- Vaccine and Infectious Disease Division, Fred Hutchinson Cancer Research Center, Seattle, WA, United States
Antibodies against foreign antigens are a critical component of the overall immune response and can facilitate pathogen clearance during a primary infection and also protect against subsequent infections. Dysregulation of the antibody response can lead to an autoimmune disease, malignancy, or enhanced infection. Since the experimental delineation of a distinct B cell lineage in 1965, various methods have been developed to understand antigen-specific B cell responses in the context of autoimmune diseases, primary immunodeficiencies, infection, and vaccination. In this review, we summarize the established techniques and discuss new and emerging technologies for probing the B cell response in vitro and in vivo by taking advantage of the specificity of B cell receptor (BCR)-associated and secreted antibodies. These include ELISPOT, flow cytometry, mass cytometry, and fluorescence microscopy to identify and/or isolate primary antigen-specific B cells. We also present our approach to identify rare antigen-specific B cells using magnetic enrichment followed by flow cytometry. Once these cells are isolated, in vitro proliferation assays and adoptive transfer experiments in mice can be used to further characterize antigen-specific B cell activation, function, and fate. Transgenic mouse models of B cells targeting model antigens and of B cell signaling have also significantly advanced our understanding of antigen-specific B cell responses in vivo.
Introduction
In his Nobel lecture in 1908, Paul Ehrlich likened the antibody-antigen interaction to a lock and key. He reasoned that antitoxins (antibodies) contained in a solution in the serum of immunized animals must be identical to a cellular receptor “for a really well-made key will not open different locks at the same time” (1). It took almost five decades before immunofluorescence microscopy was used to confirm the cellular origin of antibodies (2). Major strides in the B cell and antibody field followed in the 1970s with the development of hybridoma technology to produce monoclonal antibodies and the discovery that somatic rearrangement during B cell differentiation was responsible for antibody diversification (3, 4). The subsequent explosion of available monoclonal antibodies led to revolutionary diagnostic, therapeutic, and research reagents to distinguish different types of immune cells (5). Together, these discoveries have allowed us to probe humoral immunity at the level of the antigen-specific B cell.
Why Study B Cell Responses? Opportunities for Applying Techniques to Study Antigen-Specific B Cells
Methods to probe the antigen-specific B cell response have advanced our understanding of how to harness the remarkable breadth of the B cell repertoire and the exquisite specificity of the individual B cell in developing (1) vaccine candidates that elicit protective antibodies; (2) antibodies that prevent disease when given prophylactically; and (3) antibodies that can be given as therapy after the onset of disease. Many of the vaccines currently available were originally developed empirically either by inactivating, attenuating, or administering a subunit of the pathogen. However, vaccine development against pathogens that are traditionally difficult to vaccinate against may rely on a deeper investigation of the B cell response to the antigens exposed on the surface of these pathogens.
For HIV-1, the discovery of broadly neutralizing antibodies (bnAbs) that protect against infection across diverse viral isolates has intensified efforts to understand the developmental pathway of the rare B cells that produce these antibodies (6–9). Insights into the ontogeny of these rare B cells could allow the design of a step-wise vaccine regimen that stimulates the germ-line precursor to expand and mature to produce circulating bnAbs which could protect against HIV acquisition (10, 11). For RSV, stabilized versions of the fusion (F) protein in the pre-fusion conformation have led to insights in the B cell's response to infection and has generated potentially safer and more efficacious vaccine candidates (12, 13). Influenza also performs fusion through the stem region of the hemagglutinin protein, and the identification of B cells that target this relatively conserved site has spurred research on the development of a universal influenza vaccine (14–16). Like RSV, HIV, and influenza, the fusion proteins of EBV and CMV exist in a pre-fusion conformation, and stabilization in their pre-fusion states could greatly accelerate vaccine development against these pathogens (17–19). Rare memory B cells producing antibodies specific for the EBV fusion machinery have been isolated; these can neutralize both B cell and epithelial cell infection (20). A new paradigm in malaria vaccine development is also emerging with the discovery of IgM+ and IgD+ memory B cells targeting the Merozoite Surface Protein 1, that rapidly respond to malaria re-infection (21). Further, highly potent neutralizing antibodies targeting a novel and conserved site on the Circumsporozoite Protein have been isolated from B cells (22). Together, these examples demonstrate the importance of studying antigen-specific humoral responses to infectious diseases. The solutions to the crystal structures of surface proteins for a variety of pathogens, the conformational stabilization of these antigens, and the application of the methods summarized in this review, to probe antigen-specific B cell responses, have created new opportunities for systematic and rational vaccine design for HIV, RSV, EBV, malaria, and many other pathogens.
The study of B cell responses has not only informed vaccine design but has also advanced our understanding of antibody-mediated autoimmune diseases, such as rheumatoid arthritis and systemic lupus erythematosus (23, 24). Up to 20% of mature, naïve B cells have receptors with the capacity to bind self-antigens (25). Although these cells are potentially pathogenic, the deletion of B cells with high affinity to self-antigen through apoptosis, anergy of B cells with low affinity to self-antigen, and the absence of T cell help combine together to protect against autoimmune disease in mice (26). The study of autoantigen-specific B cells and a detailed analysis of B cell subsets with pathogenic potential in humans could lead to a better understanding of how to prevent and treat autoimmune diseases.
Although the term antigen-specific B cell is used throughout this mini-review to denote the analysis of B cells based on binding between the B cell receptor (BCR) and a specific antigen used as bait, it is important to keep in mind that BCRs within the polyclonal B cell repertoire exhibit a spectrum of polyreactivity. On one end of the spectrum, a highly polyreactive BCR is able to bind multiple structurally unrelated antigens with physiologically relevant affinities. The frequency of polyreactivity in the normal adult human B cell repertoire has been estimated to be 4% of naïve B cells, 23% of IgG+ memory B cells, and 26% of intestinal IgA+ and IgG+ plasmablasts (27–29). On the other end of the spectrum, a mono reactive BCR is activated only when it encounters a single cognate antigen. Although there are exceptions, the accumulation of somatic hypermutations within the variable regions of the BCR during the process of affinity maturation is generally thought to lead to increased affinity and specificity for the cognate antigen (30, 31).
Ex vivo Methods to Identify Antigen-Specific Primary B Cells
Several general techniques are commonly used to identify antigen-specific B cells (Table 1). The B cell enzyme linked immunospot (ELISPOT) technique relies on the principle of capturing the secreted antibody in the vicinity of each cell. In the B cell ELISPOT, antibody secreting B cells (ASCs) present in a sample or differentiated in vitro are added to plates coated with the antigen of interest. Antigen-specific antibodies will bind in close proximity to the location of the individual B cells producing those antibodies. Enzyme or fluorescent labeled secondary antibodies are then used to visualize spots of antibody secretion and binding to plate-bound antigen at the location of the ASCs. Each spot corresponds to antibody produced from a single antigen-specific B cell and therefore the technique is extremely sensitive. Secondary antibodies conjugated to combinatorial colored beads can also be used to detect the antibodies secreted from individual B cells with the advantage of multiplexing the assay (32). One limitation of the assay is its requirement for antibody secretion by B cells thereby limiting the assay to only a subset of B cells in the repertoire, namely ASCs (33). Memory B cells can be stimulated in vitro to differentiate into ASCs prior to addition to the antigen-coated plate (34). Further, the antigen-specific B cells identified by ELISPOT are generally not available for downstream analysis.
Limiting dilution is another technique that has been used to isolate antigen-specific B cells. In this approach, primary cells can be diluted serially until individual B cells are separated in microwell plates (36). The B cells can then be cultured and expanded ex vivo and/or immortalized using EBV such that each well contains a monoclonal antibody (3, 37, 38). Antigen-specific B cells can be selected by screening the culture supernatants for monoclonal antibodies that bind an antigen of interest. Although antibodies can be sequenced and cloned, the requirement for an ex vivo culture prior to selection precludes determination of the transcriptional profile of the original B cell in this approach. This technique can potentially be time-consuming and laborious, but the use of microfluidics and robotics has greatly improved the throughput for selecting antigen-specific B cells (39). Advances in single cell next generation sequencing technology have allowed high throughput transcriptional profiling and sequencing of paired immunoglobulin heavy and light chains (40). In this approach, antigen specificity can be tested after monoclonal antibodies are cloned and produced using the sequencing data. This method can be useful in identifying antigen-specific B cells that have undergone clonal expansion after vaccination or acute infection (41).
Flow cytometry is the most common method used for single cell analysis and isolation (39). Flow cytometry-based analysis of antigen-specific B cells is dependent on labeling antigen with a fluorescent tag to allow detection. Fluorochromes can either be attached covalently via chemical conjugation to the antigen, expressed as a recombinant fusion protein, or attached non-covalently by biotinylating the antigen. After biotinylation, fluorochrome-conjugated streptavidin is added to generate a labeled tetramer of the antigen. Biotinylation of the antigen at a ratio ≤1 biotin to 1 antigen is important, since each streptavidin has the potential to bind four biotins. If the ratio of biotin to antigen is >1:1, then clumping and precipitation of the antigen out of solution can occur as soon as streptavidin is added. Alternatively, site directed biotinylation can be accomplished by adding either an AviTag or BioEase tag to the recombinant antigen prior to expression (77, 78). When site-specific biotinylation is utilized, researchers must keep in mind that the tag may occlude an epitope from recognition by B cells which can be problematic for vaccine antigens. Further, for proteins that oligomerize, multiple tags may be incorporated, possibly resulting in aggregation.
Another important consideration is the potential for confounding by B cells in the repertoire that bind to the fluorochrome, streptavidin, or any linkers rather than to the antigen of interest. Binding between fluorochromes, linkers, or streptavidin and BCRs from humans and mice never exposed to these antigens are generally of low affinity, and these BCRs are generally expressed by naïve and potentially polyreactive B cells (62, 79, 80). Dual labeling, in which the same antigen is separately labeled with two different fluorochromes, can be used to identify double positive B cells and remove confounding by B cells that bind the fluorochrome (12, 42). However, even when tetramers are utilized for dual labeling, streptavidin-specific B cells will contaminate the double positive population. To fully remove confounding from the fluorochrome, streptavidin, and linkers, a “decoy” tetramer can be used to identify these contaminating B cells (21, 26). In this approach, the same fluorochrome used to identify antigen-specific B cells is conjugated to a different fluorochrome such that the emission spectrum is altered by fluorescence resonance energy transfer (FRET) (26). Decoy-binding B cells can therefore be excluded from the true antigen-specific B cells. Notably, it is critical to use the same source of fluorochrome conjugated streptavidin in the tetramer and decoy reagent, because conjugation methods, recombinant streptavidin, and protein fluorochromes like R-phycoerythrin vary enough from company to company to alter some of the epitopes available for B cells to bind.
One weakness of the flow cytometric approach is the reliance on antigens that can be readily conjugated to a fluorochrome or biotinylated. In addition to recombinant proteins and synthesized peptides, labeled polysaccharides, lipids, haptens, virus-like particles, and pseudo viruses have also been used to identify antigen-specific cells by flow cytometry (33, 43–59). Further, epitope-specific B cells have been identified by screening bacteriophage-displays or microarray peptide libraries with polyclonal antibodies targeting the native antigen to select conformational epitopes that can be fused to fluorescent proteins for use in flow cytometry (47, 60). With technologic advancements increasing the number of simultaneously measurable parameters, antigen-specific B cells can be further characterized by cell surface markers and intracellular staining. Additionally, the immunoglobulin capture assay is a flow cytometry-based adaptation of the ELISPOT assay in which a streptavidin-conjugated anti-CD45 antibody carrying four biotinylated anti-IgG antibodies is used to simultaneously bind plasmablasts and capture secreted antibody followed by fluorescent-labeled antigen to detect antigen-specific plasmablasts (61). The mean fluorescence intensity measured by flow cytometry and normalized to the level of BCR expression also provides a measure of the relative amount of antigen binding to a B cell and can be used as a rough surrogate for binding affinity (79, 81, 82). Pre-incubation of B cells with increasing concentrations of a monomeric antigen prior to labeling with tetrameric antigen can also be used to further quantify binding affinity. Cells expressing high affinity BCRs will bind monomeric antigen at low concentrations, whereas low affinity BCRs will require higher concentrations of monomeric antigen to compete with and inhibit tetramer binding (26). Individual cells can also be isolated by fluorescence activated cell sorting (FACS) for downstream analysis, including BCR sequencing and cloning, BCR affinity measurement, in vitro proliferation, and transcriptional profiling.
Methods for Rare Antigen-Specific B Cells
Methods have recently been developed to further improve the sensitivity for detecting rare antigen-specific B cells. Magnetic nanoparticles conjugated to antibodies targeting the fluorochrome on the antigen of interest, allow for the enrichment of antigen-specific B cells prior to flow cytometry (20, 26, 80, 83). This approach is particularly useful for detecting rare antigen-specific naïve B cells, autoreactive B cells, memory B cells, and plasmablasts (21, 26, 47, 50). The magnetic enrichment strategy allows for the analysis of significantly more cells in a shorter period of time by concentrating the cells of interest prior to flow cytometry (Figure 1). Notably, as with any method that seeks to identify a population of cells at a very low frequency, the background and noise inherent in the detection system is magnified with respect to the signal of interest, especially when that signal is weak. Therefore, to detect the antigen-specific population of interest, the following considerations are critical: (1) Using decoys to exclude B cells of unwanted specificities; (2) careful design of flow cytometry panels to avoid emission spillover into the channel for the antigen of interest; and (3) choosing the brightest fluorochromes, like R-phycoerythrin or allophycocyanin.
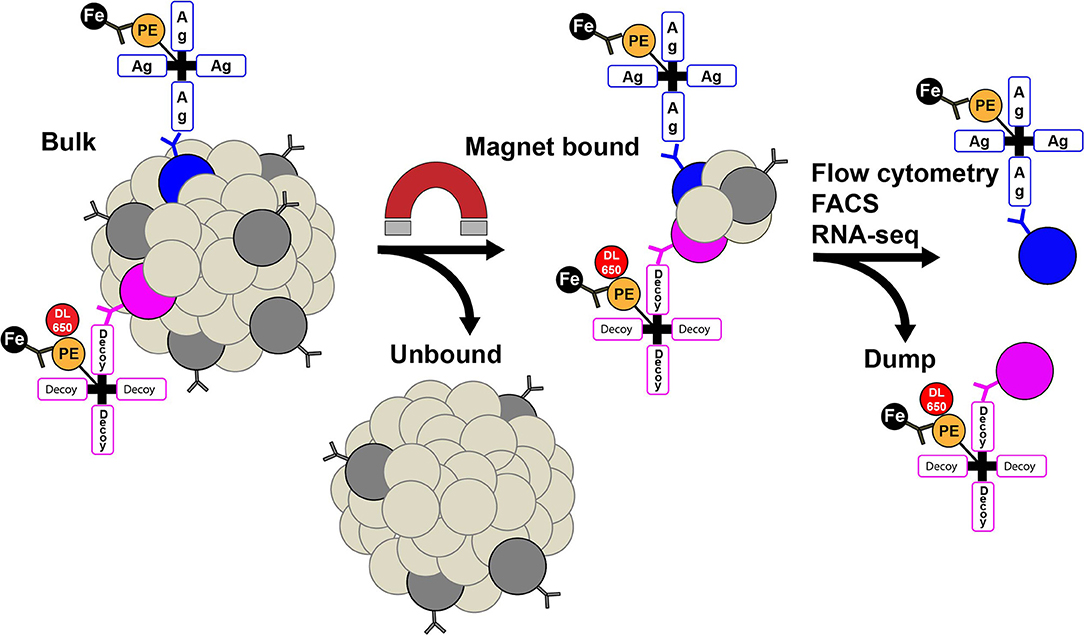
Figure 1. Rare antigen (Ag)-specific B cells can be identified using tetramers conjugated to a fluorochrome, followed by magnetic (Fe) nanoparticles that bind the fluorochrome, magnetic enrichment, and flow cytometry. B cells are shown in dark gray with a B cell receptor on the surface. PE, phycoerythrin; DL650, DyLight 650; FACS, fluorescent activated cell sorting.
In vivo Assays for Studying Antigen-Specific Primary B Cell Responses
In vivo methods to probe antigen-specific B cell responses in the presence of other antigen-presenting cells and T cell helpers, have increased our mechanistic understanding of the humoral immune response during vaccination, infection, and autoimmunity. Adoptively transferred B cells can be distinguished from recipient lymphocytes by taking advantage of mouse strains with allelic variations in CD45 or mice devoid of B cells. The adoptively transferred B cells can come from wild-type mice or from mice expressing transgenic BCRs (Table 2), and antigen-specific B cells can be analyzed using the techniques described above.
Microscopy is another general technique that has been used to identify antigen-specific cells in vivo and offers the advantage of direct visualization. In the first reported application of this technique to demonstrate the cellular origin of antibodies in 1955, fluorescein-conjugated antibodies against ovalbumin and human immunoglobulin were used to stain tissue sections of the spleen from hyperimmune rabbits (2). Since then, other groups have fluorescently labeled antigens to localize antigen-specific B cells by microscopy (62, 65). Advances in laser capture dissection microscopy, already used in the T cell field, also provide an opportunity for isolating individual antigen-specific B cells for downstream analysis, including sequencing and cloning of the BCR or transcriptional profiling (66). However, antigen staining of BCRs in situ can be challenging depending on the binding of antigens from pathogens to other cellular receptors or an alteration of BCR specificity during tissue fixation or processing. Two-photon or multiphoton microscopy has the ability to resolve images at greater depths and with less photobleaching than confocal microscopy (67, 68). As a result, this technology has allowed real-time imaging in living, intact lymphoid tissues of mice, permitting the direct in vivo observation of immune cell interactions. The dynamic movements and interactions of antigen-specific B cells can be studied in vivo by combining an adoptive transfer of individual B cells (isolated by limiting dilution or FACS) with two-photon microscopy (63, 69, 70).
Emerging Techniques for Studying Antigen-Specific Primary B Cell Responses
Humanized mouse models are powerful tools for translating experiments in mice to applications in humans. Transgenic mice that produce humanized cytokines by knock-in replacement can be used to support human hematopoietic stem cells (104). Transgenic mice with complete humanization of the mouse immunoglobulin loci provide an opportunity for recapitulating the breadth of the human B cell repertoire and serve as a valuable tool for therapeutic antibody discovery (71). However, one caveat is that the allele frequencies found in the B cell repertoires of these mouse models may not necessarily recapitulate those found in humans (72).
Mass cytometry has the potential to provide further high-dimensional analysis of antigen-specific B cells. In this method, heavy metal ion tags rather than fluorochromes are used to label cells. Since data is collected as time-of-flight mass spectrometry, up to 42 unique parameters can be simultaneously measured from a single sample without significant spillover between channels or the need for compensation. Mass cytometry with heavy metal-labeled tetramers can be constructed using streptavidin (73). Mass cytometry with metal-labeled peptide-MHC tetramers has been used successfully to identify and characterize antigen-specific T cells, but to our knowledge has not yet been applied to antigen-specific B cells (73, 74). One limitation of this approach is that cells are unavailable for downstream analysis since they are vaporized by a plasma torch to atomize the ion tags. However, by simultaneously detecting many more surface markers and intracellular cytokines, transcription factors, and detecting more signaling molecules from individual cells than previously possible with traditional fluorescent labels, the application of mass cytometry with dimensionality reduction algorithms could help dissect the complexity of the B cell compartment, provide a higher resolution view of B cell development, and reveal novel subsets of antigen-specific B cells involved in mediating autoimmune diseases or protection against infection.
On the horizon, single cell RNA-sequencing (RNA-seq) technologies have the potential to revolutionize the study of antigen-specific immune cells (75, 76). The ability to generate a library of tetramers with unique barcodes could allow the simultaneous examination of gene expression profiles from a large number of cells with different antigen specificities in a single experiment. Combining barcoded tetramers with oligonucleotide-conjugated antibodies and RNA-seq to simultaneously measure the protein and gene expression of antigen-specific cells could further increase the amount of unbiased multi-omic information about individual antigen-specific cells in normal and disease states and aid the rational design of vaccines and therapeutics (105–107).
Conclusions and Horizons
The ongoing analysis of antigen-specific B cell responses has led to the development of new diagnostic, therapeutic, and research reagents. Methods for studying antigen-specific B cell responses are being increasingly applied to tackle diseases like HIV, RSV, and autoimmune diseases, in which the immune response either fails to protect or clear disease, or where it enhances disease or is responsible for the disease itself. Considerable opportunities exist on the horizon for applying these methods to a myriad of diseases in which B cells play an active role.
Author Contributions
JB and JT reviewed the literature, generated figures and tables, and wrote the manuscript.
Funding
JB was supported by T32-AI118690 and JT was supported by R01-AI122912 from the National Institute of Allergy and Infectious Diseases, National Institutes of Health.
Conflict of Interest Statement
The authors declare that the research was conducted in the absence of any commercial or financial relationships that could be construed as a potential conflict of interest.
Acknowledgments
We would like to thank Andrew McGuire, Anton Sholukh, Blair DeBuysscher, Ashley Sherrid, Paula Culver, and Kristin Fitzpatrick for critical reading of the manuscript.
References
1. Ehrlich P. Nobel lecture on partial functions of the cell. In: Himmileweit F, editor. The Collected Papers of Paul Ehrlich. London: Elsevier (1908). p. 1–624.
2. Coons AH, Leduc EH, Connolly JM. Studies on antibody production. I. A method for the histochemical demonstration of specific antibody and its application to a study of the hyperimmune rabbit. J Exp Med. (1955) 102:49–60. doi: 10.1084/jem.102.1.49
3. Kohler G, Milstein C. Continuous cultures of fused cells secreting antibody of predefined specificity. Nature. (1975) 256:495–7.
4. Hozumi N, Tonegawa S. Evidence for somatic rearrangement of immunoglobulin genes coding for variable and constant regions. Proc Natl Acad Sci USA. (1976) 73:3628–32.
5. Bernard A, Boumsell L. The clusters of differentiation (CD) defined by the First International Workshop on human leucocyte differentiation antigens. Hum Immunol. (1984) 11:1–10.
6. Saunders KO, Wang L, Joyce MG, Yang ZY, Balazs AB, Cheng C, et al. Broadly neutralizing human immunodeficiency virus type 1 antibody gene transfer protects nonhuman primates from mucosal simian-human immunodeficiency virus infection. J Virol. (2015) 89:8334–45. doi: 10.1128/JVI.00908-15
7. Gautam R, Nishimura Y, Gaughan N, Gazumyan A, Schoofs T, Buckler-White A, et al. A single injection of crystallizable fragment domain-modified antibodies elicits durable protection from SHIV infection. Nat Med. (2018) 24:610–6. doi: 10.1038/s41591-018-0001-2
8. Mascola JR, Haynes BF. HIV-1 neutralizing antibodies: understanding nature's pathways. Immunol Rev. (2013) 254:225–44. doi: 10.1111/imr.12075
9. Fera D, Schmidt AG, Haynes BF, Gao F, Liao HX, Kepler TB, et al. Affinity maturation in an HIV broadly neutralizing B-cell lineage through reorientation of variable domains. Proc Natl Acad Sci USA. (2014) 111:10275–80. doi: 10.1073/pnas.1409954111
10. Bonsignori M, Kreider EF, Fera D, Meyerhoff RR, Bradley T, Wiehe K, et al. Staged induction of HIV-1 glycan-dependent broadly neutralizing antibodies. Sci Transl Med. (2017) 9:eaai7514. doi: 10.1126/scitranslmed.aai7514
11. Kwong PD, Mascola JR. HIV-1 Vaccines based on antibody identification, B cell ontogeny, and epitope structure. Immunity. (2018) 48:855–71. doi: 10.1016/j.immuni.2018.04.029
12. Gilman MS, Castellanos CA, Chen M, Ngwuta JO, Goodwin E, Moin SM, et al. Rapid profiling of RSV antibody repertoires from the memory B cells of naturally infected adult donors. Sci Immunol. (2016) 1:eaaj1879. doi: 10.1126/sciimmunol.aaj1879
13. McLellan JS, Chen M, Leung S, Graepel KW, Du X, Yang Y, et al. Structure of RSV fusion glycoprotein trimer bound to a prefusion-specific neutralizing antibody. Science. (2013) 340:1113–7. doi: 10.1126/science.1234914
14. Yassine HM, Boyington JC, McTamney PM, Wei CJ, Kanekiyo M, Kong WP, et al. Hemagglutinin-stem nanoparticles generate heterosubtypic influenza protection. Nat Med. (2015) 21:1065–70. doi: 10.1038/nm.3927
15. McCarthy KR, Watanabe A, Kuraoka M, Do KT, McGee CE, Sempowski GD, et al. Memory B cells that cross-react with group 1 and group 2 influenza A viruses are abundant in adult human repertoires. Immunity. (2018) 48:174–184.e9. doi: 10.1016/j.immuni.2017.12.009
16. Joyce MG, Wheatley AK, Thomas PV, Chuang GY, Soto C, Bailer RT, et al. Vaccine-induced antibodies that neutralize group 1 and group 2 influenza A viruses. Cell. (2016) 166:609–23. doi: 10.1016/j.cell.2016.06.043
17. Kirchmeier M, Fluckiger AC, Soare C, Bozic J, Ontsouka B, Ahmed T, et al. Enveloped virus-like particle expression of human cytomegalovirus glycoprotein B antigen induces antibodies with potent and broad neutralizing activity. Clin Vaccine Immunol. (2014) 21:174–80. doi: 10.1128/CVI.00662-13
18. Schleiss MR, Permar SR, Plotkin SA. Progress toward development of a vaccine against congenital cytomegalovirus infection. Clin Vaccine Immunol. (2017) 24:e00268-17. doi: 10.1128/CVI.00268-17
19. Si Z, Zhang J, Shivakoti S, Atanasov I, Tao CL, Hui WH, et al. Different functional states of fusion protein gB revealed on human cytomegalovirus by cryo electron tomography with Volta phase plate. PLoS Pathog. (2018) 14:e1007452. doi: 10.1371/journal.ppat.1007452
20. Snijder J, Ortego MS, Weidle C, Stuart AB, Gray MD, McElrath MJ, et al. An antibody targeting the fusion machinery neutralizes dual-tropic infection and defines a site of vulnerability on Epstein-Barr virus. Immunity. (2018) 48:799–811.e9. doi: 10.1016/j.immuni.2018.03.026
21. Krishnamurty AT, Thouvenel CD, Portugal S, Keitany GJ, Kim KS, Holder A, et al. Somatically hypermutated plasmodium-specific IgM(+) memory B cells are rapid, plastic, early responders upon malaria rechallenge. Immunity. (2016) 45:402–14. doi: 10.1016/j.immuni.2016.06.014
22. Kisalu NK, Idris AH, Weidle C, Flores-Garcia Y, Flynn BJ, Sack BK, et al. A human monoclonal antibody prevents malaria infection by targeting a new site of vulnerability on the parasite. Nat Med. (2018) 24:408–16. doi: 10.1038/nm.4512
23. Mandik-Nayak L, Ridge N, Fields M, Park AY, Erikson J. Role of B cells in systemic lupus erythematosus and rheumatoid arthritis. Curr Opin Immunol. (2008) 20:639–45. doi: 10.1016/j.coi.2008.08.003
24. Shlomchik MJ. Sites and stages of autoreactive B cell activation and regulation. Immunity. (2008) 28:18–28. doi: 10.1016/j.immuni.2007.12.004
25. Meffre E, Wardemann H. B-cell tolerance checkpoints in health and autoimmunity. Curr Opin Immunol. (2008) 20:632–8. doi: 10.1016/j.coi.2008.09.001
26. Taylor JJ, Martinez RJ, Titcombe PJ, Barsness LO, Thomas SR, Zhang N, et al. Deletion and anergy of polyclonal B cells specific for ubiquitous membrane-bound self-antigen. J Exp Med. (2012) 209:2065–77. doi: 10.1084/jem.20112272
27. Tiller T, Tsuiji M, Yurasov S, Velinzon K, Nussenzweig MC, Wardemann H. Autoreactivity in human IgG+ memory B cells. Immunity. (2007) 26:205–13. doi: 10.1016/j.immuni.2007.01.009
28. Wardemann H, Yurasov S, Schaefer A, Young JW, Meffre E, Nussenzweig MC. Predominant autoantibody production by early human B cell precursors. Science. (2003) 301:1374–7. doi: 10.1126/science.1086907
29. Benckert J, Schmolka N, Kreschel C, Zoller MJ, Sturm A, Wiedenmann B, et al. The majority of intestinal IgA+ and IgG+ plasmablasts in the human gut are antigen-specific. J Clin Invest. (2011) 121:1946–55. doi: 10.1172/JCI44447
30. Manivel V, Sahoo NC, Salunke DM, Rao KV. Maturation of an antibody response is governed by modulations in flexibility of the antigen-combining site. Immunity. (2000) 13:611–20. doi: 10.1016/S1074-7613(00)00061-3
31. Manivel V, Bayiroglu F, Siddiqui Z, Salunke DM, Rao KV. The primary antibody repertoire represents a linked network of degenerate antigen specificities. J Immunol. (2002) 169:888–97. doi: 10.4049/jimmunol.169.2.888
32. Harriman WD, Collarini EJ, Sperinde GV, Strandh M, Fatholahi MM, Dutta A, et al. Antibody discovery via multiplexed single cell characterization. J Immunol Methods. (2009) 341:135–45. doi: 10.1016/j.jim.2008.11.009
33. Saletti G, Cuburu N, Yang JS, Dey A, Czerkinsky C. Enzyme-linked immunospot assays for direct ex vivo measurement of vaccine-induced human humoral immune responses in blood. Nat Protoc. (2013) 8:1073–87. doi: 10.1038/nprot.2013.058
34. Walsh PN, Friedrich DP, Williams JA, Smith RJ, Stewart TL, Carter DK, et al. Optimization and qualification of a memory B-cell ELISpot for the detection of vaccine-induced memory responses in HIV vaccine trials. J Immunol Methods. (2013) 394:84–93. doi: 10.1016/j.jim.2013.05.007
35. Czerkinsky CC, Nilsson LA, Nygren H, Ouchterlony O, Tarkowski A. A solid-phase enzyme-linked immunospot (ELISPOT) assay for enumeration of specific antibody-secreting cells. J Immunol Methods. (1983) 65:109–21.
36. Blanchard-Rohner G, Galli G, Clutterbuck EA, Pollard AJ. Comparison of a limiting dilution assay and ELISpot for detection of memory B-cells before and after immunisation with a protein-polysaccharide conjugate vaccine in children. J Immunol Methods. (2010) 358:46–55. doi: 10.1016/j.jim.2010.03.014
37. Yu X, McGraw PA, House FS, Crowe JE Jr. An optimized electrofusion-based protocol for generating virus-specific human monoclonal antibodies. J Immunol Methods. (2008) 336:142–51. doi: 10.1016/j.jim.2008.04.008
38. Traggiai E, Becker S, Subbarao K, Kolesnikova L, Uematsu Y, Gismondo MR, et al. An efficient method to make human monoclonal antibodies from memory B cells: potent neutralization of SARS coronavirus. Nat Med. (2004) 10:871–5. doi: 10.1038/nm1080
39. Gross A, Schoendube J, Zimmermann S, Steeb M, Zengerle R, Koltay P. Technologies for single-cell isolation. Int J Mol Sci. (2015) 16:16897–919. doi: 10.3390/ijms160816897
40. DeKosky BJ, Ippolito GC, Deschner RP, Lavinder JJ, Wine Y, Rawlings BM, et al. High-throughput sequencing of the paired human immunoglobulin heavy and light chain repertoire. Nat Biotechnol. (2013) 31:166–9. doi: 10.1038/nbt.2492
41. Wang B, Kluwe CA, Lungu OI, DeKosky BJ, Kerr SA, Johnson EL, et al. Facile discovery of a diverse panel of anti-ebola virus antibodies by immune repertoire mining. Sci Rep. (2015) 5:13926. doi: 10.1038/srep13926
42. Moody MA, Haynes BF. Antigen-specific B cell detection reagents: use and quality control. Cytometry A. (2008) 73:1086–92. doi: 10.1002/cyto.a.20599
43. Allie SR, Bradley JE, Mudunuru U, Schultz MD, Graf BA, Lund FE, et al. The establishment of resident memory B cells in the lung requires local antigen encounter. Nat Immunol. (2019) 20:97–108. doi: 10.1038/s41590-018-0260-6
44. DeWitt WS, Yu KKQ, Wilburn DB, Sherwood A, Vignali M, Day CL, et al. A diverse lipid antigen-specific TCR repertoire is clonally expanded during active tuberculosis. J Immunol. (2018) 201:888–96. doi: 10.4049/jimmunol.1800186
45. Franz B, May KF Jr, Dranoff G, Wucherpfennig K. Ex vivo characterization and isolation of rare memory B cells with antigen tetramers. Blood. (2011) 118:348–57. doi: 10.1182/blood-2011-03-341917
46. Gaebler C, Gruell H, Velinzon K, Scheid JF, Nussenzweig MC, Klein F. Isolation of HIV-1-reactive antibodies using cell surface-expressed gp160Deltac(BaL.). J Immunol Methods. (2013) 397:47–54. doi: 10.1016/j.jim.2013.09.003
47. Hamilton JA, Li J, Wu Q, Yang P, Luo B, Li H, et al. General approach for tetramer-based identification of autoantigen-reactive B cells: characterization of La- and snRNP-reactive B cells in autoimmune BXD2 mice. J Immunol. (2015) 194:5022–34. doi: 10.4049/jimmunol.1402335
48. Havenar-Daughton C, Sarkar A, Kulp DW, Toy L, Hu X, Deresa I, et al. The human naive B cell repertoire contains distinct subclasses for a germline-targeting HIV-1 vaccine immunogen. Sci Transl Med. (2018) 10:eaat0381. doi: 10.1126/scitranslmed.aat0381
49. Khaskhely N, Mosakowski J, Thompson RS, Khuder S, Smithson SL, Westerink MA. Phenotypic analysis of pneumococcal polysaccharide-specific B cells. J Immunol. (2012) 188:2455–63. doi: 10.4049/jimmunol.1102809
50. Laudenbach M, Tucker AM, Runyon SP, Carroll FI, Pravetoni M. The frequency of early-activated hapten-specific B cell subsets predicts the efficacy of vaccines for nicotine dependence. Vaccine. (2015) 33:6332–9. doi: 10.1016/j.vaccine.2015.09.015
51. Malkiel S, Jeganathan V, Wolfson S, Manjarrez Orduno N, Marasco E, Aranow C, et al. Checkpoints for autoreactive B cells in the peripheral blood of lupus patients assessed by flow cytometry. Arthritis Rheumatol. (2016) 68:2210–20. doi: 10.1002/art.39710
52. Moody MA, Yates NL, Amos JD, Drinker MS, Eudailey JA, Gurley TC, et al. HIV-1 gp120 vaccine induces affinity maturation in both new and persistent antibody clonal lineages. J Virol. (2012) 86:7496–507. doi: 10.1128/JVI.00426-12
53. Nanton MR, Lee SJ, Atif SM, Nuccio SP, Taylor JJ, Baumler AJ, et al. Direct visualization of endogenous Salmonella-specific B cells reveals a marked delay in clonal expansion and germinal center development. Eur J Immunol. (2015) 45:428–41. doi: 10.1002/eji.201444540
54. Scherer EM, Smith RA, Simonich CA, Niyonzima N, Carter JJ, Galloway DA. Characteristics of memory B cells elicited by a highly efficacious HPV vaccine in subjects with no pre-existing immunity. PLoS Pathog. (2014) 10:e1004461. doi: 10.1371/journal.ppat.1004461
55. Whittle JR, Wheatley AK, Wu L, Lingwood D, Kanekiyo M, Ma SS, et al. Flow cytometry reveals that H5N1 vaccination elicits cross-reactive stem-directed antibodies from multiple Ig heavy-chain lineages. J Virol. (2014) 88:4047–57. doi: 10.1128/JVI.03422-13
56. Weitkamp JH, Kallewaard N, Kusuhara K, Feigelstock D, Feng N, Greenberg HB, et al. Generation of recombinant human monoclonal antibodies to rotavirus from single antigen-specific B cells selected with fluorescent virus-like particles. J Immunol Methods. (2003) 275:223–37. doi: 10.1016/S0022-1759(03)00013-9
57. McHeyzer-Williams LJ, Cool M, McHeyzer-Williams MG. Antigen-specific B cell memory: expression and replenishment of a novel b220(-) memory b cell compartment. J Exp Med. (2000) 191:1149–66. doi: 10.1084/jem.191.7.1149
58. Newman J, Rice JS, Wang C, Harris SL, Diamond B. Identification of an antigen-specific B cell population. J Immunol Methods. (2003) 272:177–87. doi: 10.1016/S0022-1759(02)00499-4
59. McHeyzer-Williams MG, McHeyzer-Williams LJ, Fanelli Panus J, Bikah G, Pogue-Caley RR, Driver DJ, et al. Antigen-specific immunity. Th cell-dependent B cell responses. Immunol Res. (2000) 22:223–36. doi: 10.1385/IR:22:2-3:223
60. Sholukh AM, Mukhtar MM, Humbert M, Essono SS, Watkins JD, Vyas HK, et al. Isolation of monoclonal antibodies with predetermined conformational epitope specificity. PLoS ONE. (2012) 7:e38943. doi: 10.1371/journal.pone.0038943
61. Pinder CL, Kratochvil S, Cizmeci D, Muir L, Guo Y, Shattock RJ, et al. Isolation and characterization of antigen-specific plasmablasts using a novel flow cytometry-based Ig capture assay. J Immunol. (2017) 199:4180–8. doi: 10.4049/jimmunol.1701253
62. Taylor JJ, Pape KA, Jenkins MK. A germinal center-independent pathway generates unswitched memory B cells early in the primary response. J Exp Med. (2012) 209:597–606. doi: 10.1084/jem.20111696
63. Suzuki K, Grigorova I, Phan TG, Kelly LM, Cyster JG. Visualizing B cell capture of cognate antigen from follicular dendritic cells. J Exp Med. (2009) 206:1485–93. doi: 10.1084/jem.20090209
64. Garside P, Ingulli E, Merica RR, Johnson JG, Noelle RJ, Jenkins MK. Visualization of specific B and T lymphocyte interactions in the lymph node. Science. (1998) 281:96–9.
65. Krautler NJ, Suan D, Butt D, Bourne K, Hermes JR, Chan TD, et al. Differentiation of germinal center B cells into plasma cells is initiated by high-affinity antigen and completed by Tfh cells. J Exp Med. (2017) 214:1259–67. doi: 10.1084/jem.20161533
66. Zhu J, Peng T, Johnston C, Phasouk K, Kask AS, Klock A, et al. Immune surveillance by CD8alphaalpha+ skin-resident T cells in human herpes virus infection. Nature. (2013) 497:494–7. doi: 10.1038/nature12110
67. Cahalan MD, Parker I, Wei SH, Miller MJ. Two-photon tissue imaging: seeing the immune system in a fresh light. Nat Rev Immunol. (2002) 2:872–80. doi: 10.1038/nri935
68. Miller MJ, Wei SH, Parker I, Cahalan MD. Two-photon imaging of lymphocyte motility and antigen response in intact lymph node. Science. (2002) 296:1869–73. doi: 10.1126/science.1070051
69. Zaretsky I, Atrakchi O, Mazor RD, Stoler-Barak L, Biram A, Feigelson SW, et al. ICAMs support B cell interactions with T follicular helper cells and promote clonal selection. J Exp Med. (2017) 214:3435–48. doi: 10.1084/jem.20171129
70. Victora GD, Schwickert TA, Fooksman DR, Kamphorst AO, Meyer-Hermann M, Dustin ML, et al. Germinal center dynamics revealed by multiphoton microscopy with a photoactivatable fluorescent reporter. Cell. (2010) 143:592–605. doi: 10.1016/j.cell.2010.10.032
71. Lee EC, Liang Q, Ali H, Bayliss L, Beasley A, Bloomfield-Gerdes T, et al. Complete humanization of the mouse immunoglobulin loci enables efficient therapeutic antibody discovery. Nat Biotechnol. (2014) 32:356–63. doi: 10.1038/nbt.2825
72. Sok D, Briney B, Jardine JG, Kulp DW, Menis S, Pauthner M, et al. Priming HIV-1 broadly neutralizing antibody precursors in human Ig loci transgenic mice. Science. (2016) 353:1557–60. doi: 10.1126/science.aah3945
73. Newell EW, Sigal N, Bendall SC, Nolan GP, Davis MM. Cytometry by time-of-flight shows combinatorial cytokine expression and virus-specific cell niches within a continuum of CD8+ T cell phenotypes. Immunity. (2012) 36:142–52. doi: 10.1016/j.immuni.2012.01.002
74. Newell EW, Sigal N, Nair N, Kidd BA, Greenberg HB, Davis MM. Combinatorial tetramer staining and mass cytometry analysis facilitate T-cell epitope mapping and characterization. Nat Biotechnol. (2013) 31:623–9. doi: 10.1038/nbt.2593
75. Bentzen AK, Such L, Jensen KK, Marquard AM, Jessen LE, Miller NJ, et al. T cell receptor fingerprinting enables in-depth characterization of the interactions governing recognition of peptide-MHC complexes. Nat Biotechnol. (2018) 36: 1191–6. doi: 10.1038/nbt.4303
76. Bentzen AK, Marquard AM, Lyngaa R, Saini SK, Ramskov S, Donia M, et al. Large-scale detection of antigen-specific T cells using peptide-MHC-I multimers labeled with DNA barcodes. Nat Biotechnol. (2016) 34:1037–45. doi: 10.1038/nbt.3662
77. Beckett D, Kovaleva E, Schatz PJ. A minimal peptide substrate in biotin holoenzyme synthetase-catalyzed biotinylation. Protein Sci. (1999) 8:921–9.
78. Schwarz E, Oesterhelt D, Reinke H, Beyreuther K, Dimroth P. The sodium ion translocating oxalacetate decarboxylase of Klebsiella pneumoniae. Sequence of the biotin-containing alpha-subunit and relationship to other biotin-containing enzymes. J Biol Chem. (1988) 263:9640–5.
79. Pape KA, Maul RW, Dileepan T, Paustian AS, Gearhart PJ, Jenkins MK. Naive B cells with high-avidity germline-encoded antigen receptors produce persistent IgM(+) and transient IgG(+) memory B cells. Immunity. (2018) 48:1135–1143.e4. doi: 10.1016/j.immuni.2018.04.019
80. Pape KA, Taylor JJ, Maul RW, Gearhart PJ, Jenkins MK. Different B cell populations mediate early and late memory during an endogenous immune response. Science. (2011) 331:1203–7. doi: 10.1126/science.1201730
81. Chao G, Lau WL, Hackel BJ, Sazinsky SL, Lippow SM, Wittrup KD. Isolating and engineering human antibodies using yeast surface display. Nat Protoc. (2006) 1:755–68. doi: 10.1038/nprot.2006.94
82. Smith MJ, Hinman RM, Getahun A, Kim S, Packard TA, Cambier JC. Silencing of high-affinity insulin-reactive B lymphocytes by anergy and impact of the NOD genetic background in mice. Diabetologia. (2018) 61:2621–32. doi: 10.1007/s00125-018-4730-z
83. Smith MJ, Packard TA, O'Neill SK, Hinman RM, Rihanek M, Gottlieb PA, et al. Detection and enrichment of rare antigen-specific B cells for analysis of phenotype and function. J Vis Exp. (2017) 120: 1–8. doi: 10.3791/55382
84. Mason DY, Jones M, Goodnow CC. Development and follicular localization of tolerant B lymphocytes in lysozyme/anti-lysozyme IgM/IgD transgenic mice. Int Immunol. (1992) 4:163–75.
85. Shih TA, Roederer M, Nussenzweig MC. Role of antigen receptor affinity in T cell-independent antibody responses in vivo. Nat Immunol. (2002) 3:399–406. doi: 10.1038/ni776
86. Herzenberg LA, Stall AM, Braun J, Weaver D, Baltimore D, Herzenberg LA, et al. Depletion of the predominant B-cell population in immunoglobulin mu heavy-chain transgenic mice. Nature. (1987) 329:71–3. doi: 10.1038/329071a0
87. Rusconi S, Kohler G. Transmission and expression of a specific pair of rearranged immunoglobulin mu and kappa genes in a transgenic mouse line. Nature. (1985) 314:330–4.
88. Dougan SK, Ogata S, Hu CC, Grotenbreg GM, Guillen E, Jaenisch R, et al. IgG1+ ovalbumin-specific B-cell transnuclear mice show class switch recombination in rare allelically included B cells. Proc Natl Acad Sci USA. (2012) 109:13739–44. doi: 10.1073/pnas.1210273109
89. Kenny JJ, Finkelman F, Macchiarini F, Kopp WC, Storb U, Longo DL. Alteration of the B cell surface phenotype, immune response to phosphocholine and the B cell repertoire in M167 mu plus kappa transgenic mice. J Immunol. (1989) 142:4466–74.
90. Storb U, Pinkert C, Arp B, Engler P, Gollahon K, Manz J, et al. Transgenic mice with mu and kappa genes encoding antiphosphorylcholine antibodies. J Exp Med. (1986) 164:627–41. doi: 10.1084/jem.164.2.627
91. Nemazee DA, Burki K. Clonal deletion of B lymphocytes in a transgenic mouse bearing anti-MHC class I antibody genes. Nature. (1989) 337:562–6.
92. Jacobsen JT, Mesin L, Markoulaki S, Schiepers A, Cavazzoni CB, Bousbaine D, et al. One-step generation of monoclonal B cell receptor mice capable of isotype switching and somatic hypermutation. J Exp Med. (2018) 215:2686–95. doi: 10.1084/jem.20172064
93. Okamoto M, Murakami M, Shimizu A, Ozaki S, Tsubata T, Kumagai S, et al. A transgenic model of autoimmune hemolytic anemia. J Exp Med. (1992) 175:71–9. doi: 10.1084/jem.175.1.71
94. Erikson J, Radic MZ, Camper SA, Hardy RR, Carmack C, Weigert M. Expression of anti-DNA immunoglobulin transgenes in non-autoimmune mice. Nature. (1991) 349:331–4.
95. Chen C, Radic MZ, Erikson J, Camper SA, Litwin S, Hardy RR, et al. Deletion and editing of B cells that express antibodies to DNA. J Immunol. (1994) 152:1970–82.
96. Hannum LG, Ni D, Haberman AM, Weigert MG, Shlomchik MJ. A disease-related rheumatoid factor autoantibody is not tolerized in a normal mouse: implications for the origins of autoantibodies in autoimmune disease. J Exp Med. (1996) 184:1269–78.
97. Shlomchik MJ, Zharhary D, Saunders T, Camper SA, Weigert MG. A rheumatoid factor transgenic mouse model of autoantibody regulation. Int Immunol. (1993) 5:1329–41.
98. Litzenburger T, Fassler R, Bauer J, Lassmann H, Linington C, Wekerle H, et al. B lymphocytes producing demyelinating autoantibodies: development and function in gene-targeted transgenic mice. J Exp Med. (1998) 188:169–80. doi: 10.1084/jem.188.1.169
99. Hulbert C, Riseili B, Rojas M, Thomas JW. B cell specificity contributes to the outcome of diabetes in nonobese diabetic mice. J Immunol. (2001) 167:5535–8. doi: 10.4049/jimmunol.167.10.5535
100. Jardine JG, Ota T, Sok D, Pauthner M, Kulp DW, Kalyuzhniy O, et al. HIV-1 VACCINES. Priming a broadly neutralizing antibody response to HIV-1 using a germline-targeting immunogen. Science. (2015) 349:156–61. doi: 10.1126/science.aac5894
101. Lin YC, Pecetta S, Steichen JM, Kratochvil S, Melzi E, Arnold J, et al. One-step CRISPR/Cas9 method for the rapid generation of human antibody heavy chain knock-in mice. EMBO J. (2018) 37:e99243. doi: 10.15252/embj.201899243
102. Ota T, Doyle-Cooper C, Cooper AB, Doores KJ, Aoki-Ota M, Le K, et al. B cells from knock-in mice expressing broadly neutralizing HIV antibody b12 carry an innocuous B cell receptor responsive to HIV vaccine candidates. J Immunol. (2013) 191:3179–85. doi: 10.4049/jimmunol.1301283
103. Tian M, Cheng C, Chen X, Duan H, Cheng HL, Dao M, et al. Induction of HIV neutralizing antibody lineages in mice with diverse precursor repertoires. Cell. (2016) 166:1471–1484.e18. doi: 10.1016/j.cell.2016.07.029
104. Rongvaux A, Willinger T, Martinek J, Strowig T, Gearty SV, Teichmann LL, et al. Development and function of human innate immune cells in a humanized mouse model. Nat Biotechnol. (2014) 32:364–72. doi: 10.1038/nbt.2858
105. Shahi P, Kim SC, Haliburton JR, Gartner ZJ, Abate AR. Abseq: ultrahigh-throughput single cell protein profiling with droplet microfluidic barcoding. Sci Rep. (2017) 7:44447. doi: 10.1038/srep44447
106. Peterson VM, Zhang KX, Kumar N, Wong J, Li L, Wilson DC, et al. Multiplexed quantification of proteins and transcripts in single cells. Nat Biotechnol. (2017) 35:936–9. doi: 10.1038/nbt.3973
Keywords: B cells, B lymphocyte subsets, humoral immune response, antigens, vaccines
Citation: Boonyaratanakornkit J and Taylor JJ (2019) Techniques to Study Antigen-Specific B Cell Responses. Front. Immunol. 10:1694. doi: 10.3389/fimmu.2019.01694
Received: 30 April 2019; Accepted: 08 July 2019;
Published: 24 July 2019.
Edited by:
Botond Z. Igyártó, Baylor Scott & White Research Institute (BSWRI), United StatesReviewed by:
Kishore Alugupalli, Thomas Jefferson University, United StatesJohn Cambier, University of Colorado School of Medicine, United States
Copyright © 2019 Boonyaratanakornkit and Taylor. This is an open-access article distributed under the terms of the Creative Commons Attribution License (CC BY). The use, distribution or reproduction in other forums is permitted, provided the original author(s) and the copyright owner(s) are credited and that the original publication in this journal is cited, in accordance with accepted academic practice. No use, distribution or reproduction is permitted which does not comply with these terms.
*Correspondence: Jim Boonyaratanakornkit, amJvb255YXJAZnJlZGh1dGNoLm9yZw==