- 1Department of Immunology, Fraunhofer Institute for Cell Therapy and Immunology, Leipzig, Germany
- 2Department of Gastroenterology, Hepatology and Endocrinology, Hannover Medical School, Hannover, Germany
The use of biomarkers in diagnosis, therapy and prognosis has gained increasing interest over the last decades. In particular, the analysis of biomarkers in cancer patients within the pre- and post-therapeutic period is required to identify several types of cells, which carry a risk for a disease progression and subsequent post-therapeutic relapse. Cancer stem cells (CSCs) are a subpopulation of tumor cells that can drive tumor initiation and can cause relapses. At the time point of tumor initiation, CSCs originate from either differentiated cells or adult tissue resident stem cells. Due to their importance, several biomarkers that characterize CSCs have been identified and correlated to diagnosis, therapy and prognosis. However, CSCs have been shown to display a high plasticity, which changes their phenotypic and functional appearance. Such changes are induced by chemo- and radiotherapeutics as well as senescent tumor cells, which cause alterations in the tumor microenvironment. Induction of senescence causes tumor shrinkage by modulating an anti-tumorigenic environment in which tumor cells undergo growth arrest and immune cells are attracted. Besides these positive effects after therapy, senescence can also have negative effects displayed post-therapeutically. These unfavorable effects can directly promote cancer stemness by increasing CSC plasticity phenotypes, by activating stemness pathways in non-CSCs, as well as by promoting senescence escape and subsequent activation of stemness pathways. At the end, all these effects can lead to tumor relapse and metastasis. This review provides an overview of the most frequently used CSC markers and their implementation as biomarkers by focussing on deadliest solid (lung, stomach, liver, breast and colorectal cancers) and hematological (acute myeloid leukemia, chronic myeloid leukemia) cancers. Furthermore, it gives examples on how the CSC markers might be influenced by therapeutics, such as chemo- and radiotherapy, and the tumor microenvironment. It points out, that it is crucial to identify and monitor residual CSCs, senescent tumor cells, and the pro-tumorigenic senescence-associated secretory phenotype in a therapy follow-up using specific biomarkers. As a future perspective, a targeted immune-mediated strategy using chimeric antigen receptor based approaches for the removal of remaining chemotherapy-resistant cells as well as CSCs in a personalized therapeutic approach are discussed.
Introduction
In 2018, according to the GLOBOCAN study, the malignant neoplasms with the highest mortality were lung (1.76 million deaths), stomach (783,000 deaths), liver (782,000 deaths), breast (627,000 deaths), and colorectal cancers (551,000 deaths) as well as blood cancers including leukemia (309,000 deaths) (1). All of these cancers are heterogeneous tumors containing cells with various stem cell properties, as described below. Already in 1877, Virchow's student Cohnheim noticed this cell population and pointed out that it possesses an embryonic character (2). Today, those cells are called cancer stem cells (CSCs) or tumor-initiating cells (TICs) and are seen as drivers of tumor establishment and growth (2–5), often correlated to aggressive, heterogeneous and therapy-resistant tumors (6, 7). Upon application of therapeutic regimens such as chemo- or radiotherapy the composition of tumor cell subpopulations changes (6, 8). At first, tumor cells with a high proliferative capacity are targeted and depleted causing a decrease in tumor size while CSCs survive (9). Additionally, some tumor cells will become senescent [therapy-induced senescence (TIS)], and subsequently can cause a change in the tumor microenvironment (TME) with tumor promoting effects due to the senescence-associated secretory phenotype (SASP) (6, 10–12).
It is well-known that CSCs are resistant to treatment and can cause tumor relapses (13). However, under the therapeutic pressure and changed microenvironment CSCs can be newly generated. In this case, these cells do originate from non-CSCs or from therapy-induced senescent tumor cells (14–18). It is therefore of importance to characterize these cells in detail and to understand their origin at the time of tumor initiation and tumor relapse.
This review underlines the role for a thorough investigation of tumors especially in the post-therapeutic period. Such post-therapeutic or therapy follow-up diagnostics are not conducted in the clinic on a regular basis, yet. The importance of specific biomarkers that analyze several parameters, such as CSCs phenotypes, senescence and TME composition, will allow the detection of therapy-resistant CSCs that cause tumor recurrence. A precise elimination of those cells of risk in a timely fashion using targeted cellular therapeutic approaches as the second line therapy is discussed in this study.
CSCs and Their Origin at Tumor Initiation
Tumor initiation can either be driven by transformed differentiated cells or transformed tissue resident stem cells (19) (compare Figure 1). The transformation can take place during tissue regeneration and can additionally, be initiated and/or accelerated as a response to infections, toxins, radiation or metabolic influences causing mutations (20, 21). During the transformation process, oncogenes are overexpressed and tumor suppressors are inactivated promoting uncontrolled growth of the cells (19). As a consequence, cells de-differentiate and acquire stem cell characteristics (19). The transformation of tissue resident stem cells or their progeny is believed to presuppose a different set of genomic changes allowing uncontrolled, niche-independent proliferation (5, 22). As stem cells already possess unlimited growth potential, it is believed that the transformation of stem cells and their progeny requires only few genomic changes (5, 22, 23). For example, the low mutagenic changes, identified in more than 10% of gastric cancers suggest that these tumors arise from tissue resident stem cell populations (24). Two stem cell populations have been identified in gastric cancers: slow cycling cells expressing the transcription factor Mist1 in the gastric corpus and Leucine-rich repeat-containing G-protein coupled receptor 5 (Lgr5)-expressing cells in the gastric antrum (25–27). Both populations have been linked to cancer generation in mouse models (24, 26, 27). In colon cancers, recent studies in mice have shown that even differentiated intestinal epithelial cells can be potential CSCs (28). The fact that adult differentiated cells, tissue resident stem cells or their progeny can promote tumor generation has also been shown in the liver. Cell tracking, in vitro and in vivo studies showed that liver cancer can originate from adult hepatocytes (29–32) as well as from hepatoblasts and hepatic progenitors (31, 32).
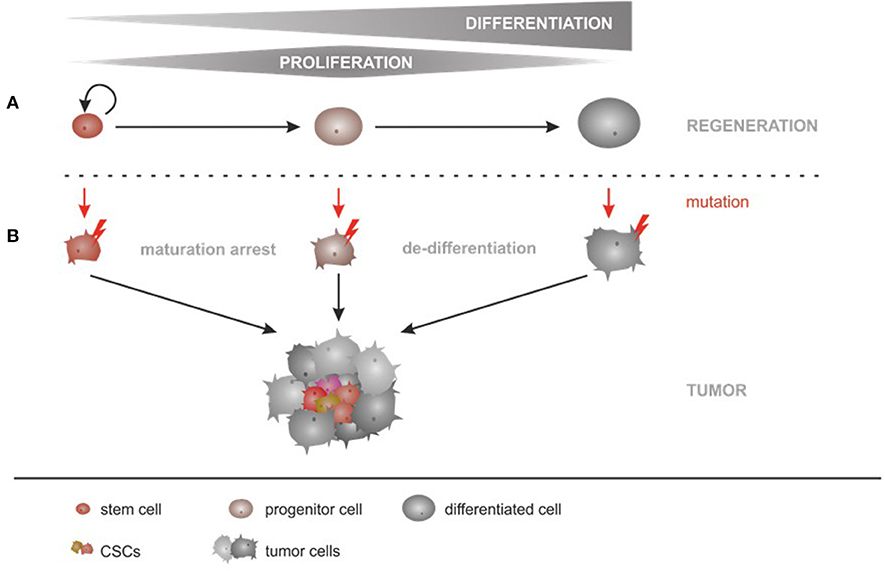
Figure 1. The origin of CSCs at tumor initiation: The two hypotheses of CSC generation. (A) The proliferation and differentiation of adult tissue resident stem cells is part of the physiological regeneration program that maintains tissue homeostasis. Adult tissue resident stem cells divide asymmetrically and generate transient amplifying cells, which possess a high proliferative capacity. These cells terminally differentiate; a process during which they will lose their proliferative capacity to finally support organ homeostasis. (B) Tumors can be generated by step-wise accumulation of several mutations (red lightening) that transform differentiated cells and cause a de-differentiation. Tissue resident stem cells as well as their progeny can accumulate mutations that lead to uncontrolled and niche independent growth. Heterogeneous tumors are generated. CSCs share phenotypic characteristics and several markers have been described in solid as well as in liquid cancers.
Tumor type, prognosis and aggressiveness are also influenced by the origin of the tumor, as analyzed for instance in breast cancers (33–35). Breast tumors originating from luminal progenitors are associated with a good prognosis, except those overexpressing Her2 (34, 36). Tumors originating from basal-like progenitors show a very aggressive phenotype (34).
In squamous cell carcinomas the differentiation phenotype seems to be influenced by the cell of origin and the kind of driver mutation, both responsible for the invasiveness and aggressiveness of the tumor (37, 38). Loss of the phosphatase and tensin homolog (Pten) as well as the liver kinase B1 (Lkb1) in lung epithelia causes tumor formation of highly penetrant tumors. These tumors are rarely metastatic and are characterized by a differentiated phenotype (37). Basal cells located within the trachea and main bronchi have been shown to self-renew and to form heterogeneous spheres (39). These basal stem cells can cause basal cell hyperplasia or epithelial hypoplasia, finally resulting in squamous cell metaplasia or dysplasia, which are discussed as precursors of squamous cell lung carcinomas (SCC) (39, 40). Studies by Fukui et al. suggest that high basal cell signatures correlate to a clinically aggressive phenotype in lung adenocarcinoma (40). Adenocarcinomas are considered to originate from sub-segmental airways of the bronchioalveolar stem cells or Type I and Type II pneumocytes (39). These bronchioalveolar stem cells are quiescent in healthy lungs but can enter proliferation cycles and could be targets of mutations causing transformation (39, 41). In mouse models, data indicate that small cell lung cancers (SCLC) can also originate from other cell types, i.e., neuroendocrine cells (42).
While in solid tumors the origin is heavily discussed, in hematological tumors the situation seems to be clearer. In acute myeloid leukemia (AML), the cell of origin is thought to be a hematopoietic stem or progenitor cell (43). However, a subgroup of human AML has been shown to share expression profiles with lymphoid T-cell progenitors. The authors showed that under oncogenic conditions, DN2 (double negative 2) T-cell progenitors process into lymphoid, biphenotypic, and myeloid leukemia cells (43–45). In chronic myeloid leukemia (CML), the cell of origin is characterized by the expression of the Bcr-Abl oncogene, generated from a chromosomal translocation between chromosome 9 and 22 (46, 47). This molecular aberration defines the chronic phase in CML, which progresses into blast crisis upon additional mutations that promote self-renewal (46, 47). While leukemic stem cells (LSCs) are well-defined and characterized in AML and CML, the concept of CSCs in acute lymphoblastic leukemia (ALL) and also in non-hodgkin lymphoma (NHL) is less established (48–50).
Tumors generated on the basis of CSCs are believed to follow a unidirectional hierarchy, in which only the CSC population is able to initiate tumor growth (51). At the time point of tumor initiation, it is suggested that cancer stem cells divide asymmetrically to maintain the CSC pool (52). These asymmetric divisions generate transient amplifying cells, which are undergoing symmetric divisions; therefore having a high proliferative capacity (51, 52). Based on recent data from hematological cancers (AML), the hierarchical model proposed by Bonnet and Dick (43) is most likely a simplified description. It is now believed that the organization of CSCs (in solid as well as in hematological cancers) is more complex (52–56). In contrast to the CSC model in which only a small subpopulation of cells is able to promote tumor initiation and growth, the clonal evolution model states that genetically unstable cells accumulate genomic and genetic alterations over time causing an increase in tumor aggressiveness, resistance and heterogeneity (5, 57). Both models are not mutually exclusive, which can be explained by the cellular plasticity (plasticity model) that suggests, that different cellular states can interconvert (as explained later) (5, 57).
Because CSCs have been shown to cause tumor initiation and tumor relapses, the search for biomarkers that characterize these cells and allow therapeutic as well as prognostic prediction or follow-up is ongoing. The most prominent markers of solid and hematological tumors are described in the following section.
Biomarkers for CSC Populations in Solid Cancers
In solid cancers, the clinical use of CSC specific biomarkers is very limited, besides the use of the carcinoembryonic antigen (CAE), fragments of the cytokeratin 19 (YFRA 21-1) (58) and the alpha-fetoprotein (AFP) that is expressed by cancer stem cells (58, 59). Importantly, most markers expressed in CSCs can also be found in adult tissue resident stem cell populations, human embryonic stem cells (hESC) or adult tissues (60). Additionally, most markers label heterogeneous stem cell populations pointing to the fact that their characterization and isolation has to be based on marker combinations using several surface markers or combinations of extracellular as well as intracellular markers; to identify and isolate cells that promote tumor initiation, resistance and relapse.
Below, a short summary of the most prominent markers is provided. CSC markers that could have potential usefulness within therapeutic, diagnostic, and prognostic approaches are pointed out (compare Tables 1–7) and focus on most deadliest tumors of lung, liver, breast, stomach, and colorectal as well as AML and CML. Tables 1–7 provide an extensive list of markers expressed in CSCs. A comparison shows that several markers are expressed in several tumor types.
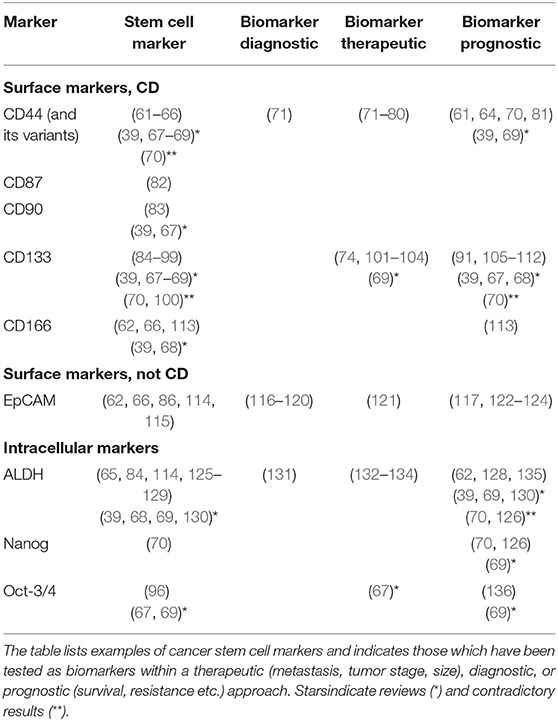
Table 1. Examples of lung cancer stem cell markers and their use as diagnostic, predictive, or therapeutic biomarkers.
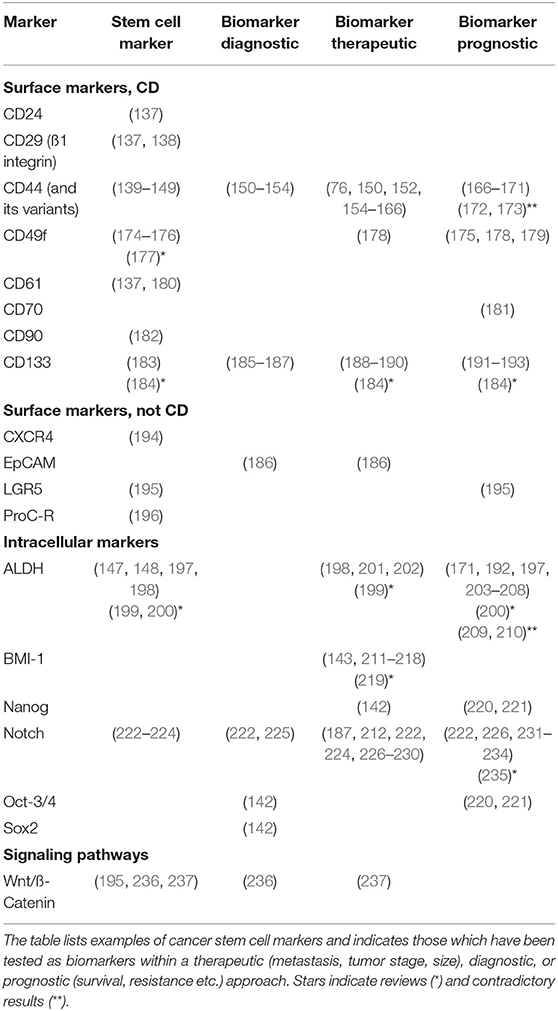
Table 2. Examples of breast cancer stem cell markers and their use as diagnostic, predictive, or therapeutic biomarkers.
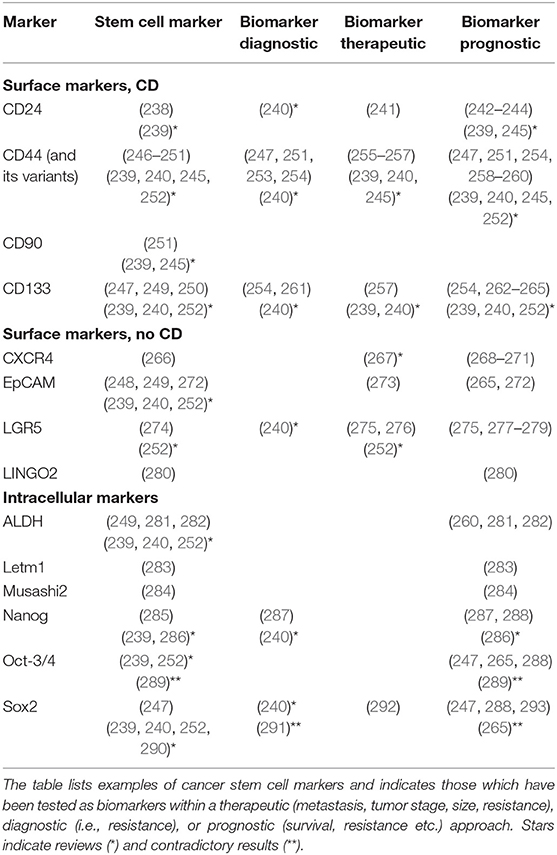
Table 3. Examples of gastric cancer stem cell markers and their use as diagnostic, predictive, or therapeutic biomarkers.
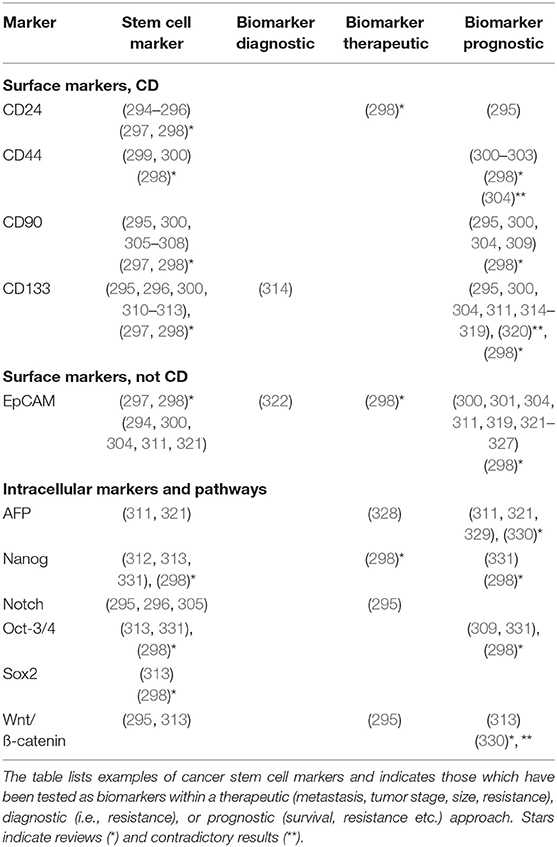
Table 4. Examples of liver cancer stem cell markers and their use as diagnostic, predictive, or therapeutic biomarkers.
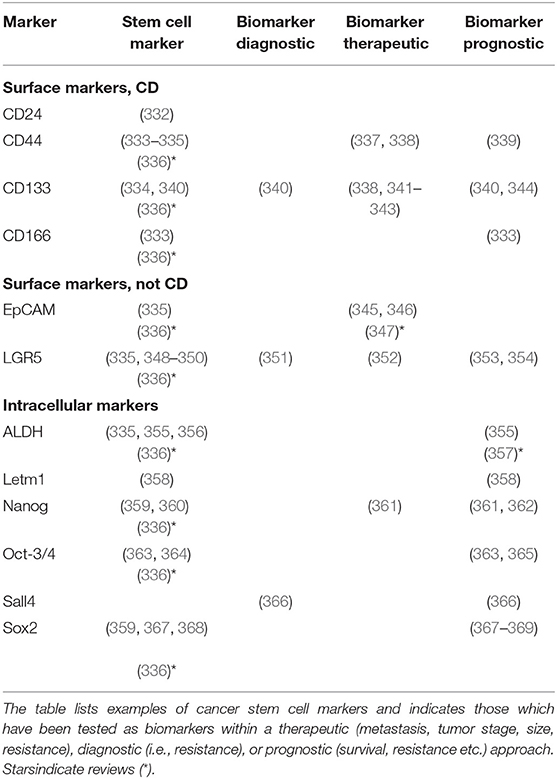
Table 5. Examples of colorectal cancer stem cell markers and their use as diagnostic, predictive, or therapeutic biomarkers.
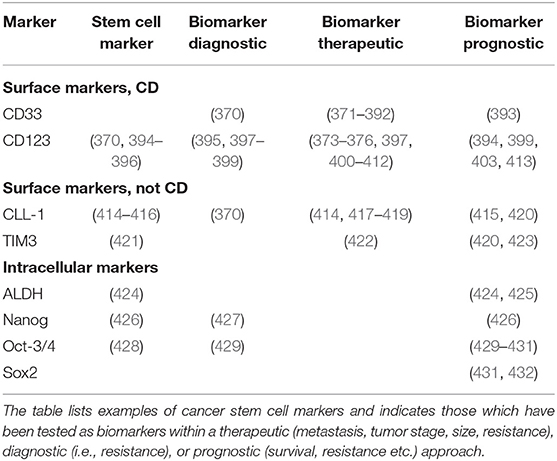
Table 6. Examples of AML cancer stem cell markers and their use as diagnostic, predictive, or therapeutic biomarkers.
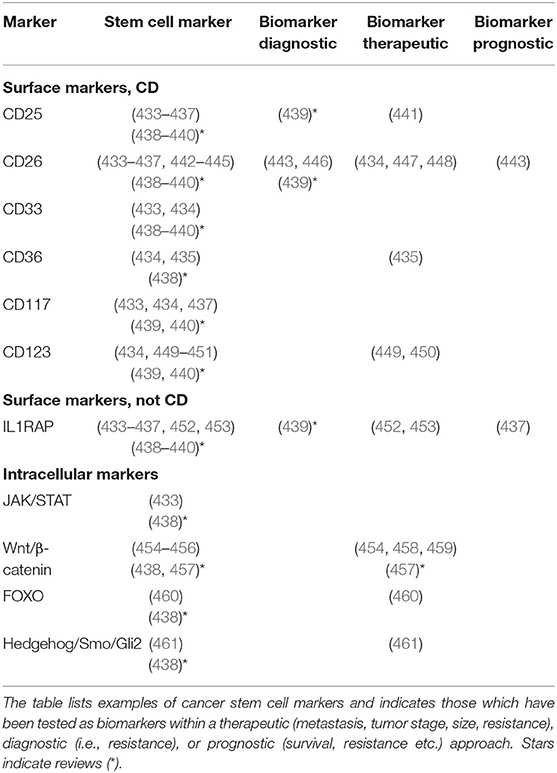
Table 7. Examples of CML cancer stem cell markers and their use as diagnostic, predictive, or therapeutic biomarkers.
CD44
CD44 is a biomarker which is not only expressed in solid but also in hematological cancers (see below). Its expression is associated with increased proliferation, self-renewal and metastasis (61, 149, 462, 463). For example, in colorectal cancers, expression of CD44/CD166 characterizes a cell population able to form tumor spheres, suggesting anchorage-independent proliferation of these cells (333). In other studies, CD44high/CD133high cells showed increased tumorigenic capabilities (334). Also in breast cancers, the percentage of CD44+/CD24−/CK+/CD45− cells was shown to be increased in malignant lesions compared to non-malignant lesions (139). A significant decrease in proliferation and migration of breast cancer cells was observed after the knock-down of CD44 (140). In gastric cancers, the knock-down of CD44 reduced sphere formation and caused decreased tumor growth in severe combined immunodeficiency mice (246). In many tumors (e.g., breast and liver), CD44 is expressed as isoform and its expression has been associated with increased cancer stem cell properties (141). In lung cancers, CD44v9 expression correlates significantly with early-stage lung adenocarcinoma and epidermal growth factor receptor (EGFR) mutations (464). Variants of CD44 are also expressed in gastric cancers and promote tumor initiation (248).
The CSC marker CD44 has been indicated as a biomarker for diagnostic, therapeutic, and prognostic approaches (compare Tables 1–5). In gastric cancer patients, CD44+ circulating tumor cells correlated with a poor prognosis (465). In colorectal cancers, a prognostic quantitative real-time PCR was established to analyze the expression of CD44v2 showing that a high expression correlated with a worse prognosis (339). In gastric cancers, the expression of CD44 and CD90 correlated with distant metastasis and could therefore be used as a diagnostic biomarker (251) and was suggested as a biomarker for treatment response (253). Therapeutic approaches targeting CD44 have been made using e.g. adenoviral delivery of siRNA in vitro (337). Furthermore, CD44-targeting drug conjugated aptamers (76) or hyaluronic acid coated onto nanoparticles have been in the focus of research (155). Antibody-based photosensitizer conjugates for combined fluorescent detection and photo-immunotherapy (PIT) of CD44-expressing cells in triple-negative breast cancers (TNBC) (150) or other antibody-based approaches tested in safety studies (466–468).
CD133
The biomarker CD133 (Prominin-1) is expressed on hESCs and rarely found on normal tissue cells (60). The marker has been additionally identified in tumors of breast, liver, stomach, and colon (compare Tables 1–5) and has also been described as a marker that characterizes cells with high tumorigenicity and a high ability to form spheroids (184, 469). In breast cancers, its expression correlates with N-cadherin expression that was found to be significantly higher in patients with metastasis (191). In lung cancers, the expression of CD133 has been correlated to epithelial to mesenchymal transitions (EMT), in combination with other additional stem cell markers, such as BMI1 (84).
The expression of CD44 and CD133 in colorectal cancers can predict metastasis (470), however, no correlation to patient outcome could be detected (471). In breast cancers, CD133 mRNA was suggested to be suitable for prognosis prediction (193, 472) and CD133 protein has been correlated to a poor prognosis (193). Pre-clinical therapeutic approaches cover antibody-based targeting of colorectal (341, 342) as well as breast cancers (188) (compare Tables 1–5).
EpCAM
The epithelial cell adhesion molecule (EpCAM, CD326) is expressed on CSCs in various tumor types including colon and hepatocellular cancers (473–476). Furthermore, it is expressed in non-transformed tissues such as epithelial cells (476), and various stem and progenitor cells (477, 478). EpCAM is involved in proliferation and differentiation as well as in cell signaling and formation and maintenance of organ morphology (479). In cancer tissue, EpCAM is homogeneously expressed on the cell surface, while in epithelia it is expressed on the basolateral side (476).
In breast cancers, the expression of EpCAM is correlated to CSC-like phenotypes that promote formation of bone metastases in mice (480). In lung cancers, the expression of EpCAM is often associated with the expression of CD44 and CD166. Triple positive cells show increased clonogenicity, spheroid formation, self-renewal capacity, and show increased resistance to both 5-fluouracil and cisplatin (62).
As one of the first CSC markers, EpCAM has been evaluated as a therapeutic biomarker (compare Tables 1–5). Targeting EpCAM with different antibody formats has been performed in colorectal as well as breast cancers (347). In colorectal cancers, a therapeutic approach targeting EpCAM+ cells with aptamers has been performed in pre-clinical conditions (345, 346).
Intracellular Biomarkers as Regulators of Stemness in Solid Cancers
Both embryonic and CSCs show unlimited growth, invasive capacity and are characterized by an undifferentiated cellular state (481). This feature depends on transitions between epithelial and mesenchymal states, regulated by a network of intracellular pluripotency transcription factors. As reviewed by Hadjimichael et al. and also described by others pluripotency in ESC is regulated by a core-network of transcription factors, consisting amongst others of Oct-3/4, Sox2, Nanog, Klf4, and c-MYC as well as signaling pathways such as the Jak/Stat, Wnt/ß-catenin, Hedgehog/Notch, TGF-ß as well as FGF signaling pathways (367, 482, 483). The core-pluripotency network consisting of Nanog, Oct-3/4 and Sox2 (described in detail below) activates genes of self-renewal and suppresses genes involved in differentiation (482). Pluripotency factors as well as signaling pathways have been indicated as biomarkers for CSCs as shortly described below (compare Tables 1–5). Of note, the tables do not include all biomarkers, however describe the most abundant ones reported in the literature.
Sox2
The transcription factor Sox2 belongs to the SRY-related HMG-box (SOX) family, and is involved in the maintenance of an undifferentiated cellular phenotype (367). Its aberrant expression in cancers often leads to increased chemotherapy resistance and asymmetric divisions, as observed in colorectal cancers (368). In those, Sox2 expression correlates with a stem cell state and with a decreased expression of the caudal-related homeobox transcription factor 2 (CDX2), which could serve as a prognostic marker for a poor prognosis (367, 368). In gastric cancers, expression of Sox2 correlates with the tumor stage as well as with a poor prognosis (247, 288). The formation of tumor spheroids in vitro also correlates to the overexpression of CD44 and CD133 as well as the transcription factors Sox2, Nanog and Oct-3/4 (247). However, in another study, Sox2 levels were downregulated in gastric cancers in comparison to normal tissue and high Sox2 expression correlated with decreased metastasis and a better prognosis for the patient due to increased p21 levels (293). Therefore, the oncogenic functions of Sox2 are controversially discussed in gastric cancers, in which Sox2 might also have tumor-suppressor functions. These different functions seem to depend on the cancer origin and cellular context (484).
Oct-3/4
Oct-3/4, also known as POU5F1, belongs to the POU homeobox gene family and is also a regulator of pluripotency in mammalian stem cell population. Oct-3/4 is upregulated in several cancers and may support the neoplastic transformation and resistance (485). In colorectal cancer cells, Oct-3/4 causes increased migration and liver metastasis (363, 486) correlating with poor survival (365). As reviewed by Prabavathy et al. Oct-3/4 expression is correlated to increased self-renewal and metastasis in lung cancer cells (67). A meta-analysis showed that Oct-3/4 expression in lung cancer was associated with poor outcomes concerning the differentiation degree, the TNM Classification of Malignant Tumors (TNM) and lymphatic metastasis (136). In hepatocellular carcinoma (HCC) Oct-3/4 expression was correlating with tumor size and recurrence (309).
Nanog
Nanog is a homeobox domain transcription factor widely expressed in human cancers (487). In colorectal tumors its expression was significantly increased in CD133+ cells, and on the basis of a univariate analysis, Nanog expression correlated linearly to liver and lymph node metastasis and the TNM stage. It might therefore be useful as a prognostic biomarker in post-operative liver metastasis (362). In breast cancer, expression of Nanog and Oct-3/4 has been correlated to a poor prognosis of the patient as well as EMT (220, 221). In HCC cell lines Nanog expression drives selfrenewal and invasion, metastasis, and drug resistance (298).
Biomarkers for CSC Populations in Hematological Cancer
CSC biomarkers of AML and CML have been listed in Tables 6, 7. They indicate commonly used markers and point out possible functions of these markers as biomarkers in prognosis, therapy, and diagnosis. Below a short introduction of the most relevant markers is given.
CD44
As mentioned above, CD44 is a common marker shared by many cancers (60). In hematological cancers, CD44 expression is functionally associated with chemotherapy resistance (488, 489). The expression of CD44 in AML is significantly correlated with a poor overall survival (OS) (490). Furthermore, CD44 was shown to be significantly higher expressed in non-remission AML patients (490). A highly relevant function of CD44 for LSCs is the adhesion to the bone marrow niche (491).
CD123 and CD33
In hematological malignancies, such as AML, CD123 as well as CD33 have been described as the “classical” CSC markers (492, 493). CD123 is a marker expressed on LSCs (395, 397, 494), but not exclusively (395, 398). In AML patients, CD123 expression correlates to the therapy response rate (413, 495), the relapse risk (403), and a shorter disease-free period and OS (399, 413). CD123 has been associated with increased proliferation and differentiation (494, 496).
CD33 is historically, the most commonly used marker for AML stem cells, with clinical implementation of CD33 targeting, dating back to the Food and Drug Administration (FDA) approval of gemtuzumab ozogamicin (GO) in 2000 (497). CD33 is highly expressed on blasts in around 85–90% of AML patients (433, 438, 497) and also expressed at higher densities in CML (433, 438) but less on healthy hematopoietic stem cells (HSCs). These cells are additionally characterized by expression of CD25, CD26, and Interleukin-1 receptor accessory protein (IL-1RAP) and also other markers (440).
CLL-1
The C-type lectin-like molecule-1 (CLL-1) is a promising alternative to the “classical” LSC targets (414). The majority of AML patients shows CLL-1+ LSCs, a marker not being expressed on HSCs (370, 414–416). Compared to CD33, CLL-1 was not only more frequently and stronger expressed on LSCs, but also not or more weakly expressed on normal tissues leading to reduced off-target effects after treatment with a respective antibody-drug conjugate. Therefore, CLL-1 might be a more suitable and specific LSC target than CD33 (414). A high expression of CLL-1 is associated with poor prognosis (420) and a faster relapse (415) in AML. Interestingly, controversial observations have been made using CLL-1 as a biomarker after chemotherapy. The diagnostic value of CLL-1 is discussed controversially: while Zhang et al. showed that CLL-1 was increased after chemotherapy (371), others showed that there is no difference between CLL-1 expression at diagnosis and at relapse (415) or even detected a decreased CLL-1 expression at relapse (370). The relevance of CLL-1 as a prognostic biomarker for chemotherapy failure or relapse is therefore still unclear. Its expression is not detectablewithin the chronic phase of CML (440).
TIM-3
Another “non-classical” LSC biomarker is T-cell immunoglobulin and mucin 3 (TIM-3), that is highly expressed on LSCs but not expressed on healthy HSCs (498). It is correlated to a poor prognosis (420) and treatment failure (423). Stem cell properties of TIM-3+ cells were confirmed by engraftment in a xenograft mouse model (421).
Intracellular Biomarkers as Regulators of Stemness in Hematological Cancers
The core-network of pluripotency associated transcription factors as well as signaling pathways have also been analyzed in hematological cancers. Fifty AML patients have been analyzed for the expression of Sox proteins, which are overexpressed in 10–22% of the patients. The analysis showed that high levels of Sox proteins may have a prognostic value (432). The analysis of Oct-3/4 expression correlated with an unfavorable prognosis and is associated with FMS-like tyrosine kinase 3-internal tandem duplications (FLT3-ITD) (430).
Activation of stemness-associated pathways especially in CML has been shown to promote extensive proliferation and has been linked to the onset of blast crisis, which is associated with a loss of differentiation of the leukemia initiating cells. An important impact on this effect has the Wnt/ß-catenin pathway (46) that promotes HSC proliferation, independent of the bone marrow niche (5, 22, 499). Especially, resistance to the tyrosine kinase inhibitor imatinib has been shown to correlate to an increased nuclear localization of ß-catenin (454, 458, 500). Inhibitors targeting the Wnt pathway have been shown to be of advantage for example in long-term cell cultures (500). Additionally, the hedgehog pathway has been suggested to be involved in chemotherapeutic resistance in CML, which is also characteristic for chronic phase CML cells (47). Mouse studies also indicate the involvement of the hedgehog pathway (46, 47), which has been implicated as a therapeutic biomarker for CML (456, 461).
To summarize, CSCs at tumor initiation originate from either differentiated cells or adult tissue resident stem cells (5, 19, 22). Several data indicate that the origin strongly correlates to the aggressiveness of the tumor. Therefore, extra- and intracellular biomarkers that characterize CSCs have been identified and implemented to be of diagnostic and prognostic advantage. However, stem cells are subject to a high degree of plasticity modulated by the TME (19), that is significantly changed by chemo- and radiotherapies and composed of several different cell types. In the following section, focus will be laying on senescent tumor cells as part of the TME as they have long-term influence on TME and CSC development and progression.
The Escape of Cancer Stem Cells From Therapy
At the moment first-line therapeutic treatments in progressed tumors include in the most cases surgery, chemo- as well as radiotherapies (501) (compare Figure 2). Those have been shown to induce DNA damage and to trigger senescence in cancer cells, a process known as therapy-induced senescence (TIS) (10, 502, 503). TIS will cause a decreased tumor size and attracts immune cells such as neutrophils, monocytes as well as T-cells toward senescent tumor site (503). However, over a long-term period the anti-tumorigenic effects of TIS are lost and the cancer might gain stemness causing tumor relapses (Figure 2).
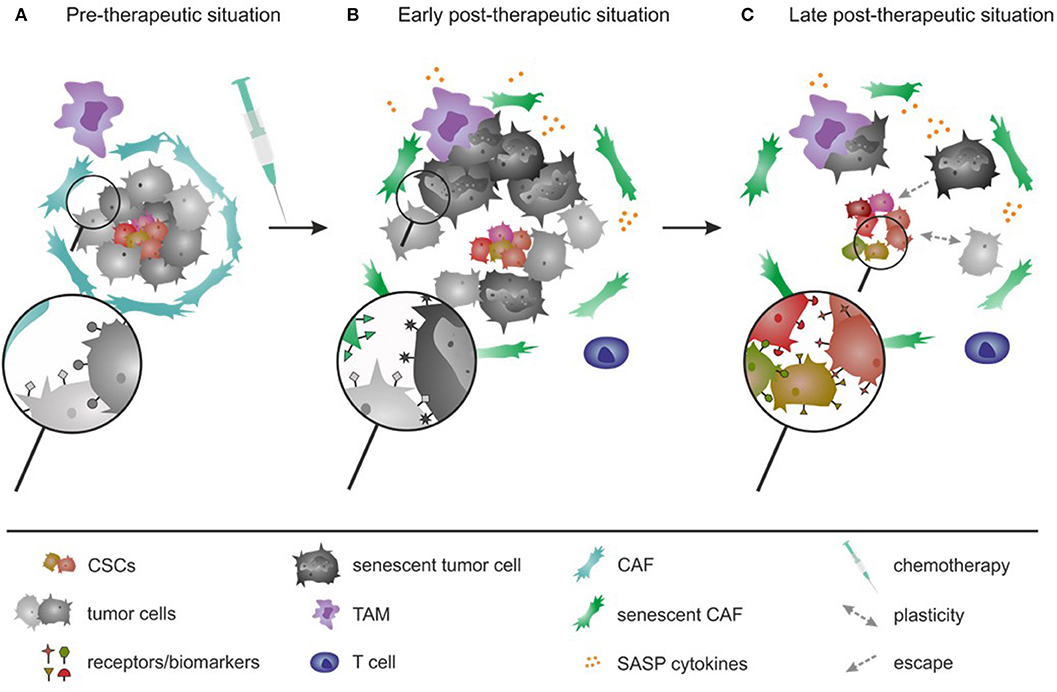
Figure 2. Kinetic of tumor development in pre-, early-, and late-therapeutic period upon application of chemo- and/or radiation therapy: current situation in the clinic. (A) In the pre-therapeutic situation, heterogeneous tumors are composed of several cell types, including CSC, tumor cells, TAMs, and CAFs; all characterized by biomarkers. (B) In the early post-therapeutic period, upon application of the first-line treatment (that currently uses mostly chemo- or radiotherapeutic regimens) several important changes occur in the tumor, in particular: tumor cells or CAFs die due to the therapy or become senescent, whereas CSCs mostly survive the treatment. Senescent cells (tumor cells and CAFs) attract immune cells toward the senescent site via SASP. The SASP therefore plays a positive role and attracts immune cells in this early post-therapeutic situation. Attracted immune cells promote the clearance of dead, of necrotic, and senescent tumor cells and CAFs. (C) In the late post-therapeutic situation uncleared senescent tumor cells and senescent CAFs and SASP thereof play a negative (pro-tumorigenic) role and support tumor development. SASP molecules provide stimulating factors for CSCs for further uncontrolled proliferation as well as their maintenance. Also, remaining senescent tumor cells acquire additional mutations that promote activation of a stemness phenotype and finally a tumor relapse.
Therapy-Induced Senescence: Its Hallmarks, Biomarkers, and Its Role in CSC Generation
Agents that induce DNA damage such as chemo- and radiation therapies have been identified to trigger senescence in differentiated cancer cells (10). TIS has been in the research focus, because it significantly contributes to the long-term outcome of patients (12). The DNA damage response ultimately activates one or several tumor suppressors pathways [p53, p16 (Ink4a), p21 (Waf1), and retinoblastoma (RB)], that trigger and maintain the senescence growth arrest (504). However, it is important to mention that the senescence phenotype can also be induced in cancer cells which lack functional p53 and RB protein (504). TIS and senescence in general, are recognized as a double-edged sword, that on the one hand leads to the attraction of immune cells, inflammation, and elimination of senescent tumor cells and correlates with a positive post-treatment prognosis and treatment outcome (505–507). On the other hand, senescence can play a strong pro-tumorigenic role that supports CSC generation, as described below.
Senescent cells are characterized by biochemical and morphological changes such as flattening and/or nuclear enlargement (508). There are several classical biomarkers of cellular senescence and they comprise: senescence-associated beta-galactosidase (SA-ß-gal) activity, expression of p53 protein, the amount of p53 in the nucleus, increase in expression of p14 (Arf), p16 (Ink4a) and p21 (Waf1), SASP, and often senescence-associated heterochromatic foci (SAHF) (12, 505, 507, 509–515). Furthermore, senescent cells display low Ki67 levels and show levels of heterochromatin protein 1 (HP1) gamma (516), as well as di- or tri-methylated lysine 9 of histone H3 (H3K9Me2/3) and histone H2A variant (macroH2A) (505, 517, 518). The usefulness of telomere length as a biomarker of senescence has been questioned (505).
Biomarkers that underline the effect of a therapeutic approach based on the induction of senescence have to be evaluated carefully and quite often simultaneously. The investigation of senescence markers after post-operative chemotherapy in muscle-invasive bladder cancer (MIBC) revealed that the simultaneous expression of several markers involved in the p53 pathway has to be checked to correctly assess the pathological outcome of MIBC (509). The analysis revealed that the expression of p14 (Arf) was associated with an impaired response to chemotherapy and poor prognosis, whereas p21 (Waf1) expression was related to reduced tumor cell proliferation (509).
TIS can play an anti-cancerous role (503). As demonstrated in our studies in premalignant and malignant liver disease, the induction of senescence leads to a so-called “senescence surveillance” mechanism, which relies on innate and adaptive immune cells. These cells clear senescent premalignant cells, thereby protecting premalignant liver from cancer development (535, 536). Interestingly, in further studies, we could show that the chemokine (monocyte chemoattractant protein 1, MCP-1) axis is of importance for the induction and maintenance of senescence and for the sufficient immune surveillance in the liver (525). Several biomarkers of senescence were found to correlate with a disease-free survival or with an improved OS in several solid cancers (516, 524). One such indicator, a lysosomal-beta-galactosidase (GLB1) that hydrolyzes beta-galactose from glycoconjugates and represents the origin of SA-ß-gal, was reported as a reliable senescence biomarker in prostate cancer (516). Inhibition of the cyclin-dependent kinase 4/6 (CDK)-RB pathway by a novel drug, SHR6390, resulted in reducing the levels of Ser780-phosphorylated RB protein and correlated with the G1 arrest as well as with cellular senescence in a wide range of human RB+ tumor cells in vitro (520). Xiang et al. identified seven senescence-associated genes (SAGs, Table 8) significantly decreased in senescent cells and increased in HCC tissues (524). Interestingly, those SAGs were strongly associated with OS, especially in Asian populations, and had a better predictive accuracy in comparison to serum AFP in predicting OS of HCC patients (524). Recently, Smolle et al. reviewed and underlined the role of members of the inhibition of growth (ING) family. These act as tumor suppressors, regulating cell cycle, apoptosis, and cellular senescence. The authors proposed them as clinically useful biomarkers in the detection and prognosis of lung cancer (523).
In line with the positive role of senescence, evidence exists regarding the role of TIS in turning “cold” tumors toward a “hot” phenotype that results in activating immune responses against tumor antigens (503). As reported in Her2+ breast cancer patients treated with Trastuzumab and chemotherapy, the treatment-induced epitope spreading was characterized by increased antibody responses not only to the tumor antigen Her2, but also to endogenous CEA, insulin-like growth factor-binding protein 2 (IGFBP2), and p53 (521).
TIS is a very important protective mechanism that is induced immediately after chemo- or radiation therapy. TIS mediates the recognition and clearance of senescent tumor cells by immune cells (503, 535). Induction of TIS after the therapy is associated with a better prognosis and OS (524). However, if senescent tumor cells are not cleared in a timely fashion, their role at a later time points shifts from positive to negative, as discussed in the section below.
Negative Role of TIS: Cancer Progression
Several studies report a pro-tumorigenic effect of TIS leading to cancer recurrence and support of tumor development (503). Uncleared senescent cells acquire additional mutations, thereby escaping the cell cycle arrest and transform into malignant cells (536). Moreover, factors secreted by senescent cells are also reported to play a strong tumor-promoting role (526).
Was et al. suggested that senescent human colon cancer cells (HCT116) that appear during a doxorubicin-based therapy enter a “dormant” cellular state, survive the treatment, and cause tumor re-growth (537). Importantly, the recent findings by Scuric et al. suggest a long-term effect of chemotherapy and/or radiation exposure upon TIS (11). In this study, markers of cellular senescence, including higher DNA damage and lower telomerase activity, were observed many years later in breast cancer survivors (11). Elevated levels of a soluble tumor necrosis factor (TNF)-receptor-II, a pro-inflammatory biomarker and one of the main SASP molecules, were also detected (11). A negative effect of SASP was correlated to a p53 single-nucleotide polymorphism (SNP) at codon 72 which is correlated to increased risk of breast cancers (538). Using a humanized mouse model, Gunaratna et al. showed that SASP caused an increased invasion of pro-inflammatory macrophages (522). However, the inflammation proceeded into a chronic inflammation with pro-tumorigenic action and tumor-associated macrophages (TAMs) contributed to angiogenesis and increased tumor growth rates (522). Also, senescent cancer-associated fibroblasts (CAFs) and, in particular, expression of Caveolin-1 (CAV1) promote tumor invasion in pancreatic cancer (539). Moreover, in clinicopathological characteristics of patients, a high CAV1 expression directly correlates with higher levels of serum tumor antigens, with the rate of advanced tumor stage, and with significantly worse outcomes in both overall and disease-free survival (539).
It has been suggested that cancer therapies, especially chemo- and radiotherapies, possess long- and late-term pro-tumorigenic side effects and could therefore contribute to the relapse of the malignant disease they were intended to treat (540). Such long-term effects could be caused by the decreased removal of senescent cells, as described below.
Cancer Stemness: Senescence Escape
As mentioned above, cells undergoing senescence can still escape the senescence program and become malignant while acquiring additional mutations (519, 535, 536) (Figure 2). In our studies, we observed a spontaneous mutation [a deficiency in p19 (Arf)] in Ras-expressing hepatocytes, which resulted in a full-blown HCC development using a Ras-induced precancerous liver disease model (535, 536). The reversibility of TIS can be caused through the inactivation of tumor suppressors p53, p16 (Ink4A), p19 (Ink4d), and/or RB (504, 507, 519). Additionally, the over-expression of CDC2/CDK1 and survivin can promote cancer stem cell survival and can also promote the development of polyploidy (507). In general, mutations in CDKN2A, coding for p16 (Ink4a, CDKN2A), p21 (Waf1, CDKN1A), and p27 (Kip1, CDKN1B) as well as E2F3 and EZH2, and a high c-MYC expression might result in low percentages of senescent cells (504, 519). Moreover, particular mutations completely protect melanoma cells from cell cycle arrest upon chemotherapy: DMBC29 melanoma cells that carried a EZH2S412C mutation, expressed c-MYC at a low level and a wild type of CDKN2A did not undergo senescence, in contrast to many melanoma cells treated with vemurafenib and trametinib (519).
An escape of cells from senescence was also identified by Milanovic et al. in B-cell lymphoma studies (14). In those studies, the researchers showed that senescent cells substantially upregulated an adult tissue stem cell signature and activated Wnt signaling (14). This senescence-associated stemness was an unexpected cell-autonomous phenotype that caused the generation of cells with a higher tumorigenic potential in vitro (14).
However, escape from senescence is not the only pathway that promotes an increase in the cancer stemness phenotype. Stemness within the tumor tissue is also regulated indirectly by signaling molecules which support the maintenance of stemness in CSCs as well as non-CSCs, as described in the following sections.
Cancer Stemness: SASP and CSC Maintenance
The stemness phenotype within a tumor can also be mediated via SASP (526). Several studies address the strong pro-tumorigenic phenotype (526) whose cytokines can mediate the maintenance of CSCs. The most prominent interleukins (IL) of SASP are IL-1,−6, and−8 (526). These cytokines can influence the CSC phenotype and functionality and therefore influence the plasticity phenotype of CSCs.
Using breast cancer cell lines, Di et al. showed that an induction of senescence in mesenchymal stem cells by hydrogen peroxide treatment causes an increased secretion of the inflammatory cytokine IL-6, which led to a higher migratory capacity of breast cancer cells in vitro as well as in xenotransplants (541). An increase in the aggressive metastatic chemoresistant phenotype upon inflammatory cytokine stimulation such as IL-1ß, IL-6, and RANTES (regulated on activation, normal T cell expressed, and secreted) was also observed by others (533, 534). Our own work indicated that IL-8 blocks differentiation of hepatocellular premalignant cells, a pathway mediated via mammalian target of rapamycin complex 1 (mTORC1) kinase, that causes an increase in chemotherapy resistance (532). An increase in tumorigenicity and EMT of breast cancer cells has been shown to correlate to an increased expression of CD44 or CSC-like properties and be caused by the senescence-associated IL-8 and IL-6 (527–529). Pathways that might be involved in such cellular reprogramming processes are the JAK2/STAT3 signaling pathway (542), the IL-6/STAT3 and NOTCH cross-talk signaling (187, 530) as well NFκB-IL-6 signaling axis, responsible for the generation of CSCs (531). Interestingly, interference with those pathways by aspirin increased chemosensitivity and decreased self-renewal in breast cancer cells (531). In colorectal cancer cells the inflammatory cytokine IL-6 mediates deacetylation, which subsequently activates NANOG transcription and accumulation of stemness phenotypes, correlating with malignant progression and poor prognosis (543).
To summarize, TIS on the one hand has positive effects that eliminates differentiated tumor cells and also causes invasion of immune cells with anti-tumorigenic functions. On the other hand, senescence causes negative effects that are reflected by pro-tumorigenic functions causing CSC development and a gain of cancer stemness (Figure 2).
An additional level of complexity is added by the plasticity of CSCs as well as non-CSCs, which also causes increased cancer stemness, resistance, and relapse. Examples are given in the next paragraph.
Cancer Stemness: Plasticity of CSCs and Non-CSCs
Cancer stemness is not only triggered by senescence escape and acquisition of stemness phenotypes or supported by maintenance of stemness (544) but also by the plasticity of CSCs and non-CSCs, altogether causing tumor relapses after treatment, as described below.
Plasticity is regulated by the TME that is very heterogeneous and consists of CAFs, TAMs, and neutrophils as well as of cancer-associated adipocytes, tumor-infiltrating lymphocytes, and cancer cells with or without stem cell characteristics (545). Therefore, a clear separation between SASP effects and plasticity cannot be made as several direct and also indirect regulatory networks are involved (Figure 2).
Mechanistically, plasticity of cells is a characteristic that ensures robust tissue regeneration and homeostasis (546, 547) and describes the phenotypic and molecular changes of tumor cells increasing stemness and reflecting the tumor's ability to self-renew (18, 548). This phenotype is ultimately closely linked to EMT (15, 548). As described, the transition from the epithelial to mesenchymal state is associated with defined regulatory networks, chromatin remodeling and gene expression programs that are specific to the epithelial, mesenchymal or hybrid cellular state (15–18). Plasticity increases the complexity by suggesting that CSCs can switch between different cellular states, characterized by the expression of surface markers as well as transcription factors (18, 56). Examples for this come from the analysis of different tumor cells: Chaffer et al. demonstrated that CD44low cells (non-CSCs) can switch to a CD44high phenotype (CSCs) resulting in mammosphere formation, a phenotype that could be induced by upregulation of the zinc finger E-box binding homeobox 1 (ZEB1) protein expression induced by TGF-ß (548), which is a major cytokine of the TME (545). In NSCLC cell lines, two distinct CSC subpopulations have been described by expression of CD133 and the aldehyde dehydrogenase (ALDH) (549). ALDHs compose an enzyme superfamily with metabolic functions. The analysis of its activity is often used to identify CSCs (550, 551). Analyzing CD133 and ALDH activity, Akunuru et al. separated cancer stem/progenitor cells (CD133+, ALDHhigh) from non-CSCs (CD133− or ALDHlow) and showed that non-CSCs can interconvert into CSCs. The latter process is activated by TGF-ß signaling or signaling by the zinc finger protein SNAI (Snail) transcription factor family. The described process underlines the dynamic plasticity of CSC/non-CSCs cells (549). After TGF-ß treatment, the authors observed an increase in IL-1ß and IL-6 as well as an increase in CD133+ and ALDHhigh subpopulations (549).
Interferon-ß (IFN-ß) as well as Oncostatin M (OSM), also cytokines within the TME, have been shown to regulate CSC phenotypes (552). Activation of IFN-ß signaling pathways in non-CSCs blocks the expression of CD44 and Snail, which causes a decrease tumor sphere formation and additionally inhibits invasion (552). In contrast, OSM induces a stemness phenotype in non-CSCs (552). One of the major regulators of colorectal tumor plasticity (either CSCs or cancer cells) are the Wnt-ß-catenin and the KRAS/BRAF/ERK pathways, which have been implicated to regulate tumorsphere formation, self-renewal as well as resistance, as reviewed by Pereira et al. (553) and Zhan et al. (554). Activation of Wnt-signaling increased sphere and clone formation as well as drug resistance (555, 556). Acquisition of stemness was also described by Perekatt et al. using transgenic mice to analyze the function of Wnt-signaling in tumorigenesis and de-differentiation in the gut (28). The authors show that the inactivation of Smad 4, a factor that regulates the differentiation program, promoted the development of adenomas with characteristics of activated Wnt signaling over long-term periods (28). Such Wnt activation can correlate with increased treatment resistance as reviewed by Mohammed et al. (557). Also in gastric cancer, activation of the Wnt pathway causes an increase in CD44 as well as Oct-3/4 expression and correlates with an increased proliferation (558).
As described above, a gain of stemness due to SASP and CSC maintenance or by plasticity of CSCs and non-CSCs, can cause increased resistance (Figure 2). CSCs (pre-existing or post-therapeutically generated de novo) can escape the treatment by the expression of drug exporters and detoxification proteins, entrance into dormancy as well as resistance to DNA damage induced cell death (4, 15, 185, 559, 560). Their survival causes tumor relapses (Figure 2). To interfere with the relapse, several strategies have been under investigation to block CSC resistance and growth (9, 13), as described below (Figures 3, 4).
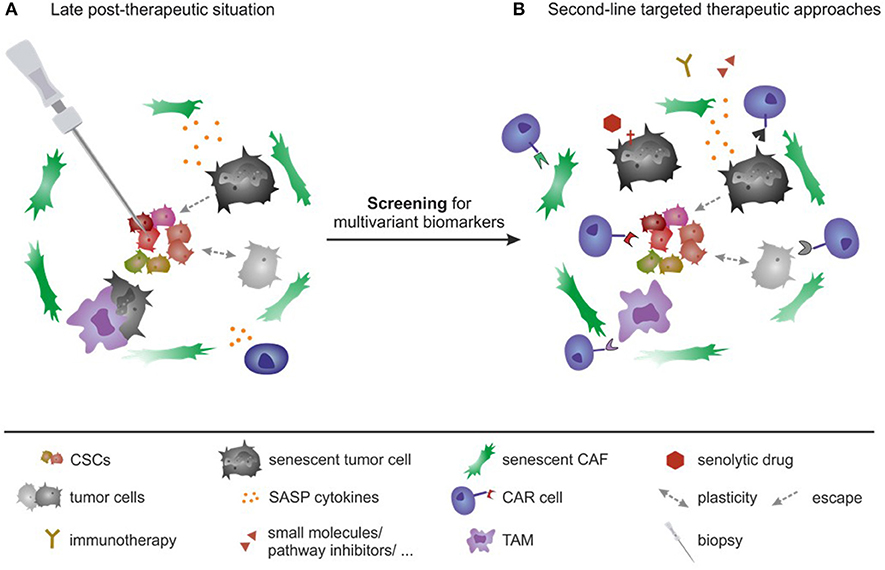
Figure 3. Targeted personalized second-line therapy as a future perspective. (A) Analysis of post-therapeutic biopsy samples: follow-up studies need to be included into regular clinical post-therapeutic relapse analysis. After therapy, local biopsies of remaining tumor tissue and/or satellite tissue should be taken periodically (even after several years post-therapy) and a multivariant analysis for biomarkers has to be performed, including the analysis of CSC biomarkers, pro-inflammatory cytokines, senescent markers as well as markers for CAFs. (B) Targeted second-line therapy needs to be performed based on the analysis described in (A) and will include a specific targeted eradication of remaining cells that could promote tumor relapse and metastasis. Targeted therapies comprise CAR-based approaches targeting CSCs as well as senescent cells or CAFs and TAMs. They also include senolytic drugs to deplete senescent cells independent of CAR approaches.
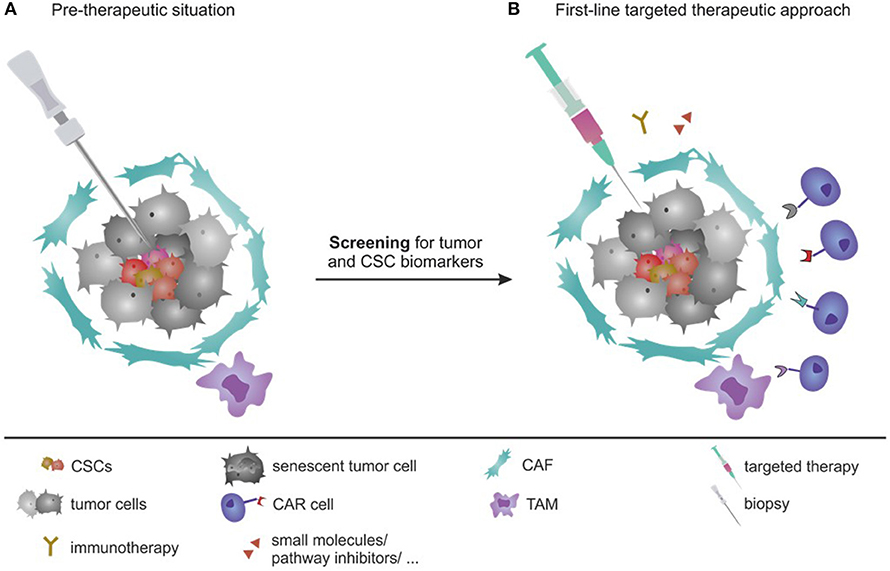
Figure 4. Targeted personalized first-line therapy as a future perspective. (A) Pre-therapeutic period: local biopsies before the therapy would allow to determine the heterogenic composition of the tumor, consisting of several biomarkers to be analyzed (CSC, CAFs, and TAMs biomarkers, tumor cell antigens, as well as e.g., T-cell compositions). (B) First-line targeted personalized therapeutic approach—therapeutic regimens could combine several approaches: the chemotherapy and small molecules (both selected based on tumor genotype), combined with immunotherapies (antibodies and checkpoint inhibitors based on tumor and analysis of T-cell phenotype), as well as CAR cell-based therapies targeting CSCs, CAFs, and TAMs. Combination therapy will allow a precise and efficient targeting of the heterogenic tumor composition from the beginning on.
Eradication of CSCs: New Targeted Approaches
Targeting CSCs has been in the focus of research for many years (13). As reviewed by Shibata and Hoque, the combination of CSC-targeted therapies and conventional non-targeted therapies can result in a decreased chemoresistance (9). Approaches of CSC-targeted therapies include kinase inhibitors as well as targeting stem cell associated pathways such as Wnt and β-catenin, some of which have already entered the clinical phase (9, 13). Immunological approaches that target CSCs via MHC-restricted killing include adoptive cell transfer, targeting checkpoint inhibitors as well as antibody-based approaches and vaccination. MHC-unrestricted killing based on NK-, γδT-, and chimeric antigen receptor (CAR) T-cell approaches have been established (561, 562). Currently, these approaches are performed after failures of the first-line therapies.
Based on the promising results of CAR T-cellular therapy in treating hematological diseases, CAR T-cell-based approaches have also moved forward into the therapy of solid cancers (563, 564). Although, CAR T-cell-based approaches face difficulties in treating solid cancers, their therapeutic use could be a promising alternative (563, 564).
CAR Therapies Targeting CSCs
Targeting CD133+ CSCs
Targeting CD133+ CSCs in solid cancers has shown quite promising preclinical results either using monotherapeutic approaches (565, 566) or using combinational approaches together with cytostatic agents (567). Recently, a clinical trial testing CD133-directed CAR T-cells in patients with ALL, AML, breast, brain, liver, pancreatic and ovarian cancers as well as colorectal cancers has been completed (NCT02541370, Table 9). Initial results showed feasibility, safety, and efficacy of CD133-directed CAR T-cells in patients. Especially, HCC patients who were not responsive to sorafenib showed a median progression-free survival of 7 months (568). In all patients the duration of response ranged from 9 to 63 weeks; three patients showed a continued response at the time of publication. Stable disease was observed in 14 out of 23 patients for 9 weeks to 15.7 months and 21 patients did not show detectable signs of metastasis (568).
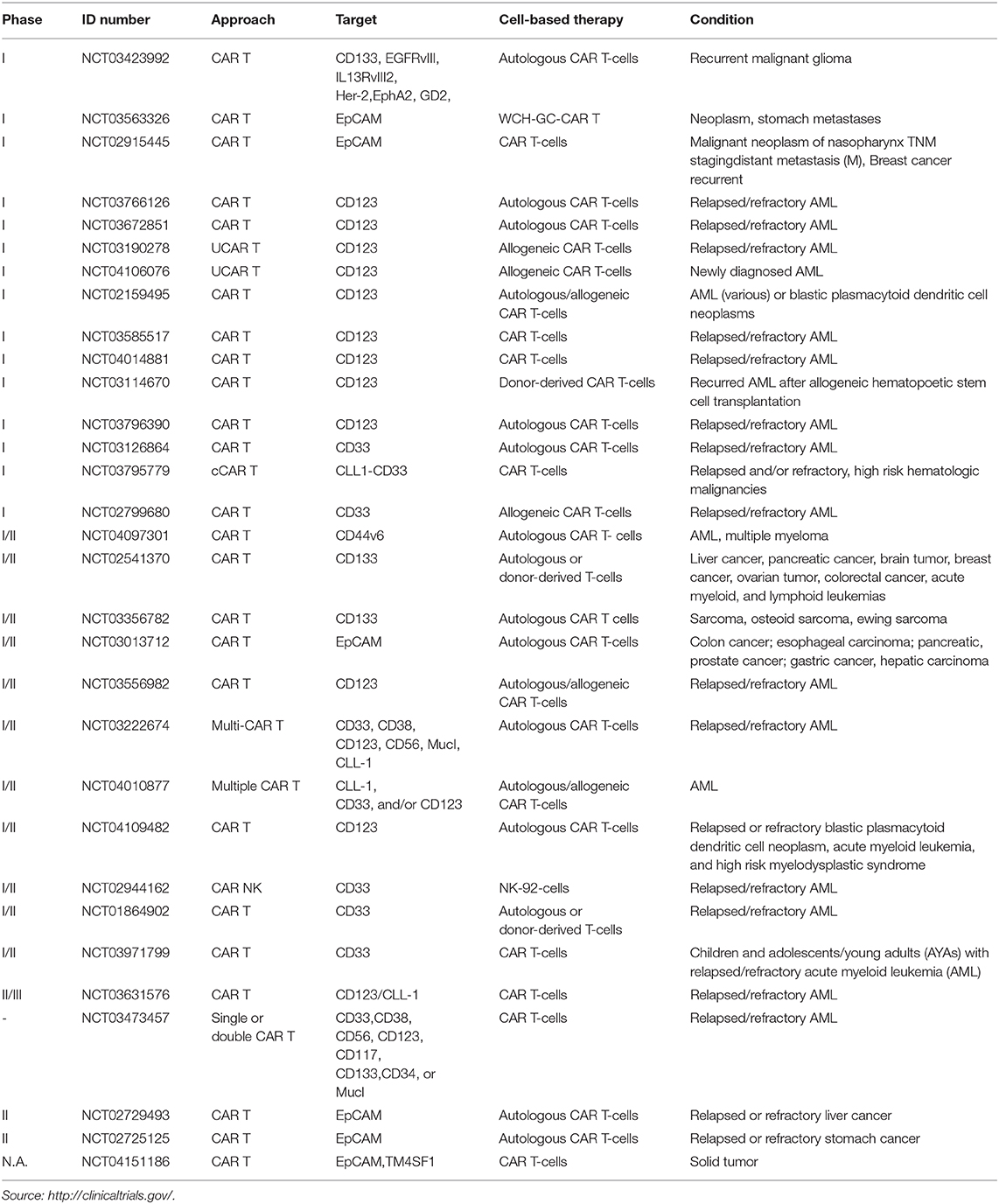
Table 9. Overview of clinical trials using current CAR-cell-based approaches in solid and hematological cancers targeting CSC.
Additional studies (Table 9) are ongoing for the treatment of relapsed or refractory AML (NCT03473457), relapsed or late staged sarcoma (NCT03356782), as well as glioma (NCT03423992). A case study of a patient receiving CD133-directed CAR T-cells after previous chemo- and radiotherapy as well as EGFR-directed CAR T-cell therapy reported a partial response for a period of 4.5 months (569). However, severe toxicities affecting the skin, the oral mucosa, and the gastrointestinal tract were reported (569).
Targeting CD44+ CSCs
Although CD44 is a very prominent CSC antigen, only few CAR-based approaches targeting CD44 have been developed. Early approaches that entered clinical trials included monoclonal antibodies and antibody-conjugates. First studies involving 186Re-conjugated antibody against the splice variant CD44v6 showed advantageous effects at first, however a long-term stable disease was only observed in one patient (570, 571). Likewise, the CD44-directed monoclonal antibody RG7356 showed only modest success in clinical trials with AML patients (572) and solid tumors (468). Tijink et al. coupled the CD44v6-directed antibody bivatuzumab to the cytotoxic antimicrotubule agent mertansine to produce an antibody-prodrug conjugate (573). Bivatuzumab mertansine was administered to seven patients and two of them showed stable disease during the therapy phase. However, one patient with squamous cell carcinoma of the esophagus died after treatment due to toxic epidermal necrolysis, which caused the premature cancelation of this trial (573). Because of this fatality, two clinical trials that were conducted in parallel for patients with metastatic breast cancer (574) and head and neck squamous cell carcinoma (575) had to be terminated.
Still, there are some promising approaches involving CD44v6-directed CAR therapies. For instance, cytokine-induced killer (CIK) cells carrying a CAR against CD44v6 showed anti-cancer effects against sarcoma in vitro and in vivo (576). Furthermore, a phase I/IIa clinical trial using CD44v6-directed CAR T-cells for AML and multiple myeloma patients is currently recruiting (NCT04097301) (Table 9).
Targeting EpCAM+ CSCs
Pre-clinical as well as clinical studies targeting EpCAM+ cancer cells using monoclonal antibodies or CAR constructs have been performed to date using co-culture and xenograft approaches (577–579) (Table 9). Combination therapy of EpCAM-directed CAR NK-92-cells and regorafenib, a potent multikinase inhibitor, resulted in a synergistic antitumor effect using for example colorectal cancer cells or xenograft models (580). CAR T-cells targeting EpCAM have been shown to significantly block tumor growth in xenografts and to secrete cytotoxic cytokines, including interferon-γ (IFN-γ) and tumor necrosis factor alpha (TNF-α) in vitro (579). Additionally, an injection of EpCAM-directed CAR T-cells led to delayed disease progression in immunodeficient mice with peritoneal ovarian and colorectal xenografts (581). Currently, there are several clinical trials with EpCAM-directed CAR T-cells listed for patients with various malignancies: three trials are ongoing (NCT02915445, NCT03563326, and NCT03013712), one trial is not yet recruiting (NCT04151186), and four trials are listed with unknown status (NCT02725125, NCT02728882, NCT02735291, and NCT02729493) (Table 9).
LSC-Directed CAR Therapies
In the field of CAR therapeutics, CD123 and CD33 are frequent targets for AML-specific CAR cells (Table 9). CAR T- and CAR NK-92-cells redirected against CD33 have entered clinical trials (Table 9). Case reports show a good tolerability of CD33-directed CAR NK-92-cells (372), but disease progression after treatment with CD33-directed CAR T-cells was still present (387). Currently, numerous clinical trials using CAR T-cells targeting CD123 are ongoing. NCT03672851 with two participants had to be terminated due to adverse effects (582). Furthermore, first studies implement CLL-1 as a target of CAR T-cells [Table 9; (419), NCT04010877 and NCT03222674].
Next Generation CARs and Targeting of CSCs in Combinational Therapies
For the more efficient CSC elimination, different approaches that have been developed can be used, i.e., tandem CAR T-cells (TanCAR) (583) as well as single universal (U) tricistronic transgene CAR T-cells (UCAR T-cells) (584). Multi-targeting of Her2, IL-13 receptor subunit alpha-2 (IL13Rα2), and ephrin-A2 (EphA2) was shown to overcome antigenic heterogeneity in 15 primary GBM samples and to increase the therapeutic success using xenograft models (584). Targeting two or more antigens may increase the risk for on-target/off-tumor toxicity, since most of the antigens are not only expressed on malignant cells, but also on healthy cells (60, 585). Improved safety, specificity, and flexibility can be obtained using universal CARs (UniCAR) or split, universal and programmable (SUPRA) CARs (585–589). Both consist of an inert and universal CAR construct without a single chain variable fragment (scFv) adaptor molecule domain in combination with a multiple tumor-targeting scFv adaptor molecule (585, 588, 589). In both cases, the activity of CAR T-cells can be regulated by the dosage of the scFv adaptor molecules or by introducing competitive molecules, such as leucine zippers as a regulator for the SUPRA CARs (588, 589). Additional safety of CAR T-cells can be achieved by the induction of suicide genes, e.g., iCasp9 (590, 591) or by inhibitory CAR (iCAR) constructs, in which signaling domains consist of an immuno-inhibitory receptor [e.g., CTLA-4 or PD-1; (592)]. An antigen only expressed on the surface of healthy cells is a target of iCAR and therefore the the attack of non-tumorigenic cells is greatly reduced (592). Specificity can be improved by using synthetic Notch (synNotch) receptors. The binding of synNotch specific to the antigen induces the cleavage of an intracellular domain and activates in turn the transcription of a second CAR, specific to another tumor antigen (593).
To enhance the targeting of solid tumors using CAR-based approaches, the combination treatment with conventional chemotherapeutic drugs could be a novel strategy to enhance antitumor response. To test this approach, NK-92 cells were modified with an EGFR-directed CAR construct against renal cell carcinoma (RCC) cell lines (594). In combination with the multikinase inhibitor cabozantinib, EGFR-directed CAR NK-92 cells showed synergistic effects in vitro and in vivo (594). Cabozantinib also caused a decrease of the anti-inflammatory PD-L1 surface expression in renal cell carcinoma cell lines (594). Furthermore, cabozantinib is known to reduce tumor infiltration of immuno-modulatory subpopulations like regulatory T-cells (Tregs) and myeloid-derived suppressor cells (MDSCs) (594, 595).
The combination of the multikinase inhibitor sunitinib and CAR T -cells targeting carbonic anhydrase IX (CAIX) has been shown to be of advantage as sunitinib reduces immunosuppressive components of the TME (596). Improvements could also be made using Her2-directed CAR NK-92-cells (92/5.137.z) in combination with apatinib (597). Treatment with CAR NK-92 alone resulted in an efficient elimination of small Her2+ tumor xenografts in vivo, but not in an elimination of larger solid tumors in gastric cancers (597). A combinatorial treatment with apatinib increased CAR NK-92 cell infiltration into these larger tumor xenografts and resulted in an enhanced antitumor efficacy of the cells (597).
In AML, early approaches focused on the targeting of single markers; combinatorial therapies, targeting more than one marker, have been tested here as well (598). Haubner et al. analyzed optimal combinations of different LSC markers and concluded that CD33/TIM-3 or CLL-1/TIM-3 combinatorial targeting is most suitable since these markers maximally cover AML cells and are minimally co-expressed on HSCs (370). Interestingly, the combination of CD33 and CD123 was found unsuitable (370). Approaches that already implement combinatorial targeting of AML LSCs include tri-specific killer engagers against CD33 and CD123 (373), compound CAR T-cells against CD33 and CD123 (374) or CLL-1 and CD33 (i.e., NCT03795779), universal CAR T-cells against CD33 and CD123 (375), and CAR CIK-cells against CD33 and CD123 (376).
Future Perspectives
Studies obtained in the last 5–10 years confirmed the importance and the urgent need of diagnostic screening of the TME not only before the treatment, but also at several stages in the post-therapeutic period. This is within the context of personalized therapies that are based on the idea to identify the best therapeutic approach for the patient. This approach should be based on the tumors molecular signature, involving the TME. The best and the most appropriate therapeutic options, which match each individual patient's requirements will increase the therapeutic efficacy and will cause fewer side effects.
The particular value of post-therapeutic local biopsies is that they enable the evaluation of tumor relapse risk on the basis of multivariate biomarkers and also provide information on therapeutically addressable targets within the remaining tumor tissue. In-time detection of tumor-promoting cells, which re-emerge in the post-therapeutic period (Figure 3), will allow an application of the individualized and precise second-line therapy in a timely fashion. Detection of tumor cells with stemness phenotypes will allow for their directed and specific targeting using the second-line treatments, depending on a different mode of action (4, 560). This secondary specific therapy can include, targeted therapies such as e.g., immunotherapies, CAR NK-, and CAR T-cells that mediate a precise eradication of several types of cells: CSCs, CAFs, and/or remaining senescent cells. To increase the specificity and therapeutic outcome and to decrease severe side effects, CAR-based therapeutics are constantly being optimized, as discussed in the section above. Special needs are: improvement of target specificity in combination with decreased off-target effects. In addition, secondary therapies could also include senolytic drugs that selectively kill senescent cells as it was discussed in a recent comprehensive review by Short et al. (599). These therapies cause very low or minor side-effects after their administration (599). In the post-therapeutic period, however, it is important to focus on the biomarkers of CSCs as well as the biomarkers of senescent tumor cells, tumor-promoting SASP molecules, CAFs and TAMs. These cells and molecules strongly influence tumor relapse and their monitoring and their in-time elimination is crucial (Figure 3). As currently available blood test systems are not sensitive enough to detect local changes in the TME, other methods for instance local biopsies and subsequent multivariant analysis of obtained tissues should be used whenever possible and even after many years upon the first-line therapy (Figure 3).
The analysis of multivariant biomarker, however is not only of importance within the post-therapeutic situation. A detailed understanding of the tumor composition before the treatment could allow straight forward first line therapies (Figure 4). Target analysis includes CSCs, CAFs, tumors cells and TAMs, and other tumor-promoting cells. Therapeutic options such as chemotherapy and radiotherapy in combination with small molecules and immunotherapies (CAR cells) could tremendously improve the outcome of the first-line approaches and predict relapses (Figure 4). Combinations already in the first-line therapy are especially required in advanced stages of malignant disease.
In conclusion, our review gives an overview of the most important biomarkers of CSCs in the TME. Furthermore, we underline the value of local biopsies and precise diagnostics and screening of biomarkers in both pre- and post-therapeutic situations (Figures 3, 4). We suggest the implementation of those strategies in the first and second-line personalized therapy required to eradicate the remaining tumor-promoting senescent tumor cells, CAFs, TAMs, and finally CSCs to protect from tumor recurrence.
The high costs are one point of contention regarding the biopsies and their analysis as well as the implementation of immunotherapies into the first and secondary line targeted therapies. However, considering the costs for therapies, comprising resection, and medication strategies, as well as the patient's sufferings due to a re-emerged full-blown cancer, the targeted therapy will help to save the patients and clinics from high personnel, emotional, and medicinal costs.
Author Contributions
SF, UK-B, and TY performed a conceptualization for the review and defined the future perspectives. LW, A-KK, HS, RK, SD, AS, A-RB, TY, SF, and UK-B analyzed the publications and created the figures and tables. All authors contributed to the article and approved the submitted version.
Funding
This study was supported by the Fraunhofer Society, the Fraunhofer Cluster of Immune Mediated Diseases (CIMD), and the Leistungs- und Transferzentrum Chemie- und Biosystemtechnik which is supported by Sächsische Aufbaubank (SAB). This work was also supported in part by the Fritz Thyssen Foundation (REF.10.16.1.031MN) and by the German Research Foundation (YE 151/2-1, AOBJ: 618426) to TY.
Conflict of Interest
The authors declare that the research was conducted in the absence of any commercial or financial relationships that could be construed as a potential conflict of interest.
Acknowledgments
The authors thank the colleagues from the Fraunhofer Cluster of Immune Mediated Diseases (CIMD), the MAVO Consortium ELITE NK cells for their helpful discussion, and proof reading of the manuscript. Study design, collection of data, analysis, decision to publish, and preparation of the manuscript were not influenced.
References
1. Bray F, Ferlay J, Soerjomataram I, Siegel RL, Torre LA, Jemal A. Global cancer statistics 2018: globocan estimates of incidence and mortality worldwide for 36 cancers in 185 countries. CA Cancer J Clin. (2018) 68:394–424. doi: 10.3322/caac.21492
2. Capp J-P. Cancer stem cells: from historical roots to a new perspective. J Oncol. (2019) 2019:5189232. doi: 10.1155/2019/5189232
3. Spillane JB, Henderson MA. Cancer stem cells: a review. ANZ J Surg. (2007) 77:464–8. doi: 10.1111/j.1445-2197.2007.04096.x
4. Phi LT, Sari IN, Yang Y-G, Lee S-H, Jun N, Kim KS, et al. Cancer Stem Cells (CSCs) in drug resistance and their therapeutic implications in cancer treatment. Stem Cells Int. (2018) 2018:5416923. doi: 10.1155/2018/5416923
5. Reya T, Morrison SJ, CLarke MF, Weissman IL. Stem cells, cancer, and cancer stem cells. Nature. (2001) 414:105–11. doi: 10.1038/35102167
6. Ayob AZ, Ramasamy TS. Cancer stem cells as key drivers of tumour progression. J Biomed Sci. (2018) 25:20. doi: 10.1186/s12929-018-0426-4
7. Kuşoglu A, Biray Avci Ç. Cancer stem cells: a brief review of the current status. Gene. (2019) 681:80–5. doi: 10.1016/j.gene.2018.09.052
8. Arnold CR, Mangesius J, Skvortsova I-I, Ganswindt U. The role of cancer stem cells in radiation resistance. Front. Oncol. (2020) 10:164. doi: 10.3389/fonc.2020.00164
9. Shibata M, Hoque MO. Targeting cancer stem cells: a strategy for effective eradication of cancer. Cancers. (2019) 11:732. doi: 10.3390/cancers11050732
10. Zeng S, Shen WH, Liu L. Senescence and cancer. Cancer Transl Med. (2018) 4:70–4. doi: 10.4103/ctm.ctm_22_18
11. Scuric Z, Carroll JE, Bower JE, Ramos-Perlberg S, Petersen L, Esquivel S, et al. Biomarkers of aging associated with past treatments in breast cancer survivors. NPJ Breast Cancer. 3:1–7. doi: 10.1038/s41523-017-0050-6
12. Fan DN, Schmitt CA. Detecting markers of therapy-induced senescence in cancer cells. In: Nikiforov MA, editor. Oncogene-Induced Senescence: Methods and Protocols. New York, NY: Humana Press (2017). p. 41–52.
13. Desai A, Yan Y, Gerson SL. Concise reviews: cancer stem cell targeted therapies: toward clinical success. Stem Cells Transl Med. (2019) 8:75–81. doi: 10.1002/sctm.18-0123
14. Milanovic M, Fan DN, Belenki D, Däbritz JH, Zhao Z, Yu Y, et al. Senescence-associated reprogramming promotes cancer stemness. Nature. (2018) 553:96–100. doi: 10.1038/nature25167
15. Gupta PB, Pastushenko I, Skibinski A, Blanpain C, Kuperwasser C. Phenotypic plasticity: driver of cancer initiation, progression, and therapy resistance. Cell Stem Cell. (2019) 24:65–78. doi: 10.1016/j.stem.2018.11.011
16. Poli V, Fagnocchi L, Zippo A. Tumorigenic cell reprogramming and cancer plasticity: interplay between signaling, microenvironment, and epigenetics. Stem Cells Int. (2018) 2018:4598195. doi: 10.1155/2018/4598195
17. Angelis ML de, Francescangeli F, La Torre F, Zeuner A. Stem cell plasticity and dormancy in the development of cancer therapy resistance. Front. Oncol. (2019) 9:626. doi: 10.3389/fonc.2019.00626
18. Yuan S, Norgard RJ, Stanger BZ. Cellular plasticity in cancer. Cancer Discov. (2019) 9:837–51. doi: 10.1158/2159-8290.CD-19-0015
19. Hanahan D, Weinberg RA. Hallmarks of cancer: the next generation. Cell. (2011) 144:646–74. doi: 10.1016/j.cell.2011.02.013
20. Basu AK. DNA damage, mutagenesis and cancer. Int J Mol Sci. (2018) 19:970. doi: 10.3390/ijms19040970
21. Blackadar CB. Historical review of the causes of cancer. World J Clin Oncol. (2016) 7:54–86. doi: 10.5306/wjco.v7.i1.54
22. Li L, Neaves WB. Normal stem cells and cancer stem cells: the niche matters. Cancer Res. (2006) 66:4553–7. doi: 10.1158/0008-5472.CAN-05-3986
23. Sell S. Cellular origin of cancer: dedifferentiation or stem cell maturation arrest? Environ Health Perspect. (1993) 101(Suppl. 5):15–26. doi: 10.1289/ehp.93101s515
24. Hayakawa Y, Fox JG, Wang TC. The origins of gastric cancer from gastric stem cells: lessons from mouse models. Cell Mol Gastroenterol Hepatol. (2017) 3:331–8. doi: 10.1016/j.jcmgh.2017.01.013
25. Koulis A, Buckle A, Boussioutas A. Premalignant lesions and gastric cancer: current understanding. World J Gastroentero Oncol. (2019) 11:665–78. doi: 10.4251/wjgo.v11.i9.665
26. Hata M, Hayakawa Y, Koike K. Gastric stem cell and cellular origin of cancer. Biomedicines. (2018) 6:100. doi: 10.3390/biomedicines6040100
27. Li X-B, Yang G, Zhu L, Tang Y-L, Zhang C, Ju Z, et al. Gastric Lgr5+ stem cells are the cellular origin of invasive intestinal-type gastric cancer in mice. Cell Res. (2016) 26:838–49. doi: 10.1038/cr.2016.47
28. Perekatt AO, Shah PP, Cheung S, Jariwala N, Wu A, Gandhi V, et al. SMAD4 suppresses WNT-driven dedifferentiation and oncogenesis in the differentiated gut epithelium. Cancer Res. (2018) 78:4878–90. doi: 10.1158/0008-5472.CAN-18-0043
29. Mu X, Español-Suñer R, Mederacke I, Affò S, Manco R, Sempoux C, et al. Hepatocellular carcinoma originates from hepatocytes and not from the progenitor/biliary compartment. J Clin Invest. (2015) 125:3891–903. doi: 10.1172/JCI77995
30. Jörs S, Jeliazkova P, Ringelhan M, Thalhammer J, Dürl S, Ferrer J, et al. Lineage fate of ductular reactions in liver injury and carcinogenesis. J Clin Invest. (2015) 125:2445–57. doi: 10.1172/JCI78585
31. Holczbauer Á, Factor VM, Andersen JB, Marquardt JU, Kleiner DE, Raggi C, et al. Modeling pathogenesis of primary liver cancer in lineage-specific mouse cell types. Gastroenterology. (2013) 145:221–31. doi: 10.1053/j.gastro.2013.03.013
32. Oikawa T. Cancer stem cells and their cellular origins in primary liver and biliary tract cancers. Hepatology. (2016) 64:645–51. doi: 10.1002/hep.28485
33. Polyak K. Breast cancer: origins and evolution. J Clin Invest. (2007) 117:3155–63. doi: 10.1172/JCI33295
34. Zarzynska JM. Chapter 12: The role of stem cells in breast cancer. In: Van Pham P, editor. Breast Cancer - From Biology to Medicine. VNU-HCM University of Science; INTECH Open. (2017) 231–49. doi: 10.5772/66904
35. Zhou J, Chen Q, Zou Y, Chen H, Qi L, Chen Y. Stem cells and cellular origins of breast cancer: updates in the rationale, controversies, and therapeutic implications. Front. Oncol. (2019) 9:820. doi: 10.3389/fonc.2019.00820
36. Wang J, Xu B. Targeted therapeutic options and future perspectives for HER2-positive breast cancer. Sig Transduct Target Ther. (2019) 4:1–22. doi: 10.1038/s41392-019-0069-2
37. Sánchez-Danés A, Blanpain C. Deciphering the cells of origin of squamous cell carcinomas. Nat Rev Cancer. (2018) 18:549–61. doi: 10.1038/s41568-018-0024-5
38. Yamano S, Gi M, Tago Y, Doi K, Okada S, Hirayama Y, et al. Role of deltaNp63(pos)CD44v(pos) cells in the development of n-nitroso-tris-chloroethylurea-induced peripheral-type mouse lung squamous cell carcinomas. Cancer Sci. (2016) 107:123–32. doi: 10.1111/cas.12855
39. Hardavella G, George R, Sethi T. Lung cancer stem cells—characteristics, phenotype. Transl Lung Cancer Res. (2016) 5:272–9. doi: 10.21037/tlcr.2016.02.01
40. Fukui T, Shaykhiev R, Agosto-Perez F, Mezey JG, Downey RJ, Travis WD, et al. Lung adenocarcinoma subtypes based on expression of human airway basal cell genes. Eur Respir J. (2013) 42:1332–44. doi: 10.1183/09031936.00144012
41. Kim CF, Jackson EL, Woolfenden AE, Lawrence S, Babar I, Vogel S, et al. Identification of bronchioalveolar stem cells in normal lung and lung cancer. Cell. (2005) 121:823–35. doi: 10.1016/j.cell.2005.03.032
42. Sutherland KD, Proost N, Brouns I, Adriaensen D, Song J-Y, Berns A. Cell of origin of small cell lung cancer: inactivation of trp53 and rb1 in distinct cell types of adult mouse lung. Cancer Cell. (2011) 19:754–64. doi: 10.1016/j.ccr.2011.04.019
43. Bonnet D, Dick JE. Human acute myeloid leukemia is organized as a hierarchy that originates from a primitive hematopoietic cell. Nat Med. (1997) 3:730–7. doi: 10.1038/nm0797-730
44. Czeh M, Rosenbauer F. Uncovering a new cellular origin for acute myeloid leukemia with lineage plasticity. Mol Cell Oncol. (2017) 4:e1268241. doi: 10.1080/23723556.2016.1268241
45. Riemke P, Czeh M, Fischer J, Walter C, Ghani S, Zepper M, et al. Myeloid leukemia with transdifferentiation plasticity developing from T-cell progenitors. EMBO J. (2016) 35:2399–416. doi: 10.15252/embj.201693927
46. Stuart SA, Minami Y, Wang JY. The CML stem cell: evolution of the progenitor. Cell CyCLe. (2009) 8:1338–43. doi: 10.4161/cc.8.9.8209
47. Zhou H, Xu R. Leukemia stem cells: the root of chronic myeloid leukemia. Protein Cell. (2015) 6:403–12. doi: 10.1007/s13238-015-0143-7
48. Ebinger S, Özdemir EZ, Ziegenhain C, Tiedt S, Castro Alves C, Grunert M, et al. Characterization of rare, dormant, and therapy-Resistant cells in acute lymphoblastic leukemia. Cancer Cell. (2016) 30:849–62. doi: 10.1016/j.ccell.2016.11.002
49. Martinez-CLiment JA, Fontan L, Gascoyne RD, Siebert R, Prosper F. Lymphoma stem cells: enough evidence to support their existence? Haematologica. (2010) 95:293–302. doi: 10.3324/haematol.2009.013318
50. Lang F, Wojcik B, Rieger MA. Stem cell hierarchy and clonal evolution in acute lymphoblastic leukemia. Stem Cells Int. (2015) 2015:137164. doi: 10.1155/2015/137164
51. Afify SM, Seno M. Conversion of stem cells to cancer stem cells: undercurrent of cancer initiation. Cancers. (2019) 11:345. doi: 10.3390/cancers11030345
52. Bu P, Chen K-Y, Lipkin SM, Shen X. Asymmetric division: a marker for cancer stem cells? Oncotarget. (2013) 4:950–1. doi: 10.18632/oncotarget.1029
53. Goardon N, Marchi E, Atzberger A, Quek L, Schuh A, Soneji S, et al. Coexistence of lMPP-like and gMP-like leukemia stem cells in acute myeloid leukemia. Cancer Cell. (2011) 19:138–52. doi: 10.1016/j.ccr.2010.12.012
54. Quek L, Otto GW, Garnett C, Lhermitte L, Karamitros D, Stoilova B, et al. Genetically distinct leukemic stem cells in human CD34- acute myeloid leukemia are arrested at a hemopoietic precursor-like stage. J Exp Med. (2016) 213:1513–35. doi: 10.1084/jem.20151775
55. Chopra M, Bohlander SK. The cell of origin and the leukemia stem cell in acute myeloid leukemia. Genes Chromosomes Cancer. (2019) 58:850–8. doi: 10.1002/gcc.22805
56. Cabrera MC, Hollingsworth RE, Hurt EM. Cancer stem cell plasticity and tumor hierarchy. World J Stem Cells. (2015) 7:27–36. doi: 10.4252/wjsc.v7.i1.27
57. Rich JN. Cancer stem cells: understanding tumor hierarchy and heterogeneity. Medicine. (2016) 95:S2–7. doi: 10.1097/MD.0000000000004764
58. Berger DP, Mertelsmann R editors. Das Rote Buch: Hämatologie und Internistische Onkologie. Landsberg am Lech: ecomed MEDIZIN (2017). p. 1400.
59. Sell S. Alpha-fetoprotein, stem cells and cancer: how study of the production of alpha-fetoprotein during chemical hepatocarcinogenesis led to reaffirmation of the stem cell theory of cancer. Tumour Biol. (2008) 29:161–80. doi: 10.1159/000143402
60. Kim W-T, Ryu CJ. Cancer stem cell surface markers on normal stem cells. BMB Rep. (2017) 50:285–98. doi: 10.5483/BMBRep.2017.50.6.039
61. Su J, Wu S, Wu H, Le Li, Guo T. CD44 is functionally crucial for driving lung cancer stem cells metastasis through Wnt/β-catenin-FoxM1-Twist signaling. Mol Carcinog. (2016) 55:1962–73. doi: 10.1002/mc.22443
62. Satar NA, Fakiruddin KS, Lim MN, Mok PL, Zakaria N, Fakharuzi NA, et al. Novel triplepositive markers identified in human nonsmall cell lung cancer cell line with chemotherapy-resistant and putative cancer stem cell characteristics. Oncol Rep. (2018) 40:669–81. doi: 10.3892/or.2018.6461
63. Hu B, Ma Y, Yang Y, Zhang L, Han H, Chen J. CD44 promotes cell proliferation in non-small cell lung cancer. Oncol Lett. (2018) 15:5627–33. doi: 10.3892/ol.2018.8051
64. Suda K, Murakami I, Yu H, Kim J, Tan A-C, Mizuuchi H, et al. CD44 facilitates epithelial-to-Mesenchymal transition phenotypic change at acquisition of resistance to EGFR kinase inhibitors in lung cancer. Mol Cancer Ther. (2018) 17:2257–65. doi: 10.1158/1535-7163.MCT-17-1279
65. Nishino M, Ozaki M, Hegab AE, Hamamoto J, Kagawa S, Arai D, et al. Variant CD44 expression is enriching for a cell population with cancer stem cell-like characteristics in human lung adenocarcinoma. J Cancer. (2017) 8:1774–85. doi: 10.7150/jca.19732
66. Zakaria N, Yusoff NM, Zakaria Z, Lim MN, Baharuddin PJ, Fakiruddin KS, et al. Human non-small cell lung cancer expresses putative cancer stem cell markers and exhibits the transcriptomic profile of multipotent cells. BMC Cancer. (2015) 15:84. doi: 10.1186/s12885-015-1086-3
67. Prabavathy D, SwaRNAlatha Y, Ramadoss N. Lung cancer stem cells-origin, characteristics and therapy. Stem Cell Investig. (2018) 5:6. doi: 10.21037/sci.2018.02.01
68. Testa U, Castelli G, Pelosi E. Lung cancers: molecular characterization, clonal heterogeneity and evolution, and cancer stem cells. Cancers. (2018) 10:248. doi: 10.3390/cancers10080248
69. Maiuthed A, Chantarawong W, Chanvorachote P. Lung cancer stem cells and cancer stem cell-targeting natural compounds. Anticancer Res. (2018) 38:3797–809. doi: 10.21873/anticanres.12663
70. Park E, Park SY, Sun P-L, Jin Y, Kim JE, Jheon S, et al. Prognostic significance of stem cell-related marker expression and its correlation with histologic subtypes in lung adenocarcinoma. Oncotarget. (2016) 7:42502–12. doi: 10.18632/oncotarget.9894
71. Alamgeer M, Neil Watkins D, Banakh I, Kumar B, Gough DJ, Markman B, et al. A phase IIa study of HA-irinotecan, formulation of hyaluronic acid and irinotecan targeting CD44 in extensive-stage small cell lung cancer. Invest New Drugs. (2018) 36:288–98. doi: 10.1007/s10637-017-0555-8
72. Quan YH, Lim J-Y, Choi BH, Choi Y, Choi YH, Park J-H, et al. Self-targeted knockdown of CD44 improves cisplatin sensitivity of chemoresistant non-small cell lung cancer cells. Cancer Chemother Pharmacol. (2019) 83:399–410. doi: 10.1007/s00280-018-3737-y
73. Kawano Y, Iwama E, Tsuchihashi K, Shibahara D, Harada T, Tanaka K, et al. CD44 variant-dependent regulation of redox balance in EGFR mutation-positive non-small cell lung cancer: a target for treatment. Lung Cancer. (2017) 113:72–8. doi: 10.1016/j.lungcan.2017.09.008
74. Huang X, Wan J, Leng D, Zhang Y, Yang S. Dual-targeting nanomicelles with CD133 and CD44 aptamers for enhanced delivery of gefitinib to two populations of lung cancer-initiating cells. Exp Ther Med. (2020) 19:192–204. doi: 10.3892/etm.2019.8220
75. Luo Y, Wang X, Du D, Lin Y. Hyaluronic acid-conjugated apoferritin nanocages for lung cancer targeted drug delivery. Biomater Sci. (2015) 3:1386–94. doi: 10.1039/C5BM00067J
76. Alshaer W, Hillaireau H, Vergnaud J, Ismail S, Fattal E. Functionalizing liposomes with anti-CD44 aptamer for selective targeting of cancer cells. Bioconjug Chem. (2015) 26:1307–13. doi: 10.1021/bc5004313
77. Song Y, Cai H, Yin T, Huo M, Ma P, Zhou J, et al. PaCLitaxel-loaded redox-sensitive nanopartiCLes based on hyaluronic acid-vitamin E succinate conjugates for improved lung cancer treatment. Int J Nanomed. (2018) 13:1585–600. doi: 10.2147/IJN.S155383
78. Parashar P, Rathor M, Dwivedi M, Saraf SA. Hyaluronic acid decorated naringenin nanoparticles: appraisal of chemopreventive and curative potential for lung cancer. Pharmaceutics. (2018) 10:33. doi: 10.3390/pharmaceutics10010033
79. Tian Y, Zhang H, Qin Y, Li D, Liu Y, Wang H, et al. Overcoming drug-resistant lung cancer by paCLitaxel-loaded hyaluronic acid-coated liposomes targeted to mitochondria. Drug Dev Ind Pharm. (2018) 44:2071–82. doi: 10.1080/03639045.2018.1512613
80. Zhang W, Xu W, Lan Y, He X, Liu K, Liang Y. Antitumor effect of hyaluronic-acid-modified chitosan nanopartiCLes loaded with siRNA for targeted therapy for non-small cell lung cancer. Int J Nanomedicine. (2019) 14:5287–301. doi: 10.2147/IJN.S203113
81. Shinohara S, Hanagiri T, Taira A, Takenaka M, Oka S, Chikaishi Y, et al. Immunohistochemical expression and serum levels of CD44 as prognostic indicators in patients with non-small cell lung cancer. Oncology. (2016) 90:327–38. doi: 10.1159/000445951
82. Qiu X, Wang Z, Li Y, Miao Y, Ren Y, Luan Y. Characterization of sphere-forming cells with stem-like properties from the small cell lung cancer cell line H446. Cancer Lett. (2012) 323:161–70. doi: 10.1016/j.canlet.2012.04.004
83. Yan X, Luo H, Zhou X, Zhu B, Wang Y, Bian X. Identification of CD90 as a marker for lung cancer stem cells in a549 and h446 cell lines. Oncol Rep. (2013) 30:2733–40. doi: 10.3892/or.2013.2784
84. Koren A, Rijavec M, Kern I, Sodja E, Korosec P, Cufer T. BMI1, ALDH1A1, and CD133 transcripts connect epithelial-mesenchymal transition to cancer stem cells in lung carcinoma. Stem Cells Int. (2016) 2016:9714315. doi: 10.1155/2016/9714315
85. Alama A, Gangemi R, Ferrini S, Barisione G, Orengo AM, Truini M, et al. CD133-Positive cells from non-Small cell lung cancer show distinct sensitivity to cisplatin and afatinib. Arch Immunol Ther Exp. (2015) 63:207–14. doi: 10.1007/s00005-015-0330-5
86. Eramo A, Lotti F, Sette G, Pilozzi E, Biffoni M, Di Virgilio A, et al. Identification and expansion of the tumorigenic lung cancer stem cell population. Cell Death Differ. (2008) 15:504–14. doi: 10.1038/sj.cdd.4402283
87. Huang Z, Yu H, Zhang J, Jing H, Zhu W, Li X, et al. Correlation of cancer stem cell markers and immune cell markers in resected non-small cell lung cancer. J Cancer. (2017) 8:3190–7. doi: 10.7150/jca.20172
88. Li L, Li J-C, Yang H, Zhang X, Liu L-L, Li Y, et al. Expansion of cancer stem cell pool initiates lung cancer recurrence before angiogenesis. Proc Natl Acad Sci USA. (2018) 115:E8948–57. doi: 10.1073/pnas.1806219115
89. Liu X, Wei H, Liu Y, Zhao M, Wu S, Yang Y. Construction of high sensitive CD133 immune PLGA magnetic spheres platform for lung cancer stem cells isolation and its property evaluation. J Biomed Nanotechnol. (2018) 14:1066–74. doi: 10.1166/jbn.2018.2562
90. Su Y-J, Lin W-H, Chang Y-W, Wei K-C, Liang C-L, Chen S-C, et al. Polarized cell migration induces cancer type-specific CD133/integrin/Src/Akt/GSK3β/β-catenin signaling required for maintenance of cancer stem cell properties. Oncotarget. (2015) 6:38029–45. doi: 10.18632/oncotarget.5703
91. Bertolini G, D'Amico L, Moro M, Landoni E, Perego P, Miceli R, et al. Microenvironment-modulated metastatic CD133+/CXCR4+/EpCAM- lung cancer-Initiating cells sustain tumor dissemination and correlate with poor prognosis. Cancer Res. (2015) 75:3636–49. doi: 10.1158/0008-5472.CAN-14-3781
92. Chen Y, Zhang F, Tsai Y, Yang X, Yang L, Duan S, et al. IL-6 signaling promotes DNA repair and prevents apoptosis in CD133+ stem-like cells of lung cancer after radiation. Radiat Oncol. (2015) 10:227. doi: 10.1186/s13014-015-0534-1
93. Zhao W, Luo Y, Li B, Zhang T. Tumorigenic lung tumorospheres exhibit stem-like features with significantly increased expression of CD133 and ABCG2. Mol Med Rep. (2016) 14:2598–606. doi: 10.3892/mmr.2016.5524
94. Koyama K, Katsurada N, Jimbo N, Tachihara M, Tamura D, Nakata K, et al. Overexpression of CD 133 and bCL-2 in non-small cell lung cancer with neuroendocrine differentiation after transformation in ALK rearrangement-positive adenocarcinoma. Pathol Int. (2019) 69:294–9. doi: 10.1111/pin.12782
95. Moro M, Bertolini G, Caserini R, Borzi C, Boeri M, Fabbri A, et al. Establishment of patient derived xenografts as functional testing of lung cancer aggressiveness. Sci. Rep. (2017) 7:6689. doi: 10.1038/s41598-017-06912-7
96. Zhao C, Setrerrahmane S, Xu H. Enrichment and characterization of cancer stem cells from a human non-small cell lung cancer cell line. Oncol Rep. (2015) 34:2126–32. doi: 10.3892/or.2015.4163
97. Sun F-F, Hu Y-H, Xiong L-P, Tu X-Y, Zhao J-H, Chen S-S, et al. Enhanced expression of stem cell markers and drug resistance in sphere-forming non-small cell lung cancer cells. Int J Clin Exp Pathol. (2015) 8:6287–300.
98. Yu J, Wang S, Zhao W, Duan J, Wang Z, Chen H, et al. Mechanistic exploration of cancer stem cell marker voltage-Dependent calcium channel α2δ1 subunit-mediated chemotherapy resistance in small-cell lung cancer. Clin Cancer Res. (2018) 24:2148–58. doi: 10.1158/1078-0432.CCR-17-1932
99. Sarvi S, Mackinnon AC, Avlonitis N, Bradley M, Rintoul RC, Rassl DM, et al. CD133+ cancer stem-like cells in small cell lung cancer are highly tumorigenic and chemoresistant but sensitive to a novel neuropeptide antagonist. Cancer Res. (2014) 74:1554–65. doi: 10.1158/0008-5472.CAN-13-1541
100. Meng X, Li M, Wang X, Wang Y, Ma D. Both CD133+ and CD133- subpopulations of a549 and h446 cells contain cancer-initiating cells. Cancer Sci. (2009) 100:1040–6. doi: 10.1111/j.1349-7006.2009.01144.x
101. Huang X, Huang J, Leng D, Yang S, Yao Q, Sun J, et al. Gefitinib-loaded DSPE-PEG2000 nanomicelles with CD133 aptamers target lung cancer stem cells. World J Surg Oncol. (2017) 15:167. doi: 10.1186/s12957-017-1230-4
102. Ma J, Zhuang H, Zhuang Z, Lu Y, Xia R, Gan L, et al. Development of docetaxel liposome surface modified with CD133 aptamers for lung cancer targeting. Artif Cells Nanomed Biotechnol. (2018) 46:1864–71. doi: 10.1080/21691401.2017.1394874
103. Zhou J, Sun J, Chen H, Peng Q. Promoted delivery of salinomycin sodium to lung cancer cells by dual targeting PLGA hybrid nanopartiCLes. Int J Oncol. (2018) 53:1289–300. doi: 10.3892/ijo.2018.4474
104. Zhou J, Sun M, Jin S, Fan L, Zhu W, Sui X, et al. Combined using of paclitaxel and salinomycin active targeting nanostructured lipid carriers against non-small cell lung cancer and cancer stem cells. Drug Deliv. (2019) 26:281–9. doi: 10.1080/10717544.2019.1580799
105. Bertolini G, Roz L, Perego P, Tortoreto M, Fontanella E, Gatti L, et al. Highly tumorigenic lung cancer CD133+ cells display stem-like features and are spared by cisplatin treatment. Proc Natl Acad Sci USA. (2009) 106:16281–6. doi: 10.1073/pnas.0905653106
106. Wang D, Wen G-M, Hou W, Xia P. The roles of CD133 expression in the patients with non-small cell lung cancer. Cancer Biomark. (2018) 22:385–94. doi: 10.3233/CBM-170835
107. Miyata T, Oyama T, Yoshimatsu T, Higa H, Kawano D, Sekimura A, et al. The Clinical significance of cancer stem cell markers ALDH1A1 and CD133 in lung adenocarcinoma. Anticancer Res. (2017) 37:2541–7. doi: 10.21873/anticanres.11597
108. Su C, Xu Y, Li X, Ren S, Zhao C, Hou L, et al. Predictive and prognostic effect of CD133 and cancer-testis antigens in stage Ib-IIIA non-small cell lung cancer. Int J Clin Exp Pathol. (2015) 8:5509–18.
109. Qiu Z-X, Zhao S, Mo X-M, Li W-M. Overexpression of PROM1 (CD133) confers poor prognosis in non-small cell lung cancer. Int J Clin Exp Pathol. (2015) 8:6589–95.
110. Wen G-M, Mou F-F, Hou W, Wang D, Xia P. Integrative analysis of CD133 mRNA in human cancers based on data mining. Stem Cell Rev Rep. (2019) 15:23–34. doi: 10.1007/s12015-018-9865-2
111. Sowa T, Menju T, Sonobe M, Nakanishi T, Shikuma K, Imamura N, et al. Association between epithelial-mesenchymal transition and cancer stemness and their effect on the prognosis of lung adenocarcinoma. Cancer Med. (2015) 4:1853–62. doi: 10.1002/cam4.556
112. Chen E, Zeng Z, Bai B, Zhu J, Song Z. The prognostic value of CSCs biomarker CD133 in NSCLC: a meta-analysis. Oncotarget. (2016) 7:56526–39. doi: 10.18632/oncotarget.10964
113. Zhao M, Zhang Y, Zhang H, Wang S, Zhang M, Chen X, et al. Hypoxia-induced cell stemness leads to drug resistance and poor prognosis in lung adenocarcinoma. Lung Cancer. (2015) 87:98–106. doi: 10.1016/j.lungcan.2014.11.017
114. Hanssen A, Wagner J, Gorges TM, Taenzer A, Uzunoglu FG, Driemel C, et al. Characterization of different CTC subpopulations in non-small cell lung cancer. Sci. Rep. (2016) 6:28010. doi: 10.1038/srep28010
115. Jahchan NS, Lim JS, Bola B, Morris K, Seitz G, Tran KQ, et al. Identification and targeting of long-term tumor-Propagating cells in small cell lung cancer. Cell Rep. (2016) 16:644–56. doi: 10.1016/j.celrep.2016.06.021
116. Chen L, Peng M, Li N, Song Q, Yao Y, Xu B, et al. Combined use of epCAM and FRα enables the high-efficiency capture of circulating tumor cells in non-small cell lung cancer. Sci. Rep. (2018) 8:1188. doi: 10.1038/s41598-018-19391-1
117. Wit S de, Rossi E, Weber S, Tamminga M, Manicone M, Swennenhuis JF, et al. Single tube liquid biopsy for advanced non-small cell lung cancer. Int J Cancer. (2019) 144:3127–37. doi: 10.1002/ijc.32056
118. Zamay GS, Kolovskaya OS, Ivanchenko TI, Zamay TN, Veprintsev DV, Grigorieva VL, et al. Development of DNA aptamers to native EpCAM for isolation of lung circulating tumor cells from human blood. Cancers. (2019) 11:351. doi: 10.3390/cancers11030351
119. Rud AK, Boye K, Fodstad Ø, Juell S, Jørgensen LH, Solberg S, et al. Detection of disseminated tumor cells in lymph nodes from patients with early stage non-small cell lung cancer. Diagn Pathol. (2016) 11:50. doi: 10.1186/s13000-016-0504-4
120. Gao W, Huang T, Yuan H, Yang J, Jin Q, Jia C, et al. Highly sensitive detection and mutational analysis of lung cancer circulating tumor cells using integrated combined immunomagnetic beads with a droplet digital PCR chip. Talanta. (2018) 185:229–36. doi: 10.1016/j.talanta.2018.03.083
121. Alibolandi M, Ramezani M, Abnous K, Sadeghi F, Atyabi F, Asouri M, et al. In vitro and in vivo evaluation of therapy targeting epithelial-cell adhesion-molecule aptamers for non-small cell lung cancer. J Control Release. (2015) 209:88–100. doi: 10.1016/j.jconrel.2015.04.026
122. Thompson JC, Fan R, Black T, Yu GH, Savitch SL, Chien A, et al. Measurement and immunophenotyping of pleural fluid epCAM-positive cells and CLusters for the management of non-small cell lung cancer patients. Lung Cancer. (2019) 127:25–33. doi: 10.1016/j.lungcan.2018.11.020
123. Zhou N, Wang H, Liu H, Xue H, Lin F, Meng X, et al. MTA1-upregulated epCAM is associated with metastatic behaviors and poor prognosis in lung cancer. J Exp Clin Cancer Res. (2015) 34:157. doi: 10.1186/s13046-015-0263-1
124. Wit S de, van Dalum G, Lenferink AT, Tibbe AG, Hiltermann TJ, Groen HJ, et al. The detection of epCAM(+) and epCAM(-) circulating tumor cells. Sci. Rep. (2015) 5:12270. doi: 10.1038/srep12270
125. Gao F, Zhou B, Xu J-C, Gao X, Li S-X, Zhu G-C, et al. The role of LGR5 and ALDH1A1 in non-small cell lung cancer: cancer progression and prognosis. Biochem Biophys Res Commun. (2015) 462:91–8. doi: 10.1016/j.bbrc.2015.04.029
126. Koh YW, Han J-H, Haam S, Jung J. ALDH1 expression correlates with an epithelial-like phenotype and favorable prognosis in lung adenocarcinoma: a study based on immunohistochemistry and mRNA expression data. J Cancer Res Clin Oncol. (2019) 145:1427–36. doi: 10.1007/s00432-019-02906-2
127. Codony-Servat J, Codony-Servat C, Cardona AF, Giménez-Capitán A, Drozdowskyj A, Berenguer J, et al. Cancer stem cell biomarkers in EGFR-mutation-positive non-small-cell lung cancer. Clin Lung Cancer. (2019) 20:167–77. doi: 10.1016/j.CLlc.2019.02.005
128. Jiang F, Qiu Q, Khanna A, Todd NW, Deepak J, Xing L, et al. Aldehyde dehydrogenase 1 is a tumor stem cell-associated marker in lung cancer. Mol Cancer Res. (2009) 7:330–8. doi: 10.1158/1541-7786.MCR-08-0393
129. Masciale V, Grisendi G, Banchelli F, D'Amico R, Maiorana A, Sighinolfi P, et al. Isolation and identification of cancer stem-like cells in adenocarcinoma and squamous cell carcinoma of the lung: a Pilot study. Front. Oncol. (2019) 9:1394. doi: 10.3389/fonc.2019.01394
130. Codony-Servat J, Verlicchi A, Rosell R. Cancer stem cells in small cell lung cancer. Transl Lung Cancer Res. (2016) 5:16–25. doi: 10.3978/j.issn.2218-6751.2016.01.01
131. Rossi A, Voigtlaender M, Klose H, Schlüter H, Schön G, Loges S, et al. High aldehyde dehydrogenase levels are detectable in the serum of patients with lung cancer and may be exploited as screening biomarkers. J Oncol. (2019) 2019:8970645. doi: 10.1155/2019/8970645
132. Liu X, Wang L, Cui W, Yuan X, Lin L, Cao Q, et al. Targeting ALDH1A1 by disulfiram/copper complex inhibits non-small cell lung cancer recurrence driven by ALDH-positive cancer stem cells. Oncotarget. (2016) 7:58516–30. doi: 10.18632/oncotarget.11305
133. Kang JH, Lee S-H, Lee J-S, Nam B, Seong TW, Son J, et al. Aldehyde dehydrogenase inhibition combined with phenformin treatment reversed nSCLC through aTP depletion. Oncotarget. (2016) 7:49397–410. doi: 10.18632/oncotarget.10354
134. Wang N-N, Wang L-H, Li Y, Fu S-Y, Xue X, Jia L-N, et al. Targeting ALDH2 with disulfiram/copper reverses the resistance of cancer cells to microtubule inhibitors. Exp Cell Res. (2018) 362:72–82. doi: 10.1016/j.yexcr.2017.11.004
135. Morise M, Hishida T, Takahashi A, Yoshida J, Ohe Y, Nagai K, et al. CLinicopathological significance of cancer stem-like cell markers in high-grade neuroendocrine carcinoma of the lung. J Cancer Res Clin Oncol. (2015) 141:2121–30. doi: 10.1007/s00432-015-1985-3
136. Li S-J, Huang J, Zhou X-D, Zhang W-B, Lai Y-T, Che G-W. CLinicopathological and prognostic significance of oct-4 expression in patients with non-small cell lung cancer: a systematic review and meta-analysis. J Thorac Dis. (2016) 8:1587–600. doi: 10.21037/jtd.2016.06.01
137. Kouros-Mehr H, Bechis SK, Slorach EM, Littlepage LE, Egeblad M, Ewald AJ, et al. GATA-3 links tumor differentiation and dissemination in a luminal breast cancer model. Cancer Cell. (2008) 13:141–52. doi: 10.1016/j.ccr.2008.01.011
138. Barnawi R, Al-Khaldi S, Colak D, Tulbah A, Al-Tweigeri T, Fallatah M, et al. β1 Integrin is essential for fascin-mediated breast cancer stem cell function and disease progression. Int J Cancer. (2019) 145:830–41. doi: 10.1002/ijc.32183
139. Da Cruz Paula A, Leitão C, Marques O, Rosa AM, Santos AH, Rêma A, et al. Molecular characterization of CD44+/CD24-/Ck+/CD45- cells in benign and malignant breast lesions. Virchows Arch. (2017) 470:311–22. doi: 10.1007/s00428-017-2068-4
140. Nam K, Oh S, Lee KM, Yoo SA, Shin I. CD44 regulates cell proliferation, migration, and invasion via modulation of c-Src transcription in human breast cancer cells. Cell Signal. (2015) 27:1882–94. doi: 10.1016/j.cellsig.2015.05.002
141. Zhang H, Brown RL, Wei Y, Zhao P, Liu S, Liu X, et al. CD44 splice isoform switching determines breast cancer stem cell state. Genes Dev. (2019) 33:166–79. doi: 10.1101/gad.319889.118
142. Zhang L, Xu L, Zhang F, Vlashi E. Doxycycline inhibits the cancer stem cell phenotype and epithelial-to-mesenchymal transition in breast cancer. Cell Cycle. (2017) 16:737–45. doi: 10.1080/15384101.2016.1241929
143. Ge G, Zhou C, Ren Y, Tang X, Wang K, Zhang W, et al. Enhanced SLC34A2 in breast cancer stem cell-like cells induces chemotherapeutic resistance to doxorubicin via SLC34A2-Bmi1-ABCC5 signaling. Tumour Biol. (2016) 37:5049–62. doi: 10.1007/s13277-015-4226-0
144. Colacino JA, Azizi E, Brooks MD, Harouaka R, Fouladdel S, MCDermott SP, et al. Heterogeneity of human breast stem and progenitor cells as revealed by transcriptional profiling. Stem Cell Reports. (2018) 10:1596–609. doi: 10.1016/j.stemcr.2018.03.001
145. Hu J, Li G, Zhang P, Zhuang X, Hu G. A CD44v+ subpopulation of breast cancer stem-like cells with enhanced lung metastasis capacity. Cell Death Dis. (2017) 8:e2679. doi: 10.1038/cddis.2017.72
146. Ji P, Zhang Y, Wang S-J, Ge H-L, Zhao G-P, Xu Y-C, et al. CD44hiCD24lo mammosphere-forming cells from primary breast cancer display resistance to multiple chemotherapeutic drugs. Oncol Rep. (2016) 35:3293–302. doi: 10.3892/or.2016.4739
147. Li W, Ma H, Zhang J, Zhu L, Wang C, Yang Y. Unraveling the roles of CD44/CD24 and ALDH1 as cancer stem cell markers in tumorigenesis and metastasis. Sci. Rep. (2017) 7:13856. doi: 10.1038/s41598-017-14364-2
148. Louhichi T, Ziadi S, Saad H, Dhiab MB, Mestiri S, Trimeche M. CLinicopathological significance of cancer stem cell markers CD44 and ALDH1 expression in breast cancer. Breast Cancer. (2018) 25:698–705. doi: 10.1007/s12282-018-0875-3
149. Cho Y, Lee H-W, Kang H-G, Kim H-Y, Kim S-J, Chun K-H. Cleaved CD44 intracellular domain supports activation of stemness factors and promotes tumorigenesis of breast cancer. Oncotarget. (2015) 6:8709–21. doi: 10.18632/oncotarget.3325
150. Jin J, Krishnamachary B, Mironchik Y, Kobayashi H, Bhujwalla ZM. Phototheranostics of CD44-positive cell populations in triple negative breast cancer. Sci. Rep. (2016) 6:27871. doi: 10.1038/srep27871
151. Qiu Y, Zhou B, Yang X, Long D, Hao Y, Yang P. Novel single-cell analysis platform based on a solid-State zinc-Coadsorbed carbon quantum dots electrochemiluminescence probe for the evaluation of CD44 expression on breast cancer cells. ACS Appl Mater Interfaces. (2017) 9:16848–56. doi: 10.1021/acsami.7b02793
152. Wang Z, Sau S, Alsaab HO, Iyer AK. CD44 directed nanomicellar payload delivery platform for selective anticancer effect and tumor specific imaging of triple negative breast cancer. Nanomedicine. (2018) 14:1441–54. doi: 10.1016/j.nano.2018.04.004
153. Yaghjyan L, Stoll E, Ghosh K, Scott CG, Jensen MR, Brandt KR, et al. Tissue-based associations of mammographic breast density with breast stem cell markers. Breast Cancer Res. (2017) 19:100. doi: 10.1186/s13058-017-0889-3
154. Yang R-M, Fu C-P, Fang J-Z, Xu X-D, Wei X-H, Tang W-J, et al. Hyaluronan-modified superparamagnetic iron oxide nanopartiCLes for bimodal breast cancer imaging and photothermal therapy. Int J Nanomedicine. (2017) 12:197–206. doi: 10.2147/IJN.S121249
155. MunTIMadugu E, Kumar R, Saladi S, Rafeeqi TA, Khan W. CD44 targeted chemotherapy for co-eradication of breast cancer stem cells and cancer cells using polymeric nanopartiCLes of salinomycin and paclitaxel. Colloids Surf B Biointerfaces. (2016) 143:532–46. doi: 10.1016/j.colsurfb.2016.03.075
156. Agrawal S, Dwivedi M, Ahmad H, Chadchan SB, Arya A, Sikandar R, et al. CD44 targeting hyaluronic acid coated lapatinib nanocrystals foster the efficacy against triple-negative breast cancer. Nanomedicine. (2018) 14:327–37. doi: 10.1016/j.nano.2017.10.010
157. Aguirre-Alvarado C, Segura-Cabrera A, Velázquez-Quesada I, Hernández-Esquivel MA, García-Pérez CA, Guerrero-Rodríguez SL, et al. Virtual screening-driven repositioning of etoposide as CD44 antagonist in breast cancer cells. Oncotarget. (2016) 7:23772–84. doi: 10.18632/oncotarget.8180
158. Chen J, He H, Deng C, Yin L, Zhong Z. Saporin-loaded CD44 and EGFR dual-targeted nanogels for potent inhibition of metastatic breast cancer in vivo. Int J Pharm. (2019) 560:57–64. doi: 10.1016/j.ijpharm.2019.01.040
159. Chen J, Ouyang J, Chen Q, Deng C, Meng F, Zhang J, et al. EGFR and CD44 dual-Targeted multifunctional hyaluronic acid nanogels boost protein delivery to ovarian and breast cancers in vitro and in vivo. ACS Appl Mater Interfaces. (2017) 9:24140–7. doi: 10.1021/acsami.7b06879
160. Fan W, Wang X, Ding B, Cai H, Wang X, Fan Y, et al. Thioaptamer-conjugated CD44-targeted delivery system for the treatment of breast cancer in vitro and in vivo. J Drug Target. (2016) 24:359–71. doi: 10.3109/1061186X.2015.1077850
161. Fan Y, Wang Q, Lin G, Shi Y, Gu Z, Ding T. Combination of using prodrug-modified cationic liposome nanocomplexes and a potentiating strategy via targeted co-delivery of gemcitabine and docetaxel for CD44-overexpressed triple negative breast cancer therapy. Acta Biomater. (2017) 62:257–72. doi: 10.1016/j.actbio.2017.08.034
162. Fu W, Sun H, Zhao Y, Chen M, Yang L, Yang X, et al. Targeted delivery of CD44s-siRNA by ScFv overcomes de novo resistance to cetuximab in triple negative breast cancer. Mol Immunol. (2018) 99:124–33. doi: 10.1016/j.molimm.2018.05.010
163. Han N-K, Shin DH, Kim JS, Weon KY, Jang C-Y, Kim J-S. Hyaluronan-conjugated liposomes encapsulating gemcitabine for breast cancer stem cells. Int J Nanomedicine. (2016) 11:1413–25. doi: 10.2147/IJN.S95850
164. Liang D-S, Zhang W-J, Wang A-T, Su H-T, Zhong H-J, Qi X-R. Treating metastatic triple negative breast cancer with CD44/neuropilin dual molecular targets of multifunctional nanopartiCLes. Biomaterials. (2017) 137:23–36. doi: 10.1016/j.biomaterials.2017.05.022
165. Xie X, Huang X, Tang H, Ye F, Yang L, Guo X, et al. Diallyl disulfide inhibits breast cancer stem cell progression and glucose metabolism by targeting CD44/PKM2/AMPK signaling. Curr Cancer Drug Targets. (2018) 18:592–9. doi: 10.2174/1568009617666171024165657
166. Yang C, He Y, Zhang H, Liu Y, Wang W, Du Y, et al. Selective killing of breast cancer cells expressing activated CD44 using CD44 ligand-coated nanopartiCLes in vitro and in vivo. Oncotarget. (2015) 6:15283–96. doi: 10.18632/oncotarget.3681
167. McFarlane S, Coulter JA, Tibbits P, O'Grady A, McFarlane C, Montgomery N, et al. CD44 increases the efficiency of distant metastasis of breast cancer. Oncotarget. (2015) 6:11465–76. doi: 10.18632/oncotarget.3410
168. Rico MJ, Perroud HA, Herrera C, Alasino CM, Roggero EA, Pezzotto SM, et al. Putative biomarkers of response to treatment in breast cancer patients: a pilot assay. Cancer Invest. (2017) 35:377–85. doi: 10.1080/07357907.2017.1309545
169. Sanmartín E, Ortiz-Martínez F, Pomares-Navarro E, García-Martínez A, Rodrigo-Baños M, García-Escolano M, et al. CD44 induces FOXP3 expression and is related with favorable outcome in breast carcinoma. Virchows Arch. (2017) 470:81–90. doi: 10.1007/s00428-016-2045-3
170. Seo AN, Lee HJ, Kim EJ, Jang MH, Kim YJ, Kim JH, et al. Expression of breast cancer stem cell markers as predictors of prognosis and response to trastuzumab in HER2-positive breast cancer. Br J Cancer. (2016) 114:1109–16. doi: 10.1038/bjc.2016.101
171. Da Cruz Paula A, Marques O, Sampaio R, Rosa A, Garcia J, Rêma A, et al. Characterization of CD44+ALDH1+Ki-67- cells in non-malignant and neoplastic lesions of the breast. Anticancer Res. (2016) 36:4629–38. doi: 10.21873/anticanres.11013
172. Rabinovich I, Sebastião AP, Lima RS, Urban CD, Junior ES, Anselmi KF, et al. Cancer stem cell markers ALDH1 and CD44+/CD24- phenotype and their prognosis impact in invasive ductal carcinoma. Eur J Histochem. (2018) 62:2943. doi: 10.4081/ejh.2018.2943
173. Yaghjyan L, Esnakula AK, Scott CG, Wijayabahu AT, Jensen MR, Vachon CM. Associations of mammographic breast density with breast stem cell marker-defined breast cancer subtypes. Cancer Causes Control. (2019) 30:1103–11. doi: 10.1007/s10552-019-01207-w
174. Gómez-Miragaya J, Palafox M, Paré L, Yoldi G, Ferrer I, Vila S, et al. Resistance to taxanes in triple-negative breast cancer associates with the dynamics of a CD49f+ tumor-Initiating population. Stem Cell Reports. (2017) 8:1392–407. doi: 10.1016/j.stemcr.2017.03.026
175. Ye F, Zhong X, Qiu Y, Yang L, Wei B, Zhang Z, et al. CD49f can act as a biomarker for local or distant recurrence in breast cancer. J Breast Cancer. (2017) 20:142–9. doi: 10.4048/jbc.2017.20.2.142
176. Gomez-Miragaya J, González-Suárez E. Tumor-initiating CD49f cells are a hallmark of chemoresistant triple negative breast cancer. Mol Cell Oncol. (2017) 4:e1338208. doi: 10.1080/23723556.2017.1338208
177. Krebsbach PH, Villa-Diaz LG. The role of integrin α6 (CD49f) in stem cells: more than a conserved biomarker. Stem Cells Dev. (2017) 26:1090–9. doi: 10.1089/scd.2016.0319
178. Hu T, Zhou R, Zhao Y, Wu G. Integrin α6/Akt/Erk signaling is essential for human breast cancer resistance to radiotherapy. Sci. Rep. (2016) 6:33376. doi: 10.1038/srep33376
179. Ye F, Qiu Y, Li L, Yang L, Cheng F, Zhang H, et al. The presence of epCAM(-)/CD49f(+) cells in breast cancer is associated with a poor clinical outcome. J Breast Cancer. (2015) 18:242–8. doi: 10.4048/jbc.2015.18.3.242
180. Yeo SK, Wen J, Chen S, Guan J-L. Autophagy differentially regulates distinct breast cancer stem-like cells in murine models via EGFR/Stat3 and Tgfβ/Smad signaling. Cancer Res. (2016) 76:3397–410. doi: 10.1158/0008-5472.CAN-15-2946
181. Liu L, Yin B, Yi Z, Liu X, Hu Z, Gao W, et al. Breast cancer stem cells characterized by CD70 expression preferentially metastasize to the lungs. Breast Cancer. (2018) 25:706–16. doi: 10.1007/s12282-018-0880-6
182. Wang X, Liu Y, Zhou K, Zhang G, Wang F, Ren J. Isolation and characterization of CD105+/CD90+ subpopulation in breast cancer mDA-MB-231 cell line. Int J Clin Exp Pathol. (2015) 8:5105–12.
183. Sansone P, Berishaj M, Rajasekhar VK, Ceccarelli C, Chang Q, Strillacci A, et al. Evolution of cancer stem-like cells in endocrine-resistant metastatic breast cancers is mediated by stromal microvesiCLes. Cancer Res. (2017) 77:1927–41. doi: 10.1158/0008-5472.CAN-16-2129
184. Brugnoli F, Grassilli S, Al-Qassab Y, Capitani S, Bertagnolo V. CD133 in breast cancer cells: more than a stem cell marker. J Oncol. (2019) 2019:7512632. doi: 10.1155/2019/7512632
185. Zhang D, Sun B, Zhao X, Ma Y, Ji R, Gu Q, et al. Twist1 expression induced by sunitinib accelerates tumor cell vasculogenic mimicry by increasing the population of CD133+ cells in triple-negative breast cancer. Mol Cancer. (2014) 13:207. doi: 10.1186/1476-4598-13-207
186. Brugnoli F, Grassilli S, Lanuti P, Marchisio M, Al-Qassab Y, Vezzali F, et al. Up-modulation of pLC-β2 reduces the number and malignancy of triple-negative breast tumor cells with a CD133+/EpCAM+ phenotype: a promising target for preventing progression of TNBC. BMC Cancer. (2017) 17:617. doi: 10.1186/s12885-017-3592-y
187. Sansone P, Ceccarelli C, Berishaj M, Chang Q, Rajasekhar VK, PeRNA F, et al. Self-renewal of CD133(hi) cells by IL6/Notch3 signalling regulates endocrine resistance in metastatic breast cancer. Nat Commun. (2016) 7:10442. doi: 10.1038/ncomms10442
188. Swaminathan SK, Roger E, Toti U, Niu L, Ohlfest JR, Panyam J. CD133-targeted paCLitaxel delivery inhibits local tumor recurrence in a mouse model of breast cancer. J Control Release. (2013) 171:280–7. doi: 10.1016/j.jconrel.2013.07.014
189. Brugnoli F, Grassilli S, Piazzi M, Palomba M, Nika E, Bavelloni A, et al. In triple negative breast tumor cells, pLC-β2 promotes the conversion of CD133high to CD133low phenotype and reduces the CD133-related invasiveness. Mol Cancer. (2013) 12:165. doi: 10.1186/1476-4598-12-165
190. Latorre E, Carelli S, Raimondi I, D'Agostino V, Castiglioni I, Zucal C, et al. The ribonucleic complex HuR-MALAT1 represses CD133 expression and suppresses epithelial-Mesenchymal transition in breast cancer. Cancer Res. (2016) 76:2626–36. doi: 10.1158/0008-5472.CAN-15-2018
191. Bock C, Kuhn C, Ditsch N, Krebold R, Heublein S, Mayr D, et al. Strong correlation between n-cadherin and CD133 in breast cancer: role of both markers in metastatic events. J Cancer Res Clin Oncol. (2014) 140:1873–81. doi: 10.1007/s00432-014-1750-z
192. Nozaki Y, Tamori S, Inada M, Katayama R, Nakane H, Minamishima O, et al. Correlation between c-Met and ALDH1 contributes to the survival and tumor-sphere formation of ALDH1 positive breast cancer stem cells and predicts poor Clinical outcome in breast cancer. Genes Cancer. (2017) 8:628–39. doi: 10.18632/genesandcancer.148
193. Joseph C, Arshad M, Kurozomi S, Althobiti M, Miligy IM, Al-Izzi S, et al. Overexpression of the cancer stem cell marker CD133 confers a poor prognosis in invasive breast cancer. Breast Cancer Res Treat. (2019) 174:387–99. doi: 10.1007/s10549-018-05085-9
194. Zhang M, Tsimelzon A, Chang C-H, Fan C, Wolff A, Perou CM, et al. Intratumoral heterogeneity in a trp53-null mouse model of human breast cancer. Cancer Discov. (2015) 5:520–33. doi: 10.1158/2159-8290.CD-14-1101
195. Yang L, Tang H, Kong Y, Xie X, Chen J, Song C, et al. LGR5 promotes breast cancer progression and maintains stem-Like cells through activation of wnt/β-Catenin signaling. Stem Cells. (2015) 33:2913–24. doi: 10.1002/stem.2083
196. Wang D, Cai C, Dong X, Yu QC, Zhang X-O, Yang L, et al. Identification of multipotent mammary stem cells by protein C receptor expression. Nature. (2015) 517:81–4. doi: 10.1038/nature13851
197. Ginestier C, Hur MH, Charafe-Jauffret E, Monville F, Dutcher J, Brown M, et al. ALDH1 is a marker of normal and malignant human mammary stem cells and a predictor of poor clinical outcome. Cell Stem Cell. (2007) 1:555–67. doi: 10.1016/j.stem.2007.08.014
198. Kim R-J, Park J-R, Roh K-J, Choi A-R, Kim S-R, Kim P-H, et al. High aldehyde dehydrogenase activity enhances stem cell features in breast cancer cells by activating hypoxia-inducible factor-2α. Cancer Lett. (2013) 333:18–31. doi: 10.1016/j.canlet.2012.11.026
199. Rodriguez-Torres M, Allan AL. Aldehyde dehydrogenase as a marker and functional mediator of metastasis in solid tumors. Clin Exp Metastasis. (2016) 33:97–113. doi: 10.1007/s10585-015-9755-9
200. Tomita H, Tanaka K, Tanaka T, Hara A. Aldehyde dehydrogenase 1A1 in stem cells and cancer. Oncotarget. (2016) 7:11018–32. doi: 10.18632/oncotarget.6920
201. Liu P Kumar IS Brown S Kannappan V Tawari PE Tang JZ . Disulfiram targets cancer stem-like cells and reverses resistance and cross-resistance in acquired paCLitaxel-resistant triple-negative breast cancer cells. Br J Cancer. (2013) 109:1876–85. doi: 10.1038/bjc.2013.534
202. Matsunaga N, Ogino T, Hara Y, Tanaka T, Koyanagi S, Ohdo S. Optimized dosing schedule based on circadian dynamics of mouse breast cancer stem cells improves the antitumor effects of aldehyde dehydrogenase inhibitor. Cancer Res. (2018) 78:3698–708. doi: 10.1158/0008-5472.CAN-17-4034
203. Charafe-Jauffret E, Ginestier C, Iovino F, Tarpin C, Diebel M, Esterni B, et al. Aldehyde dehydrogenase 1-positive cancer stem cells mediate metastasis and poor Clinical outcome in inflammatory breast cancer. Clin Cancer Res. (2010) 16:45–55. doi: 10.1158/1078-0432.CCR-09-1630
204. Liu Y, Lv D-l, Duan J-j, Xu S-l, Zhang J-f, Yang X-j, et al. ALDH1A1 expression correlates with CLinicopathologic features and poor prognosis of breast cancer patients: a systematic review and meta-analysis. BMC Cancer. (2014) 14:444. doi: 10.1186/1471-2407-14-444
205. Marcato P, Dean CA, Liu R-Z, Coyle KM, Bydoun M, Wallace M, et al. Aldehyde dehydrogenase 1A3 influences breast cancer progression via differential retinoic acid signaling. Mol Oncol. (2015) 9:17–31. doi: 10.1016/j.molonc.2014.07.010
206. Khoury T, Ademuyiwa FO, Chandrasekhar R, Chandraseekhar R, Jabbour M, Deleo A, et al. Aldehyde dehydrogenase 1A1 expression in breast cancer is associated with stage, triple negativity, and outcome to neoadjuvant chemotherapy. Mod Pathol. (2012) 25:388–97. doi: 10.1038/modpathol.2011.172
207. Woodward WA, Krishnamurthy S, Lodhi A, Xiao L, Gong Y, Cristofanilli M, et al. Aldehyde dehydrogenase1 immunohistochemical staining in primary breast cancer cells independently predicted overall survival but did not correlate with the presence of circulating or disseminated tumors cells. J Cancer. (2014) 5:360–7. doi: 10.7150/jca.7885
208. Zhong Y, Shen S, Zhou Y, Mao F, Guan J, Lin Y, et al. ALDH1 is a better clinical indicator for relapse of invasive ductal breast cancer than the CD44+/CD24- phenotype. Med Oncol. (2014) 31:864. doi: 10.1007/s12032-014-0864-0
209. Tan EY, Thike AA, Tan PH. ALDH1 expression is enriched in breast cancers arising in young women but does not predict outcome. Br J Cancer. (2013) 109:109–13. doi: 10.1038/bjc.2013.297
210. Collina F, Di Bonito M, Li Bergolis V, Laurentiis M de, Vitagliano C, Cerrone M, et al. Prognostic value of cancer stem cells markers in triple-negative breast cancer. Biomed Res Int. (2015) 2015:158682. doi: 10.1155/2015/158682
211. Srinivasan M, Bharali DJ, Sudha T, Khedr M, Guest I, Sell S, et al. Downregulation of BMI1 in breast cancer stem cells suppresses tumor growth and proliferation. Oncotarget. (2017) 8:38731–42. doi: 10.18632/oncotarget.16317
212. Kim S-H, Singh SV. The role of polycomb group protein BMI-1 and notch4 in breast cancer stem cell inhibition by benzyl isothiocyanate. Breast Cancer Res Treat. (2015) 149:681–92. doi: 10.1007/s10549-015-3279-5
213. Yuan W, Yuan Y, Zhang T, Wu S. Role of BMI-1 in regulation of ionizing irradiation-induced epithelial-mesenchymal transition and migration of breast cancer cells. PLoS ONE. (2015) 10:e0118799. doi: 10.1371/jouRNAl.pone.0118799
214. Yan Y, Wang Y, Zhao P, Ma W, Hu Z, Zhang K. BMI-1 promotes self-Renewal of radio- and temozolomide (TMZ)-Resistant breast cancer cells. Reprod Sci. (2017) 24:1620–9. doi: 10.1177/1933719117697255
215. Gong X-F, Yu A-L, Tang J, Wang C-L, He J-R, Chen G-Q, et al. MicroRNA-630 inhibits breast cancer progression by directly targeting BMI1. Exp Cell Res. (2018) 362:378–85. doi: 10.1016/j.yexcr.2017.11.039
216. Griffith J, Andrade D, Mehta M, Berry W, Benbrook DM, Aravindan N, et al. Silencing BMI1 radiosensitizes human breast cancer cells by inducing DNA damage and autophagy. Oncol Rep. (2017) 37:2382–90. doi: 10.3892/or.2017.5478
217. Ojo D, Lin X, Wu Y, Cockburn J, Bane A, Tang D. Polycomb complex protein BMI1 confers resistance to tamoxifen in estrogen receptor positive breast cancer. Cancer Lett. (2018) 426:4–13. doi: 10.1016/j.canlet.2018.03.048
218. Elango R, Vishnubalaji R, Manikandan M, Binhamdan SI, Siyal A-A, Alshawakir YA, et al. Concurrent targeting of BMI1 and CDK4/6 abrogates tumor growth in vitro and in vivo. Sci. Rep. (2019) 9:13696. doi: 10.1038/s41598-019-50140-0
219. Janaki Ramaiah M, Vaishnave S. BMI1 and PTEN are key determinants of breast cancer therapy: a plausible therapeutic target in breast cancer. Gene. (2018) 678:302–11. doi: 10.1016/j.gene.2018.08.022
220. Wang D, Lu P, Zhang H, Luo M, Zhang X, Wei X, et al. Oct-4 and nanog promote the epithelial-mesenchymal transition of breast cancer stem cells and are associated with poor prognosis in breast cancer patients. Oncotarget. (2014) 5:10803–15. doi: 10.18632/oncotarget.2506
221. Yang F, Zhang J, Yang H. OCT4, SOX2, and NANOG positive expression correlates with poor differentiation, advanced disease stages, and worse overall survival in HER2+ breast cancer patients. Onco Targets Ther. (2018) 11:7873–81. doi: 10.2147/OTT.S173522
222. D'Angelo RC, Ouzounova M, Davis A, Choi D, Tchuenkam SM, Kim G, et al. Notch reporter activity in breast cancer cell lines identifies a subset of cells with stem cell activity. Mol Cancer Ther. (2015) 14:779–87. doi: 10.1158/1535-7163.MCT-14-0228
223. Rodilla V, Dasti A, Huyghe M, Lafkas D, Laurent C, Reyal F, et al. Luminal progenitors restrict their lineage potential during mammary gland development. PLoS Biol. (2015) 13:e1002069. doi: 10.1371/jouRNAl.pbio.1002069
224. Mamaeva V, Niemi R, Beck M, Özliseli E, Desai D, Landor S, et al. Inhibiting notch activity in breast cancer stem cells by glucose functionalized nanoparticles carrying γ-secretase inhibitors. Mol Ther. (2016) 24:926–36. doi: 10.1038/mt.2016.42
225. Anjanappa M, Hao Y, Simpson ER, Bhat-Nakshatri P, Nelson JB, Tersey SA, et al. A system for detecting high impact-low frequency mutations in primary tumors and metastases. Oncogene. (2018) 37:185–96. doi: 10.1038/onc.2017.322
226. Baker A, Wyatt D, Bocchetta M, Li J, Filipovic A, Green A, et al. Notch-1-PTEN-ERK1/2 signaling axis promotes hER2+ breast cancer cell proliferation and stem cell survival. Oncogene. (2018) 37:4489–504. doi: 10.1038/s41388-018-0251-y
227. Diluvio G, Del Gaudio F, Giuli MV, Franciosa G, Giuliani E, Palermo R, et al. NOTCH3 inactivation increases triple negative breast cancer sensitivity to gefitinib by promoting eGFR tyrosine dephosphorylation and its intracellular arrest. Oncogenesis. (2018) 7:42. doi: 10.1038/s41389-018-0051-9
228. Ran Y, Hossain F, Pannuti A, Lessard CB, Ladd GZ, Jung J, et al. γ-Secretase inhibitors in cancer clinical trials are pharmacologically and functionally distinct. Embo Mol Med. (2017) 9:950–66. doi: 10.15252/emmm.201607265
229. Sizemore GM, Balakrishnan S, Hammer AM, Thies KA, Trimboli AJ, Wallace JA, et al. Stromal pTEN inhibits the expansion of mammary epithelial stem cells through jagged-1. Oncogene. (2017) 36:2297–308. doi: 10.1038/onc.2016.383
230. Bhola NE, Jansen VM, Koch JP, Li H, Formisano L, Williams JA, et al. Treatment of triple-Negative breast cancer with TORC1/2 inhibitors sustains a drug-Resistant and notch-dependent cancer stem cell population. Cancer Res. (2016) 76:440–52. doi: 10.1158/0008-5472.CAN-15-1640-T
231. Leontovich AA, Jalalirad M, Salisbury JL, Mills L, Haddox C, Schroeder M, et al. NOTCH3 expression is linked to breast cancer seeding and distant metastasis. Breast Cancer Res. (2018) 20:105. doi: 10.1186/s13058-018-1020-0
232. Dou X-W, Liang Y-K, Lin H-Y, Wei X-L, Zhang Y-Q, Bai J-W, et al. Notch3 maintains luminal phenotype and suppresses tumorigenesis and metastasis of breast cancer via trans-activating estrogen receptor-α. Theranostics. (2017) 7:4041–56. doi: 10.7150/thno.19989
233. Wieland E, Rodriguez-Vita J, Liebler SS, Mogler C, Moll I, Herberich SE, et al. Endothelial notch1 activity facilitates metastasis. Cancer Cell. (2017) 31:355–67. doi: 10.1016/j.ccell.2017.01.007
234. Gu X, Lu C, He D, Lu Y, Jin J, Liu D, et al. Notch3 negatively regulates chemoresistance in breast cancers. Tumour Biol. (2016) 37. doi: 10.1007/s13277-016-5412-4
235. Janghorban M, Xin L, Rosen JM, Zhang XH-F. Notch signaling as a regulator of the tumor immune response: to target or not to target? Front Immunol. (2018) 9:1649. doi: 10.3389/fimmu.2018.01649
236. Siddharth S, Goutam K, Das S, Nayak A, Nayak D, Sethy C, et al. Nectin-4 is a breast cancer stem cell marker that induces wNT/β-catenin signaling via pi3k/Akt axis. Int J Biochem Cell Biol. (2017) 89:85–94. doi: 10.1016/j.biocel.2017.06.007
237. Jang G-B, Hong I-S, Kim R-J, Lee S-Y, Park S-J, Lee E-S, et al. Wnt/β-catenin small-molecule inhibitor CWP232228 preferentially inhibits the growth of breast cancer stem-like cells. Cancer Res. (2015) 75:1691–702. doi: 10.1158/0008-5472.CAN-14-2041
238. Zhang C, Li C, He F, Cai Y, Yang H. Identification of CD44+CD24+ gastric cancer stem cells. J Cancer Res Clin Oncol. (2011) 137:1679–86. doi: 10.1007/s00432-011-1038-5
239. Brungs D, Aghmesheh M, Vine KL, Becker TM, Carolan MG, Ranson M. Gastric cancer stem cells: evidence, potential markers, and clinical implications. J Gastroenterol. (2016) 51:313–26. doi: 10.1007/s00535-015-1125-5
240. Li K, Dan Z, Nie Y-Q. Gastric cancer stem cells in gastric carcinogenesis, progression, prevention and treatment. World J Gastroenterol. (2014) 20:5420–6. doi: 10.3748/wjg.v20.i18.5420
241. Jiao X-L, Zhao C, Niu M, Chen D. Downregulation of CD24 inhibits invasive growth, facilitates apoptosis and enhances chemosensitivity in gastric cancer AGS cells. Eur Rev Med Pharmacol Sci. (2013) 17:1709–15.
242. Fujikuni N, Yamamoto H, Tanabe K, Naito Y, Sakamoto N, Tanaka Y, et al. Hypoxia-mediated CD24 expression is correlated with gastric cancer aggressiveness by promoting cell migration and invasion. Cancer Sci. (2014) 105:1411–20. doi: 10.1111/cas.12522
243. Wu J-X, Zhao Y-Y, Wu X, An H-X. CLinicopathological and prognostic significance of CD24 overexpression in patients with gastric cancer: a meta-analysis. PLoS ONE. (2014) 9:e114746. doi: 10.1371/jouRNAl.pone.0114746
244. Zhao H, Wen J, Dong X, He R, Gao C, Zhang W, et al. Identification of AQP3 and CD24 as biomarkers for carcinogenesis of gastric intestinal metaplasia. Oncotarget. (2017) 8:63382–91. doi: 10.18632/oncotarget.18817
245. Fu Y, Du P, Zhao J, Hu C'e, Qin Y, Huang G. Gastric cancer stem cells: mechanisms and therapeutic approaches. Yonsei Med J. (2018) 59:1150–8. doi: 10.3349/ymj.2018.59.10.1150
246. Takaishi S, Okumura T, Tu S, Wang SS, Shibata W, Vigneshwaran R, et al. Identification of gastric cancer stem cells using the cell surface marker CD44. Stem Cells. (2009) 27:1006–20. doi: 10.1002/stem.30
247. Zhang X, Hua R, Wang X, Huang M, Gan L, Wu Z, et al. Identification of stem-like cells and clinical significance of candidate stem cell markers in gastric cancer. Oncotarget. (2016) 7:9815–31. doi: 10.18632/oncotarget.6890
248. Lau WM, Teng E, Chong HS, Lopez KA, Tay AY, Salto-Tellez M, et al. CD44v8-10 is a cancer-specific marker for gastric cancer stem cells. Cancer Res. (2014) 74:2630–41. doi: 10.1158/0008-5472.CAN-13-2309
249. Nguyen PH, Giraud J, Chambonnier L, Dubus P, Wittkop L, Belleannée G, et al. Characterization of biomarkers of tumorigenic and chemoresistant cancer stem cells in human gastric carcinoma. Clin Cancer Res. (2017) 23:1586–97. doi: 10.1158/1078-0432.CCR-15-2157
250. Nosrati A, Naghshvar F, Khanari S. Cancer stem cell markers CD44, CD133 in primary gastric adenocarcinoma. Int J Mol Cell Med. (2014) 3:279–86.
251. Shu X, Liu H, Pan Y, Sun L, Yu L, Sun L, et al. Distinct biological characterization of the CD44 and CD90 phenotypes of cancer stem cells in gastric cancer cell lines. Mol Cell Biochem. (2019) 459:35–47. doi: 10.1007/s11010-019-03548-1
252. Bekaii-Saab T, El-Rayes B. Identifying and targeting cancer stem cells in the treatment of gastric cancer. Cancer. (2017) 123:1303–12. doi: 10.1002/cncr.30538
253. Watanabe T, Okumura T, Hirano K, Yamaguchi T, Sekine S, Nagata T, et al. Circulating tumor cells expressing cancer stem cell marker CD44 as a diagnostic biomarker in patients with gastric cancer. Oncol Lett. (2017) 13:281–8. doi: 10.3892/ol.2016.5432
254. Lu L, Wu M, Sun L, Li W, Fu W, Zhang X, et al. CLinicopathological and prognostic significance of cancer stem cell markers CD44 and CD133 in patients with gastric cancer: a comprehensive meta-analysis with 4729 patients involved. Medicine. (2016) 95:e5163. doi: 10.1097/MD.0000000000005163
255. Jang E, Kim E, Son H-Y, Lim E-K, Lee H, Choi Y, et al. Nanovesicle-mediated systemic delivery of microRNA-34a for CD44 overexpressing gastric cancer stem cell therapy. Biomaterials. (2016) 105:12–24. doi: 10.1016/j.biomaterials.2016.07.036
256. Yao H-J, Zhang Y-G, Sun L, Liu Y. The effect of hyaluronic acid functionalized carbon nanotubes loaded with salinomycin on gastric cancer stem cells. Biomaterials. (2014) 35:9208–23. doi: 10.1016/j.biomaterials.2014.07.033
257. Chen H, Lin J, Shan Y, Zhengmao L. The promotion of nanopartiCLe delivery to two populations of gastric cancer stem cells by CD133 and CD44 antibodies. Biomed Pharmacothe. (2019) 115:108857. doi: 10.1016/j.biopha.2019.108857
258. Kodama H, Murata S, Ishida M, Yamamoto H, Yamaguchi T, Kaida S, et al. Prognostic impact of CD44-positive cancer stem-like cells at the invasive front of gastric cancer. Br J Cancer. (2017) 116:186–94. doi: 10.1038/bjc.2016.401
259. Yoon C, Park DJ, Schmidt B, Thomas NJ, Lee H-J, Kim TS, et al. CD44 expression denotes a subpopulation of gastric cancer cells in which hedgehog signaling promotes chemotherapy resistance. Clin Cancer Res. (2014) 20:3974–88. doi: 10.1158/1078-0432.CCR-14-0011
260. Senel F, Kökenek Unal TD, Karaman H, Inanç M, Aytekin A. Prognostic value of cancer stem cell markers CD44 and ALDH1/2 in gastric cancer cases. Asian Pac J Cancer Prev. (2017) 18:2527–31. doi: 10.22034/APJCP.2017.18.9.2527
261. Howard R, Al Diffalha S, Pimiento J, Mejia J, Enderling H, Giuliano A, et al. CD133 expression as a helicobacter pylori-independent biomarker of gastric cancer progression. Anticancer Res. (2018) 38:4443–8. doi: 10.21873/anticanres.12746
262. Xia P, Song C-L, Liu J-F, Wang D, Xu X-Y. Prognostic value of circulating CD133(+) cells in patients with gastric cancer. Cell Prolif . (2015) 48:311–7. doi: 10.1111/cpr.12175
263. Yiming L, Yunshan G, Bo M, Yu Z, Tao W, Gengfang L, et al. CD133 overexpression correlates with CLinicopathological features of gastric cancer patients and its impact on survival: a systematic review and meta-analysis. Oncotarget. (2015) 6:42019–27. doi: 10.18632/oncotarget.5714
264. Hashimoto K, Aoyagi K, Isobe T, Kouhuji K, Shirouzu K. Expression of CD133 in the cytoplasm is associated with cancer progression and poor prognosis in gastric cancer. Gastric Cancer. (2014) 17:97–106. doi: 10.1007/s10120-013-0255-9
265. Chen X-L, Chen X-Z, Wang Y-G, Du He, Lu Z-H, Liu K, et al. Clinical significance of putative markers of cancer stem cells in gastric cancer: a retrospective cohort study. Oncotarget. (2016) 7:62049–69. doi: 10.18632/oncotarget.11384
266. Fujita T, Chiwaki F, Takahashi R-U, Aoyagi K, Yanagihara K, Nishimura T, et al. Identification and characterization of CXCR4-Positive gastric cancer stem cells. PLoS ONE. (2015) 10:e0130808. doi: 10.1371/jouRNAl.pone.0130808
267. Xue L-J, Mao X-B, Ren L-L, Chu X-Y. Inhibition of CXCL12/CXCR4 axis as a potential targeted therapy of advanced gastric carcinoma. Cancer Med. (2017) 6:1424–36. doi: 10.1002/cam4.1085
268. Yang W, Lai Z, Li Y, Mu J, Yang M, Xie J, et al. Immune signature profiling identified prognostic factors for gastric cancer. Chin J Cancer Res. (2019) 31:463–70. doi: 10.21147/j.issn.1000-9604.2019.03.08
269. Yu C, Zhang Y. Characterization of the prognostic values of CXCR family in gastric cancer. Cytokine. (2019) 123:154785. doi: 10.1016/j.cyto.2019.154785
270. Jiang Q, Sun Y, Liu X. CXCR4 as a prognostic biomarker in gastrointestinal cancer: a meta-analysis. Biomarkers. (2019) 24:510–6. doi: 10.1080/1354750X.2019.1637941
271. Han M, Lv S, Zhang Y, Yi R, Huang B, Fu H, et al. The prognosis and CLinicopathology of CXCR4 in gastric cancer patients: a meta-analysis. Tumour Biol. (2014) 35:4589–97. doi: 10.1007/s13277-013-1603-4
272. Dai M, Yuan F, Fu C, Shen G, Hu S, Shen G. Relationship between epithelial cell adhesion molecule (EpCAM) overexpression and gastric cancer patients: a systematic review and meta-analysis. PLoS ONE. (2017) 12:e0175357. doi: 10.1371/jouRNAl.pone.0175357
273. Knödler M, Körfer J, Kunzmann V, Trojan J, Daum S, Schenk M, et al. Randomised phase iI trial to investigate catumaxomab (anti-EpCAM × anti-CD3) for treatment of peritoneal carcinomatosis in patients with gastric cancer. Br J Cancer. (2018) 119:296–302. doi: 10.1038/s41416-018-0150-6
274. Nakajima T, Uehara T, Maruyama Y, Iwaya M, Kobayashi Y, Ota H. Distribution of Lgr5-positive cancer cells in intramucosal gastric signet-ring cell carcinoma. Pathol Int. (2016) 66:518–23. doi: 10.1111/pin.12451
275. Xi HQ, Cai AZ, Wu XS, Cui JX, Shen WS, Bian SB, et al. Leucine-rich repeat-containing g-protein-coupled receptor 5 is associated with invasion, metastasis, and could be a potential therapeutic target in human gastric cancer. Br J Cancer. (2014) 110:2011–20. doi: 10.1038/bjc.2014.112
276. Gong X, Azhdarinia A, Ghosh SC, Xiong W, An Z, Liu Q, et al. LGR5-Targeted antibody-drug conjugate eradicates gastrointestinal tumors and prevents recurrence. Mol Cancer Ther. (2016) 15:1580–90. doi: 10.1158/1535-7163.MCT-16-0114
277. Xi H-Q, Cui J-X, Shen W-S, Wu X-S, Bian S-B, Li J-Y, et al. Increased expression of Lgr5 is associated with chemotherapy resistance in human gastric cancer. Oncol Rep. (2014) 32:181–8. doi: 10.3892/or.2014.3207
278. Liu X-S, Lin X-K, Mei Y, Ahmad S, Yan C-X, Jin H-L, et al. Regulatory T cells promote overexpression of Lgr5 on gastric cancer cells via TGF-beta1 and confer poor prognosis in gastric cancer. Front Immunol. (2019) 10:1741. doi: 10.3389/fimmu.2019.01741
279. Huang T, Qiu X, Xiao J, Wang Q, Wang Y, Zhang Y, et al. The prognostic role of leucine-rich repeat-containing G-protein-coupled receptor 5 in gastric cancer: a systematic review with meta-analysis. Clin Res Hepatol Gastroenterol. (2016) 40:246–53. doi: 10.1016/j.CLinre.2015.07.009
280. Jo JH, Park SB, Park S, Lee HS, Kim C, Jung DE, et al. Novel gastric cancer stem cell-Related marker lINGO2 is associated with cancer cell phenotype and patient outcome. Int J Mol Sci. (2019) 20:555. doi: 10.3390/ijms20030555
281. Nishikawa S, Konno M, Hamabe A, Hasegawa S, Kano Y, Ohta K, et al. Aldehyde dehydrogenase high gastric cancer stem cells are resistant to chemotherapy. Int J Oncol. (2013) 42:1437–42. doi: 10.3892/ijo.2013.1837
282. Di Wu, Mou Y-P, Chen K, Cai J-Q, Zhou Y-C, Pan Y, et al. Aldehyde dehydrogenase 3A1 is robustly upregulated in gastric cancer stem-like cells and associated with tumorigenesis. Int J Oncol. (2016) 49:611–22. doi: 10.3892/ijo.2016.3551
283. Li H, Piao L, Xu D, Xuan Y. LETM1 is a potential biomarker that predicts poor prognosis in gastric adenocarcinoma. Exp Mol Pathol. (2019) 112:104333. doi: 10.1016/j.yexmp.2019.104333
284. Yang Z, Li J, Shi Y, Li L, Guo X. Increased musashi 2 expression indicates a poor prognosis and promotes malignant phenotypes in gastric cancer. Oncol Lett. (2019) 17:2599–606. doi: 10.3892/ol.2019.9889
285. Wang B, Chen Q, Cao Y, Ma X, Yin C, Jia Y, et al. LGR5 is a gastric cancer stem cell marker associated with stemness and the EMT signature genes NANOG, NANOGP8, PRRX1, TWIST1, and BMI1. PLoS ONE. (2016) 11:e0168904. doi: 10.1371/jouRNAl.pone.0168904
286. Santaliz-Ruiz LE, Xie X, Old M, Teknos TN, Pan Q. Emerging role of nanog in tumorigenesis and cancer stem cells. Int J Cancer. (2014) 135:2741–8. doi: 10.1002/ijc.28690
287. Lin T, Ding Y-Q, Li J-M. Overexpression of nanog protein is associated with poor prognosis in gastric adenocarcinoma. Med Oncol. (2012) 29:878–85. doi: 10.1007/s12032-011-9860-9
288. Basati G, Mohammadpour H, Emami Razavi A. Association of high expression levels of SOX2, NANOG, and OCT4 in gastric cancer tumor tissues with progression and poor prognosis. J Gastrointest Cancer. (2019) 51:41–7. doi: 10.1007/s12029-018-00200-x
289. Chen Z, Xu W-R, Qian H, Zhu W, Bu X-F, Wang S, et al. Oct4, a novel marker for human gastric cancer. J Surg Oncol. (2009) 99:414–9. doi: 10.1002/jso.21270
290. Carrasco-Garcia E, Álvarez-Satta M, García-Puga M, Ribeiro ML, Arevalo S, Arauzo-Bravo M, et al. Therapeutic relevance of SOX9 stem cell factor in gastric cancer. Expert Opin Ther Targets. (2019) 23:143–52. doi: 10.1080/14728222.2019.1559826
291. Luo J, Yan R, He X, He J. SOX2 inhibits cell proliferation and metastasis, promotes apoptotic by downregulating cCND1 and pARP in gastric cancer. Am J Transl Res. (2018) 10:639–47.
292. Hütz K, Mejías-Luque R, Farsakova K, Ogris M, Krebs S, Anton M, et al. The stem cell factor SOX2 regulates the tumorigenic potential in human gastric cancer cells. Carcinogenesis. (2014) 35:942–50. doi: 10.1093/carcin/bgt410
293. Chen Y, Huang Y, Zhu L, Chen M, Huang Y, Zhang J, et al. SOX2 inhibits metastasis in gastric cancer. J Cancer Res Clin Oncol. (2016) 142:1221–30. doi: 10.1007/s00432-016-2125-4
294. Tsai S-C, Lin C-C, Shih T-C, Tseng R-J, Yu M-C, Lin Y-J, et al. The miR-200b-ZEB1 circuit regulates diverse stemness of human hepatocellular carcinoma. Mol Carcinog. (2017) 56:2035–47. doi: 10.1002/mc.22657
295. Wang R, Sun Q, Wang P, Liu M, Xiong S, Luo J, et al. Notch and wnt/β-catenin signaling pathway play important roles in activating liver cancer stem cells. Oncotarget. (2016) 7:5754–68. doi: 10.18632/oncotarget.6805
296. Wang R, Li Y, Tsung A, Huang H, Du Q, Yang M, et al. iNOS promotes CD24+CD133+ liver cancer stem cell phenotype through a TACE/ADAM17-dependent notch signaling pathway. Proc Natl Acad Sci USA. (2018) 115:E10127–36. doi: 10.1073/pnas.1722100115
297. Sukowati CHC. Heterogeneity of hepatic cancer stem cells. Adv Exp Med Biol. (2019) 1139:59–81. doi: 10.1007/978-3-030-14366-4_4
298. Xiang Y, Yang T, Pang B-Y, Zhu Y, Liu YN. The progress and prospects of putative biomarkers for liver cancer stem cells in hepatocellular carcinoma. Stem Cells Int. (2016) 2016:7614971. doi: 10.1155/2016/7614971
299. Asai R, Tsuchiya H, Amisaki M, Makimoto K, Takenaga A, Sakabe T, et al. CD44 standard isoform is involved in maintenance of cancer stem cells of a hepatocellular carcinoma cell line. Cancer Med. (2019) 8:773–82. doi: 10.1002/cam4.1968
300. Liu R, Shen Y, Nan K, Mi B, Wu T, Guo J, et al. Association between expression of cancer stem cell markers and poor differentiation of hepatocellular carcinoma: a meta-analysis (PRISMA). Medicine. (2015) 94:e1306. doi: 10.1097/MD.0000000000001306
301. Choi GH, Kim GI, Yoo JE, Na DC, Han DH, Roh YH, et al. Increased expression of circulating cancer stem cell markers during the perioperative period predicts early recurrence after curative resection of hepatocellular carcinoma. Ann Surg Oncol. (2015) 22(Suppl. 3):S1444–52. doi: 10.1245/s10434-015-4480-9
302. Luo Y, Tan Y. Prognostic value of CD44 expression in patients with hepatocellular carcinoma: meta-analysis. Cancer Cell Int. (2016) 16:47. doi: 10.1186/s12935-016-0325-2
303. Morine Y, Imura S, Ikemoto T, Iwahashi S, Saito Yu, Shimada M. CD44 expression is a prognostic factor in patients with intrahepatic cholangiocarcinoma after surgical resection. Anticancer Res. (2017) 37:5701–5. doi: 10.21873/anticanres.12007
304. Kim BH, Park J-W, Kim JS, Lee S-K, Hong EK. Stem cell markers predict the response to sorafenib in patients with hepatocellular carcinoma. Gut Liver. (2019) 13:342–8. doi: 10.5009/gnl18345
305. Luo J, Wang P, Wang R, Wang J, Liu M, Xiong S, et al. The notch pathway promotes the cancer stem cell characteristics of CD90+ cells in hepatocellular carcinoma. Oncotarget. (2016) 7:9525–37. doi: 10.18632/oncotarget.6672
306. Yoshida M, Yamashita T, Okada H, Oishi N, Nio K, Hayashi T, et al. Sorafenib suppresses extrahepatic metastasis de novo in hepatocellular carcinoma through inhibition of mesenchymal cancer stem cells characterized by the expression of CD90. Sci. Rep. (2017) 7:11292. doi: 10.1038/s41598-017-11848-z
307. Zhang K, Che S, Su Z, Zheng S, Zhang H, Yang S, et al. CD90 promotes cell migration, viability and sphere-forming ability of hepatocellular carcinoma cells. Int J Mol Med. (2018) 41:946–54. doi: 10.3892/ijmm.2017.3314
308. Zhu L, Zhang W, Wang J, Liu R. Evidence of CD90+CXCR4+ cells as circulating tumor stem cells in hepatocellular carcinoma. Tumour Biol. (2015) 36:5353–60. doi: 10.1007/s13277-015-3196-6
309. Zhao RC, Zhou J, Chen KF, Gong J, Liu J, He JY, et al. The prognostic value of combination of CD90 and OCT4 for hepatocellular carcinoma after curative resection. Neoplasma. (2016) 63:288–98. doi: 10.4149/neo_2016_036
310. Suetsugu A, Nagaki M, Aoki H, Motohashi T, Kunisada T, Moriwaki H. Characterization of CD133+ hepatocellular carcinoma cells as cancer stem/progenitor cells. Biochem Biophys Res Commun. (2006) 351:820–4. doi: 10.1016/j.bbrc.2006.10.128
311. Li M-M, Tang Y-Q, Gong Y-F, Cheng W, Li H-L, Kong F-E, et al. Development of an oncogenic dedifferentiation SOX signature with prognostic significance in hepatocellular carcinoma. BMC Cancer. (2019) 19:851. doi: 10.1186/s12885-019-6041-2
312. Liu K, Hao M, Ouyang Y, Zheng J, Chen D. CD133+ cancer stem cells promoted by VEGF accelerate the recurrence of hepatocellular carcinoma. Sci. Rep. (2017) 7:41499. doi: 10.1038/srep41499
313. Cheung PF, Cheung TT, Yip CW, Ng LW, Fung SW, Lo CM, et al. Hepatic cancer stem cell marker granulin-epithelin precursor and β-catenin expression associate with recurrence in hepatocellular carcinoma. Oncotarget. (2016) 7:21644–57. doi: 10.18632/oncotarget.7803
314. Jun SY, Jeon S-J, Yoon J-Y, Lee J-J, Yoon HR, Choi M-H, et al. The positive correlation of TIPRL with lC3 and CD133 contributes to cancer aggressiveness: potential biomarkers for early liver cancer. Sci. Rep. (2019) 9:16802. doi: 10.1038/s41598-019-53191-5
315. Vilchez V, Turcios L, Zaytseva Y, Stewart R, Lee EY, Maynard E, et al. Cancer stem cell marker expression alone and in combination with microvascular invasion predicts poor prognosis in patients undergoing transplantation for hepatocellular carcinoma. Am J Surg. (2016) 212:238–45. doi: 10.1016/j.amjsurg.2015.12.019
316. Chen D, Li Z, Cheng Q, Wang Y, Qian L, Gao J, et al. Genetic alterations and expression of PTEN and its relationship with cancer stem cell markers to investigate pathogenesis and to evaluate prognosis in hepatocellular carcinoma. J Clin Pathol. (2019) 72:588–96. doi: 10.1136/jCLinpath-2019-205769
317. Ding Q, Xia Y, Ding S, Lu P, Sun L, Liu M. An alternatively spliced variant of CXCR3 mediates the metastasis of CD133+ liver cancer cells induced by CXCL9. Oncotarget. (2016) 7:14405–14. doi: 10.18632/oncotarget.7360
318. Su R, Nan H, Guo H, Ruan Z, Jiang L, Song Y, et al. Associations of components of PTEN/AKT/mTOR pathway with cancer stem cell markers and prognostic value of these biomarkers in hepatocellular carcinoma. Hepatol Res. (2016) 46:1380–91. doi: 10.1111/hepr.12687
319. Yu G-F, Lin X, Luo R-C, Fang W-Y. Nuclear CD133 expression predicts poor prognosis for hepatocellular carcinoma. Int J Clin Exp Pathol. (2018) 11:2092–9.
320. Chen Y-L, Lin P-Y, Ming Y-Z, Huang W-C, Chen R-F, Chen P-M, et al. The effects of the location of cancer stem cell marker CD133 on the prognosis of hepatocellular carcinoma patients. BMC Cancer. (2017) 17:474. doi: 10.1186/s12885-017-3460-9
321. Seino S, Tsuchiya A, Watanabe Y, Kawata Y, Kojima Y, Ikarashi S, et al. Clinical outcome of hepatocellular carcinoma can be predicted by the expression of hepatic progenitor cell markers and serum tumour markers. Oncotarget. (2018) 9:21844–60. doi: 10.18632/oncotarget.25074
322. Felden J von, Schulze K, Krech T, Ewald F, Nashan B, Pantel K, et al. Circulating tumor cells as liquid biomarker for high HCC recurrence risk after curative liver resection. Oncotarget. (2017) 8:89978–87. doi: 10.18632/oncotarget.21208
323. Dai X-M, Huang T, Yang S-L, Zheng X-M, Chen GG, Zhang T. Peritumoral EpCAM is an independent prognostic marker after curative resection of HBV-Related hepatocellular carcinoma. Dis Markers. (2017) 2017:8495326. doi: 10.1155/2017/3765279
324. Ko C-J, Li C-J, Wu M-Y, Chu P-Y. Overexpression of epithelial cell adhesion molecule as a predictor of poor outcome in patients with hepatocellular carcinoma. Exp Ther Med. (2018) 16:4810–6. doi: 10.3892/etm.2018.6794
325. Shen J, Wang W-S, Zhu X-L, Ni C-F. High epithelial cell adhesion molecule-Positive circulating tumor cell count predicts poor survival of patients with unresectable hepatocellular carcinoma treated with transcatheter arterial chemoembolization. J Vasc Interv Radiol. (2018) 29:1678–84. doi: 10.1016/j.jvir.2018.07.030
326. Noh C-K, Wang HJ, Kim CM, Kim J, Yoon SY, Lee GH, et al. EpCAM as a predictive marker of tumor recurrence and survival in patients who underwent surgical resection for hepatocellular carcinoma. Anticancer Res. (2018) 38:4101–9. doi: 10.21873/anticanres.12700
327. Zhou L, Zhu Y. The EpCAM overexpression is associated with Clinicopathological significance and prognosis in hepatocellular carcinoma patients: a systematic review and meta-analysis. Int J Surg. (2018) 56:274–80. doi: 10.1016/j.ijsu.2018.06.025
328. Zhu M, Li W, Lu Y, Dong X, Chen Y, Lin B, et al. Alpha fetoprotein antagonizes apoptosis induced by paclitaxel in hepatoma cells in vitro. Sci. Rep. (2016) 6:26472. doi: 10.1038/srep26472
329. Jin J, Niu X, Zou L, Li L, Li S, Han J, et al. AFP mRNA level in enriched circulating tumor cells from hepatocellular carcinoma patient blood samples is a pivotal predictive marker for metastasis. Cancer Lett. (2016) 378:33–7. doi: 10.1016/j.canlet.2016.04.033
330. Lorente L. New prognostic biomarkers of mortality in patients undergoing liver transplantation for hepatocellular carcinoma. World J Gastroenterol. (2018) 24:4230–42. doi: 10.3748/wjg.v24.i37.4230
331. Chang T-S, Wu Y-C, Chi C-C, Su W-C, Chang P-J, Lee K-F, et al. Activation of IL6/IGFIR confers poor prognosis of HBV-related hepatocellular carcinoma through induction of OCT4/NANOG expression. Clin Cancer Res. (2015) 21:201–10. doi: 10.1158/1078-0432.CCR-13-3274
332. Was H Czarnecka J Kominek A Barszcz K BeRNAs T Piwocka K . Some chemotherapeutics-treated colon cancer cells display a specific phenotype being a combination of stem-like and senescent cell features. Cancer Biol Ther. (2018) 19:63–75. doi: 10.1080/15384047.2017.1385675
333. Manhas J, Bhattacharya A, Agrawal SK, Gupta B, Das P, Deo SV, et al. Characterization of cancer stem cells from different grades of human colorectal cancer. Tumour Biol. (2016) 37:14069–81. doi: 10.1007/s13277-016-5232-6
334. Zhou J-Y, Chen M, Ma L, Wang X, Chen Y-G, Liu S-L. Role of CD44(high)/CD133(high) HCT-116 cells in the tumorigenesis of colon cancer. Oncotarget. (2016) 7:7657–66. doi: 10.18632/oncotarget.7084
335. Leng Z, Xia Q, Chen J, Li Y, Xu J, Zhao E, et al. Lgr5+CD44+EpCAM+ strictly defines cancer stem cells in human colorectal cancer. Cell Physiol Biochem. (2018) 46:860–72. doi: 10.1159/000488743
336. Zhou Y, Xia L, Wang H, Oyang L, Su M, Liu Q, et al. Cancer stem cells in progression of colorectal cancer. Oncotarget. (2018) 9:33403–15. doi: 10.18632/oncotarget.23607
337. Lee SY, Kim KA, Kim CH, Kim YJ, Lee J-H, Kim HR. CD44-shRNA recombinant adenovirus inhibits cell proliferation, invasion, and migration, and promotes apoptosis in HCT116 colon cancer cells. Int J Oncol. (2017) 50:329–36. doi: 10.3892/ijo.2016.3801
338. Tsunekuni K, Konno M, Haraguchi N, Koseki J, Asai A, Matsuoka K, et al. CD44/CD133-positive colorectal cancer stem cells are sensitive to trifluridine exposure. Sci Rep. (2019) 9:14861. doi: 10.1038/s41598-019-50968-6
339. Ozawa M, Ichikawa Y, Zheng Y-W, Oshima T, Miyata H, Nakazawa K, et al. Prognostic significance of CD44 variant 2 upregulation in colorectal cancer. Br J Cancer. (2014) 111:365–74. doi: 10.1038/bjc.2014.253
340. Lim SH, Jang J, Park JO, Kim K-M, Kim ST, Park YS, et al. CD133-positive tumor cell content is a predictor of early recurrence in colorectal cancer. J Gastrointest Oncol. (2014) 5:447–56. doi: 10.3978/j.issn.2078-6891.2014.071
341. Ning S-T, Lee S-Y, Wei M-F, Peng C-L, Lin SY-F, Tsai M-H, et al. Targeting colorectal cancer stem-like cells with anti-CD133 antibody-Conjugated SN-38 nanopartiCLes. ACS Appl Mater Interfaces. (2016) 8:17793–804. doi: 10.1021/acsami.6b04403
342. Zhao L, Yang Y, Zhou P, Ma H, Zhao X, He X, et al. Targeting CD133high colorectal cancer cells in vitro and in vivo with an asymmetric bispecific antibody. J Immunother. (2015) 38:217–28. doi: 10.1097/CJI.0000000000000086
343. Schmohl JU, Gleason MK, Dougherty PR, Miller JS, Vallera DA. Heterodimeric bispecific single chain variable fragments (ScFv) killer engagers (BiKEs) enhance NK-cell activity against CD133+ colorectal cancer cells. Target Oncol. (2016) 11:353–61. doi: 10.1007/s11523-015-0391-8
344. Zhao Y, Peng J, Zhang E, Jiang N, Li J, Zhang Q, et al. CD133 expression may be useful as a prognostic indicator in colorectal cancer, a tool for opTIMizing therapy and supportive evidence for the cancer stem cell hypothesis: a meta-analysis. Oncotarget. (2016) 7:10023–36. doi: 10.18632/oncotarget.7054
345. AlShamaileh H, Wang T, Xiang D, Yin W, Tran PH-L, Barrero RA, et al. Aptamer-mediated survivin RNAi enables 5-fluorouracil to eliminate colorectal cancer stem cells. Sci Rep. (2017) 7:5898. doi: 10.1038/s41598-017-05859-z
346. Xiang D, Shigdar S, Bean AG, Bruce M, Yang W, Mathesh M, et al. Transforming doxorubicin into a cancer stem cell killer via EpCAM aptamer-mediated delivery. Theranostics. (2017) 7:4071–86. doi: 10.7150/thno.20168
347. Boesch M, Spizzo G, Seeber A. Concise review: aggressive colorectal cancer: role of epithelial cell adhesion molecule in cancer stem cells and epithelial-to-Mesenchymal transition. Stem Cells Transl Med. (2018) 7:495–501. doi: 10.1002/sctm.17-0289
348. Sousa e Melo F de, Kurtova AV, Harnoss JM, Kljavin N, Hoeck JD, Hung J, et al. A distinct role for Lgr5+ stem cells in primary and metastatic colon cancer. Nature. (2017) 543:676–80. doi: 10.1038/nature21713
349. Shimokawa M, Ohta Y, Nishikori S, Matano M, Takano A, Fujii M, et al. Visualization and targeting of Lgr5+ human colon cancer stem cells. Nature. (2017) 545:187–92. doi: 10.1038/nature22081
350. Cortina C, Turon G, Stork D, HeRNAndo-Momblona X, Sevillano M, Aguilera M, et al. A genome editing approach to study cancer stem cells in human tumors. Embo Mol Med. (2017) 9:869–79. doi: 10.15252/emmm.201707550
351. Baker A-M, Graham TA, Elia G, Wright NA, Rodriguez-Justo M. Characterization of Lgr5 stem cells in colorectal adenomas and carcinomas. Sci Rep. (2015) 5:8654. doi: 10.1038/srep08654
352. Junttila MR, Mao W, Wang X, Wang B-E, Pham T, Flygare J, et al. Targeting Lgr5+ cells with an antibody-drug conjugate for the treatment of colon cancer. Sci Transl Med. (2015) 7:314ra186. doi: 10.1126/scitranslmed.aac7433
353. He S, Zhou H, Zhu X, Hu S, Fei M, Wan D, et al. Expression of Lgr5, a marker of intestinal stem cells, in colorectal cancer and its CLinicopathological significance. Biomed Pharmacother. (2014) 68:507–13. doi: 10.1016/j.biopha.2014.03.016
354. Jiang Y, Li W, He X, Zhang H, Jiang F, Chen Z. Lgr5 expression is a valuable prognostic factor for colorectal cancer: evidence from a meta-analysis. BMC Cancer. (2015) 15:948. doi: 10.1186/s12885-015-1985-3
355. Vishnubalaji R, Manikandan M, Fahad M, Hamam R, Alfayez M, Kassem M, et al. Molecular profiling of ALDH1+ colorectal cancer stem cells reveals preferential activation of mAPK, FAK, and oxidative stress pro-survival signalling pathways. Oncotarget. (2018) 9:13551–64. doi: 10.18632/oncotarget.24420
356. Kozovska Z, Patsalias A, Bajzik V, Durinikova E, Demkova L, Jargasova S, et al. ALDH1A inhibition sensitizes colon cancer cells to chemotherapy. BMC Cancer. (2018) 18:656. doi: 10.1186/s12885-018-4572-6
357. Kahlert C, Gaitzsch E, Steinert G, Mogler C, Herpel E, Hoffmeister M, et al. Expression analysis of aldehyde dehydrogenase 1A1 (ALDH1A1) in colon and rectal cancer in association with prognosis and response to chemotherapy. Ann Surg Oncol. (2012) 19:4193–201. doi: 10.1245/s10434-012-2518-9
358. Piao L, Feng Y, Yang Z, Qi W, Li H, Han H, et al. LETM1 is a potential cancer stem-like cell marker and predicts poor prognosis in colorectal adenocarcinoma. Pathol Res Pract. (2019) 215:152437. doi: 10.1016/j.prp.2019.152437
359. Yang L, Ding C, Tang W, Yang T, Liu M, Wu H, et al. INPP4B exerts a dual function in the stemness of colorectal cancer stem-like cells through regulating sox2 and nanog expression. Carcinogenesis. (2019) 41:78–90. doi: 10.1093/carcin/bgz110
360. Yao C, Su L, Shan J, Zhu C, Liu L, Liu C, et al. IGF/STAT3/NANOG/Slug signaling axis simultaneously controls epithelial-Mesenchymal transition and stemness maintenance in colorectal cancer. Stem Cells. (2016) 34:820–31. doi: 10.1002/stem.2320
361. Wang H, Liu B, Wang J, Li J, Gong Y, Li S, et al. Reduction of NANOG mediates the inhibitory effect of aspirin on tumor growth and stemness in colorectal cancer. Cell Physiol Biochem. (2017) 44:1051–63. doi: 10.1159/000485405
362. Xu F, Dai C, Zhang R, Zhao Y, Peng S, Jia C. Nanog: a potential biomarker for liver metastasis of colorectal cancer. Dig Dis Sci. (2012) 57:2340–6. doi: 10.1007/s10620-012-2182-8
363. Fujino S, Miyoshi N. Oct4 gene expression in primary colorectal cancer promotes liver metastasis. Stem Cells Int. (2019) 2019:7896524. doi: 10.1155/2019/7896524
364. Lee JH, Yun CW, Han Y-S, Kim S, Jeong D, Kwon HY, et al. Melatonin and 5-fluorouracil co-suppress colon cancer stem cells by regulating cellular prion protein-Oct4 axis. J Pineal Res. (2018) 65:e12519. doi: 10.1111/jpi.12519
365. Miyoshi N, Fujino S, Ohue M, Yasui M, Takahashi Y, Sugimura K, et al. The POU5F1 gene expression in colorectal cancer: a novel prognostic marker. Surg Today. (2018) 48:709–15. doi: 10.1007/s00595-018-1644-9
366. Ardalan Khales S, Abbaszadegan MR, Abdollahi A, Raeisossadati R, Tousi MF, Forghanifard MM. SALL4 as a new biomarker for early colorectal cancers. J Cancer Res Clin Oncol. (2015) 141:229–35. doi: 10.1007/s00432-014-1808-y
367. Lundberg IV, Edin S, Eklöf V, Öberg Å, Palmqvist R, Wikberg ML. SOX2 expression is associated with a cancer stem cell state and down-regulation of CDX2 in colorectal cancer. BMC Cancer. (2016) 16:471. doi: 10.1186/s12885-016-2509-5
368. Takeda K, Mizushima T, Yokoyama Y, Hirose H, Wu X, Qian Y, et al. Sox2 is associated with cancer stem-like properties in colorectal cancer. Sci Rep. (2018) 8:17639. doi: 10.1038/s41598-018-36251-0
369. Miller TJ, McCoy MJ, Hemmings C, Bulsara MK, Iacopetta B, Platell CF. The prognostic value of cancer stem-like cell markers SOX2 and CD133 in stage iII colon cancer is modified by expression of the immune-related markers FoxP3, PD-L1 and CD3. Pathology. (2017) 49:721–30. doi: 10.1016/j.pathol.2017.08.007
370. Haubner S, PeRNA F, Köhnke T, Schmidt C, Berman S, Augsberger C, et al. Coexpression profile of leukemic stem cell markers for combinatorial targeted therapy in AML. Leukemia. (2019) 33:64–74. doi: 10.1038/s41375-018-0180-3
371. Zhang CC, Yan Z, Pascual B, Jackson-Fisher A, Huang DS, Zong Q, et al. Gemtuzumab ozogamicin (GO) inclusion to induction chemotherapy eliminates leukemic initiating cells and significantly improves survival in mouse models of acute myeloid leukemia. Neoplasia. (2018) 20:1–11. doi: 10.1016/j.neo.2017.10.008
372. Tang X, Yang L, Li Z, Nalin AP, Dai H, Xu T, et al. First-in-man clinical trial of CAR NK-92 cells: safety test of CD33-CAR NK-92 cells in patients with relapsed and refractory acute myeloid leukemia. Am J Cancer Res. (2018) 8:1083–9.
373. Braciak TA, Roskopf CC, Wildenhain S, Fenn NC, Schiller CB, Schubert IA, et al. Dual-targeting triplebody 33-16-123 (SPM-2) mediates effective redirected lysis of primary blasts from patients with a broad range of AML subtypes in combination with natural killer cells. Oncoimmunology. (2018) 7:e1472195. doi: 10.1080/2162402X.2018.1472195
374. Petrov JC, Wada M, Pinz KG, Yan LE, Chen KH, Shuai X, et al. Compound CAR T-cells as a double-pronged approach for treating acute myeloid leukemia. Leukemia. (2018) 32:1317–26. doi: 10.1038/s41375-018-0075-3
375. Cartellieri M, Feldmann A, Koristka S, Arndt C, Loff S, Ehninger A, et al. Switching CAR T cells on and off: a novel modular platform for retargeting of t cells to AML blasts. Blood Cancer J. (2016) 6:e458. doi: 10.1038/bcj.2016.61
376. Pizzitola I, Anjos-Afonso F, Rouault-Pierre K, Lassailly F, Tettamanti S, Spinelli O, et al. Chimeric antigen receptors against CD33/CD123 antigens efficiently target primary acute myeloid leukemia cells in vivo. Leukemia. (2014) 28:1596–605. doi: 10.1038/leu.2014.62
377. Vallera DA, Felices M, McElmurry R, McCullar V, Zhou X, Schmohl JU, et al. IL15 trispecific killer engagers (Trike) make natural killer cells specific to CD33+ targets while also inducing persistence, in vivo expansion, and enhanced function. Clin Cancer Res. (2016) 22:3440–50. doi: 10.1158/1078-0432.CCR-15-2710
378. Hoseini SS, Guo H, Wu Z, Hatano MN, Cheung N-KV. A potent tetravalent t-cell-engaging bispecific antibody against CD33 in acute myeloid leukemia. Blood Adv. (2018) 2:1250–8. doi: 10.1182/bloodadvances.2017014373
379. Kovtun Y, Noordhuis P, Whiteman KR, Watkins K, Jones GE, Harvey L, et al. IMGN779, a novel CD33-Targeting antibody–drug conjugate with DNA-Alkylating activity, exhibits potent antitumor activity in models of AML. Mol Cancer Ther. (2018) 17:1271–9. doi: 10.1158/1535-7163.MCT-17-1077
380. Vasu S, He S, Cheney C, Gopalakrishnan B, Mani R, Lozanski G, et al. Decitabine enhances anti-CD33 monoclonal antibody BI 836858-mediated natural killer ADCC against AML blasts. Blood. (2016) 127:2879–89. doi: 10.1182/blood-2015-11-680546
381. Kloess S, Ede Valverde da Silva A, Oberschmidt O, Gardlowski T, Matthies N, Vyas M, et al. Triplebody mediates increased anti-Leukemic reactivity of IL-2 activated donor natural killer (NK) cells and impairs viability of their CD33-expressing NK subset. Front Immunol. (2017) 8:1100. doi: 10.3389/fimmu.2017.01100
382. Schneider D, Xiong Y, Hu P, Wu D, Chen W, Ying T, et al. A unique human immunoglobulin heavy chain variable domain-only CD33 CAR for the treatment of acute myeloid leukemia. Front. Oncol. (2018) 8:539. doi: 10.3389/fonc.2018.00539
383. Kenderian SS, Ruella M, Shestova O, Klichinsky M, Aikawa V, Morrissette JJ, et al. CD33-specific chimeric antigen receptor T cells exhibit potent preClinical activity against human acute myeloid leukemia. Leukemia. (2015) 29:1637–47. doi: 10.1038/leu.2015.52
384. Hills RK, Castaigne S, Appelbaum FR, Delaunay J, Petersdorf S, Othus M, et al. Addition of gemtuzumab ozogamicin to induction chemotherapy in adult patients with acute myeloid leukaemia: a meta-analysis of individual patient data from randomised controlled trials. Lancet Oncol. (2014) 15:986–96. doi: 10.1016/S1470-2045(14)70281-5
385. Gamis AS, Alonzo TA, Meshinchi S, Sung L, Gerbing RB, Raimondi SC, et al. Gemtuzumab ozogamicin in children and adolescents with de novo acute myeloid leukemia improves event-free survival by reducing relapse risk: results from the randomized phase iII children's oncology group trial AAML0531. JCO. (2014) 32:3021–32. doi: 10.1200/JCO.2014.55.3628
386. Stein EM, Walter RB, Erba HP, Fathi AT, Advani AS, Lancet JE, et al. A phase 1 trial of vadastuximab talirine as monotherapy in patients with CD33-positive acute myeloid leukemia. Blood. (2018) 131:387–96. doi: 10.1182/blood-2017-06-789800
387. Wang Q-S, Wang Y, Lv H-Y, Han Q-W, Fan H, Guo B, et al. Treatment of CD33-directed chimeric antigen receptor-modified T cells in one patient with relapsed and refractory acute myeloid leukemia. Mol Ther. (2015) 23:184–91. doi: 10.1038/mt.2014.164
388. Zahler S, Bhatia M, Ricci A, Roy S, Morris E, Harrison L, et al. A phase I Study of reduced-Intensity conditioning and allogeneic stem cell transplantation followed by dose escalation of targeted consolidation immunotherapy with gemtuzumab ozogamicin in children and adolescents with CD33+ acute myeloid leukemia. Biol Blood Marrow Transpl. (2016) 22:698–704. doi: 10.1016/j.bbmt.2016.01.019
389. Niktoreh N, Lerius B, Zimmermann M, Gruhn B, Escherich G, Bourquin J-P, et al. Gemtuzumab ozogamicin in children with relapsed or refractory acute myeloid leukemia: a report by Berlin-Frankfurt-Münster study group. Haematologica. (2019) 104:120–7. doi: 10.3324/haematol.2018.191841
390. Tarlock K, Alonzo TA, Gerbing RB, Raimondi SC, Hirsch BA, Sung L, et al. Gemtuzumab ozogamicin reduces relapse risk in FLT3/ITD acute myeloid leukemia: a report from the children's oncology group. Clin Cancer Res. (2016) 22:1951–7. doi: 10.1158/1078-0432.CCR-15-1349
391. Amadori S, Suciu S, Selleslag D, Aversa F, Gaidano G, Musso M, et al. Gemtuzumab ozogamicin versus best supportive care in older patients with newly diagnosed acute myeloid leukemia unsuitable for intensive chemotherapy: results of the randomized phase III EORTC-GIMEMA AML-19 trial. J Clin Oncol. (2016) 34:972–9. doi: 10.1200/JCO.2015.64.0060
392. Portwood S, Puchalski RA, Walker RM, Wang ES. Combining IMGN779, a novel anti-CD33 antibody-drug conjugate (ADC), with the pARP inhibitor, olaparib, results in enhanced anti-Tumor activity in preclinical acute myeloid leukemia (AML) models. Blood. (2016) 128:1645. doi: 10.1182/blood.V128.22.1645.1645
393. Pollard JA, Loken M, Gerbing RB, Raimondi SC, Hirsch BA, Aplenc R, et al. CD33 expression and its association with gemtuzumab ozogamicin response: results from the randomized phase III children's oncology group trial AAML0531. J Clin Oncol. (2016) 34:747–55. doi: 10.1200/JCO.2015.62.6846
394. Yabushita T, Satake H, Maruoka H, Morita M, Katoh D, Shimomura Y, et al. Expression of multiple leukemic stem cell markers is associated with poor prognosis in de novo acute myeloid leukemia. Leuk Lymphoma. (2018) 59:2144–51. doi: 10.1080/10428194.2017.1410888
395. Al-Mawali A, Gillis D, Lewis I. Immunoprofiling of leukemic stem cells CD34+/CD38-/CD123+ delineate FLT3/ITD-positive CLones. J Hematol Oncol. (2016) 9:61. doi: 10.1186/s13045-016-0292-z
396. Jordan CT, Upchurch D, Szilvassy SJ, Guzman ML, Howard DS, Pettigrew AL, et al. The interleukin-3 receptor alpha chain is a unique marker for human acute myelogenous leukemia stem cells. Leukemia. (2000) 14:1777–84. doi: 10.1038/sj.leu.2401903
397. Xie LH, Biondo M, Busfield SJ, Arruda A, Yang X, Vairo G, et al. CD123 target validation and preClinical evaluation of ADCC activity of anti-CD123 antibody CSL362 in combination with NKs from AML patients in remission. Blood Cancer J. (2017) 7:e567. doi: 10.1038/bcj.2017.52
398. Bras AE, Haas V de, van Stigt A, Jongen-Lavrencic M, Beverloo HB, Te Marvelde JG, et al. CD123 expression levels in 846 acute leukemia patients based on standardized immunophenotyping. Cytometry B Clin Cytom. (2019) 96:134–42. doi: 10.1002/cyto.b.21745
399. Arai N, Homma M, Abe M, Baba Y, Murai S, Watanuki M, et al. Impact of CD123 expression, analyzed by immunohistochemistry, on Clinical outcomes in patients with acute myeloid leukemia. Int J Hematol. (2019) 109:539–44. doi: 10.1007/s12185-019-02616-y
400. Bonifant CL, Szoor A, Torres D, Joseph N, Velasquez MP, Iwahori K, et al. CD123-engager T cells as a novel immunotherapeutic for acute myeloid leukemia. Mol Ther. (2016) 24:1615–26. doi: 10.1038/mt.2016.116
401. Li F, Sutherland MK, Yu C, Walter RB, Westendorf L, Valliere-Douglass J, et al. Characterization of SGN-CD123A, a potent CD123-directed antibody-drug conjugate for acute myeloid leukemia. Mol Cancer Ther. (2018) 17:554–64. doi: 10.1158/1535-7163.MCT-17-0742
402. Kovtun Y, Jones GE, Adams S, Harvey L, Audette CA, Wilhelm A, et al. A CD123-targeting antibody-drug conjugate, IMGN632, designed to eradicate AML while sparing normal bone marrow cells. Blood Adv. (2018) 2:848–58. doi: 10.1182/bloodadvances.2018017517
403. Han L, Jorgensen JL, Brooks C, Shi C, Zhang Q, Nogueras González GM, et al. Antileukemia efficacy and mechanisms of action of SL-101, a novel anti-CD123 antibody conjugate, in acute myeloid leukemia. Clin Cancer Res. (2017) 23:3385–95. doi: 10.1158/1078-0432.CCR-16-1904
404. Fan D, Li Z, Zhang X, Yang Y, Yuan X, Zhang X, et al. AntiCD3Fv fused to human interleukin-3 deletion variant redirected T cells against human acute myeloid leukemic stem cells. J Hematol Oncol. (2015) 8:18. doi: 10.1186/s13045-015-0109-5
405. Busfield SJ, Biondo M, Wong M, Ramshaw HS, Lee EM, Ghosh S, et al. Targeting of acute myeloid leukemia in vitro and in vivo with an anti-CD123 mAb engineered for optimal ADCC. Leukemia. (2014) 28:2213–21. doi: 10.1038/leu.2014.128
406. Williams BA, Wang X-H, Leyton JV, Maghera S, Deif B, Reilly RM, et al. CD16+NK-92 and anti-CD123 monoclonal antibody prolongs survival in primary human acute myeloid leukemia xenografted mice. Haematologica. (2018) 103:1720–9. doi: 10.3324/haematol.2017.187385
407. Hutmacher C, Volta L, Rinaldi F, Murer P, Myburgh R, Manz MG, et al. Development of a novel fully-human anti-CD123 antibody to target acute myeloid leukemia. Leuk Res. (2019) 84:106178. doi: 10.1016/j.leukres.2019.106178
408. Thokala R, Olivares S, Mi T, Maiti S, Deniger D, Huls H, et al. Redirecting specificity of t cells using the sleeping beauty system to express chimeric antigen receptors by mix-and-matching of VL and VH domains targeting CD123+ tumors. PLoS ONE. (2016) 11:e0159477. doi: 10.1371/jouRNAl.pone.0159477
409. Oberschmidt O, Morgan M, Huppert V, Kessler J, Gardlowski T, Matthies N, et al. Development of automated separation, expansion, and quality control protocols for clinical-scale manufacturing of primary human NK cells and alpharetroviral chimeric antigen receptor engineering. Hum Gene Ther Methods. (2019) 30:102–20. doi: 10.1089/hgtb.2019.039
410. Tasian SK, Kenderian SS, Shen F, Ruella M, Shestova O, Kozlowski M, et al. Optimized depletion of chimeric antigen receptor T cells in murine xenograft models of human acute myeloid leukemia. Blood. (2017) 129:2395–407. doi: 10.1182/blood-2016-08-736041
411. He SZ, Busfield S, Ritchie DS, Hertzberg MS, Durrant S, Lewis ID, et al. A phase 1 study of the safety, pharmacokinetics and anti-leukemic activity of the anti-CD123 monoclonal antibody CSL360 in relapsed, refractory or high-risk acute myeloid leukemia. Leuk Lymphoma. (2015) 56:1406–15. doi: 10.3109/10428194.2014.956316
412. Al-Hussaini M, Rettig MP, Ritchey JK, Karpova D, Uy GL, Eissenberg LG, et al. Targeting CD123 in acute myeloid leukemia using a T-cell-directed dual-affinity retargeting platform. Blood. (2016) 127:122–31. doi: 10.1182/blood-2014-05-575704
413. Zahran AM, Aly SS, Rayan A, El-Badawy O, Fattah MA, Ali AM, et al. Survival outcomes of CD34+CD38-LSCs and their expression of CD123 in adult AML patients. Oncotarget. (2018) 9:34056–65. doi: 10.18632/oncotarget.26118
414. Jiang Y-P, Liu BY, Zheng Q, Panuganti S, Chen R, Zhu J, et al. CLT030, a leukemic stem cell-targeting CLL1 antibody-drug conjugate for treatment of acute myeloid leukemia. Blood Adv. (2018) 2:1738–49. doi: 10.1182/bloodadvances.2018020107
415. van Rhenen A, van Dongen GA, Kelder A, Rombouts EJ, Feller N, Moshaver B, et al. The novel AML stem cell associated antigen CLL-1 aids in discrimination between normal and leukemic stem cells. Blood. (2007) 110:2659–66. doi: 10.1182/blood-2007-03-083048
416. Bill M, Aggerholm A, Kjeldsen E, Roug AS, Hokland P, Nederby L. Revisiting CLEC12A as leukaemic stem cell marker in AML: highlighting the necessity of precision diagnostics in patients eligible for targeted therapy. Br J Haematol. (2019) 184:769–81. doi: 10.1111/bjh.15711
417. Leong SR, Sukumaran S, Hristopoulos M, Totpal K, Stainton S, Lu E, et al. An anti-CD3/anti–CLL-1 bispecific antibody for the treatment of acute myeloid leukemia. Blood. (2017) 129:609–18. doi: 10.1182/blood-2016-08-735365
418. Lin TY, Zhu Y, Li Y, Zhang H, Ma AH, Long Q, et al. Daunorubicin-containing CLL1-targeting nanomicelles have anti-leukemia stem cell activity in acute myeloid leukemia. Nanomedicine. (2019) 20:102004. doi: 10.1016/j.nano.2019.04.007
419. Kenderian SS, Ruella M, Shestova O, Klichinsky M, Kim M, Soderquist C, et al. Targeting CLEC12A with chimeric antigen receptor T Cells can overcome the chemotherapy refractoriness of leukemia stem cells. Biol. Blood Marrow Transpl. (2017) 23:S247–8. doi: 10.1016/j.bbmt.2016.12.413
420. Darwish NHE, Sudha T, Godugu K, Elbaz O, Abdelghaffar HA, Hassan EEA, et al. Acute myeloid leukemia stem cell markers in prognosis and targeted therapy: potential impact of BMI-1, TIM-3 and CLL-1. Oncotarget. (2016) 7:57811–20. doi: 10.18632/oncotarget.11063
421. Kikushige Y, Shima T, Takayanagi S-i, Urata S, Miyamoto T, Iwasaki H, et al. TIM-3 is a promising target to selectively kill acute myeloid leukemia stem cells. Cell Stem Cell. (2010) 7:708–17. doi: 10.1016/j.stem.2010.11.014
422. Homayouni V, Ganjalikhani-hakemi M, Rezaei A, Khanahmad H, Behdani M, Lomedasht FK. Preparation and characterization of a novel nanobody against T-cell immunoglobulin and mucin-3 (TIM-3). Iran J Basic Med Sci. (2016) 19:1201–8. doi: 10.15171/ijb.1427
423. Dama P, Tang M, Fulton N, Kline J, Liu H. Gal9/TIM-3 expression level is higher in AML patients who fail chemotherapy. J. ImmunoTher Cancer. (2019) 7:175. doi: 10.1186/s40425-019-0611-3
424. van Hoang T, Buss EC, Wang W, Hoffmann I, Raffel S, Zepeda-Moreno A, et al. The rarity of ALDH(+) cells is the key to separation of normal versus leukemia stem cells by ALDH activity in AML patients. Int J Cancer. (2015) 137:525–36. doi: 10.1002/ijc.29410
425. Blume R, Rempel E, Manta L, Saeed BR, Wang W, Raffel S, et al. The molecular signature of AML with increased ALDH activity suggests a stem cell origin. Leuk Lymphoma. (2018) 59:2201–10. doi: 10.1080/10428194.2017.1422862
426. Voeltzel T, Flores-Violante M, Zylbersztejn F, Lefort S, Billandon M, Jeanpierre S, et al. A new signaling cascade linking BMP4, BMPR1A, ΔNp73 and NANOG impacts on stem-like human cell properties and patient outcome. Cell Death Dis. (2018) 9:1011. doi: 10.1038/s41419-018-1042-7
427. Kakiuchi S, Minami Y, Miyata Y, Mizutani Y, Goto H, Kawamoto S, et al. NANOG expression as a responsive biomarker during treatment with hedgehog signal inhibitor in acute myeloid leukemia. Int J Mol Sci. (2017) 18:486. doi: 10.3390/ijms18030486
428. Picot T, Kesr S, Wu Y, Aanei CM, Flandrin-Gresta P, Tondeur S, et al. Potential role of OCT4 in leukemogenesis. Stem Cells Dev. (2017) 26:1637–47. doi: 10.1089/scd.2017.0134
429. Yin J-Y, Tang Q, Zhai L-L, Zhou L-Y, Qian J, Lin J, et al. High expression of OCT4 is frequent and may cause undesirable treatment outcomes in patients with acute myeloid leukemia. Tumour Biol. (2015) 36:9711–6. doi: 10.1007/s13277-015-3731-5
430. Xiang Y, Zhou X. Octamer-binding transcription factor 4 correlates with complex karyotype, fLT3-ITD mutation and poorer risk stratification, and predicts unfavourable prognosis in patients with acute myeloid leukaemia. Hematology. (2018) 23:721–8. doi: 10.1080/10245332.2018.1482050
431. Zhang L-Y, Yuan Y-Q, Zhou D-M, Wang Z-Y, Ju S-G, Sun Y, et al. Impact of global and gene-specific DNA methylation in de novo or relapsed acute myeloid leukemia patients treated with decitabine. Asian Pac J Cancer Prev. (2016) 17:431–7. doi: 10.7314/APJCP.2016.17.1.431
432. Tosic N, Petrovic I, Grujicic NK, Davidovic S, Virijevic M, Vukovic NS, et al. Prognostic significance of SOX2, SOX3, SOX11, SOX14 and SOX18 gene expression in adult de novo acute myeloid leukemia. Leuk Res. (2018) 67:32–8. doi: 10.1016/j.leukres.2018.02.001
433. Sadovnik I, Hoelbl-Kovacic A, Herrmann H, Eisenwort G, Cerny-Reiterer S, Warsch W, et al. Identification of CD25 as STAT5-Dependent growth-Regulator of leukemic stem cells in PH+ CML. Clin Cancer Res. (2015) 22:2051–61. doi: 10.1158/1078-0432.CCR-15-0767
434. Herrmann H, Sadovnik I, Cerny-Reiterer S, Rülicke T, Stefanzl G, Willmann M, et al. Dipeptidylpeptidase IV (CD26) defines leukemic stem cells (LSC) in chronic myeloid leukemia. Blood. (2014) 123:3951–62. doi: 10.1182/blood-2013-10-536078
435. Landberg N, Palffy S von, Askmyr M, Lilljebjörn H, Sandén C, Rissler M, et al. CD36 defines primitive chronic myeloid leukemia cells less responsive to imatinib but vulnerable to antibody-based therapeutic targeting. Haematologica. (2018) 103:447–55. doi: 10.3324/haematol.2017.169946
436. Warfvinge R, Geironson L, Sommarin MN, Lang S, Karlsson C, Roschupkina T, et al. Single-cell molecular analysis defines therapy response and immunophenotype of stem cell subpopulations in CML. Blood. (2017) 129:2384–94. doi: 10.1182/blood-2016-07-728873
437. Landberg N, Hansen N, Askmyr M, Ågerstam H, Lassen C, Rissler M, et al. IL1RAP expression as a measure of leukemic stem cell burden at diagnosis of chronic myeloid leukemia predicts therapy outcome. Leukemia. (2016) 30:255–8. doi: 10.1038/leu.2015.135
438. Houshmand M, Simonetti G, Circosta P, Gaidano V, Cignetti A, Martinelli G, et al. Chronic myeloid leukemia stem cells. Leukemia. (2019) 33:1543–56. doi: 10.1038/s41375-019-0490-0
439. Sadovnik I, Herrmann H, Eisenwort G, Blatt K, Hoermann G, Mueller N, et al. Expression of CD25 on leukemic stem cells in BCR-ABL1+ CML: potential diagnostic value and functional implications. Exp Hematol. (2017) 51:17–24. doi: 10.1016/j.exphem.2017.04.003
440. Valent P, Sadovnik I, Eisenwort G, Bauer K, Herrmann H, Gleixner KV, et al. Immunotherapy-Based targeting and elimination of leukemic stem cells in AML and CML. Int J Mol Sci. (2019) 20. doi: 10.3390/ijms20174233
441. Madhumathi J, Sridevi S, Verma RS. CD25 targeted therapy of chemotherapy resistant leukemic stem cells using DR5 specific TRAIL peptide. Stem Cell Res. (2017) 19:65–75. doi: 10.1016/j.scr.2017.01.001
442. Bocchia M, Sicuranza A, Abruzzese E, Iurlo A, Sirianni S, Gozzini A, et al. Residual peripheral blood CD26+ leukemic stem cells in chronic myeloid leukemia patients during TKI therapy and during treatment-free remission. Front. Oncol. (2018) 8:194. doi: 10.3389/fonc.2018.00194
443. Culen M, Borsky M, Nemethova V, Razga F, Smejkal J, Jurcek T, et al. Quantitative assessment of the CD26+ leukemic stem cell compartment in chronic myeloid leukemia: patient-subgroups, prognostic impact, and technical aspects. Oncotarget. (2016) 7:33016–24. doi: 10.18632/oncotarget.9108
444. Galimberti S, Grassi S, Baratè C, Guerrini F, Ciabatti E, Perutelli F, et al. The polycomb BMI1 protein is co-expressed with CD26+ in leukemic stem cells of chronic myeloid leukemia. Front. Oncol. (2018) 8:555. doi: 10.3389/fonc.2018.00555
445. Zhou S, Zhu X, Liu W, Cheng F, Zou P, You Y, et al. Comparison of chronic myeloid leukemia stem cells and hematopoietic stem cells by global proteomic analysis. Biochem Biophys Res Commun. (2020) 522:362–7. doi: 10.1016/j.bbrc.2019.11.092
446. Raspadori D, Pacelli P, Sicuranza A, Abruzzese E, Iurlo A, Cattaneo D, et al. Flow cytometry assessment of CD26+ leukemic stem cells in peripheral blood: a simple and rapid new diagnostic tool for chronic myeloid leukemia. Cytometry B Clin Cytom. (2019) 96:294–9. doi: 10.1002/cyto.b.21764
447. Willmann M, Sadovnik I, Eisenwort G, Entner M, Bernthaler T, Stefanzl G, et al. Evaluation of cooperative antileukemic effects of nilotinib and vildagliptin in Ph+ chronic myeloid leukemia. Exp Hematol. (2018) 57:50–9.e6. doi: 10.1016/j.exphem.2017.09.012
448. Zhou S, Li W, Xiao Y, Zhu X, Zhong Z, Li Q, et al. A novel chimeric antigen receptor redirecting T-cell specificity towards CD26+ cancer cells. Leukemia. (2020). doi: 10.1038/s41375-020-0824-y
449. Frolova O, Benito J, Brooks C, Wang R-Y, Korchin B, Rowinsky EK, et al. SL-401 and SL-501, targeted therapeutics directed at the interleukin-3 receptor, inhibit the growth of leukaemic cells and stem cells in advanced phase chronic myeloid leukaemia. Br J Haematol. (2014) 166:862–74. doi: 10.1111/bjh.12978
450. Nievergall E, Ramshaw HS, Yong AS, Biondo M, Busfield SJ, Vairo G, et al. Monoclonal antibody targeting of IL-3 receptor α with CSL362 effectively depletes CML progenitor and stem cells. Blood. (2014) 123:1218–28. doi: 10.1182/blood-2012-12-475194
451. Zhang J, Zhao A, Sun L, Chen W, Zhang H, Chen Z, et al. Selective surface marker and miRNA profiles of CD34+ blast-derived microvesiCLes in chronic myelogenous leukemia. Oncol Lett. (2017) 14:1866–74. doi: 10.3892/ol.2017.6336
452. Ågerstam H, Hansen N, Palffy S von, Sandén C, Reckzeh K, Karlsson C, et al. IL1RAP antibodies block IL-1-induced expansion of candidate CML stem cells and mediate cell killing in xenograft models. Blood. (2016) 128:2683–93. doi: 10.1182/blood-2015-11-679985
453. Warda W, Larosa F, Neto Da Rocha M, Trad R, Deconinck E, Fajloun Z, et al. CML hematopoietic stem cells expressing IL1RAP can be targeted by chimeric antigen receptor-engineered T Cells. Cancer Res. (2019) 79:663–75. doi: 10.1158/0008-5472.CAN-18-1078
454. Zhou H, Mak PY, Mu H, Mak DH, Zeng Z, Cortes J, et al. Combined inhibition of β-catenin and Bcr-Abl synergistically targets tyrosine kinase inhibitor-resistant blast crisis chronic myeloid leukemia blasts and progenitors in vitro and in vivo. Leukemia. (2017) 31:2065–74. doi: 10.1038/leu.2017.87
455. Jing Hu, Min Feng, Zhang-Ling Liu, Yi Liu, Zheng-Lan Huang, Hui Li, et al. Potential role of Wnt/β-catenin signaling in blastic transformation of chronic myeloid leukemia: cross talk between β-catenin and BCR-ABL. Tumor Biol. (2016) 37:15859–72. doi: 10.1007/s13277-016-5413-3
456. de Cássia Viu Carrara R, Fontes AM, Abraham KJ, Orellana MD, Haddad SK, Palma PVB, et al. Expression differences of genes in the PI3K/AKT, WNT/b-catenin, SHH, NOTCH and MAPK signaling pathways in CD34+ hematopoietic cells obtained from chronic phase patients with chronic myeloid leukemia and from healthy controls. Clin Transl Oncol. (2018) 20:542–9. doi: 10.1007/s12094-017-1751-x
457. Masamoto Y, Kurokawa M. Targeting chronic myeloid leukemia stem cells: can transcriptional program be a druggable target for cancers? Stem Cell Investig. (2018) 5:10. doi: 10.21037/sci.2018.03.05
458. Zhao Y, Masiello D, McMillian M, Nguyen C, Wu Y, Melendez E, et al. CBP/catenin antagonist safely eliminates drug-resistant leukemia-initiating cells. Oncogene. (2016) 35:3705–17. doi: 10.1038/onc.2015.438
459. Jin B, Wang C, Li J, Du X, Ding K, Pan J. Anthelmintic niclosamide disrupts the interplay of p65 and FOXM1/beta-catenin and eradicates leukemia stem cells in chronic myelogenous leukemia. Clin Cancer Res. (2017) 23:789–803. doi: 10.1158/1078-0432.CCR-16-0226
460. Pellicano F, Scott MT, Helgason GV, Hopcroft LE, Allan EK, Aspinall-O'Dea M, et al. The antiproliferative activity of kinase inhibitors in chronic myeloid leukemia cells is mediated by FOXO transcription factors. Stem Cells. (2014) 32:2324–37. doi: 10.1002/stem.1748
461. Sadarangani A, Pineda G, Lennon KM, Chun H-J, Shih A, Schairer AE, et al. GLI2 inhibition abrogates human leukemia stem cell dormancy. J Transl Med. (2015) 13:98. doi: 10.1186/s12967-015-0453-9
462. Cui J, Li P, Liu X, Hu H, Wei W. Abnormal expression of the notch and Wnt/β-catenin signaling pathways in stem-like ALDHHI CD44+ cells correlates highly with Ki-67 expression in breast cancer. Oncol Lett. (2015) 9:1600–6. doi: 10.3892/ol.2015.2942
463. Ponta H, Sherman L, Herrlich PA. CD44: from adhesion molecules to signalling regulators. Nat Rev Mol Cell Biol. (2003) 4:33–45. doi: 10.1038/nrm1004
464. Akamine T, Tagawa T, Ijichi K, Toyokawa G, Takamori S, Hirai F, et al. The significance of CD44 variant 9 in resected lung adenocarcinoma: correlation with pathological early-stage and EGFR mutation. Ann Surg Oncol. (2019) 26:1544–51. doi: 10.1245/s10434-018-07137-2
465. Li M, Zhang B, Zhang Z, Liu X, Qi X, Zhao J, et al. Stem cell-like circulating tumor cells indicate poor prognosis in gastric cancer. Biomed Res Int. (2014) 2014:981261. doi: 10.1155/2014/981261
466. Stroomer JW, Roos JC, Sproll M, Quak JJ, Heider KH, Wilhelm BJ, et al. Safety and biodistribution of 99mTechnetium-labeled anti-CD44v6 monoclonal antibody BIWA 1 in head and neck cancer patients. Clin Cancer Res. (2000) 6:3046–55.
467. Börjesson PK, Postema EJ, Roos JC, Colnot DR, Marres HA, van Schie MH, et al. Phase I therapy study with 186Re-labeled humanized monoclonal antibody BIWA 4 (Bivatuzumab) in patients with head and neck squamous cell carcinoma. Clin Cancer Res. (2003) 9(10 Pt 2):3961–72s.
468. Menke-van der Houven van Oordt CW, Gomez-Roca C, van Herpen C, Coveler AL, Mahalingam D, Verheul HMW, et al. First-in-human phase I clinical trial of RG7356, an anti-CD44 humanized antibody, in patients with advanced, CD44-expressing solid tumors. Oncotarget. (2016) 7:80046–58. doi: 10.18632/oncotarget.11098
469. Li Z. CD133: a stem cell biomarker and beyond. Exp Hematol Oncol. (2013) 2:17. doi: 10.1186/2162-3619-2-17
470. Ren F, Sheng WQ, Du X. CD133: a cancer stem cells marker, is used in colorectal cancers. World J Gastroenterol. (2013) 19:2603–11. doi: 10.3748/wjg.v19.i17.2603
471. Gazzaniga P, Gradilone A, Petracca A, Nicolazzo C, Raimondi C, Iacovelli R, et al. Molecular markers in circulating tumour cells from metastatic colorectal cancer patients. J Cell Mol Med. (2010) 14:2073–7. doi: 10.1111/j.1582-4934.2010.01117.x
472. Xia P. CD133 mRNA may be a suitable prognostic marker for human breast cancer. Stem Cell Investig. (2017) 4:87. doi: 10.21037/sci.2017.10.03
473. Trzpis M, MCLaughlin PM, Leij LM de, Harmsen MC. Epithelial cell adhesion molecule: more than a carcinoma marker and adhesion molecule. Am J Pathol. (2007) 171:386–95. doi: 10.2353/ajpath.2007.070152
474. Cirulli V, Crisa L, Beattie GM, Mally MI, Lopez AD, Fannon A, et al. KSA antigen Ep-CAM mediates cell-cell adhesion of pancreatic epithelial cells: morphoregulatory roles in pancreatic islet development. J Cell Biol. (1998) 140:1519–34. doi: 10.1083/jcb.140.6.1519
475. Huang L, Yang Y, Yang F, Liu S, Zhu Z, Lei Z, et al. Functions of EpCAM in physiological processes and diseases (review). Int J Mol Med. (2018) 42:1771–85. doi: 10.3892/ijmm.2018.3764
476. Balzar M, Winter MJ, Boer CJ de, Litvinov SV. The biology of the 17-1A antigen (Ep-CAM). J Mol Med. (1999) 77:699–712. doi: 10.1007/s001099900038
477. Schmelzer E, Zhang L, Bruce A, Wauthier E, Ludlow J, Yao H-l, et al. Human hepatic stem cells from fetal and postnatal donors. J Exp Med. (2007) 204:1973–87. doi: 10.1084/jem.20061603
478. Kamimoto K, Kaneko K, Kok CY-Y, Okada H, Miyajima A, Itoh T. Heterogeneity and stochastic growth regulation of biliary epithelial cells dictate dynamic epithelial tissue remodeling. Elife. (2016) 5:15034. doi: 10.7554/eLife.15034
479. Schnell U, Cirulli V, Giepmans BN. EpCAM: structure and function in health and disease. Biochim Biophys Acta. (2013) 1828:1989–2001. doi: 10.1016/j.bbamem.2013.04.018
480. Hiraga T, Ito S, Nakamura H. EpCAM expression in breast cancer cells is associated with enhanced bone metastasis formation. Int J Cancer. (2016) 138:1698–708. doi: 10.1002/ijc.29921
481. Kim J, Orkin SH. Embryonic stem cell-specific signatures in cancer: insights into genomic regulatory networks and implications for medicine. Genome Med. (2011) 3:75. doi: 10.1186/gm291
482. Hadjimichael C, Chanoumidou K, Papadopoulou N, Arampatzi P, Papamatheakis J, Kretsovali A. Common stemness regulators of embryonic and cancer stem cells. World J Stem Cells. (2015) 7:1150–84. doi: 10.4252/wjsc.v7.i9.1150
483. Rosner MH, Vigano MA, Ozato K, TIMmons PM, Poirier F, Rigby PW, et al. A POU-domain transcription factor in early stem cells and germ cells of the mammalian embryo. Nature. (1990) 345:686–92. doi: 10.1038/345686a0
484. Carrasco-Garcia E, Santos JC, Garcia I, Brianti M, García-Puga M, Pedrazzoli J, et al. Paradoxical role of SOX2 in gastric cancer. Am J Cancer Res. (2016) 6:701–13.
485. Wang Y-J, Herlyn M. The emerging roles of Oct4 in tumor-initiating cells. Am J Physiol Cell Physiol. (2015) 309:C709–18. doi: 10.1152/ajpcell.00212.2015
486. Dai X, Ge J, Wang X, Qian X, Zhang C, Li X. OCT4 regulates epithelial-mesenchymal transition and its knockdown inhibits colorectal cancer cell migration and invasion. Oncol Rep. (2013) 29:155–60. doi: 10.3892/or.2012.2086
487. Jeter CR, Yang T, Wang J, Chao H-P, Tang DG. Concise review: NANOG in cancer stem cells and tumor development: an update and outstanding questions. Stem Cells. (2015) 33:2381–90. doi: 10.1002/stem.2007
488. Quéré R, Andradottir S, Brun AC, Zubarev RA, Karlsson G, Olsson K, et al. High levels of the adhesion molecule CD44 on leukemic cells generate acute myeloid leukemia relapse after withdrawal of the initial transforming event. Leukemia. (2010) 25:515–26. doi: 10.1038/leu.2010.281
489. Wang N-S, Wei M, Ma W-l, Meng W, Zheng W-l. Knockdown of CD44 enhances chemosensitivity of acute myeloid leukemia cells to ADM Ara-C. Tumour Biol. (2014) 35:3933–40. doi: 10.1007/s13277-013-1523-3
490. Huang X, Li D, Li T, Zhao BO, Chen X. Prognostic value of the expression of phosphatase and tensin homolog and CD44 in elderly patients with refractory acute myeloid leukemia. Oncol Lett. (2015) 10:103–10. doi: 10.3892/ol.2015.3189
491. Zhou H-S, Carter BZ, Andreeff M. Bone marrow niche-mediated survival of leukemia stem cells in acute myeloid leukemia: Yin and Yang. Cancer Biol Med. (2016) 13:248–59. doi: 10.20892/j.issn.2095-3941.2016.0023
492. Testa U, Pelosi E, Castelli G. CD123 as a therapeutic target in the treatment of hematological malignancies. Cancers. (2019) 11:1358. doi: 10.3390/cancers11091358
493. Pollyea DA, Jordan CT. Therapeutic targeting of acute myeloid leukemia stem cells. Blood. (2017) 129:1627–35. doi: 10.1182/blood-2016-10-696039
494. Wittwer NL, Brumatti G, Marchant C, Sandow JJ, Pudney MK, Dottore M, et al. High CD123 levels enhance proliferation in response to IL-3, but reduce chemotaxis by downregulating CXCR4 expression. Blood Adv. (2017) 1:1067–79. doi: 10.1182/bloodadvances.2016002931
495. Faez A, Subh SA-M. Impact of leukemia stem cells phenotype expression on response to induction therapy in acute myeloid leukemia patients. Cardiovasc Hematol Disord Drug Targets. (2020) 20:145–151. doi: 10.2174/1871529X19666190719105954
496. Zhou J, Chng W-J. Identification and targeting leukemia stem cells: the path to the cure for acute myeloid leukemia. World J Stem Cells. (2014) 6:473–84. doi: 10.4252/wjsc.v6.i4.473
497. Davis JR, Benjamin DJ, Jonas BA. New and emerging therapies for acute myeloid leukaemia. J Investig Med. (2018) 66:1088–95. doi: 10.1136/jim-2018-000807
498. Kikushige Y, Miyamoto T. Identification of TIM-3 as a leukemic stem cell surface molecule in primary acute myeloid leukemia. Oncology. (2015) 89(Suppl. 1):28–32. doi: 10.1159/000431062
499. Grainger S, Traver D, Willert K. Wnt signaling in hematological malignancies. Prog Mol Biol Transl Sci. (2018) 153:321–41. doi: 10.1016/bs.pmbts.2017.11.002
500. Zhao Y, Wu K, Wu Y, Melendez E, Smbatyan G, Massiello D, et al. Characterization of imatinib resistant CML leukemic stem/Initiating cells and their sensitivity to CBP/Catenin antagonists. Curr Mol Pharmacol. (2018) 11:113–21. doi: 10.2174/1874467210666170919155739
501. Blaudszun A-R, Moldenhauer G, Schneider M, Philippi A. A photosensitizer delivered by bispecific antibody redirected T lymphocytes enhances cytotoxicity against EpCAM-expressing carcinoma cells upon light irradiation. J Control Release. (2015) 197:58–68. doi: 10.1016/j.jconrel.2014.10.025
502. Hosoya N, Miyagawa K. Targeting DNA damage response in cancer therapy. Cancer Sci. (2014) 105:370–88. doi: 10.1111/cas.12366
503. Ruhland MK, Coussens LM, Stewart SA. Senescence and cancer: an evolving inflammatory paradox. Biochim Biophys Acta. (2016) 1865:14–22. doi: 10.1016/j.bbcan.2015.10.001
504. Ewald JA, Desotelle JA, Wilding G, Jarrard DF. Therapy-induced senescence in cancer. J Natl Cancer Inst. (2010) 102:1536–46. doi: 10.1093/jnci/djq364
505. Dodig S, Cepelak I, Pavić I. Hallmarks of senescence and aging. Biochem Med. (2019) 29:030501. doi: 10.11613/BM.2019.030501
506. Lee S, Lee J-S. Cellular senescence: a promising strategy for cancer therapy. BMB Rep. (2019) 52:35–41. doi: 10.5483/BMBRep.2019.52.1.294
507. Chakradeo S, Elmore LW, Gewirtz DA. Is senescence reversible? Curr Drug Targets. (2016) 17:460–6. doi: 10.2174/1389450116666150825113500
508. Zhao H, Darzynkiewicz Z. Biomarkers of cell senescence assessed by imaging cytometry. Methods Mol Biol. (2013) 965:83–92. doi: 10.1007/978-1-62703-239-1_5
509. Aljabery F, Shabo I, Gimm O, Jahnson S, Olsson H. The expression profile of p14, p53 and p21 in tumour cells is associated with disease-specific survival and the outcome of postoperative chemotherapy treatment in muscle-invasive bladder cancer. Urol Oncol. (2018) 36:530.e7-530.e18. doi: 10.1016/j.urolonc.2018.05.025
510. Fallah M, Mohammadi H, Shaki F, Hosseini-Khah Z, Moloudizargari M, Dashti A, et al. Doxorubicin and liposomal doxorubicin induce senescence by enhancing nuclear factor kappa B and mitochondrial membrane potential. Life Sci. (2019) 232:116677. doi: 10.1016/j.lfs.2019.116677
511. Hayman L, Chaudhry WR, Revin VV, Zhelev N, Bourdon J-C. What is the potential of p53 isoforms as a predictive biomarker in the treatment of cancer? Expert Rev Mol Diagn. (2019) 19:149–59. doi: 10.1080/14737159.2019.1563484
512. Moraes JK de, Wagner VP, Fonseca FP, Amaral-Silva GK, Farias CB de, Pilar EF, et al. Activation of BDNF/TrkB/Akt pathway is associated with aggressiveness and unfavorable survival in oral squamous cell carcinoma. Oral Dis. (2019) 25:1925–36. doi: 10.1111/odi.13190
513. Lin T, Hou P-F, Meng S, Chen F, Jiang T, Li M-L, et al. Emerging roles of p53 related lncRNAs in cancer progression: a systematic review. Int J Biol Sci. (2019) 15:1287–98. doi: 10.7150/ijbs.33218
514. van den Bossche J, Deben C, Pauw I de, Lambrechts H, Hermans C, Deschoolmeester V, et al. In vitro study of the polo-like kinase 1 inhibitor volasertib in non-small-cell lung cancer reveals a role for the tumor suppressor p53. Mol Oncol. (2019) 13:1196–213. doi: 10.1002/1878-0261.12477
515. Zamorano-León JJ, Ballesteros S, las Heras N de, Alvarez-Sala L, La SeRNA-Soto M de, Zekri-Nechar K, et al. Effect of pectin on the expression of proteins associated with mitochondrial biogenesis and cell senescence in HT29-human colorectal adenocarcinoma cells. Prev Nutr Food Sci. (2019) 24:187–96. doi: 10.3746/pnf.2019.24.2.187
516. Wagner J, Damaschke N, Yang B, Truong M, Guenther C, McCormick J, et al. Overexpression of the novel senescence marker β-Galactosidase (GLB1) in prostate cancer predicts reduced PSA recurrence. PLoS ONE. (2015) 10:e0124366. doi: 10.1371/jouRNAl.pone.0124366
517. Zhang R, Chen W, Adams PD. Molecular dissection of formation of senescence-Associated heterochromatin foci?†. Mol Cell Biol. (2007) 27:2343–58. doi: 10.1128/MCB.02019-06
518. Bernadotte A, Mikhelson VM, Spivak IM. Markers of cellular senescence. telomere shortening as a marker of cellular senescence. Aging. (2016) 8:3–11. doi: 10.18632/aging.100871
519. Hartman ML, Sztiller-Sikorska M, Czyz M. Whole-exome sequencing reveals novel genetic variants associated with diverse phenotypes of melanoma cells. Mol Carcinog. (2019) 58:588–602. doi: 10.1002/mc.22953
520. Long F, He Y, Fu H, Li Y, Bao X, Wang Q, et al. PreClinical characterization of SHR6390, a novel CDK 4/6 inhibitor, in vitro and in human tumor xenograft models. Cancer Sci. (2019) 110:1420–30. doi: 10.1111/cas.13957
521. Knutson KL, CLynes R, Shreeder B, Yeramian P, Kemp KP, Ballman K, et al. Improved survival of HER2+ breast cancer patients treated with trastuzumab and chemotherapy is associated with host antibody immunity against the HER2 intracellular domain. Cancer Res. (2016) 76:3702–10. doi: 10.1158/0008-5472.CAN-15-3091
522. Gunaratna RT, Santos A, Luo L, Nagi C, Lambertz I, Spier M, et al. Dynamic role of the codon 72 p53 single-Nucleotide polymorphism in mammary tumorigenesis in a humanized mouse model. Oncogene. (2019) 38:3535–50. doi: 10.1038/s41388-018-0630-4
523. Smolle E, Fink-Neuboeck N, Lindenmann J, Smolle-Juettner F, Pichler M. The biological and clinical relevance of inhibitor of growth (ING) genes in non-Small cell lung cancer. Cancers. (2019) 11. doi: 10.3390/cancers11081118
524. Xiang X-H, Yang L, Zhang X, Ma X-H, Miao R-C, Gu J-X, et al. Seven-senescence-associated gene signature predicts overall survival for asian patients with hepatocellular carcinoma. World J Gastroenterol. (2019) 25:1715–28. doi: 10.3748/wjg.v25.i14.1715
525. Eggert T, Wolter K, Ji J, Ma C, Yevsa T, Klotz S, et al. Distinct functions of senescence-associated immune responses in liver tumor surveillance and tumor progression. Cancer Cell. (2016) 30:533–47. doi: 10.1016/j.ccell.2016.09.003
526. Coppé J-P, Desprez P-Y, Krtolica A, Campisi J. The senescence-associated secretory phenotype: the dark side of tumor suppression. Annu Rev Pathol. (2010) 5:99–118. doi: 10.1146/annurev-pathol-121808-102144
527. Ma F, Chen D, Chen F, Chi Y, Han Z, Feng X, et al. Human umbilical cord mesenchymal stem cells promote breast cancer metastasis by interleukin-8- and interleukin-6-dependent induction of CD44(+)/CD24(-) cells. Cell Transplant. (2015) 24:2585–99. doi: 10.3727/096368915X687462
528. Ortiz-Montero P, Londoño-Vallejo A, Vernot J-P. Senescence-associated IL-6 and IL-8 cytokines induce a self- and cross-reinforced senescence/inflammatory milieu strengthening tumorigenic capabilities in the MCF-7 breast cancer cell line. Cell Commun Signal. (2017) 15:17. doi: 10.1186/s12964-017-0172-3
529. Yamaguchi N, Nakayama Y, Yamaguchi N. Down-regulation of forkhead box protein A1 (FOXA1) leads to cancer stem cell-like properties in tamoxifen-resistant breast cancer cells through induction of interleukin-6. J Biol Chem. (2017) 292:8136–48. doi: 10.1074/jbc.M116.763276
530. Peng D, Tanikawa T, Li W, Zhao L, Vatan L, Szeliga W, et al. Myeloid-Derived suppressor cells endow stem-like qualities to breast cancer cells through IL6/STAT3 and NO/NOTCH cross-talk signaling. Cancer Res. (2016) 76:3156–65. doi: 10.1158/0008-5472.CAN-15-2528
531. Saha S, Mukherjee S, Khan P, Kajal K, Mazumdar M, Manna A, et al. Aspirin suppresses the acquisition of chemoresistance in breast cancer by disrupting an NFκB-IL6 signaling axis responsible for the generation of cancer stem cells. Cancer Res. (2016) 76:2000–12. doi: 10.1158/0008-5472.CAN-15-1360
532. Wolf B, Krieg K, Falk C, Breuhahn K, Keppeler H, Biedermann T, et al. Inducing differentiation of premalignant hepatic cells as a novel therapeutic strategy in hepatocarcinoma. Cancer Res. (2016) 76:5550–61. doi: 10.1158/0008-5472.CAN-15-3453
533. Oh K, Lee O-Y, Park Y, Seo MW, Lee D-S. IL-1β induces IL-6 production and increases invasiveness and estrogen-independent growth in a TG2-dependent manner in human breast cancer cells. BMC Cancer. (2016) 16:724. doi: 10.1186/s12885-016-2746-7
534. Gallo M, Frezzetti D, Roma C, Chicchinelli N, Barbieri A, Arra C, et al. RANTES and IL-6 cooperate in inducing a more aggressive phenotype in breast cancer cells. Oncotarget. (2018) 9:17543–53. doi: 10.18632/oncotarget.24784
535. Yevsa T, Kang T-W, Zender L. Immune surveillance of pre-cancerous senescent hepatocytes limits hepatocellular carcinoma development. Oncoimmunology. (2012) 1:398–9. doi: 10.4161/onci.19128
536. Kang T-W, Yevsa T, Woller N, Hoenicke L, Wuestefeld T, Dauch D, et al. Senescence surveillance of pre-malignant hepatocytes limits liver cancer development. Nature. (2011) 479:547–51. doi: 10.1038/nature10599
537. Was H Barszcz K Czarnecka J Kowalczyk A Bernas T Uzarowska E . Bafilomycin A1 triggers proliferative potential of senescent cancer cells in vitro and in NOD/SCID mice. Oncotarget. (2016) 8:9303–22. doi: 10.18632/oncotarget.14066
538. Koboldt DC, Fulton RS, McLellan MD, Schmidt H, Kalicki-Veizer J, McMichael JF, et al. Comprehensive molecular portraits of human breast tumours. Nature. (2012) 490:61–70. doi: 10.1038/nature11412
539. Yamao T, Yamashita Y-I, Yamamura K, Nakao Y, Tsukamoto M, Nakagawa S, et al. Cellular senescence, represented by expression of caveolin-1, in cancer-associated fibroblasts promotes tumor invasion in pancreatic cancer. Ann Surg Oncol. (2019) 26:1552–9. doi: 10.1245/s10434-019-07266-2
540. Jabbour W, Wion D. Biomarkers of aging associated with past treatments in breast cancer survivors: when therapy-induced pathways turn out to be potential therapeutic targets. NPJ Breast Cancer. (2018) 4:1–2. doi: 10.1038/s41523-018-0058-6
541. Di G-H, Liu Y, Lu Y, Liu J, Wu C, Duan H-F. IL-6 secreted from senescent mesenchymal stem cells promotes proliferation and migration of breast cancer cells. PLoS ONE. (2014) 9:e113572. doi: 10.1371/jouRNAl.pone.0113572
542. Marotta LL, Almendro V, Marusyk A, Shipitsin M, Schemme J, Walker SR, et al. The JAK2/STAT3 signaling pathway is required for growth of CD44+CD24− stem cell-like breast cancer cells in human tumors. J Clin Invest. (2011) 121:2723–35. doi: 10.1172/JCI44745
543. Wang T, Song P, Zhong T, Wang X, Xiang X, Liu Q, et al. The inflammatory cytokine IL-6 induces FRA1 deacetylation promoting colorectal cancer stem-like properties. Oncogene. (2019) 38:4932–47. doi: 10.1038/s41388-019-0763-0
544. Dou Z, Berger SL. Senescence elicits stemness: a surprising mechanism for cancer relapse. Cell Metab. (2018) 27:710–1. doi: 10.1016/j.cmet.2018.03.009
545. Poltavets V, Kochetkova M, Pitson SM, Samuel MS. The role of the extracellular matrix and its molecular and cellular regulators in cancer cell plasticity. Front. Oncol. (2018) 8:431. doi: 10.3389/fonc.2018.00431
546. Aloia L, McKie MA, Huch M. Cellular plasticity in the adult liver and stomach. J Physiol. (2016) 594:4815–25. doi: 10.1113/JP271769
547. Chen Y, Wong PP, Sjeklocha L, Steer CJ, Sahin MB. Mature hepatocytes exhibit unexpected plasticity by direct dedifferentiation into liver progenitor cells in culture. Hepatology. (2012) 55:563–74. doi: 10.1002/hep.24712
548. Chaffer CL, Marjanovic ND, Lee T, Bell G, Kleer CG, Reinhardt F, et al. Poised chromatin at the ZEB1 promoter enables breast cancer cell plasticity and enhances tumorigenicity. Cell. (2013) 154:61–74. doi: 10.1016/j.cell.2013.06.005
549. Akunuru S, James Zhai Q, Zheng Y. Non-small cell lung cancer stem/progenitor cells are enriched in multiple distinct phenotypic subpopulations and exhibit plasticity. Cell Death Dis. (2012) 3:e352. doi: 10.1038/cddis.2012.93
550. Vassalli G. Aldehyde dehydrogenases: not just markers, but functional regulators of stem cells. Stem Cells Int. (2019) 2019:3904645. doi: 10.1155/2019/3904645
551. Clark DW, Palle K. Aldehyde dehydrogenases in cancer stem cells: potential as therapeutic targets. Ann Transl Med. (2016) 4:518. doi: 10.21037/atm.2016.11.82
552. Doherty MR, Parvani JG, Tamagno I, Junk DJ, Bryson BL, Cheon HJ, et al. The opposing effects of interferon-beta and oncostatin-M as regulators of cancer stem cell plasticity in triple-negative breast cancer. Breast Cancer Res. (2019) 21:1–12. doi: 10.1186/s13058-019-1136-x
553. Pereira L, Mariadason JM, Hannan RD, Dhillon AS. Implications of epithelial-mesenchymal plasticity for heterogeneity in colorectal cancer. Front. Oncol. (2015) 5:13. doi: 10.3389/fonc.2015.00013
554. Zhan T, Ambrosi G, Wandmacher AM, Rauscher B, Betge J, Rindtorff N, et al. MEK inhibitors activate Wnt signalling and induce stem cell plasticity in colorectal cancer. Nat Commun. (2019) 10:2197. doi: 10.1038/s41467-019-09898-0
555. Legge DN, Shephard AP, Collard TJ, Greenhough A, Chambers AC, CLarkson RW, et al. BCL-3 promotes a cancer stem cell phenotype by enhancing β-catenin signalling in colorectal tumour cells. Dis Model Mech. (2019) 12:037697. doi: 10.1242/dmm.037697
556. Liu D, Du L, Chen D, Ye Z, Duan H, Tu T, et al. Reduced CD146 expression promotes tumorigenesis and cancer stemness in colorectal cancer through activating wnt/β-catenin signaling. Oncotarget. (2016) 7:40704–18. doi: 10.18632/oncotarget.9930
557. Mohammed MK, Shao C, Wang J, Wei Q, Wang X, Collier Z, et al. Wnt/beta-catenin signaling plays an ever-expanding role in stem cell self-renewal, tumorigenesis and cancer chemoresistance. Genes Dis. (2016) 3:11–40. doi: 10.1016/j.gendis.2015.12.004
558. Mao J, Fan S, Ma W, Fan P, Wang B, Zhang J, et al. Roles of wnt/β-catenin signaling in the gastric cancer stem cells proliferation and salinomycin treatment. Cell Death Dis. (2014) 5:e1039. doi: 10.1038/cddis.2013.515
559. Correnti M, Raggi C. Stem-like plasticity and heterogeneity of circulating tumor cells: current status and prospect challenges in liver cancer. Oncotarget. (2017) 8:7094–115. doi: 10.18632/oncotarget.12569
560. Shibue T, Weinberg RA. EMT, CSCs, and drug resistance: the mechanistic link and Clinical implications. Nat Rev Clin Oncol. (2017) 14:611–29. doi: 10.1038/nrCLinonc.2017.44
561. Zhang D, Tang DG, Rycaj K. Cancer stem cells: regulation programs, immunological properties and immunotherapy. Semin Cancer Biol. (2018) 52:94–106. doi: 10.1016/j.semcancer.2018.05.001
562. Pan Q, Li Q, Liu S, Ning N, Zhang X, Xu Y, et al. Concise review: targeting cancer stem cells using immunologic approaches. Stem Cells. (2015) 33:2085–92. doi: 10.1002/stem.2039
563. Schmidts A, Maus MV. Making CAR T cells a solid option for solid tumors. Front Immunol. (2018) 9:2593. doi: 10.3389/fimmu.2018.02593
564. Long KB, Young RM, Boesteanu AC, Davis MM, Melenhorst JJ, Lacey SF, et al. CAR T cell therapy of non-hematopoietic malignancies: detours on the road to clinical success. Front Immunol. (2018) 9:2740. doi: 10.3389/fimmu.2018.02740
565. Zhu X, Prasad S, Gaedicke S, Hettich M, Firat E, Niedermann G. Patient-derived glioblastoma stem cells are killed by CD133-specific CAR T cells but induce the T cell aging marker CD57. Oncotarget. (2015) 6:171–84. doi: 10.18632/oncotarget.2767
566. Hu B, Zou Y, Zhang L, Tang J, Niedermann G, Firat E, et al. Nucleofection with plasmid DNA for CRISPR/Cas9-mediated inactivation of programmed cell death protein 1 in CD133-specific CAR T Cells. Hum Gene Ther. (2019) 30:446–58. doi: 10.1089/hum.2017.234
567. Klapdor R, Wang S, Hacker U, Büning H, Morgan M, Dörk T, et al. Improved killing of ovarian cancer stem cells by combining a novel chimeric antigen receptor-Based immunotherapy and chemotherapy. Hum Gene Ther. (2017) 28:886–96. doi: 10.1089/hum.2017.168
568. Wang Y, Chen M, Wu Z, Tong C, Dai H, Guo Y, et al. CD133-directed CAR T cells for advanced metastasis malignancies: a phase I trial. Oncoimmunology. (2018) 7:e1440169. doi: 10.1080/2162402X.2018.1440169
569. Feng K-C, Guo Y-L, Liu Y, Dai H-R, Wang Y, Lv H-Y, et al. Cocktail treatment with EGFR-specific and CD133-specific chimeric antigen receptor-modified T cells in a patient with advanced cholangiocarcinoma. J Hematol Oncol. (2017) 10:4. doi: 10.1186/s13045-016-0378-7
570. Colnot DR, Quak JJ, Roos JC, van Lingen A, Wilhelm AJ, van Kamp GJ, et al. Phase I therapy study of 186Re-labeled chimeric monoclonal antibody U36 in patients with squamous cell carcinoma of the head and neck. J Nucl Med. (2000) 41:1999–2010.
571. Colnot DR, Ossenkoppele GJ, Roos JC, Quak JJ, Bree Rd, Börjesson PK, et al. Reinfusion of unprocessed, granulocyte colony-stimulating factor-stimulated whole blood allows dose escalation of 186Relabeled chimeric monoclonal antibody U36 radioimmunotherapy in a phase I dose escalation study. Clin Cancer Res. (2002) 8:3401–6.
572. Vey N, Delaunay J, Martinelli G, Fiedler W, Raffoux E, Prebet T, et al. Phase I clinical study of RG7356, an anti-CD44 humanized antibody, in patients with acute myeloid leukemia. Oncotarget. (2016) 7:32532–42. doi: 10.18632/oncotarget.8687
573. Tijink BM, Buter J, Bree Rd, Giaccone G, Lang MS, Staab A, et al. A phase I dose escalation study with anti-CD44v6 bivatuzumab mertansine in patients with incurable squamous cell carcinoma of the head and neck or esophagus. Clin Cancer Res. (2006) 12:6064–72. doi: 10.1158/1078-0432.CCR-06-0910
574. Rupp U, Schoendorf-Holland E, Eichbaum M, Schuetz F, Lauschner I, Schmidt P, et al. Safety and pharmacokinetics of bivatuzumab mertansine in patients with CD44v6-positive metastatic breast cancer: final results of a phase I study. Anticancer Drugs. (2007) 18:477–85. doi: 10.1097/CAD.0b013e32801403f4
575. Riechelmann H, Sauter A, Golze W, Hanft G, Schroen C, Hoermann K, et al. Phase I trial with the CD44v6-targeting immunoconjugate bivatuzumab mertansine in head and neck squamous cell carcinoma. Oral Oncol. (2008) 44:823–9. doi: 10.1016/j.oraloncology.2007.10.009
576. Leuci V, Casucci GM, Grignani G, Rotolo R, Rossotti U, Vigna E, et al. CD44v6 as innovative sarcoma target for CAR-redirected CIK cells. Oncoimmunology. (2018) 7:e1423167. doi: 10.1080/2162402X.2017.1423167
577. Zhou Y, Wen P, Li M, Li Y, Li X-A. Construction of chimeric antigen receptor-modified T cells targeting EpCAM and assessment of their anti-tumor effect on cancer cells. Mol Med Rep. (2019) 20:2355–64. doi: 10.3892/mmr.2019.10460
578. Armstrong A, Eck SL. EpCAM: a new therapeutic target for an old cancer antigen. Cancer Biol Ther. (2003) 2:320–6. doi: 10.4161/cbt.2.4.451
579. Zhang B-L, Li D, Gong Y-L, Huang Y, Qin D-Y, Jiang L, et al. Preclinical evaluation of chimeric antigen receptor-modified T Cells specific to epithelial cell adhesion molecule for treating colorectal cancer. Hum Gene Ther. (2019) 30:402–12. doi: 10.1089/hum.2018.229
580. Zhang Q, Zhang H, Ding J, Liu H, Li H, Li H, et al. Combination therapy with EPCAM-CAR-NK-92 cells and regorafenib against human colorectal cancer models. J Immunol Res. (2018) 2018:1–11. doi: 10.1155/2018/4263520
581. Ang WX, Li Z, Chi Z, Du S-H, Chen C, Tay JCK, et al. Intraperitoneal immunotherapy with T cells stably and transiently expressing anti-EpCAM CAR in xenograft models of peritoneal carcinomatosis. Oncotarget. (2017) 8:13545–59. doi: 10.18632/oncotarget.14592
582. Study Evaluating Safety Efficacy of CAR-T Cells Targeting CD123 in Patients with Acute Leukemia. CLinicalTrials.gov (2020). Available online at: https://clinicaltrials.gov/ct2/show/NCT03672851?term=NCT03672851&draw=2&rank=1 (accessed April 27, 2020).
583. Hegde M, Mukherjee M, Grada Z, Pignata A, Landi D, Navai SA, et al. Tandem CAR T cells targeting HER2 and IL13Rα2 mitigate tumor antigen escape. J Clin Invest. (2016) 126:3036–52. doi: 10.1172/JCI83416
584. Bielamowicz K, Fousek K, Byrd TT, Samaha H, Mukherjee M, Aware N, et al. Trivalent CAR T cells overcome interpatient antigenic variability in glioblastoma. Neuro Oncol. (2018) 20:506–18. doi: 10.1093/neuonc/nox182
585. Guedan S, Calderon H, Posey AD, Maus MV. Engineering and design of chimeric antigen receptors. Mol Ther Methods Clin Dev. (2019) 12:145–56. doi: 10.1016/j.omtm.2018.12.009
586. Minutolo NG, Hollander EE, Powell DJ. The emergence of universal immune receptor T cell therapy for cancer. Front Oncol. (2019) 9:176. doi: 10.3389/fonc.2019.00176
587. MacKay M, Afshinnekoo E, Rub J, Hassan C, Khunte M, Baskaran N, et al. The therapeutic landscape for cells engineered with chimeric antigen receptors. Nature Biotechnol. (2020) 38:233–44. doi: 10.1038/s41587-019-0329-2
588. Bachmann M. The uni CAR system: a modular CAR T cell approach to improve the safety of CAR T cells. Immunol Lett. (2019) 211:13–22. doi: 10.1016/j.imlet.2019.05.003
589. Cho JH, Collins JJ, Wong WW. Universal chimeric antigen receptors for multiplexed and logical control of T cell responses. Cell. (2018) 173:1426–38.e11. doi: 10.1016/j.cell.2018.03.038
590. Hübner J, Hoseini SS, Suerth JD, Hoffmann D, Maluski M, Herbst J, et al. Generation of genetically engineered precursor T-Cells from human umbilical cord blood using an optimized alpharetroviral vector platform. Mol Ther. (2016) 24:1216–26. doi: 10.1038/mt.2016.89
591. Gargett T, Brown MP. The inducible caspase-9 suicide gene system as a “safety switch” to limit on-target, off-tumor toxicities of chimeric antigen receptor T cells. Front Pharmacol. (2014) 5:235. doi: 10.3389/fphar.2014.00235
592. Fedorov VD, Themeli M, Sadelain M. PD-1– and CTLA-4–based inhibitory chimeric antigen receptors (ICARs) divert off-Target immunotherapy responses. Sci Transl Med. (2013) 5:215ra172. doi: 10.1126/scitranslmed.3006597
593. Roybal KT, Rupp LJ, Morsut L, Walker WJ, McNally KA, Park JS, et al. Precision tumor recognition by T Cells with combinatorial antigen-sensing circuits. Cell. (2016) 164:770–9. doi: 10.1016/j.cell.2016.01.011
594. Zhang Q, Tian K, Xu J, Zhang H, Li L, Fu Q, et al. Synergistic effects of cabozantinib and EGFR-specific CAR-NK-92 cells in renal cell carcinoma. J Immunol Res. (2017) 2017:6915912. doi: 10.1155/2017/6915912
595. Kwilas AR, Ardiani A, Donahue RN, Aftab DT, Hodge JW. Dual effects of a targeted small-molecule inhibitor (cabozantinib) on immune-mediated killing of tumor cells and immune tumor microenvironment permissiveness when combined with a cancer vaccine. J Transl Med. (2014) 12:294. doi: 10.1186/s12967-014-0294-y
596. Li H, Ding J, Lu M, Liu H, Miao Y, Li L, et al. CAIX-specific CAR-T cells and sunitinib show synergistic effects against metastatic renal cancer models. J Immunother. (2019) 43:16–28. doi: 10.1097/CJI.0000000000000301
597. Wu X, Huang S. HER2-specific chimeric antigen receptor-engineered natural killer cells combined with apatinib for the treatment of gastric cancer. Bull Cancer. (2019) 106:946–58. doi: 10.1016/j.bulcan.2019.03.012
598. PeRNA F, Berman SH, Soni RK, Mansilla-Soto J, Eyquem J, Hamieh M, et al. Integrating proteomics and transcriptomics for systematic combinatorial chimeric antigen receptor therapy of AML. Cancer Cell. (2017) 32:506–19.e5. doi: 10.1016/j.ccell.2017.09.004
Keywords: cancer stem cells, senescence, targeted therapy, CAR cells, biomarkers, precision therapy
Citation: Walcher L, Kistenmacher A-K, Suo H, Kitte R, Dluczek S, Strauß A, Blaudszun A-R, Yevsa T, Fricke S and Kossatz-Boehlert U (2020) Cancer Stem Cells—Origins and Biomarkers: Perspectives for Targeted Personalized Therapies. Front. Immunol. 11:1280. doi: 10.3389/fimmu.2020.01280
Received: 29 February 2020; Accepted: 20 May 2020;
Published: 07 August 2020.
Edited by:
Frank Preijers, Radboud University Nijmegen Medical Centre, NetherlandsReviewed by:
Alessandro Poggi, San Martino Hospital (IRCCS), ItalyXinhui Wang, Harvard Medical School, United States
Copyright © 2020 Walcher, Kistenmacher, Suo, Kitte, Dluczek, Strauß, Blaudszun, Yevsa, Fricke and Kossatz-Boehlert. This is an open-access article distributed under the terms of the Creative Commons Attribution License (CC BY). The use, distribution or reproduction in other forums is permitted, provided the original author(s) and the copyright owner(s) are credited and that the original publication in this journal is cited, in accordance with accepted academic practice. No use, distribution or reproduction is permitted which does not comply with these terms.
*Correspondence: Tetyana Yevsa, eWV2c2EudGV0eWFuYUBtaC1oYW5ub3Zlci5kZQ==; Uta Kossatz-Boehlert, dXRhLmtvc3NhdHotYm9laGxlcnRAaXppLmZyYXVuaG9mZXIuZGU=
†These authors have contributed equally to this work and share first authorship
‡These authors have contributed equally to this work