- 1Molecular and Cellular Biology Program, Dartmouth College, Hanover, NH, United States
- 2Thayer School of Engineering, Dartmouth College, Hanover, NH, United States
The field of HIV research relies heavily on non-human primates, particularly the members of the macaque genus, as models for the evaluation of candidate vaccines and monoclonal antibodies. A growing body of research suggests that successful protection of humans will not solely rely on the neutralization activity of an antibody's antigen binding fragment. Rather, immunological effector functions prompted by the interaction of the immunoglobulin G constant region and its cognate Fc receptors help contribute to favorable outcomes. Inherent differences in the sequences, expression, and activities of human and non-human primate antibody receptors and immunoglobulins have the potential to produce disparate results in the observations made in studies conducted in differing species. Having a more complete understanding of these differences, however, should permit the more fluent translation of observations between model organisms and the clinic. Here we present a guide to such translations that encompasses not only what is presently known regarding the affinity of the receptor-ligand interactions but also the influence of expression patterns and allelic variation, with a focus on insights gained from use of this model in HIV vaccines and passive antibody therapy and treatment.
Introduction
Advances in the treatment and prevention of HIV have relied heavily on the macaque animal model. Infection of animals with simian immunodeficiency virus (SIV) or chimeric simianized human immunodeficiency virus (SHIV) allows researchers to both model transmission and induce in a variety of macaque species a disease state characterized by sequelae closely resembling those found over the course of human HIV infection (1–5), against which therapeutic interventions can be compared. The similarities of the macaque model to humans has permitted the screening of drug candidates prior to elaborate and expensive human trials, which has contributed to the development of lifesaving drugs such as tenofovir (6, 7). In addition to its successes in the development of small molecule inhibitors, work in macaques has demonstrated that vaccines and antibodies are capable of mediating protection against SIV/SHIV infection, offering important insights into the characteristics of effective immunological responses that serve as a model for vaccine research and development.
However, the high-profile failures of all but one of the vaccine candidates tested in humans to protect against HIV-1 infection also suggest the limitations of the macaque model. For all of the beneficial contributions the model has made to the understanding and treatment of HIV, discrepancies between outcomes in macaque studies and in the clinic suggest that a better understanding of how human and macaque immunobiology differ may be necessary in order to more reliably draw translatable conclusions from animal studies. Fundamental differences between humans and macaques, here focusing particularly in the area of humoral immune responses, mean that in some cases, the result of a vaccination study or a passive transfer experiment conducted in macaques may not be recapitulated if attempted in humans. Promisingly, with a more complete understanding of this interspecies diversity, it should be possible to better translate observations likely to be meaningful to human protection and therapy. While numerous Fc receptors for IgG exist, many of these, including FcRL5, mucins, and TRIM21 (8–10), have yet to be evaluated thoroughly in the macaque model. However, recent work has greatly enhanced our knowledge of IgG, FcγR, and FcRn biology in rhesus macaques. This review summarizes the current understanding of what differences exist in the setting of receptor and antibody interactions between humans and non-human primates, with a particular emphasis on those facets that have the potential to affect the evaluation of candidate vaccines and antibody-based strategies for the prevention or treatment of HIV-1 infection in humans.
Antibodies in HIV Prevention and Therapy
A wealth of data from non-human primate studies has demonstrated that monoclonal antibodies can provide sterilizing protection from viral challenge (11–13); additionally, because humoral immune responses are often correlates of vaccine-mediated protection, it has become clear that closer inspection of immunoglobulin biology in macaques has the potential to significantly advance research and development of vaccines and therapeutic antibodies. This potential was made all the more apparent following the conclusion of the single successful human vaccine trial to date, the RV144 trial conducted in Thailand, in which a moderate degree of protection was observed (14). A subsequent study of vaccine-induced immune responses identified binding, rather than broadly neutralizing, antibodies directed against the envelope glycoprotein as a correlate of reduced risk of infection (15). Furthermore, additional correlates analysis suggested that binding antibody responses with certain characteristics, such as a bias toward IgG3, away from IgA, and with antibody-dependent cell-mediated cytotoxicity (ADCC), and complement cascade-initiating activity were associated with reduced risk (15–18). These findings reinforce those of numerous other studies in both humans and macaques (summarized in Table 1) that have concluded that innate immune effector functions, such as ADCC, correlate with and may directly contribute to improved outcomes at all stages of HIV infection, from potentially blocking acquisition of the virus altogether to maintaining a lower viral load and delaying the onset of AIDS.
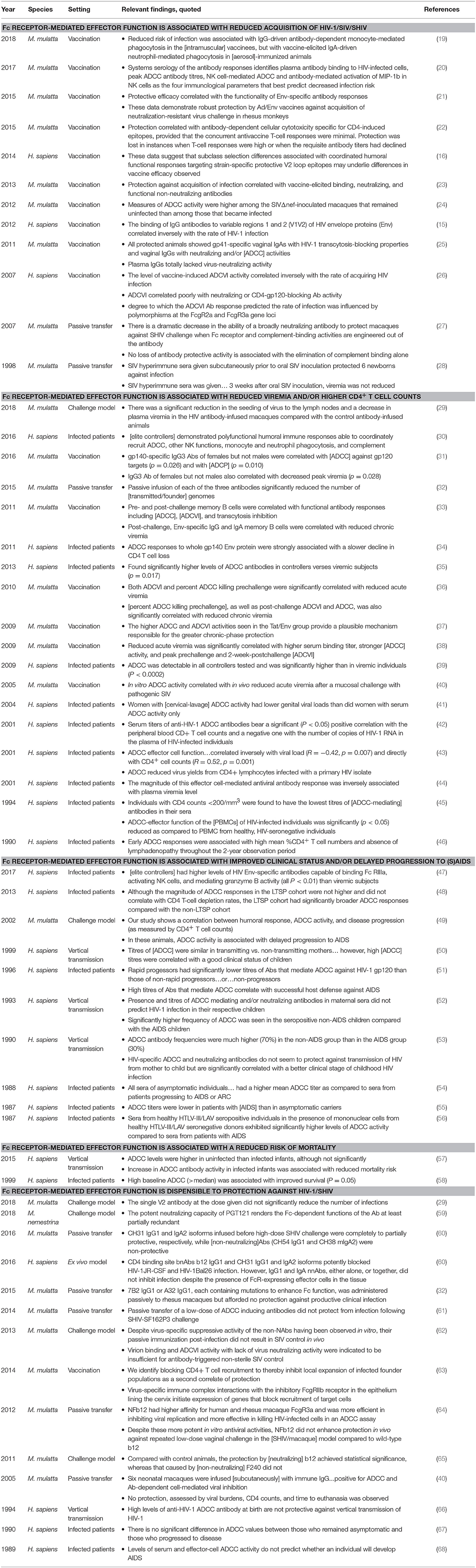
Table 1. A partial summary of human and NHP studies that investigated the role of antiviral antibody effector functions in the context of (S)HIV infection.
One of the most illustrative examples is the work of Hessell et al. which compared the protective efficacy of the broadly neutralizing antibody b12 with variants engineered to lack affinity for Fcγ receptors (FcγR) and/or the complement cascade-initiating protein C1q. Passive transfer of the native b12 was able to successfully block infection of rhesus macaques (27). Comparative analysis of the engineered variant lacking affinity for cellular antibody receptors to the crystallizable fragment (Fc) of IgG demonstrated that effector function driven by FcγRs, but not complement, contributed to protection. Similarly, humanized mouse models of HIV prevention and therapy have led to similar observations regarding the contribution of effector functions to the in vitro effect of other broadly neutralizing antibodies (69–71). However, given the lack of impact of effector function on the protection afforded by the neutralizing antibody PGT121 (59), and the inability of Fc glycosylation changes that result in enhanced ADCC activity specifically to enhance b12's protective efficacy (64), the generalizability of this observation to other antibodies, and the specific FcγR-dependent effector activities that may play a role in protection, remain to be determined. Nonetheless, a wealth of diverse data from studies of vaccines, monoclonal antibodies, and natural infection converge to point toward the importance of Fc receptor-mediated antiviral activities in vivo. Thus, fully leveraging the macaque model of HIV to identify vaccine and antibody candidates that bear desirable effector function profiles requires accounting for the ways in which immunoglobulins and their receptors differ between non-human primates and humans.
Antibody Receptors
The considerable interspecies diversity existing between the members of the FcγR family (itself a member of the immunoglobulin superfamily) (Figure 1) dramatically complicates comparisons of immune responses in humans and macaques. When cross-linked through their binding of antigen-antibody immune complexes, FcγRs transmit activating or inhibitory signals to the immune cell, depending on the identity of the signaling domain associated with the receptor in question. By enabling innate immune effector cells to potently respond to antigenic targets that the humoral immune system has adapted to recognize, FcγRs serve as a critical bridge between the arms of the immune system. Among other outcomes, FcγR engagement can lead to the release of cytokines, the direct killing of virus-infected, antibody-opsonized cells via ADCC, and phagocytosis and subsequent antigen processing and display (72).
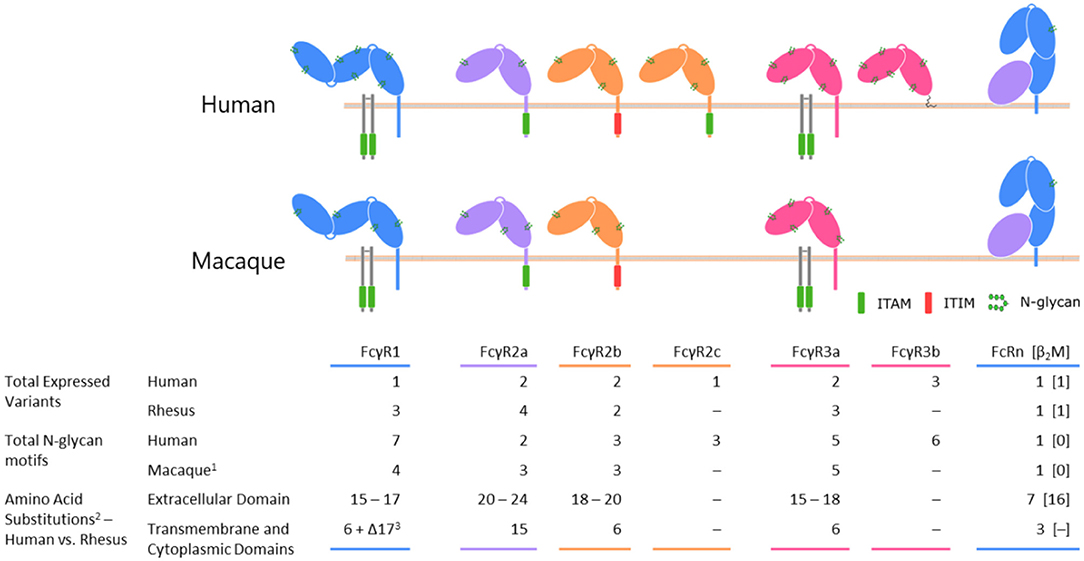
Figure 1. A comparison of selected Fc receptors between humans and rhesus macaque species. Differences in protein coding sequences reflects insertions, deletions, and sites of polymorphism.
(1) The number of N glycan motifs is conserved across rhesus and cynomolgus macaques.
(2) The ranges of values represent the minimum and maximum changes involved in shifting from human to rhesus; exact numbers depend on the allelic variants being compared.
(3) Rhesus macaque FcγRI is truncated by 17 amino acids at its C terminus relative to the human variant.
This review will also briefly consider a receptor unrelated to the FcγR family—the MHC class I-like neonatal Fc receptor (FcRn). Much like the Fcγ receptors, FcRn acts as an important link between arms of the immune system, connecting humoral, and cellular immunity through its diverse activities. The receptor's capacity to distinguish monomeric IgG from that found in immune complex both imparts IgG with a lengthy serum half-life (73), and ensures that captured antigens are efficiently processes and presented to T cells (74, 75).
The differences in the sequences, ligand binding affinities, and expression patterns of human and macaque IgG and IgG receptors raise the possibility that a potent effector function-driven immune response may have a different phenotypic character in each species. This situation is further complicated by the frequent use of species-mismatched reagents and cell lines for in vitro activity assays. For example, the high baseline activity of macaque natural killer cells has driven most analyses of the ADCC potential found in the sera of vaccinated animals to be performed using human cell lines (40, 76). Similarly, the macaque/SHIV model is routinely used to evaluate the ability of passively transferred human antibodies to protect against infection (11–13). Absent a convincing case that the subclass of the transfused antibody or the receptor profile of the effector cell lines do not differ meaningfully between species, caution is required when attempting to extrapolate the findings of such studies from one species to another. Such risks may be mitigated, however, if given the means to more confidently translate observations between primate species and humans.
Distinctions Between Human and NHP IgG Types
The other participant in the FcγR-antibody interaction, immunoglobulin G, introduces an additional dimension that must be accounted for when translating observations between macaques and humans. Primate species nominally share the same IgG subclasses, having IgG1 through IgG4, numbered according to serum prevalence, but in the case of humans and macaques, the similarities largely end with this convention. Macaque IgG subclasses are in fact a much less structurally and functionally diverse group of proteins than those found in humans (Figure 2). For example, human IgG3 features an elongated hinge, up to 62 amino acids in length (81, 82), for which no analog has been found in any species of macaque. Human IgG2, on the other hand, has a shortened hinge, and exists in several disulfide bond-based structural isoforms whose activity profile can differ (83). The similarities between macaque subclasses do not end with their sequences, however; the functional activity of macaque IgG, assessed by the ability to promote monocyte phagocytosis and natural killer cell degranulation, and binding to FcγR is relatively consistent across each of the four subclasses in rhesus (80) and cynomologus (77), as compared to the more distinct activity and binding profiles observed for human IgG subclasses.
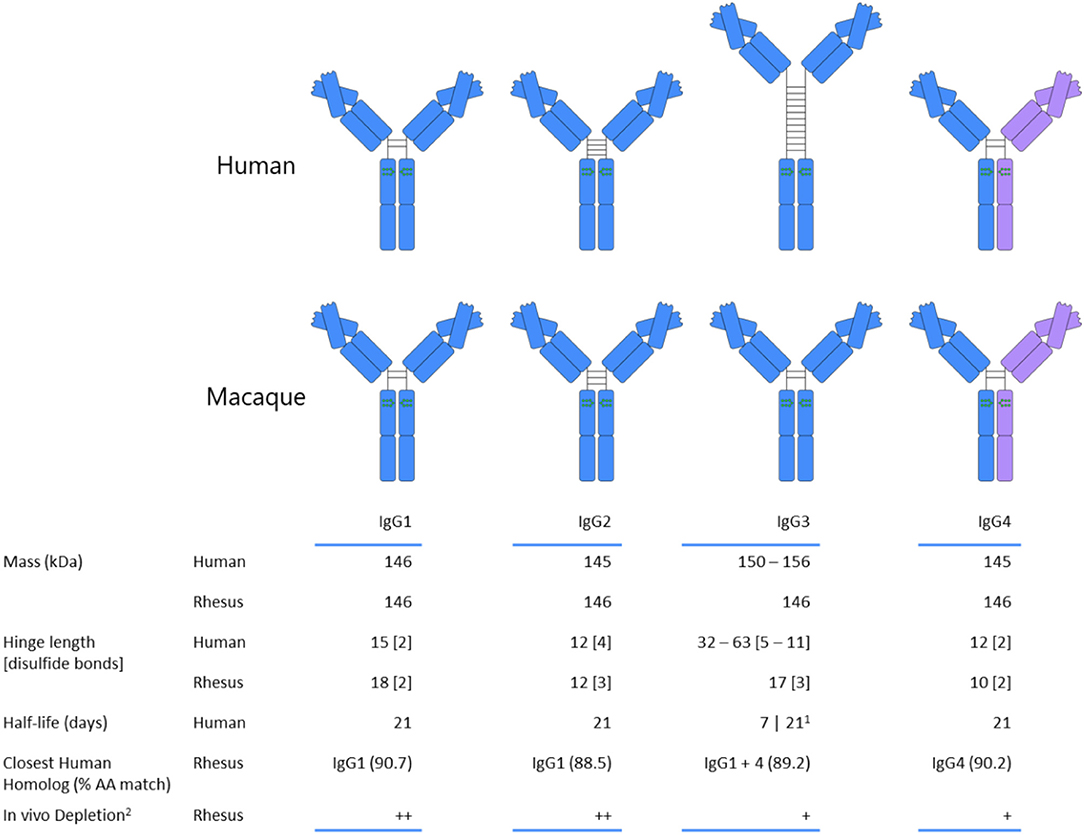
Figure 2. Comparison of human and rhesus macaque subclass properties. Human IgG3 is depicted with the most commonly expressed number of hinge unit repeats. IgG4 coloration reflects the potential for Fab arm exchange, which is a feature of human and rhesus IgG4, but not of cynomolgus macaques. References: (77–79).
(1) The allotype-dependent inclusion or absence of His435 determines half-life of human IgG3, with histidine-containing having a longer half-life.
(2) Plus signs denote the relative degree and durability of the decline in the CD8+ T cell population based on treatment with subclass-switched anti-CD8 monoclonal antibody (80).
The human IgG response, conversely, can progress through better delineated phases during the course of infection, phases which may have arisen because they can collectively lead to an immune response activity profile that rises and falls when appropriate – fighting infection early on, and having the capacity to wane before the damage caused by inflammation is too severe. Within this framework, the role of IgG3, the first subclass within the immunoglobulin locus and the form that tends to be observed early on in infection (84), is to rapidly activate the immune response, particularly the effector cells of the innate immune system. In keeping with this role, human IgG3 strongly interacts with Fcγ receptors (85) and is a potent promoter of phagocytosis and degranulation. Switching downstream in the locus to IgG1 gives rise to this abundant, long-lived molecule. In fact, it has been theorized that the relative affinities of the human subclasses differ as beneficial mutations accumulate and switching to downstream subclasses occurs (86). By virtue of their generally reduced affinity for FcγR (85), subsequent or direct class-switching to IgG2 or IgG4 reduces activating potential, leading to the formation of less potent immune complexes (78). IgG4 may be particularly well-suited for this role because of its ability to undergo the process of Fab arm exchange, through which naturally-occurring bispecific antibodies are produced (87, 88). Because bivalent targeting normally aids in immune complex formation, the inability to avidly bind may further reduce the inflammatory potential of IgG4. Indeed, in humans, switching to IgG4 is often associated with amelioration of antibody-mediated allergy (89–92). Cynomolgus macaque antibodies cannot perform this Fab arm exchange (77, 93), and the quantity of IgG4 in macaques is thought to be quite minor (79, 80, 94).
Further, while the IgG subclasses are generally ordered from most to least activating in the immunoglobulin locus of humans (IgG3, IgG1, IgG2, IgG4), they are ordered from least to most activating in rhesus (IgG4, IgG3, IgG2, IgG1) (80, 95), though again, the differences in activity across rhesus and cynomolgus macaque subclasses are considerably less pronounced than in humans, and IgG sequences suggest this may hold in other macaque species as well (96). Left unanswered is the question of what significance observed polymorphisms in macaque IgG subclasses may hold—evidence of polymorphisms despite limited sequencing efforts may suggest the presence of significant allotypic diversity in rhesus and cynomolgus macaques (95, 97). It is likely that, to date, immunoglobulin sequence variation existing within macaque species has been undersampled.
While little is known about the progression of subclass switching over time in macaques, in humans, an early but waning IgG3 response has been observed in the setting of both natural infection and vaccination (16, 98, 99). Repetitive protein boosts in the setting of vaccination have also been observed to increase the magnitude of the IgG4 response (16, 17, 99). Most importantly, the range of effector activity differences and structural distinctions among subclasses are considerably more broad in humans than in macaques (80, 100). When making immunological comparisons between species, it is important to consider the ways in which human antibodies have been functionally honed that are not shared by the non-human primates relied on as research models.
Distinctions Between Human and NHP FcγR
Members of the FcγR family share several common traits. Typically, two extracellular C-like domains are responsible for binding residues of IgG located in the lower hinge region (101–105). A molecule of IgG is bound by a single Fcγ receptor (106–108) through contacts made with both heavy chain constituents of the IgG homodimer. The character of those contacts differs between the protomers, with each side tending to favor a different class of intermolecular interactions (109). The extracellular domains of the receptors are followed by a transmembrane domain and a cytoplasmic domain, which may contain an activating or inhibitory signaling motif. In the absence of an intrinsic signaling motif, the task of communicating the antibody-mediated binding and receptor clustering is handled through association with the Fc receptor γ chain or other accessory receptors. An overview of the similarities and differences between receptors can be found in Figure 1.
Organization of this review will follow according to the type of signal a receptor transduces. Fcγ receptor crosslinking may lead to the generation of an activating signal though the involvement of an immunoreceptor tyrosine-based activating motif (ITAM) located in the cytoplasmic domain of the receptor or on the Fc receptor γ chain [reviewed in (72, 110–112)]. Briefly, activating receptors include the human and macaque FcγRI, FcγRIIa, and FcγRIIIa receptors. FcγRIIb, bearing an immunoreceptor tyrosine-based inhibitory motif (ITIM), is then the sole member of the inhibitory category of FcγR. The FcγRIIIb receptor found exclusively on human granulocytes, and apparently not present in the macaque genome, represents a special case as it lacks the normal transmembrane and cytoplasmic domains and is instead anchored to the cell membrane via a lipid chain. Although not as well-characterized as the other human Fcγ receptors, much of the available evidence suggests that FcγRIIIb contributes to the activation of granulocytes and that it is most appropriately included in the ITAM-bearing category of receptors.
FcγRI
Among members of the Fcγ receptor family, FcγRI stands apart by virtue of its three extracellular domains and high ligand-binding affinity. As in humans, the receptor's elevated affinity in rhesus and cynomolgus macaques, has been measured in the low nanomolar to high picomolar range (77, 100), allows it to readily bind circulating monomeric IgG (85). As Figure 1 illustrates, the cytoplasmic domain of FcγRI lacks an intrinsic ITAM and this receptor instead utilizes the Fc receptor common γ chain to activate the cell (113, 114).
Characterization of FcγRI's role initially presented a challenge to researchers as the failure to express the receptor in humans apparently does not lead to any obvious immunodeficiency or associate with more frequent infections (115). Furthermore, the high concentration of IgG in circulation coupled with the receptor's high affinity suggested that FcγRI would be fully occupied by monomeric IgG at steady state (110). However, the conservation of this receptor across primate species and the rarity of individuals with expression defects suggests an important biological role. It is now thought that a primary role of FcγRI may be in antigen processing and display, as well as triggering the release of cytokines (116–118).
The single major allele of FcγRI expressed by humans (NP_000557) shares a high degree of amino acid sequence identity (~90%) with the macaque variants characterized to date, although several noteworthy differences do exist. The three major rhesus variants and one cynomolgus variant differ from the human receptor at four predicted contact residues and lack three N-linked glycosylation motifs found in the human molecule. Furthermore, in rhesus macaques (see NP_001244233), but not cynomolgus (NP_001270969), there is a 17 amino acid truncation at the intracellular C terminus. The consequences of this deletion, if any, for the receptor's association with the common γ chain have not been determined.
Within the macaque genus, FcγRI's extracellular domain phenotype is extremely similar, with the most common rhesus allele (FcγRI-1) and the major cynomolgus allele sharing identical extracellular domains. Furthermore, the rhesus FcγRI-2 allele differs from these at only two extracellular residues and the FcγRI-3 allele at just one. None of these polymorphic positions are predicted to contact the IgG Fc based on homology models. This high degree of conservation is also thought to extend to FcγRI's pattern of expression by immune cells. In keeping with its role in antigen presentation, the receptor is expressed by dendritic cells (117, 119), monocytes, and macrophages (120) (Table 2). Granulocytes are also capable of expressing FcγRI following induction by interferon γ (130, 131).
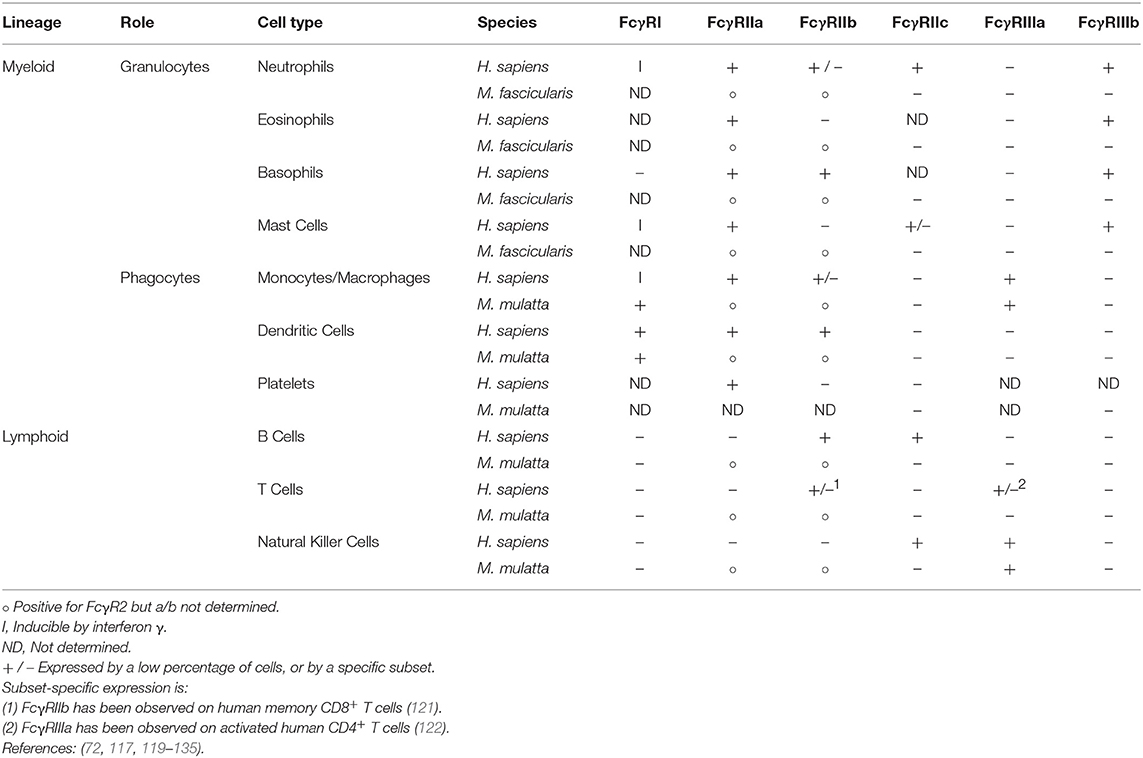
Table 2. Expression of members of Fcγ receptor family by immune cell subsets in humans and macaques.
In light of the high degree of homology shared by human and macaque FcγRI, it should come as no great surprise that the receptors have strikingly similar preferences for IgG subclasses. Within a fully human context (receptor and IgG), FcγRI binds IgG1 and IgG3 with similarly high affinities, and has an intermediate preference for IgG4. The receptor demonstrates significantly reduced affinity for IgG2 (77, 85). In rhesus macaques, however, FcγRI has nearly equivalent affinities for all four subclasses of rhesus IgG (100); this trend is also observed in cynomolgus macaques (77). The cause of this difference is not the sequence of the receptors, but the IgG. This becomes apparent when mixing receptors and antibodies of different species—human FcγRI has relatively uniform affinity for different subclasses of macaque IgG while macaque receptors exhibit preferences between human subclasses (77, 100). Human IgG2 contains a unique motif (VAGP) in its upper CH2 domain, a motif not present in any macaque subclass or other human subclasses [instead—LLGGP, for all but human IgG4; accession numbers: [human] P01857; P01859; P01860; P08161 [rhesus macaque] see (79)], that impairs binding to FcγRI and accounts for this observation (136). Interestingly, human IgG4 has a slightly altered motif (FLGGP) at this position, which may explain the intermediate recognition of this subclass.
The high degree of FcγRI conservation between humans and macaques imposes fewer restrictions on the comparison process than is the case for other receptors handled in this review. IgG1, which is perhaps the most likely candidate for comparison due to its prevalence in vivo and among licensed therapeutics (137–139), is a particularly straightforward case and FcγRI-bearing macaque cells are expected to recognize a passively transfused human antibody near natively. In the case of human IgG2 and IgG4 however, there is evidence to suggest that while macaque FcγRI mirrors the human receptor's hierarchy of subclass preference outlined above, the receptor's affinity may be up to 4-fold stronger than in humans (77, 100). Since human IgG2 and IgG4 Fc domains have been used for monoclonal antibodies in instances where a silent Fc profile is desired (139), as well as for extending the half-life of therapeutic proteins by expression as Fc fusions, the heightened inflammatory potential of these molecules when evaluated in a macaque model may need to be taken into account.
FcγRIIa
A sharp increase in complexity, both with and between species, occurs when surveying the most widely expressed activating receptor—FcγRIIa (140). This receptor plays a central role in the phagocytosis of antibody-opsonized antigens, which it triggers by means of an intrinsic ITAM located in its cytoplasmic domain (110).
Humans express two major allelic variants of FcγRIIa, which are distinguished by the identity of the amino acid found at position 131 of the extracellular domain (141). Expression of an arginine at this location results in weakened binding of IgG, particularly for IgG2 (142). Conversely, the histidine-containing variant demonstrates substantially higher affinity across multiple subclasses, including IgG2, and can effectively bind immune complexes composed of this subclass (85, 143). The consequences of this polymorphism can be dramatic. In the treatment of cancer, multiple monoclonal antibodies indicated for a range of malignancies have demonstrated differing responses based on the allelic makeup of the recipients (144). Protection against extracellular bacteria, often mediated by IgG2 targeting polysaccharide antigens, can also be impaired by the lower affinity allele; this may lead to an increase in the frequency and severity of certain infections among R131 individuals, particularly those homozygous for the allele (145–147). In the context of HIV, infected individuals that are homozygous for the lower affinity R131 variant experience a more rapid decline in CD4+ T cells than subjects having one or two copies of the high affinity H131 allele (148). Interestingly, no impact of this polymorphism on risk of infection was observed in the Vax004 HIV vaccine trial (149).
In macaques, a considerably greater number of major allelic variants have been characterized. Based on records in GenBank, numerous variants of this receptor have been defined in rhesus, pigtailed and cynomolgus macaques. While H131 is the minor allele in several human populations (150, 151), most macaque FcγRIIa alleles feature a histidine at this position and therefore resemble the high affinity human receptor in sequence and antibody recognition (100). The FcγRIIa-4 allele of rhesus macaques is an important exception to this trend, having a proline substitution at this residue that is likely detrimental to the receptor's affinity for IgG (100). Similarly, although the FcγRIIa-1 allele includes the critical histidine, it also contains an additional N-linked glycan motif at position 128, a residue that contacts the Fc region of IgG in the cocrystal structure of human proteins (152), which apparently interferes with the receptor's IgG affinity (100). The consequence of these differences is that alleles 1 (at least when produced recombinantly) and 4 have reduced affinity for macaque IgG compared to alleles 2 and 3 (100), creating high and low affinity variation analogous to the R131/H131 polymorphism in humans. Although the exact allelic frequencies have not been reported, the allotypic variants have been roughly numbered by prevalence (100). Accordingly, the FcγRIIa-1 allele is expected to occur most frequently, and its impact on passive transfer experiments and vaccine studies could prove to be substantial, provided that the native receptor is glycosylated similarly to the recombinantly-produced receptor utilized in in vitro studies. Relevant to the potential for differences between macaque and human FcγRII to drive differences in outcomes of antibody infusions between species, it is clear from evaluation of the binding profiles of engineered IgG sequence variants across human and rhesus receptors, that greater phenotypic differences are observed for FcγRII as compared to FcγRIII between species (153).
Receptor variation is not limited to the extracellular domain of FcγRIIa. While the four reported receptor alleles of rhesus macaques are identical intracellularly, they differ from the human and cynomolgus macaque variants in the sequence of their signaling domains. The activating ITAM motif takes the form of two semi-conserved sequences separated by six to eight amino acids. In the available sequences reviewed ([human] P12318; [rhesus] H9BMP0; [cynomolgus] Q8SPW4), the first ITAM unit takes the form Tyr-Met-Thr-Leu. Rhesus macaques repeat this sequence for the second iteration of the motif. Humans and cynomolgus macaques on the other hand use the slightly altered sequence of Tyr-Leu-Thr-Leu (154). This is in addition to a handful of residues flanking the second ITAM that are conserved among the available macaque sequences, but that differ when compared to the human receptors. The consequences of these substitutions, if any, are not known.
The comparison of sequence diversity alone is not sufficient to capture all of the differences between human and macaque FcγRIIa; among other factors, the patterns of expression must also be accounted for when translating observations between species. In humans, FcγRIIa is the most commonly expressed Fc receptor of monocytes and macrophages (155), and is also expressed by dendritic cells and granulocytes. Analysis of expression in macaques has been complicated by the fact that the extracellular domain of FcγRIIa and the inhibitory FcγRIIb receptor have even more highly conserved amino acid sequences (95%) than is the case in humans (92%) (100) ([human] P12318 vs. P31994; [rhesus] H9BMP0 vs. F7GVR0; [cynomolgus] Q8SPW4 vs. Q8SPW3). Difficulty in obtaining flow cytometry reagents capable of reliably discriminating between the receptors has meant that much of the literature published to date omits the specific form of the receptor expressed by a given cell type, reporting instead only the presence or absence of FcγRII (156). Even so, there is data that suggests that cynomolgus and pigtail macaque neutrophils express 3–5 fold more total FcγRII than their human counterparts (77, 156), which are known to express only the activating form of the receptor (132). Given this known difference in expression levels and additional differences potentially associated with activating vs. inhibitory and allotypic FcγRII composition, there is the potential for altered neutrophil biology in non-human primate model systems.
FcγRIII
In many of the studies summarized in Table 1, subjects producing antibodies capable of carrying out directed killing of virally-infected cells through the process of antibody-dependent cell-mediated cytotoxicity (ADCC) experienced significantly better clinical outcomes. In macaques and most, but not all, humans, the cell type most commonly associated with performing ADCC, natural killer (NK) cells, exclusively express FcγRIIIa, making the receptor a potent potential contributor to antiviral effector function. Humans express two major allelic variants of FcγRIIIa that are distinguished based on differing levels of activity attributed to a single polymorphic residue in the extracellular domain—position 158 in this case (157, 158). Expression of a valine at this position results in greater affinity for human IgG1 and IgG3, and to a lesser extent IgG4, as compared to the phenylalanine-containing variant (85). Although it is found less frequently in the population (159), the V158 allele can confer significant benefits. For example, V158 individuals respond more strongly to anti-tumor monoclonal antibody therapy, an observation attributed to enhanced ADCC directed against the tumor cells (160–162).
The extracellular domain of rhesus macaque FcγRIIIa also exhibits a polymorphism at position 158. However, the isoleucine expressed by the FcγRIIIa-1 and−2 alleles has similar side chain chemistry to the valine of FcγRIIIa-3 and as a result, the extracellular domains of known rhesus alleles resemble the high affinity human receptor in both sequence and affinity (153). Interestingly, the FcγRIIIa-2 allele, which is identical to FcγRIIIa-1 extracellularly but carries a pair of polymorphisms that introduce valine residues to the transmembrane domain and membrane-proximal region of the cytoplasmic domain (positions 229 and 233, respectively), is associated with more complete depletion of B cells following treatment with rituximab (163). More broadly, the amino acid sequences of the non-human primates surveyed maintained > 90% homology to humans in their extracellular domains. In each of the macaque sequences though, there is an altered residue that leads to the elimination of a N-glycan motif known to be modified in humans. This is potentially offset by the introduction of a new motif close by, but it is not known whether this new site is accessible for glycosylation.
Based on biophysical and functional data gathered thus far (77, 100, 153), one can reasonably conclude that the macaque forms of the receptor are a fair approximation of the high affinity human allele, FcγRIIIa V158. Unfortunately, the prevalence of the lower affinity F158 allele in humans exceeds that of the high affinity allele (164–166). All else being equal, this difference in allelic frequencies suggests that most humans may be likely to generate a less robust ADCC response than a macaque would based on the lower affinity of their FcγRIIIa receptor. Significantly, human polymorphic variation at this position has been associated with rate of infection among low risk HIV vaccine recipients, and development of Kaposi's sarcoma, an AIDS defining illness, in HIV infected men (148, 167).
Human granulocytes uniquely express a variant of FcγRIII that is apparently not found in any species of macaque—FcγRIIIb (168). The receptor's homology to the extracellular domain of human FcγRIIIa and the weight of experimental evidence generated to date argue for the inclusion of FcγRIIIb alongside the activating Fcγ receptors. This classification has been far from straightforward however (169), in part because FcγRIIIb uniquely lacks both a transmembrane domain and cytoplasmic signaling domains, instead being tethered to the cell membrane via a glycosylphosphatidylinositol (GPI) anchor (168). Even so, upregulation of FcγRIIIb in response to engagement has been observed as well as an association with Syk signaling in neutrophils (170). In order to transduce such signals, FcγRIIIb would require an accessory signaling element, the proposed candidates for which include the complement receptor (171) and FcγRIIa (172). The latter receptor has been hypothesized to act in synergy with FcγRIIIb to increase the flux of Ca2+ into granulocytes and enhance their effector functions (173), which include the release of cytotoxic granules (174) and phagocytosis (175, 176). Conversely, a competing view of FcγRIIIb holds that the receptor merely serves as a decoy that competes with activating receptors for immune complexes. Experimental support for this model lies in the observation that enhancing the affinity of IgG for FcγRIII broadly resulted in lower levels of neutrophil ADCC (177). This alternate hypothesis raises the possibility that human granulocytes may have a means to receive an inhibitory input that is not available to macaque effector cells, potentially further skewing signaling in neutrophils.
Inhibitory Receptor
FcγRIIb
All humans and macaques express a single form of inhibitory FcγR—FcγRIIb. The receptor contains an intrinsic ITIM in its cytoplasmic domain that allows it to rein in immune responses. FcγRIIb is abundantly expressed by phagocytic cells (120, 132) and is typically the sole Fcγ receptor found on human B cells (132, 133). It is additionally expressed by liver sinusoidal endothelial cells, where it is thought to play an role in recognition and uptake of immune complexes (178, 179). Evaluation of the role of this receptor on immune cells has been especially hampered by the limited availability of staining reagents capable of discriminating FcγRIIa from FcγRIIb. In humans, an FcγRIIb-specific reagent is available (132), however, its specificity toward macaque receptors has not been reported. All major human variants of FcγRIIb feature identical extracellular domains and contain an arginine at residue 131 (P31994), making them phenotypically more similar to the low affinity variant of the activating FcγRIIa (85, 153). Of the two human alleles which have been characterized, the rarer variant contains an isoleucine to threonine mutation in the transmembrane domain, at residue 232. This change renders the receptor incompatible with inclusion in lipid rafts and as a result, unable to signal (180, 181).
In contrast to humans, both reported alleles of rhesus macaque FcγRIIb feature a histidine at position 131 and therefore more closely resemble the high affinity variants of FcγRIIa. This is superceded, however, in the FcγRIIb-2 allele by a leucine to proline substitution at residue 88, a site immediately adjacent to a predicted Fc contact residue, which abrogates the receptor's affinity for all subclasses of both rhesus macaque and human IgG (100). While this variant is the less common of the two alleles, its precise frequency has yet to be reported. However, it is possible that the inclusion of FcγRIIb-2 animals in a study could have significant consequences. Intracellularly, FcγRIIb is well-conserved between humans and macaques; the cytoplasmic domains differ at just two residues ([human] P31994; [rhesus] F7GVR0; [cynomolgus] Q8SPW3). Of these differences, one is a conservative change from isoleucine in humans to valine in rhesus macaques. Furthermore, neither of the mutations occurs proximal to the signaling motif.
That FcγRIIb is the sole inhibitory Fc receptor in humans and macaques means the consequences of the interspecies FcγRIIb differences have the potential to be far-reaching. For example, according to one proposed model of SIV and HIV infection, FcγRIIb engagement at the mucosa somewhat paradoxically has been suggested to contribute to protection by generating an inhibitory signal that slows the migration of CD4+ T cells to the region and deprives the virus of host cells for replication (63). However, the high affinity phenotype of macaque FcγRIIb could mean that the receptor stands on an equal footing (or greater, in the case of FcγRIIa alleles 1 and 4) with the activating receptors in the competition for immune complexes—a scenario that is not recapitulated in humans where even FcγRIIa-R131 has higher affinity for IgG than FcγRIIb (85, 100).
Additional Receptors
FcγRIIc
In ~20–45% of humans (182, 183), an unequal genetic crossover between the activating cytoplasmic domain of FcγRIIa and the extracellular domain of FcγRIIb results in the expression of the receptor FcγRIIc (184) on B cells and natural killer cells (134, 135). FcγRIIc provides an additional means of activating immune cells and is capable of triggering ADCC in natural killer cells (185). In the context of HIV infection, the receptor is noteworthy based on the observation that a single nucleotide polymorphism located in an intron of FcγRIIc was associated with greater vaccine efficacy in the RV144 trial (186). A similar receptor has not been reported in macaques.
Neonatal Fc Receptor (FcRn)
The similarities between macaques and humans that make these non-human primates a tractable model for the study of HIV also make them useful for determining the pharmacokinetic properties of biologic drugs. Cynomolgus macaques in particular are a widely utilized species for the evaluation of antibodies and Fc fusion proteins (187), biologics that depend heavily on FcRn to achieve half-lives that can extend beyond 3 weeks (73, 188–191).
FcRn is a heterodimeric protein composed of a β2-microglobulin (β2m) subunit and an MHC class I-like heavy chain (192–194). Diverse tissues and cell types express FcRn (195–198), but it functions principally in intestinal epithelia, vascular endothelia, and syncytiotrophoblasts (199–203) where the receptor's ability to bind to the Fc portion of IgG in a pH-dependent manner, having high affinity under acidic conditions (pH < 6.5) and relatively low affinity at a physiological pH of 7.4 (204–206), permits the uptake, transfer, and release of IgG across compartments of the body. This property of FcRn enables the maternofetal transfer of IgG across the placenta (207) and the absorption of IgG present in maternal milk by the intestinal epithelium of neonatal rodents (204, 208). FcRn's high level of expression in vascular endothelial cells leads to the sorting and recycling of endocytosed IgG from acidified endosomes, where the receptor's affinity for IgG is high, to circulation, where the physiological pH causes the release of IgG (73, 189–191). Additionally, FcRn can contribute to the accelerated processing of antigens derived from multivalent immune complexes and their subsequent presentation by both forms of major histocompatibility complex (74, 75).
The heavy chain of FcRn is well-conserved among the macaque species commonly used in biomedical research; the most frequent variants are identical to one another (accession numbers: NM_001257520, NM_001284551, and XM_011768102). Among the ten residues that differ between humans and macaques, none occur in a location known to contact the Fc, or in the receptor's N-linked glycan sequon (209). Similarly, of the four polymorphisms observed in cynomolgus macaques, only two occur in the extracellular domain and neither involves putative IgG Fc contact residues (210). Interestingly, a promoter polymorphism in FcRn has been identified to associate with reduced levels of serum IgG, though the impact of this polymorphism on the half-life or biodistribution of passively administered mAb or polyclonal samples remains to be defined (211).
The β2-microglobulin subunit that makes up the remainder of the heterodimeric receptor is somewhat more diverse than the heavy chain, both between species of macaques and when macaques are compared to humans; the N-terminal isoleucine required for interaction with IgG (212) is present in all species under review, and the impact of sequence diversity in this protein has been less well-studied in all species.
Conclusions
The sheer number of factors to consider when translating observations between macaques and humans makes the process a challenging, multidimensional one. Differences in the structures and activities of IgG subclasses, and polymorphisms in protein sequence and post-translational modification of antibody receptors are a subset of the many relevant considerations. Copy number variation, splice variants, and alleles with sequence variation outside of coding regions have been associated with a diversity of phenotypes in humans (183, 213–217), and are presumed to exist in NHP. A number of differences in the patterns of cellular expression of FcγRs between species are well-established, and while genetically-associated differences in expression levels likely exist, they have not been fully characterized. Linkage disequilibrium between FcRs and a diversity of major and minor alleles make it difficult to define the potential effect of these polymorphisms with high confidence even in large cohorts. Thus, the process of precisely translating results between species is a complex and potentially intractable challenge. However, the highly significant contributions to drug development demonstrates that macaques can nonetheless serve as an excellent preclinical model.
When considering exceptional scenarios, several simplifying assumptions may help to smooth the process. The first—treating the members of the macaque genus largely interchangeably—has already achieved widespread adoption (156) as the shifting landscape of cost, conservation, and ethical considerations have driven changes in the decades since the model's introduction in the preferred macaque species, and among NHP more generally, for biomedical research (218). In light of the considerable similarities between macaque species (including shared allotypic diversity) outlined in this review, it is our view that under common research circumstances, this practice serves as a useful simplifying assumption; however, exceptions do exist. For example, evaluation of passive infusion of human IgG4 in NHP is one such example. Human IgG4 can undergo Fab arm exchange with endogenous human IgG4, but would not exchange with any serum antibody in some, though not all macaque species. Similarly, outcomes of vaccination studies in humans have been associated with the subclasses of antibodies induced. However, the extensive differences between the repertoire of subclasses in humans and the relatively more monolithic structural and activity profiles of NHP IgG types may pose challenges to easy translation of vaccine studies. These differences may preclude attempts to model means to induce specific subclasses by altered regimens, adjuvants, and immunogens, or to explore the relevance of class-switching patterns observed in humans to challenge outcomes.
Of the cell types and FcγR-mediated immune processes potentially impacted by the differences in human and macaque biology, two in particular stand out as deserving of additional comment. Unlike the low affinity phenotype of human FcγRIIb, the inclusion of a histidine at a critical contact residue in macaques grants equivalent affinity (or greater, in the case of alleles 1 and 4) to that of the activating FcγRIIa. While this has the potential to alter the ratio of activating and inhibitory signals relative to what is observed in humans for any cell type expressing a mix of receptors, the effect could be even more pronounced in B cells where FcγRIIb is the only member of the family expressed.
Neutrophil biology is another aspect of macaque immunity likely to differ significantly from that of humans based on differences in Fcγ receptors. The reasons for this are twofold. The first is the substantially higher level of expression of FcγRII receptor that has been observed on macaque neutrophils. This expression profile potentially contributes to a stronger response by these cells in macaques, although this assumes that macaque neutrophils, like those of humans, express FcγRIIa, and do not express inhibitory the FcγRIIb, which could offset the larger number of activating receptors. The second major difference is the unique expression of FcγRIIIb, which is absent from macaques, by human neutrophils, although the effect of expression of this receptor is more difficult to determine as its role has not been definitively determined.
The recent history of biomedical research well-illustrates the utility of the members of the macaque genus for modeling human diseases and evaluating therapeutic interventions. Much of the progress left to be made in understanding the non-human primate model resides on the margins and need only be considered under particular circumstances. However, consideration of the interspecies variability that has been defined to date and the gaps in our present understanding, if filled, have the potential to make macaques an even more enabling model organism. These insights in turn may increase the rate at which pre-clinical observations are converted to clinical success stories.
Author Contributions
All authors listed have made a substantial, direct and intellectual contribution to the work, and approved it for publication.
Funding
This work was supported by the Bill and Melinda Gates Foundation (OPP1114729) and National Institutes of Health NIAID and NIGMS (R01AI131975) and NIAID (P01AI120756 and R01AI129801).
Conflict of Interest Statement
The authors declare that the research was conducted in the absence of any commercial or financial relationships that could be construed as a potential conflict of interest.
The reviewer AC declared a past co-authorship with one of the authors MA to the handling editor.
References
1. Letvin NL, Daniel MD, Sehgal PK, Desrosiers RC, Hunt RD, Waldron LM, et al. Induction of AIDS-like disease in macaque monkeys with T-cell tropic retrovirus STLV-III. Science. (1985) 230:71–3. doi: 10.1126/science.2412295
2. Kestler H, Kodama T, Ringler D, Marthas M, Pedersen N, Lackner A, et al. Induction of AIDS in rhesus monkeys by molecularly cloned simian immunodeficiency virus. Science. (1990) 248:1109–12. doi: 10.1126/science.2160735
3. Hirsch VM, Dapolito G, Johnson PR, Elkins WR, London WT, Montali RJ, et al. Induction of AIDS by simian immunodeficiency virus from an African green monkey: species-specific variation in pathogenicity correlates with the extent of in vivo replication. J Virol. (1995) 69:955–67.
4. Reimann KA, Li JT, Veazey R, Halloran M, Park IW, Karlsson GB, et al. A chimeric simian/human immunodeficiency virus expressing a primary patient human immunodeficiency virus type 1 isolate env causes an AIDS-like disease after in vivo passage in rhesus monkeys. J Virol. (1996) 70:6922–8.
5. Reimann KA, Parker RA, Seaman MS, Beddall M, Peterson L, Kenneth C, et al. Pathogenicity of simian-human immunodeficiency virus SHIV-89 . 6P and SIVmac Is attenuated in cynomolgus macaques and associated with early T-lymphocyte responses pathogenicity of simian-human immunodeficiency virus SHIV-89 . 6P and SIVmac Is Attenuated i. J Virol. (2005) 79:8878–85. doi: 10.1128/JVI.79.14.8878
6. Tsai CC, Follis KE, Sabo A, Grant R, Bischofberger N. Efficacy of 9-(2-phosphonylmethoxyethyl)adenine treatment against chronic simian immunodeficiency virus infection in macaques. J Infect Dis. (1995) 171:1338–43. doi: 10.1093/infdis/171.5.1338
7. Van Rompay KK, Cherrington JM, Marthas ML, Berardi CJ, Mulato AS, Spinner A, et al. 9-[2-(Phosphonomethoxy)propyl]adenine therapy of established simian immunodeficiency virus infection in infant rhesus macaques. Antimicrob Agents Chemother. (1996) 40:2586–91. doi: 10.1128/AAC.40.11.2586
8. Wilson TJ, Fuchs A, Colonna M. Cutting edge: human FcRL4 and FcRL5 are receptors for IgA and IgG. J Immunol. (2012) 188:4741–5. doi: 10.4049/jimmunol.1102651
9. Fahrbach KM, Malykhina O, Stieh DJ, Hope TJ. Differential binding of IgG and IgA to mucus of the female reproductive tract. PLoS ONE. (2013) 8:e76176. doi: 10.1371/journal.pone.0076176
10. Foss S, Watkinson R, Sandlie I, James LC, Andersen JT. TRIM21: a cytosolic Fc receptor with broad antibody isotype specificity. Immunol Rev. (2015) 268:328–39. doi: 10.1111/imr.12363
11. Mascola JR, Lewis MG, Stiegler G, Harris D, VanCott TC, Hayes D, et al. Protection of Macaques against pathogenic simian/human immunodeficiency virus 89.6PD by passive transfer of neutralizing antibodies. J Virol. (1999) 73:4009–18.
12. Shibata R, Igarashi T, Haigwood N, Buckler-White A, Ogert R, Ross W, et al. Neutralizing antibody directed against the HIV-1 envelope glycoprotein can completely block HIV-1/SIV chimeric virus infections of macaque monkeys. Nat Med. (1999) 5:204–10. doi: 10.1038/5568
13. Mascola JR, Stiegler G, VanCott TC, Katinger H, Carpenter CB, Hanson CE, et al. Protection of macaques against vaginal transmission of a pathogenic HIV-1/SIV chimeric virus by passive infusion of neutralizing antibodies. Nat Med. (2000) 6:207–10. doi: 10.1097/00002030-200102001-00018
14. Rerks-Ngarm S, Pitisuttithum P, Nitayaphan S, Kaewkungwal J, Chiu J, Paris R, et al. Vaccination with ALVAC and AIDSVAX to Prevent HIV-1 Infection in Thailand. N Engl J Med. (2009) 361:2209–20. doi: 10.1056/NEJMoa0908492
15. Haynes BF, Gilbert PB, McElrath MJ, Zolla-Pazner S, Tomaras GD, Alam SM, et al. Immune-correlates analysis of an HIV-1 vaccine efficacy trial. N Engl J Med. (2012) 366:1275–86. doi: 10.1056/NEJMoa1113425
16. Chung AW, Ghebremichael M, Robinson H, Brown E, Choi I, Lane S, et al. Polyfunctional Fc-effector profiles mediated by IgG subclass selection distinguish RV144 and VAX003 vaccines. Sci Transl Med. (2014) 6:228–38. doi: 10.1126/scitranslmed.3007736
17. Yates NL, Liao H-X, Fong Y, deCamp A, Vandergrift NA, Williams WT, et al. Vaccine-induced Env V1-V2 IgG3 correlates with lower HIV-1 infection risk and declines soon after vaccination. Sci Transl Med. (2014) 6:228ra39. doi: 10.1126/scitranslmed.3007730
18. Perez LG, Martinez DR, DeCamp AC, Pinter A, Berman PW, Francis D, et al. V1V2-specific complement activating serum IgG as a correlate of reduced HIV-1 infection risk in RV144. PLoS ONE. (2017) 12:e180720. doi: 10.1371/journal.pone.0180720
19. Ackerman ME, Das J, Pittala S, Broge T, Linde C, Suscovich TJ, et al. Route of immunization defines multiple mechanisms of vaccine-mediated protection against SIV. Nat Med. (2018) 24:1. doi: 10.1038/s41591-018-0161-0
20. Bradley T, Pollara J, Santra S, Vandergrift N, Pittala S, Bailey-Kellogg C, et al. Pentavalent HIV-1 vaccine protects against simian-human immunodeficiency virus challenge. Nat Commun. (2017) 8:1–15. doi: 10.1038/ncomms15711
21. Barouch DH, Alter G, Broge T, Linde C, Ackerman ME, Brown EP, et al. Protective efficacy of adenovirus/protein vaccines against SIV challenges in rhesus monkeys. Science. (2015) 349:320–4. doi: 10.1126/science.aab3886
22. Fouts TR, Bagley K, Prado IJ, Bobb KL, Schwartz JA, Xu R, et al. Balance of cellular and humoral immunity determines the level of protection by HIV vaccines in rhesus macaque models of HIV infection. Proc Natl Acad Sci USA. (2015) 112:E992–9. doi: 10.1073/pnas.1423669112
23. Barouch DH, Stephenson KE, Borducchi EN, Smith K, Stanley K, et al. XProtective efficacy of a global HIV-1 mosaic vaccine against heterologous SHIV challenges in rhesus monkeys. Cell. (2013) 155:531–9. doi: 10.1016/j.cell.2013.09.061
24. Alpert MD, Harvey JD, Lauer WA, Reeves RK, Piatak M, Carville A, et al. ADCC develops over time during persistent infection with live-attenuated SIV and is associated with complete protection against SIVmac251 Challenge. PLoS Pathog. (2012) 8:e1002890. doi: 10.1371/journal.ppat.1002890
25. Bomsel M, Tudor D, Drillet AS, Alfsen A, Ganor Y, Roger MG, et al. Immunization with HIV-1 gp41 subunit virosomes induces mucosal antibodies protecting nonhuman primates against vaginal SHIV challenges. Immunity. (2011) 34:269–80. doi: 10.1016/j.immuni.2011.01.015
26. Forthal DN, Gilbert PB, Landucci G, Phan T. Recombinant gp120 vaccine-induced antibodies inhibit clinical strains of HIV-1 in the presence of Fc receptor-bearing effector cells and correlate inversely with HIV infection rate. J Immunol. (2007) 178:6596–603. doi: 10.4049/jimmunol.178.10.6596
27. Hessell AJ, Hangartner L, Hunter M, Havenith CEG, Beurskens FJ, Bakker JM, et al. Fc receptor but not complement binding is important in antibody protection against HIV. Nature. (2007) 449:101–4. doi: 10.1038/nature06106
28. Van Rompay KK, Berardi CJ, Dillard-Telm S, Tarara RP, Canfield DR, Valverde CR, et al. Passive immunization of newborn rhesus macaques prevents oral simian immunodeficiency virus infection. J Infect Dis. (1998) 177:1247–59.
29. Hessell AJ, Shapiro MB, Powell R, Malherbe DC, McBurney SP, Pandey S, et al. Reduced cell-associated DNA and improved viral control in macaques following passive transfer of a single Anti-V2 monoclonal antibody and repeated Simian/Human immunodeficiency virus challenges. J Virol. (2018) 92:1–24. doi: 10.1128/JVI.02198-17
30. Ackerman ME, Mikhailova A, Brown EP, Dowell KG, Walker BD, Bailey-Kellogg C, Suscovich TJ, et al. Polyfunctional HIV-specific antibody responses are associated with spontaneous HIV control. PLoS Pathog. (2016) 12:e1005315. doi: 10.1371/journal.ppat.1005315
31. Mohanram V, Demberg T, Musich T, Tuero I, Vargas-Inchaustegui DA, Miller-Novak L, et al. B cell responses associated with vaccine-induced delayed SIVmac251 acquisition in female rhesus macaques. J Immunol. (2016) 197:2316–24. doi: 10.4049/jimmunol.1600544
32. Santra S, Tomaras GD, Warrier R, Nicely NI, Liao HX, Pollara J, et al. Human non-neutralizing HIV-1 envelope monoclonal antibodies limit the number of founder viruses during SHIV mucosal infection in rhesus macaques. PLoS Pathog. (2015) 11:e1005042. doi: 10.1371/journal.ppat.1005042
33. Brocca-Cofano E, McKinnon K, Demberg T, Venzon D, Hidajat R, Xiao P, et al. Vaccine-elicited SIV and HIV envelope-specific IgA and IgG memory B cells in rhesus macaque peripheral blood correlate with functional antibody responses and reduced viremia. Vaccine. (2011) 29:3310–9. doi: 10.1016/j.vaccine.2011.02.066
34. Chung AW, Navis M, Isitman G, Wren L, Silvers J, Amin J, et al. Activation of NK cells by ADCC antibodies and HIV disease progression. J Acquir Immune Defic Syndr. (2011) 58:127–31. doi: 10.1097/QAI.0b013e31822c62b9
35. Lambotte O, Pollara J, Boufassa F, Moog C, Venet A, Haynes BF, et al. High antibody-dependent cellular cytotoxicity responses are correlated with strong CD8 T cell viral suppressive activity but not with B57 Status in HIV-1 Elite Controllers. PLoS ONE. (2013) 8:e74855. doi: 10.1371/journal.pone.0074855
36. Xiao P, Zhao J, Patterson LJ, Brocca-Cofano E, Venzon D, Kozlowski PA, et al. Multiple vaccine-elicited nonneutralizing antienvelope antibody activities contribute to protective efficacy by reducing both acute and chronic viremia following simian/human immunodeficiency virus SHIV89.6P challenge in rhesus macaques. J Virol. (2010) 84:7161–73. doi: 10.1128/JVI.00410-10
37. Florese RH, Demberg T, Xiao P, Kuller L, Larsen K, Summers LE, et al. Contribution of nonneutralizing vaccine-elicited antibody activities to improved protective efficacy in rhesus macaques immunized with Tat/Env compared with multigenic vaccines. J Immunol. (2009) 182:3718–27. doi: 10.4049/jimmunol.0803115
38. Hidajat R, Xiao P, Zhou Q, Venzon D, Summers LE, Kalyanaraman VS, et al. Correlation of vaccine-elicited systemic and mucosal nonneutralizing antibody activities with reduced acute viremia following intrarectal simian immunodeficiency virus SIV mac251 challenge of rhesus macaques. J Virol. (2009) 83:791–801. doi: 10.1128/JVI.01672-08
39. Lambotte O, Ferrari G, Moog C, Yates NL, Liao H-X, Parks RJ, et al. Heterogeneous neutralizing antibody and antibody-dependent cell cytotoxicity responses in HIV-1 elite controllers. AIDS. (2009) 23:897–906. doi: 10.1097/QAD.0b013e328329f97d
40. Gomez-Roman VR, Patterson LJ, Venzon D, Liewehr D, Aldrich K, Florese R, et al. Vaccine-elicited antibodies mediate antibody-dependent cellular cytotoxicity correlated with significantly reduced acute viremia in rhesus macaques challenged with SIVmac251. J Immunol. (2005) 174:2185–9. doi: 10.4049/jimmunol.174.4.2185
41. Nag P, Kim J, Sapiega V, Landay AL, Bremer JW, Mestecky J, et al. Women with cervicovaginal antibody-dependent cell-mediated cytotoxicity have lower genital HIV-1 RNA loads. J Infect Dis. (2004) 190:1970–8. doi: 10.1086/425582
42. Ahmad R, Sindhu STAK, Toma E, Morisset R, Vincelette J, Menezes J, et al. Evidence for a correlation between antibody-dependent cellular cytotoxicity-mediating anti-HIV-1 antibodies and prognostic predictors of HIV infection. J Clin Immunol. (2001) 21:227–33. doi: 10.1023/A:1011087132180
43. Forthal DN, Landucci G, Keenan B. Relationship between antibody-dependent cellular cytotoxicity, plasma HIV type 1 RNA, and CD4+ lymphocyte count. AIDS Res Hum Retroviruses. (2001) 17:553–61. doi: 10.1089/08892220151126661
44. Forthal DN, Landucci G, Daar ES. Antibody from Patients with Acute Human Immunodeficiency Virus (HIV) Infection Inhibits Primary Strains of HIV Type 1 in the presence of natural-killer effector cells. Society. (2001) 75:6953–61. doi: 10.1128/JVI.75.15.6953
45. Ahmad A, Morisset R, Thomas R, Menezes J. Evidence for a defect of antibody-dependent cellular cytotoxic (ADCC) effector function and anti-HIV gp120/41-specific ADCC-mediating antibody titres in HIV-infected individuals. J Acquir Immune Defic Syndr. (1994) 7:428–37.
46. Sawyer LA, Katzenstein DA, Hendry RM, Boone EJ, Vujcic LK, Williams CC, et al. Possible beneficial effects of neutralizing antibodies and antibody-dependent, cell-mediated cytotoxicity in human immunodeficiency virus infection. AIDS Res Hum Retroviruses. (1990) 6:341–56. doi: 10.1089/aid.1990.6.341
47. Madhavi V, Wines BD, Amin J, Emery S, ENCORE1 Study Group, Lopez E, et al. HIV-1 Env- and Vpu-Specific antibody-dependent cellular cytotoxicity responses associated with elite control of HIV. J Virol. (2017) 91:1–16. doi: 10.1128/JVI.00700-17
48. Wren LH, Chung AW, Isitman G, Kelleher AD, Parsons MS, Amin J, et al. Specific antibody-dependent cellular cytotoxicity responses associated with slow progression of HIV infection. Immunology. (2013) 138:116–23. doi: 10.1111/imm.12016
49. Banks ND, Kinsey N, Clements J, Hildreth JEK. Sustained antibody-dependent cell-mediated cytotoxicity (ADCC) in SIV-infected macaques correlates with delayed progression to AIDS. AIDS Res Hum Retroviruses. (2002) 18:1197–205. doi: 10.1089/08892220260387940
50. Tranchat C, Van de Perre P, Simonon-Sorel A, Karita E, Benchaïb M, Lepage P, et al. Maternal humoral factors associated with perinatal human immunodeficiency virus type-1 transmission in a cohort from Kigali, Rwanda, 1988-1994. J Infect. (1999) 39:213–20. doi: 10.1016/S0163-4453(99)90052-X
51. Baum LL, Cassutt KJ, Knigge K, Khattri R, Margolick J, Rinaldo C, et al. HIV-1 gp120-specific antibody-dependent cell-mediated cytotoxicity correlates with rate of disease progression. J Immunol. (1996) 157:2168–73.
52. Broliden K, Sievers E, Tovo PA, Moschese V, Scarlatti G, Broliden PA, et al. Antibody-dependent cellular cytotoxicity and neutralizing activity in sera of HIV-1-infected mothers and their children. Clin Exp Immunol. (1993) 93:56–64. doi: 10.1111/j.1365-2249.1993.tb06497.x
53. Ljunggren K, Moschese V, Broliden PA, Giaquinto C, Quinti I, Fenyo EM, et al. Antibodies mediating cellular cytotoxicity and neutralization correlate with a better clinical stage in children born to human immunodeficiency virus-infected mothers. J Infect Dis. (1990) 161:198–202. doi: 10.1093/infdis/161.2.198
54. Goudsmit J, Ljunggren K, Smit L, Jondal M, Fenyö EM, Jonda M. Biological significance of the antibody response to HIV antigens expressed on the cell surface. Arch Virol. (1988) 103:189–206.
55. Ljunggren K, Böttiger B, Biberfeld G, Karlson A, Fenyö EM, Jondal M. Antibody-dependent cellular cytotoxicity-inducing antibodies against human immunodeficiency virus. Presence at different clinical stages. J Immunol. (1987) 139:2263–7.
56. Rook AH, Lane HC, Folks T, McCoy S, Alter H, Fauci AS. Sera from HTLV-III/LAV antibody-positive individuals mediate antibody-dependent cellular cytotoxicity against HTLV-III/LAV-infected T cells. J Immunol. (1987) 138:1064–7.
57. Milligan C, Richardson BA, John-Stewart G, Nduati R, Overbaugh J. Passively Acquired Antibody-Dependent Cellular Cytotoxicity (ADCC) activity in HIV-infected infants is associated with reduced mortality. Cell Host Microbe. (2015) 17:500–6. doi: 10.1016/j.chom.2015.03.002
58. Forthal DN, Landucci G, Haubrich R, Keenan B, Kuppermann BD, Tilles JG, et al. Antibody-dependent cellular cytotoxicity independently predicts survival in severely immunocompromised human immunodeficiency virus-infected patients. J Infect Dis. (1999) 180:1338–41.
59. Parsons MS, Lee WS, Kristensen AB, Amarasena T, Khoury G, Wheatley AK, et al. Fc-dependent functions are redundant to efficacy of anti-HIV antibody PGT121 in macaques. J Clin Invest. (2018) 129:182–91. doi: 10.1172/JCI122466
60. Astronomo RD, Santra S, Ballweber-Fleming L, Westerberg KG, Mach L, Hensley-McBain T, et al. Neutralization Takes Precedence Over IgG or IgA Isotype-related Functions in Mucosal HIV-1 antibody-mediated protection. EBioMedicine. (2016) 14:97–111. doi: 10.1016/j.ebiom.2016.11.024
61. Dugast AS, Chan Y, Hoffner M, Licht A, Nkolola J, Li H, et al. Lack of protection following passive transfer of polyclonal highly functional low-dose non-neutralizing antibodies. PLoS ONE. (2014) 9:e97229. doi: 10.1371/journal.pone.0097229
62. Nakane T, Nomura T, Shi S, Nakamura M, Naruse TK, Kimura A. Limited impact of passive non-neutralizing antibody immunization in acute SIV infection on viremia control in rhesus macaques. PLoS ONE. (2013) 8:e73453. doi: 10.1371/journal.pone.0073453
63. Smith AJ, Wietgrefe SW, Shang L, Reilly CS, Southern PJ, Perkey KE, et al. Live simian immunodeficiency virus vaccine correlate of protection: immune complex-inhibitory Fc receptor interactions that reduce target cell availability. J Immunol. (2014) 193:3126–33. doi: 10.4049/jimmunol.1400822
64. Moldt B, Shibata-Koyama M, Rakasz EG, Schultz N, Kanda Y, Dunlop DC, et al. A nonfucosylated variant of the anti-HIV-1 monoclonal antibody b12 has enhanced FcγRIIIa-mediated antiviral activity in vitro but does not improve protection against mucosal SHIV challenge in macaques. J Virol. (2012) 86:6189–96. doi: 10.1128/JVI.00491-12
65. Burton DR, Hessell AJ, Keele BF, Johan P, Ketas TA, Moldt B. Limited or no protection by weakly or nonneutralizing antibodies against vaginal SHIV challenge of macaques compared with a strongly neutralizing antibody. Proc Natl Acad Sci USA. (2011) 108:11181–6. doi: 10.1073/pnas.1103012108
66. Jenkins M, Landers D, Williams-Herman D, Wara D, Viscarello RR, Hammill HA, et al. Association between Anti-Human Immunodeficiency Virus Type 1 (HIV-1) antibody-dependent cellular cytotoxicity antibody titers at birth and vertical transmission of HIV-1. J Infect Dis. (1994) 170:308–12. doi: 10.2307/30134706
67. Dalgleish A, Sinclair A, Steel M, Beatson D, Ludlam C, Habeshaw J. Failure of ADCC to predict HIV-associated disease progression or outcome in a haemophiliac cohort. Clin Exp Immunol. (1990) 81:5–10. doi: 10.1111/j.1365-2249.1990.tb05283.x
68. Ojo-Amaize E, Nishanian PG, Heitjan DF, Rezai A, Esmail I, Korns E, et al. Serum and effector-cell antibody-dependent cellular cytotoxicity (ADCC) activity remains high during human immunodeficiency virus (HIV) disease progression. J Clin Immunol. (1989) 9:454–61. doi: 10.1007/BF00918014
69. Pietzsch J, Gruell H, Bournazos S, Donovan BM, Klein F, Diskin R, et al. A mouse model for HIV-1 entry. Proc Natl Acad Sci USA. (2012) 109:15859–64. doi: 10.1073/pnas.1213409109
70. Bournazos S, Klein F, Pietzsch J, Seaman MS, Nussenzweig MC, Ravetch JV. Broadly Neutralizing Anti-HIV-1 antibodies require fc effector functions for in vivo activity. Cell. (2014) 158:1243–53. doi: 10.1016/j.cell.2014.08.023
71. Lu C-L, Murakowski DK, Bournazos S, Schoofs T, Sarkar D, Halper-Stromberg A, et al. Enhanced clearance of HIV-1-infected cells by broadly neutralizing antibodies against HIV-1 in vivo. Science. (2016) 352:1001–4. doi: 10.1126/science.aaf1279
72. Ackerman ME, Nimmerjahn F editors. Antibody Fc. London: Elsevier. (2014). doi: 10.1016/C2011-0-07091-6
73. Junghans RP, Anderson CL. The protection receptor for IgG catabolism is the beta2-microglobulin-containing neonatal intestinal transport receptor. Proc Natl Acad Sci USA. (1993) 93:5512–6. doi: 10.1073/pnas.93.11.5512
74. Qiao S-W, Kobayashi K, Johansen F-E, Sollid LM, Andersen JT, Milford E, et al. Dependence of antibody-mediated presentation of antigen on FcRn. Proc Natl Acad Sci USA. (2008) 105:9337–42. doi: 10.1073/pnas.0801717105
75. Baker K, Qiao S-W, Kuo TT, Aveson VG, Platzer B, Andersen J-T, et al. Neonatal Fc receptor for IgG (FcRn) regulates cross-presentation of IgG immune complexes by CD8-CD11b+ dendritic cells. Proc Natl Acad Sci USA. (2011) 108:9927–32. doi: 10.1073/pnas.1019037108
76. Vowels BR, Gershwin ME, Gardner MB, McGraw TP. Natural killer cell activity of rhesus macaques against retrovirus-pulsed CD4+ target cells. AIDS Res Hum Retroviruses. (1990) 6:905–18. doi: 10.1089/aid.1990.6.905
77. Warncke M, Calzascia T, Coulot M, Balke N, Touil R, Kolbinger F, et al. Different adaptations of IgG effector function in human and nonhuman primates and implications for therapeutic antibody treatment. J Immunol. (2012) 188:4405–11. doi: 10.4049/jimmunol.1200090
78. Vidarsson G, Dekkers G, Rispens T. IgG subclasses and allotypes: from structure to effector functions. Front Immunol. (2014) 5:520. doi: 10.3389/fimmu.2014.00520
79. Scinicariello F, Engleman CN, Jayashankar L, McClure HM, Attanasio R. Rhesus macaque antibody molecules: sequences and heterogeneity of alpha and gamma constant regions. Immunology. (2004) 111:66–74. doi: 10.1111/j.1365-2567.2004.01767.x
80. Boesch AW, Osei-Owusu NY, Crowley AR, Chu TH, Chan YN, Weiner JA, et al. Biophysical and Functional Characterization of Rhesus Macaque IgG Subclasses. Front Immunol. (2016) 7:589. doi: 10.3389/fimmu.2016.00589
81. Saluk PH, Clem LW. The unique molecular weight of the heavy chain from human IgG3. J Immunol. (1971) 107:298–301.
82. Michaelsen TE, Frangione B, Franklin EC. Primary structure of the “hinge” region of human IgG3. Probable quadruplication of a 15-amino acid residue basic unit. J Biol Chem. (1977) 252:883–9.
83. Dillon TM, Ricci MS, Vezina C, Flynn GC, Liu YD, Rehder DS, et al. Structural and functional characterization of disulfide isoforms of the human IgG2 subclass. J Biol Chem. (2008) 283:16206–15. doi: 10.1074/jbc.M709988200
84. Ferrante A, Beard LJ, Feldman RG. IgG subclass distribution of antibodies to bacterial and viral antigens. Pediatr Infect Dis J. (1990) 9:S16–24.
85. Bruhns P, Iannascoli B, England P, Mancardi DA, Fernandez N, Jorieux S, et al. Specificity and affinity of human Fcgamma receptors and their polymorphic variants for human IgG subclasses. Blood. (2009) 113:3716–25. doi: 10.1182/blood-2008-09-179754
86. Collins AM, Jackson KJL. A temporal model of human IgE and IgG antibody function. Front Immunol. (2013) 4:235. doi: 10.3389/fimmu.2013.00235
87. van der Neut Kolfschoten M, Schuurman J, Losen M, Bleeker WK, Martinez-Martinez P, Vermeulen E, et al. Anti-inflammatory activity of human IgG4 antibodies by dynamic fab arm exchange. Science. (2007) 317:1554–7. doi: 10.1126/science.1144603
88. Labrijn AF, Buijsse AO, Van Den Bremer ETJ, Verwilligen AYW, Bleeker WK, Thorpe SJ, et al. Therapeutic IgG4 antibodies engage in Fab-arm exchange with endogenous human IgG4 in vivo. Nat Biotechnol. (2009) 27:767–71. doi: 10.1038/nbt.1553
89. Gehlhar K, Schlaak M, Becker WM, Bufe A. Monitoring allergen immunotherapy of pollen-allergic patients: the ratio of allergen-specific IgG4 to IgG1 correlates with clinical outcome. Clin Exp Allergy. (1999) 29:497–506. doi: 10.1046/j.1365-2222.1999.00525.x
90. Torres Lima M, Wilson D, Pitkin L, Roberts A, Nouri-Aria K, Jacobson M, Walker S, et al. Grass pollen sublingual immunotherapy for seasonal rhinoconjunctivitis: a randomized controlled trial. Clin Exp Allergy. (2002) 32:507–14. doi: 10.1046/j.0954-7894.2002.01327.x
91. Matsui EC, Diette GB, Krop EJM, Aalberse RC, Smith AL, Curtin-Brosnan J, et al. Mouse allergen-specific immunoglobulin G and immunoglobulin G4 and allergic symptoms in immunoglobulin E-sensitized laboratory animal workers. Clin Exp Allergy. (2005) 35:1347–53. doi: 10.1111/j.1365-2222.2005.02331.x
92. Boonpiyathad T, Meyer N, Moniuszko M, Sokolowska M, Eljaszewicz A, Wirz OF, et al. High-dose bee venom exposure induces similar tolerogenic B-cell responses in allergic patients and healthy beekeepers. Allergy Eur J Allergy Clin Immunol. (2017) 72:407–15. doi: 10.1111/all.12966
93. Jacobsen FW, Padaki R, Morris AE, Aldrich TL, Armitage RJ, Allen MJ, et al. Molecular and functional characterization of cynomolgus monkey IgG subclasses. J Immunol. (2011) 186:341–9. doi: 10.4049/jimmunol.1001685
94. Martin LN. Chromatographic fractionation of rhesus monkey (Macaca mulatta) IgG subclasses using DEAE cellulose and protein A-Sepharose. J Immunol Methods. (1982) 50:319–29. doi: 10.1016/0022-1759(82)90170-3
95. Ramesh A, Darko S, Hua A, Overman G, Ransier A, Francica JR, et al. Structure and diversity of the rhesus macaque immunoglobulin loci through multiple de novo genome assemblies. Front Immunol. (2017) 8:407. doi: 10.3389/fimmu.2017.01407
96. Nguyen DC, Sanghvi R, Scinicariello F, Pulit-Penaloza J, Hill N, Attanasio R. Cynomolgus and pigtail macaque IgG subclasses: Characterization of IGHG genes and computational analysis of IgG/Fc receptor binding affinity. Immunogenetics. (2014) 66:361–77. doi: 10.1007/s00251-014-0775-4
97. Calvas P, Apoil P, Fortenfant F, Roubinet F, Andris J, Capra D, et al. Characterization of the three immunoglobulin G subclasses of macaques. Scand J Immunol. (1999) 49:595–610. doi: 10.1046/j.1365-3083.1999.00540.x
98. Dugast A-S, Stamatatos L, Tonelli A, Suscovich TJ, Licht AF, Mikell I, Ackerman ME, et al. Independent evolution of Fc- and Fab-mediated HIV-1-specific antiviral antibody activity following acute infection. Eur J Immunol. (2014) 44:2925–37. doi: 10.1002/eji.201344305
99. Karnasuta C, Akapirat S, Madnote S, Savadsuk H, Puangkaew J, Rittiroongrad S, et al. Comparison of antibody responses induced by RV144, VAX003, and VAX004 vaccination regimens. AIDS Res Hum Retroviruses. (2017) 33:410–23. doi: 10.1089/aid.2016.0204
100. Chan YN, Boesch AW, Osei-Owusu NY, Emileh A, Crowley AR, Cocklin SL, et al. IgG binding characteristics of rhesus macaque FcγR. J Immunol. (2016) 197:2936–47. doi: 10.4049/jimmunol.1502252
101. Duncan AR, Woof JM, Partridge LJ, Burton DR, Winter G. Localization of the binding site for the human high-affinity Fc receptor on IgG. Nature. (1988) 332:563–4. doi: 10.1038/332563a0
102. Lund J, Winter G, Jones PT, Pound JD, Tanaka T, Walker MR, et al. Human Fc gamma RI and Fc gamma RII interact with distinct but overlapping sites on human IgG. J Immunol. (1991) 147:2657–62.
103. Lund J, Pound JD, Jones PT, Duncan AR, Bentley T, Goodall M, et al. Multiple binding sites on the CH2 domain of IgG for mouse FcγR11. Mol Immunol. (1992) 29:53–9. doi: 10.1016/0161-5890(92)90156-R
104. Sarmay G, Lund J, Rozsnyay Z, Gergely J, Jefferis R. Mapping and comparison of the interaction sites on the Fc region of IgG responsible for triggering antibody dependent cellular cytotoxicity (ADCC) through different types of human Fcγ receptor. Mol Immunol. (1992) 29:633–9. doi: 10.1016/0161-5890(92)90200-H
105. Morgan A, Jones ND, Nesbitt AM, Chaplin L, Bodmer MW, Emtage JS. The N-terminal end of the CH2 domain of chimeric human IgG1 anti-HLA-DR is necessary for C1q, Fc gamma RI and Fc gamma RIII binding. Immunology. (1995) 86:319–24.
106. Koolwijk P, Spierenburg GT, Frasa H, Boot JH, van de Winkel JG, Bast BJ. Interaction between hybrid mouse monoclonal antibodies and the human high-affinity IgG FCR, huFc gamma RI, on U937. Involvement of only one of the mIgG heavy chains in receptor binding. J Immunol. (1989) 143:1656–62.
107. Ghirlando R, Keown MB, Mackay GA, Lewis MS, Unkeless JC, Gould HJ. Stoichiometry and thermodynamics of the interaction between the Fc fragment of human IgG1 and its low-affinity receptor FcγRIII. Biochemistry. (1995) 34:13320–7. doi: 10.1021/bi00041a007
108. Kato K, Sautès-Fridman C, Yamada W, Kobayashi K, Uchiyama S, Kim H, et al. Structural basis of the interaction between IgG and Fcγ receptors. J Mol Biol. (2000) 295:3351. doi: 10.1006/jmbi.1999.3351
109. Radaev S, Motyka S, Fridman WH, Sautes-Fridman C, Sun PD. The structure of a human type III Fcgamma receptor in complex with Fc. J Biol Chem. (2001) 276:16469–77. doi: 10.1074/jbc.M100350200
110. Nimmerjahn F, Ravetch JV. Fcgamma receptors as regulators of immune responses. Nat Rev Immunol. (2008) 8:34–47. doi: 10.1038/nri2206
111. Getahun A, Cambier JC. Of ITIMs, ITAMs, and ITAMis: Revisiting immunoglobulin Fc receptor signaling. Immunol Rev. (2015) 268:66–73. doi: 10.1111/imr.12336
112. Bournazos S. IgG Fc receptors: evolutionary considerations. In: Ahmed R, Aktories K, Casadevall A, Compans RW, Galan JE, Garcia-Sastre A, Malissen B, Palme K, Rappuoli R, Iwasaki A, Akira S, editors. Current Topics in Microbiology and Immunology. Berlin; Heidelberg: Springer (2019). p. 1–11. doi: 10.1007/82_2019_149
113. Ernst LK, Duchemin AM, Anderson CL. Association of the high-affinity receptor for IgG (Fc gamma RI) with the gamma subunit of the IgE receptor. Proc Natl Acad Sci USA. (1993) 90:6023–7. doi: 10.1073/pnas.90.13.6023
114. Scholl PR, Geha RS. Physical association between the high-affinity IgG receptor (Fc gamma RI) and the gamma subunit of the high-affinity IgE receptor (Fc epsilon RI gamma). Proc Natl Acad Sci USA. (1993) 90:8847–50. doi: 10.1073/pnas.90.19.8847
115. van de Winkel JG, de Wit TP, Ernst LK, Capel PJ, Ceuppens JL. Molecular basis for a familial defect in phagocyte expression of IgG receptor I (CD64). J Immunol. (1995) 154:2896–903.
116. Indik ZK, Hunter S, Huang MM, Pan XQ, Chien P, Kelly C, et al. The high affinity Fc gamma receptor (CD64) induces phagocytosis in the absence of its cytoplasmic domain: the gamma subunit of Fc gamma RIIIA imparts phagocytic function to Fc gamma RI. Exp Hematol. (1994) 22:599–606.
117. Fanger NNA, Voigtlaender D, Liu C, Swink S, Wardwell K, Fisher J, et al. Characterization of expression, cytokine regulation, and effector function of the high affinity IgG receptor Fc gamma RI (CD64) expressed on human blood dendritic cells. J Immunol. (1997) 158:3090–8.
118. Okayama Y, Hagaman DD, Metcalfe DD. A Comparison of mediators released or generated by IFN- -treated human mast cells following aggregation of Fc RI or Fc RI. J Immunol. (2001) 166:4705–12. doi: 10.4049/jimmunol.166.7.4705
119. O'Doherty U, Ignatius R, Bhardwaj N, Pope M. Generation of monocyte-derived dendritic cells from precursors in rhesus macaque blood. J Immunol Methods. (1997) 207:185–94. doi: 10.1016/S0022-1759(97)00119-1
120. Carter DL, Shieh TM, Blosser RL, Chadwick KR, Margolick JB, Hildreth JE, et al. CD56 identifies monocytes and not natural killer cells in rhesus macaques. Cytometry. (1999) 37:41–50. doi: 10.1002/(SICI)1097-0320(19990901)37:1<41::AID-CYTO5>3.0.CO;2-4
121. Starbeck-Miller GR, Badovinac VP, Barber DL, Harty JT. Cutting edge: expression of Fc RIIB tempers memory CD8 t cell function in vivo. J Immunol. (2014) 192:35–9. doi: 10.4049/jimmunol.1302232
122. Chauhan AK, Chen C, Moore TL, DiPaolo RJ. Induced expression of FcγRIIIa (CD16a) on CD4+ T cells triggers generation of IFN-γhigh subset. J Biol Chem. (2015) 290:5127–40. doi: 10.1074/jbc.M114.599266
123. Anselmino LM, Perussia B, Thomas LL. Human basophils selectively express the FcγRII (CDw32) subtype of IgG receptor. J Allergy Clin Immunol. (1989) 84:907–14. doi: 10.1016/0091-6749(89)90388-6
124. Rogers KA, Scinicariello F, Attanasio R. IgG Fc receptor III homologues in nonhuman primate species: genetic characterization and ligand interactions. J Immunol. (2006) 177:3848–56. doi: 10.4049/jimmunol.177.6.3848
125. Zhao W, Kepley CL, Morel PA, Okumoto LM, Fukuoka Y, Schwartz LB. Fc RIIa, Not Fc RIIb, is constitutively and functionally expressed on skin-derived human mast cells. J Immunol. (2006) 177:694–701. doi: 10.4049/jimmunol.177.1.694
126. Choi EI, Wang R, Peterson L, Letvin NL, Reimann KA. Use of an anti-CD16 antibody for in vivo depletion of natural killer cells in rhesus macaques. Immunology. (2008) 124:215–22. doi: 10.1111/j.1365-2567.2007.02757.x
127. Meknache N, Jonsson F, Laurent J, Guinnepain M-T, Daeron M. Human basophils express the glycosylphosphatidylinositol-anchored low-affinity IgG Receptor Fc RIIIB (CD16B). J Immunol. (2009) 182:2542–50. doi: 10.4049/jimmunol.0801665
128. Cady CT, Powell MS, Harbeck RJ, Giclas PC, Murphy JR, Katial RK, et al. IgG antibodies produced during subcutaneous allergen immunotherapy mediate inhibition of basophil activation via a mechanism involving both FcγRIIA and FcγRIIB. Immunol Lett. (2010) 130:57–65. doi: 10.1016/j.imlet.2009.12.001
129. Cassard L, Jönsson F, Arnaud S, Daëron M. Fcγ Receptors inhibit mouse and human basophil activation. J Immunol. (2012) 189:2995–3006. doi: 10.4049/jimmunol.1200968
130. Perussia B, Dayton ET, Lazarus R, Fanning V, Trinchieri G. Immune interferon induces the receptor for monomeric IgG1 on human monocytic and myeloid cells. J Exp Med. (1983) 158:1092–113. doi: 10.1084/jem.158.4.1092
131. Okayama Y, Kirshenbaum AS, Metcalfe DD. Expression of a functional high-affinity IgG Receptor, FcγRI, on human mast cells: up-regulation by IFN-γ. J Immunol. (2000) 164:4332–39. doi: 10.4049/jimmunol.164.8.4332
132. Veri MC, Gorlatov S, Li H, Burke S, Johnson S, Stavenhagen J, et al. Monoclonal antibodies capable of discriminating the human inhibitory Fcγ-receptor IIB (CD32B) from the activating Fcγ-receptor IIA (CD32A): Biochemical, biological and functional characterization. Immunology. (2007) 121:392–404. doi: 10.1111/j.1365-2567.2007.02588.x
133. Sestak K, Scheiners C, Wu XW, Hollemweguer E. Identification of anti-human CD antibodies reactive with rhesus macaque peripheral blood cells. Vet Immunol Immunopathol. (2007) 119:21–6. doi: 10.1016/j.vetimm.2007.06.011
134. Metes D, Ernst LK, Chambers WH, Sulica A, Herberman RB, Morel PA. Expression of functional CD32 molecules on human NK cells is determined by an allelic polymorphism of the FcgammaRIIC gene. Blood. (1998) 91:2369–80.
135. Li X, Wu J, Ptacek T, Redden DT, Brown EE, Alarcon GS, et al. Allelic-dependent expression of an activating Fc receptor on B cells enhances humoral immune responses. Sci Transl Med. (2013) 5:216ra175-216ra175. doi: 10.1126/scitranslmed.3007097
136. Chappel MS, Isenman DE, Everett M, Xu YY, Dorrington KJ, Klein MH. Identification of the Fc gamma receptor class I binding site in human IgG through the use of recombinant IgG1/IgG2 hybrid and point-mutated antibodies. Proc Natl Acad Sci USA. (1991) 88:9036–40. doi: 10.1073/pnas.88.20.9036
137. Morell A, Skvaril F, van Loghem E, Kleemola M. Human IgG subclasses in maternal and fetal serum. Vox Sang. (1971) 21:481–92.
138. van der Giessen M, Rossouw E, van Veen TA, van Loghem E, Zegers BJ, Sander PC. Quantification of IgG subclasses in sera of normal adults and healthy children between 4 and 12 years of age. Clin Exp Immunol. (1975) 21:501–9.
139. Irani V, Guy AJ, Andrew D, Beeson JG, Ramsland PA, Richards JS. Molecular properties of human IgG subclasses and their implications for designing therapeutic monoclonal antibodies against infectious diseases. Mol Immunol. (2015) 67:171–82. doi: 10.1016/j.molimm.2015.03.255
140. Bruhns P. Properties of mouse and human IgG receptors and their contribution to disease models. Blood. (2012) 119:5640–9. doi: 10.1182/blood-2012-01-380121
141. Clark MR, Clarkson SB, Ory PA, Stollman N, Goldstein IM. Molecular basis for a polymorphism involving Fc receptor II on human monocytes. J Immunol. (1989) 143:1731–4.
142. Sanders LA, Feldman RG, Voorhorst-Ogink MM, de Haas M, Rijkers GT, Capel PJ, et al. Human immunoglobulin G (IgG) Fc Receptor IIA (CD32) Polymorphism and IgG2-Mediated Bacterial Phagocytosis by neutrophils. Infect Immun. (1995) 63:73–81.
143. Warmerdam PA, van de Winkel JG, Gosselin EJ, Capel PJ. Molecular basis for a polymorphism of human Fc gamma receptor II (CD32). J Exp Med. (1990) 172:19–25. doi: 10.1084/jem.172.1.19
144. Mellor JD, Brown MP, Irving HR, Zalcberg JR, Dobrovic A. A critical review of the role of Fc gamma receptor polymorphisms in the response to monoclonal antibodies in cancer. J Hematol Oncol. (2013) 6:1. doi: 10.1186/1756-8722-6-1
145. Platonov, Kuijper, Vershinina, Shipulin, Westerdaal, Fijen, Van DE Winkel. Meningococcal disease and polymorphism of FcgammaRIIa (CD32) in late complement component-deficient individuals. Clin Exp Immunol. (1998) 111:97–101. doi: 10.1046/j.1365-2249.1998.00484.x
146. Yee a M, Phan HM, Zuniga R, Salmon JE, Musher DM. Association between Fc gamma RIIa-R131 allotype and bacteremic pneumococcal pneumonia. Clin Infect Dis. (2000) 30:25–8. doi: 10.1086/313588
147. Endeman H, Cornips MC, Grutters JC, van den Bosch JM, Ruven HJ, van Velzen-Blad H, et al. The Fcgamma receptor IIA-R/R131 genotype is associated with severe sepsis in community-acquired pneumonia. Clin Vaccine Immunol. (2009) 16:1087–90. doi: 10.1128/cvi.00037-09
148. Forthal DN, Landucci G, Bream J, Jacobson LP, Phan TB, Montoya B. FcγRIIa Genotype Predicts Progression of HIV Infection. J Immunol. (2007) 179:7916–23. doi: 10.4049/jimmunol.179.11.7916
149. Forthal DN, Gabriel EE, Wang A, Landucci G, Phan TB. Association of Fcγ receptor IIIa genotype with the rate of HIV infection after gp120 vaccination. Blood. (2012) 120:2836–42. doi: 10.1182/blood-2012-05-431361
150. Osborne JM, Chacko GW, Brandt JT, Anderson CL. Ethnic variation in frequency of an allelic polymorphism of human FcγRIIA determined with allele specific oligonucleotide probes. J Immunol Methods. (1994) 173:207–17. doi: 10.1016/0022-1759(94)90299-2
151. Norsworthy P, Theodoridis E, Botto M, Athanassiou P, Beynon H, Gordon C, et al. Overrepresentation of the Fcγ receptor type IIA R131/R131 genotype in Caucasoid systemic lupus erythematosus patients with autoantibodies to C1q and glomerulonephritis. Arthritis Rheum. (1999) 42:1828–32. doi: 10.1002/1529-0131(199909)42:9<1828::AID-ANR6>3.0.CO;2-F
152. Ramsland PA, Farrugia W, Bradford TM, Sardjono CT, Esparon S, Trist HM, et al. Structural Basis for Fc{gamma}RIIa Recognition of Human IgG and formation of inflammatory signaling complexes. J Immunol. (2011) 187:3208–17. doi: 10.4049/jimmunol.1101467
153. Boesch AW, Miles AR, Chan YN, Osei-Owusu NY, Ackerman ME. IgG Fc variant cross-reactivity between human and rhesus macaque FcγRs. MAbs. (2017) 9:455–65. doi: 10.1080/19420862.2016.1274845
154. Haj AK, Arbanas JM, Yamniuk AP, Karl JA, Bussan HE, Drinkwater KY, et al. Characterization of mauritian cynomolgus macaque FcγR alleles using long-read sequencing. J Immunol. (2019) 202:151–9. doi: 10.4049/jimmunol.1800843
155. Richards JO, Karki S, Lazar GA, Chen H, Dang W, Desjarlais JR. Optimization of antibody binding to FcgammaRIIa enhances macrophage phagocytosis of tumor cells. Mol Cancer Ther. (2008) 7:2517–27. doi: 10.1158/1535-7163.MCT-08-0201
156. Trist HM, Tan PS, Wines BD, Ramsland PA, Orlowski E, Stubbs J, et al. Polymorphisms and Interspecies Differences of the Activating and Inhibitory Fc RII of macaca nemestrina influence the binding of human IgG subclasses. J Immunol. (2014) 192:792–803. doi: 10.4049/jimmunol.1301554
157. Ravetch JV, Perussia B. Alternative membrane forms of Fc gamma RIII(CD16) on human natural killer cells and neutrophils. Cell type-specific expression of two genes that differ in single nucleotide substitutions. J Exp Med. (1989) 170:481–97.
158. Wu J, Edberg JC, Redecha PB, Bansal V, Guyre PM, Coleman K, et al. A novel polymorphism of FcgammaRIIIa (CD16) alters receptor function and predisposes to autoimmune disease. J Clin Invest. (1997) 100:1059–70. doi: 10.1172/JCI119616
159. Leppers-van de Straat FG, Jansen M, Kobayashi T, Yoshie H, Sugita N, van de Winkel JG, et al. A novel PCR-based method for direct Fcγ receptor IIIa (CD16) allotyping. J Immunol Methods. (2002) 242:127–32. doi: 10.1016/s0022-1759(00)00240-4
160. Weng WK, Levy R. Two immunoglobulin G fragment C receptor polymorphisms independently predict response to rituximab in patients with follicular lymphoma. J Clin Oncol. (2003) 21:3940–7. doi: 10.1200/JCO.2003.05.013
161. Ghielmini M, Schmitz SFH, Cogliatti S, Bertoni F, Waltzer U, Fey MF, et al. Effect of single-agent rituximab given at the standard schedule or as prolonged treatment in patients with mantle cell lymphoma: a study of the Swiss group for clinical cancer research (SAKK). J Clin Oncol. (2005) 23:705–11. doi: 10.1200/JCO.2005.04.164
162. Friedberg JW. Unique toxicities and resistance mechanisms associated with monoclonal antibody therapy. ASH Educ B. (2005) 2005:329–34. doi: 10.1182/asheducation-2005.1.329
163. Miller CJ, Genescà M, Abel K, Montefiori D, Forthal D, Bost K, et al. Antiviral antibodies are necessary for control of simian immunodeficiency virus replication. J Virol. (2007) 81:5024–35. doi: 10.1128/JVI.02444-06
164. Morgan AW, Griffiths B, Ponchel F, Montague BMN, Ali M, Gardner PP, et al. Fcγ receptor type IIIA is associated with rheumatoid arthritis in two distinct ethnic groups. Arthritis Rheum. (2000) 43:2328–34. doi: 10.1002/1529-0131(200010)43:10<2328::AID-ANR21>3.0.CO;2-Z
165. Aksu K, Kitapcioglu G, Keser G, Berdeli A, Karabulut G, Kobak S, et al. FcgammaRIIa, IIIa and IIIb gene polymorphisms in Behçet's disease: do they have any clinical implications? Clin Exp Rheumatol. (2008) 26:S77–83.
166. Gulen F, Tanac R, Altinoz S, Berdeli A, Zeyrek D, Koksoy H, et al. The Fc gammaRIIa polymorphism in Turkish children with asthma bronchial and allergic rhinitis. Clin Biochem. (2007) 40:392–6. doi: 10.1016/j.clinbiochem.2006.11.014
167. Lehrnbecher TL, Foster CB, Zhu S, Venzon D, Steinberg SM, Wyvill K, et al. Variant genotypes of FcgammaRIIIA influence the development of Kaposi's sarcoma in HIV-infected men. Blood. (2000) 95:2386–90.
168. Selvaraj P, Rosse WF, Silber R, Springer TA. The major Fc receptor in blood has a phosphatidylinositol anchor and is deficient in paroxysmal nocturnal haemoglobinuria. Nature. (1988) 333:565–7. doi: 10.1038/333565a0
169. Treffers LW, van Houdt M, Bruggeman CW, Heineke MH, Zhao XW, vander Heijden J, et al. FcγRIIIb restricts antibody-dependent destruction of cancer cells by human neutrophils. Front Immunol. (2018) 9:3124. doi: 10.3389/fimmu.2018.03124
170. Fernandes MJG, Rollet-Labelle E, Paré G, Marois S, Tremblay M-L, Teillaud J-L, et al. CD16b associates with high-density, detergent-resistant membranes in human neutrophils. Biochem J. (2006) 393:351–9. doi: 10.1042/BJ20050129
171. Galon J, Gauchat JF, Mazières N, Spagnoli R, Storkus W, Lötze M, Bonnefoy JY, et al. Soluble Fcgamma receptor type III (FcgammaRIII, CD16) triggers cell activation through interaction with complement receptors. J Immunol. (1996) 157:1184–92.
172. Chuang FY, Sassaroli M, Unkeless JC. Convergence of Fc gamma receptor IIA and Fc gamma receptor IIIB signaling pathways in human neutrophils. J Immunol. (2000) 164:350–60. doi: 10.4049/jimmunol.164.1.350
173. Vossebeld PJM, Kessler J, von dem Borne AEGK, Roos D, Verhoeven AJ. Heterotypic FcyR clusters evoke a synergistic Ca2+ response in human neutrophils. J Biol Chem. (1995) 270:10671–9. doi: 10.1074/jbc.270.18.10671
174. Huizinga TW, Dolman KM, van der Linden NJ, Kleijer M, Nuijens JH, von dem Borne AE, et al. Phosphatidylinositol-linked FcRIII mediates exocytosis of neutrophil granule proteins, but does not mediate initiation of the respiratory burst. J Immunol. (1990) 144:1432–7.
175. Shibata-Koyama M, Iida S, Misaka H, Mori K, Yano K, Shitara K, et al. Nonfucosylated rituximab potentiates human neutrophil phagocytosis through its high binding for FcγRIIIb and MHC class II expression on the phagocytotic neutrophils. Exp Hematol. (2009) 37:309–21. doi: 10.1016/j.exphem.2008.11.006
176. Golay J, Da Roit F, Bologna L, Ferrara C, Leusen JH, Rambaldi A, et al. Glycoengineered CD20 antibody obinutuzumab activates neutrophils and mediates phagocytosis through CD16B more efficiently than rituximab. Blood. (2013) 122:3482–91. doi: 10.1182/blood-2013-05-504043
177. Derer S, Glorius P, Schlaeth M, Lohse S, Klausz K, Muchhal U, et al. Increasing FcaγRIIa affinity of an FcaγRIII-optimized anti-EGFR antibody restores neutrophil-mediated cytotoxicity. MAbs. (2014) 6:409–21. doi: 10.4161/mabs.27457
178. Mousavi SA, Sporstøl M, Fladeby C, Kjeken R, Barois N, Berg T. Receptor-mediated endocytosis of immune complexes in rat liver sinusoidal endothelial cells is mediated by FcγRIIb2. Hepatology. (2007) 46:871–84. doi: 10.1002/hep.21748
179. Ganesan LP, Kim J, Wu Y, Mohanty S, Phillips GS, Birmingham DJ, et al. Fc RIIb on liver sinusoidal endothelium clears small immune complexes. J Immunol. (2012) 189:4981–8. doi: 10.4049/jimmunol.1202017
180. Floto RA, Clatworthy MR, Heilbronn KR, Rosner DR, MacAry PA, Rankin A, et al. Loss of function of a lupus-associated FcgammaRIIb polymorphism through exclusion from lipid rafts. Nat Med. (2005) 11:1056–8. doi: 10.1038/nm1288
181. Kono H, Kyogoku C, Suzuki T, Tsuchiya N, Honda H, Yamamoto K, et al. FcyRIIB Ile232Thr transmembrane polymorphism associated with human systemic lupus erythematosus decreases affinity to lipid rafts and attenuates inhibitory effects on B cell receptor signaling. Hum Mol Genet. (2005) 14:2881–92. doi: 10.1093/hmg/ddi320
182. Ernst LK, Metes D, Herberman RB, Morel PA. Allelic polymorphisms in the FcγRIIC gene can influence its function on normal human natural killer cells. J Mol Med. (2002) 80:248–57. doi: 10.1007/s00109-001-0294-2
183. Breunis WB, van Mirre E, Bruin M, Geissler J, de Boer M, Peters M, et al. Copy number variation of the activating FCGR2C gene predisposes to idiopathic thrombocytopenic purpura. Blood. (2007) 111:1029–38. doi: 10.1182/blood-2007-03-079913
184. Warmerdam PAM, Nabben NMJM, Van de Graaf SAR, Van de Winkel JGJ, Capel PJA. The human low affinity immunoglobulin G Fc receptor IIC gene is a result of an unequal crossover event. J Biol Chem. (1993) 268:7346–9.
185. Metes D, Galatiuc C, Moldovan I, Morel PA, Chambers WH, DeLeo AB, et al. Expression and function of Fc gamma RII on human natural killer cells. Nat Immun. (1994) 13:289–300.
186. Li SS, Gilbert PB, Tomaras GD, Kijak G, Ferrari G, Thomas R, et al. FCGR2C polymorphisms associate with HIV-1 vaccine protection in RV144 trial. J Clin Invest. (2014) 124:3879–90. doi: 10.1172/JCI75539
187. Liu L. Pharmacokinetics of monoclonal antibodies and Fc-fusion proteins. Protein Cell. (2017) 9:15–32. doi: 10.1007/s13238-017-0408-4.
188. Spiegelberg HL, Fishkin BG. The catabolism of human G immunoglobulins of different heavy chain subclasses. 3. The catabolism of heavy chain disease proteins and of Fc fragments of myeloma proteins. Clin Exp Immunol. (1972) 10:599–607.
189. Ghetie V, Hubbard JG, Kim JK, Tsen MF, Lee Y, Ward ES. Abnormally short serum half - lives of IgG in beta 2 - microglobulin - deficient mice. Eur J Immunol. (1996) 26:690–6.
190. Roopenian DC, Christianson GJ, Sproule TJ, Brown AC, Akilesh S, Jung N, et al. The MHC class I-like IgG receptor controls perinatal IgG transport, IgG homeostasis, and fate of IgG-Fc-coupled drugs. J Immunol. (2003) 170:3528–33. doi: 10.4049/jimmunol.170.7.3528
191. Ward ES. Evidence to support the cellular mechanism involved in serum IgG homeostasis in humans. Int Immunol. (2003) 15:187–95. doi: 10.1093/intimm/dxg018
192. Simister NE, Mostov KE. An Fc receptor structurally related to MHC class I antigens. Nature. (1989) 337:184–7. doi: 10.1038/337184a0
193. Burmeister WP, Huber AH, Bjorkman PJ. Crystal structure of the complex of rat neonatal Fc receptor with Fc. Nature. (1994) 372:379–83. doi: 10.1038/372379a0
194. Burmeister WP, Gastinel LN, Simister NE, Blum ML, Bjorkman PJ. Crystal structure at 2.2 Å resolution of the MHC-related neonatal Fc receptor. Nature. (1994) 372:336–43. doi: 10.1038/372336a0
195. Haymann JP, Levraud JP, Bouet S, Kappes V, Hagège J, Nguyen G, et al. Characterization and localization of the neonatal Fc receptor in adult human kidney. J Am Soc Nephrol. (2000) 11:632–9.
196. Zhu X, Meng G, Dickinson BL, Li X, Mizoguchi E, Miao L, et al. MHC class I-related neonatal Fc receptor for IgG is functionally expressed in monocytes, intestinal macrophages, and dendritic cells. J Immunol. (2001) 166:3266–76. doi: 10.4049/jimmunol.166.5.3266
197. Schlachetzki F, Zhu C, Pardridge WM. Expression of the neonatal Fc receptor (FcRn) at the blood-brain barrier. J Neurochem. (2002) 81:203–6. doi: 10.1046/j.1471-4159.2002.00840.x
198. Spiekermann GM, Finn PW, Ward ES, Dumont J, Dickinson BL, et al. Receptor-mediated immunoglobulin G transport across mucosal barriers in adult life: functional expression of FcRn in the mammalian lung. J Exp Med. (2002) 196:303–10. doi: 10.1084/jem.20020400
199. Leach JL, Sedmak DD, Osborne JM, Rahill B, Lairmore MD, Anderson CL. Isolation from human placenta of the IgG transporter, FcRn, and localization to the syncytiotrophoblast: implications for maternal-fetal antibody transport. J Immunol. (1996) 157:3317–22.
200. Simister NE, Story CM, Chen HL, Hunt JS. An IgG - transporting Fc receptor expressed in the syncytiotrophoblast of human placenta. Eur J Immunol. (1996) 26:1527–31.
201. Israel EJ, Taylor S, Wu Z, Mizoguchi E, Blumberg RS, Bhan A, Simister NE. Expression of the neonatal Fc receptor, FcRn, on human intestinal epithelial cells. Immunology. (1997) 92:69–74. doi: 10.1046/j.1365-2567.1997.00326.x
202. Borvak J, Richardson J, Medesan C, Antohe F, Radu C, Simionescu M, et al. Functional expression of the MHC class I-related receptor, FcRn, in endothelial cells of mice. IntImmunol. (1998) 10:1289–98.
203. Shah U, Dickinson BL, Blumberg RS, Simister NE, Lencer WI, Walker AW. Distribution of the IgG Fc Receptor, FcRn, in the Human Fetal Intestine. Pediatr Res. (2003) 53:295–301. doi: 10.1203/01.PDR.0000047663.81816.E3
204. Jones EA, Waldmann TA. The mechanism of intestinal uptake and transcellular transport of IgG in the neonatal rat. J Clin Invest. (1972) 51:2916–27. doi: 10.1172/JCI107116
205. Rodewald R. pH-dependent binding of immunoglobulins to intestinal cells of the neonatal rat. J Cell Biol. (1976) 71:666–9. doi: 10.1083/jcb.71.2.666
206. Simister NE, Rees AR. Isolation and characterization of an Fc receptor from neonatal rat intestine. Eur J Immunol. (1985) 15:733–8.
207. Firan M, Bawdon R, Radu C, Ober RJ, Eaken D, Antohe F, et al. The MHC class I-related receptor, FcRn, plays an essential role in the maternofetal transfer of gamma-globulin in humans. Int Immunol. (2001) 13:993–1002. doi: 10.1093/intimm/13.8.993
208. Israel EJ, Patel VK, Taylor SF, Marshak-Rothstein A, Simister NE. Requirement for a beta 2-microglobulin-associated Fc receptor for acquisition of maternal IgG by fetal and neonatal mice. J Immunol. (1995) 154:6246–51.
209. Martin WL, West AP, Gan L, Bjorkman PJ. Crystal structure at 2.8 A of an FcRn/heterodimeric Fc complex: mechanism of pH-dependent binding. Mol Cell. (2001) 7:867–77.
210. Uno Y, Utoh M, Iwasaki K. Polymorphisms of neonatal Fc receptor in cynomolgus and rhesus macaques. Drug Metab Pharmacokinet. (2014) 29:427–30. doi: 10.2133/dmpk.DMPK-14-NT-033
211. Shubin Z, Tagaya Y, Poonia B. Functional polymorphisms in rhesus macaque FCGRT and β2-m. Immunogenetics. (2018) 70:179–83. doi: 10.1007/s00251-017-1022-6
212. Vaughn DE, Milburn CM, Penny DM, Martin WL, Johnson JL, Bjorkman PJ. Identification of critical IgG binding epitopes on the neonatal Fc receptor. J Mol Biol. (1997) 274:597–607. doi: 10.1006/jmbi.1997.1388
213. Su K, Li X, Edberg JC, Wu J, Ferguson P, Kimberly RP. A promoter haplotype of the immunoreceptor tyrosine-based inhibitory motif-bearing Fc RIIb alters receptor expression and associates with autoimmunity. II. differential binding of GATA4 and Yin-Yang1 transcription factors and correlated receptor expression. J Immunol. (2004) 172:7192–9. doi: 10.4049/jimmunol.172.11.7192
214. Willcocks LC, Lyons PA, Clatworthy MR, Robinson JI, Yang W, Newland SA, et al. Copy number of FCGR3B, which is associated with systemic lupus erythematosus, correlates with protein expression and immune complex uptake. J Exp Med. (2008) 205:1573–82. doi: 10.1084/jem.20072413
215. Breunis WB, Van Mirre E, Geissler J, Laddach N, Wolbink G, Van Schoot E, et al. Copy number variation at the FCGR locus includes FCGR3A, FCGR2C and FCGR3B but not FCGR2A and FCGR2B. Hum Mutat. (2009) 30:640–50. doi: 10.1002/humu.20997
216. Van Der Heijden J, Geissler J, Van Mirre E, Van Deuren M, Van Der Meer JWM, Salama A, et al. A novel splice variant of FcγRIIa: A risk factor for anaphylaxis in patients with hypogammaglobulinemia. J Allergy Clin Immunol. (2013) 131:1408–16.e5. doi: 10.1016/j.jaci.2013.02.009
217. van der Heijden J, Nagelkerke S, Zhao X, Geissler J, Rispens T, van den Berg TK, et al. Haplotypes of Fc RIIa and Fc RIIIb polymorphic variants influence IgG-mediated responses in neutrophils. J Immunol. (2014) 192:2715–21. doi: 10.4049/jimmunol.1203570
Keywords: non-human primate, rhesus, cynomolgus, HIV, SIV, IgG, Fcγ receptor, neonatal Fc receptor
Citation: Crowley AR and Ackerman ME (2019) Mind the Gap: How Interspecies Variability in IgG and Its Receptors May Complicate Comparisons of Human and Non-human Primate Effector Function. Front. Immunol. 10:697. doi: 10.3389/fimmu.2019.00697
Received: 01 November 2018; Accepted: 13 March 2019;
Published: 08 April 2019.
Edited by:
Gabriella Scarlatti, San Raffaele Hospital (IRCCS), ItalyReviewed by:
Davide Corti, Vir Biotechnology, SwitzerlandAmy W. Chung, The University of Melbourne, Australia
Copyright © 2019 Crowley and Ackerman. This is an open-access article distributed under the terms of the Creative Commons Attribution License (CC BY). The use, distribution or reproduction in other forums is permitted, provided the original author(s) and the copyright owner(s) are credited and that the original publication in this journal is cited, in accordance with accepted academic practice. No use, distribution or reproduction is permitted which does not comply with these terms.
*Correspondence: Margaret E. Ackerman, bWFyZ2FyZXQuZS5hY2tlcm1hbkBkYXJ0bW91dGguZWR1