- 1Division of Infectious Diseases and Immunology, Department of Biomolecular Health Sciences, Utrecht University, Utrecht, Netherlands
- 2Division of Clinical Microbiology, Department of Laboratory Medicine, Karolinska Institute, Stockholm, Sweden
- 3Department of Clinical Microbiology, Karolinska University Laboratory, Stockholm, Sweden
Cathelicidins are short cationic peptides that are part of the innate immune system. At first, these peptides were studied mostly for their direct antimicrobial killing capacity, but nowadays they are more and more appreciated for their immunomodulatory functions. In this review, we will provide a comprehensive overview of the various effects cathelicidins have on the detection of damage- and microbe-associated molecular patterns, with a special focus on their effects on Toll-like receptor (TLR) activation. We review the available literature based on TLR ligand types, which can roughly be divided into lipidic ligands, such as LPS and lipoproteins, and nucleic-acid ligands, such as RNA and DNA. For both ligand types, we describe how direct cathelicidin-ligand interactions influence TLR activation, by for instance altering ligand stability, cellular uptake and receptor interaction. In addition, we will review the more indirect mechanisms by which cathelicidins affect downstream TLR-signaling. To place all this information in a broader context, we discuss how these cathelicidin-mediated effects can have an impact on how the host responds to infectious organisms as well as how these effects play a role in the exacerbation of inflammation in auto-immune diseases. Finally, we discuss how these immunomodulatory activities can be exploited in vaccine development and cancer therapies.
Introduction
The discovery of penicillin in 1928 by Alexander Fleming was a major breakthrough in medicine. Ever since, the use of antibiotics saved millions of lives around the world, curing infections that were previously life threatening (1). However, due to the continuous expansion of antibiotic resistance among clinically relevant bacterial species, novel antimicrobials are urgently required to counter infections by these pathogens (2). One promising alternative to conventional antibiotics is the use of host defense peptides (HDPs), which refers to a large family of peptides with varying functions, including direct antimicrobial activity against a wide variety of bacterial, fungal and viral pathogens. Of special interest is the more recent description of the immunomodulatory functions of these peptides, which provides additional opportunities for potential clinical applications (3).
One group of HDPs that has been extensively studied in the context of their immunomodulatory activity is the cathelicidin family. This peptide family can be found in nearly all vertebrate species and has been shown to have a major impact on host responses toward various highly conserved microbe-associated molecular patterns (MAMPs). MAMPs activate the innate immune system through pattern recognition receptors (PRRs), of which Toll-like receptors (TLRs) are the most well-known receptor family. TLRs can be roughly divided into two subgroups; extracellular TLRs and intracellular TLRs, that recognize microbial membrane components and extracellular proteins or nucleic acids, respectively (4).
In this review, we aim to summarize the current knowledge on the mechanisms by which cathelicidins affect TLR activation and downstream signaling as well as how this impacts immune responses during both infections and sterile inflammation, including auto-immune responses.
Cathelicidins
Cathelicidins belong to the family of HDPs with each cathelicidin being encoded by a single gene, consisting of four exons. Cathelicidins are translated as a pre-pro-peptide, consisting of a signal peptide on the N-terminus that directs the peptide to secretory granules, followed by the highly conserved cathelin domain and ending in the active mature peptide at the C-terminus (5). Cathelicidins, such as the human cathelicidin LL-37, are commonly secreted by neutrophils in their biologically inactive pro-peptide form and require cleavage by extracellular enzymes such as elastase or proteinase-3 to release the biologically active C-terminal peptide (6). In the human skin, proteases such as kallikrein-5 have also been shown to cleave the LL-37 pro-peptide (hCAP18) once it is secreted by epithelial cells and keratinocytes. This leads not only to release of the active LL-37 peptide, but also to many different smaller fragments, such as LL-23, LL-29, and KS-27 (7). The mature cathelicidin peptides are highly variable in both amino-acid sequence and size, which leads to considerable differences in their 3D structure. They can contain to α-helices, β-hairpins, extended structures or form cyclic peptides. Some cathelicidins are rich in specific tryptophan, proline or arginine residues, while others are arranged in short tandem repeats (8–10). Since the mature peptides are highly diverse, not all cathelicidins will have similar activities which is important to keep in mind when studying these peptides. Importantly, despite these highly diverse structures, most peptides have a characteristic amphipathic nature and a net positive charge (8, 11).
Cathelicidins are expressed in nearly all vertebrates. In some species, only one cathelicidin has been identified, like human (LL-37), mouse (CRAMP) and dog (K9CATH). Other species, like chicken, horse, pig and cattle, express multiple cathelicidins (10). The main source of LL-37 in humans are neutrophils, which store the inactive pro-peptide in their secretory granules (12) and secrete them upon activation (13, 14). However, other cell types, including lymphocytes, macrophages, epithelial cells and keratinocytes, can also produce cathelicidins (15–17). Under homeostatic conditions, cathelicidins reach in vivo concentrations of around 0.2–0.5 μM in the plasma (12, 18), 0.2–2.0 μM in the lung mucosa (18), 0.01–1.1 μM in sweat (19), 0–4.4 μM in ascites fluid and 4–6 μM in saliva (18). Many cathelicidins are strongly upregulated during infection due to TLR activation by MAMPs, such as LPS, LTA and flagellin (20, 21). In addition, cathelicidins can be upregulated when tissues are damaged or by exposure to specific compounds, such as vitamin D3, butyrate and PGE2 (22–25). Under extreme conditions, for example in psoriatic lesions, more than 300 μM cathelicidin can be detected (26).
While best known for their direct antimicrobial activity against a broad spectrum of bacteria (27–29), viruses (30–32), fungi (33, 34), and parasites (35, 36), it is now well-established that these peptides also have the potential to modulate immune responses in various ways. This includes regulation of neutrophil and monocyte chemotaxis (37–39), induction of chemokine expression (27, 40), skewing of macrophage polarization (41), influencing phagocytosis (27, 42–44), and regulation of both extracellular and intracellular TLR activation (27, 40, 45–49). Due to this plethora of effects, it is perhaps not surprising that the reduced expression or total lack of cathelicidins is correlated with increased risk of infection (50, 51) but also has an impact on the development of autoimmune diseases (52–55).
Cathelicidins Inhibit The Activation of Lipid-Sensing TLRs
Lipid-Sensing TLRs
Extracellular TLRs are important in the detection of bacteria-derived lipid-containing molecules. Detection of such lipids is often the first step in the initiation of an immune response against many bacterial pathogens. Bacterial lipid-containing molecules that can activate TLRs include lipopolysaccharides (LPS) from the Gram-negative bacterial outer membrane (TLR4), lipoteichoic acids (LTA) from the Gram-positive bacterial cell wall and diverse di- and tri-acylated bacterial lipoproteins (TLR1/2/6). During activation, TLRs form homo- or heterodimers that are the basis of the TLR receptor complex. However, various co-receptors, such as MD-2 and CD14 have been shown to improve ligand detection by TLRs. Upon stimulation, TLR4 forms a receptor complex consisting of a TLR4 homodimer and two MD-2 proteins (4, 56, 57). The expression of the CD14 co-receptor can further enhance LPS detection and cellular responses. The soluble LPS-binding protein (LBP) can further act as a chaperone by extracting LPS from the bacterial membrane or bacterial-derived outer membrane vesicles and delivering it to the TLR4 receptor complex. TLR2 on the other hand forms heterodimers with either TLR1 or TLR6 (58, 59). These TLR2 heterodimers are responsible for the recognition of a variety of MAMPs, including LTA, di- and tri-acylated bacterial lipoproteins such as the highly common Braun lipoprotein in E. coli, lipoarabinomannan from mycobacteria, zymosan from fungi and hemagglutinin from measles viruses. In addition, synthetic lipoproteins based on these natural ligands, such as the di-acylated Pam2CSK4 (TLR2/6) and tri-acylated Pam3CSK4 (TLR1/2), are commonly used as TLR2 ligands for in vitro studies. Similar to TLR4 activation, expression of CD14 further increases the detection efficiency of TLR1/2/6 receptor complexes (56). Both TLR4 and TLR1/2/6 signal via the MyD88-dependent pathway, which ultimately leads to activation of NF-κB and AP-1 and thereby to the secretion of pro-inflammatory cytokines (56, 60). Importantly, TLR4 can also be present in endosomal compartments where activation can lead to TRIF-mediated signaling pathways, leading to the production of anti-inflammatory cytokines like IL-10 and type I interferons, predominantly IFN-β (61) (Figure 1).
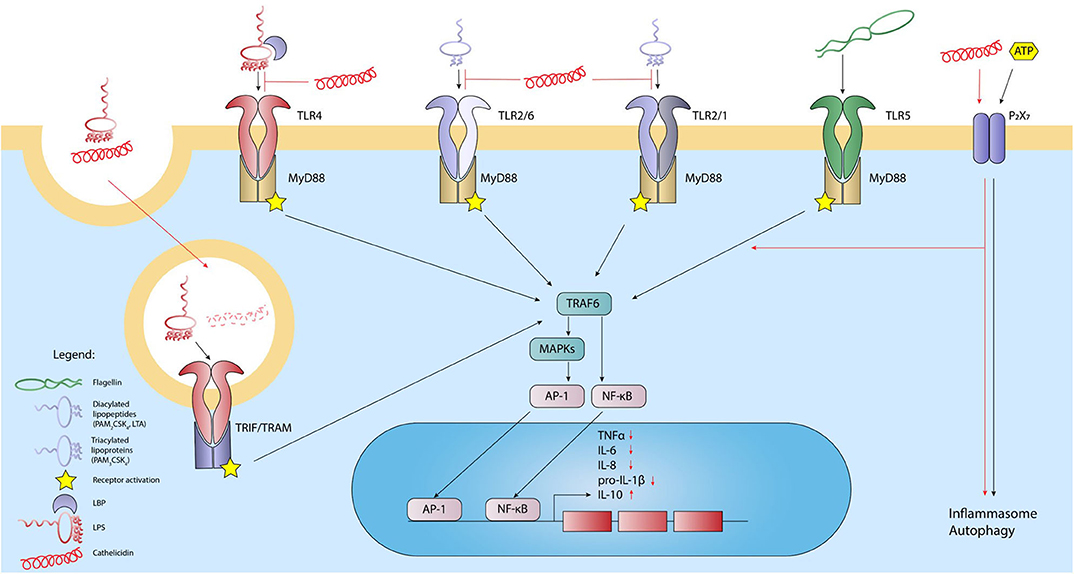
Figure 1. Cathelicidins inhibit the activation of lipid-sensing TLRs and modulate the response of other extracellular receptors. Activation of TLR4 by LPS, TLR1/2, or-2/6 heterodimers by LTA, and TLR5 by bacterial flagellin leads to activation of the intracellular domain. Through the adaptor MyD88 and subsequent downstream signaling through MAPKs, NFkB, and AP-1 are released and translocated to the nucleus, leading to transcription of inflammatory cytokines. LPS can also activate TLR4 located in endosomal compartments, which leads to activation through adaptors TRIF and TRAM. Additionally, P2X7 receptor responds to extracellular ATP, which leads to inflammasome formation. In general, cathelicidins inhibit lipid-induced TLR-activation and thereby the downstream inflammatory response by neutralizing lipid MAMPs. LPS-cathelicidin complexes can be taken up, after which the cathelicidins are degraded to allow LPS-induced TLR4 stimulation. Finally, cathelicidins can signal through the P2X7 receptor by which the peptides can stimulate inflammasome formation and autophagy. Black lines: normal signaling; red lines: effects exerted by cathelicidins.
Cathelicidins Inhibit LPS-Induced TLR4 Activation
Due to the fact that cathelicidins were initially described for their antimicrobial and membrane disruptive activity, many studies have focused on elucidating how cathelicidins interact with bacterial membranes or specific membrane components, such as LPS and LTA. Through these studies, it has become clear that cathelicidins are attracted to the bacterial membrane via electrostatic interactions between the negatively charged lipids in the bacterial outer membrane and the positively charged peptide (62). Indeed, loss of negatively charged phosphate-groups in the LPS core-region, for example due to mutations in LPS-controlling genes such as PhoP/PhoQ or PmrB/PmrA, reduces the susceptibility of Gram-negative bacteria to host defence peptides, like cathelicidins (63). While LPS-cathelicidin interaction may be important for eliciting antimicrobial activity, it was first shown in 1994 by Hirata et al. (64), that the 18 kDa rabbit cationic protein (CAP18) also exerts LPS-neutralizing activity, which drastically inhibits the inflammatory responses toward LPS both in vitro and in vivo (65–67). Later studies showed that this LPS-cathelicidin interaction resulted in reduced TLR4 activation and was not limited to hexa-acylated E. coli LPS (68–72), but was also observed in the context of penta-acylated P. aeruginosa LPS and the tetra-acylated P. gingivalis LPS (68, 70, 73). For several cathelicidins, including the human LL-37, it has now been shown that direct complex formation between LPS and cathelicidins plays an important role in preventing the binding of LPS to the TLR4 receptor complex, thereby reducing immune activation (66, 74–79). More detailed studies on LL-37 showed that binding of E. coli LPS occurs in a two-step process, with strong ionic interactions being followed by lower affinity interactions that are more dependent on entropic forces, such as interaction between hydrophobic regions of LPS and LL-37 (79, 80). That LPS neutralization is at least partially dependent on this ionic interaction is underlined by the fact that citrullinated LL-37 loses its ability to reduce the LPS-induced activation of macrophages (81, 82). Importantly, cathelicidins can influence LPS-induced TLR4 activation at different stages. Chicken cathelicidin-2 (CATH-2) and LL-37 have been shown to directly penetrate the bacterial membrane and bind to membrane lipids during the bacterial killing process (79, 80). Furthermore, several cathelicidins are able to bind LPS which was already bound to LBP or are capable of reducing the LPS concentration on the host cell surface (67, 76, 83, 84), suggesting competition with cell surface receptors (Figure 1). Important to note is that the mature cathelicidin peptides are highly diverse, which explains why some cathelicidins exert a strong inhibitory effect, while others do not seem to affect TLR4 activation by LPS at all (27). Similarly, cleavage of cathelicidins, which can alter the overall peptide charge or structure, can influence the LPS binding as well. For example, LL-23, a cleaved biological variant of LL-37 containing 23 amino acids, is unable to neutralize LPS (85), whereas the 31 amino acid long LL-31 is still able to inhibit the LPS-induced TLR4 activation (68).
While the interaction between cathelicidins and LPS is important for the regulation of TLR4 activation, several studies have suggested more indirect TLR4 regulation by cathelicidins. LL-37 pre-incubation, for instance, still leads to a reduction of the LPS-induced immune response in vitro, albeit to a lesser extent compared to LPS-LL-37 co-incubation (71). Furthermore, in human monocytes, LPS-mediated p50/p105 as well as TNF induced protein 2 expression were strongly inhibited by LL-37, in contrast to the much milder effects of LL-37 on for instance RELB, CCL4 and CXCL1 (71, 72). Similarly, LPS stimulation of bone marrow-derived macrophages from CRAMP knockout mice results in enhanced IL-10 production compared to stimulation of wildtype macrophages. However, no difference in TNF or MIP-2 production was observed between wildtype and CRAMP knockout cells (86). This selective influence on TLR4 activation could be the result of regulating the expression or functions of signaling molecules downstream of TLR4. The murine cathelicidin CRAMP for instance, reduced MyD88 synthesis and impaired the interaction between MyD88 and IRAK in murine macrophages upon LPS stimulation. In addition, CRAMP inhibited the phosphorylation of p38 and ERK downstream in this cascade, leading to a strong reduction of TNF production (86). Similarly, LL-37 was shown to inhibit the LPS-induced translocation of the NF-κB subunits p50 and p65, also resulting in a strong reduction of TNF (71, 87) and reduces the LPS-induced upregulation of TREM-1 by MyD88 (88). However, as these studies co-incubated cells with LPS and cathelicidins, it is difficult to assess to what extent these effects are just the result of reduced TLR4 activation due to the blocking of receptor activation. Nevertheless, it has been suggested that LL-37 can interact with intracellular GAPDH, an important enzyme in the glycolysis pathway, which subsequently promotes MAPK activation and chemokine expression (71). Such effects on MAPK activation can also have an impact on LPS-induced signaling pathways, which also use MAPK as intermediate signaling molecules. However, more research is needed to clarify to what extent these indirect regulatory effects of cathelicidins influence activation of TLR4 as well as other TLRs (Figure 1).
While most studies have clearly shown an inhibitory effect of cathelicidins on LPS-induced immune activation, there are also indications that in specific cases the interaction between LPS and cathelicidins can lead to enhanced cellular activation. This effect was first shown by Shaykhiev et al. (89), where LL-37-LPS complexes were shown to be taken up more efficiently in vitro by human bronchial epithelial cells, which subsequently led to enhanced intracellular TLR4 activation and increased IL-6 production (89). Similarly, a human adenocarcinoma colonic epithelial cell line also responded with an enhanced inflammatory response toward LPS-LL-37 complexes compared to LPS by itself (24) (Figure 1). Nevertheless, increasing the cellular uptake of LPS does not always lead to an enhanced response. For instance, cathelicidin-mediated uptake of LPS was also observed in cultured human liver sinusoidal endothelial cells; however, this did not lead to an altered immune activation in these cells (90).
Cathelicidins Inhibit LTA-Induced Activation of TLR1/2/6
Whereas the effects of cathelicidins on LPS-induced TLR4 activation are very well-studied, the influence of cathelicidins on lipid-induced TLR1/2/6 activation is less well-known. Nevertheless, several cathelicidins were shown to inhibit LTA- or Pam3CSK4-induced TLR1/2 activation and Pam2CSK4-induced TLR2/6 activation. This includes LL-37-mediated inhibition of TNF and IL-6 release in LTA-stimulated PBMCs and dendritic cells, as well as inhibition of LTA-induced TNF release in macrophages by several cathelicidins from different species (27, 72, 91). However, similar to the inhibition of LPS-induced activation, not all cathelicidins are able to reduce TLR1/2/6-activation or might be less effective (27, 68, 72, 75). Like LPS-neutralization, the mechanism by which cathelicidins inhibit TLR2 has also been associated with direct interaction between cathelicidins and the TLR2 ligands. Using isothermal titration calorimetry as well as competition assays, both chicken CATH-2 and human LL-37 were shown to bind LTA and Pam3CSK4 directly (77, 79, 92), albeit with a lower affinity compared to their binding to LPS (77, 78). In addition, citrullinated LL-37 loses its ability to inhibit LTA-induced activation of macrophages (81), indicating that interaction with LTA, similar as with LPS, is dependent on ionic interactions. Despite the observed interaction between LL-37 and LTA, LL-37 is not able to inhibit TLR2 activation on all cell types. In human primary bronchial cells, co-incubation of LL-37 enhanced the Pam3CSK4-induced expression of IL-8 and IL-6, while no effect of LL-37 was observed in relation to LTA-induced keratinocyte activation (93). This indicates that the function of cathelicidins might differ in the context of different cell types. In addition, as TLR2 and TLR4 signaling pathways share many downstream signaling molecules, it is likely that the indirect effects of cathelicidins on downstream TLR signaling pathways related to TLR4 activation will also play a role in the cathelicidin-mediated regulation of TLR2 activation, although no proof has been provided for this yet (Figure 1).
Cathelicidins Enhance The Activation of Nucleic Acid-Sensing TLRs
Nucleic Acid-Sensing TLRs
Foreign nucleic acids from invading viruses, as well as several bacteria, are sensed by several intracellular PRRs. These include several DNA- and RNA-receptors in the cytoplasm, as well as specific TLRs (TLR3, -7, -8, and-9) expressed in endolysosomal compartments (94). Depending on the localization of a pathogen as well as the stage of infection, receptors at these different cellular compartments can be activated. For instance, viruses are obligate intracellular parasites, meaning they rely completely on host cells for their replication and survival and replicate their genome within the host cytoplasm. Alternatively, viral nucleic acids can be found extracellularly as well in apoptotic particles, which can be engulfed by host cells and thereby end up in TLR-containing endolysosomes (95). In contrast to extracellular TLRs, which can rapidly respond to ligands in the extracellular environment, several barriers exist for the activation of intracellular TLRs by nucleic acids. Firstly, due to the many nucleases present in the extracellular environment, most free extracellular nucleic-acids are degraded before cells have the opportunity to respond (96, 97). Secondly, because the nucleic acid sensing TLRs are located intracellularly, cells have to actively endocytose the DNA or RNA for it to reach its complementary TLR receptor (98, 99).
TLR9 recognizes unmethylated CpG-containing DNA motifs in bacterial genomic DNA and viral double stranded DNA (dsDNA). The unmethylated CpG-containing DNA motifs in bacterial DNA are mimicked by synthetic CpG oligodeoxynucleotides (ODN), which are widely used in experimental systems (94, 100, 101). TLR21 is the avian equivalent of TLR9, which also recognizes CpG-ODN (102). TLR7 and -8 are highly homologous to each other due to gene duplication and recognize viral single stranded RNA (ssRNA), RNA from bacteria such as group B Streptococci and possibly siRNAs as well. The synthetic agonists for TLR7 and -8 are antiviral nucleoside analogs such as R848 and imiquimod. TLR3 recognizes viral double-stranded RNA (dsRNA), which is mimicked by the synthetic analog polyinosinic-polycytidylic acid [poly(I:C)]. TLR3 might also respond to some ssRNA viruses, most likely during the replication phase when they copy their RNA (56, 94, 100, 101, 103).
Like most extracellular TLRs, TLR7, -8, and -9 signal through the MyD88-dependent signaling pathway, resulting in the secretion of proinflammatory cytokines. In addition, activation of the highly expressed TLR7 and -9 in plasmacytoid DCs (pDCs) results in high levels of type-I interferons, like IFN-α, which is important during anti-viral responses. TLR3 on the other hand activates the TRIF-TRAF pathway in a MyD88 independent manner, which leads to IFN-β secretion (94, 101, 103).
Cathelicidins Promote Nucleic Acid Stability and Endocytosis
Cathelicidins have been found to play an important role in improving the detection of nucleic acids by cells. First of all, the positively charged cathelicidins can directly interact with the negatively charged DNA or RNA through ionic interactions, which protects it from degradation by DNAses and RNAses that are abundantly present in the extracellular environment (48, 49, 98, 104, 105). Through this interaction, cathelicidins can stabilize DNA and RNA released from damaged or dying cells as well as DNA released by bacteria, for instance during the process of biofilm formation. Once nucleic acids are bound by cathelicidins and protected from degradation, cathelicidins can assist in improving the uptake of DNA by different cell types, such as macrophages, dendritic cells and B cells (48, 49, 106, 107). While this increase in uptake could theoretically be the result of increased DNA stability, increased uptake has also been observed in the context of short DNA oligos with a phosphorothioate backbone, which are resistant to DNAse degradation due to a sulfur group replacing an oxygen group on the DNA-backbone (48, 107). Furthermore, it has been shown that the positively charged peptide can act as a bridge between the nucleic acids and proteoglycans on the cell surface, which appears to be involved in the lipid-raft-mediated uptake of these cathelicidin-nucleic acid complexes (105) (Figure 2A).
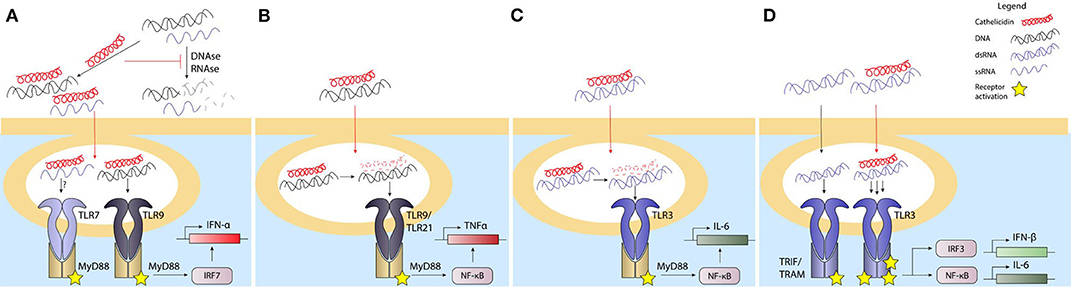
Figure 2. Cathelicidins influence the response to nucleic acids depending on the cell type. Extracellular nucleotides are an inflammatory signal through various pathways. Phagocytosis or endocytosis of DNA and/or RNA is required for their delivery to endosomal compartments leads to activation of TLR3 by dsRNA, TLR7/8 by ssRNA, and TLR9 by bacterial or self-DNA. Activation of the TLR response leads to activation of the NFkB and/or IRF transcription factors, an subsequently to inflammatory cytokine production. Extracellular DNAses and RNAses degrade the nucleotides, which prevents uptake by phagocytic cells and reduces TLR activation in the endosomal compartements. Cathelicidins can bind to extracellular DNA/RNA to stabilize it and prevent it from degradation by DNAses or RNAses. The uptake of DNA/RNA-cathelicidin complexes is enhanced and this complex formation enhances TLR9 and possibly TLR7 activation in pDCs (A). The uptake of DNA-cathelicidin complexes by macrophages (B) or RNA-cathelicidin-complexes by bronchial epithelial cells (C) is enhanced as well, but the cathelicidin is degraded in order to DNA or RNA to activate their respective TLRs. In keratinocytes, the dsRNA-cathelicidin complex is transported to the endosome and enhances the TLR3 stimulation (D). Black lines: normal signaling or route; red lines: effects exerted by cathelicidins.
The ability of cathelicidins to increase DNA stability and enhance DNA internalization is also important during NETosis, the process by which neutrophils undergo cell death by expelling their DNA into a neutrophil extracellular trap (NET) to trap invading microbes. LL-37-DNA complexes for instance, are formed within NETs produced by neutrophils that are infected by mycobacteria. These LL-37-covered NETs, still containing mycobacteria, are more efficiently internalized by macrophages, which allows the macrophages to kill the mycobacteria in lysosomal compartments in an LL-37-dependent manner (108). Cathelicidins are also commonly found within NETs under other circumstances (104, 108, 109), where they can both contribute to the antimicrobial activity of the NET, as well as prevent the NETs from being degraded by bacterial nucleases (104).
Cathelicidins Influence TLR Responses To Nucleic Acids Depending on the Cell Type
Plasmacytoid Dendritic Cells (pDCs)
pDCs play an important role in many inflammatory processes, which include wound healing, antiviral and antibacterial responses, but also autoinflammatory responses (49, 110–113). Within all these processes, their main role is the production of high quantities of IFN-α, which is produced upon activation of the endosomally located TLR7 by ssRNA or TLR9 by DNA (113). pDCs were the first cell type in which LL-37 was shown to enhance DNA-induced IFN-α responses in a TLR9-dependent manner (49). Shortly after, a similar finding was done in the context of ssRNA-LL-37-complexes, which enhanced IFN-α production in pDCs in a TLR7-dependent manner (98). While both these processes depend on the stabilization of nucleic acids by LL-37 to allow endocytosis, it was recently shown that the structural organization of DNA-LL-37-complexes is another important step in TLR9 activation. Schmidt et al. showed that DNA, when bound to LL-37, forms columnar complexes where the spacing between the DNA strands is related to the structure of the LL-37 molecules. This spacing created by LL-37 is crucial, as it approximates the width of the TLR9 ectodomain (114, 115). This ensures that the TLR9 molecules bound to the DNA do not interfere with each other, as the LL-37-mediated spacing leaves enough room for binding of other TLR9 molecules to parallel DNA strands. Furthermore, it was shown that the outside of the TLR9 ectodomain, which is not in contact with the DNA bound in the binding pocket, can interact with an adjacent DNA strand, which improves the binding affinity of the whole DNA-complex (114). Overall, the compactness of the DNA-induced by the binding with LL-37 provides an optimal spatial arrangement for the DNA to bind a high number of TLR9 receptors, which boosts the downstream signaling and IFN-α release (Figure 2B). However, similar to the indirect effects of cathelicidins on TLR4 activation, LL-37 could also play a more indirect role in TLR activation in pDCs. In these cells, autophagy is needed to deliver viral TLR7 ligands to compartments containing TLRs in order to induce an antiviral response (116). While LL-37 has not yet been shown to directly activate autophagy in pDCs, it has been shown to boost autophagy in other phagocytic cell types. Vitamin D3 for instance, a potent LL-37 inducer, triggered autophagy in human macrophages in an LL-37-dependent manner by downregulation of the PI3K/Akt/mTOR pathway (117–119), which improved the intracellular killing of Mycobacterium tuberculosis (119).
Macrophages, Conventional Dendritic Cells and B Cells
Besides pDCs, other cell types, such as macrophages (120, 121), conventional DCs (cDCs) (122, 123) and B cells (124–126), express nucleic acid recognizing TLRs. However, in contrast to pDCs, these cells do not produce IFN-α upon nucleic acid sensing, but mostly signal through MyD88-dependent proinflammatory signaling pathways, mainly involving MAPK and NF-κB (126, 127). B cells show an increased TLR9 activation upon stimulation with DNA-LL-37-complexes, which results in enhanced surface expression of activation markers CD40 and CD86, as well as increased production of IL-6 and IgG (107, 128). In human cDCs, which express TLR8 but not TLR9, ssRNA-LL-37 complexes increase the surface expression of CD80 and CD86 activation markers as well as production of IL-6 and TNF. Interestingly, IFN-α, derived from pDCs activated by ssRNA-LL-37-complexes, can further enhance the activation of cDCs by these same complexes (98).
Similar to B cells, macrophages express TLR9 in endosomal compartments and induce a proinflammatory response that includes TNF production upon DNA detection. However, where LL-37 was shown to improve responses in B cells, it has a very limited ability to enhance activation of macrophages toward DNA when tested in murine RAW264.7 macrophages. Alternatively, cathelicidins from several other species, including equine CATH-2, chicken CATH-2 and porcine PR-39, but not murine CRAMP, were able to enhance TNF responses in these cells (27). Importantly, while TLR9 activation in pDCs was shown to be dependent on the sustained complex formation between DNA and LL-37 (114), chicken macrophage activation by chicken CATH-2-DNA complexes depends on the actual release of cathelicidin from the DNA within the endosomal compartment (48). This release was the result of peptide degradation due to endosomal proteases and was a requirement for TLR21 activation. Interestingly, while exogenous CRAMP appears unable to enhance DNA-induced macrophage responses, endogenous CRAMP expression improves DNA-induced macrophage activation by upregulating TNF, IL-6 and IL-12p40 production, likely due to direct interaction between DNA, CRAMP and the endosomal TLR9 (129) (Figure 2C). While macrophage responses toward RNA-LL-37 complexes are less well-studied, some studies show that stimulation of RAW264.7 cells or alveolar macrophages with RNA-LL-37 complexes results in reduced IL-6 expression, which indicates that this complex formation actually has an inhibitory effect on the activation of TLR3 in these cells (130–132).
Keratinocytes and Epithelial Cells
Keratinocytes are crucial in the protection against skin infections and are a major source of LL-37 during both infections as well as wound-healing processes (133–136). The secretion of LL-37 by these cells strongly contributes to direct antimicrobial activity in the skin and enhances bacterial internalization by the keratinocytes (133). Besides acting as an antimicrobial factor, LL-37 can also influence nucleic acid detection by keratinocytes in several ways. First of all, whereas keratinocytes normally only express low levels of TLR9, LL-37 strongly induces the expression of this TLR in a dose-dependent manner, thereby increasing the capacity of keratinocytes to respond to endocytosed DNA (137–139). Secondly, expression of LL-37 during infection or skin damage gives LL-37 the opportunity to interact with host-DNA or -RNA released from the damaged tissue and influence the cellular uptake of these nucleic acids. Interestingly, while keratinocytes have an increased DNA uptake when DNA is complexed with LL-37, this DNA does not end up in endosomes, but in the cytoplasm. This alternative localization could be the reason why TLR9 cannot be activated by LL-37-DNA-complexes in keratinocytes, while consecutive stimulation with LL-37 and DNA does lead to a strong type I IFN response, possibly due to the increased expression of TLR9 induced by LL-37 (139). Nevertheless, while LL-37 promotes cytoplasmic uptake of DNA and many cytoplasmic nucleic acid receptors exist, such as the inflammasome-activating DNA receptor AIM2, the cytoplasmic localization of LL-37-DNA-complexes does not lead to activation of AIM2 nor does it activate the inflammasome-mediated release of IL-1β. The lack of AIM2 activation could potentially be caused by steric hindrance by LL-37, which might prevent the binding of self-DNA to AIM2 (140, 141) and thereby could play a role in the prevention of autoimmunity. While the studies mentioned here have mostly focused on the interaction between LL-37 and host-DNA, the upregulation of TLR9 expression could of course also influence the detection of bacterial DNA released passively by dying bacteria or actively during the programmed cell death during bacterial biofilm formation (142, 143). In contrast to LL-37-DNA complexes, complexes of LL-37 with dsRNA are in fact capable of reaching endosomal compartments in both human epidermal keratinocytes and human bronchial epithelial cells, which results in the activation of TLR3 (130, 131, 144, 145). TLR3 activation by RNA-LL-37-complexes depends on different processes in both cell types. In keratinocytes, complex formation between LL-37 and the dsRNA provides an RNA structure where the intercalating LL-37 provides optimal spacing between RNA molecules to bind a higher number of TLR3 molecules per RNA molecule, enhancing IFN-β and IL-6 production, with a similar mechanism as LL-37-DNA-mediated activation of TLR9 in pDCs. Human bronchial epithelial cells on the other hand require the dissociation of LL-37 from the LL-37-RNA-complex to activate TLR3, which is caused by a decrease in pH and protease activation in endolysosomal compartments. Degradation of LL-37 then allows the RNA to bind to the TLR3 receptor, which is reminiscent of the mechanism by which TLR21 is activated by CATH-2-DNA complexes in chicken macrophages (130, 131) (Figure 2D). Together, all these studies demonstrate both the complexity by which cathelicidins can influence nucleic acid-sensing as well as the different requirements that TLR activation by RNA/DNA-cathelicidin-complexes has depending on the cell type and species investigated.
Limited Effects of Cathelicidins on TLR5 Activation
While the effects of cathelicidins on the previously described TLRs are well-studied, the influence of cathelicidins on TLR5 activation remains less well-understood. TLR5 detects the conserved flagellin protein present in the flagella of Gram-negative bacteria and its activation leads to pro-inflammatory cytokine production via MyD88- and NF-κB-signaling pathways (56). Some studies have shown that LL-37 enhances the flagellin-induced IL-8 secretion by adult human keratinocytes (93, 146), which was depended on P2X7 receptor signaling and Scr/Akt pathway activation (146). In human bronchial epithelial cells, co-incubation of LL-37 and flagellin resulted in an increased IL-8 and IL-6 secretion (93), regulated via the PI3K/p38 pathway (147). On the other hand, LL-37 had no or a slightly inhibitory effect on the flagellin activation in human dendritic cells (91), macrophages (81), PBMCs (68, 87), or TLR5-tranfected HEK cells (77) (Figure 1). This shows that the influence of cathelicidins on TLR5 is highly dependent on the cell type, although more research is required to understand the mechanisms underlying these differences.
Cathelicidins Activate The Inflammasome Via P2X7
Another innate immune mechanism involved in sensing microbe- or damage-related signals involves the inflammasome. The formation of the inflammasome complex can be triggered by a diverse set of environmental stimuli, including ATP, cytokines and TLR ligands, and might be affected by cathelicidins as well. Inflammasome activation and signaling results in the conversion of pro-IL-1β and pro-IL-18 into mature IL-1β and IL-18. ATP is one of most common ligands studied in this respect, triggering the inflammasome formation via the P2X7 receptor, leading to the activation of caspase-1 and thereby the cleavage of pro-IL-1β to active IL-1β (148). However, this P2X7-mediated inflammasome activation can also be induced by other ligands, such as LL-37. This has for instance been shown by LL-37 treatment of LPS-activated monocytes or stimulation of macrophages with both LL-37 and P. aeruginosa, which in both cases leads to P2X7-dependent IL-1β release (149, 150). In addition, NET-associated LL-37 has been found to activate caspase-1 in a P2X7 receptor dependent fashion in macrophages, leading to IL-1β and IL-18 release (151), which provides yet another function for LL-37 in NETs.
The Influence of Cathelicidins on TLR Activation in Health and Disease
Cathelicidin-Mediated TLR Regulation Balances the Inflammatory Response to Bacterial Infection
Cathelicidin-Mediated TLR Regulation in vitro
Cathelicidins are capable of reducing the inflammatory response of the immune system by inhibiting LPS- or LTA-induced TLR activation. This reduction is dependent on the direct interaction between cathelicidins and these lipid-containing molecules. Until recently, these effects were mostly studied in the context of purified TLR ligands and little was known about how cathelicidins affect TLR signaling in the context of a complete bacterium. However, some recent studies are now shedding some light on how cathelicidins balance inflammation in the context of whole bacterial cells. For instance, human adenocarcinoma colonic epithelial cells produce higher amounts of LL-37 upon activation, which prevents internalization of the enteric pathogenic Gram-negative bacterium S. typhimurium. Alternatively, knockdown of LL-37 increases Salmonella invasion in enterocytes and allows for more efficient immune evasion by these bacteria due to lower TLR4 expression and a reduced IL-1β response (152). While reducing the invasion and internalization of Salmonella in colonic epithelial cells, LL-37 enhances the clearance of Mycobacterium avium subsp. paratuberculosis (MAP), a bacterium causing chronic diarrheic intestinal infections in domestic and wild ruminants, by increasing bacterial uptake in murine macrophages. In addition, macrophage treatment with LL-37 suppresses TLR2 upregulation and thereby the production of tissue-damaging inflammatory cytokines released during MAP infection (153), as well as during A. fumigatus infection (154).
Besides the ability of cathelicidins to regulate TLR expression they can also influence bacterial-induced TLR activation through direct interaction with these bacteria. Importantly, the ability of cathelicidins to regulate bacterial-induced TLR activation directly is highly dependent on bacterial viability. For instance, when cathelicidins such as human LL-37, chicken CATH-2 or porcine PMAP-36 are co-incubated with heat-inactivated E. coli or P. aeruginosa, they strongly reduce macrophage responses against these bacteria by blocking TLR2 and TLR4 activation through direct interaction with the lipoproteins and LPS normally activating these TLRs. However, when these cathelicidins are co-incubated with live E. coli or P. aeruginosa, no inhibition is observed as long as these peptides remain below bactericidal concentrations. Importantly, when instead bactericidal concentrations are used, inhibition of macrophage activation can be observed again. Alternatively, using cathelicidins that lack antimicrobial activity, but possess LPS-neutralizing activity, such as the canine K9CATH, it was shown that these peptides can in fact reduce macrophage activation in the context of killed bacteria, but not in the context of viable bacteria (73, 77, 92). This viability-dependent regulation of TLR activation provides an elegant way for the host to respond to bacterial molecules only when needed. At the start of an infection, activation of the immune system leads to the production and release of cathelicidins and cytokines from both macrophages and neutrophils at the site of infection. These cathelicidins will target and fight the bacteria to reduce the bacterial burden at the site of infection. During this phase, cathelicidins will only be able to neutralize the LPS- and lipoprotein-induced inflammatory responses against already killed bacteria, while still allowing a response against any remaining viable bacteria. This leads to a balancing act, where a reduction or increase of viable bacteria, i.e., a reduced or increased threat, also leads to a corresponding reduced or enhanced inflammatory response. Therefore, this cathelicidin-mediated regulation based on bacterial viability could be an important factor in maintaining a proportional inflammatory response based on the present bacterial threat and thereby limiting excessive inflammation which can lead to unwanted tissue damage (Figure 3).
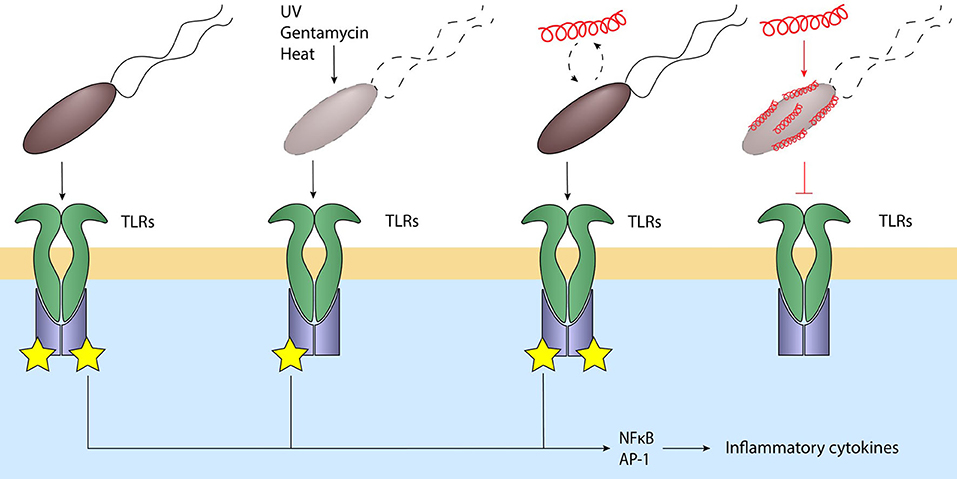
Figure 3. Cathelicidins balance the immune response to bacteria. Live bacteria activate various TLRs through their MAMPs, leading to NFkB and AP-1 activation and inflammatory signaling. Bacteria killed by for example UV, heat or gentamycin still activate these TLRs. Addition of cathelicidins to viable bacteria does not inhibit TLR activation; however, cathelicidin-killed bacteria or addition of cathelicidins to non-viable bacteria strongly inhibits TLR activation. This silent killing reduces the inflammatory response and thereby the subsequent inflammatory tissue damage when the bacteria are already killed and are no longer a threat. Black lines: normal signaling; red lines: effects exerted by cathelicidins.
Cathelicidin-Mediated TLR Modulation in vivo
While the strong antimicrobial potential of cathelicidins has driven the in vivo testing of these peptides, many studies have also investigated how these peptides influence immune activation during infection. A common tool for testing the effects of cathelicidins on infection and inflammation in vivo is the clnp-null mouse model, which lacks the expression of the only murine cathelicidin CRAMP. Using this model, it has been shown that mice lacking cathelicidin expression were more prone to necrotic skin infection caused by Group A Streptococcus (155), P. aeruginosa infection of the cornea (50), S. aureus-induced endophthalmitis (156), cecal-ligation and puncture-induced sepsis (157), dextran sulfate sodium (DSS)-induced colitis (158), K. pneumoniae-induced lung infection (159), caerulein-induced experimental acute pancreatitis (160) and meningitis-induced by intracerebral injection S. pneumoniae (161, 162). In general, these CRAMP-deficient mice suffered from a higher bacterial load, increased proinflammatory cytokine production and increased tissue damage. In addition, two studies also reported an increased influx of neutrophils in clnp-null mice (50, 162). These findings indicate the importance of cathelicidins not only in reducing the pathogenic burden during infections, but also their importance in limiting inflammation. Similarly, alternative models relying on exogenous treatment with cathelicidins, or transgenic overexpression of LL-37, show comparable results. For instance, intravenous administration of LL-37 in a cecal-ligation and puncture-induced sepsis mouse model improved the survival of these mice and reduced the bacterial load in the blood and peritoneum. In addition, LL-37 reduced the levels of several proinflammatory cytokines in the peritoneal fluids as well as in the serum, despite causing an increase in NET formation by neutrophils (163, 164). In a similar fashion, intratracheal treatment of A. fumigatus-instilled mice with exogenous LL-37, as well as transgenic overexpression of LL-37, causes enhanced fungal clearance, reduced lung damage and less proinflammatory cytokine production (154). Taken together, all these studies show a potent antimicrobial and anti-inflammatory role for cathelicidins during both bacterial and fungal infections. However, despite the cathelicidin-related anti-inflammatory effect seen in many of these studies, it remains difficult to distinguish if these anti-inflammatory effects can be partially caused by direct TLR inhibition or by alternative immunomodulation or that the effects can be explained by a lower bacterial burden due to the antimicrobial activity of these cathelicidins.
In an effort to separate these effects, several studies have now used different types of inactivated bacteria to investigate the direct anti-inflammatory role of cathelicidins in vivo. For instance, inflammatory responses induced by intratracheal administration of heat-inactivated P. aeruginosa or S. aureus isolates in mice can be inhibited by co-administration or post-administration of chicken CATH-2 (73, 165). In addition, intratracheal administration of chicken CATH-2-killed P. aeruginosa induces less neutrophil influx and inflammatory cytokine production compared to either administration of heat-killed or gentamicin-killed P. aeruginosa (73). These findings indicate that it is likely that the reduction in inflammatory markers observed upon treatment of a bacterial infection with cathelicidins, is not only the result of a reduced bacterial burden due to antimicrobial activity but is indeed also affected by direct inhibition of immune activation by cathelicidins.
Nucleic Acid-Cathelicidin Complexes Can Lead to Autoimmune Disease
Cathelicidins have been extensively described for various protective functions that are beneficial for host survival. These functions include their direct antimicrobial activity against Gram-positive and Gram-negative bacteria (27, 74, 166), inhibition of viral replication (132) or direct antiviral activity (167), promotion of wound healing (110) as well as their ability to modulate immune responses (15, 37, 71), which has been shown to protect against excessive inflammation (77). However, their ability to improve nucleic acid detection may also lead to the onset of various autoimmune diseases.
The pathways leading to excessive inflammation in autoimmune diseases are often complex and involve numerous cell types. For SLE, psoriasis and diabetes, these processes most likely start with some type of tissue damage that initiates TLR signaling and autoimmune inflammation (49, 54, 168). In diabetes, cell death of the insulin producing β-cells of the pancreas initiates a cascade that leads to more cell death and subsequently more inflammation (54). In SLE and psoriasis, anti-DNA and -RNA antibodies can be found that most likely are the result of DNA released upon tissue damage and play an important role in the exacerbation of these diseases (168, 169). In 2007, Lande et al. were first to link these processes to cathelicidins by describing how LL-37 enhances DNA-induced inflammation in psoriatic skin lesions (49). In these lesions, the release of DNA and RNA from damaged tissue binds LL-37 (49, 98), which is expressed at extremely high concentrations (up to 300 μM) by either keratinocytes or neutrophils under these conditions (26). These complexes then stimulate pDC-derived IFN-α production that subsequently drives the activation of cDCs and T cells, which in turn exacerbate the tissue damage (98). In addition, enhanced activation of B cells by DNA-LL-37 complexes also increases the production of anti-DNA antibodies (128). In diabetes, the complex formation of these anti-DNA antibodies with DNA and CRAMP triggers pDC activation, which again leads to high IFN-α production. These high levels of IFN-α in turn increase T cell activation and thereby autoreactivity against pancreatic β-cells (54). Other complexes that can increase auto-inflammation include RNA-LL-37 complexes. These trigger TLR8-mediated cytokine production and can induce neutrophil NETosis in psoriatic skin (170). Similarly, anti-RNA immunocomplexes were shown to activate neutrophil NETosis in SLE, which results in the release of additional NET-derived DNA into the extracellular environment (168). Importantly, as these NETs are coated with both LL-37 and anti-DNA antibodies, they can serve as new ligands for pDC activation and thus IFN-α production, which leads to a further exacerbation of the inflammatory response (169).
Cathelicidins as Adjuvant for Vaccination
Vaccination strategies aim to induce a modest immune response against one or more specific pathogens, in order for a host to be able to respond with a humoral response toward such pathogens when they are encountered later in life. To induce such a specific immune response, vaccination therapies require one or more antigens in the form of whole live, inactivated or attenuated viruses or bacteria, or alternatively, specific microbial components, such as outer membrane vesicles or specific viral or bacterial proteins. However, as not all antigens can stimulate the immune system sufficiently to build an immune memory, adjuvants, including several TLR agonists, are commonly used to improve the strength of the immune response during vaccination. Since cathelicidins can modulate these TLR responses, their possible role as adjuvant during vaccinations has been investigated in various studies. For instance, intranasal vaccination with attenuated pseudorabies virus (PRV), complexed with CpG-DNA and a bactenecin-derived innate defense regulator (IDR) peptide, resulted in an enhanced protection of piglets (171). Similarly, the combination of an IDR peptide and CpG-DNA as adjuvants for a pertussis toxoid vaccine improved in vitro DC maturation, cytokine production and expression of surface activation markers, while also enhancing in vivo antigen presentation and specific IgG1 and IgG2a antibody titers (172, 173). While the mechanisms behind these improved responses are hard to discern, improvement of DNA-induced TLR9 responses by these peptides could very well play a role in this. On the other hand, cathelicidins have also been shown to improve vaccination responses toward various viral pathogens in the absence of CpG-DNA as an adjuvant. Intramuscular or intranasal administration of piglets with inactivated porcine reproductive and respiratory syndrome virus (PRRSV) microparticles complexed with an IDR peptide or LL-37 enhanced the response toward the antigens in vitro; however, in vivo only little improvement in vaccination efficacy was observed (132). In addition, intranasal vaccination of mice using a nanoparticle-based vaccine for an H1N1-ovalbumin influenza virus, also benefits from IDR peptides as adjuvant, which together with c-di-AMP, induced a strong humoral and cellular immune response (174). Furthermore, subcutaneous vaccination of mice with the HPV E7 epitope of human papillomavirus (HPV) using CRAMP as adjuvant, reduced HPV-induced tumor growth (175). Finally, besides their effect on anti-viral vaccinations, IDR peptides were also shown to improve vaccination efficiency against other types of pathogens. This includes intravenous administration of an IDR peptide as an adjunctive therapy for an oral administered anti-malarial therapy, which strongly enhanced the protection against late-stage malarial infection in mice (176). Alternatively, using an IDR peptide as adjuvant resulted in a balanced increase in IgG1 and IgG2a antibody titers upon subcutaneous vaccination of beef calves, using a mix of Mycoplasma bovis subunits and IDR peptide. However, in this last study it is unclear whether this is due to the addition of the IDR peptide, since a control without IDR peptide is missing (177). Together, these results indicate the potential usefulness of cathelicidins and other similar host defense peptides in vaccination therapies; however, more detailed studies will be required to discriminate the contribution of direct immunomodulatory activities from the TLR-modulatory activities of these peptides in such vaccination therapies.
Cathelicidins as Anticancer Therapy
Besides their role in inflammation, both cathelicidins and TLRs play an important role in the development and progression of cancer. TLR2 activation for instance, has been suggested as a possible therapeutic target, with local administration of a TLR2/6 agonist resulting in reduced tumor growth and prolonged survival in a pancreatic carcinoma mouse model (178). Alternatively, TLR9 activation by CpG-ODN has been shown to reduce metastasis and improve survival in pancreatic cancer (179) and neuroblastoma mouse models (180). As LL-37 has been shown to improve DNA-induced TLR9 activation, it might not be surprising that co-administration of CpG-ODN and LL-37 enhanced survival in a mouse ovarian-tumor model compared to CpG-ODN alone and that the LL-37-CpG-ODN combination enhanced the activation and proliferation of NK-cells, but not of T cells or macrophages, in the peritoneal cavity (181). On the other hand, inhibition of LPS-induced TLR4 activation reduces the migration and invasion capacity of the SW480 cancer cell line (182) and reduces pancreatic tumorigenesis in mice (183). While not specifically tested with cathelicidins, peptides such as LL-37 could also provide efficient inhibition of TLR4 and could thereby be of therapeutic value in tumors which have strongly enhanced TLR4 expression, for example numerous ovarian epithelial cancers (184). Furthermore, cathelicidins can also have beneficial anti-cancer effects in the absence of specific TLR ligands. In gastric or colon tumors, where the expression of LL-37 is reduced (185), treatment with LL-37 activates caspase-independent apoptosis and reduces tumor progression (186). Additionally, the application of CRAMP as adjuvant for HPV vaccination reduces tumor growth, albeit no direct anti-tumor effects of CRAMP were observed when used to treat HPV-induced tumors (175).
Nevertheless, overexpression of LL-37 has also been linked to increased tumor growth, enhanced invasiveness and bad prognosis in malignant melanomas and ovarian, lung, prostate and breast cancers, by stimulating the growth receptors of the EGFR and ERB-family (187). In addition, increased TLR expression in ovarian and pancreatic cancers is associated with poor clinical outcome (188, 189). Together, this shows that cathelicidins, either directly or through modulation of TLR activation, can be useful in the development of novel anti-cancer therapies, but that the potential negative effects of these peptides should not be overlooked. In this respect, modification of synthetic cathelicidin-like peptides could provide a solution to these issues, by structurally improving the peptide to increase desirable effects while at the same time limiting unwanted side effects.
Conclusion and Outlook
The fact that cathelicidins are a highly conserved part of the innate immune system in vertebrates, together with their apparent multifunctional nature, has led to a large interest in these peptides across various disciplines, including e.g., microbiology, immunology, oncology and dermatology. This multidisciplinary approach has uncovered a wide variety of effects that these peptides can exert. However, the potency of these different effects can vary strongly. Among their immunoregulatory functions, the regulation of TLR activation can be counted among their more potent functions, with cathelicidins being able to strongly reduce LPS- or LTA- induced TLR activation and almost completely inhibiting TLR-mediated inflammatory responses when they kill bacteria. On the other hand, DNA can be transformed from a quickly degradable extracellular factor into a very potent inflammatory signal in the context of various auto-immune diseases. While direct interaction between cathelicidins and TLR ligands in most of these cases plays an important role in regulating TLR activation, more research is required to uncover the more complex direct effects of cathelicidins on signaling pathways such as the induction of autophagy. Autophagy can play a role in an enormous set of different intracellular pathways. Activation of autophagy by cathelicidins could also have a major impact on TLR signaling besides those effects mentioned in this review. Importantly, while cathelicidins are conserved among vertebrates, the amino acid composition and 3D structure of the mature peptides vary a lot. This means that one should be careful to extrapolate findings between different cathelicidins without supporting laboratory evidence.
Finally, the literature discussed in this review shows that cathelicidins could have strong prophylactic and therapeutic value aside from their antimicrobial activities. This includes dampening inflammation when treating infections to prevent sepsis as well as improving vaccine responses through an improved immune response against target antigens. In addition, it has been shown using structurally modified cathelicidins that certain functions can be enhanced or, alternatively, be limited. Thus, cathelicidin derivatives can be designed with specific therapeutic properties while limiting any unwanted side effects. Overall, it may be concluded that, despite the fact that cathelicidins have been discovered nearly 30 years ago, the elucidation of new properties and functions in recent years continues to provide more insight in the physiological roles and potential applications of cathelicidins.
Author Contributions
MS and MC have written the review. RH designed the graphics. HH, EV, and RH, carefully read and corrected the text and wrote paragraphs of their expertise.
Funding
This research was funded by an NWO-TTW Perspectief grant [Grant No. 14924].
Conflict of Interest
The authors declare that the research was conducted in the absence of any commercial or financial relationships that could be construed as a potential conflict of interest.
References
1. Fraenkel GJ. Penicillin at the beginning. Ann Diagn Pathol. 2:422–4. doi: 10.1016/S1092-9134(98)80043-9
2. Tacconelli E, Carrara E, Savoldi A, Harbarth S, Mendelson M, Monnet DL, et al. Discovery, research, and development of new antibiotics: the WHO priority list of antibiotic-resistant bacteria and tuberculosis. Lancet Infect Dis. (2018) 18:318–27. doi: 10.1016/S1473-3099(17)30753-3
3. Mookherjee N, Anderson MA, Haagsman HP, Davidson DJ. Antimicrobial host defence peptides: functions and clinical potential. Nat Rev Drug Discov. (2020) 19:311–332. doi: 10.1038/s41573-019-0058-8
4. Takeda K, Kaisho T, Akira S. Toll-like receptors. Annu Rev Immunol. (2003) 21:335–76. doi: 10.1146/annurev.immunol.21.120601.141126
5. Zanetti M. Cathelicidins, multifunctional peptides of the innate immunity. J Leukoc Biol. (2004) 75:39–48. doi: 10.1189/jlb.0403147
6. Zaiou M, Nizet V, Gallo RL. Antimicrobial and protease inhibitory functions of the human cathelicidin (hCAP18/LL-37) prosequence. J Invest Dermatol. (2003) 120:810–6. doi: 10.1046/j.1523-1747.2003.12132.x
7. Yamasaki K, Schauber J, Coda A, Lin H, Dorschner RA, Schechter NM, et al. Kallikrein-mediated proteolysis regulates the antimicrobial effects of cathelicidins in skin. FASEB J. (2006) 20:2068–80. doi: 10.1096/fj.06-6075com
8. Zasloff M. Antimicrobial peptides in health and disease. N Engl J Med. (2002) 347:1199–200. doi: 10.1056/NEJMe020106
9. Durr UH, Sudheendra US, Ramamoorthy A. LL-37, the only human member of the cathelicidin family of antimicrobial peptides. Biochim Biophys Acta. (2006) 1758:1408–25. doi: 10.1016/j.bbamem.2006.03.030
10. Kosciuczuk EM, Lisowski P, Jarczak J, Strzalkowska N, Jozwik A, Horbanczuk J, et al. Cathelicidins: family of antimicrobial peptides. A review. Mol Biol Rep. (2012) 39:10957–70. doi: 10.1007/s11033-012-1997-x
11. Zhang LJ, Gallo RL. Antimicrobial peptides. Curr Biol. (2016) 26:R14–9. doi: 10.1016/j.cub.2015.11.017
12. Sorensen O, Cowland JB, Askaa J, Borregaard N. An ELISA for hCAP-18, the cathelicidin present in human neutrophils and plasma. J Immunol Methods. (1997) 206:53–9. doi: 10.1016/S0022-1759(97)00084-7
13. van Dijk A, Tersteeg-Zijderveld MH, Tjeerdsma-van Bokhoven JL, Jansman AJ, Veldhuizen EJA, Haagsman HP. Chicken heterophils are recruited to the site of Salmonella infection and release antibacterial mature cathelicidin-2 upon stimulation with LPS. Mol Immunol. (2009) 46:1517–26. doi: 10.1016/j.molimm.2008.12.015
14. Wan M, Sabirsh A, Wetterholm A, Agerberth B, Haeggstrom JZ. Leukotriene B4 triggers release of the cathelicidin LL-37 from human neutrophils: novel lipid-peptide interactions in innate immune responses. FASEB J. (2007) 21:2897–905. doi: 10.1096/fj.06-7974com
15. van Harten RM, van Woudenbergh E, van Dijk A, Haagsman HP. Cathelicidins: immunomodulatory antimicrobials. Vaccines. (2018) 6:63. doi: 10.3390/vaccines6030063
16. Bals R, Wang X, Zasloff M, Wilson JM. The peptide antibiotic LL-37/hCAP-18 is expressed in epithelia of the human lung where it has broad antimicrobial activity at the airway surface. Proc Natl Acad Sci USA. (1998) 95:9541–6. doi: 10.1073/pnas.95.16.9541
17. Schauber J, Svanholm C, Termen S, Iffland K, Menzel T, Scheppach W, et al. Expression of the cathelicidin LL-37 is modulated by short chain fatty acids in colonocytes: relevance of signalling pathways. Gut. (2003) 52:735–41. doi: 10.1136/gut.52.5.735
18. Byfield FJ, Wen Q, Leszczynska K, Kulakowska A, Namiot Z, Janmey PA, et al. Cathelicidin LL-37 peptide regulates endothelial cell stiffness and endothelial barrier permeability. Am J Physiol Cell Physiol. (2011) 300:C105–12. doi: 10.1152/ajpcell.00158.2010
19. Lopez-Garcia B, Lee PH, Yamasaki K, Gallo RL. Anti-fungal activity of cathelicidins and their potential role in Candida Albicans skin infection. J Invest Dermatol. (2005) 125:108–15. doi: 10.1111/j.0022-202X.2005.23713.x
20. Horibe K, Nakamichi Y, Uehara S, Nakamura M, Koide M, Kobayashi Y, et al. Roles of cathelicidin-related antimicrobial peptide in murine osteoclastogenesis. Immunology. (2013) 140:344–51. doi: 10.1111/imm.12146
21. Ruan Y, Shen T, Wang Y, Hou M, Li J, Sun T. Antimicrobial peptide LL-37 attenuates LTA induced inflammatory effect in macrophages. Int Immunopharmacol. (2013) 15:575–80. doi: 10.1016/j.intimp.2013.01.012
22. Kulkarni NN, Yi Z, Huehnken C, Agerberth B, Gudmundsson GH. Phenylbutyrate induces cathelicidin expression via the vitamin D receptor: linkage to inflammatory and growth factor cytokines pathways. Mol Immunol. (2015) 63:530–9. doi: 10.1016/j.molimm.2014.10.007
23. Mily A, Rekha RS, Kamal SM, Arifuzzaman AS, Rahim Z, Khan L, et al. Significant effects of oral phenylbutyrate and vitamin D3 adjunctive therapy in pulmonary tuberculosis: a randomized controlled trial. PLoS ONE. (2015) 10:e0138340. doi: 10.1371/journal.pone.0138340
24. Marin M, Holani R, Shah CB, Odeon A, Cobo ER. Cathelicidin modulates synthesis of Toll-like receptors (TLRs) 4 and 9 in colonic epithelium. Mol Immunol. (2017) 91:249–58. doi: 10.1016/j.molimm.2017.09.011
25. Tada H, Shimizu T, Nagaoka I, Takada H. Vitamin D3 analog maxacalcitol (OCT) induces hCAP-18/LL-37 production in human oral epithelial cells. Biomed Res. (2016) 37:199–205. doi: 10.2220/biomedres.37.199
26. Ong PY, Ohtake T, Brandt C, Strickland I, Boguniewicz M, Ganz T, et al. Endogenous antimicrobial peptides and skin infections in atopic dermatitis. N Engl J Med. (2002) 347:1151–60. doi: 10.1056/NEJMoa021481
27. Coorens M, Scheenstra MR, Veldhuizen EJA, Haagsman HP. Interspecies cathelicidin comparison reveals divergence in antimicrobial activity, TLR modulation, chemokine induction and regulation of phagocytosis. Sci Rep. (2017) 7:40874. doi: 10.1038/srep40874
28. Arzese A, Skerlavaj B, Tomasinsig L, Gennaro R, Zanetti M. Antimicrobial activity of SMAP-29 against the Bacteroides fragilis group and clostridia. J Antimicrob Chemother. (2003) 52:375–81. doi: 10.1093/jac/dkg372
29. Benincasa M, Skerlavaj B, Gennaro R, Pellegrini A, Zanetti M. In vitro and in vivo antimicrobial activity of two alpha-helical cathelicidin peptides and of their synthetic analogs. Peptides. (2003) 24:1723–31. doi: 10.1016/j.peptides.2003.07.025
30. Currie SM, Findlay EG, McHugh BJ, Mackellar A, Man T, Macmillan D, et al. The human cathelicidin LL-37 has antiviral activity against respiratory syncytial virus. PLoS ONE. (2013) 8:e73659. doi: 10.1371/journal.pone.0073659
31. Harcourt JL, McDonald M, Svoboda P, Pohl J, Tatti K, Haynes LM. Human cathelicidin, LL-37, inhibits respiratory syncytial virus infection in polarized airway epithelial cells. BMC Res Notes. (2016) 9:11. doi: 10.1186/s13104-015-1836-y
32. Tripathi S, Tecle T, Verma A, Crouch E, White M, Hartshorn KL. The human cathelicidin LL-37 inhibits influenza A viruses through a mechanism distinct from that of surfactant protein D or defensins. J Gen Virol. (2013) 94:40–9. doi: 10.1099/vir.0.045013-0
33. Benincasa M, Scocchi M, Pacor S, Tossi A, Nobili D, Basaglia G, et al. Fungicidal activity of five cathelicidin peptides against clinically isolated yeasts. J Antimicrob Chemother. (2006) 58:950–9. doi: 10.1093/jac/dkl382
34. Ordonez SR, Amarullah IH, Wubbolts RW, Veldhuizen EJA, Haagsman HP. Fungicidal mechanisms of cathelicidins LL-37 and CATH-2 revealed by live-cell imaging. Antimicrob Agents Chemother. (2014) 58:2240–8. doi: 10.1128/AAC.01670-13
35. Cauchard S, Van Reet N, Buscher P, Goux D, Grotzinger J, Leippe M, et al. Killing of trypanozoon parasites by the equine cathelicidin eCATH1. Antimicrob Agents Chemother. (2016) 60:2610–9. doi: 10.1128/AAC.01127-15
36. Rico-Mata R, De Leon-Rodriguez LM, Avila EE. Effect of antimicrobial peptides derived from human cathelicidin LL-37 on entamoeba histolytica trophozoites. Exp Parasitol. (2013) 133:300–6. doi: 10.1016/j.exppara.2012.12.009
37. De Y, Chen Q, Schmidt AP, Anderson GM, Wang JM, Wooters J, et al. LL-37, the neutrophil granule- and epithelial cell-derived cathelicidin, utilizes formyl peptide receptor-like 1 (FPRL1) as a receptor to chemoattract human peripheral blood neutrophils, monocytes, and T cells. J Exp Med. (2000) 192:1069–74. doi: 10.1084/jem.192.7.1069
38. Tjabringa GS, Ninaber DK, Drijfhout JW, Rabe KF, Hiemstra PS. Human cathelicidin LL-37 is a chemoattractant for eosinophils and neutrophils that acts via formyl-peptide receptors. Int Arch Allergy Immunol. (2006) 140:103–12. doi: 10.1159/000092305
39. Zhang Z, Cherryholmes G, Chang F, Rose DM, Schraufstatter I, Shively JE. Evidence that cathelicidin peptide LL-37 may act as a functional ligand for CXCR2 on human neutrophils. Eur J Immunol. (2009) 39:3181–94. doi: 10.1002/eji.200939496
40. Scott MG, Davidson DJ, Gold MR, Bowdish D, Hancock RE. The human antimicrobial peptide LL-37 is a multifunctional modulator of innate immune responses. J Immunol. (2002) 169:3883–91. doi: 10.4049/jimmunol.169.7.3883
41. van der Does AM, Beekhuizen H, Ravensbergen B, Vos T, Ottenhoff TH, van Dissel JT, et al. LL-37 directs macrophage differentiation toward macrophages with a proinflammatory signature. J Immunol. (2010) 185:1442–9. doi: 10.4049/jimmunol.1000376
42. Lishko VK, Moreno B, Podolnikova NP, Ugarova TP. Identification of human cathelicidin peptide LL-37 as a ligand for macrophage integrin αMβ2 (Mac-1, CD11b/CD18) that promotes phagocytosis by opsonizing bacteria. Res Rep Biochem. (2016) 2016:39–55. doi: 10.2147/RRBC.S107070
43. Wan M, van der Does AM, Tang X, Lindbom L, Agerberth B, Haeggstrom JZ. Antimicrobial peptide LL-37 promotes bacterial phagocytosis by human macrophages. J Leukoc Biol. (2014) 95:971–81. doi: 10.1189/jlb.0513304
44. Zhang X, Bajic G, Andersen GR, Christiansen SH, Vorup-Jensen T. The cationic peptide LL-37 binds Mac-1 (CD11b/CD18) with a low dissociation rate and promotes phagocytosis. Biochim Biophys Acta. (2016) 1864:471–8. doi: 10.1016/j.bbapap.2016.02.013
45. Molhoek EM, van Dijk A, Veldhuizen EJA, Haagsman HP, Bikker FJ. Improved proteolytic stability of chicken cathelicidin-2 derived peptides by D-amino acid substitutions and cyclization. Peptides. (2011) 32:875–80. doi: 10.1016/j.peptides.2011.02.017
46. Okuda D, Yomogida S, Tamura H, Nagaoka I. Determination of the antibacterial and lipopolysaccharide-neutralizing regions of guinea pig neutrophil cathelicidin peptide CAP11. Antimicrob Agents Chemother. (2006) 50:2602–7. doi: 10.1128/AAC.00331-06
47. Baumann A, Demoulins T, Python S, Summerfield A. Porcine cathelicidins efficiently complex and deliver nucleic acids to plasmacytoid dendritic cells and can thereby mediate bacteria-induced IFN-α responses. J Immunol. (2014) 193:364–71. doi: 10.4049/jimmunol.1303219
48. Coorens M, van Dijk A, Bikker F, Veldhuizen EJA, Haagsman HP. Importance of endosomal cathelicidin degradation to enhance DNA-induced chicken macrophage activation. J Immunol. (2015) 195:3970–7. doi: 10.4049/jimmunol.1501242
49. Lande R, Gregorio J, Facchinetti V, Chatterjee B, Wang YH, Homey B, et al. Plasmacytoid dendritic cells sense self-DNA coupled with antimicrobial peptide. Nature. (2007) 449:564–9. doi: 10.1038/nature06116
50. Huang LC, Reins RY, Gallo RL, McDermott AM. Cathelicidin-deficient (Cnlp -/-) mice show increased susceptibility to Pseudomonas Aeruginosa keratitis. Invest Ophthalmol Vis Sci. (2007) 48:4498–508. doi: 10.1167/iovs.07-0274
51. Yamasaki K, Gallo RL. Rosacea as a disease of cathelicidins and skin innate immunity. J Investig Dermatol Symp Proc. (2011) 15:12–5. doi: 10.1038/jidsymp.2011.4
52. Brauner H, Luthje P, Grunler J, Ekberg NR, Dallner G, Brismar K, et al. Markers of innate immune activity in patients with type 1 and type 2 diabetes mellitus and the effect of the anti-oxidant coenzyme Q10 on inflammatory activity. Clin Exp Immunol. (2014) 177:478–82. doi: 10.1111/cei.12316
53. Schauber J, Rieger D, Weiler F, Wehkamp J, Eck M, Fellermann K, et al. Heterogeneous expression of human cathelicidin hCAP18/LL-37 in inflammatory bowel diseases. Eur J Gastroenterol Hepatol. (2006) 18:615–21. doi: 10.1097/00042737-200606000-00007
54. Diana J, Simoni Y, Furio L, Beaudoin L, Agerberth B, Barrat F, et al. Crosstalk between neutrophils, B-1a cells and plasmacytoid dendritic cells initiates autoimmune diabetes. Nat Med. (2013) 19:65–73. doi: 10.1038/nm.3042
55. Sun J, Furio L, Mecheri R, van der Does AM, Lundeberg E, Saveanu L, et al. Pancreatic β-cells limit autoimmune diabetes via an immunoregulatory antimicrobial peptide expressed under the influence of the gut microbiota. Immunity. (2015) 43:304–17. doi: 10.1016/j.immuni.2015.07.013
56. De Nardo D. Toll-like receptors: activation, signalling and transcriptional modulation. Cytokine. (2015) 74:181–9. doi: 10.1016/j.cyto.2015.02.025
57. Takeda K, Akira S. Toll-like receptors in innate immunity. Int Immunol. (2005) 17:1–14. doi: 10.1093/intimm/dxh186
58. Jin MS, Kim SE, Heo JY, Lee ME, Kim HM, Paik SG, et al. Crystal structure of the TLR1-TLR2 heterodimer induced by binding of a tri-acylated lipopeptide. Cell. (2007) 130:1071–82. doi: 10.1016/j.cell.2007.09.008
59. Kang JY, Nan X, Jin MS, Youn SJ, Ryu YH, Mah S, et al. Recognition of lipopeptide patterns by toll-like receptor 2-Toll-like receptor 6 heterodimer. Immunity. (2009) 31:873–84. doi: 10.1016/j.immuni.2009.09.018
60. Balka KR, De Nardo D. Understanding early TLR signaling through the myddosome. J Leukoc Biol. (2019) 105:339–51. doi: 10.1002/JLB.MR0318-096R
61. Aksoy E, Taboubi S, Torres D, Delbauve S, Hachani A, Whitehead MA, et al. The p110δ isoform of the kinase PI(3)K controls the subcellular compartmentalization of TLR4 signaling and protects from endotoxic shock. Nat Immunol. (2012) 13:1045–54. doi: 10.1038/ni.2426
62. Brogden KA. Antimicrobial peptides: pore formers or metabolic inhibitors in bacteria?. Nat Rev Microbiol. (2005) 3:238–50. doi: 10.1038/nrmicro1098
63. Olaitan AO, Morand S, Rolain JM. Mechanisms of polymyxin resistance: acquired and intrinsic resistance in bacteria. Front Microbiol. (2014) 5:643. doi: 10.3389/fmicb.2014.00643
64. Hirata M, Shimomura Y, Yoshida M, Morgan JG, Palings I, Wilson D, et al. Characterization of a rabbit cationic protein (CAP18) with lipopolysaccharide-inhibitory activity. Infect Immun. (1994) 62:1421–6. doi: 10.1128/IAI.62.4.1421-1426.1994
65. Larrick JW, Hirata M, Shimomoura Y, Yoshida M, Zheng H, Zhong J, et al. Rabbit CAP18 derived peptides inhibit gram negative and gram positive bacteria. Prog Clin Biol Res. (1994) 388:125–35.
66. Nagaoka I, Hirota S, Niyonsaba F, Hirata M, Adachi Y, Tamura H, et al. Cathelicidin family of antibacterial peptides CAP18 and CAP11 inhibit the expression of TNF-alpha by blocking the binding of LPS to CD14(+) cells. J Immunol. (2001) 167:3329–38. doi: 10.4049/jimmunol.167.6.3329
67. Nagaoka I, Hirota S, Niyonsaba F, Hirata M, Adachi Y, Tamura H, et al. Augmentation of the lipopolysaccharide-neutralizing activities of human cathelicidin CAP18/LL-37-derived antimicrobial peptides by replacement with hydrophobic and cationic amino acid residues. Clin Diagn Lab Immunol. (2002) 9:972–82. doi: 10.1128/CDLI.9.5.972-982.2002
68. Molhoek EM, den Hertog AL, de Vries AM, Nazmi K, Veerman EC, Hartgers FC, et al. Structure-function relationship of the human antimicrobial peptide LL-37 and LL-37 fragments in the modulation of TLR responses. Biol Chem. (2009) 390:295–303. doi: 10.1515/BC.2009.037
69. Molhoek EM, van Dijk A, Veldhuizen EJA, Dijk-Knijnenburg H, Mars-Groenendijk RH, Boele LC, et al. Chicken cathelicidin-2-derived peptides with enhanced immunomodulatory and antibacterial activities against biological warfare agents. Int J Antimicrob Agents. (2010) 36:271–4. doi: 10.1016/j.ijantimicag.2010.06.001
70. Scott A, Weldon S, Buchanan PJ, Schock B, Ernst RK, McAuley DF, et al. Evaluation of the ability of LL-37 to neutralise LPS in vitro and ex vivo. PLoS ONE. (2011) 6:e26525. doi: 10.1371/journal.pone.0026525
71. Mookherjee N, Brown KL, Bowdish DM, Doria S, Falsafi R, Hokamp K, et al. Modulation of the TLR-mediated inflammatory response by the endogenous human host defense peptide LL-37. J Immunol. (2006) 176:2455–64. doi: 10.4049/jimmunol.176.4.2455
72. Mookherjee N, Wilson HL, Doria S, Popowych Y, Falsafi R, Yu JJ, et al. Bovine and human cathelicidin cationic host defense peptides similarly suppress transcriptional responses to bacterial lipopolysaccharide. J Leukoc Biol. (2006) 80:1563–74. doi: 10.1189/jlb.0106048
73. Coorens M, Banaschewski JHB, Baer BJ, Yamashita C, van Dijk A, Haagsman HP, et al. Killing of Pseudomonas Aeruginosa by chicken cathelicidin-2 is immunogenically silent, preventing lung inflammation in vivo. Infect Immun. (2017) 85:e00546–17. doi: 10.1128/IAI.00546-17
74. Turner J, Cho Y, Dinh NN, Waring AJ, Lehrer RI. Activities of LL-37, a cathelin-associated antimicrobial peptide of human neutrophils. Antimicrob Agents Chemother. (1998) 42:2206–14. doi: 10.1128/AAC.42.9.2206
75. Nell MJ, Tjabringa GS, Wafelman AR, Verrijk R, Hiemstra PS, Drijfhout JW, et al. Development of novel LL-37 derived antimicrobial peptides with LPS and LTA neutralizing and antimicrobial activities for therapeutic application. Peptides. (2006) 27:649–60. doi: 10.1016/j.peptides.2005.09.016
76. Nagaoka I, Yomogida S, Tamura H, Hirata M. Antibacterial cathelicidin peptide CAP11 inhibits the lipopolysaccharide (LPS)-induced suppression of neutrophil apoptosis by blocking the binding of LPS to target cells. Inflamm Res. (2004) 53:609–22. doi: 10.1007/s00011-004-1300-2
77. Coorens MV, Schneider AF, de Groot AM, van Dijk A, Meijerink M, et al. Cathelicidins inhibit Escherichia Coli-induced TLR2 and TLR4 activation in a viability-dependent manner. J Immunol. (2017) 199:1418–28. doi: 10.4049/jimmunol.1602164
78. Scheenstra MR, van den Belt M, Tjeerdsma-van Bokhoven JLM, Schneider AFV, Ordonez SR, van Dijk A, et al. Cathelicidins PMAP-36, LL-37 and CATH-2 are similar peptides with different modes of action. Sci Rep. (2019) 9:4780. doi: 10.1038/s41598-019-41246-6
79. Schneider VA, Coorens M, Ordonez SR, Tjeerdsma-van Bokhoven JL, Posthuma G, van Dijk A, et al. Imaging the antimicrobial mechanism(s) of cathelicidin-2. Sci Rep. (2016) 6:32948. doi: 10.1038/srep32948
80. Sochacki KA, Barns KJ, Bucki R, Weisshaar JC. Real-time attack on single Escherichia Coli cells by the human antimicrobial peptide LL-37. Proc Natl Acad Sci USA. (2011) 108:E77–81. doi: 10.1073/pnas.1101130108
81. Koziel J, Bryzek D, Sroka A, Maresz K, Glowczyk I, Bielecka E, et al. Citrullination alters immunomodulatory function of LL-37 essential for prevention of endotoxin-induced sepsis. J Immunol. (2014) 192:5363–72. doi: 10.4049/jimmunol.1303062
82. Al-Adwani S, Wallin C, Balhuizen MD, Veldhuizen EJA, Coorens M, Landreh M, et al. Studies on citrullinated LL-37: detection in human airways, antibacterial effects and biophysical properties. Sci Rep. (2020) 10:2376. doi: 10.1038/s41598-020-59071-7
83. Rosenfeld Y, Papo N, Shai Y. Endotoxin (lipopolysaccharide) neutralization by innate immunity host-defense peptides. Peptide properties and plausible modes of action. J Biol Chem. (2006) 281:1636–43. doi: 10.1074/jbc.M504327200
84. Rosenfeld Y, Shai Y. Lipopolysaccharide (endotoxin)-host defense antibacterial peptides interactions: role in bacterial resistance and prevention of sepsis. Biochim Biophys Acta. (2006) 1758:1513–22. doi: 10.1016/j.bbamem.2006.05.017
85. Wang G, Elliott M, Cogen AL, Ezell EL, Gallo RL, Hancock RE. Structure, dynamics, and antimicrobial and immune modulatory activities of human LL-23 and its single-residue variants mutated on the basis of homologous primate cathelicidins. Biochemistry. (2012) 51:653–64. doi: 10.1021/bi2016266
86. Pinheiro da Silva F, Gallo RL, Nizet V. Differing effects of exogenous or endogenous cathelicidin on macrophage toll-like receptor signaling. Immunol Cell Biol. (2009) 87:496–500. doi: 10.1038/icb.2009.19
87. Nijnik A, Pistolic J, Wyatt A, Tam S, Hancock RE. Human cathelicidin peptide LL-37 modulates the effects of IFN-gamma on APCs. J Immunol. (2009) 183:5788–98. doi: 10.4049/jimmunol.0901491
88. Amatngalim GD, Nijnik A, Hiemstra PS, Hancock RE. Cathelicidin peptide LL-37 modulates TREM-1 expression and inflammatory responses to microbial compounds. Inflammation. (2011) 34:412–25. doi: 10.1007/s10753-010-9248-6
89. Shaykhiev R, Sierigk J, Herr C, Krasteva G, Kummer W, Bals R. The antimicrobial peptide cathelicidin enhances activation of lung epithelial cells by LPS. FASEB J. (2010) 24:4756–66. doi: 10.1096/fj.09.151332
90. Suzuki K, Murakami T, Hu Z, Tamura H, Kuwahara-Arai K, Iba T, et al. Human host defense cathelicidin peptide LL-37 enhances the lipopolysaccharide uptake by liver sinusoidal endothelial cells without cell activation. J Immunol. (2016) 196:1338–47. doi: 10.4049/jimmunol.1403203
91. Kandler K, Shaykhiev R, Kleemann P, Klescz F, Lohoff M, Vogelmeier C, et al. The anti-microbial peptide LL-37 inhibits the activation of dendritic cells by TLR ligands. Int Immunol. (2006) 18:1729–36. doi: 10.1093/intimm/dxl107
92. Schneider VAF, Coorens M, Tjeerdsma-van Bokhoven JLM, Posthuma G, van Dijk A, Veldhuizen EJA, et al. Imaging the antistaphylococcal activity of CATH-2: mechanism of attack and regulation of inflammatory response. mSphere. (2017) 2:e00370–17. doi: 10.1128/mSphere.00370-17
93. Filewod NC, Pistolic J, Hancock RE. Low concentrations of LL-37 alter IL-8 production by keratinocytes and bronchial epithelial cells in response to proinflammatory stimuli. FEMS Immunol Med Microbiol. (2009) 56:233–40. doi: 10.1111/j.1574-695X.2009.00571.x
94. Blasius AL, Beutler B. Intracellular toll-like receptors. Immunity. (2010) 32:305–15. doi: 10.1016/j.immuni.2010.03.012
95. Lester SN, Li K. Toll-like receptors in antiviral innate immunity. J Mol Biol. (2014) 426:1246–64. doi: 10.1016/j.jmb.2013.11.024
96. Gilliet M, Lande R. Antimicrobial peptides and self-DNA in autoimmune skin inflammation. Curr Opin Immunol. (2008) 20:401–7. doi: 10.1016/j.coi.2008.06.008
97. Barra GB, Santa Rita TH, de Almeida Vasques J, Chianca CF, Nery LF, Santana Soares Costa S. EDTA-mediated inhibition of DNases protects circulating cell-free DNA from ex vivo degradation in blood samples. Clin Biochem. (2015) 48:976–81. doi: 10.1016/j.clinbiochem.2015.02.014
98. Ganguly D, Chamilos G, Lande R, Gregorio J, Meller S, Facchinetti V, et al. Self-RNA-antimicrobial peptide complexes activate human dendritic cells through TLR7 and TLR8. J Exp Med. (2009) 206:1983–94. doi: 10.1084/jem.20090480
99. Gilliet M, Cao W, Liu YJ. Plasmacytoid dendritic cells: sensing nucleic acids in viral infection and autoimmune diseases. Nat Rev Immunol. (2008) 8:594–606. doi: 10.1038/nri2358
100. Bauer S, Pigisch S, Hangel D, Kaufmann A, Hamm S. Recognition of nucleic acid and nucleic acid analogs by toll-like receptors 7, 8 and 9. Immunobiology. (2008) 213:315–28. doi: 10.1016/j.imbio.2007.10.010
101. von Landenberg P, Bauer S. Nucleic acid recognizing toll-like receptors and autoimmunity. Curr Opin Immunol. (2007) 19:606–10. doi: 10.1016/j.coi.2007.10.004
102. Keestra AM, de Zoete MR, Bouwman LI, van Putten JP. Chicken TLR21 is an innate CpG DNA receptor distinct from mammalian TLR9. J Immunol. (2010) 185:460–7. doi: 10.4049/jimmunol.0901921
103. Chen JQ, Szodoray P, Zeher M. Toll-like receptor pathways in autoimmune diseases. Clin Rev Allergy Immunol. (2016) 50:1–17. doi: 10.1007/s12016-015-8473-z
104. Neumann A, Vollger L, Berends ET, Molhoek EM, Stapels DA, Midon M, et al. Novel role of the antimicrobial peptide LL-37 in the protection of neutrophil extracellular traps against degradation by bacterial nucleases. J Innate Immun. (2014) 6:860–8. doi: 10.1159/000363699
105. Sandgren S, Wittrup A, Cheng F, Jonsson M, Eklund E, Busch S, et al. The human antimicrobial peptide LL-37 transfers extracellular DNA plasmid to the nuclear compartment of mammalian cells via lipid rafts and proteoglycan-dependent endocytosis. J Biol Chem. (2004) 279:17951–6. doi: 10.1074/jbc.M311440200
106. Lazzaretto B, Fadeel B. Intra- and extracellular degradation of neutrophil extracellular traps by macrophages and dendritic cells. J Immunol. (2019) 203:2276–90. doi: 10.4049/jimmunol.1800159
107. Hurtado P, Peh CA. LL-37 promotes rapid sensing of CpG oligodeoxynucleotides by B lymphocytes and plasmacytoid dendritic cells. J Immunol. (2010) 184:1425–35. doi: 10.4049/jimmunol.0902305
108. Stephan A, Batinica M, Steiger J, Hartmann P, Zaucke F, Bloch W, et al. LL37:DNA complexes provide antimicrobial activity against intracellular bacteria in human macrophages. Immunology. (2016) 148:420–32. doi: 10.1111/imm.12620
109. von Kockritz-Blickwede M, Goldmann O, Thulin P, Heinemann K, Norrby-Teglund A, Rohde M, et al. Phagocytosis-independent antimicrobial activity of mast cells by means of extracellular trap formation. Blood. (2008) 111:3070–80. doi: 10.1182/blood-2007-07-104018
110. Gregorio J, Meller S, Conrad C, Di Nardo A, Homey B, Lauerma A, et al. Plasmacytoid dendritic cells sense skin injury and promote wound healing through type I interferons. J Exp Med. (2010) 207:2921–30. doi: 10.1084/jem.20101102
111. Piccioli D, Sammicheli C, Tavarini S, Nuti S, Frigimelica E, Manetti AG, et al. Human plasmacytoid dendritic cells are unresponsive to bacterial stimulation and require a novel type of cooperation with myeloid dendritic cells for maturation. Blood. (2009) 113:4232–9. doi: 10.1182/blood-2008-10-186890
112. Rahman T, Brown AS, Hartland EL, van Driel IR, Fung KY. Plasmacytoid dendritic cells provide protection against bacterial-induced colitis. Front Immunol. (2019) 10:608. doi: 10.3389/fimmu.2019.00608
113. Reizis B. Plasmacytoid dendritic cells: development, regulation, and function. Immunity. (2019) 50:37–50. doi: 10.1016/j.immuni.2018.12.027
114. Schmidt NW, Jin F, Lande R, Curk T, Xian W, Lee C, et al. Liquid-crystalline ordering of antimicrobial peptide-DNA complexes controls TLR9 activation. Nat Mater. (2015) 14:696–700. doi: 10.1038/nmat4298
115. Lee EY, Zhang C, Di Domizio J, Jin F, Connell W, Hung M, et al. Helical antimicrobial peptides assemble into protofibril scaffolds that present ordered dsDNA to TLR9. Nat Commun. (2019) 10:1012. doi: 10.1038/s41467-019-08868-w
116. Lee HK, Lund JM, Ramanathan B, Mizushima N, Iwasaki A. Autophagy-dependent viral recognition by plasmacytoid dendritic cells. Science. (2007) 315:1398–401. doi: 10.1126/science.1136880
117. Suares A, Tapia C, Gonzalez-Pardo V. VDR agonists down regulate PI3K/Akt/mTOR axis and trigger autophagy in kaposi's sarcoma cells. Heliyon. (2019) 5:e02367. doi: 10.1016/j.heliyon.2019.e02367
118. Yuk JM, Shin DM, Lee HM, Yang CS, Jin HS, Kim KK, et al. Vitamin D3 induces autophagy in human monocytes/macrophages via cathelicidin. Cell Host Microbe. (2009) 6:231–43. doi: 10.1016/j.chom.2009.08.004
119. Rekha RS, Rao Muvva SS, Wan M, Raqib R, Bergman P, Brighenti S, et al. Phenylbutyrate induces LL-37-dependent autophagy and intracellular killing of Mycobacterium tuberculosis in human macrophages. Autophagy. (2015) 11:1688–99. doi: 10.1080/15548627.2015.1075110
120. De Nardo D, De Nardo CM, Nguyen T, Hamilton JA, Scholz GM. Signaling crosstalk during sequential TLR4 and TLR9 activation amplifies the inflammatory response of mouse macrophages. J Immunol. (2009) 183:8110–8. doi: 10.4049/jimmunol.0901031
121. Gantier MP, Tong S, Behlke MA, Xu D, Phipps S, Foster PS, et al. TLR7 is involved in sequence-specific sensing of single-stranded RNAs in human macrophages. J Immunol. (2008) 180:2117–24. doi: 10.4049/jimmunol.180.4.2117
122. Hemont C, Neel A, Heslan M, Braudeau C, Josien R. Human blood mDC subsets exhibit distinct TLR repertoire and responsiveness. J Leukoc Biol. (2013) 93:599–609. doi: 10.1189/jlb.0912452
123. Perrot I, Deauvieau F, Massacrier C, Hughes N, Garrone P, Durand I, et al. TLR3 and Rig-like receptor on myeloid dendritic cells and rig-like receptor on human NK cells are both mandatory for production of IFN-gamma in response to double-stranded RNA. J Immunol. (2010) 185:2080–8. doi: 10.4049/jimmunol.1000532
124. Bourke E, Bosisio D, Golay J, Polentarutti N, Mantovani A. The toll-like receptor repertoire of human B lymphocytes: inducible and selective expression of TLR9 and TLR10 in normal and transformed cells. Blood. (2003) 102:956–63. doi: 10.1182/blood-2002-11-3355
125. Freeman SA, Jaumouille V, Choi K, Hsu BE, Wong HS, Abraham L, et al. Toll-like receptor ligands sensitize B-cell receptor signalling by reducing actin-dependent spatial confinement of the receptor. Nat Commun. (2015) 6:6168. doi: 10.1038/ncomms7168
126. Kuraoka M, Snowden PB, Nojima T, Verkoczy L, Haynes BF, Kitamura D, et al. BCR and endosomal TLR signals synergize to increase AID expression and establish central B cell tolerance. Cell Rep. (2017) 18:1627–35. doi: 10.1016/j.celrep.2017.01.050
127. Suthers AN, Sarantopoulos S. TLR7/TLR9- and B cell receptor-signaling crosstalk: promotion of potentially dangerous B cells. Front Immunol. (2017) 8:775. doi: 10.3389/fimmu.2017.00775
128. Gestermann N, Di Domizio J, Lande R, Demaria O, Frasca L, Feldmeyer L, et al. Netting neutrophils activate autoreactive B cells in lupus. J Immunol. (2018) 200:3364–71. doi: 10.4049/jimmunol.1700778
129. Nakagawa Y, Gallo RL. Endogenous intracellular cathelicidin enhances TLR9 activation in dendritic cells and macrophages. J Immunol. (2015) 194:1274–84. doi: 10.4049/jimmunol.1402388
130. Singh D, Qi R, Jordan JL, San Mateo L, Kao CC. The human antimicrobial peptide LL-37, but not the mouse ortholog, mCRAMP, can stimulate signaling by poly(I:C) through a FPRL1-dependent pathway. J Biol Chem. (2013) 288:8258–68. doi: 10.1074/jbc.M112.440883
131. Singh D, Vaughan R, Kao CC. LL-37 peptide enhancement of signal transduction by Toll-like receptor 3 is regulated by pH: identification of a peptide antagonist of LL-37. J Biol Chem. (2014) 289:27614–24. doi: 10.1074/jbc.M114.582973
132. Levast B, Hogan D, van Kessel J, Strom S, Walker S, Zhu J, et al. Synthetic cationic peptide IDR-1002 and human cathelicidin LL37 modulate the cell innate response but differentially impact PRRSV replication in vitro. Front Vet Sci. (2019) 6:233. doi: 10.3389/fvets.2019.00233
133. Braff MH, Zaiou M, Fierer J, Nizet V, Gallo RL. Keratinocyte production of cathelicidin provides direct activity against bacterial skin pathogens. Infect Immun. (2005) 73:6771–81. doi: 10.1128/IAI.73.10.6771-6781.2005
134. Gallo RL, Nakatsuji T. Microbial symbiosis with the innate immune defense system of the skin. J Invest Dermatol. (2011) 131:1974–80. doi: 10.1038/jid.2011.182
135. Heilborn JD, Nilsson MF, Kratz G, Weber G, Sorensen O, Borregaard N, et al. The cathelicidin anti-microbial peptide LL-37 is involved in re-epithelialization of human skin wounds and is lacking in chronic ulcer epithelium. J Invest Dermatol. (2003) 120:379–89. doi: 10.1046/j.1523-1747.2003.12069.x
136. Sorensen OE, Cowland JB, Theilgaard-Monch K, Liu L, Ganz T, Borregaard N. Wound healing and expression of antimicrobial peptides/polypeptides in human keratinocytes, a consequence of common growth factors. J Immunol. (2003) 170:5583–9. doi: 10.4049/jimmunol.170.11.5583
137. Lebre MC, van der Aar AM, van Baarsen L, van Capel TM, Schuitemaker JH, Kapsenberg ML, et al. Human keratinocytes express functional toll-like receptor 3, 4, 5, and 9. J Invest Dermatol. (2007) 127:331–41. doi: 10.1038/sj.jid.5700530
138. Miller LS, Modlin RL. Human keratinocyte toll-like receptors promote distinct immune responses. J Invest Dermatol. (2007) 127:262–3. doi: 10.1038/sj.jid.5700559
139. Morizane S, Yamasaki K, Muhleisen B, Kotol PF, Murakami M, Aoyama Y, et al. Cathelicidin antimicrobial peptide LL-37 in psoriasis enables keratinocyte reactivity against TLR9 ligands. J Invest Dermatol. (2012) 132:135–43. doi: 10.1038/jid.2011.259
140. Dombrowski Y, Schauber J. Cathelicidin LL-37: a defense molecule with a potential role in psoriasis pathogenesis. Exp Dermatol. (2012) 21:327–30. doi: 10.1111/j.1600-0625.2012.01459.x
141. Morizane S, Gallo RL. Antimicrobial peptides in the pathogenesis of psoriasis. J Dermatol. (2012) 39:225–30. doi: 10.1111/j.1346-8138.2011.01483.x
142. Ibanez de Aldecoa AL, Zafra O, Gonzalez-Pastor JE. Mechanisms and regulation of extracellular DNA release and its biological roles in microbial communities. Front Microbiol. (2017) 8:1390. doi: 10.3389/fmicb.2017.01390
143. Montanaro L, Poggi A, Visai L, Ravaioli S, Campoccia D, Speziale P, et al. Extracellular DNA in biofilms. Int J Artif Organs. (2011) 34:824–31. doi: 10.5301/ijao.5000051
144. Lee EY, Takahashi T, Curk T, Dobnikar J, Gallo RL, Wong CLG. Crystallinity of double-stranded RNA-antimicrobial peptide complexes modulates toll-like receptor 3-mediated inflammation. ACS Nano. (2017) 11:12145–55. doi: 10.1021/acsnano.7b05234
145. Takiguchi T, Morizane S, Yamamoto T, Kajita A, Ikeda K, Iwatsuki K. Cathelicidin antimicrobial peptide LL-37 augments interferon-β expression and antiviral activity induced by double-stranded RNA in keratinocytes. Br J Dermatol. (2014) 171:492–8. doi: 10.1111/bjd.12942
146. Nijnik A, Pistolic J, Filewod NC, Hancock RE. Signaling pathways mediating chemokine induction in keratinocytes by cathelicidin LL-37 and flagellin. J Innate Immun. (2012) 4:377–86. doi: 10.1159/000335901
147. Pistolic J, Cosseau C, Li Y, Yu JJ, Filewod NC, Gellatly S, et al. Host defence peptide LL-37 induces IL-6 expression in human bronchial epithelial cells by activation of the NF-kappaB signaling pathway. J Innate Immun. (2009) 1:254–67. doi: 10.1159/000171533
148. He Y, Hara H, Nunez G. Mechanism and regulation of NLRP3 inflammasome activation. Trends Biochem Sci. (2016) 41:1012–21. doi: 10.1016/j.tibs.2016.09.002
149. Elssner A, Duncan M, Gavrilin M, Wewers MD. A novel P2X7 receptor activator, the human cathelicidin-derived peptide LL37, induces IL-1 beta processing and release. J Immunol. (2004) 172:4987–94. doi: 10.4049/jimmunol.172.8.4987
150. McHugh BJ, Wang R, Li HN, Beaumont PE, Kells R, Stevens H, et al. Cathelicidin is a fire alarm, generating protective NLRP3-dependent airway epithelial cell inflammatory responses during infection with Pseudomonas aeruginosa. PLoS Pathog. (2019) 15:e1007694. doi: 10.1371/journal.ppat.1007694
151. Kahlenberg JM, Carmona-Rivera C, Smith CK, Kaplan MJ. Neutrophil extracellular trap-associated protein activation of the NLRP3 inflammasome is enhanced in lupus macrophages. J Immunol. (2013) 190:1217–26. doi: 10.4049/jimmunol.1202388
152. Marin M, Holani R, Blyth ADG, Drouin D, Odeon A, Cobo ER. Human cathelicidin improves colonic epithelial defenses against Salmonella typhimurium by modulating bacterial invasion, TLR4 and pro-inflammatory cytokines. Cell Tissue Res. (2019) 376:433–42. doi: 10.1007/s00441-018-02984-7
153. Cirone KM, Lahiri P, Holani R, Tan YL, Arrazuria R, De Buck J, et al. Synthetic cathelicidin LL-37 reduces Mycobacterium avium subsp. paratuberculosis internalization and pro-inflammatory cytokines in macrophages. Cell Tissue Res. (2020) 379:207–17. doi: 10.1007/s00441-019-03098-4
154. Luo XL, Li JX, Huang HR, Duan JL, Dai RX, Tao RJ, et al. LL37 Inhibits Aspergillus Fumigatus infection via directly binding to the fungus and preventing excessive inflammation. Front Immunol. (2019) 10:283. doi: 10.3389/fimmu.2019.00283
155. Nizet V, Ohtake T, Lauth X, Trowbridge J, Rudisill J, Dorschner RA, et al. Innate antimicrobial peptide protects the skin from invasive bacterial infection. Nature. (2001) 414:454–7. doi: 10.1038/35106587
156. Talreja D, Singh PK, Kumar A. In vivo role of TLR2 and MyD88 signaling in eliciting innate immune responses in staphylococcal endophthalmitis. Invest Ophthalmol Vis Sci. (2015) 56:1719–32. doi: 10.1167/iovs.14-16087
157. Ho J, Chan H, Liang Y, Liu X, Zhang L, Li Q, et al. Cathelicidin preserves intestinal barrier function in polymicrobial sepsis. Crit Care. (2020) 24:47. doi: 10.1186/s13054-020-2754-5
158. Tai EK, Wu WK, Wang XJ, Wong HP, Yu L, Li ZJ, et al. Intrarectal administration of mCRAMP-encoding plasmid reverses exacerbated colitis in Cnlp(-/-) mice. Gene Ther. (2013) 20:187–93. doi: 10.1038/gt.2012.22
159. Kovach MA, Ballinger MN, Newstead MW, Zeng X, Bhan U, Yu FS, et al. Cathelicidin-related antimicrobial peptide is required for effective lung mucosal immunity in gram-negative bacterial pneumonia. J Immunol. (2012) 189:304–11. doi: 10.4049/jimmunol.1103196
160. Deng YY, Shamoon M, He Y, Bhatia M, Sun J. Cathelicidin-related antimicrobial peptide modulates the severity of acute pancreatitis in mice. Mol Med Rep. (2016) 13:3881–5. doi: 10.3892/mmr.2016.5008
161. Kress E, Merres J, Albrecht LJ, Hammerschmidt S, Pufe T, Tauber SC, et al. CRAMP deficiency leads to a pro-inflammatory phenotype and impaired phagocytosis after exposure to bacterial meningitis pathogens. Cell Commun Signal. (2017) 15:32. doi: 10.1186/s12964-017-0190-1
162. Merres J, Hoss J, Albrecht LJ, Kress E, Soehnlein O, Jansen S, et al. Role of the cathelicidin-related antimicrobial peptide in inflammation and mortality in a mouse model of bacterial meningitis. J Innate Immun. (2014) 6:205–18. doi: 10.1159/000353645
163. Hosoda H, Nakamura K, Hu Z, Tamura H, Reich J, Kuwahara-Arai K, et al. Antimicrobial cathelicidin peptide LL37 induces NET formation and suppresses the inflammatory response in a mouse septic model. Mol Med Rep. (2017) 16:5618–26. doi: 10.3892/mmr.2017.7267
164. Hu Z, Murakami T, Suzuki K, Tamura H, Reich J, Kuwahara-Arai K, et al. Antimicrobial cathelicidin peptide LL-37 inhibits the pyroptosis of macrophages and improves the survival of polybacterial septic mice. Int Immunol. (2016) 28:245–53. doi: 10.1093/intimm/dxv113
165. Banaschewski BJH, Baer B, Arsenault C, Jazey T, Veldhuizen EJA, Delport J, et al. The antibacterial and anti-inflammatory activity of chicken cathelicidin-2 combined with exogenous surfactant for the treatment of cystic fibrosis-associated pathogens. Sci Rep. (2017) 7:15545. doi: 10.1038/s41598-017-15558-4
166. Veldhuizen EJA, Brouwer EC, Schneider VA, Fluit AC. Chicken cathelicidins display antimicrobial activity against multiresistant bacteria without inducing strong resistance. PLoS ONE. (2013) 8:e61964. doi: 10.1371/journal.pone.0061964
167. He M, Zhang H, Li Y, Wang G, Tang B, Zhao J, et al. Cathelicidin-derived antimicrobial peptides inhibit zika virus through direct inactivation and interferon pathway. Front Immunol. (2018) 9:722. doi: 10.3389/fimmu.2018.00722
168. Garcia-Romo GS, Caielli S, Vega B, Connolly J, Allantaz F, Xu Z, et al. Netting neutrophils are major inducers of type I IFN production in pediatric systemic lupus erythematosus. Sci Transl Med. (2011) 3:73ra20. doi: 10.1126/scitranslmed.3001201
169. Lande R, Ganguly D, Facchinetti V, Frasca L, Conrad C, Gregorio J, et al. Neutrophils activate plasmacytoid dendritic cells by releasing self-DNA-peptide complexes in systemic lupus erythematosus. Sci Transl Med. (2011) 3:73ra19. doi: 10.1126/scitranslmed.3001180
170. Herster F, Bittner Z, Archer NK, Dickhofer S, Eisel D, Eigenbrod T, et al. Neutrophil extracellular trap-associated RNA and LL37 enable self-amplifying inflammation in psoriasis. Nat Commun. (2020) 11:105. doi: 10.1038/s41467-019-13756-4
171. Cao D, Li H, Jiang Z, Cheng Q, Yang Z, Xu C, et al. CpG oligodeoxynucleotide synergizes innate defense regulator peptide for enhancing the systemic and mucosal immune responses to pseudorabies attenuated virus vaccine in piglets in vivo. Int Immunopharmacol. (2011) 11:748–54. doi: 10.1016/j.intimp.2011.01.028
172. Kindrachuk J, Jenssen H, Elliott M, Townsend R, Nijnik A, Lee SF, et al. A novel vaccine adjuvant comprised of a synthetic innate defence regulator peptide and CpG oligonucleotide links innate and adaptive immunity. Vaccine. (2009) 27:4662–71. doi: 10.1016/j.vaccine.2009.05.094
173. Garlapati S, Eng NF, Kiros TG, Kindrachuk J, Mutwiri GK, Hancock RE, et al. Immunization with PCEP microparticles containing pertussis toxoid, CpG ODN and a synthetic innate defense regulator peptide induces protective immunity against pertussis. Vaccine. (2011) 29:6540–8. doi: 10.1016/j.vaccine.2011.07.009
174. Schulze K, Ebensen T, Babiuk LA, Gerdts V, Guzman CA. Intranasal vaccination with an adjuvanted polyphosphazenes nanoparticle-based vaccine formulation stimulates protective immune responses in mice. Nanomedicine. (2017) 13:2169–78. doi: 10.1016/j.nano.2017.05.012
175. Liu C, Chu X, Sun P, Feng X, Huang W, Liu H, et al. Synergy effects of polyinosinic-polycytidylic acid, CpG oligodeoxynucleotide, and cationic peptides to adjuvant HPV E7 epitope vaccine through preventive and therapeutic immunization in a TC-1 grafted mouse model. Hum Vaccin Immunother. (2018) 14:931–40. doi: 10.1080/21645515.2017.1420446
176. Achtman AH, Pilat S, Law CW, Lynn DJ, Janot L, Mayer ML, et al. Effective adjunctive therapy by an innate defense regulatory peptide in a preclinical model of severe malaria. Sci Transl Med. (2012) 4:135ra64. doi: 10.1126/scitranslmed.3003515
177. Prysliak T, Maina T, Yu L, Suleman M, Jimbo S, Perez-Casal J. Induction of a balanced IgG1/IgG2 immune response to an experimental challenge with Mycoplasma bovis antigens following a vaccine composed of Emulsigen, IDR peptide1002, and poly IC: Vaccine. (2017) 35:6604–10. doi: 10.1016/j.vaccine.2017.10.037
178. Schneider C, Schmidt T, Ziske C, Tiemann K, Lee KM, Uhlinsky V, et al. Tumour suppression induced by the macrophage activating lipopeptide MALP-2 in an ultrasound guided pancreatic carcinoma mouse model. Gut. (2004) 53:355–61. doi: 10.1136/gut.2003.026005
179. Pratesi G, Petrangolini G, Tortoreto M, Addis A, Belluco S, Rossini A, et al. Therapeutic synergism of gemcitabine and CpG-oligodeoxynucleotides in an orthotopic human pancreatic carcinoma xenograft. Cancer Res. (2005) 65:6388–93. doi: 10.1158/0008-5472.CAN-05-0602
180. Brignole C, Marimpietri D, Di Paolo D, Perri P, Morandi F, Pastorino F, et al. Therapeutic targeting of TLR9 inhibits cell growth and induces apoptosis in neuroblastoma. Cancer Res. (2010) 70:9816–26. doi: 10.1158/0008-5472.CAN-10-1251
181. Chuang CM, Monie A, Wu A, Mao CP, Hung CF. Treatment with LL-37 peptide enhances antitumor effects induced by CpG oligodeoxynucleotides against ovarian cancer. Hum Gene Ther. (2009) 20:303–13. doi: 10.1089/hum.2008.124
182. Rakhesh M, Cate M, Vijay R, Shrikant A, Shanjana A. A TLR4-interacting peptide inhibits lipopolysaccharide-stimulated inflammatory responses, migration and invasion of colon cancer SW480 cells. Oncoimmunology. (2012) 1:1495–506. doi: 10.4161/onci.22089
183. Ochi A, Nguyen AH, Bedrosian AS, Mushlin HM, Zarbakhsh S, Barilla R, et al. MyD88 inhibition amplifies dendritic cell capacity to promote pancreatic carcinogenesis via Th2 cells. J Exp Med. (2012) 209:1671–87. doi: 10.1084/jem.20111706
184. Kelly MG, Alvero AB, Chen R, Silasi DA, Abrahams VM, Chan S, et al. TLR-4 signaling promotes tumor growth and paclitaxel chemoresistance in ovarian cancer. Cancer Res. (2006) 66:3859–68. doi: 10.1158/0008-5472.CAN-05-3948
185. Piktel E, Niemirowicz K, Wnorowska U, Watek M, Wollny T, Gluszek K, et al. The Role of cathelicidin LL-37 in cancer development. Arch Immunol Ther Exp. (2016) 64:33–46. doi: 10.1007/s00005-015-0359-5
186. Ren SX, Cheng AS, To KF, Tong JH, Li MS, Shen J, et al. Host immune defense peptide LL-37 activates caspase-independent apoptosis and suppresses colon cancer. Cancer Res. (2012) 72:6512–23. doi: 10.1158/0008-5472.CAN-12-2359
187. Wu WK, Wang G, Coffelt SB, Betancourt AM, Lee CW, Fan D, et al. Emerging roles of the host defense peptide LL-37 in human cancer and its potential therapeutic applications. Int J Cancer. (2010) 127:1741–7. doi: 10.1002/ijc.25489
188. Muccioli M, Benencia F. Toll-like receptors in ovarian cancer as targets for immunotherapies. Front Immunol. (2014) 5:341. doi: 10.3389/fimmu.2014.00341
Keywords: macrophages, dendritic cells, antimicrobial peptides, cathelicidins, LL-37, Toll-like receptors, MAMPs, DAMPs
Citation: Scheenstra MR, van Harten RM, Veldhuizen EJA, Haagsman HP and Coorens M (2020) Cathelicidins Modulate TLR-Activation and Inflammation. Front. Immunol. 11:1137. doi: 10.3389/fimmu.2020.01137
Received: 06 March 2020; Accepted: 11 May 2020;
Published: 09 June 2020.
Edited by:
Mark Hulett, La Trobe University, AustraliaReviewed by:
Felix Ngosa Toka, Ross University School of Veterinary Medicine, Saint Kitts and NevisAlan L. Scott, Johns Hopkins University, United States
Copyright © 2020 Scheenstra, van Harten, Veldhuizen, Haagsman and Coorens. This is an open-access article distributed under the terms of the Creative Commons Attribution License (CC BY). The use, distribution or reproduction in other forums is permitted, provided the original author(s) and the copyright owner(s) are credited and that the original publication in this journal is cited, in accordance with accepted academic practice. No use, distribution or reproduction is permitted which does not comply with these terms.
*Correspondence: Maaike R. Scheenstra, bS5yLnNjaGVlbnN0cmFAdXUubmw=