- 1Department of Microbiology and Immunology, Shanghai Jiao Tong University School of Medicine, Shanghai Institute of Immunology, Shanghai, China
- 2Key Laboratory of Medical Molecular Virology of MOE/MOH, Shanghai Public Health Clinical Center, Fudan University, Shanghai, China
- 3Shanghai-MOST Key Laboratory of Health and Disease Genomics, Chinese National Human Genome Center at Shanghai, Shanghai, China
- 4Department of Microbiology, Perelman School of Medicine, University of Pennsylvania, Philadelphia, PA, United States
- 5TB Center, Shanghai Emerging and Re-emerging Infectious Disease Institute, Fudan University, Shanghai, China
Mycobacterial lipoproteins are considered to be involved in both virulence and immunoregulatory processes during Mycobacterium tuberculosis (M.tb) infection. In our previous investigations on the immunoreactivity of more than 30 M.tb proteins in active TB patients, we identified mycobacterial lipoprotein Z (LppZ) as one of the most immune dominant antigens. How LppZ triggers immune responses is still unclear. In this study, we analyzed LppZ-mediated innate and adaptive immunity using a murine air pouch model and an M.tb infection model, respectively. We found that LppZ could not only recruit inflammatory cells but also induce the production of proinflammatory cytokines inside the pouches. LppZ could also induce strong Th1 responses following immunization and confer protection against challenge with M.tb virulent strain H37Rv at a similar level to BCG vaccination but with less pathological damage in the lungs. Furthermore, we revealed the presence of LppZ-specific functional CD4+ T cells in the lungs of the challenged mice that were capable of secreting double or triple cytokines, including IFN-γ, IL-2, and TNF-α. Our study thus demonstrates that LppZ is of strong immunogenicity during M.tb infection in both humans and mice and has the ability to trigger effective innate and cellular immunity. Considering the limitations of candidate antigens in the pipeline of TB vaccine development, LppZ-mediated immune protection against M.tb challenge in the mouse model implies its potential application in vaccine development.
Introduction
Tuberculosis (TB), caused by Mycobacterium tuberculosis (M.tb) infection, remains one of the leading infectious diseases worldwide. The high prevalence of latent tuberculosis infection (LTBI), resulting from incomplete clearance after initial M.tb infection, contributes to the high incidence of active TB in epidemiological regions (1). Therefore, in addition to the requirement for rapid diagnosis and treatment, vaccine development to prevent M.tb infection and the reactivation of LTBI are urgently needed for TB control. Bacille Calmette-Guérin (BCG), the live attenuated Mycobacterium bovis strain, is the only licensed TB vaccine at present (2). Although it provides protection against miliary and meningeal TB in childhood, it exhibits less efficacy in preventing pulmonary TB in both teenagers and adults (3). Several TB vaccine candidates have entered the pipeline for clinical trials (4). However, the antigens being used for vaccine development are still very limited in number and effect. Ag85 family members are the most investigated, including Ag85A, Ag85B, and Ag85C (5,6). Other mycobacterial antigens, such as Rv0733 (7), Rv3615c (8), HspX (9), and Mtb8.4 (10) are also under investigation for their potential as vaccine candidate antigens with no obvious success. A recent successful phase 2b trial on M72/AS01E (Mtb32A and Mtb39A) highlights the incentive of exploring new antigens in TB vaccine design (11). Therefore, it is still of paramount importance to screen and identify novel candidate antigens that have high immunogenicity and appropriate immune-regulatory activity for the purpose of TB vaccine design.
In a previous study, we have investigated more than 30 M.tb derived proteins for cellular immune responses in patients with active TB (7). Amongst them, lipoprotein Z (LppZ) was identified as one of the most immune dominant antigens (7). LppZ, encoded by rv3006, belongs to lipoprotein family with around 100 members in M.tb (12). It was found prevalent in a range of mycobacterium species, including M. leprae, M. smegmatis, M. marinum, and M. bovis, and was detectable in both the membrane fraction and in the culture supernatant of M.tb (13–15). LppZ was demonstrated to have the highest antibody reactivity in TB patients when compared to Ag85A or other lipoproteins such as PstS-1 (38 kDa antigen) and PstS-2, which are also abundant among culture filtrate proteins (14, 16). Furthermore, LppZ was shown to be sero-reactive in both cavitary and non-cavitary TB patients and to have sero-diagnostic potential for TB (17). In view of its strong immune responsiveness in active TB patients, whether and how LppZ might exert immune regulation was considered worthy of further exploration.
In the present study, T cell responsiveness to LppZ was extensively analyzed in active TB patients. Subsequently, the immunogenicity and protective efficacy of LppZ were determined systematically in murine models to provide initial evidence of the potential of LppZ as a new TB vaccine candidate antigen.
Materials and Methods
Study Subjects
All pulmonary TB patients (n = 86) and healthy volunteers (n = 48) enrolled in this study were adults vaccinated with the BCG Shanghai strain (Shanghai Institute of Biological Products Co. Ltd., Shanghai, China) at birth. TB patients were in-patients of Shanghai Public Health Clinical Center (SHAPHC, Shanghai, China) and signed voluntary informed consents in accordance with the Declaration of Helsinki. The healthy volunteers were defined as having no medical history of M.tb infection, a normal physical examination (including blood test, serum chemistry, chest X-rays) with no disease symptoms and no evidence of latent M.tb infection (negative in T-SPOT.TB assay). The study was approved by the Ethical Committee of Shanghai Jiao Tong University School of Medicine (SJTUSM).
Mice and Ethics Statement
C57BL/6 female mice (6–8 weeks old) were purchased from Shanghai Laboratory Animal Center (Shanghai, China) and maintained under specific-pathogen-free (SPF) conditions in the animal facility of SJTUSM. The protocols of animal experiments were approved by the Animal Ethics Committee of SJTUSM, and were performed under the Guide for the Care and Use of Laboratory Animals.
Expression and Purification of Recombinant M.tb Antigens
The recombinant plasmids were obtained from NIH BEI Research Resources Repository (NIAID, NIH), including pMRLB.7 containing rv3875 (Protein ESAT6) (NR-36431), pMRLB.41 containing rv3804c (Protein Ag85A) (NR-13292), pMRLB.46 containing rv3874 (Protein CFP-10) (NR-13297) and pMRLB.54 containing rv3006 (Protein LppZ) (NR-13304) from M.tb. An ESAT6 and CFP-10 fusion gene (E6C10) was prepared through co-cloning into expressing vector pET-28α. Recombinant expressing plasmids were transformed to Escherichia coli BL21 (DE3) strain, and the proteins were induced with isopropyl-β-D-thiogalactoside (IPTG) (Beyotime, Jiangsu, China) for 4 h at 30°C. Bacteria were lysed and the proteins were purified by affinity chromatography with Ni-NTA His-Bind Resin (Merck Millipore, Darmstadt, Germany) as previously described (18). The purity of the proteins was monitored by 12% SDS-PAGE and further confirmed by Western blotting using anti-LppZ or anti-Ag85A mouse polyclonal antibodies and goat anti-mouse IgG antibody (Cwbio, Beijing, China). The protein bands on PVDF membrane (Bio-Rad, Hercules, CA) were visualized by adding ECL solution (Millipore, Boston, MA). The membranes were scanned on a Molecular Imager ChemiDoc XRS+ machine (Bio-Rad). Endotoxins were removed by using Triton X-114 (Sigma Aldrich, St. Louis, MO) two-phase separation as previously described (18). The remaining endotoxin in purified proteins was detected by using Tachyleus Amebocyte Lysate Kits (Gulangyu, Xiamen, China) according to the manufacturer's protocol. To verify the absence of LPS interference in the functional assay, an in vitro stimulation experiment was performed on Raw264.7 cells where the cells were incubated with DMEM medium (GIBCO, Grand Island, NY), LppZ (10 μg/mL) plus Polymyxin B (PMB, 10 μg/mL, Merck), lipopolysaccharide (LPS, 500 ng/mL, Sigma Aldrich), or with LPS plus PMB. After 24 h of incubation, cell culture supernatants were collected, and TNF-α and IL-6 levels were measured by ELISA kits (eBioscience, San Diego, CA).
Murine Air Pouch Model
Dorsal air pouches were induced in mice. In brief, 4 mL sterile-filtered air was injected subcutaneously into the back of C57BL/6 female mice. After 3 days, the pouches were re-inflated with 3 mL sterile air. Three days later 1 mL 0.5% carboxymethylcellulose (CMC) (Sigma Aldrich) mixed with 50 μg LppZ or Ag85A was injected. Injection of 1 mL 0.5% CMC served as a negative control. At 4 h and 24 h post-injection, mice were sacrificed and the pouches were lavaged with 3 mL sterile PBS. The lavage fluid was centrifuged at 486 × g for 8 min at 4°C. Cells and supernatants were collected separately and subjected to further analyses.
Cellular Analysis in the Pouches
Cells collected from the pouches were labeled with FITC-anti-mouse CD11b, PE-anti-mouse F4/80 (both from Biolegend, San Diego, CA) and APC-anti-mouse Ly6G (BD Biosciences, Franklin Lakes, NJ) antibodies, and acquired with a FACS CantoII flow cytometer (BD Biosciences). Data were analyzed by FlowJo software 7.5 (FlOWJO LLC, Treestar Inc., Ashland, OR). Neutrophils were defined as CD11b+Ly6GhiF4/80− and macrophages as CD11b+F4/80hiLy6G−.
Myeloperoxidase Assay
Leukocyte myeloperoxidase (MPO) activity in the exudates was detected by measuring the H2O2-dependent oxidation of 3,3′,5,5′-tetramethylbenzidine (TMB) as described previously (19). Aliquots of 30 μL supernatants were incubated with 120 μL TMB substrate (eBioscience) for 5 min at room temperature. The reaction was stopped by adding 2N H2SO4. The optical density was measured at 450 nm in a PowerWaveXS2 microplate spectrophotometer (BioTek Instruments Inc., VT). All the samples were assayed in duplicate.
Mouse Immunization and M.tb H37Rv Challenge
C57BL/6 female mice (12 mice per group) were immunized subcutaneously with 10 μg LppZ or Ag85A emulsified with adjuvant mixture containing 250 μg dimethyl dioctadecylammonium bromide (DDA) and 25 μg monophosphoryl lipid A (MPL) (both from Sigma Aldrich) in 200 μL PBS three times with 2-weeks intervals as previously described (7). Mice immunized with the adjuvant mixture only were treated as a negative control, while one dose of 5 × 106 BCG Shanghai strain (Shanghai) served as a positive control. Four weeks after the final immunization, mice were subjected to immunological analysis (6 mice per group) or H37Rv challenge (6 mice per group).
For H37Rv challenge, mice were exposed to 100–200 colony-forming units (CFUs) bacteria in an automatic inhalation system (Glas-Col Model #A4212 099c, Terre Haute, IN) in the ABSL-3 animal facility at SHAPHC (7). At 4 weeks post-challenge, mice were sacrificed and the lobes of the lungs were separated for enumerating bacterium CFU, hematoxylin and eosin (H&E) staining, and immunological analysis. More specifically, part of the lungs from infected mice including left lung, right middle and inferior lobes, were homogenized to calculate bacterial numbers as previously described (20). The right post-caval lobes from each mouse were used for H&E staining. The right superior lung lobes were used for immunological analysis. Bacterial loads in the spleens were determined by CFU counting.
Isolation of Human Peripheral Blood Mononuclear Cells (PBMCs)
Heparin anticoagulant human peripheral blood (5–10 mL) was collected. PBMCs were isolated by Ficoll-hypaque density-gradient centrifugation with LymphoprepTM solution (Axis Shield, Oslo, Norway) at 800 × g for 20 min at room temperature. The mononuclear cell layer was collected and washed once with RPMI 1640 culture medium (GIBCO). PBMCs were resuspended in cell culture medium (RPMI 1640 culture medium containing 10% fetal bovine serum (FBS), 100 U/mL penicillin, and 100 μg/mL streptomycin) (all from GIBCO) and subjected to further immunological analysis.
Harvest of Mouse Splenocytes and Pulmonary Immune Cells
The lungs of bacteria-challenged mice were finely minced and digested with 1 mg/mL Collagenase IV (Life Technologies, Waltham, MA) and 200 μg/mL DNase I (Fermantas, Waltham, MA). Single cell suspensions were obtained through 0.15 mm sieves. Pulmonary lymphocytes and splenocytes from immunized mice were isolated by Ficoll-hypaque density gradient centrifugation. Cells were resuspended in mouse cell culture medium (RPMI 1640 culture medium containing 10% FBS, 50 μM 2-mercaptoethanol, 1 mM L-glutamine, 100 U/mL penicillin, and 100 μg/mL streptomycin) for further immunological analysis.
Antigen-Specific Interferon-Gamma (IFN-γ) Release Assay
Antigen-specific IFN-γ release was detected by enzyme-linked immunospot (ELISpot) assay according to the manufacturers' instructions (human IFN-γ ELISpot kit: U-CyTech, Utrecht, Netherlands; mouse IFN-γ ELISpot kit: BD Biosciences). Briefly, 96-well PVDF plates (Millipore) were coated with anti-human or anti-mouse IFN-γ coating antibody overnight at 4°C. Freshly isolated cells (2.5 × 105 in 200 μL culture medium) were incubated with 10 μg/mL M.tb antigen (LppZ, E6C10 fusion protein, Ag85A), tuberculin PPD (Statens Serum Institut, SSI, Copenhagen, Denmark), 5 μg/mL Concanavalin A (ConA) or 2.5 μg/mL phytohemagglutinin (PHA) (both from Sigma Aldrich) for 20 h, respectively. IFN-γ secreting cells were detected by biotin-labeled detection antibody and horseradish peroxidase (HRP) conjugated streptavidin. Coloration was developed with AEC substrate solution for 30 min at room temperature in the dark. The reaction was stopped by thoroughly rinsing the PVDF membrane with demineralized water. The plates were air-dried and the spots were counted by ELISpot Reader (BioReader Model 4000; Bio-Sys GmbH, Karben, Germany). The number of antigen-specific IFN-γ producing cells was calculated as spot-forming units (SFUs) per 2.5 × 105 cells.
Detection of Cytokine Secretion in Mouse Lymphocytes
Freshly isolated splenocytes or pulmonary immune cells were resuspended at a concentration of 1 × 107cells/mL. Cells were stimulated with 10 μg/mL antigens (LppZ and Ag85A) or tuberculin PPD for 20 h or treated with 50 ng/mL phorbol 12-myristate 13-acetate (PMA) and 500 ng/mL Ionomycin (both from Sigma Aldrich) for 5 h. GolgiStop solution (BD Biosciences) was added to cell culture during the last 5 h of ex vivo stimulation. For surface staining, cells were incubated with PE-Cy5-anti-mouse CD3 (eBioscience), Pacific Blue-anti-mouse CD4 (Biolegend), APC-Cy7-anti-mouse CD8 antibodies, biotin-Annexin V and PerCP-streptavidin (BD Biosciences) for 30 min in the dark at 4°C. After washing with PBS containing 2% FBS, cells were fixed and permeated by the Cytofix/Cytoperm reagent from Fixation/Permeabilization kit (BD Biosciences). Cells were incubated with FITC-anti-mouse IFN-γ, PE-anti-mouse IL-2 (both from BD Biosciences), and APC-anti-mouse TNF-α (Biolegend) antibodies for 45 min at 4°C. Cells were washed, resuspended in PBS and acquired with a FACS CantoII flow cytometer (BD Bioscience) in 2 h. Data were analyzed by using FlowJo software 7.5 (Treestar Inc.).
Pathological Analysis of Lungs From H37Rv Infected Mice
The right post-caval lobe of the right lung was fixed in 4% neutral-buffered paraformaldehyde (PFA) solution for 24 h. The tissues were embedded with paraffin and sectioned with a thickness of 5 μm. H&E staining was performed to detect tissue damage, lymphocyte infiltration, and local inflammation in the lungs. Slides were analyzed in a double-blinded fashion by a pathologist using QwinPlus software of Leica microscope (Leica Model DMI3000 M; Leica, Wetzlar, Germany). The score was obtained by measuring the consolidation area of the whole field of vision (amplification 40) and calculated by the following formula: total area of consolidation/area of whole field of vision × 100%. The results were recorded as the mean of at least five fields from each group (n=6).
Cytokine Detection by ELISA
Enzyme-linked immunosorbent assay (ELISA) was performed to detect IL-1β, IL-6, and TNF-α in the supernatants from air pouch lavages according to the manufacturer's instructions (all kits were from eBioscience).
Statistical Analysis
All the data were shown as mean ± SEM. Statistical analyses were performed by using GraphPad Prism 5 software (Graphpad software Inc., CA, USA). Statistical differences were assessed by the unpaired t-test for the data with Gaussian distribution and by Mann-Whitney test for those with non-Gaussian distribution. Unless stated, p < 0.05 was considered statistically significant.
Results
LppZ Displays Strong Antigenicity in Both Onset and Retreated Active TB Patients Which Is Comparable to ESAT6 and CFP-10
To verify the high antigenicity of LppZ in active TB patients (7, 21), LppZ was expressed prokaryotically and purified by affinity chromatography followed by the removal of endotoxin. The purity of LppZ was determined by Coomassie blue staining and validated by Western blotting using anti-6 × His tag antibody, and mouse and rabbit anti-LppZ polyclonal antibodies (Supplementary Figure 1). The numbers of LppZ-specific IFN-γ producing cells in the periphery of TB patients and healthy controls (HCs) were detected by an ELISpot assay upon stimulation with purified LppZ (22) (Supplementary Figure 2). A fusion protein of ESAT6 and CFP-10 (E6C10) was used to determine M.tb-specific immune status in TB patients, while PHA was used as a positive control (Figure 1A). Our results showed that both initial and retreated TB patients had significantly higher responsiveness than the HCs specific to LppZ. They had more IFN-γ producing cells, which was similar to the differential responses to E6C10 (Figure 1A). Correlation analysis revealed that TB patients responded to LppZ in a manner parallel to E6C10 (Figure 1B). Since LppZ induced strong IFN-γ production similar to E6C10 in the active TB patients, receiver operating characteristic (ROC) curve analysis was performed to evaluate the diagnostic potential of LppZ-specific IFN-γ production in TB. It was obvious that LppZ-induced cellular response was able to efficiently discriminate TB patients from HCs with the area under the curve of ROC (AUC) value of 0.8739. The sensitivity and specificity were 50% and 97.92%, respectively (Figure 1C), which was similar to the results with E6C10 (Figure 1D). LPS interference in LppZ protein stimulation was excluded (Supplementary Figure 3).These results revealed that LppZ had high antigenicity for cellular immunity in active TB patients.
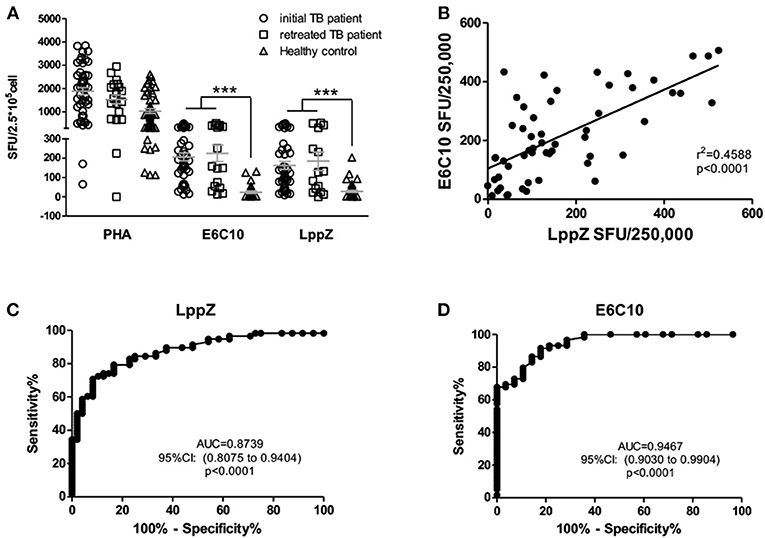
Figure 1. LppZ induces high cellular responsiveness in TB patients. (A) The numbers of IFN-γ producing cells in TB patients (including initial TB patients and retreated TB patients) and HCs were assessed by ELISpot assay after stimulation with E6C10 fusion protein or purified LppZ protein. PHA served as a positive control. (B) Correlation analyses between LppZ- and E6C10-specific IFN-γ producing cells. (C,D) ROC curve analyses of LppZ- (C) and E6C10-specific (D) IFN-γ-producing cells in distinguishing TB patients and HCs. ***p < 0.0001.
LppZ Triggers Proinflammatory Responses in a Mouse Air Pouch Model
To explore the regulation of strong cellular immune responses by LppZ, a mouse air pouch model (Supplementary Figure 4) was applied to recapitulate the innate immunity initiated at the early stage upon M.tb infection. Sterile-filtered air (4 mL) was injected subcutaneously to mechanically disrupt the connective tissues for the formation of the pouches. This facilitated the infiltration of immune cells. By injecting target antigens into the pouches to trigger the host innate response the characteristics of the subsequent inflammation could be further investigated (23–25). As shown in Figure 2A, injection of LppZ in 0.5% CMC drove a dramatically greater accumulation of inflammatory cells into the pouches when compared to the CMC-only group. Interestingly, injection of Ag85A in 0.5% CMC induced moderate cell infiltration inside the pouches, much less than when LppZ was used.
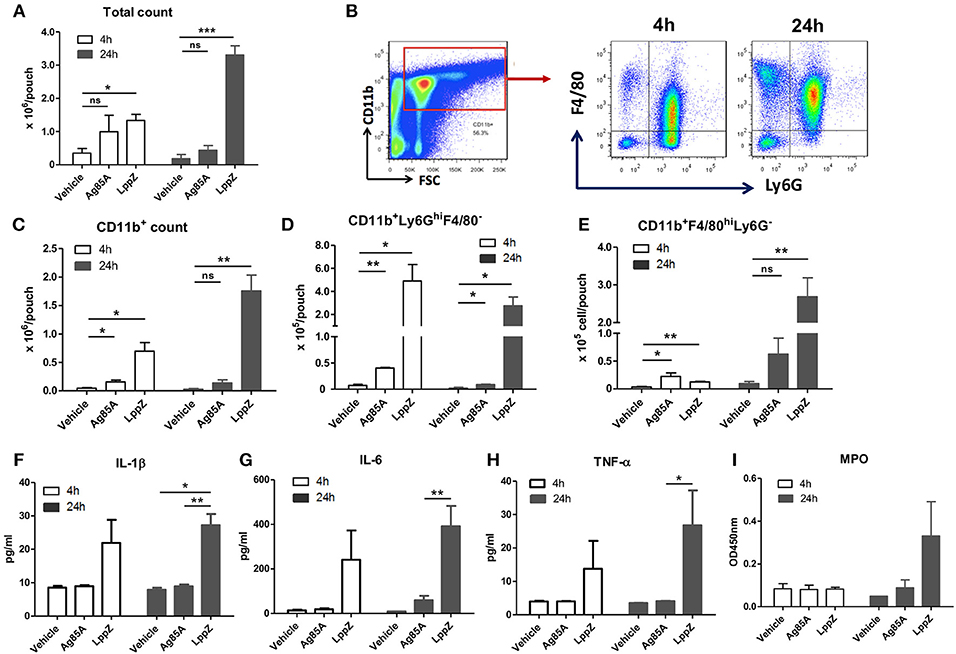
Figure 2. LppZ recruits innate cells and induces pro-inflammatory cytokine production in the murine air pouch model. After injecting CMC (vehicle), LppZ (LppZ) or Ag85A (Ag85A) recombinant protein in the pouches, mice were sacrificed at 4 h and 24 h. Cells in the pouches were collected. (A) Total cell numbers recruited in the pouches. (B) Representative graphs showing the gating strategy for analysis of infiltrating cell subsets. The absolute numbers of CD11b+ cells (C), CD11b+Ly6GhiF4/80− neutrophils (D) and CD11b+Ly6G−F4/80+ macrophages (E) were analyzed by flow cytometry. The levels of IL-1β (F), IL-6 (G), TNF-α (H), and myeloperoxidase (MPO) (I) were measured in the exudates (n = 3–6 mice per group). *p < 0.05, **p < 0.01, ***p < 0.001.
The types of immune cells recruited in the pouches were further defined (Figure 2B). LppZ injection led to the increased infiltration of CD11b+ myeloid cells when compared to other groups (Figure 2C). More precisely, at 4 h the CD11b+Ly6GhiF4/80− neutrophils were much more abundant than the CD11b+Ly6G−F4/80hi macrophages in both LppZ- and Ag85A-treated pouches whereas the absolute numbers of both cells were increased more in LppZ-treated pouches than in Ag85A-treated pouches (Figures 2D,E, white bar). At 24 h after either LppZ or Ag85A treatment the absolute number of the macrophages was increased whereas that of the neutrophils in the pouches decreased. Although Ag85A treatment induced macrophage accumulation in the pouches, the increase in macrophages was more dramatic in LppZ-injected than in Ag85A-injected pouches (Figures 2D,E, black bar). Few neutrophils and macrophages were detectable in vehicle-treated groups. Furthermore, when key pro-inflammatory cytokines were measured in the exudates of the pouches there were significantly higher levels of IL-1β, IL-6 and TNF-α upon LppZ treatment when compared to vehicle or Ag85A-treated groups. Interestingly, no dramatic difference in pro-inflammatory cytokines was observed between vehicle- and Ag85A-injected pouches (Figures 2F–H). Myeloperoxidase (MPO) is one of the key enzymes released by inflammatory cells during inflammation and, consistent with the dramatic recruitment of inflammatory cells and the augmented pro-inflammatory cytokine release in the pouches upon LppZ injection, the MPO level also increased significantly in LppZ-induced exudates at 24 h whereas vehicle or Ag85A treatment induced little MPO in the pouches (Figure 2I). Thus, these results strongly suggested that LppZ triggers inflammatory responses largely through the recruitment of inflammatory cells and the induction of pro-inflammatory cytokine production.
LppZ Induces Strong Th1 Responses With Enhanced T Cell Multi-Functionality in Immunized Mice
M.tb-specific Th1 immune responses are considered to play critical roles in the protection against TB (26). The ability of LppZ to trigger efficient T cell responses was further investigated. C57BL/6 mice were immunized with LppZ and Ag85A was used as an experimental control (Supplementary Figure 5a). Subsequent analysis of antigen-specific cellular responses in the spleens showed that LppZ was able to induce the generation of antigen-specific IFN-γ producing cells (Figure 3A, black bar), to a degree similar to Ag85A immunization (Figure 3A, twill bar).
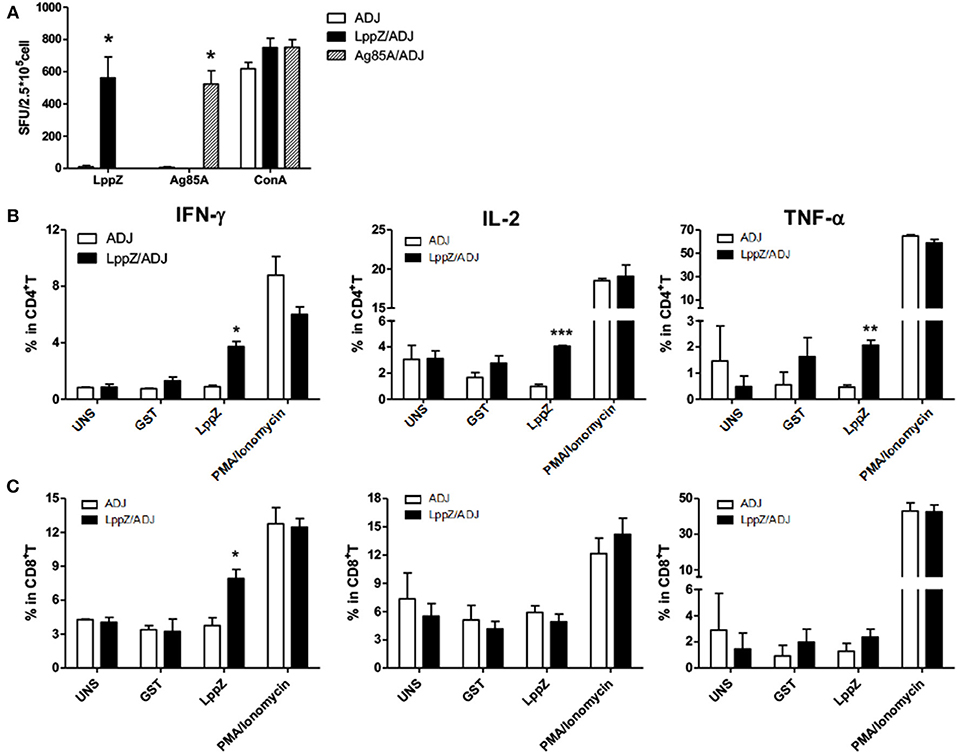
Figure 3. LppZ evokes increased IFN-γ and TNF-α release from splenocytes of immunized mice. (A) C57BL/6 female mice (6–8 weeks old) were immunized with LppZ (LppZ/ADJ) or Ag85A (Ag85A/ADJ) recombinant proteins emulsified with adjuvant, or adjuvant only (ADJ). Splenocytes from different immunization group were collected. For ex vivo stimulation, 2.5 × 105 cells were incubated with LppZ (10 μg/mL) or Ag85A (10 μg/mL) for 20 h. Antigen-specific IFN-γ-producing cells were measured by ELISpot assay. ConA was treated as a positive control. (B,C) 5 × 105 cells were incubated with LppZ (10 μg/mL) for 20 h. Antigen-specific IFN-γ, TNF-α, and IL-2 producing CD4+ (B) or CD8+ (C) T cells from the ADJ or LppZ/ADJ group were measured by flow cytometry. PMA/Ionomycin was a positive control and GST was an irrelevant control. UNS: without ex vivo antigen stimulation. *p < 0.05, **p < 0.01, ***p < 0.001.
T cells from the spleens of LppZ immunized mice were further defined as functional subsets based on antigen-specific cytokine release. No significant differences were observed in the absolute numbers of CD4+ or CD8+ T cells with or without LppZ immunization (data not shown). However, LppZ could trigger antigen-specific CD4+ and CD8+ T cell responses after immunization: more LppZ-specific Th1-type CD4+ T cells that released cytokines IFN-γ, IL-2, and TNF-α were induced in LppZ-immunized mice when compared to those in the ADJ group (Figure 3B). LppZ evoked the generation of more IFN-γ releasing CD8+ T cells than ADJ did alone (Figure 3C). There were no significant differences between LppZ- and ADJ-injected groups in the frequencies of cytokine-releasing T cells upon stimulation with GST (an irrelevant control to exclude non-specific responses induced by trace contaminants in E.coli-derived proteins) or upon PMA/Ionomycin stimulation (positive control).
Antigen-specific polyfunctional T cells are those releasing two or three cytokines simultaneously. They are considered to be the main fighters against M.tb infection (27, 28). LppZ-specific polyfunctional T cells were compared between LppZ-immunized and control mice. It was obvious that more IFN-γ+IL-2+TNF-α+ CD4+ T cells and IFN-γ+TNF-α+ CD4+ T cells were detectable in LppZ-immunized mice than in the ADJ group. In addition, CD4+ T cells producing either IFN-γ or IL-2 alone were increased more by the LppZ vaccination (Figure 4A). Similarly, IFN-γ+CD8+ T cells were induced more by LppZ treatment when compared to ADJ group (Figure 4C). However, in ADJ- and LppZ-treated mice, the percentages of single, double and triple cytokine-producing cells were similar both in CD4+ (Figure 4B) and CD8+ T cells (Figure 4D). In addition, equivalent levels of single or double cytokine releasing cells were induced upon PMA/Ionomycin stimulation in both CD4+ and CD8+ T cells in the two groups (Figures 4E,F).
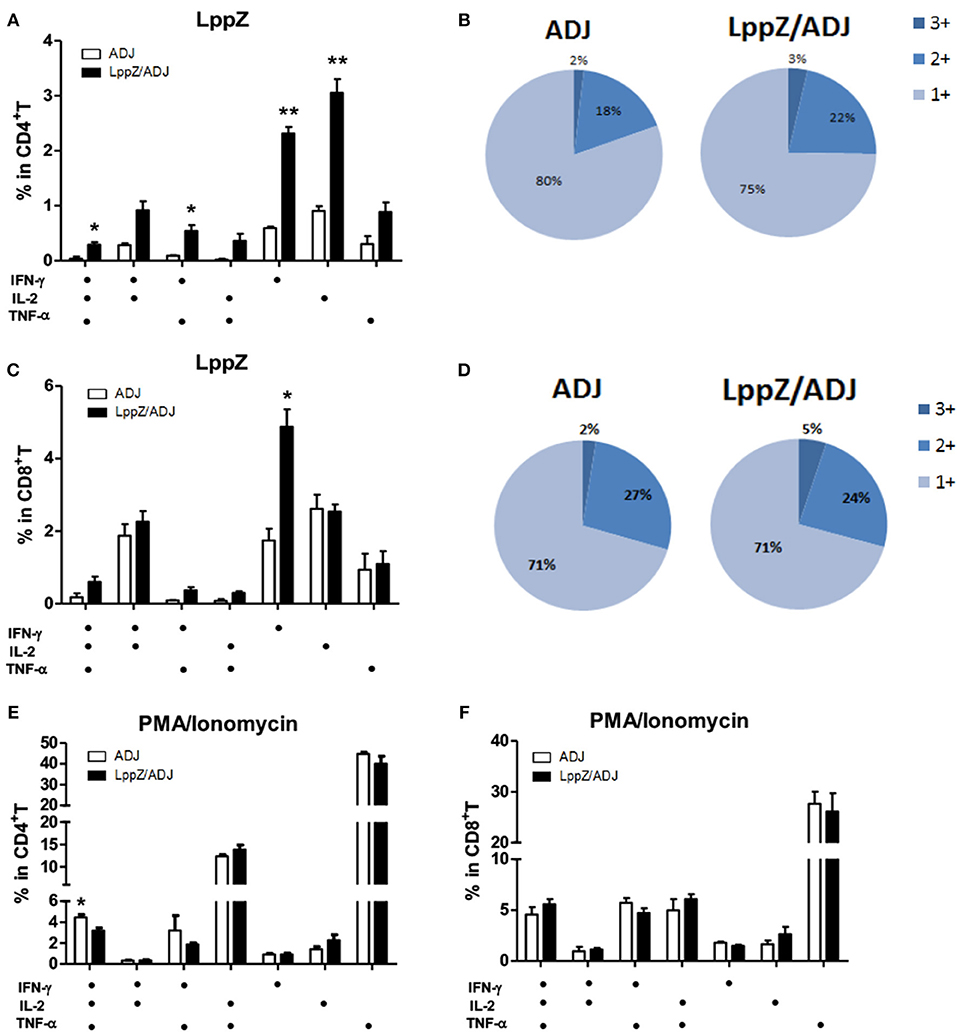
Figure 4. Polyfunctionality of CD4+ or CD8+ T cells responding to LppZ in immunized mice. Splenocytes from each mouse were collected and stimulated ex vivo with LppZ or PMA/Ionomycin. Frequencies of antigen-specific CD4+ (A) or CD8+ (C) T cells expressing single and multiple cytokines were analyzed by flow cytometry. Single or multiple cytokine production is shown on the x-axis of the bar chart (n = 3–6 mice per group). The percentages of CD4+ (B) and CD8+ (D) T cell expressing single (1+), double (2+) or triple (3+) cytokines are represented as pie charts. (E,F): frequencies of cytokine-producing CD4+ (E) or CD8+ (F) T cells upon PMA/Ionomycin stimulation *p < 0.05, **p < 0.01.
LppZ Immunization Protects Lungs From Injury With Less Bacilli Load
Four weeks after LppZ, Ag85A or BCG immunization, a low-dose aerosol inhalation challenge (100–200 CFUs per mice) with virulent M.tb H37Rv strain was used to determine the protective efficacy against infection (Supplementary Figure 5b). Four weeks after the challenge, the ADJ group presented the highest bacterial load in both lungs (Log10CFU = 5.002 ± 0.137) and spleens (Log10CFU = 3.154 ± 0.393), whereas BCG vaccination strongly reduced bacterial burdens developed in the two organs (Lung: Log10CFU = 4.077 ± 0.077; Spleen: Log10CFU = 1.939 ± 0.220). Notably, LppZ-immunized mice showed diminished bacterial loads in the lungs (Log10CFU = 4.498 ± 0.133) and spleens (Log10CFU = 2.082 ± 0.286) when compared to the ADJ group, an effect similar to that of Ag85A immunization (Lung: Log10CFU = 4.538 ± 0.053; Spleen: Log10CFU = 2.141 ± 0.290) and BCG vaccination (Figures 5A,B). Lung pathology of the challenged mice was also evaluated. Associated with the low bacilli loads in the lungs in LppZ-immunized mice, there was a less-pronounced lung inflammation characterized by less infiltration of immune cells in local regions and less alveolar tissue damage. In contrast, mice from the ADJ group showed much more severe inflammation (Figure 5C, arrow). LppZ-immunized mice exhibited the lowest pathological score in the lungs among the four groups based on H&E staining (Figure 5D). Our results thus demonstrated that LppZ immunization gave efficient protection against M.tb infection with less tissue damage.
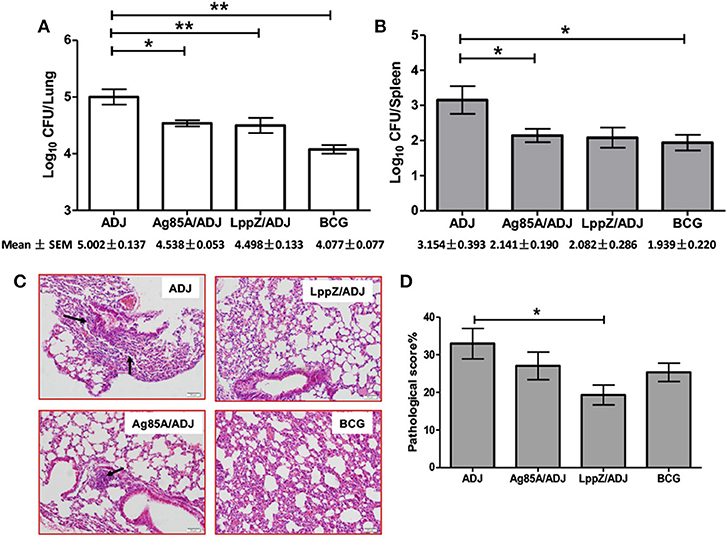
Figure 5. Bacteria load and lung histopathology after aerosol infection with virulent H37Rv strain. Six C57BL/6 female mice were immunized with purified Ag85A (Ag85A/ADJ) or LppZ (LppZ/ADJ) recombinant protein emulsified with adjuvant. Adjuvant only (ADJ) and BCG (BCG) immunization were used as controls. Four weeks after the last immunization, mice were challenged with the virulent M.tb strain H37Rv. Bacteria load in the lungs (A) and spleens (B) were enumerated and represented as the mean log10 CFU/organ ± SEM. Lung pathological scores (C) and representative pathological changes based on H&E staining (scale bar = 50 μm) (D) are indicated. *p < 0.05, **p < 0.01.
More LppZ-Specific Polyfunctional T Cell Responses Are Present in the Lungs After H37Rv Challenge in LppZ Immunized Mice
Regional immune responses play important roles in the protection against M.tb infection (29). One lobe from the lungs of each immunized mouse after bacteria challenge was pooled and LppZ-specific T cell functionality was analyzed. Interestingly, LppZ induced different cytokine releasing patterns when compared to Ag85A. More LppZ-specific IL-2 and TNF-α producing CD4+ T cells were present in the lungs of the challenged LppZ-immunized mice when compared to the ADJ group, whereas Ag85A immunization led to more accumulation of Ag85A-specific IFN-γ+, IL-2+, and TNF-α+ CD4+ T cells in the lungs (Figure 6A). An increase in IFN-γ production in CD8+ T cells also was greater in both LppZ- and Ag85A-immunized mice than in the ADJ group (Figure 6B).
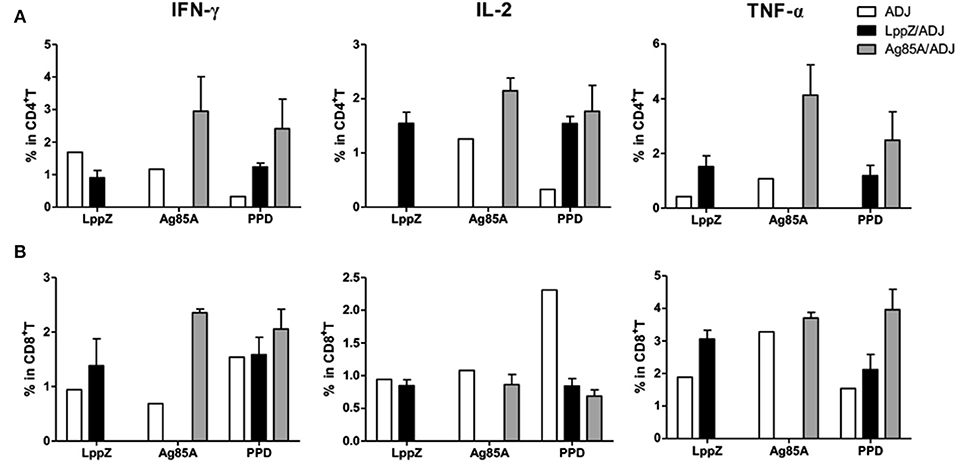
Figure 6. M.tb antigen-specific Th1-type cytokine production in CD4+ or CD8+ T cells from the lungs of virulent H37Rv-challenged mice. Four weeks after bacteria challenge, mice were sacrificed and lymphocytes from the lungs were collected. For ex vivo stimulation, 5 × 105 cells from different groups (ADJ, LppZ/ADJ or Ag85A/ADJ) were incubated with 10 μg/mL LppZ, Ag85A or PPD respectively, for 20 h. Intracellular cytokine profile for IFN-γ, TNF-α and IL-2 in individual cells from different groups were measured by flow cytometry of CD4+ (A) or CD8+ (B) T cells.
Antigen-specific polyfunctional T cells in the lungs of the H37Rv-challenged mice were also analyzed. LppZ immunization significantly increased the frequencies of multiple cytokine-releasing CD4+ and CD8+ T cells. In contrast, in challenged mice that had received ADJ alone, all LppZ-specific T cells produced single cytokines (Figures 7A,D). In challenged Ag85A-immunized mice, both Ag85A-specific double and triple cytokine releasing CD4+ and CD8+ T cells were more abundant in the lungs than in the ADJ control mice (Figures 7B,E). PPD-specific cellular immune responses, either CD4+ or CD8+ T cells, in the lungs after bacteria challenge were also compared between LppZ and Ag85A immunized mice. The frequencies of PPD-specific polyfunctional T cells in challenged LppZ- and Ag85A-immunized mice were higher than in ADJ control mice and were similar between the two groups (Figures 7C,F). These results revealed that LppZ immunization generated regional antigen-specific T cell immunity after bacterial challenge, which was similar to Ag85A immunization.
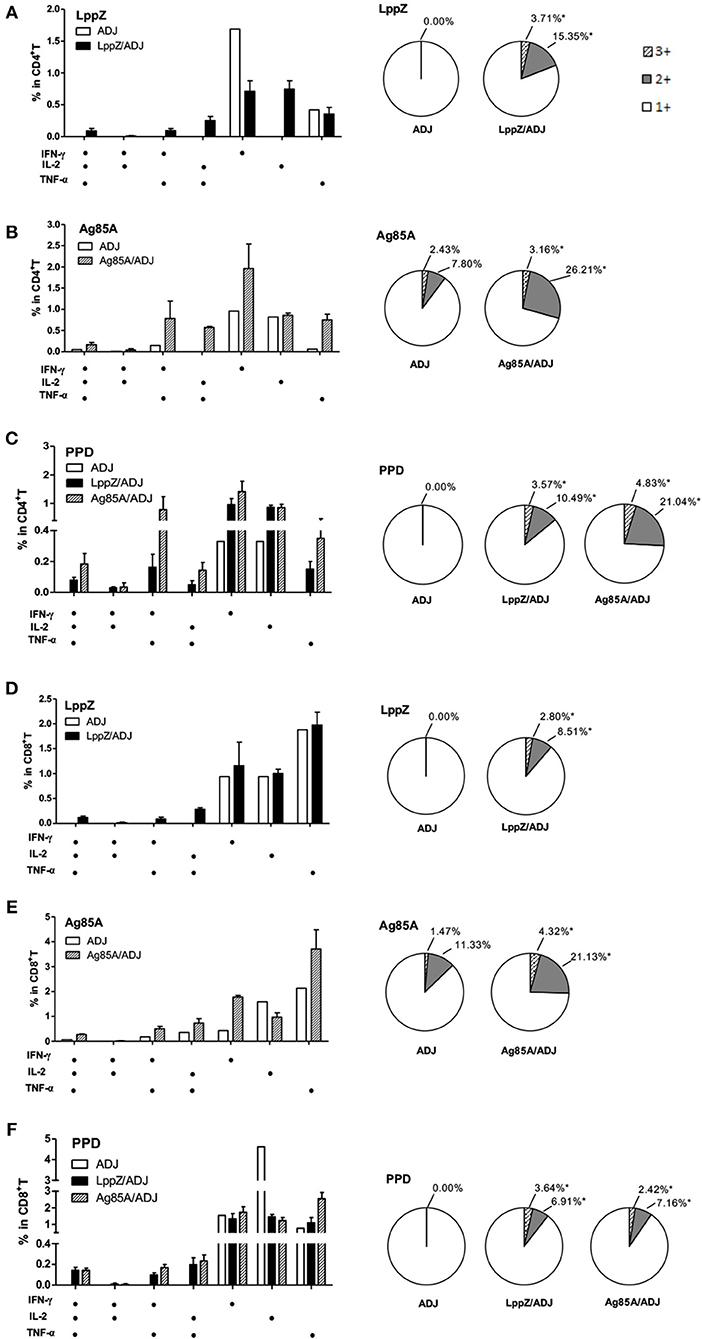
Figure 7. Polyfunctional CD4+ and CD8+ T cell distribution in the lungs of the immunized mice after H37Rv challenge. Pulmonary lymphocytes from different groups (ADJ, LppZ/ADJ or Ag85A/ADJ) were collected and stimulated ex vivo with LppZ (A,D), Ag85A (B,E) or PPD (C,F). Frequencies of antigen-specific CD4+ or CD8+ T cells producing single or multiple cytokines were analyzed by flow cytometry. Single or multiple cytokine production was shown on the x-axis of the bar chart. The percentages of T cells expressing single (1+), double (2+) or triple (3+) cytokines were represented as pie charts. *p < 0.05.
Discussion
Because of the declining protection from BCG immunization in adult populations, the prime-boost strategy with a subunit vaccine is probably appropriate and calls for the identification of new candidate antigens (30–32). We propose LppZ as such a candidate. Owing to their cellular distribution and secreted nature, the LppZ lipoprotein family mediates bacterial interactions with the hosts through cell adhesion, invasion and transmembrane signaling (33). As investigated here, LppZ exhibited strong immunoreactivity in cellular responses in addition to the high humoral IgA responses in TB patients that were reported previously (21), confirming its dominant antigenicity among mycobacterial antigens.
Several lipoproteins previously have been previously reported to be involved in immunosuppressive processes. For instance, LpqH (19-kDa antigen), LprG and LprI exerted an immunosuppressive function at the innate immunity stage through toll-like receptors (34–39). This might help bacteria to escape from the initial immune surveillance. On the contrary, we have observed in this study that LppZ induced more dramatic innate immunity in the air pouch model with rapid recruitment of neutrophils at 4 h and the accumulation of macrophages and neutrophils in the pouches at 24 h. In the innate immune responses that follow M.tb infection, neutrophils and macrophages are the main components possessing strong phagocytosis properties and they occur earlier than T cell immunity. Rapid recruitment of neutrophils is the very first event in the fight against bacterial pathogens. They are responsible for the initiation of necrosis within the granuloma (40), and undergo killing processes and degranulation in response to M.tb infection. However, macrophages are considered to be the component to restrict bacilli survival and proliferation with more efficacy (41). What is more, alveolar macrophages, constituting the majority of cells collected from bronchoalveolar lavage, exert anti-inflammatory functions to control infection in the lung (21). Considering the membrane localization of LppZ in the bacteria, the presence of LppZ probably favors the elimination of bacteria through inducing a robust innate immunity once the bacteria encounter innate cells.
It is widely accepted that M.tb-specific T cell immunity is crucial for the protection against M.tb infection, especially polyfunctional T cells with the capacity for producing multiple cytokines (28, 42, 43). On the one hand, IFN-γ is one of the key cytokines produced by CD4+ T cells during M.tb infection. Subsequent activation of macrophages by IFN-γ is involved in the protection against TB (44–46). The anti-mycobacterial function of CD8+ T cells also depends on IFN-γ (47). TNF-α, on the other hand, can stimulate the production of reactive nitrogen intermediates (RNIs), restrict mycobacterial growth within macrophages and prevent necrosis of macrophages (48, 49). IL-2 tends to promote the proliferation and maturation of T cells and plays an important role in the control of M.tb infection as well (20, 31, 42, 43). In the present work, mice immunized with LppZ exhibited a strong capacity to generate antigen-specific Th1 immune responses, which was largely consistent with efficient protection upon M.tb challenge. More interestingly, LppZ immunization exerted protection against M.tb challenge similar to BCG or Ag85A immunization but with less pulmonary damage present in the lungs (Figure 5). Analyzing the immunological patterns, it was apparent that the frequencies of polyfunctional CD4+ and CD8+ T cells were moderate in LppZ-immunized mice after challenge when compared to BCG or Ag85A immunization (Figure 7). Similarly, the generation of mono-cytokine producing CD4+ and CD8+ T cells was also less pronounced (Figure 6) suggesting that while the induction of Th1 immunity by LppZ immunization protects against M.tb infection it mediates moderate inflammatory reactions. The protective efficacy together with the low pulmonary injury seen after the challenge of LppZ-immunized mice supports the further development of LppZ as one of the antigens in subunit booster vaccines in the future.
Cumulatively, we have reported in this study that LppZ showed strong immunogenicity during M.tb infection in humans as well as in mice. In mice, it was apparently able to induce innate and adaptive immunity and exerted efficient protection against M.tb infection similar to the current BCG vaccine. Considering the difficulty in substituting recombinant BCG in countries that are using BCG as primary vaccination and the limitations of the candidate antigens for booster vaccine development, further studies on LppZ-mediated protection in prime-boost animal models are warranted.
Ethics Statement
This study was carried out in accordance with the recommendations of the Ethical Committee of Shanghai Jiao Tong University School of Medicine with written informed consent from all subjects. All subjects gave written informed consent in accordance with the Declaration of Helsinki. The protocol was approved by the Ethical Committee of Shanghai Jiao Tong University School of Medicine.
Author Contributions
YW, XF, HS, and GZ: conceived the project. YC, XF, and YW: designed experiments. YC, JX, YLi, Y-jX, YLiu, SW, and PJ: performed experiments. Y-qX and SL: collected samples and clinical data. YC, JX, YLi, Y-jX, XF, and YW: analyzed data. YC, JX, Y-jX, and YW: wrote the manuscript. YC, JX, SL, XF, and YW: Obtained funding. GZ, HS, and SL are senior authors.
Funding
This work was supported by grants from Chinese National Mega Science and Technology Program on Infectious Diseases (2018ZX10731301, 2018ZX10302301, and 2013ZX10003007), Shanghai Academic Research Leader Project (2018XD1403300), the National Natural Science Foundation of China (81501361, 81873868, and 31771004), the Fundamental Research Funds for the Central Universities (17X100040012) and Doctoral Innovation Fund Projects from Shanghai Jiao Tong University School of Medicine (BXJ201803).
Conflict of Interest Statement
The authors declare that the research was conducted in the absence of any commercial or financial relationships that could be construed as a potential conflict of interest.
Acknowledgments
We acknowledge Prof. Zhao-jun Wang and Chen-yun Wu (Shanghai Jiao Tong University School of Medicine) for the help of the establishment of the murine air pouch model. We also acknowledge Prof. Bing Su from our institute as well as adjunct professor of Yale University, and Dr. Douglas B. Lowrie from SHAPHC for editing the manuscript.
Supplementary Material
The Supplementary Material for this article can be found online at: https://www.frontiersin.org/articles/10.3389/fimmu.2018.03190/full#supplementary-material
References
2. Behr MA, Wilson MA, Gill WP, Salamon H, Schoolnik GK, Rane S, et al. Comparative genomics of BCG vaccines by whole-genome DNA microarray. Science (1999) 284:1520–3. doi: 10.1126/science.284.5419.1520
4. Frick M. The Tuberculosis Vaccine Pipelines: A New Path to the Same Destination? HIV, HCV & TB Pipeline Report. Treatment Action Group (2015).
5. Huygen K, Lozes E, Gilles B, Drowart A, Palfliet K, Jurion F, et al. Mapping of TH1 helper T-cell epitopes on major secreted mycobacterial antigen 85A in mice infected with live Mycobacterium bovis BCG. Infect Immun. (1994) 62:363–70.
6. Launois P, DeLeys R, Niang MN, Drowart A, Andrien M, Dierckx P, et al. T-cell-epitope mapping of the major secreted mycobacterial antigen Ag85A in tuberculosis and leprosy. Infect Immun. (1994) 62:3679–87.
7. Xiao Y, Sha W, Tian Z, Chen Y, Ji P, Sun Q, et al. Adenylate kinase: a novel antigen for immunodiagnosis and subunit vaccine against tuberculosis. J Mol Med. (2016) 94:823–34. doi: 10.1007/s00109-016-1392-5
8. Kong H, Dong C, Xiong S. A novel vaccine p846 encoding Rv3615c, Mtb10.4, and Rv2660c elicits robust immune response and alleviates lung injury induced by Mycobacterium infection. Hum Vaccin Immunother. (2014) 10:378–90. doi: 10.4161/hv.27121
9. Wang X, Zhang J, Liang J, Zhang Y, Teng X, Yuan X, et al. Protection against Mycobacterium tuberculosis infection offered by a new multistage subunit vaccine correlates with increased number of IFN-gamma+ IL-2+ CD4+ and IFN-gamma+ CD8+ T cells. PLoS ONE (2015) 10:e0122560. doi: 10.1371/journal.pone.0122560
10. Liu W, Li J, Niu H, Lin X, Li R, Wang Y, et al. Immunogenicity and protective efficacy of multistage vaccine candidates (Mtb8.4-HspX and HspX-Mtb8.4) against Mycobacterium tuberculosis infection in mice. Int Immunopharmacol. (2017) 53:83–9. doi: 10.1016/j.intimp.2017.10.015
11. Van Der Meeren O, Hatherill M, Nduba V, Wilkinson RJ, Muyoyeta M, Van Brakel E, et al. Phase 2b controlled trial of M72/AS01E vaccine to prevent tuberculosis. N Engl J Med. (2018) 379:1621–34. doi: 10.1056/NEJMoa1803484
12. Sutcliffe IC, Harrington DJ. Lipoproteins of Mycobacterium tuberculosis: an abundant and functionally diverse class of cell envelope components. FEMS Microbiol Rev. (2004) 28:645–59. doi: 10.1016/j.femsre.2004.06.002
13. Xiong Y, Chalmers MJ, Gao FP, Cross TA, Marshall AG. Identification of Mycobacterium tuberculosis H37Rv integral membrane proteins by one-dimensional gel electrophoresis and liquid chromatography electrospray ionization tandem mass spectrometry. J Proteome Res. (2005) 4:855–61. doi: 10.1021/pr0500049
14. Malen H, Berven FS, Fladmark KE, Wiker HG. Comprehensive analysis of exported proteins from Mycobacterium tuberculosis H37Rv. Proteomics (2007) 7:1702–18. doi: 10.1002/pmic.200600853
15. de Souza GA, Leversen NA, Malen H, Wiker HG. Bacterial proteins with cleaved or uncleaved signal peptides of the general secretory pathway. J Proteomics (2011) 75:502–10. doi: 10.1016/j.jprot.2011.08.016
16. Malen H, Softeland T, Wiker HG. Antigen analysis of Mycobacterium tuberculosis H37Rv culture filtrate proteins. Scand J Immunol. (2008) 67:245–52. doi: 10.1111/j.1365-3083.2007.02064.x
17. Sartain MJ, Slayden RA, Singh KK, Laal S, Belisle JT. Disease state differentiation and identification of tuberculosis biomarkers via native antigen array profiling. Mol Cell Proteomics (2006) 5:2102–13. doi: 10.1074/mcp.M600089-MCP200
18. Yang X, Wu JB, Liu Y, Xiong Y, Ji P, Wang SJ, et al. Identification of mycobacterial bacterioferritin B for immune screening of tuberculosis and latent tuberculosis infection. Tuberculosis (2017) 107:119–25. doi: 10.1016/j.tube.2017.08.005
19. Maione F, Paschalidis N, Mascolo N, Dufton N, Perretti M, D'Acquisto F. Interleukin 17 sustains rather than induces inflammation. Biochem Pharmacol. (2009) 77:878–87. doi: 10.1016/j.bcp.2008.11.011
20. Kang H, Yuan Q, Ma H, Hu ZD, Han DP, Wu K, et al. Enhanced protective efficacy against Mycobacterium tuberculosis afforded by BCG prime-DNA boost regimen in an early challenge mouse model is associated with increased splenic interleukin-2-producing CD4 T-cell frequency post-vaccination. Immunology (2014) 143:661–9. doi: 10.1111/imm.12348
21. Xiao J, Xiong Y, Chen Y, Xiao Y, Ji P, Li Y, et al. Determination of lipoprotein Z-specific IgA in tuberculosis and latent tuberculosis infection. Front Cell Infect Microbiol. (2017) 7:495. doi: 10.3389/fcimb.2017.00495
22. Lalvani A, Pathan AA, McShane H, Wilkinson RJ, Latif M, Conlon CP, et al. Rapid detection of Mycobacterium tuberculosis infection by enumeration of antigen-specific T cells. Am J Respir Crit Care Med. (2001) 163:824–8. doi: 10.1164/ajrccm.163.4.2009100
23. Yoshino S, Bacon PA, Blake DR, Scott DL, Wainwright AC, Walton KW. A model of persistent antigen-induced chronic inflammation in the rat air pouch. Br J Exp Pathol. (1984) 65:201–14.
24. Bower MA, Constant SL, Mendez S. Necator americanus: the Na-ASP-2 protein secreted by the infective larvae induces neutrophil recruitment in vivo and in vitro. Exp Parasitol. (2008) 118:569–75. doi: 10.1016/j.exppara.2007.11.014
25. Wu C, Chen Q, Fang Y, Wu J, Han Y, Wang Y, et al. Schistosoma japonicum egg specific protein SjE16.7 recruits neutrophils and induces inflammatory hepatic granuloma initiation. PLoS Negl Trop Dis. (2014) 8:e2703. doi: 10.1371/journal.pntd.0002703
26. Ottenhoff TH, Kaufmann SH. Vaccines against tuberculosis: where are we and where do we need to go? PLoS Pathog. (2012) 8:e1002607. doi: 10.1371/journal.ppat.1002607
27. Behar SM. Antigen-specific CD8(+) T cells and protective immunity to tuberculosis. Adv Exp Med Biol. (2013) 783:141–63. doi: 10.1007/978-1-4614-6111-1_8
28. Lewinsohn DA, Lewinsohn DM, Scriba TJ. Polyfunctional CD4(+) T cells as targets for tuberculosis vaccination. Front Immunol. (2017) 8:1262. doi: 10.3389/fimmu.2017.01262
29. Schwander S, Dheda K. Human lung immunity against Mycobacterium tuberculosis: insights into pathogenesis and protection. Am J Respir Crit Care Med. (2011) 183:696–707. doi: 10.1164/rccm.201006-0963PP
30. Dalmia N, Ramsay AJ. Prime-boost approaches to tuberculosis vaccine development. Expert Rev Vaccines (2012) 11:1221–33. doi: 10.1586/erv.12.94
31. Hu Z, Wong KW, Zhao HM, Wen HL, Ji P, Ma H, et al. Sendai virus mucosal vaccination Establishes lung-resident memory CD8 T cell immunity and boosts BCG-primed protection against TB in mice. Mol Ther. (2017) 25:1222–33. doi: 10.1016/j.ymthe.2017.02.018
32. Nieuwenhuizen NE, Kaufmann SHE. Next-generation vaccines based on bacille calmette-guerin. Front Immunol. (2018) 9:121. doi: 10.3389/fimmu.2018.00121
33. Sutcliffe IC, Russell RR. Lipoproteins of gram-positive bacteria. J Bacteriol. (1995) 177:1123–8.
34. Garbe T, Harris D, Vordermeier M, Lathigra R, Ivanyi J, Young D. Expression of the Mycobacterium tuberculosis 19-kilodalton antigen in Mycobacterium smegmatis: immunological analysis and evidence of glycosylation. Infect Immun. (1993) 61:260–7.
35. Lopez M, Sly LM, Luu Y, Young D, Cooper H, Reiner NE. The 19-kDa Mycobacterium tuberculosis protein induces macrophage apoptosis through Toll-like receptor-2. J Immunol. (2003) 170:2409–16. doi: 10.4049/jimmunol.170.5.2409
36. Gehring AJ, Dobos KM, Belisle JT, Harding CV, Boom WH. Mycobacterium tuberculosis LprG (Rv1411c): a novel TLR-2 ligand that inhibits human macrophage class II MHC antigen processing. J Immunol. (2004) 173:2660–8. doi: 10.4049/jimmunol.173.4.2660
37. Sanchez A, Espinosa P, Garcia T, Mancilla R. The 19 kDa Mycobacterium tuberculosis lipoprotein (LpqH) induces macrophage apoptosis through extrinsic and intrinsic pathways: a role for the mitochondrial apoptosis-inducing factor. Clin Dev Immunol. (2012) 2012:950503. doi: 10.1155/2012/950503
38. Gaur RL, Ren K, Blumenthal A, Bhamidi S, Gonzalez-Nilo FD, Jackson M, et al. LprG-mediated surface expression of lipoarabinomannan is essential for virulence of Mycobacterium tuberculosis. PLoS Pathog. (2014) 10:e1004376. doi: 10.1371/journal.ppat.1004376
39. Sethi D, Mahajan S, Singh C, Lama A, Hade MD, Gupta P, et al. Lipoprotein LprI of Mycobacterium tuberculosis acts as a lysozyme inhibitor. J Biol Chem. (2016) 291:2938–53. doi: 10.1074/jbc.M115.662593
40. Turner O, Basaraba R, Frank A, Orme IM. Granuloma formation in mouse and guinea pig models of experimental tuberculosis. In: D. Boros, editor. Granulomatous Infections and Inflammation: Cellular and Molecular Mechanisms. Washigton, DC: ASM Press (2003).
41. Weiss G, Schaible UE. Macrophage defense mechanisms against intracellular bacteria. Immunol Rev. (2015) 264:182–203. doi: 10.1111/imr.12266
42. Scriba TJ, Tameris M, Mansoor N, Smit E, van der Merwe L, Isaacs F, et al. Modified vaccinia Ankara-expressing Ag85A, a novel tuberculosis vaccine, is safe in adolescents and children, and induces polyfunctional CD4+ T cells. Eur J Immunol. (2010) 40:279–90. doi: 10.1002/eji.200939754
43. Leroux-Roels I, Forgus S, De Boever F, Clement F, Demoitie MA, Mettens P, et al. Improved CD4(+) T cell responses to Mycobacterium tuberculosis in PPD-negative adults by M72/AS01 as compared to the M72/AS02 and Mtb72F/AS02 tuberculosis candidate vaccine formulations: a randomized trial. Vaccine (2013) 31:2196–206. doi: 10.1016/j.vaccine.2012.05.035
44. Huygen K, Abramowicz D, Vandenbussche P, Jacobs F, De Bruyn J, Kentos A, et al. Spleen cell cytokine secretion in Mycobacterium bovis BCG-infected mice. Infect Immun. (1992) 60:2880–6
45. Cooper AM, Flynn JL. The protective immune response to Mycobacterium tuberculosis. Curr Opin Immunol. (1995) 7:512–6. doi: 10.1016/0952-7915(95)80096-4
46. Jung YJ, LaCourse R, Ryan L, North RJ. Evidence inconsistent with a negative influence of T helper 2 cells on protection afforded by a dominant T helper 1 response against Mycobacterium tuberculosis lung infection in mice. Infect Immun. (2002) 70:6436–43. doi: 10.1128/IAI.70.11.6436-6443.2002
47. Tascon RE, Stavropoulos E, Lukacs KV, Colston MJ. Protection against Mycobacterium tuberculosis infection by CD8+ T cells requires the production of gamma interferon. Infect Immun. (1998) 66:830–4.
48. Clay H, Volkman HE, Ramakrishnan L. Tumor necrosis factor signaling mediates resistance to mycobacteria by inhibiting bacterial growth and macrophage death. Immunity (2008) 29:283–94. doi: 10.1016/j.immuni.2008.06.011
Keywords: lipoprotein Z, tuberculosis, innate immunity, T cell immunity, immune protection
Citation: Chen Y, Xiao J, Li Y, Xiao Y, Xiong Y, Liu Y, Wang S, Ji P, Zhao G, Shen H, Lu S, Fan X and Wang Y (2019) Mycobacterial Lipoprotein Z Triggers Efficient Innate and Adaptive Immunity for Protection Against Mycobacterium tuberculosis Infection. Front. Immunol. 9:3190. doi: 10.3389/fimmu.2018.03190
Received: 01 August 2018; Accepted: 31 December 2018;
Published: 16 January 2019.
Edited by:
Marina De Bernard, University of Padova, ItalyReviewed by:
Lilliana Radoshevich, University of Iowa, United StatesAmélia M. Sarmento, Fernando Pessoa University, Portugal
J. Claire Hoving, University of Cape Town, South Africa
Copyright © 2019 Chen, Xiao, Li, Xiao, Xiong, Liu, Wang, Ji, Zhao, Shen, Lu, Fan and Wang. This is an open-access article distributed under the terms of the Creative Commons Attribution License (CC BY). The use, distribution or reproduction in other forums is permitted, provided the original author(s) and the copyright owner(s) are credited and that the original publication in this journal is cited, in accordance with accepted academic practice. No use, distribution or reproduction is permitted which does not comply with these terms.
*Correspondence: Ying Wang, eXdhbmdAc2licy5hYy5jbg==
Xiao-yong Fan, eHlmYW4wMDhAZnVkYW4uZWR1LmNu
†These authors have contributed equally to this work