- Department of Infectious Diseases, College of Veterinary Sciences, University of Georgia, Athens, GA, United States
Well-adapted pathogens have evolved to survive the many challenges of a robust immune response. Defending against all host antimicrobials simultaneously would be exceedingly difficult, if not impossible, so many co-evolved organisms utilize immunomodulatory tools to subvert, distract, and/or evade the host immune response. Bordetella spp. present many examples of the diversity of immunomodulators and an exceptional experimental system in which to study them. Recent advances in this experimental system suggest strategies for interventions that tweak immunity to disrupt bacterial immunomodulation, engaging more effective host immunity to better prevent and treat infections. Here we review advances in the understanding of respiratory pathogens, with special focus on Bordetella spp., and prospects for the use of immune-stimulatory interventions in the prevention and treatment of infection.
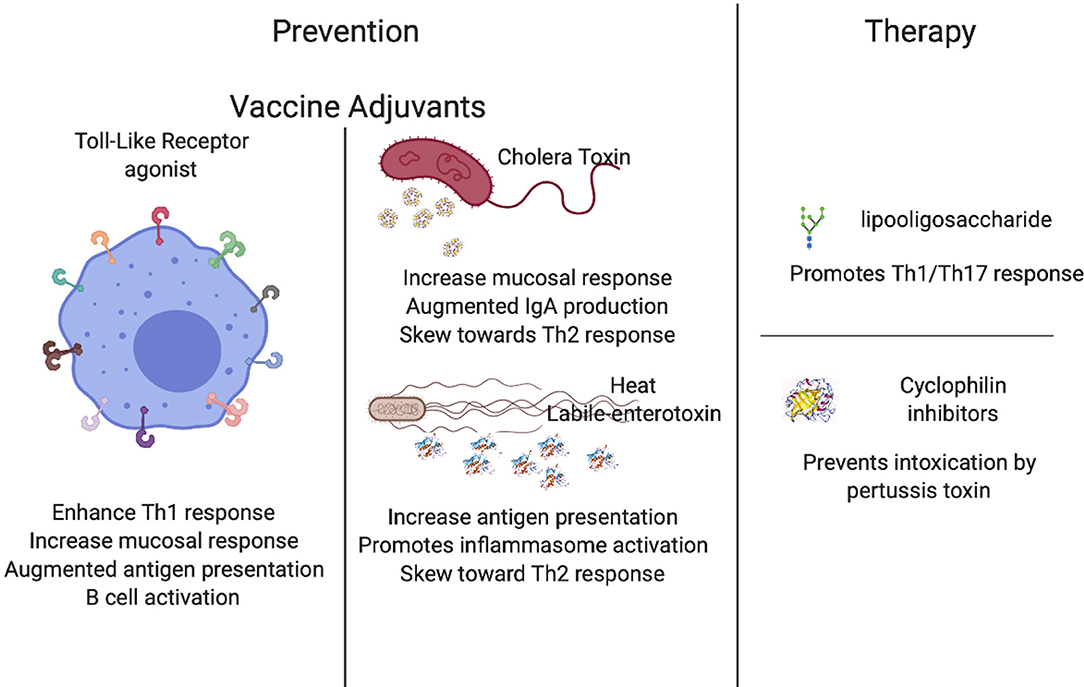
Graphical Abstract. Areas of investigation focused on the use of immunomodulation in prevention and therapy of infectious diseases. Created with BioRender.
Introduction to the Strategy of Immunomodulation for Health
We are exposed to vast numbers of pathogens during our lifespan, but only a small number manage to cause disease. Invading bacteria face a hostile environment in hosts with arrays of antimicrobial compounds and components of immunity. To persist in such an environment, bacteria must find a way to survive this onslaught of antibacterials. The strategy of resisting them all may be exceedingly challenging or impossible; instead, most of the best-studied pathogens have mechanisms that allow them to evade the full effects of host defenses (1–12). In this review, we will consider examples of novel approaches in vaccine and therapeutic development that have been guided by the better understanding of bacterial immunomodulatory abilities. We will focus on findings with Bordetella spp., considering novel adjuvants that enhance host immune response and new immunostimulatory therapies that can augment the most effective aspects of the host immune response. The results highlighted in this review demonstrate that the manipulation and/or disruption of bacterial immunomodulatory properties are providing a highly promising approach that could replace antibiotics in a near future. Understanding the mechanisms that bacteria utilize to manipulate host immune response, as well as the immune signaling pathways that lead to greater protective immunity, can guide the development of targeted interventions that can enhance the host immune response to more effectively kill the bacterial hazard.
The Bordetellae; Biology; and Experimental System
Pertussis disease is caused by B. pertussis, a highly transmissible human pathogen that causes a respiratory illness also known as the 100-day cough (13). Among the proposed reasons for its resurgence are waning immunity (13), the end of the “honeymoon period” (14), the past vaccination calendar (15), and the failure of the current acellular vaccine to confer sterilizing immunity and long-lasting herd immunity. The increase in the number of cases is associated with more advanced diagnostic tools than ever before, allowing for an increase in the number of identified cases (16–31), but also with increased morbidity and mortality that creates an unambiguous imperative for improved prevention methods.
Vaccination has greatly increased life expectancy by preventing several historically notorious infectious diseases (32–36). However, we are witnessing a rise in several preventable diseases previously thought to be controlled (37), such as pertussis (38). Around 1945, a whole-cell vaccine against B. pertussis was introduced, causing an unprecedented decrease in the number of reported pertussis cases. However, due to undesirable adverse effects such as fever, erythema, swelling, drowsiness and others, this was replaced in several industrialized countries by an acellular vaccine that contains between 3 to 5 bacterial proteins (39–44). Despite the fact that both types of vaccines generate antibodies that impede bacterial adhesion and have bactericidal action, these have not been sufficient to halt the increase in the number of cases. In response to this increase a boost was introduced to extend immunological memory, and new vaccination strategies targeted to pregnant women and close family have also been introduced as an attempt to protect highly susceptible newborns (45–48).
As the number of cases continues to increase, the scientific community is working to understand the causes that drive this reemergence (13, 49). Amongst the proposed causes of this increase are, limitation to the protection conferred by the current acellular vaccine. Not only does the acquired anamnestic response wane rapidly (50), but the acellular vaccine still allows for bacterial colonization of the nasal cavity and shedding. Combined, these factors illuminate the fact that the current vaccines used in most industrialized countries still permit transmission of pertussis from host to host (51–54), which has even more significant impacts when considered in tandem with the rise of anti-vaccination movements. Yet another cause for the increase is the differences detected in the immune response triggered by the whole cell vaccine (Th17) vs. the acellular vaccine (Th2) (51, 55–57). It is important to highlight that while neither whole-cell nor acellular vaccines confer long-lasting immunity, and the merits of both responses have been debated in recent years, the general consensus agrees on advantages to skewing T cell response toward Th1/Th17 immunity (58–61).
The “gold standard” of immunity to pertussis is considered to be the classical Th1/Th17 T cell response induced by convalescent immunity (62); however, there is significant cumulative evidence that infection-induced immunity is imperfect and shorter-lived than it could be (50). Current discoveries contribute to better understanding of the immune response to Bordetellae, and the important role that CD4 resident T cells play in a local memory response has been recently demonstrated (63). Another hypothesis is that Bordetellae are evolving, and due to the genome plasticity and adaptability of this pathogen, current isolates of B. pertussis have lost some of the antigens included in the acellular vaccine. This phenomenon is referred to as “vaccine driven evolution,” which helps justify why immunity is not as robust as it has previously been (64–67).
These are only some of the potential causes that are currently being considered, and it is most likely an uneven combination of all of them that is truly driving this pertussis resurgence. Although the whole-cell vaccine is still used, the trend is shifting toward a safer acellular vaccine, and efforts on improving their performance and the length of protective memory these generate will be discussed in this manuscript.
The current strategy for the development of vaccines is driven by the hypothesis that antibodies provide strong protection. As a consequence, most of the current acellular vaccines are highly safe and generate a rapid antibody response that is protective, albeit limited (68, 69). Importantly, infection triggers a complex and well-orchestrated sequence of responses involving many interacting components of innate and adaptive immunity, directed by several signaling pathways that present numerable known, and probably many more unknown, opportunities to interfere in the succession of events that can skew the resulting immune response.
Bordetellae harbor multiple mechanisms that allow them to modulate the host immune response (1, 70, 71). Some of the proteins that these organisms utilize to manipulate immune cells include adenylate cyclase toxin (ACT), a pore forming protein that leads to the deregulation of cAMP levels within target cells (72, 73); type 3 secretion system (T3SS), a needle-like structure that injects toxins within mammalian cells (74–76); a type 6 secretion system that uses a phage-like mechanism to inject molecules (77); pertussis toxin (PTX), which prevents G proteins from interacting with G protein-coupled receptors on the cell membrane and therefore interfering with intracellular communication (78–80); and filamentous hemagglutinin (FHA), which binds signaling receptors, enables adhesion to epithelial cells and interferes with cytokine production (81, 82). Based on these studies of various immunomodulators we can now begin to adjust the way we design preventative and responsive medications to fight bacterial infections in more effective ways.
A good understanding of the sequential reactions of the immune response (and bacterial manipulation of them) is key to improving the induction and maintenance of robust long-lasting protective immunity. Some of the Bordetella spp. virulence factors are already being investigated for treatments, such as PTX for human immunodeficiency virus (HIV) treatment (83–89). Understanding how we can alter bacterial ability to sense and respond to the host to modulate its response can lead to treatments and therapies that focus on the enhancement of more appropriate and effective host immune responses.
Immunotherapy in Prevention
Adjuvants
The Bordetella pertussis acellular vaccine has not completely blocked the spread of pertussis because it allows for colonization of the nasal cavity (48) and provides only temporary protection (13). Adjuvants are well-documented for their potential to increase vaccine performance, and some adjuvants such as CpG oligodeoxynuceotides or alum are commonly found in vaccine formulations (90, 91). There are a plethora of adjuvants that can potentiate the performance of a vaccine and can be classified into two main groups: Toll-like receptors agonists (92–94) and mucosal adjuvants (58, 95–97). These two distinct classes have been closely considered for their contributions to pertussis vaccines as well as therapeutics (98–103), yielding highly promising enhancing properties.
Toll-Like Receptors Agonists
Toll-Like Receptors (TLRs) are highly sophisticated sentinels that recognize specific pathogen-associated molecular patterns (PAMPs). The differential activation of TLRs is one of the main determinants for an efficient immune response against pathogens. Under this premise, researchers have been working on the addition of TLR agonists to vaccines with the expectation that activating different TLRs will command the type of T cell response produced (104) and will ultimately enhance host protective immunity (105).
One of the best studied Toll-Like Receptors is TLR2, which recognizes a broad spectrum of bacterial cell wall components, including lipopolypeptides, peptidoglycan, and lipochoic acids, that trigger different signals that shape the immune response against the bacterial threat (106). It has been demonstrated that the use of TLR2 agonists as adjuvants to already developed vaccines increases immunity, especially in neonates (93). This feature is highly relevant to the design of vaccines against diseases that primarily affect newborns and young infants (93). Moreover, TLR2 agonists in combination with the BCG vaccine can enhance protection against Mycobacterium tuberculosis (107), skewing the cellular response toward Th1 (100), and resulting in a more robust protective memory response, further promoting its use in vaccinology. TLR2 has been also correlated with an efficient response to B. pertussis infections (108), and some preliminary data has revealed that the use of these agonists enhances protection against infection by pertussis (58, 100). Altogether, these data suggest that TLR2 agonists may be promising candidates to combine with current or new vaccines to enhance the protective response.
Similarly, TLR4 appears a good candidate for vaccine enhancement because it recognizes lipopolysaccharide (LPS) molecules, which are commonly present on the surface of most bacteria. Agonists of TLR4 enhance the performance of several vaccines including viral, bacterial, and even mycobacterial (109–113). One important aspect is its promotion of mucosal immunity (114–116), which is critical for the generation of protection against certain infections including gut and respiratory diseases like pertussis (117–119), although this increase is achieved via mucosal delivery of the vaccine rather than systemic (120). Molecular evidence has revealed that the addition of a TLR4 ligand to the acellular pertussis vaccine resulted in a shift from a Th2-dominant response to additional induction of Th17 (121, 122). The abundant immunological evidence that highlights the role of TLR4 in the immune response to B. pertussis (102, 123–130) indicates that TLR4 agonists are promising candidate for the generation of more robust protective immunity.
TLR5 (131) is also a highly plausible candidate to augment vaccine performance since it recognizes flagella, which are present in a multitude of bacterial species. Previous literature has indicated that ectopic expression of flagella in Bordetella spp. leads to faster clearance of the infection (132), and it was later revealed that TLR5 activates antigen-presenting cells, increasing T cell response (133) (manuscript in preparation), and may ultimately contribute to the more rapid clearance previously reported. In several other microorganisms, the addition of TLR5 agonists have resulted in an increased performance of the vaccine (134–141). Altogether these data suggest that TLR5 agonists could significantly increase the performance of the current acellular pertussis vaccine.
TLR7 recognizes single-stranded RNA (142–153) and has been demonstrated to be a promising vaccine adjuvant for protection against several microorganisms (154, 155). Similar to TLR2, the TLR7 agonist augments immunity in newborns, the most susceptible population (93, 102, 143, 156, 157). The addition of a TLR7 agonist to an alum-adjuvant of pertussis vaccine skewed the immune response toward Th1/Th17 and significantly decreased colonization (98), providing preliminary data to further pursue this agonist in other animal models.
Lastly, TLR9 recognizes unmethylated CpG oligodeoxynucleotides and promotes IL-6 secretion and consequent B cell activation (158–168). It has been demonstrated that enhancement of TLR9 receptors augment activity of antigen-presenting cells in neonates (93, 102, 169). Addition of a TLR9 agonist to the acellular pertussis vaccine resulted in greater stimulation of B and T cells and a shift to Th1, as well as higher antibody titers (81, 170–174), suggesting that an agonist of TLR9 is also a candidate to add to the current pertussis vaccines. These have the potential to be widely used agonists, as most of the current vaccine's efficacy is measured as an increase in antibody titers.
Altogether, these results demonstrate that TLR agonists are great candidates to be used as vaccine adjuvants to increase protective immunity. Interestingly, some of the TLR agonists substantially augment vaccine performance in newborns and infants, which represent the most susceptible population (93, 169) although there are substantial hurdles to applying this knowledge. Moreover, preliminary data obtained with TLR2, and TLR7 agonists demonstrate the improved performance of the current B. pertussis vaccine and indicates that the use of adjuvants can feasibly potentiate and augment the generation of protective immunity (58, 98, 100).
Mucosal Adjuvants
Adjuvants have been used to potentiate, enhance, or accelerate vaccine effects since the 1920s (105) and the field has greatly evolved since. Mucosal adjuvants include cholera toxin, heat-labile enterotoxin, and other compounds have been studied for their particular ability to increase protection on mucosal surfaces (175). These are of extreme importance, not only because of the aforementioned increase in vaccine performance, but also because the delivery method involving intranasal vaccination has a lot of potential for improving the delivery of the vaccine and increasing acceptance among needle-phobic population. In the following paragraphs we will detail the mechanisms of action and the data compiled for some of the most promising mucosal adjuvants.
Cholera toxin (CT) and heat-labile enterotoxin of Escherichia coli (LT) are highly antigenic; however, due to their toxicity, they are not ideal candidates for human therapies. Recently, safe forms of these toxins created via genetic manipulation have been utilized as adjuvants to enhance the function of mucosal vaccines (103, 176–181). The mechanism behind this augmented immune response induced by CT is an increase in the permeability of the mucosal epithelium, enhanced antigen presentation, the consequent promotion of dendritic cell maturation, increased IgA response, and finally, the generation of complex stimulatory and inhibitory effects on T cell proliferation and cytokine production such as IL-4, IL-5, IL-6, and IL-10 that skew the response toward a Th2-type (177, 182). CTA1 is the subunit responsible for the immunomodulatory activity in conjunction with ERdj5 in the endoplasmic reticulum, which is the target for CT. In the absence of ERdj5, mice failed to produce inflammatory cytokines, indicating that CT action requires ERdj5 (183). Similarly, the calcium-binding protein S100A4 is required for efficient antigen presentation and enhanced activity of CT, as it is necessary for the humoral and cellular response (184). CT has been tested as an adjuvant for pertussis vaccine and preliminary data suggests that it substantially improves mucosal protection by augmenting IgA levels (183, 185), and it has even been suggested that this adjuvant may be safe for use in humans (186, 187). Some studies have revealed that conjugation of CT with pertussis toxoid added to the current acellular vaccine (188) or Fimbriae (Fim2) (189) are highly promising candidates to improve the generation of protective immunity from these vaccines.
Similar to CT, the heat-labile enterotoxin from E. coli (LT) promotes an antigen-specific response inducing IgA antibodies, Th17 response, and the enhancement of long-lasting protective immunity (190) while also being safe for use in humans (191). LT promotes maturation of dendritic cells, antigen-specific IL-17 positive cells, and production of IL-1α, IL-1β, and IL-23 by dendritic cells. Trials in animals have revealed the efficacy of this adjuvant at enhancing mucosal response (192). LT promotes dendritic cell maturation enhancing IL-1β production through activation of caspase-1 and the NLRP3 inflammasome complex. Simultaneously, LT enhances LPS-induced IL-1α and IL-23 expression through activation of ERK MAPK in dendritic cells inducing the development of Th17 T cells (193). Interestingly, LT derivatives LTK63 (non-toxic mutant of LT) and LTR72 (which retains partial enzymatic activity) revealed two distinct phenotypes characterized by stimulation of IL-12 and TNF-α production by macrophages, resulting in enhanced Th1 responses with the LTK63 adjuvants. In contrast, LTR72 suppresses LPS-induced IL-12 production, increases type 2 responses, inhibits Th1 response, and facilitates clearance of bacterial burden (194), demonstrating that both subunits of the toxin have particular activities that can be beneficial for the improvement of the current acellular pertussis vaccine.
Another mucosal adjuvant that is widely investigated is retinoic acid, a powerful immunomodulator that interferes with growth, differentiation, and other aspects of the cell life cycle. Importantly, retinoic acid is also essential in the generation of mucosal immunity, the promotion of tolerogenic effects, the generation of a robust innate and adaptive immune response, and moreover, it also acts as a negative regulator of IgE production (195–197). It has been hypothesized that retinoic acid plays a fundamental role in sustaining mucosal homeostasis by down-regulating IgE levels (197). Its performance as an adjuvant has been studied in several organisms and the plethora of results obtained have revealed that retinoic acid is a promising candidate to use as an adjuvant of mucosal vaccines by itself or encapsulated in nanoparticles (198–203). Unfortunately, its activity in conjunction with the pertussis vaccine has not yet been assessed.
The use of biopolymers in mucosally-administered vaccines has substantially improved the current vaccine formulations and has great potential for the future (204). Some of the presently investigated biopolymers include alginate (205–212) and gellan (213, 214). Although these are still in early stages of study, other biopolymers, such as chitosan (95–97, 215–232), starch (233), and β-glucan (234–241), have already been tested in animal trials with encouraging success. While the use of biopolymers is still rising, this area of investigation is highly promising, especially for enhancement of mucosal protection. Mucosal delivery has been explored for pertussis immunization from different approaches that have resulted in hopeful results in which Th17 response was enhanced and the animals were more robustly protected against challenge (58, 170, 242, 243).
To summarize, several mucosal adjuvants are being investigated, some of which are derived from toxins while still others are derived from biopolymers. Both act to enhance the performance of vaccines, particularly those that can be orally or intranasally delivered, usually in cases in which mucosal protection is a key component of immunity. However, these further demonstrate that different strategies and approaches can be used to improve the performance of the current vaccines to produce and enhance individual and herd immunity.
Novel Vaccination Strategies
The combination of BCG and acellular pertussis vaccination has been shown to reduce the mortality rate of pertussis (244–247). Immunological studies unraveling the underlying mechanism by which protection against pertussis is enhanced are necessary. Some groups have focused on the addition of antigens to the current vaccine in order to improve performance. After demonstrating via in vitro experiments that the autotransporter BrkA would be a good candidate to generate antibodies that kill Bordetella spp., BrkA has been tested as an adjuvant of the current acellular pertussis vaccine, the results of which revealed robust lung protection against infection with B. pertussis (248, 249). Two other autotransporters, Vag8 (250, 251) and SphB1, when added to the current pertussis vaccine resulted in improved protection against B. pertussis infection (252). Adenylate cyclase toxin (ACT), when added to a current vaccine formulation significantly decreased inflammation and increased the generation of protective immunity (253, 254). BcfA (colonization factor A) has been used as adjuvant in the current vaccine, and the preliminary data obtained with the murine model reveals that the addition of this adjuvant shifts the T cell response toward Th1/Th17 (255).
Live vaccines have the potential to induce strong mucosal protection, but suffer from concern about their risk. An exciting new vaccine candidate against B. pertussis is the live attenuated vaccine, BPZE1, which has been shown to induce a robust local B and T cell response (256–282) despite genetically engineered mutations that render it relatively safe (283, 284). Excitingly, phase I trials demonstrate that the intranasal formulation of the vaccine transiently colonizes the nasal cavity, leading to the generation of stronger immunity (264, 268).
Several groups are currently working on the development of outer membrane vesicles and outer membrane proteins in protection against B. pertussis as well as cross-protection against several Bordetella spp. and characterizing the immune response as well as protective immunity (285–295). In animal studies, immunization with outer membrane vesicles led to not only better humoral and cellular (Th17) memory, but also to a significant increase in IgA titers, which is one of the major hurdles of current vaccination strategies against this pathogen (296–298). It is important to highlight that the increase in IgA responses upon immunization with outer membrane vesicles is only obtained when these are administrated mucosally (299). The classic delivery for OMV's, which is subcutaneous or intraperitoneal immunization, does not induce IgA responses and this novel delivery method provides a great advance, as it can be administered with more ease and induces an even better immunological response. The increase in mucosal protection has led to efforts toward improved nasal delivery approaches and a thermostable spray containing outer membrane vesicles has been developed. This spray significantly improves delivery and decreases the discomfort other intranasal formulations might cause. Importantly, this delivery method still maintains all the outstanding qualities of the classical delivery of these purified outer membrane vesicles (300).
Finally, another highly promising strategy is focused on the disruption of bacterial ability to manipulate the host immune response. Under the premise that bacteria harbor mechanisms that allow them to sense and respond to host immunity, disrupting these pathways would allow for the generation of more robust protective immunity. A live attenuated vaccine in which immunomodulatory mechanisms are disrupted might confer cross-protection against classical Bordetellae, which are known to share many antigens. Although this is only the first study for this method of vaccine design (manuscript in revision), this novel approach has great potential for the generation of new vaccine candidates and possibly therapeutics.
Immunotherapy in Treatment
LOS-Derived Oligosaccharide Glycoconjugates
Pertussis toxin (PTX) in an inactivated form (PTd) functions as a major protective antigen, stimulating production of toxin-neutralizing antibodies which can protect against damage caused by the toxin, but do not target the bacteria itself (301, 302); however, it also demonstrates possible partial reversion back to its toxic active form (303, 304), which may be responsible for the reactogenicity seen in a small percentage of vaccine recipients. It is also a secretory protein, which is only loosely associated with the cell and is therefore not an ideal target for bactericidal antibodies. A more effective target is an abundant surface component such as the endotoxin lipooligosaccharide (LOS), an LPS analog with a complete absence of the O-specific polysaccharide chain that is produced by several varieties of Gram-negative bacteria (305). LOS provides significant adjuvant properties via induction of IL-12 and 1L-1β that promote Th1 and Th17 responses, respectively (306, 307). It also displays pyrogenic, mitogenic, and endotoxic activity that necessitate its conjugation or conversion to a less destructive form prior to its use in a vaccine.
LOS conjugated to protein carriers filamentous hemagglutinin, bovine serum albumin, and tetanus toxoid (TTd) successfully induce a strong bactericidal response specific to LOS presented on the surface of B. pertussis, leading to complement-mediated destruction of the cell (90, 308, 309). These protein carriers are also surface components, like LOS, and the resulting surface-associated conjugate acts as a strong target for antibody action directed against B. pertussis.
Somewhat surprisingly, another conjugate iteration in which an LOS-derived oligosaccharide is covalently linked with the secretory protein PTX yields a uniquely non-toxic and immunogenic glycoconjugate that retains the antigenic properties of PTX while also inducing the production of bactericidal antibodies. The presumed linkage at the fetuin- and glycoprotein-binding sites of PTX inactivates the enzymatic activity of the protomer A and binding properties of oligomer B, demonstrated using in vitro assays (310). Although the use of LOS appears to be highly promising, in vivo studies still need to be done to assess pharmacological parameters of safety and biodistribution.
Cyclophilin Inhibitors
PTX is internalized in cells via endocytosis and then follows a retrograde transport system to the endoplasmic reticulum. The enzymatically active (A) subunit of PTX, PTS1, detaches from the rest of the toxin in the ER and unfolds due to its thermal instability. It is then transported into the cytosol with the help of cyclophilin (Cyps), an important protein folding helper enzyme that also is required to facilitate membrane translocation from early endosomes into the cytosol of various ADP-ribosylating toxins (311–313). Inhibiting Cyps activity has been shown to in turn inhibit membrane translocation and protect cells from intoxication with PTX and others (311).
Inhibition can be achieved via the approved immunosuppressive drug cyclosporine A (CsA), which specifically inhibits Cyps activity in mammalian cells by binding directly to Cyps and forming a ternary complex. It has been used as the primary agent in immunosuppressive regimens such as grafts and transplants since the 1980s. It is now suggested that CsA might interfere with the translocation of PTS1 from the ER into the cytosol; it may also play a role in reassembling the unfolded PTS1 subunit (311).
In vitro intoxication assays performed on CHO-K1 cells demonstrated that CsA-treated cells were protected from PTX intoxication. Interestingly, up to 50% of CsA is retained intracellularly, even in the absence of extracellular inhibitor, after 18 h (314). Thus, presumably, intracellular Cyps stay inhibited over a longer period of time, explaining the toxin-resistant phenotype. This is also concomitant with the long retention of CsA in different tissues observed after CsA administration in human patients (315, 316). This inhibitor was delivered orally during trials, but its use in a mucosal spray or as a directly injectable vaccine component has yet to be investigated.
Future Directions and Conclusion
Since the years of our notoriously premature celebration of victory over infectious disease, there has been seemingly inexorable retaliation. There is now justifiable concern, shifting toward fear, about the combined threats of increasing antibiotic resistance and the failures of current vaccines due to factors including incomplete vaccine uptake, vaccine-driven evolution and other threats. However, recent advances in our understanding of immunology and the tools to manipulate it present hope for more rational targeted interventions that are focused on enhancing the natural host response. Similarly, improved understanding of strategies and mechanisms by which bacteria modulate the immune response provides new targets for treatment and prevention. In the coming years, we will likely witness an expansion in the field of immunotherapy promoted by a better understanding of the finely tuned interactions of bacteria and host.
Author Contributions
MG: original idea of the manuscript, writing, and editing. HJ: writing and editing. EH: editing and final approval.
Funding
This work was supported by the National Institutes of Health (NIH) (Grant Nos. R21 AI142678-01, R21AI140399, and RO1GM113681) and by the National Institute of Allergy and Infectious Diseases. This work has been also supported by the Catalyst Award (American Lung Association). The funders had no role in the study design, data collection, and interpretation, or the decision to submit the work for publication.
Conflict of Interest
The authors declare that the research was conducted in the absence of any commercial or financial relationships that could be construed as a potential conflict of interest.
Acknowledgments
The authors would like to acknowledge the members of the EH Lab for helpful and fruitful discussions and brainstorming.
References
1. Gestal MC, Whitesides LT, Harvill ET. Integrated signaling pathways mediate Bordetella immunomodulation, persistence, and transmission. Trends Microbiol. (2019) 27:118–30. doi: 10.1016/j.tim.2018.09.010
2. Hancock RE, Nijnik A, Philpott DJ. Modulating immunity as a therapy for bacterial infections. Nat Rev Microbiol. (2012) 10:243–54. doi: 10.1038/nrmicro2745
3. Liu Y, Islam EA, Jarvis GA, Gray-Owen SD, Russell MW. Neisseria gonorrhoeae selectively suppresses the development of Th1 and Th2 cells, and enhances Th17 cell responses, through TGF-β-dependent mechanisms. Mucosal Immunol. (2012) 5:320–31. doi: 10.1038/mi.2012.12
4. Wolfe DN, Karanikas AT, Hester SE, Kennett MJ, Harvill ET. IL-10 induction by Bordetella parapertussis limits a protective IFN-gamma response. J Immunol. (2010) 184:1392–400. doi: 10.4049/jimmunol.0803045
5. Carbonetti NH. Immunomodulation in the pathogenesis of Bordetella pertussis infection and disease. Curr Opin Pharmacol. (2007) 7:272–8. doi: 10.1016/j.coph.2006.12.004
6. Tateda K, Ishii Y, Horikawa M, Matsumoto T, Miyairi S, Pechere JC, et al. The Pseudomonas aeruginosa autoinducer N-3-oxododecanoyl homoserine lactone accelerates apoptosis in macrophages and neutrophils. Infect Immun. (2003) 71:5785–93. doi: 10.1128/IAI.71.10.5785-5793.2003
7. Ritchie AJ, Yam AO, Tanabe KM, Rice SA, Cooley MA. Modification of in vivo and in vitro T- and B-cell-mediated immune responses by the Pseudomonas aeruginosa quorum-sensing molecule N-(3-oxododecanoyl)-L-homoserine lactone. Infect Immun. (2003) 71:4421–31. doi: 10.1128/IAI.71.8.4421-4431.2003
8. Sperandio V. Pathogens' adaptation to the human host. Proc Natl Acad Sci USA. (2018) 115:9342–3. doi: 10.1073/pnas.1813379115
9. Lustri BC, Sperandio V, and Moreira CG. Bacterial chat: intestinal metabolites and signals in host-microbiota-pathogen interactions. Infect Immun. (2017) 85:e00476–17. doi: 10.1128/IAI.00476-17
10. Bäumler AJ, Sperandio V. Interactions between the microbiota and pathogenic bacteria in the gut. Nature. (2016) 535:85–93. doi: 10.1038/nature18849
11. Kendall MM, Sperandio V. What a dinner party! mechanisms and functions of interkingdom signaling in host-pathogen associations. MBio. (2016) 7:e01748. doi: 10.1128/mBio.01748-15
12. Curtis MM, Sperandio V. A complex relationship: the interaction among symbiotic microbes, invading pathogens, and their mammalian host. Mucosal Immunol. (2011) 4:133–8. doi: 10.1038/mi.2010.89
13. Kilgore PE, Salim AM, Zervos MJ, and Schmitt HJ. Pertussis: microbiology, disease, treatment, and prevention. Clin Microbiol Rev. (2016) 29, 449–486. doi: 10.1128/CMR.00083-15
14. Domenech de Cellès M, Magpantay FM, King AA, and Rohani P. The pertussis enigma: reconciling epidemiology, immunology and evolution. Proc Biol Sci. (2016) 283:20152309. doi: 10.1098/rspb.2015.2309
15. Broutin H, Viboud C, Grenfell BT, Miller MA, and Rohani P. Impact of vaccination and birth rate on the epidemiology of pertussis: a comparative study in 64 countries. Proc Biol Sci. (2010) 277:3239–45. doi: 10.1098/rspb.2010.0994
16. Miyashita N, Akaike H, Teranishi H, Kawai Y, Ouchi K, Kato T, et al. Diagnostic value of symptoms and laboratory data for pertussis in adolescent and adult patients. BMC Infect Dis. (2013) 13:129. doi: 10.1186/1471-2334-13-129
17. Subissi L, Rodeghiero C, Martini H, Litzroth A, Huygen K, Leroux-Roels G, et al. Assessment of IgA anti-PT and IgG anti-ACT reflex testing to improve Bordetella pertussis serodiagnosis in recently vaccinated subjects. Clin Microbiol Infect. (2019) pii: S1198-743X(19)30528-2. doi: 10.1016/j.cmi.2019.10.001
18. Gil-Prieto R, Walter S, San-Román-Montero J, Marín-García P, González-Escalada A, Gil-de-Miguel A. Paediatric hospitalizations due to whooping cough in Spain (1997-2017). Vaccine. (2019) 37:6342–7. doi: 10.1016/j.vaccine.2019.09.017
19. Stefanelli P. Pertussis: identification, prevention and control. Adv Exp Med Biol. (2019). doi: 10.1007/5584_2019_408
20. Wu DX, Chen Q, Yao KH, Li L, Shi W, Ke JW, et al. Pertussis detection in children with cough of any duration. BMC Pediatr. (2019) 19:236. doi: 10.1186/s12887-019-1615-3
21. Rabi A, Rokni T, Bennaoui F, Rada N, El Idrissi Slitine N, Draiss G, et al. Epidemiology of pertussis in Marrakech and contribution of molecular diagnosis. Infect Dis. (2019) 51:703–5. doi: 10.1080/23744235.2019.1637537
22. Fumimoto R, Otsuka N, Kamiya H, Sunagawa T, Tanaka-Taya K, Kamachi K, et al. Seroprevalence of IgA and IgM antibodies to Bordetella pertussis in healthy Japanese donors: assessment for the serological diagnosis of pertussis. PLoS ONE. (2019) 14:e0219255. doi: 10.1371/journal.pone.0219255
23. Dou M, Macias N, Shen F, Bard JD, Domínguez DC, Li X. Rapid and accurate diagnosis of the respiratory disease pertussis on a point-of-care biochip. EClinicalMedicine. (2019) 8:72–7. doi: 10.1016/j.eclinm.2019.02.008
24. Dou M, Sanchez J, Tavakoli H, Gonzalez JE, Sun J, Dien Bard J, et al. A low-cost microfluidic platform for rapid and instrument-free detection of whooping cough. Anal Chim Acta. (2019) 1065:71–8. doi: 10.1016/j.aca.2019.03.001
25. Markey K, Douglas-Bardsley A, Asokanathan C, Fry NK, Barkoff AM, Bacci S, et al. Improvement in serological diagnosis of pertussis by external quality assessment. J Med Microbiol. (2019) 68:741–7. doi: 10.1099/jmm.0.000926
26. Damouni Shalabi R, Srugo I, Golan-Shany O, Kugelman A, Bamberger E. Respiratory viruses frequently mimic pertussis in young infants. Pediatr Infect Dis J. (2019) 38:e107–9. doi: 10.1097/INF.0000000000002223
27. Moosa F, du Plessis M, Wolter N, Carrim M, Cohen C, von Mollendorf C, et al. Challenges and clinical relevance of molecular detection of Bordetella pertussis in South Africa. BMC Infect Dis. (2019) 19:276. doi: 10.1186/s12879-019-3869-7
28. Tascini C, Carannante N, Sodano G, Tiberio C, Atripaldi L, Di Caprio G, et al. Neonatal pertussis diagnosis: low procalcitonin level and high lymphocyte count are able to discriminate pertussis from bacterial and viral infections. New Microbiol. (2019) 42:49–51.
29. Choi GS, Huh DH, Han SB, Ahn DH, Kang KR, Kim JA, et al. Enzyme-linked immunosorbent assay for detecting anti-pertussis toxin antibody in mouse. Clin Exp Vaccine Res. (2019) 8:64–9. doi: 10.7774/cevr.2019.8.1.64
30. Saiki-Macedo S, Valverde-Ezeta J, Cornejo-Tapia A, Castillo ME, Petrozzi-Helasvuo V., Aguilar-Luis MA, et al. Identfication of viral and bacterial etiologic agents of the pertussis-like syndrome in children under 5 years old hospitalized. BMC Infect Dis. (2019) 19:75. doi: 10.1186/s12879-019-3671-6
31. Di Matola T, Miele C, Coppola M, Fumi M, Pancione Y, Sale S, et al. Utility of peripheral blood smear in rapid diagnosis of Pertussis. Int J Lab Hematol. (2019) 41:e41–2. doi: 10.1111/ijlh.12947
33. Paff ML, Nuismer SL, Ellington A, Molineux IJ, Bull JJ. Virus wars: using one virus to block the spread of another. PeerJ. (2016) 4:e2166. doi: 10.7717/peerj.2166
34. De Gregorio E, Rappuoli R. Vaccines for the future: learning from human immunology. Microb Biotechnol. (2012) 5:149–55. doi: 10.1111/j.1751-7915.2011.00276.x
35. Frishman WH. Ten secrets to a long life. Am J Med. (2019) 132:564–6. doi: 10.1016/j.amjmed.2018.12.020
36. Roush SW, Murphy TV, and Vaccine-Preventable Disease Table Working Group. Historical comparisons of morbidity and mortality for vaccine-preventable diseases in the United States. JAMA. (2007) 298:2155–63. doi: 10.1001/jama.298.18.2155
37. McKee M, Middleton J. Information wars: tackling the threat from disinformation on vaccines. BMJ. (2019) 365:l2144. doi: 10.1136/bmj.l2144
38. Rohani P, Drake JM. The decline and resurgence of pertussis in the US. Epidemics. (2011) 3:183–8. doi: 10.1016/j.epidem.2011.10.001
39. Goezsy B, Kato L. Sensitizing properties of B. pertussis in the mouse and rat. Rev Can Biol. (1964) 23:427–37.
40. Morrone G, Nunziata B, Picciotto L. [Immunitary response of the infant vaccinated with quadruple DPT-polio vaccine]. Riv Ist Sieroter Ital. (1959) 34:321–9.
41. Chen BL, Chou CT, Huang CT, Wang YT, Ko HH, Huang WC, et al. Studies on diphtheria-pertussis-tetanus combined immunization in children. II. Immune responses after the primary vaccination. J Immunol. (1957) 79:39–45.
42. Ipsen J, Bowen HE. Effects of routine immunization of children with triple vaccine (diphtheria-tetanus-pertussis). Am J Public Health Nations Health. (1955) 45:312–8. doi: 10.2105/AJPH.45.3.312
45. Caboré RN, Maertens K, Dobly A, Leuridan E, Van Damme P, and Huygen K. Influence of maternal vaccination against diphtheria, tetanus, and pertussis on the avidity of infant antibody responses to a pertussis containing vaccine in Belgium. Virulence. (2017) 8: 1245–54. doi: 10.1080/21505594.2017.1296998
46. Gaillard ME, Bottero D, Zurita ME, Carriquiriborde F, Martin Aispuro P, Bartel E, et al. Pertussis maternal immunization: narrowing the knowledge gaps on the duration of transferred protective immunity and on vaccination frequency. Front Immunol. (2017) 8:1099. doi: 10.3389/fimmu.2017.01099
47. Gkentzi D, Katsakiori P, Marangos M, Hsia Y, Amirthalingam G, Heath PT, et al. Maternal vaccination against pertussis: a systematic review of the recent literature. Arch Dis Child Fetal Neonatal Ed. (2017) 102:F456–63. doi: 10.1136/archdischild-2016-312341
48. Warfel JM, Papin JF, Wolf RF, Zimmerman LI, Merkel TJ. Maternal and neonatal vaccination protects newborn baboons from pertussis infection. J Infect Dis. (2014) 210:604–10. doi: 10.1093/infdis/jiu090
49. Black RE, Cousens S, Johnson HL, Lawn JE, Rudan I, Bassani DG, et al. Global, regional, and national causes of child mortality in 2008: a systematic analysis. Lancet. (2010) 375:1969–87. doi: 10.1016/S0140-6736(10)60549-1
50. Wendelboe AM, Van Rie A, Salmaso S, Englund JA. Duration of immunity against pertussis after natural infection or vaccination. Pediatr Infect Dis J. (2005) 24:S58–61. doi: 10.1097/01.inf.0000160914.59160.41
51. Warfel JM, Zimmerman LI, Merkel TJ. Acellular pertussis vaccines protect against disease but fail to prevent infection and transmission in a nonhuman primate model. Proc Natl Acad Sci USA. (2014) 111:787–92. doi: 10.1073/pnas.1314688110
52. Zhang X, Weyrich LS, Lavine JS, Karanikas AT, Harvill ET. Lack of cross-protection against Bordetella holmesii after pertussis vaccination. Emerg Infect Dis. (2012) 18:1771–9. doi: 10.3201/eid1811.111544
53. Lavine J, Broutin H, Harvill ET, Bjørnstad ON. Imperfect vaccine-induced immunity and whooping cough transmission to infants. Vaccine. (2010) 29:11–6. doi: 10.1016/j.vaccine.2010.10.029
54. Long GH, Karanikas AT, Harvill ET, Read AF, and Hudson PJ. Acellular pertussis vaccination facilitates Bordetella parapertussis infection in a rodent model of bordetellosis. Proc Biol Sci. (2010) 277:2017–25. doi: 10.1098/rspb.2010.0010
55. Diavatopoulos DA, and Edwards KM. What is wrong with pertussis vaccine immunity? why immunological memory to pertussis is failing. Cold Spring Harb Perspect Biol. (2017) 9:a029553. doi: 10.1101/cshperspect.a029553
56. Burdin N, Handy LK, and Plotkin SA. What is wrong with pertussis vaccine immunity? the problem of waning effectiveness of pertussis vaccines. Cold Spring Harb Perspect Biol. (2017) 9:a029454. doi: 10.1101/cshperspect.a029454
57. Bancroft T, Dillon MB, da Silva Antunes R, Paul S, Peters B, Crotty S, et al. Th1 versus Th2 T cell polarization by whole-cell and acellular childhood pertussis vaccines persists upon re-immunization in adolescence and adulthood. Cell Immunol. (2016) 304–5:35–43. doi: 10.1016/j.cellimm.2016.05.002
58. Allen AC, Wilk MM, Misiak A, Borkner L, Murphy D, and Mills KHG. Sustained protective immunity against Bordetella pertussis nasal colonization by intranasal immunization with a vaccine-adjuvant combination that induces IL-17-secreting TRM cells. Mucosal Immunol. (2018) 11:1763–76. doi: 10.1038/s41385-018-0080-x
59. Kapil P, Merkel TJ. Pertussis vaccines and protective immunity. Curr Opin Immunol. (2019) 59:72–8. doi: 10.1016/j.coi.2019.03.006
60. Lambert EE, Buisman AM, and van Els CACM. Superior B. pertussis specific CD4+ T-cell immunity imprinted by natural infection. Adv Exp Med Biol. (2019). doi: 10.1007/5584_2019_405
61. Wilk MM, Borkner L, Misiak A, Curham L, Allen AC, and Mills KHG. Immunization with whole cell but not acellular pertussis vaccines primes CD4 TRM cells that sustain protective immunity against nasal colonization with Bordetella pertussis. Emerg Microbes Infect. (2019) 8:169–85. doi: 10.1080/22221751.2018.1564630
62. Raeven RH, Brummelman J, Pennings JL, Nijst OE, Kuipers B, Blok LE, et al. Molecular signatures of the evolving immune response in mice following a Bordetella pertussis infection. PLoS ONE. (2014) 9:e104548. doi: 10.1371/journal.pone.0104548
63. Wilk MM, Misiak A, McManus RM, Allen AC, Lynch MA, and Mills KHG. Lung CD4 Tissue-resident memory t cells mediate adaptive immunity induced by previous infection of mice with Bordetella pertussis. J Immunol. (2017) 199:233–43. doi: 10.4049/jimmunol.1602051
64. Bouchez V, Hegerle N, Strati F, Njamkepo E, Guiso N. New data on vaccine antigen deficient Bordetella pertussis isolates. Vaccines. (2015) 3:751–70. doi: 10.3390/vaccines3030751
65. Azarian T, Ali A, Johnson JA, Mohr D, Prosperi M, Veras NM, et al. Phylodynamic analysis of clinical and environmental Vibrio cholerae isolates from Haiti reveals diversification driven by positive selection. MBio. (2014) 5:e01824–14. doi: 10.1128/mBio.01824-14
66. Hegerle N, and Guiso N. Antibody-mediated inhibition of Bordetella pertussis adenylate cyclase-haemolysin-induced macrophage cytotoxicity is influenced by variations in the bacterial population. Microbiology. (2014) 160(Pt 5):962–9. doi: 10.1099/mic.0.074690-0
67. Octavia S, Maharjan RP, Sintchenko V, Stevenson G, Reeves PR, Gilbert GL, et al. Insight into evolution of Bordetella pertussis from comparative genomic analysis: evidence of vaccine-driven selection. Mol Biol Evol. (2011) 28:707–15. doi: 10.1093/molbev/msq245
68. Kirimanjeswara GS, Mann PB, Harvill ET. Role of antibodies in immunity to Bordetella infections. Infect Immun. (2003) 71:1719–24. doi: 10.1128/IAI.71.4.1719-1724.2003
69. Wolfe DN, Goebel EM, Bjornstad ON, Restif O, Harvill ET. The O antigen enables Bordetella parapertussis to avoid Bordetella pertussis-induced immunity. Infect Immun. (2007) 75:4972–9. doi: 10.1128/IAI.00763-07
70. Melvin JA, Scheller EV, Miller JF, Cotter PA. Bordetella pertussis pathogenesis: current and future challenges. Nat Rev Microbiol. (2014) 12:274–88. doi: 10.1038/nrmicro3235
71. Fedele G, Bianco M, Ausiello CM. The virulence factors of Bordetella pertussis: talented modulators of host immune response. Arch Immunol Ther Exp. (2013) 61:445–57. doi: 10.1007/s00005-013-0242-1
72. Gorgojo J, Scharrig E, Gómez RM, Harvill ET, Rodríguez ME. Bordetella parapertussis circumvents neutrophil extracellular bactericidal mechanisms. PLoS ONE. (2017) 12:e0169936. doi: 10.1371/journal.pone.0169936
73. Fedele G, Schiavoni I, Adkins I, Klimova N, and Sebo P. Invasion of dendritic cells, macrophages and neutrophils by the Bordetella adenylate cyclase toxin: a subversive move to fool host immunity. Toxins. (2017) 9:E293. doi: 10.3390/toxins9100293
74. Nicholson TL, Brockmeier SL, Loving CL, Register KB, Kehrli ME, Shore SM. The Bordetella bronchiseptica type III secretion system is required for persistence and disease severity but not transmission in swine. Infect Immun. (2014) 82:1092–103. doi: 10.1128/IAI.01115-13
75. Fennelly NK, Sisti F, Higgins SC, Ross PJ, van der Heide H, Mooi FR, et al. Bordetella pertussis expresses a functional type III secretion system that subverts protective innate and adaptive immune responses. Infect Immun. (2008) 76:1257–66. doi: 10.1128/IAI.00836-07
76. Skinner JA, Pilione MR, Shen H, Harvill ET, Yuk MH. Bordetella type III secretion modulates dendritic cell migration resulting in immunosuppression and bacterial persistence. J Immunol. (2005) 175:4647–52. doi: 10.4049/jimmunol.175.7.4647
77. Coulthurst S. The Type VI secretion system: a versatile bacterial weapon. Microbiology. (2019) 165:503–15. doi: 10.1099/mic.0.000789
78. Starost LJ, Karassek S, Sano Y, Kanda T, Kim KS, Dobrindt U, et al. Pertussis Toxin Exploits host cell signaling pathways induced by meningitis-causing E. coli K1-RS218 and enhances adherence of monocytic THP-1 cells to human cerebral endothelial cells. Toxins. (2016) 8:E291. doi: 10.3390/toxins8100291
79. Suh HW, Sim YB, Park SH, Sharma N, Im HJ, Hong JS. Effect of pertussis toxin pretreated centrally on blood glucose level induced by stress. Korean J Physiol Pharmacol. (2016) 20:467–76. doi: 10.4196/kjpp.2016.20.5.467
80. Kirimanjeswara GS, Agosto LM, Kennett MJ, Bjornstad ON, Harvill ET. Pertussis toxin inhibits neutrophil recruitment to delay antibody-mediated clearance of Bordetella pertussis. J Clin Invest. (2005) 115:3594–601. doi: 10.1172/JCI24609
81. Bakhshaei P, Kazemi MH, Golara M, Abdolmaleki S, Khosravi-Eghbal R, Khoshnoodi J, et al. Investigation of the cellular immune response to recombinant fragments of filamentous hemagglutinin and pertactin of Bordetella pertussis in BALB/c mice. J Interferon Cytokine Res. (2018) 38:161–70. doi: 10.1089/jir.2017.0060
82. Villarino Romero R, Hasan S, Faé K, Holubova J, Geurtsen J, Schwarzer M, et al. Bordetella pertussis filamentous hemagglutinin itself does not trigger anti-inflammatory interleukin-10 production by human dendritic cells. Int J Med Microbiol. (2016) 306:38–47. doi: 10.1016/j.ijmm.2015.11.003
83. Rizzi C, Crippa MP, Jeeninga RE, Berkhout B, Blasi F, Poli G, et al. Pertussis toxin B-oligomer suppresses IL-6 induced HIV-1 and chemokine expression in chronically infected U1 cells via inhibition of activator protein 1. J Immunol. (2006) 176:999–1006. doi: 10.4049/jimmunol.176.2.999
84. Alfano M, Grivel JC, Ghezzi S, Corti D, Trimarchi M, Poli G, et al. Pertussis toxin B-oligomer dissociates T cell activation and HIV replication in CD4 T cells released from infected lymphoid tissue. AIDS. (2005) 19:1007–14. doi: 10.1097/01.aids.0000174446.40379.3b
85. Alfano M, Rizzi C, Corti D, Adduce L, Poli G. Bacterial toxins: potential weapons against HIV infection. Curr Pharm Des. (2005) 11:2909–26. doi: 10.2174/1381612054546725
86. Lapenta C, Spada M, Santini SM, Racca S, Dorigatti F, Poli G, et al. Pertussis toxin B-oligomer inhibits HIV infection and replication in hu-PBL-SCID mice. Int Immunol. (2005) 17:469–75. doi: 10.1093/intimm/dxh226
87. Alfano M, Vallanti G, Biswas P, Bovolenta C, Vicenzi E, Mantelli B, et al. The binding subunit of pertussis toxin inhibits HIV replication in human macrophages and virus expression in chronically infected promonocytic U1 cells. J Immunol. (2001) 166:1863–70. doi: 10.4049/jimmunol.166.3.1863
88. Alfano M, Pushkarsky T, Poli G, Bukrinsky M. The B-oligomer of pertussis toxin inhibits human immunodeficiency virus type 1 replication at multiple stages. J Virol. (2000) 74:8767–70. doi: 10.1128/JVI.74.18.8767-8770.2000
89. Alfano M, Schmidtmayerova H, Amella CA, Pushkarsky T, Bukrinsky M. The B-oligomer of pertussis toxin deactivates CC chemokine receptor 5 and blocks entry of M-tropic HIV-1 strains. J Exp Med. (1999) 190:597–605. doi: 10.1084/jem.190.5.597
90. Robbins JB, Schneerson R, Kubler-Kielb J, Keith JM, Trollfors B, Vinogradov E, et al. Toward a new vaccine for pertussis. Proc Natl Acad Sci USA. (2014) 111:3213–6. doi: 10.1073/pnas.1324149111
91. Coffman RL, Sher A, Seder RA. Vaccine adjuvants: putting innate immunity to work. Immunity. (2010) 33:492–503. doi: 10.1016/j.immuni.2010.10.002
92. Kumar S, Sunagar R, Gosselin E. Bacterial protein toll-like-receptor agonists: a novel perspective on vaccine adjuvants. Front Immunol. (2019) 10:1144. doi: 10.3389/fimmu.2019.01144
93. Surendran N, Simmons A, Pichichero ME. TLR agonist combinations that stimulate Th type I polarizing responses from human neonates. Innate Immun. (2018) 24:240–51. doi: 10.1177/1753425918771178
94. Ignacio BJ, Albin TJ, Esser-Kahn AP, Verdoes M. Toll-like receptor agonist conjugation: a chemical perspective. Bioconjug Chem. (2018) 29:587–603. doi: 10.1021/acs.bioconjchem.7b00808
95. Sinani G, Sessevmez M, Gök MK, Özgümüş S, Oya Alpar H, and Cevher E. Modified chitosan-based nanoadjuvants enhance immunogenicity of protein antigens after mucosal vaccination. Int J Pharm. (2019) 569:118592. doi: 10.1016/j.ijpharm.2019.118592
96. Mehrabi M, Montazeri H, Mohamadpour Dounighi N, Rashti A, Vakili-Ghartavol R. Chitosan-based nanoparticles in mucosal vaccine delivery. Arch Razi Inst. (2018) 73:165–76. doi: 10.22092/ari.2017.109235.1101
97. Moran HBT, Turley JL, Andersson M, Lavelle EC. Immunomodulatory properties of chitosan polymers. Biomaterials. (2018) 184:1–9. doi: 10.1016/j.biomaterials.2018.08.054
98. Misiak A, Leuzzi R, Allen AC, Galletti B, Baudner BC, D'Oro U, et al. Addition of a TLR7 agonist to an acellular pertussis vaccine enhances Th1 and Th17 responses and protective immunity in a mouse model. Vaccine. (2017) 35:5256–63. doi: 10.1016/j.vaccine.2017.08.009
99. Brummelman J, Wilk MM, Han WG, van Els CA, Mills KH. Roads to the development of improved pertussis vaccines paved by immunology. Pathog Dis. (2015) 73:ftv067. doi: 10.1093/femspd/ftv067
100. Dunne A, Mielke LA, Allen AC, Sutton CE, Higgs R, Cunningham CC, et al. A novel TLR2 agonist from Bordetella pertussis is a potent adjuvant that promotes protective immunity with an acellular pertussis vaccine. Mucosal Immunol. (2015) 8:607–17. doi: 10.1038/mi.2014.93
101. Dunne A, Marshall NA, Mills KH. TLR based therapeutics. Curr Opin Pharmacol. (2011) 11:404–11. doi: 10.1016/j.coph.2011.03.004
102. Higgins SC, Mills KH. TLR, NLR Agonists, and other immune modulators as infectious disease vaccine adjuvants. Curr Infect Dis Rep. (2010) 12:4–12. doi: 10.1007/s11908-009-0080-9
103. Pizza M, Giuliani MM, Fontana MR, Monaci E, Douce G, Dougan G, et al. Mucosal vaccines: non toxic derivatives of LT and CT as mucosal adjuvants. Vaccine. (2001) 19:2534–41. doi: 10.1016/S0264-410X(00)00553-3
104. Walsh KP, Mills KH. Dendritic cells and other innate determinants of T helper cell polarisation. Trends Immunol. (2013) 34:521–30. doi: 10.1016/j.it.2013.07.006
105. Di Pasquale A, Preiss SF, Tavares Da Silva, Garçon N. Vaccine adjuvants: from 1920 to 2015 and Beyond. Vaccines. (2015) 3:320–43. doi: 10.3390/vaccines3020320
106. Guo X, Wu N, Shang Y, Liu X, Wu T, Zhou Y, et al. The novel toll-like receptor 2 agonist SUP3 enhances antigen presentation and T cell activation by dendritic cells. Front Immunol. (2017) 8:158. doi: 10.3389/fimmu.2017.00158
107. Jiang L, Liu G, Ni W, Zhang N, Jie J, Xie F, et al. The combination of MBP and BCG-induced dendritic cell maturation through TLR2/TLR4 promotes Th1 activation in vitro and vivo. Mediators Inflamm. (2017) 2017:1953680. doi: 10.1155/2017/1953680
108. Asgarian-Omran H, Amirzargar AA, Zeerleder S, Mahdavi M, van Mierlo G, Solati S, et al. Interaction of Bordetella pertussis filamentous hemagglutinin with human TLR2: identification of the TLR2-binding domain. APMIS. (2015) 123:156–62. doi: 10.1111/apm.12332
109. Rostamian M, Bahrami F, Niknam HM. Vaccination with whole-cell killed or recombinant leishmanial protein and toll-like receptor agonists against Leishmania tropica in BALB/c mice. PLoS ONE. (2018) 13:e0204491. doi: 10.1371/journal.pone.0204491
110. Rostamian M, Niknam HM. Evaluation of the adjuvant effect of agonists of toll-like receptor 4 and 7/8 in a vaccine against leishmaniasis in BALB/c mice. Mol Immunol. (2017) 91:202–8. doi: 10.1016/j.molimm.2017.09.010
111. Lebedeva E, Bagaev A, Pichugin A, Chulkina M, Lysenko A, Tutykhina I, et al. The differences in immunoadjuvant mechanisms of TLR3 and TLR4 agonists on the level of antigen-presenting cells during immunization with recombinant adenovirus vector. BMC Immunol. (2018) 19:26. doi: 10.1186/s12865-018-0264-x
112. Goff PH, Hayashi T, He W, Yao S, Cottam HB, Tan GS, et al. Synthetic Toll-like receptor 4 (TLR4) and TLR7 ligands work additively via myd88 to induce protective antiviral immunity in mice. J Virol. (2017) 91:e01050–17. doi: 10.1128/JVI.01050-17
113. Su Y, Li D, Xing Y, Wang H, Wang J, Yuan J, et al. Subcutaneous immunization with fusion protein DnaJ-ΔA146Ply without additional adjuvants induces both humoral and cellular immunity against Pneumococcal infection partially depending on TLR4. Front Immunol. (2017) 8:686. doi: 10.3389/fimmu.2017.00686
114. Shibata N, Kunisawa J, Hosomi K, Fujimoto Y, Mizote K, Kitayama N, et al. Lymphoid tissue-resident Alcaligenes LPS induces IgA production without excessive inflammatory responses via weak TLR4 agonist activity. Mucosal Immunol. (2018) 11:693–702. doi: 10.1038/mi.2017.103
115. Reed SG, Hsu FC, Carter D, Orr MT. The science of vaccine adjuvants: advances in TLR4 ligand adjuvants. Curr Opin Immunol. (2016) 41:85–90. doi: 10.1016/j.coi.2016.06.007
116. Zaffaroni L, Peri F. Recent advances on Toll-like receptor 4 modulation: new therapeutic perspectives. Future Med Chem. (2018) 10:461–76. doi: 10.4155/fmc-2017-0172
117. Rolin O, Smallridge W, Henry M, Goodfield L, Place D, Harvill ET. Toll-like receptor 4 limits transmission of Bordetella bronchiseptica. PLoS ONE. (2014) 9:e85229. doi: 10.1371/journal.pone.0085229
118. Dadaglio G, Fayolle C, Zhang X, Ryffel B, Oberkampf M, Felix T, et al. Antigen targeting to CD11b+ dendritic cells in association with TLR4/TRIF signaling promotes strong CD8+ T cell responses. J Immunol. (2014) 193:1787–98. doi: 10.4049/jimmunol.1302974
119. Fedele G, Spensieri F, Palazzo R, Nasso M, Cheung GY, Coote JG, et al. Bordetella pertussis commits human dendritic cells to promote a Th1/Th17 response through the activity of adenylate cyclase toxin and MAPK-pathways. PLoS ONE. (2010) 5:e8734. doi: 10.1371/journal.pone.0008734
120. Boehm DT, Wolf MA, Hall JM, Wong TY, Sen-Kilic E, Basinger HD, et al. Intranasal acellular pertussis vaccine provides mucosal immunity and protects mice from. NPJ Vaccines. (2019) 4:40. doi: 10.1038/s41541-019-0136-2
121. Brummelman J, Raeven RH, Helm K, Pennings JL, Metz B, van Eden W, et al. Transcriptome signature for dampened Th2 dominance in acellular pertussis vaccine-induced CD4(+) T cell responses through TLR4 ligation. Sci Rep. (2016) 6:25064. doi: 10.1038/srep25064
122. Brummelman J, Helm K, Hamstra HJ, van der Ley P, Boog CJ, Han WG, et al. Modulation of the CD4(+) T cell response after acellular pertussis vaccination in the presence of TLR4 ligation. Vaccine. (2015) 33:1483–91. doi: 10.1016/j.vaccine.2015.01.063
123. Wolfe DN, Buboltz AM, Harvill ET. Inefficient Toll-like receptor-4 stimulation enables Bordetella parapertussis to avoid host immunity. PLoS ONE. (2009) 4:e4280. doi: 10.1371/journal.pone.0004280
124. Fedele G, Nasso M, Spensieri F, Palazzo R, Frasca L, Watanabe M, et al. Lipopolysaccharides from Bordetella pertussis and Bordetella parapertussis differently modulate human dendritic cell functions resulting in divergent prevalence of Th17-polarized responses. J Immunol. (2008) 181:208–16. doi: 10.4049/jimmunol.181.1.208
125. MacArthur I, Mann PB, Harvill ET, Preston A. IEIIS Meeting minireview: Bordetella evolution: lipid A and Toll-like receptor 4. J Endotoxin Res. (2007) 13:243–7. doi: 10.1177/0968051907082609
126. Kirimanjeswara GS, Mann PB, Pilione M, Kennett MJ, Harvill ET. The complex mechanism of antibody-mediated clearance of Bordetella from the lungs requires TLR4. J Immunol. (2005) 175:7504–11. doi: 10.4049/jimmunol.175.11.7504
127. Mann PB, Wolfe D, Latz E, Golenbock D, Preston A, Harvill ET. Comparative toll-like receptor 4-mediated innate host defense to Bordetella infection. Infect Immun. (2005) 73:8144–52. doi: 10.1128/IAI.73.12.8144-8152.2005
128. Mann PB, Elder KD, Kennett MJ, Harvill ET. Toll-like receptor 4-dependent early elicited tumor necrosis factor alpha expression is critical for innate host defense against Bordetella bronchiseptica. Infect Immun. (2004) 72:6650–8. doi: 10.1128/IAI.72.11.6650-6658.2004
129. McKay PF, King DF, Mann JF, Barinaga G, Carter D, Shattock RJ. TLR4 and TLR7/8 adjuvant combinations generate different vaccine antigen-specific immune outcomes in minipigs when administered via the ID or IN routes. PLoS ONE. (2016) 11:e0148984. doi: 10.1371/journal.pone.0148984
130. Mann PB, Kennett MJ, Harvill ET. Toll-like receptor 4 is critical to innate host defense in a murine model of bordetellosis. J Infect Dis. (2004) 189:833–6. doi: 10.1086/381898
131. Mizel SB, West AP, Hantgan RR. Identification of a sequence in human toll-like receptor 5 required for the binding of Gram-negative flagellin. J Biol Chem. (2003) 278:23624–9. doi: 10.1074/jbc.M303481200
132. Akerley BJ, Cotter PA, Miller JF. Ectopic expression of the flagellar regulon alters development of the Bordetella-host interaction. Cell. (1995) 80:611–20. doi: 10.1016/0092-8674(95)90515-4
133. Tremblay MM, Bilal MY, Houtman JC. Prior TLR5 induction in human T cells results in a transient potentiation of subsequent TCR-induced cytokine production. Mol Immunol. (2014) 57:161–70. doi: 10.1016/j.molimm.2013.09.002
134. Bargieri DY, Rosa DS, Braga CJ, Carvalho BO, Costa FT, Espíndola NM, et al. New malaria vaccine candidates based on the Plasmodium vivax merozoite surface protein-1 and the TLR-5 agonist Salmonella Typhimurium FliC flagellin. Vaccine. (2008) 26:6132–42. doi: 10.1016/j.vaccine.2008.08.070
135. Honko AN, Sriranganathan N, Lees CJ, Mizel SB. Flagellin is an effective adjuvant for immunization against lethal respiratory challenge with Yersinia pestis. Infect Immun. (2006) 74:1113–20. doi: 10.1128/IAI.74.2.1113-1120.2006
136. Letran SE, Lee SJ, Atif SM, Uematsu S, Akira S, McSorley SJ. TLR5 functions as an endocytic receptor to enhance flagellin-specific adaptive immunity. Eur J Immunol. (2011) 41:29–38. doi: 10.1002/eji.201040717
137. Taylor DN, Treanor JJ, Strout C, Johnson C, Fitzgerald T, Kavita U, et al. Induction of a potent immune response in the elderly using the TLR-5 agonist, flagellin, with a recombinant hemagglutinin influenza-flagellin fusion vaccine (VAX125, STF2.HA1 SI). Vaccine. (2011) 29:4897–902. doi: 10.1016/j.vaccine.2011.05.001
138. Kinnebrew MA, Ubeda C, Zenewicz LA, Smith N, Flavell RA, Pamer EG. Bacterial flagellin stimulates Toll-like receptor 5-dependent defense against vancomycin-resistant Enterococcus infection. J Infect Dis. (2010) 201:534–43. doi: 10.1086/650203
139. Kim JR, Holbrook BC, Hayward SL, Blevins LK, Jorgensen MJ, Kock ND, et al. Inclusion of flagellin during vaccination against influenza enhances recall responses in nonhuman primate neonates. J Virol. (2015) 89:7291–303. doi: 10.1128/JVI.00549-15
140. Song H, Xiong D, Wang J, Zhai X, Liang G. A porcine reproductive and respiratory syndrome virus vaccine candidate based on PRRSV glycoprotein 5 and the Toll-like receptor 5 agonist Salmonella typhimurium flagellin. J Mol Microbiol Biotechnol. (2015) 25:56–9. doi: 10.1159/000375496
141. Cunningham AL, Dang KM, Yu JJ, Guentzel MN, Heidner HW, Klose KE, et al. Enhancement of vaccine efficacy by expression of a TLR5 ligand in the defined live attenuated Francisella tularensis subsp. novicida strain U112ΔiglB::fljB. Vaccine. (2014) 32:5234–40. doi: 10.1016/j.vaccine.2014.07.038
142. Dowling DJ. Recent advances in the discovery and delivery of TLR7/8 agonists as vaccine adjuvants. Immunohorizons. (2018) 2:185–97. doi: 10.4049/immunohorizons.1700063
143. Dowling DJ, van Haren SD, Scheid A, Bergelson I, Kim D, Mancuso CJ, et al. TLR7/8 adjuvant overcomes newborn hyporesponsiveness to pneumococcal conjugate vaccine at birth. JCI Insight. (2017) 2:e91020. doi: 10.1172/jci.insight.91020
144. van Haren SD, Dowling DJ, Foppen W, Christensen D, Andersen P, Reed SG, et al. Age-specific adjuvant synergy: dual TLR7/8 and mincle activation of human newborn dendritic cells enables Th1 polarization. J Immunol. (2016) 197:4413–24. doi: 10.4049/jimmunol.1600282
145. van Haren SD, Ganapathi L, Bergelson I, Dowling DJ, Banks M, Samuels RC, et al. in vitro cytokine induction by TLR-activating vaccine adjuvants in human blood varies by age and adjuvant. Cytokine. (2016) 83:99–109. doi: 10.1016/j.cyto.2016.04.001
146. Ganapathi L, Van Haren S, Dowling DJ, Bergelson I, Shukla NM, Malladi SS, et al. The imidazoquinoline toll-like receptor-7/8 agonist hybrid-2 potently induces cytokine production by human newborn and adult leukocytes. PLoS ONE. (2015) 10:e0134640. doi: 10.1371/journal.pone.0134640
147. Wilkinson A, Lattmann E, Roces CB, Pedersen GK, Christensen D, Perrie Y. Lipid conjugation of TLR7 agonist Resiquimod ensures co-delivery with the liposomal Cationic Adjuvant Formulation 01 (CAF01) but does not enhance immunopotentiation compared to non-conjugated Resiquimod+CAF01. J Control Release. (2018) 291:1–10. doi: 10.1016/j.jconrel.2018.10.002
148. Collier MA, Junkins RD, Gallovic MD, Johnson BM, Johnson MM, Macintyre AN, et al. Acetalated dextran microparticles for codelivery of STING and TLR7/8 agonists. Mol Pharm. (2018) 15:4933–46. doi: 10.1021/acs.molpharmaceut.8b00579
149. McGowan DC, Herschke F, Khamlichi MD, Rosauro ML, Benedicto SMP, Pauwels F, et al. Design and synthesis of tetrahydropyridopyrimidine based toll-like receptor (TLR) 7/8 dual agonists. Bioorg Med Chem Lett. (2018) 28:3216–21. doi: 10.1016/j.bmcl.2018.08.015
150. Gadd AJR, Castelletto V, Kabova E, Shankland K, Perrie Y, Hamley I, et al. High potency of lipid conjugated TLR7 agonist requires nanoparticulate or liposomal formulation. Eur J Pharm Sci. (2018) 123:268–76. doi: 10.1016/j.ejps.2018.07.048
151. Vo HTM, Baudner BC, Sammicheli S, Iannacone M, D'Oro U, Piccioli D. Alum/toll-like receptor 7 adjuvant enhances the expansion of memory B cell compartment within the draining lymph node. Front Immunol. (2018) 9:641. doi: 10.3389/fimmu.2018.00641
152. Carignan D, Herblot S, Laliberté-Gagné M, Bolduc M, Duval M, Savard P, et al. Activation of innate immunity in primary human cells using a plant virus derived nanoparticle TLR7/8 agonist. Nanomedicine. (2018) 14:2317–27. doi: 10.1016/j.nano.2017.10.015
153. Van Hoeven N, Fox CB, Granger B, Evers T, Joshi SW, Nana GI, et al. A formulated TLR7/8 agonist is a flexible, highly potent and effective adjuvant for pandemic influenza vaccines. Sci Rep. (2017) 7:46426. doi: 10.1038/srep46426
154. Rubtsova K, Rubtsov AV, Halemano K, Li SX, Kappler JW, Santiago ML, et al. T cell production of IFNγ in response to TLR7/IL-12 stimulates optimal B cell responses to viruses. PLoS ONE. (2016) 11:e0166322. doi: 10.1371/journal.pone.0166322
155. Hu Y, Cong X, Chen L, Qi J, Wu X, Zhou M, et al. Synergy of TLR3 and 7 ligands significantly enhances function of DCs to present inactivated PRRSV antigen through TRIF/MyD88-NF-κB signaling pathway. Sci Rep. (2016) 6:23977. doi: 10.1038/srep23977
156. Aguado-Martínez A, Basto AP, Tanaka S, Ryser LT, Nunes TP, Ortega-Mora LM, et al. Immunization with a cocktail of antigens fused with OprI reduces Neospora caninum vertical transmission and postnatal mortality in mice. Vaccine. (2019) 37:473–83. doi: 10.1016/j.vaccine.2018.11.060
157. Buonsanti C, Balocchi C, Harfouche C, Corrente F, Galli Stampino L, Mancini F, et al. Novel adjuvant Alum-TLR7 significantly potentiates immune response to glycoconjugate vaccines. Sci Rep. (2016) 6:29063. doi: 10.1038/srep29063
158. Lu F, Mosley YC, Carmichael B, Brown DD, HogenEsch H. Formulation of aluminum hydroxide adjuvant with TLR agonists poly(I:C) and CpG enhances the magnitude and avidity of the humoral immune response. Vaccine. (2019) 37:1945–53. doi: 10.1016/j.vaccine.2019.02.033
159. Li N, Zhang L, Zheng B, Li W, Liu J, Zhang H, et al. RSV recombinant candidate vaccine G1F/M2 with CpG as an adjuvant prevents vaccine-associated lung inflammation, which may be associated with the appropriate types of immune memory in spleens and lungs. Hum Vaccin Immunother. (2019) 15:2684–94. doi: 10.1080/21645515.2019.1596710
160. Jin JW, Tang SQ, Rong MZ, Zhang MQ. Synergistic effect of dual targeting vaccine adjuvant with aminated β-glucan and CpG-oligodeoxynucleotides for both humoral and cellular immune responses. Acta Biomater. (2018) 78:211–23. doi: 10.1016/j.actbio.2018.08.002
161. Guan X, Chen J, Hu Y, Lin L, Sun P, Tian H, et al. Highly enhanced cancer immunotherapy by combining nanovaccine with hyaluronidase. Biomaterials. (2018) 171:198–206. doi: 10.1016/j.biomaterials.2018.04.039
162. Alkie TN, Taha-Abdelaziz K, Barjesteh N, Bavananthasivam J, Hodgins DC, Sharif S. Characterization of innate responses induced by PLGA encapsulated- and soluble TLR ligands in vitro and in vivo in chickens. PLoS ONE. (2017) 12:e0169154. doi: 10.1371/journal.pone.0169154
163. Hawksworth D. Advancing Freund's and addaVax adjuvant regimens using CpG oligodeoxynucleotides. Monoclon Antib Immunodiagn Immunother. (2018) 37:195–9. doi: 10.1089/mab.2018.0022
164. Lai CY, Yu GY, Luo Y, Xiang R, Chuang TH. Immunostimulatory activities of CpG-oligodeoxynucleotides in teleosts: toll-like receptors 9 and 21. Front Immunol. (2019) 10:179. doi: 10.3389/fimmu.2019.00179
165. Akkaya M, Akkaya B, Miozzo P, Rawat M, Pena M, Sheehan PW, et al. B cells produce type 1 IFNs in response to the TLR9 agonist CpG-A conjugated to cationic lipids. J Immunol. (2017) 199:931–40. doi: 10.4049/jimmunol.1700348
166. Ye L, Feng Z, Doycheva D, Malaguit J, Dixon B, Xu N, et al. CpG-ODN exerts a neuroprotective effect via the TLR9/pAMPK signaling pathway by activation of autophagy in a neonatal HIE rat model. Exp Neurol. (2018) 301(Pt A):70–80. doi: 10.1016/j.expneurol.2017.12.008
167. Zhang H, Yan T, Xu S, Feng S, Huang D, Fujita M, et al. Graphene oxide-chitosan nanocomposites for intracellular delivery of immunostimulatory CpG oligodeoxynucleotides. Mater Sci Eng C Mater Biol Appl. (2017) 73:144–51. doi: 10.1016/j.msec.2016.12.072
168. Zhang P, Dong S, Guo J, Yang Y, Liu C, Li B, et al. CpG-oligodeoxynucleotides improved irradiation-induced injuries by G-CSF and IL-6 up-regulation. Cell Physiol Biochem. (2017) 44:2368–77. doi: 10.1159/000486153
169. Ramirez A, Co M, Mathew A. CpG improves influenza vaccine efficacy in young adult but not aged mice. PLoS ONE. (2016) 11:e0150425. doi: 10.1371/journal.pone.0150425
170. Asokanathan C, Corbel M, Xing D. A CpG-containing oligodeoxynucleotide adjuvant for acellular pertussis vaccine improves the protective response against Bordetella pertussis. Hum Vaccin Immunother. (2013) 9:325–31. doi: 10.4161/hv.22755
171. Knight JB, Huang YY, Halperin SA, Anderson R, Morris A, Macmillan A, et al. Immunogenicity and protective efficacy of a recombinant filamentous haemagglutinin from Bordetella pertussis. Clin Exp Immunol. (2006) 144:543–51. doi: 10.1111/j.1365-2249.2006.03097.x
172. Boyd AP, Ross PJ, Conroy H, Mahon N, Lavelle EC, Mills KH. Bordetella pertussis adenylate cyclase toxin modulates innate and adaptive immune responses: distinct roles for acylation and enzymatic activity in immunomodulation and cell death. J Immunol. (2005) 175:730–8. doi: 10.4049/jimmunol.175.2.730
173. Maletto B, Rópolo A, Morón V, Pistoresi-Palencia MC. CpG-DNA stimulates cellular and humoral immunity and promotes Th1 differentiation in aged BALB/c mice. J Leukoc Biol. (2002) 72:447–54.
174. Gracia A, Polewicz M, Halperin SA, Hancock RE, Potter AA, Babiuk LA, et al. Antibody responses in adult and neonatal BALB/c mice to immunization with novel Bordetella pertussis vaccine formulations. Vaccine. (2011) 29:1595–604. doi: 10.1016/j.vaccine.2010.12.083
175. de Apostólico JS, Lunardelli VA, Coirada FC, Boscardin SB, Rosa DS. Adjuvants: classification, modus operandi, and licensing. J Immunol Res. (2016) 2016:1459394. doi: 10.1155/2016/1459394
176. Holmgren J, Adamsson J, Anjuère F, Clemens J, Czerkinsky C, Eriksson K, et al. Mucosal adjuvants and anti-infection and anti-immunopathology vaccines based on cholera toxin, cholera toxin B subunit and CpG DNA. Immunol Lett. (2005) 97:181–8. doi: 10.1016/j.imlet.2004.11.009
177. Bromander AK, Kjerrulf M, Holmgren J, Lycke N. Cholera toxin enhances antigen presentation. Adv Exp Med Biol. (1995) 371B:1501–6.
178. Holmgren J, Czerkinsky C, Lycke N, Svennerholm AM. Strategies for the induction of immune responses at mucosal surfaces making use of cholera toxin B subunit as immunogen, carrier, and adjuvant. Am J Trop Med Hyg. (1994) 50:42–54.
179. Bromander AK, Kjerrulf M, Holmgren J, Lycke N. Cholera toxin enhances alloantigen presentation by cultured intestinal epithelial cells. Scand J Immunol. (1993) 37:452–8. doi: 10.1111/j.1365-3083.1993.tb03318.x
180. Holmgren J, Lycke N, Czerkinsky C. Cholera toxin and cholera B subunit as oral-mucosal adjuvant and antigen vector systems. Vaccine. (1993) 11:1179–84. doi: 10.1016/0264-410X(93)90039-Z
181. Holmgren J, Nordqvist S, Blomquist M, Jeverstam F, Lebens M, Raghavan S. Preclinical immunogenicity and protective efficacy of an oral Helicobacter pylori inactivated whole cell vaccine and multiple mutant cholera toxin: a novel and non-toxic mucosal adjuvant. Vaccine. (2018) 36:6223–30. doi: 10.1016/j.vaccine.2018.07.073
182. Lavelle EC, Jarnicki A, McNeela E, Armstrong ME, Higgins SC, Leavy O, et al. Effects of cholera toxin on innate and adaptive immunity and its application as an immunomodulatory agent. J Leukoc Biol. (2004) 75:756–63. doi: 10.1189/jlb.1103534
183. Kim MS, Yi EJ, Kim YI, Kim SH, Jung YS, Kim SR, et al. ERdj5 in innate immune cells is a crucial factor for the mucosal adjuvanticity of cholera toxin. Front Immunol. (2019) 10:1249. doi: 10.3389/fimmu.2019.01249
184. Sun JB, Holmgren J, Larena M, Terrinoni M, Fang Y, Bresnick AR, et al. Deficiency in calcium-binding protein S100A4 impairs the adjuvant action of cholera toxin. Front Immunol. (2017) 8:1119. doi: 10.3389/fimmu.2017.01119
185. Barati B, Ebrahimi F, Nazarian S. Production of chicken egg yolk antibody (IgY) against recombinant cholera toxin B subunit and evaluation of its prophylaxis potency in mice. Iran J Immunol. (2018) 15:47–58.
186. Isaka M, Komiya T, Takahashi M, Yasuda Y, Taniguchi T, Zhao Y, et al. Recombinant cholera toxin B subunit (rCTB) as a mucosal adjuvant enhances induction of diphtheria and tetanus antitoxin antibodies in mice by intranasal administration with diphtheria-pertussis-tetanus (DPT) combination vaccine. Vaccine. (2004) 22:3061–8. doi: 10.1016/j.vaccine.2004.02.019
187. Lee SF, Halperin SA, Salloum DF, MacMillan A, Morris A. Mucosal immunization with a genetically engineered pertussis toxin S1 fragment-cholera toxin subunit B chimeric protein. Infect Immun. (2003) 71:2272–5. doi: 10.1128/IAI.71.4.2272-2275.2003
188. Isaka M, Yasuda Y, Taniguchi T, Kozuka S, Matano K, Maeyama J, et al. Mucosal and systemic antibody responses against an acellular pertussis vaccine in mice after intranasal co-administration with recombinant cholera toxin B subunit as an adjuvant. Vaccine. (2003) 21:1165–73. doi: 10.1016/S0264-410X(02)00516-9
189. Olivera N, Castuma CE, Hozbor D, Gaillard ME, Rumbo M, Gómez RM. Immunization with the recombinant Cholera toxin B fused to Fimbria 2 protein protects against Bordetella pertussis infection. Biomed Res Int. (2014) 2014:421486. doi: 10.1155/2014/421486
190. Clements JD, Norton EB. The mucosal vaccine adjuvant LT(R192G/L211A) or dmLT. mSphere. (2018) 3:e00215–18. doi: 10.1128/mSphere.00215-18
191. Albert MJ, Haridas S, Ebenezer M, Raghupathy R, Khan I. Immunization with a double-mutant (R192G/L211A) of the heat-labile enterotoxin of escherichia coli offers partial protection against campylobacter jejuni in an adult mouse intestinal colonization model. PLoS ONE. (2015) 10:e0142090. doi: 10.1371/journal.pone.0142090
192. Chang YC, Chang CY, Tsai PS, Chiou HY, Jeng CR, Pang VF, et al. Efficacy of heat-labile enterotoxin B subunit-adjuvanted parenteral porcine epidemic diarrhea virus trimeric spike subunit vaccine in piglets. Appl Microbiol Biotechnol. (2018) 102:7499–507. doi: 10.1007/s00253-018-9110-6
193. Brereton CF, Sutton CE, Ross PJ, Iwakura Y, Pizza M, Rappuoli R, et al. Escherichia coli heat-labile enterotoxin promotes protective Th17 responses against infection by driving innate IL-1 and IL-23 production. J Immunol. (2011) 186:5896–906. doi: 10.4049/jimmunol.1003789
194. Ryan EJ, McNeela E, Pizza M, Rappuoli R, O'Neill L, Mills KH. Modulation of innate and acquired immune responses by Escherichia coli heat-labile toxin: distinct pro- and anti-inflammatory effects of the nontoxic AB complex and the enzyme activity. J Immunol. (2000) 165:5750–9. doi: 10.4049/jimmunol.165.10.5750
195. Coleman MM, Basdeo SA, Coleman AM, Cheallaigh CN, Peral de Castro C, McLaughlin AM, et al. All-trans retinoic acid augments autophagy during intracellular bacterial infection. Am J Respir Cell Mol Biol. (2018) 59:548–556. doi: 10.1165/rcmb.2017-0382OC
196. Oliveira LM, Teixeira FME, Sato MN. Impact of retinoic acid on immune cells and inflammatory diseases. Mediators Inflamm. (2018) 2018:3067126. doi: 10.1155/2018/3067126
197. Seo GY, Lee JM, Jang YS, Kang SG, Yoon SI, Ko HJ, et al. Mechanism underlying the suppressor activity of retinoic acid on IL4-induced IgE synthesis and its physiological implication. Cell Immunol. (2017) 322:49–55. doi: 10.1016/j.cellimm.2017.10.001
198. Bezerra IPS, Costa-Souza BLS, Carneiro G, Ferreira LAM, de Matos Guedes HL, Rossi-Bergmann B. Nanoencapsulated retinoic acid as a safe tolerogenic adjuvant for intranasal vaccination against cutaneous leishmaniasis. Vaccine. (2019) 37:3660–7. doi: 10.1016/j.vaccine.2019.05.043
199. Mwanza-Lisulo M, Kelly P. Potential for use of retinoic acid as an oral vaccine adjuvant. Philos Trans R Soc Lond B Biol Sci. (2015) 370:20140145. doi: 10.1098/rstb.2014.0145
200. Lisulo MM, Kapulu MC, Banda R, Sinkala E, Kayamba V, Sianongo S, et al. Adjuvant potential of low dose all-trans retinoic acid during oral typhoid vaccination in Zambian men. Clin Exp Immunol. (2014) 175:468–75. doi: 10.1111/cei.12238
201. Tan X, Sande JL, Pufnock JS, Blattman JN, Greenberg PD. Retinoic acid as a vaccine adjuvant enhances CD8+ T cell response and mucosal protection from viral challenge. J Virol. (2011) 85:8316–27. doi: 10.1128/JVI.00781-11
202. Qiang Y, Xu J, Yan C, Jin H, Xiao T, Yan N, et al. Butyrate and retinoic acid imprint mucosal-like dendritic cell development synergistically from bone marrow cells. Clin Exp Immunol. (2017) 189:290–7. doi: 10.1111/cei.12990
203. Raverdeau M, Mills KH. Modulation of T cell and innate immune responses by retinoic acid. J Immunol. (2014) 192:2953–8. doi: 10.4049/jimmunol.1303245
204. Torres-Sangiao E, Holban AM, Gestal MC. Advanced nanobiomaterials: vaccines, diagnosis and treatment of infectious diseases. Molecules. (2016) 21:E867. doi: 10.3390/molecules21070867
205. Alagpulinsa DA, Cao JJL, Driscoll RK, Sîrbulescu RF, Penson MFE, Sremac M, et al. Alginate-microencapsulation of human stem cell-derived β cells with CXCL12 prolongs their survival and function in immunocompetent mice without systemic immunosuppression. Am J Transplant. (2019) 19:1930–40. doi: 10.1111/ajt.15308
206. Ibe MI, Odimegwu DC, Onuigbo EB. Alginate-coated chitosan microparticles encapsulating an oral plasmid-cured live Salmonella enterica serovar Gallinarum vaccine cause a higher expression of interferon-gamma in chickens compared to the parenteral live vaccine. Avian Pathol. (2019) 48:423–28. doi: 10.1080/03079457.2019.1616673
207. Minakshi P, Ghosh M, Brar B, Kumar R, Lambe UP, Ranjan K, et al. Nano-antimicrobials: a new paradigm for combating mycobacterial resistance. Curr Pharm Des. (2019) 25:1554–79. doi: 10.2174/1381612825666190620094041
208. Vaghasiya K, Eram A, Sharma A, Ray E, Adlakha S, Verma RK. Alginate microspheres elicit innate M1-inflammatory response in macrophages leading to bacillary killing. AAPS PharmSciTech. (2019) 20:241. doi: 10.1208/s12249-019-1458-0
209. Coelho-Rocha ND, de Castro CP, de Jesus LCL, Leclercq SY, de Cicco Sandes SH, Nunes AC, et al. Microencapsulation of lactic acid bacteria improves the gastrointestinal delivery and in situ expression of recombinant fluorescent protein. Front Microbiol. (2018) 9:2398. doi: 10.3389/fmicb.2018.02398
210. Kordbacheh E, Nazarian S, Hajizadeh A, Sadeghi D. Entrapment of LTB protein in alginate nanoparticles protects against Enterotoxigenic Escherichia coli. APMIS. (2018) 126:320–8. doi: 10.1111/apm.12815
211. Lee J, Kim YM, Kim JH, Cho CW, Jeon JW, Park JK, et al. Nasal delivery of chitosan/alginate nanoparticle encapsulated bee (Apis mellifera) venom promotes antibody production and viral clearance during porcine reproductive and respiratory syndrome virus infection by modulating T cell related responses. Vet Immunol Immunopathol. (2018) 200:40–51. doi: 10.1016/j.vetimm.2018.04.006
212. Yuan J, Guo L, Wang S, Liu D, Qin X, Zheng L, et al. Preparation of self-assembled nanoparticles of ε-polylysine-sodium alginate: a sustained-release carrier for antigen delivery. Colloids Surf B Biointerfaces. (2018) 171:406–12. doi: 10.1016/j.colsurfb.2018.07.058
213. Wu H, Bao Y, Wang X, Zhou D, Wu W. Alkyl polyglycoside, a highly promising adjuvant in intranasal split influenza vaccines. Hum Vaccin Immunother. (2017) 13:1–9. doi: 10.1080/21645515.2016.1278098
214. Bacon A, Makin J, Sizer PJ, Jabbal-Gill I, Hinchcliffe M, Illum L, et al. Carbohydrate biopolymers enhance antibody responses to mucosally delivered vaccine antigens. Infect Immun. (2000) 68:5764–70. doi: 10.1128/IAI.68.10.5764-5770.2000
215. Bagheripour MJ, Ebrahimi F, Hajizade A, Nazarian S. Immunogenicity evaluation of rBoNT/E nanovaccine after mucosal administration. Iran J Basic Med Sci. (2019) 22:353–9.
216. Díaz AG, Quinteros DA, Paolicchi FA, Rivero MA, Palma SD, Pardo RP, et al. Mucosal immunization with polymeric antigen BLSOmp31 using alternative delivery systems against Brucella ovis in rams. Vet Immunol Immunopathol. (2019) 209:70–7. doi: 10.1016/j.vetimm.2019.02.005
217. Ghaffari Marandi BH, Zolfaghari MR, Kazemi R, Motamedi MJ, Amani J. Immunization against Vibrio cholerae, ETEC, and EHEC with chitosan nanoparticle containing LSC chimeric protein. Microb Pathog. (2019) 134:103600. doi: 10.1016/j.micpath.2019.103600
218. Kole S, Qadiri SSN, Shin SM, Kim WS, Lee J, Jung SJ. Nanoencapsulation of inactivated-viral vaccine using chitosan nanoparticles: evaluation of its protective efficacy and immune modulatory effects in olive flounder (Paralichthys olivaceus) against viral haemorrhagic septicaemia virus (VHSV) infection. Fish Shellfish Immunol. (2019) 91:136–47. doi: 10.1016/j.fsi.2019.05.017
219. Korupalli C, Pan WY, Yeh CY, Chen PM, Mi FL, Tsai HW, et al. Single-injecting, bioinspired nanocomposite hydrogel that can recruit host immune cells in situ to elicit potent and long-lasting humoral immune responses. Biomaterials. (2019) 216:119268. doi: 10.1016/j.biomaterials.2019.119268
220. Muralidharan A, Russell MS, Larocque L, Gravel C, Sauvé S, Chen Z, et al. Chitosan alters inactivated respiratory syncytial virus vaccine elicited immune responses without affecting lung histopathology in mice. Vaccine. (2019) 37:4031–9. doi: 10.1016/j.vaccine.2019.06.003
221. Senevirathne A, Hewawaduge C, Hajam IA, Lalsiamthara J, Lee JH. Intranasally administered anti-Brucella subunit vaccine formulation induces protective immune responses against nasal Brucella challenge. Vet Microbiol. (2019) 228:112–8. doi: 10.1016/j.vetmic.2018.11.022
222. Singh A, Nisaa K, Bhattacharyya S, Mallick AI. Immunogenicity and protective efficacy of mucosal delivery of recombinant hcp of Campylobacter jejuni type VI secretion system (T6SS) in chickens. Mol Immunol. (2019) 111:182–97. doi: 10.1016/j.molimm.2019.04.016
223. Soh SH, Shim S, Im YB, Park HT, Cho CS, Yoo HS. Induction of Th2-related immune responses and production of systemic IgA in mice intranasally immunized with Brucella abortus malate dehydrogenase loaded chitosan nanoparticles. Vaccine. (2019) 37:1554–64. doi: 10.1016/j.vaccine.2019.02.005
224. Zhang J, Fu X, Zhang Y, Zhu W, Zhou Y, Yuan G, et al. Chitosan and anisodamine improve the immune efficacy of inactivated infectious spleen and kidney necrosis virus vaccine in Siniperca chuatsi. Fish Shellfish Immunol. (2019) 89:52–60. doi: 10.1016/j.fsi.2019.03.040
225. Hojatizade M, Soleymani M, Tafaghodi M, Badiee A, Chavoshian O, Jaafari MR. Chitosan nanoparticles loaded with whole and soluble leishmania antigens, and evaluation of their immunogenecity in a mouse model of leishmaniasis. Iran J Immunol. (2018) 15:281–93. doi: 10.22034/IJI.2018.39397
226. Huang T, Song X, Jing J, Zhao K, Shen Y, Zhang X, et al. Chitosan-DNA nanoparticles enhanced the immunogenicity of multivalent DNA vaccination on mice against Trueperella pyogenes infection. J Nanobiotechnol. (2018) 16:8. doi: 10.1186/s12951-018-0337-2
227. Malik A, Gupta M, Mani R, Gogoi H, Bhatnagar R. Trimethyl chitosan nanoparticles encapsulated protective antigen protects the mice against anthrax. Front Immunol. (2018) 9:562. doi: 10.3389/fimmu.2018.00562
228. Sun B, Yu S, Zhao D, Guo S, Wang X, Zhao K. Polysaccharides as vaccine adjuvants. Vaccine. (2018) 36:5226–34. doi: 10.1016/j.vaccine.2018.07.040
229. Lebre F, Bento D, Ribeiro J, Colaço M, Borchard G, de Lima MCP, et al. Association of chitosan and aluminium as a new adjuvant strategy for improved vaccination. Int J Pharm. (2017) 527:103–14. doi: 10.1016/j.ijpharm.2017.05.028
230. Spinner JL, Oberoi HS, Yorgensen YM, Poirier DS, Burkhart DJ, Plante M, et al. Methylglycol chitosan and a synthetic TLR4 agonist enhance immune responses to influenza vaccine administered sublingually. Vaccine. (2015) 33:5845–53. doi: 10.1016/j.vaccine.2015.08.086
231. Torlak E, Sert D. Antibacterial effectiveness of chitosan-propolis coated polypropylene films against foodborne pathogens. Int J Biol Macromol. (2013) 60:52–5. doi: 10.1016/j.ijbiomac.2013.05.013
232. Kang ML, Kang SG, Jiang HL, Guo DD, Lee DY, Rayamahji N, et al. Chitosan microspheres containing Bordetella bronchiseptica antigens as novel vaccine against atrophic rhinitis in pigs. J Microbiol Biotechnol. (2008) 18:1179–85.
233. de Paula Oliveira Santos B, Trentini MM, Machado RB, Rúbia Nunes Celes M, Kipnis A, Petrovsky N, et al. Advax4 delta inulin combination adjuvant together with ECMX, a fusion construct of four protective mTB antigens, induces a potent Th1 immune response and protects mice against Mycobacterium tuberculosis infection. Hum Vaccin Immunother. (2017) 13:2967–76. doi: 10.1080/21645515.2017.1368598
234. Horst G, Levine R, Chick R, Hofacre C. Effects of beta-1,3-glucan (AletaTM) on vaccination response in broiler chickens. Poult Sci. (2019) 98:1643–7. doi: 10.3382/ps/pey523
235. Wang H, Yang B, Wang Y, Liu F, Fernández-Tejada A, Dong S. β-Glucan as an immune activator and a carrier in the construction of a synthetic MUC1 vaccine. Chem Commun. (2018) 55:253–6. doi: 10.1039/C8CC07691J
236. Whelan AO, Flick-Smith HC, Homan J, Shen ZT, Carpenter Z, Khoshkenar P, et al. Protection induced by a Francisella tularensis subunit vaccine delivered by glucan particles. PLoS ONE. (2018) 13:e0200213. doi: 10.1371/journal.pone.0200213
237. Hung CY, Zhang H, Castro-Lopez N, Ostroff GR, Khoshlenar P, Abraham A, et al. Glucan-chitin particles enhance Th17 response and improve protective efficacy of a multivalent antigen (rCpa1) against pulmonary Coccidioides posadasii infection. Infect Immun. (2018) 86:e00070–18. doi: 10.1128/IAI.00070-18
238. Bundle DR, Paszkiewicz E, Elsaidi HRH, Mandal SS, Sarkar S. A three component synthetic vaccine containing a β-mannan T-cell peptide epitope and a β-glucan dendritic cell ligand. Molecules. (2018) 23:E1961. doi: 10.3390/molecules23081961
239. Ferreira LG, Endrighi M, Lisenko KG, de Oliveira MRD, Damasceno MR, Claudino JA, et al. Oat beta-glucan as a dietary supplement for dogs. PLoS ONE. (2018) 13:e0201133. doi: 10.1371/journal.pone.0201133
240. Deepe GS, Buesing WR, Ostroff GR, Abraham A, Specht CA, Huang H, et al. Vaccination with an alkaline extract of Histoplasma capsulatum packaged in glucan particles confers protective immunity in mice. Vaccine. (2018) 36:3359–67. doi: 10.1016/j.vaccine.2018.04.047
241. Jin Y, Li P, Wang F. β-glucans as potential immunoadjuvants: a review on the adjuvanticity, structure-activity relationship and receptor recognition properties. Vaccine. (2018) 36:5235–44. doi: 10.1016/j.vaccine.2018.07.038
242. Li P, Asokanathan C, Liu F, Khaing KK, Kmiec D, Wei X, et al. PLGA nano/micro particles encapsulated with pertussis toxoid (PTd) enhances Th1/Th17 immune response in a murine model. Int J Pharm. (2016) 513:183–90. doi: 10.1016/j.ijpharm.2016.08.059
243. Shi W, Kou Y, Jiang H, Gao F, Kong W, Su W, et al. Novel intranasal pertussis vaccine based on bacterium-like particles as a mucosal adjuvant. Immunol Lett. (2018) 198:26–32. doi: 10.1016/j.imlet.2018.03.012
244. Claesson MH. Immunological links to nonspecific effects of DTwP and BCG vaccines on infant mortality. J Trop Med. (2011) 2011:706304. doi: 10.1155/2011/706304
245. Nascimento IP, Dias WO, Quintilio W, Hsu T, Jacobs WR, Leite LC. Construction of an unmarked recombinant BCG expressing a pertussis antigen by auxotrophic complementation: protection against Bordetella pertussis challenge in neonates. Vaccine. (2009) 27:7346–51. doi: 10.1016/j.vaccine.2009.09.043
246. Nascimento IP, Dias WO, Quintilio W, Christ AP, Moraes JF, Vancetto MD, et al. Neonatal immunization with a single dose of recombinant BCG expressing subunit S1 from pertussis toxin induces complete protection against Bordetella pertussis intracerebral challenge. Microbes Infect. (2008) 10:198–202. doi: 10.1016/j.micinf.2007.10.010
247. Nascimento IP, Dias WO, Mazzantini RP, Miyaji EN, Gamberini M, Quintilio W, et al. Recombinant Mycobacterium bovis BCG expressing pertussis toxin subunit S1 induces protection against an intracerebral challenge with live Bordetella pertussis in mice. Infect Immun. (2000) 68:4877–83. doi: 10.1128/IAI.68.9.4877-4883.2000
248. Cainelli Gebara VC, Risoléo L, Lopes AP, Ferreira VR, Quintilio W, Lépine F, et al. Adjuvant and immunogenic activities of the 73kDa N-terminal alpha-domain of BrkA autotransporter and Cpn60/60kDa chaperonin of Bordetella pertussis. Vaccine. (2007) 25:621–9. doi: 10.1016/j.vaccine.2006.08.033
249. Oliver DC, Fernandez RC. Antibodies to BrkA augment killing of Bordetella pertussis. Vaccine. (2001) 20:235–41. doi: 10.1016/S0264-410X(01)00269-9
250. Marr N, Shah NR, Lee R, Kim EJ, Fernandez RC. Bordetella pertussis autotransporter Vag8 binds human C1 esterase inhibitor and confers serum resistance. PLoS ONE. (2011) 6:e20585. doi: 10.1371/journal.pone.0020585
251. Finn TM, Amsbaugh DF. Vag8, a Bordetella pertussis bvg-regulated protein. Infect Immun. (1998) 66:3985–9.
252. Suzuki K, Shinzawa N, Ishigaki K, Nakamura K, Abe H, Fukui-Miyazaki A, et al. Protective effects of in vivo-expressed autotransporters against Bordetella pertussis infection. Microbiol Immunol. (2017) 61:371–9. doi: 10.1111/1348-0421.12504
253. Boehm DT, Hall JM, Wong TY, DiVenere AM, Sen-Kilic E, Bevere JR, et al. Evaluation of adenylate cyclase toxoid antigen in acellular pertussis vaccines by using a Bordetella pertussis challenge model in mice. Infect Immun. (2018) 86:e00857–17. doi: 10.1128/IAI.00857-17
254. Wang X, Gray MC, Hewlett EL, Maynard JA. The Bordetella adenylate cyclase repeat-in-toxin (RTX) domain is immunodominant and elicits neutralizing antibodies. J Biol Chem. (2015) 290:23025. doi: 10.1074/jbc.A114.585281
255. Jennings-Gee J, Quataert S, Ganguly T, D'Agostino R, Deora R, Dubey P. The adjuvant Bordetella colonization factor A attenuates alum-induced Th2 responses and enhances Bordetella pertussis clearance from mouse lungs. Infect Immun. (2018) 86:e00935–17. doi: 10.1128/IAI.00935-17
256. Scanlon KM, Skerry C, Carbonetti NH. Novel therapies for the treatment of pertussis disease. Pathog Dis. (2015) 73:ftv074. doi: 10.1093/femspd/ftv074
257. Skerry CM, Mahon BP. A live, attenuated Bordetella pertussis vaccine provides long-term protection against virulent challenge in a murine model. Clin Vaccine Immunol. (2011) 18:187–93. doi: 10.1128/CVI.00371-10
258. Skerry CM, Cassidy JP, English K, Feunou-Feunou P, Locht C, Mahon BP. A live attenuated Bordetella pertussis candidate vaccine does not cause disseminating infection in gamma interferon receptor knockout mice. Clin Vaccine Immunol. (2009) 16:1344–51. doi: 10.1128/CVI.00082-09
259. Brennan MJ. A new whooping cough vaccine that may prevent colonization and transmission. Vaccines. (2017) 5:E43. doi: 10.3390/vaccines5040043
260. Locht C, Papin JF, Lecher S, Debrie AS, Thalen M, Solovay K, et al. Live attenuated pertussis vaccine BPZE1 protects baboons against Bordetella pertussis disease and infection. J Infect Dis. (2017) 216:117–24. doi: 10.1093/infdis/jix254
261. Locht C. Live pertussis vaccines will they protect against carriage and spread of pertussis? Clin Microbiol Infect. (2016) 22(Suppl. 5):S96–102. doi: 10.1016/j.cmi.2016.05.029
262. Locht C. Pertussis: where did we go wrong and what can we do about it? J Infect. (2016) 72(Suppl.): S34–40. doi: 10.1016/j.jinf.2016.04.020
263. Feunou PF, Kammoun H, Debrie AS, Locht C. Heterologous prime-boost immunization with live attenuated B. pertussis BPZE1 followed by acellular pertussis vaccine in mice. Vaccine. (2014) 32:4281–8. doi: 10.1016/j.vaccine.2014.06.019
264. Jahnmatz M, Amu S, Ljungman M, Wehlin L, Chiodi F, Mielcarek N, et al. B-cell responses after intranasal vaccination with the novel attenuated Bordetella pertussis vaccine strain BPZE1 in a randomized phase I clinical trial. Vaccine. (2014) 32:3350–6. doi: 10.1016/j.vaccine.2014.04.048
265. Lim A, Ng JK, Locht C, Alonso S. Protective role of adenylate cyclase in the context of a live pertussis vaccine candidate. Microbes Infect. (2014) 16:51–60. doi: 10.1016/j.micinf.2013.10.002
266. Schiavoni I, Fedele G, Quattrini A, Bianco M, Schnoeller C, Openshaw PJ, et al. Live attenuated B. pertussis BPZE1 rescues the immune functions of Respiratory Syncytial virus infected human dendritic cells by promoting Th1/Th17 responses. PLoS ONE. (2014) 9:e100166. doi: 10.1371/journal.pone.0100166
267. Schnoeller C, Roux X, Sawant D, Raze D, Olszewska W, Locht C, et al. Attenuated Bordetella pertussis vaccine protects against respiratory syncytial virus disease via an IL-17-dependent mechanism. Am J Respir Crit Care Med. (2014) 189:194–202. doi: 10.1164/rccm.201307-1227OC
268. Thorstensson R, Trollfors B, Al-Tawil N, Jahnmatz M, Bergström J, Ljungman M, et al. A phase I clinical study of a live attenuated Bordetella pertussis vaccine–BPZE1; a single centre, double-blind, placebo-controlled, dose-escalating study of BPZE1 given intranasally to healthy adult male volunteers. PLoS ONE. (2014) 9:e83449. doi: 10.1371/journal.pone.0083449
269. Kammoun H, Roux X, Raze D, Debrie AS, De Filette M, Ysenbaert T, et al. Immunogenicity of live attenuated B. pertussis BPZE1 producing the universal influenza vaccine candidate M2e. PLoS ONE. (2013) 8:e59198. doi: 10.1371/journal.pone.0059198
270. Kammoun H, Feunou PF, Foligne B, Debrie AS, Raze D, Mielcarek N, et al. Dual mechanism of protection by live attenuated Bordetella pertussis BPZE1 against Bordetella bronchiseptica in mice. Vaccine. (2012) 30:5864–70. doi: 10.1016/j.vaccine.2012.07.005
271. Li R, Cheng C, Chong SZ, Lim AR, Goh YF, Locht C, et al. Attenuated Bordetella pertussis BPZE1 protects against allergic airway inflammation and contact dermatitis in mouse models. Allergy. (2012) 67:1250–8. doi: 10.1111/j.1398-9995.2012.02884.x
272. Fedele G, Bianco M, Debrie AS, Locht C, Ausiello CM. Attenuated Bordetella pertussis vaccine candidate BPZE1 promotes human dendritic cell CCL21-induced migration and drives a Th1/Th17 response. J Immunol. (2011) 186:5388–96. doi: 10.4049/jimmunol.1003765
273. Li R, Lim A, Alonso S. Attenuated Bordetella pertussis BPZE1 as a live vehicle for heterologous vaccine antigens delivery through the nasal route. Bioeng Bugs. (2011) 2:315–9. doi: 10.4161/bbug.2.6.18167
274. Li R, Lim A, Ow ST, Phoon MC, Locht C, Chow VT, et al. Development of live attenuated Bordetella pertussis strains expressing the universal influenza vaccine candidate M2e. Vaccine. (2011) 29:5502–11. doi: 10.1016/j.vaccine.2011.05.052
275. Feunou PF, Bertout J, Locht C. T- and B-cell-mediated protection induced by novel, live attenuated pertussis vaccine in mice. Cross protection against parapertussis. PLoS ONE. (2010) 5:e10178. doi: 10.1371/journal.pone.0010178
276. Feunou PF, Kammoun H, Debrie AS, Mielcarek N, Locht C. Long-term immunity against pertussis induced by a single nasal administration of live attenuated B. pertussis BPZE1. Vaccine. (2010) 28:7047–53. doi: 10.1016/j.vaccine.2010.08.017
277. Kavanagh H, Noone C, Cahill E, English K, Locht C, Mahon BP. Attenuated Bordetella pertussis vaccine strain BPZE1 modulates allergen-induced immunity and prevents allergic pulmonary pathology in a murine model. Clin Exp Allergy. (2010) 40:933–41. doi: 10.1111/j.1365-2222.2010.03459.x
278. Li R, Lim A, Phoon MC, Narasaraju T, Ng JK, Poh WP, et al. Attenuated Bordetella pertussis protects against highly pathogenic influenza A viruses by dampening the cytokine storm. J Virol. (2010) 84:7105–13. doi: 10.1128/JVI.02542-09
279. Mielcarek N, Debrie AS, Mahieux S, Locht C. Dose response of attenuated Bordetella pertussis BPZE1-induced protection in mice. Clin Vaccine Immunol. (2010) 17:317–24. doi: 10.1128/CVI.00322-09
280. Feunou PF, Ismaili J, Debrie AS, Huot L, Hot D, Raze D, et al. Genetic stability of the live attenuated Bordetella pertussis vaccine candidate BPZE1. Vaccine. (2008) 26:5722–7. doi: 10.1016/j.vaccine.2008.08.018
281. Ho SY, Chua SQ, Foo DG, Locht C, Chow VT, Poh CL, et al. Highly attenuated Bordetella pertussis strain BPZE1 as a potential live vehicle for delivery of heterologous vaccine candidates. Infect Immun. (2008) 76:111–9. doi: 10.1128/IAI.00795-07
282. Mielcarek N, Debrie AS, Raze D, Bertout J, Rouanet C, Younes AB, et al. Live attenuated B. pertussis as a single-dose nasal vaccine against whooping cough. PLoS Pathog. (2006) 2:e65. doi: 10.1371/journal.ppat.0020065
283. Debrie AS, Coutte L, Raze D, Mooi F, Alexander F, Gorringe A, et al. Construction and evaluation of Bordetella pertussis live attenuated vaccine strain BPZE1 producing Fim3. Vaccine. (2018) 36:1345–52. doi: 10.1016/j.vaccine.2018.02.017
284. Solans L, Debrie AS, Borkner L, Aguiló N, Thiriard A, Coutte L, et al. IL-17-dependent SIgA-mediated protection against nasal Bordetella pertussis infection by live attenuated BPZE1 vaccine. Mucosal Immunol. (2018) 11:1753–62. doi: 10.1038/s41385-018-0073-9
285. Gasperini G, Biagini M, Arato V, Gianfaldoni C, Vadi A, Norais N, et al. Outer Membrane Vesicles (OMV)-based and proteomics-driven antigen selection identifies novel factors contributing to epithelial cells. Mol Cell Proteomics. (2018) 17:205–15. doi: 10.1074/mcp.RA117.000045
286. Gasperini G, Biagini M, Arato V, Gianfaldoni C, Vadi A, Norais N, et al. Outer Membrane Vesicles (OMV)-based and proteomics-driven antigen selection identifies novel factors contributing to Bordetella pertussis adhesion to epithelial cells. Mol Cell Proteomics. (2017) 17:205–15.
287. Saito M, Odanaka K, Otsuka N, Kamachi K, Watanabe M. Development of vaccines against pertussis caused by Bordetella holmesii using a mouse intranasal challenge model. Microbiol Immunol. (2016) 60:599–608. doi: 10.1111/1348-0421.12409
288. Bottero D, Zurita ME, Gaillard ME, Bartel E, Vercellini C, Hozbor D. Membrane vesicles derived from Bordetella bronchiseptica: active constituent of a new vaccine against infections caused by this pathogen. Appl Environ Microbiol. (2018) 84:e01877–17. doi: 10.1128/AEM.01877-17
289. Bottero D, Zurita ME, Gaillard ME, Bartel E, Vercellini C, Hozbor D. Membrane vesicles derived from Bordetella bronchiseptica: active constituent of a new vaccine against infections caused by this pathogen. Appl Environ Microbiol. (2017) 84:e01877–17.
290. Bottero D, Gaillard ME, Zurita E, Moreno G, Martinez DS, Bartel E, et al. Characterization of the immune response induced by pertussis OMVs-based vaccine. Vaccine. (2016) 34:3303–9. doi: 10.1016/j.vaccine.2016.04.079
291. Gaillard ME, Bottero D, Errea A, Ormazábal M, Zurita ME, Moreno G, et al. Acellular pertussis vaccine based on outer membrane vesicles capable of conferring both long-lasting immunity and protection against different strain genotypes. Vaccine. (2014) 32:931–7. doi: 10.1016/j.vaccine.2013.12.048
292. Ormazábal M, Bartel E, Gaillard ME, Bottero D, Errea A, Zurita ME, et al. Characterization of the key antigenic components of pertussis vaccine based on outer membrane vesicles. Vaccine. (2014) 32:6084–90. doi: 10.1016/j.vaccine.2014.08.084
293. Bottero D, Gaillard ME, Errea A, Moreno G, Zurita E, Pianciola L, et al. Outer membrane vesicles derived from Bordetella parapertussis as an acellular vaccine against Bordetella parapertussis and Bordetella pertussis infection. Vaccine. (2013) 31:5262–8. doi: 10.1016/j.vaccine.2013.08.059
294. Gaillard ME, Bottero D, Castuma CE, Basile LA, Hozbor D. Laboratory adaptation of Bordetella pertussis is associated with the loss of type three secretion system functionality. Infect Immun. (2011) 79:3677–82. doi: 10.1128/IAI.00136-11
295. Roberts R, Moreno G, Bottero D, Gaillard ME, Fingermann M, Graieb A, et al. Outer membrane vesicles as acellular vaccine against pertussis. Vaccine. (2008) 26:4639–46. doi: 10.1016/j.vaccine.2008.07.004
296. Raeven RH, Brummelman J, Pennings JLA, van der Maas L, Helm K, Tilstra WA, et al. Molecular and cellular signatures underlying superior immunity against Bordetella pertussis upon pulmonary vaccination. Mucosal Immunol. (2018) 11:979–93. doi: 10.1038/mi.2017.81
297. Raeven RH, van der Maas L, Tilstra W, Uittenbogaard JP, Bindels TH, Kuipers BA, et al. Immunoproteomic profiling of Bordetella pertussis outer membrane vesicle vaccine reveals broad and balanced humoral immunogenicity. J Proteome Res. (2015) 14:2929–42. doi: 10.1021/acs.jproteome.5b00258
298. Raeven RH, Brummelman J, Pennings JL, van der Maas L, Tilstra W, Helm K, et al. Bordetella pertussis outer membrane vesicle vaccine confers equal efficacy in mice with milder inflammatory responses compared to a whole-cell vaccine. Sci Rep. (2016) 6:38240. doi: 10.1038/srep38240
299. Raeven RHM, Brummelman J, Pennings JLA, van der Maas L, Helm K, Tilstra WA, et al. Molecular and cellular signatures underlying superior immunity against Bordetella pertussis upon pulmonary vaccination. Mucosal Immunol. (2018) 11:979–93. doi: 10.1038/mi.2017.110
300. Kanojia G, Raeven RHM, van der Maas L, Bindels THE, van Riet E, Metz B, et al. Development of a thermostable spray dried outer membrane vesicle pertussis vaccine for pulmonary immunization. J Control Release. (2018) 286:167–78. doi: 10.1016/j.jconrel.2018.07.035
301. Weiss AA, Patton AK, Millen SH, Chang SJ, Ward JI, Bernstein DI. Acellular pertussis vaccines and complement killing of Bordetella pertussis. Infect Immun. (2004) 72:7346–51. doi: 10.1128/IAI.72.12.7346-7351.2004
302. Coutte L, Locht C. Investigating pertussis toxin and its impact on vaccination. Future Microbiol. (2015) 10:241–54. doi: 10.2217/fmb.14.123
303. Isbrucker RA, Bliu A, Prior F. Modified binding assay for improved sensitivity and specificity in the detection of residual pertussis toxin in vaccine preparations. Vaccine. (2010) 28:2687–92. doi: 10.1016/j.vaccine.2010.01.040
304. Quentin-Millet MJ, Arminjon F, Danve B, Cadoz M, Armand J. Acellular pertussis vaccines: evaluation of reversion in a nude mouse model. J Biol Stand. (1988) 16:99–108. doi: 10.1016/0092-1157(88)90037-6
305. Kilár A, Dörnyei Á, Kocsis B. Structural characterization of bacterial lipopolysaccharides with mass spectrometry and on- and off-line separation techniques. Mass Spectrom Rev. (2013) 32:90–117. doi: 10.1002/mas.21352
306. Higgs R, Higgins SC, Ross PJ, Mills KH. Immunity to the respiratory pathogen Bordetella pertussis. Mucosal Immunol. (2012) 5:485–500. doi: 10.1038/mi.2012.54
307. Mahon BP, Ryan MS, Griffin F, Mills KH. Interleukin-12 is produced by macrophages in response to live or killed Bordetella pertussis and enhances the efficacy of an acellular pertussis vaccine by promoting induction of Th1 cells. Infect Immun. (1996) 64:5295–301.
308. Kubler-Kielb J, Vinogradov E, Lagergård T, Ginzberg A, King JD, Preston A, et al. Oligosaccharide conjugates of Bordetella pertussis and bronchiseptica induce bactericidal antibodies, an addition to pertussis vaccine. Proc Natl Acad Sci USA. (2011) 108:4087–92. doi: 10.1073/pnas.1100782108
309. Niedziela T, Letowska I, Lukasiewicz J, Kaszowska M, Czarnecka A, Kenne L, et al. Epitope of the vaccine-type Bordetella pertussis strain 186 lipooligosaccharide and antiendotoxin activity of antibodies directed against the terminal pentasaccharide-tetanus toxoid conjugate. Infect Immun. (2005) 73:7381–9. doi: 10.1128/IAI.73.11.7381-7389.2005
310. Koj S, Ługowski C, Niedziela T. [Bordetella pertussis lipooligosaccharide-derived neoglycoconjugates - new components of pertussis vaccine]. Postepy Hig Med Dosw (Online) (2015) 69:1013–30.
311. Ernst K, Eberhardt N, Mittler AK, Sonnabend M, Anastasia A, Freisinger S, et al. Pharmacological cyclophilin inhibitors prevent intoxication of mammalian cells with Bordetella pertussis toxin. Toxins. (2018) 10:E181. doi: 10.3390/toxins10050181
312. Kaiser E, Pust S, Kroll C, Barth H. Cyclophilin A facilitates translocation of the Clostridium botulinum C2 toxin across membranes of acidified endosomes into the cytosol of mammalian cells. Cell Microbiol. (2009) 11:780–95. doi: 10.1111/j.1462-5822.2009.01291.x
313. Lang AE, Ernst K, Lee H, Papatheodorou P, Schwan C, Barth H, et al. The chaperone Hsp90 and PPIases of the cyclophilin and FKBP families facilitate membrane translocation of Photorhabdus luminescens ADP-ribosyltransferases. Cell Microbiol. (2014) 16:490–503. doi: 10.1111/cmi.12228
314. Shitara Y, Takeuchi K, Nagamatsu Y, Wada S, Sugiyama Y, Horie T. Long-lasting inhibitory effects of cyclosporin A, but not tacrolimus, on OATP1B1- and OATP1B3-mediated uptake. Drug Metab Pharmacokinet. (2012) 27:368–78. doi: 10.2133/dmpk.DMPK-11-RG-096
315. Fabre I, Fabre G, Lena N, Cano JP. Kinetics of uptake and intracellular binding of Cyclosporine A in RAJI cells, in vitro. Biochem Pharmacol. (1986) 35:4261–6. doi: 10.1016/0006-2952(86)90704-5
Keywords: immunomodulation, Bordetella, Toll-receptors, adjuvants, pertussis
Citation: Gestal MC, Johnson HM and Harvill ET (2019) Immunomodulation as a Novel Strategy for Prevention and Treatment of Bordetella spp. Infections. Front. Immunol. 10:2869. doi: 10.3389/fimmu.2019.02869
Received: 16 September 2019; Accepted: 22 November 2019;
Published: 13 December 2019.
Edited by:
Roberta Antonia Diotti, Vita-Salute San Raffaele University, ItalyReviewed by:
Rene Raeven, Intravacc, NetherlandsGiorgio Fedele, Istituto Superiore di Sanità (ISS), Italy
Copyright © 2019 Gestal, Johnson and Harvill. This is an open-access article distributed under the terms of the Creative Commons Attribution License (CC BY). The use, distribution or reproduction in other forums is permitted, provided the original author(s) and the copyright owner(s) are credited and that the original publication in this journal is cited, in accordance with accepted academic practice. No use, distribution or reproduction is permitted which does not comply with these terms.
*Correspondence: Monica C. Gestal, bWNhcmdlc0BnbWFpbC5jb20=; Eric T. Harvill, aGFydmlsbEB1Z2EuZWR1