweere JM, Michaels LA, Malkovskiy AV, Lazzareschi D, Katznelson E, et al. Filamentous bacteriophage promote biofilm assembly and function. Cell Host Microbe (2015) 18:549–59. doi: 10.1016/j.chom.2015.10.013
121. Manrique P, Bolduc B, Walk ST, van der Oost J, de Vos WM, Young MJ. Healthy human gut phageome. Proc Natl Acad Sci USA. (2016) 113:10400–5. doi: 10.1073/pnas.1601060113
122. Manrique P, Dills M, Young M. The human gut phage community and its implications for health and disease. Viruses (2017) 9:141. doi: 10.3390/v9060141
123. Damron FH, Oglesby-Sherrouse AG, Wilks A, Barbier M. Dual-seq transcriptomics reveals the battle for iron during Pseudomonas aeruginosa acute murine pneumonia. Sci Rep. (2016) 6:39172. doi: 10.1038/srep39172
124. Zhou B, Xiao JF, Tuli L, Ressom HW. LC-MS-based metabolomics. Mol BioSyst. (2012) 8:470–81. doi: 10.1039/C1MB05350G
125. Gilchrist FJ, Bright-Thomas RJ, Jones AM, Smith D, Španěl P, Webb AK, et al. Hydrogen cyanide concentrations in the breath of adult cystic fibrosis patients with and without Pseudomonas aeruginosa infection. J Breath Res. (2013) 7:026010. doi: 10.1088/1752-7155/7/2/026010
126. Gilchrist FJ, Belcher J, Jones AM, Smith D, Smyth AR, Southern KW, et al. Exhaled breath hydrogen cyanide as a marker of early Pseudomonas aeruginosa infection in children with cystic fibrosis. ERJ Open Res. (2015) 1:00044–2015. doi: 10.1183/23120541.00044-2015
127. Pabary R, Huang J, Kumar S, Alton EWFW, Bush A, Hanna GB, et al. Does mass spectrometric breath analysis detect Pseudomonas aeruginosa in cystic fibrosis? Eur Respir J. (2016) 47:994–7. doi: 10.1183/13993003.00944-2015
128. Bos LDJ, Meinardi S, Blake D, Whiteson K. Bacteria in the airways of patients with cystic fibrosis are genetically capable of producing VOCs in breath. J Breath Res. (2016) 10:047103. doi: 10.1088/1752-7163/10/4/047103
Keywords: cystic fibrosis, coinfection, polymicrobial, respiratory infection, biofilm, chronic disease
Citation: Kiedrowski MR and Bomberger JM (2018) Viral-Bacterial Co-infections in the Cystic Fibrosis Respiratory Tract. Front. Immunol. 9:3067. doi: 10.3389/fimmu.2018.03067
Received: 02 August 2018; Accepted: 11 December 2018;
Published: 20 December 2018.
Edited by:
François Trottein, Center for the National Scientific Research (CNRS), FranceReviewed by:
Mark Ambrose, University of Tasmania, AustraliaIsabelle Couillin, Center for the National Scientific Research (CNRS), France
Copyright © 2018 Kiedrowski and Bomberger. This is an open-access article distributed under the terms of the Creative Commons Attribution License (CC BY). The use, distribution or reproduction in other forums is permitted, provided the original author(s) and the copyright owner(s) are credited and that the original publication in this journal is cited, in accordance with accepted academic practice. No use, distribution or reproduction is permitted which does not comply with these terms.
*Correspondence: Jennifer M. Bomberger, amJvbWJAcGl0dC5lZHU=