- 1Center for Innovative Therapies in Cystic Fibrosis, University of Ferrara, Ferrara, Italy
- 2Department of Life Sciences and Biotechnology, University of Ferrara, Ferrara, Italy
- 3Department of Neurosciences, Biomedicine and Movement, University of Verona, Verona, Italy
- 4Department of Medical Sciences, University of Ferrara, Ferrara, Italy
A hallmark of cystic fibrosis (CF) chronic respiratory disease is an extensive neutrophil infiltrate in the mucosa filling the bronchial lumen, starting early in life for CF infants. The genetic defect of the CF Transmembrane conductance Regulator (CFTR) ion channel promotes dehydration of the airway surface liquid, alters mucus properties, and decreases mucociliary clearance, favoring the onset of recurrent and, ultimately, chronic bacterial infection. Neutrophil infiltrates are unable to clear bacterial infection and, as an adverse effect, contribute to mucosal tissue damage by releasing proteases and reactive oxygen species. Moreover, the rapid cellular turnover of lumenal neutrophils releases nucleic acids that further alter the mucus viscosity. A prominent role in the recruitment of neutrophil in bronchial mucosa is played by CF bronchial epithelial cells carrying the defective CFTR protein and are exposed to whole bacteria and bacterial products, making pharmacological approaches to regulate the exaggerated neutrophil chemotaxis in CF a relevant therapeutic target. Here we revise: (a) the major receptors, kinases, and transcription factors leading to the expression, and release of neutrophil chemokines in bronchial epithelial cells; (b) the role of intracellular calcium homeostasis and, in particular, the calcium crosstalk between endoplasmic reticulum and mitochondria; (c) the epigenetic regulation of the key chemokines; (d) the role of mutant CFTR protein as a co-regulator of chemokines together with the host-pathogen interactions; and (e) different pharmacological strategies to regulate the expression of chemokines in CF bronchial epithelial cells through novel drug discovery and drug repurposing.
Lung Pathology in Cystic Fibrosis Patients: an Early Event Accompanying Whole Life
Autosomal recessive inheritance of mutations of the Cystic Fibrosis Transmembrane Conductance Regulator (CFTR) gene, encoding a chloride and bicarbonate transporting protein, is at the basis of the multiorgan Cystic Fibrosis (CF) disease (1–3). CF lung disease, characterized by chronic bacterial airway infection, neutrophilic inflammation, and dilation of bronchioles obstructed by mucus plugs, is presently the main limitation to the quality and expectancy of the life of CF patients. Although lung pathology and the mechanisms of the disease were prioritized for decades in CF research, what is between the CFTR gene defects and the overt clinical symptoms of the CF patients has still not been completely defined. Consensus has been reached that lung pathology begins in the early months of life for the majority of CF infants, often before the onset of clinical symptoms, as demonstrated by the presence of inflammatory cytokines in the bronchoalveolar lavage fluid of CF infants (4–6) and by the lung histopathology of CF infants who die within weeks or months after birth, showing bronchial lumena filled and plugged by neutrophils (7).
Different hypotheses have been proposed to link the chloride and bicarbonate transport defects of mutant CFTR protein and the onset of airway disease. Consensus on the mechanism can be summarized in that altered CFTR protein reduces the hydration, and possibly the pH, of the airway surface liquid (ASL), thus affecting the rate of the mucociliary clearance, the principal innate mechanism involved in the defense against microbial infection (8). ASL dehydration worsens the mucociliary clearance by reducing mucus fluidity in both ASL and in the submucosal glands of the airway mucosa. The precise mechanism(s) favoring the early recurrent infections with Staphilococcus aureus and Haemophilus influenzae, and the stable chronic bacterial infection with Pseudomonas aeruginosa (P. aeruginosa) that follows in at least 80% of CF teenagers, are not completely understood (9), as ALS dehydration and increased mucus viscosity are considered early predisposing events in CF lung pathophysiology (10, 11).
Hallmarks of the lung pathology of CF patients include defective mucociliary clearance and chronic bacterial infection (especially P. aeruginosa) associated with an exaggerated neutrophil dominated inflammation.
Neutrophils in CF Airway Inflammation: a Double-Edged Sword
Neutrophils are the predominant immune cells infiltrating the airway mucosa and filling the intralumenal space of bronchioles in CF patients (7). Although the recruitment of neutrophils in CF airways' begins early in life and becomes persistent, neutrophils are unable to solve CF bacterial infection. The inefficacy of neutrophils in clearing bacteria prompted a debate on the presence of a neutrophil dysfunction in CF airways, as has been extensively reviewed elsewhere (12, 13). Different in vitro and in vivo studies in human and mice models evidenced that defective CFTR expressed in CF neutrophils, which is essential for chloride transport into phagolysosome and production of HOCl, impairs bacterial killing, implicating a specific disadvantage in microbial clearance in CF airways (14–18). As an indirect confirmation of the role of CFTR in neutrophilic function, VX-770 CFTR potentiator and VRT-325 corrector partially restored the impaired bacterial killing function in neutrophils of patients bearing G551D-CFTR or F508del-CFTR mutations, respectively (19, 20).
Although defective in clearing the chronic respiratory infection of these patients, neutrophils in CF airways are exposed to bacteria and become a source of continuous release of proteases, mainly elastases, which further impair their killing ability upon cleavage of the CXCR1 chemokine receptor (21). The relevance of elastases released from neutrophils has become an intense field of investigation due to its multiple adverse effects in CF lung pathology. It has been directly correlated with the onset of bronchiectasis and the severity of lung disease. The imbalance between proteases and anti-proteases in the CF ASL has prompted researchers to consider neutrophil elastase as a relevant molecular target in this disease (22–31). Its role in CF lung tissue damage has been further increased by its effect on degradation of CFTR protein (32), which can potentially reduce the efficacy of novel CFTR modulators, and by the evidence that its expression is upregulated by the pro-inflammatory cytokine TNF-alpha (TNF-α) and the chemokine interleukin (IL)-8 (or CXCL8) in CF lung (33). Finally, it amplifies the autocrine circuitry of inflammation by potentiating the recruitment of elastase-producing neutrophils by inducing the release of the neutrophilic chemokine IL-8, acting with an autocrine mechanism on CXCR1 and with activation of TLR4 and MyD88-dependent signaling (34–36).
A second critical adverse effect of a huge amount of neutrophils is their contribution to increasing the pro-oxidant milieu of the CF ALS, as has been extensively reviewed elsewhere (37). Among the different sources of pro-oxidants in the CF airway milieu, neutrophils contribute by releasing reactive oxygen species (ROS) by mechanisms known as “frustrated phagocytosis” or as a result of continuous activation, being the neutrophil-derived ROS critical effectors of bronchial epithelial damage (38–41).
As a third critical adverse effect, the presence of a large amount of neutrophils in CF brochial lumena implies the release of abundant DNA on the surface of the mucosa, which further reduces the fluidity of the ASL and worsens the bronchial obstruction (42). For a long time, neutrophil-derived DNA was thought to be the result of the turnover of neutrophils ending in hypoxic necrosis and consequent DNA release (43). More recently, the free DNA in CF airways has been found to be derived from the Neutrophil Extracellular Traps (NETs) released by neutrophils instead of the results of hypoxic necrosis (44). NETs are part of the innate defense armamentarium that block bacteria, viruses, and parasites facilitating the phagocytosis by neutrophils. However, in the environment of CF lung infection and inflammation, the benefits of NETs seems to be overcome by the adverse effect of the release of DNA that further reduces ASL fluidity, which impairs the clearance of toxic enzymes, such as neutrophil elastase and myeloperoxidase, damaging the respiratory tissue. The balance between the pros and cons of NETs in CF lung disease is therefore critical (45, 46). A crucial issue in CF lung disease is that neutrophils in the bronchial lumena of CF patients are unable to clear the bacterial infection and are co-responsible, together with bacteria, for the tissue damage, since neutrophils release proteases and ROS and further affect the rheology of CF ASL with abundant DNA. A graphical summary is presented in Figure 1.
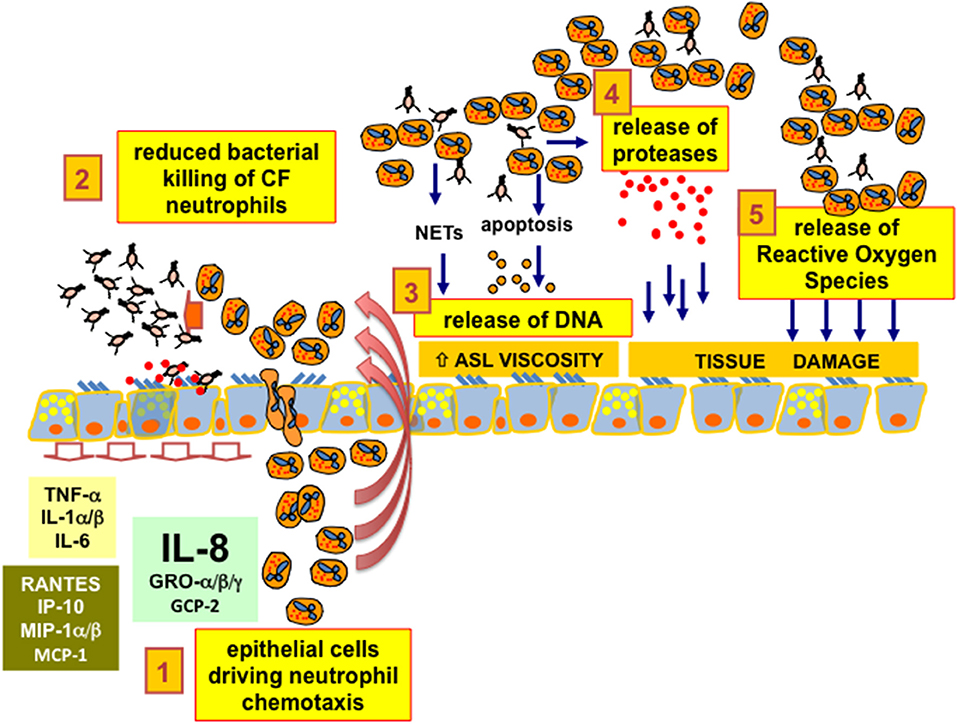
Figure 1. CF bronchial epithelial cells and neutrophil chemotaxis. 1. epithelial cells driving neutrophil chemotaxis: bronchial epithelial cells drive the chemotaxis of neutrophils inside the lumen of bronchi and bronchioles of CF patients, mainly secreting the chemokine IL-8 (CXCL8) expressed upon interaction with bacteria with ASGM1R and TLR2/5 receptors exposed on the apical membrane. 2. reduced bacterial killing of CF neutrophils: CF neutrophils present different degrees of defective killing of the bacteria in the process of phagocytosis, being unable to clear completely the recurrent and, later on, chronic bacterial infection in the airways. 3. release of DNA: CF neutrophils are continuously stimulated in releasing Neutrophil Extracellular Traps. Despite the expected increase in efficiency of bacterial clearing, DNA from NETs increases the viscosity of the Airway Surface Liquid (ASL), further worsening the beat of cilia and the effectiveness of the CF mucociliary clearance. Moreover, CF neutrophils further release DNA as a consequence of apoptosis or necrosis due to the hypoxic environment resulting from mucus plugging of the conductive airways. 4. release of proteases: CF neutrophils exposed to bacteria or bacteria-derived components are prone to exocytosis of granules containing a wide armamentarium of proteases that are usually utilized by neutrophils to disrupt the tissues to allow their migration. In particular, an abundant amount of elastase has been found in CF lungs and its concentration has been related to CF lung disease progression and severity. 5. release of Reactive Oxygen Species: the unbalanced pro-oxidant milieu of the CF ASL due to excessive ROS derives mainly from “frustrated phagocytosis” of CF neutrophils and contributes together with proteases to a neutrophil-dependent airway tissue damage, a clear side effect dependent on the CFTR defect and worsened by an exaggerated neutrophil chemotaxis in CF bronchial lumen.
Chemokines Recruiting Neutrophils and the Role of Bronchial Epithelial Cells
Elevated concentrations of cytokines have been found early in life in the bronchoalveolar lavage fluid of CF infants, even in the absence of an overt bacterial infection (4–6). Different mechanisms have been proposed to explain the early onset of sterile inflammation in CF lungs, including the role of airway surface mucus and hypoxia (47–49).
Among the soluble mediators of inflammation detected in CF bronchoalveolar lavage fluid, the most potent chemokines recruiting neutrophils have attracted particular interest, both in terms of pathophysiology and therapeutic perspectives, namely the complement system-derived C5a, the leukotriene B4 (LTB4), and the chemokine Interleukin(IL)-8.
C5a activated complement component receptor (C5aR) expressed on neutrophils was found to be critical in the defense against P. aeruginosa infection since knock-out mice deficient of C5aR were able to recruit neutrophils but succumbed to pneumonia because of the killing defect (50). Although it was earlier speculated that the C5aR decoy molecule C5L2 could be beneficial in reducing excessive inflammation in several lung diseases, including CF (51), it was later concluded that inactivation of C5aR by cleavage mediated by proteases released from CF neutrophils was at least partly responsible for the reduced killing and clearance of P. aeruginosa in CF lungs (52). Due to this critical role of C5aR in host defense, although elevated concentrations of C5a in CF airway fluids have been directly correlated with disease severity, very little effort has been invested in inhibiting the C5a-C5aR axis (53, 54).
LTB4 is released from neutrophils and macrophages in response to different stimuli, and in turn recruits and activates neutrophils (55). LTB4 has been found at elevated concentrations in different CF respiratory fluids (56–58). Because of its potent neutrophil chemotactic effect, novel drugs, or drug repositioning to inhibit either LTB4 or its receptor have been proposed in CF to reduce inflammatory-dependent tissue damage (59). Particularly relevant was the experience with BIIL 284 BS, a drug acting as an LTB4 receptor antagonist, which was tested in pre-clinical and clinical trials (60, 61). Unfortunately, the clinical trial resulted in major adverse events, as it was apparent that the drug, while effectively reducing the inflammatory response, was untowardly increasing P. aeruginosa bacterial load (61, 62), providing a first relevant alert on the delicate balance between the reduction of excessive inflammation by over-inhibiting neutrophil chemotaxis and the mandatory need of preserving a sufficient immune defense. To tackle the crucial issue of anticipating in pre-clinical assays the effect of potential anti-inflammatory molecules modulating neutrophil chemotaxis, an interesting CF in vitro model has been set-up, able to simulate closely the transepithelial neutrophil migration and the effect of candidate drugs (63). In spite of inhibiting the LTB4 receptor, such as in the previous unsuccessful experience with BIIL 284 BS (61, 62), acebilistat, a recent drug modulating LTB4 expression, has positively passed pre-clinical assays (63) and is now in clinical trials (64), keeping open the possibility of reducing excessive CF lung inflammation by modulating neutrophil chemotaxis targeting the leukotriene-LTB4 axis.
IL-8/CXCL8 is possibly the neutrophilic chemokine most extensively studied in CF lung pathophysiology. Different cells of the airway tract are known to contribute to the release IL-8; among these, bronchial epithelial cells have been highlighted as a relevant source. To dissect the specific contribution of bronchial epithelial cells in the expression of IL-8 and of several other soluble mediators of inflammation, different in vitro experimental models with immortalized cell lines or primary cell cultures have been tested and are currently utilized to investigate molecular mechanisms or novel anti-inflammatory molecules. Key pro-inflammatory challenges able to elicit inflammatory mediators in bronchial epithelial cells are different living bacteria (S. aureus, P. aeruginosa), heat-inactivated dead bacteria (Heat-Killed P. aeruginosa), flagella-defective or pili-defective recombinant strains of P. aeruginosa, single bacterial components (e.g., flagellin), P. aeruginosa clinical isolates and bacterial products from patients' airway specimens (e.g., Supernatant of Mucopurulent Material), different pro-inflammatory cytokines (e.g., TNF-α and IL-1), and oxidants such as hydrogen peroxide (65–72). Under these stimuli in vitro, bronchial epithelial cells upregulate the basal expression of many pro-inflammatory mediators, such as the cytokines (e.g., TNF-α, IL-1α/β, IL-6), chemokines attracting lympho-monocytes (e.g., IP-10, RANTES, MCP-1, MIP-1α/β), and, as anticipated, IL-8 and other chemokines attracting neutrophils (Gro α/β/γ, GCP-2) (67, 68, 70, 71, 73–81). Among these soluble mediators of inflammation, the neutrophilic chemokine IL-8 (CXCL8) is most strikingly expressed up to two orders of magnitude above the basal release (81). Wide consensus has been reached so far on the role of bronchial epithelial cells as relevant producers of the potent chemokine IL-8, which in turn forwards a strong recruiting soluble signal to neutrophils to reach the lumen of bronchi and bronchioles in the CF mucosa.
Host-Pathogen Interactions and Intracellular Signaling Modulating IL-8/CXCL8 Expression
A growing series of evidence based on longitudinal clinical investigations of CF patients is building a strong consensus that the inflammatory process could be even more deleterious to CF lung structure and function than the bacterial infection by itself (83) Sterile inflammation in CF lungs has been evidenced based on the presence of elevated concentrations of cytokines in the bronchoalveolar lavage fluid of CF infants of a few months of age (4–6). Airway surface mucus plugging and hypoxia have been proposed to explain the onset of sterile inflammation in CF lungs (47–49). The possible direct contribution of intracellular CFTR protein defects has been proposed (as described later in paragraph 5), together with mechanisms more downstream than the CFTR-dependent ion transport alterations, such as mucus plugging (84). Although inflammation in the absence of detectable bacterial infection has been demonstrated as a likely initiating event, the whole inflammatory process in CF lung is undoubtedly amplified by the occurrence of polybacterial infection. Host-pathogen interactions between bacteria and bronchial epithelial cells in CF have been most extensively studied for P. aeruginosa, the pathogen that colonizes CF airways of almost all CF patients. P. aeruginosa in a planktonic state interacts through the pili with Asialo-GM1 receptor (AGM1R) on the apical membrane of airway epithelial cells (65). Flagellum-derived flagellin protein also binds to AGM1R, together with Toll-like receptors (TLR) 2 and 5 (85). Flagellin in particular has been found to elicit a strong intracellular pro-inflammatory signaling, as shown by the single purified protein and by recombinant lab strains of P. aeruginosa, in different converging studies led by Prince et al. (68, 72, 86, 87). MyD88-dependent signaling downstream TLR2 and TLR5, elicited by P. aeruginosa, activates Mitogen-Activated Protein (MAP) Kinases such as MAPK ERK1/2 and MAPK p38, together with ribosomal S6 kinase (RSK)1/2 and heat shock protein (HSP) 27, which are directly involved in inducing the expression of the neutrophilic chemokine IL-8 (88–90). Besides the activation of MyD88-dependent signaling, P. aeruginosa is known to also potentiate the expression of IL-8 by a nucleotide-purinergic receptors loop. Interaction of the bacteria with ASGM1R and TLR5 promotes sustained release of ATP as a classical “danger signal” from the apical membrane (85, 91), interacting with purinergic receptors P2Y2R (92). This activates an intracellular calcium signaling (see paragraph 6), in which phospholipase C beta 3 (PLCB3) plays a key role (78, 93).
For transcription factors (TFs) involved in chemokine expression, the promoter elements of IL-8 gene have been widely studied (89, 94, 95). The mapping of the transcription machinery of the IL-8 gene in human bronchial epithelial cells infected with P. aeruginosa was studied not only with the aim of understanding the molecular regulation of IL-8 transcription, but also to propose novel anti-inflammatory approaches and to identify potential pharmacological targets. This issue was addressed by investigating the role of TFs on the transcription of the IL-8 gene in human bronchial epithelial cells. Functional assays were based on the transfection of TF decoy oligodeoxynucleotides, designed to interfere with the interaction of the transcription factors nuclear factor-κB (NF-κB), activating protein (AP-1), CAAT/enhancer-binding protein β (C/EBPβ, also known as NF-IL-6), C/EBP homologous protein (CHOP), and cAMP response element binding protein (CREB) with the corresponding consensus sequences identified in the IL-8 promoter. The treatment of target cells with these decoy oligonucleotides reduced the P. aeruginosa-dependent transcription of IL-8, suggesting their participation in the transcriptional machinery (89, 94). These conclusions have been recently confirmed and reviewed (95). On the contrary, IL-8 gene expression is repressed by a combination of molecular events that includes: (a) deacetylation of histones, (b) octamer-1 (Oct-1) binding, and (c) active repression by NF-κB repressing factor (NRF). Histone deacetylase-1 (HDAC-1) activity is involved in IL-8 transcription inhibition, as demonstrated by the fact that HDAC1 inhibition derepresses the expression of IL-8, which involves the recruitment of CREB binding protein (CBP)/p300 to the IL-8 promoter, resulting in hyperacetylation of histones and chromatin remodeling, thus counteracting the repression (96, 97). In terms of Oct-1 activity, it has been demonstrated that Oct-1 (the IL-8 repressor) and CCAAT/enhancer-binding protein (C/EBP) (an IL-8 activator) bind to overlapping elements within the IL-8 promoter. The role of Oct-1 as a transcriptional repressor is sustained by experimental evidence that replacing the Oct-1 repressor with C/EBP induces transcription at the IL-8 promoter (98). Similarly, binding of NRF to a negative regulatory element (NRE) in the IL-8 gene promoter (which incompletely overlaps with the NF-κB response element) also represses IL-8 transcription (99). Interestingly, the transcription factors that have been suggested to regulate IL-8 are also involved in regulating the expression of other pro-inflammatory genes, such as GRO-γ, the intercellular adhesion molecule (ICAM)-1, and the cytokines IL-1β and IL-6 (94).
The possible application of transcription factor(s) targeting (for instance, using decoy oligonucleotides) might be a potential therapeutic intervention, especially in the case delivery issues are solved. In this respect, two recently reported studies have focused on nanomaterial-based delivery systems for overcoming limitations associated with clinical applications of decoy oligonucleotides targeting pro-inflammatory transcription factors (such as those targeting NF-kB) (100, 101).
The Epigenetic Regulation of the Key Chemokines
The epigenetic regulation of key chemokines in CF occurs at the level of: (a) histone acetyltransferase (HAT)/HDAC balance, (b) histone and DNA methylation, and (c) miRNA-dependent post-transcriptional regulation. It has been recently reported that the transcriptional regulation of IL-8 and other pro-inflammatory genes involves chromatin remodeling through histone acetylation. Interestingly, there is a possible regulatory loop between IL-8 gene transcription and CFTR. In fact, NF-κB facilitates histone acetylation of IL-8 and other pro-inflammatory gene promoters and the histone acetyltransferase (HAT)/HDAC balance is sensitive to CFTR function. This conclusion is supported by the observation that cells with a reduced or absent CFTR function have a decreased HDAC2 protein, resulting in hyperacetylation of the IL-8 promoter and increased IL-8 transcription. In agreement with (a), reduced HDAC2 and HDAC2 activity is observed in cells deficient in CFTR and (b) suppression of HDAC2 expression with HDAC2 shRNA (short hairpin RNA) resulted in enhanced IL-8 expression and promoter acetylation similar to CFTR-deficient cells (102). In conclusion, there is an intrinsic alteration in the HAT/HDAC balance in cells lacking CFTR function in vitro and in native CF tissue. This mechanism provides an explanation for the apparent dysregulation of inflammatory mediators seen in the CF airway, as reduced histone deacetylation would potentially influence many genes. IL-8 hypersecretion in CF airway epithelial cells is also caused by the abnormal epigenetic regulation of IL-8 gene involving histone methylation. Under basal conditions, CF cells had increased bromodomain (Brd)3 and Brd4 recruitment and enhanced NF-κB and C/EBPβ binding to the IL-8 promoter compared to non-CF cells due to trimethylation of histone H3 at lysine 4 (H3K4me3) and DNA hypomethylation at CpG6. IL-1β increased NF-κB, C/EBPβ, and Brd4 binding. Furthermore, inhibitors of bromodomain and extra-terminal domain family (BET) proteins reduced IL-8 production in CF cells, suggesting a therapeutic target for the BET pathway (103). Regarding microRNA (miRNA) involved in controlling IL-8 production in the CF lung, three recent studies have determined how miRNAs that are aberrantly expressed in the CF airways may post-transcriptionally regulate IL-8 expression. The first report was focused on miR-155, a miRNA that is highly expressed in CF lung. Bhattacharyya and Coll found that expression of miR-155 was elevated in CF IB3-1 lung epithelial cells in culture, compared with control IB3-1/S9 cells. In addition, clinical evidence indicated that miR-155 was also highly expressed in CF lung epithelial cells and circulating CF neutrophils from CF patients. High levels of miR-155 specifically reduced levels of SHIP1, thereby promoting PI3K/Akt activation and contributing to the pro-inflammatory expression of IL-8 in CF lung epithelial cells (104). The other two studies on this topic have investigated miRNAs that are decreased in the CF lung that directly target IL-8 mRNA. Fabbri et al. identified miR-93 as a miRNA that is decreased in IB3-1 and Cufi-1 cells infected with P. aeruginosa. The possible involvement of miR-93 in IL-8 gene regulation was validated using three luciferase vectors, including one carrying the complete 3'-UTR region of the IL-8 mRNA and one carrying the same region with a mutated miR-93 site. Specifically, the results obtained indicate that, in addition to NF-κB-dependent up-regulation of IL-8 gene transcription, IL-8 protein expression is post-transcriptionally regulated by interactions of the IL-8 mRNA with the inhibitory miR-93 (105). The involvement of microRNAs in IL-8 is also supported by the study of Oglesby et al. (106), who measured the expression and function of miRNAs decreased in the CF lung. MicroRNA miR-17 was identified as a miRNA that regulates IL-8 and its expression was decreased in adult CF bronchial brushings and bronchial epithelial cells chronically stimulated with Pseudomonas-conditioned medium (106). Another microRNA involved in inflammation is miR-636, as recently demonstrated by Bardin et al. (107). By analyzing miRNAs in human primary air-liquid interface cell cultures, overexpression of miR-636 in CF patients compared to non-CF controls was shown. Functional studies demonstrated that miR-636 directly interacts with IL1R1 and RANK (two pro-inflammatory cytokine receptors), and IKBKB (which encodes IKKβ, a major protein in the NF-κB pathway). A summary of miRNA regulation is depicted in Figure 2.
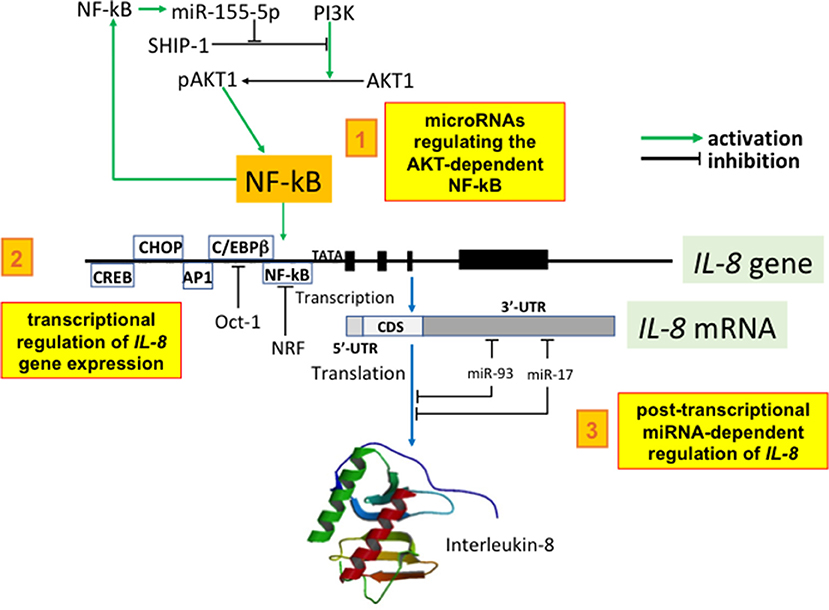
Figure 2. Selected examples of the miRNA network regulating IL-8. 1. microRNAs regulating the AKT-dependent NF-kB: miR-155-5p expression is potentiated by NF-kB and is elevated in CF lung epithelial cells and circulating CF neutrophils biopsied from CF patients. High levels of miR-155 specifically reduce the levels of SHIP1, thereby promoting PI3K/Akt activation. Phospho-Akt levels are therefore elevated in CF lung epithelial cells and can be specifically lowered by either antagomir-155 or elevated expression of SHIP1. Elevated miR-155-5p contributes to the pro-inflammatory expression of IL-8 in CF lung epithelial cells by lowering SHIP1 expression and thereby activating the PI3K/Akt signaling pathway (104). 2. transcriptional regulation of IL-8 gene expression: IL-8 promoter is under the control of transcription factors enhancing IL-8 mRNA production (examples are NF-κB, AP-1, C/EBPβ, CHOP, and CREB); furthermore, IL-8 gene expression is repressed by Oct-1 and by NF-κB repressing factor (NRF). All these transcription factors are regulated by microRNAs. 3. post-transcriptional miRNA-dependent regulation of IL-8: Fabbri et al. identified miR-93 as a miRNA that is decreased in cystic fibrosis IB3-1 and Cufi-1 cells infected with P. aeruginosa. The possible involvement of miR-93 in IL-8 gene regulation was validated using luciferase vectors and the results obtained indicate that IL-8 protein expression is post-transcriptionally regulated by interactions of the IL-8 mRNA with the inhibitory miR-93 (105). The involvement of microRNAs in IL-8 is also supported by the study of Oglesby et al. (106) who identified miR-17 as a miRNA regulating IL-8. Interestingly, its expression was decreased in adult CF bronchial brushings and bronchial epithelial cells chronically stimulated with Pseudomonas-conditioned medium (106). 5′-UTR, 3′-UTR: 5′ and 3′ untranslated region of the IL-8 mRNA; CDS, coding sequences.
CFTR Protein Defects Cooperate to Pro-inflammatory Intracellular Signaling
The origin of this abnormal inflammatory response in CF continues to be debated, with researchers unsure whether it is initiated by exogenous stimuli, such as persistent microbial infection, or by intrinsic deficiency of CFTR function, with alterations in signal transduction, or both. Dysregulation of the airway innate immune system is associated with CF, being both airway epithelial cells and immune cells susceptible to intrinsic CFTR-associated alterations in signal transduction.
No differences in TLRs expression have been observed between human CF and non-CF airway epithelial cell lines. However, increased expression of TLR2 and TLR5 on the apical membrane of the polarized human CF airway epithelial cells and in CFTR-knockout vs. WT mice were found (108, 109). These different cellular redistributions of TLRs render the CF airway epithelial cells more susceptible to host-pathogen interactions between bacterial constituents (pili and flagellin) and the receptors involved in the transduction of “danger signal” at apical membrane, favoring the release of cytokines (as described before in section host-pathogen interactions and intracellular signaling modulating IL-8/CXCL8 expression).
An intrinsic defect associated with CFTR deficiency is the susceptibility of CF airway cells to accumulate ROS, particularly during pathogen infection. Evidence shows increased oxidative stress in CF (37, 110–116). Although several studies suggest that the oxidative stress in CF is a direct consequence of mitochondrial dysfunction due to perturbed CFTR signaling, others suggest that the accumulation of ROS in CF depends on there being a reduced antioxidant capacity (113). Low mitochondrial reduced glutathione (mtGSH) levels were found in CF patient-derived tracheal cells and in CFTR-knockout mice (117, 118). Indeed, in CF patient-derived pancreatic and tracheal cells a reduction of protein expression of Cu/Zn-superoxide dismutase (SOD1) and Mn-SOD (SOD2) and a reduction in the activity of extracellular SOD (119) has been observed. The oxidative stress in CF lung may: (I) affect autophagy, compromising the expression of CFTR channel (120, 121); (II) induce mtDNA oxidation and damage, triggering inflammasome activation, and/or altering the OXPHOS activity, that in turn produces additional ROS (122, 123); and (III) destroy lung tissue, affecting the cell function and/or exacerbating the inflammatory response (37).
An inherent defective CFTR channel leads to transglutaminase (TG2) upregulation, resulting in defective autophagy with consequent accumulation of aggresomes and ROS (121). Lack of autophagy in macrophages and in airway epithelial cells result in a reduced bacterial clearance and in the accumulation of dysfunctional mitochondria, which in turn promotes secretion of pro-inflammatory cytokines, indicating that autophagy may regulate the inflammation responses by suppressing the secretion of immune mediators (124–126). In fact, the rescue of dysfunctional autophagy in CF, mediated by autophagy inducers such as MTOR inhibitor (rapamycin), TG2 inhibitor (cistamine), and/or modulators of Ca2+-dependent signaling (KB-R7943), attenuated the hyperinflammation in CF lung, improving the CFTR transport to PM and reducing ROS production and cytokine release in macrophages and in primary CF airway cells in vitro and in CF mouse models in vivo (127–132).
The hyperinflammation of the CF airway is sustained by the accumulation of dysfunctional mitochondria, as an indirect consequence of perturbed CFTR signaling, responsible for ROS production and increased inflammasome-dependent IL-1β release. Mitochondrial defects associated with an abnormal oxidative stress and inflammatory response have been found in CF. The first evidence was shown in 1979, demonstrating that mitochondrial Ca2+ uptake and oxygen consumption were altered in mitochondria isolated from CF patient-derived fibroblast (133). In the same year, the mitochondrial NADH dehydrogenase (complex-1) was found altered in CF skin fibroblasts (134), while in 1981 a deficiency of 6-phosphate dehydrogenase in CF patients was found (135). Consistent with the first observations, in CF patient-derived tracheal cells a decreased mitochondrial NADH dehydrogenase activity and mitochondrial membrane potential (Δψ) were measured, due to the down-regulation of the mt-ND4 gene that codifies for a subunit of the complex-1 essential for its assembly and activity (136). This alteration was rescued through the reintroduction of CFTR-wt, also indicating that mtGSH depletion in CF is responsible for the altered complex-1 activity (118). Recently, it has been observed that the CFTR corrector agent VX-809 and 4,6,4'-trimethylangelicin (TMA) treatment lead to partial restoration of the mitochondrial failure in CF airway cell lines, producing an improvement in complex-1 activity, Δψ generation, ANT-dependent ADP/ATP exchange, and membrane lipid peroxidation (137). These data indicate that the restoration of mitochondrial physiology is linked to the mitigation of the inflammatory response, suggesting that the mitochondrial defects found in CF airway cells contributes to the susceptibility of CF cells to bacterial infection, influencing the innate immune response.
Several pieces of evidence have shown how the hyperinflammation observed in CF lung is sustained by cell defects associated with CFTR deficiency intrinsic to epithelial, but also in inflammatory, cells. In CF patients, the differentiation of T lymphocytes to Th17 phenotype is increased (138), while monocyte-derived macrophages do not respond to IL-13/IL-4 and fail to polarize into M2 while the polarization to the M1 phenotype was unaffected (139). CF macrophages serve as a replicative niche for bacteria to avoid host defenses (140), present deficits in bacterial killing (141, 142), and produce excess cytokines (143). In synthesis, although the initiating event of CF lung inflammation between infection-derived exogenous and CFTR-related endogenous components remains a “chicken and egg” debate still without a final consensus, different converging evidence supports the hypothesis that CFTR-specific signal transduction alterations amplify the extent of the response.
In this context, analysis at a single-cell level might be very informative. For instance, Mould et al. (144) investigated inflammatory macrophage heterogeneity during acute lung inflammation in mice and performed single cell RNA sequencing of macrophages isolated from the airspaces during peak inflammation and resolution of inflammation. They found two transcriptionally distinct subdivisions of alveolar macrophages based on proliferative capacity and inflammatory programing. Of course, overcoming technical obstacles (due, for instance, to debris, and apoptotic cells) rendering difficult isolation of individual cells for single cell analyses is a key issue (145). Finally, on-a-chip devices are expected to bring key information at single-cell levels on hyperinflammation in pulmonary diseases, including CF (146–148). As far this key issue is concerned, starting from the notion that cell-to-cell variability in chemokine/cytokine secretion is largely unknown, Ramji et al. developed and validated a microfluidic device to integrate live-cell imaging of fluorescent reporter proteins with a single-cell assay of protein secretion (146). This device was used to image transcription factor dynamics in macrophages in response to LPS, followed by quantification of secretion of TNF, CCL2, CCL3, and CCL5.
Intracellular Calcium Homeostasis in the Regulation of the Expression of Chemokines
Ca2+ homeostasis is a pivotal element in regulating the immunological and physical barriers of the airway epithelium in CF. Normally, the exposure to common respiratory bacterial pathogens, such as P. aeruginosa, originates in a cytosolic Ca2+ transient (about 100 nM) in airway epithelial cell lines necessary to initiate the inflammatory response, inducing the expression, and secretion of pro-inflammatory cytokines (149). A defective CFTR channel leads to the deregulation of Ca2+ homeostasis in CF cells, which is detrimental for lung inflammation. Ca2+ signaling dysregulations were observed in several human CF airway epithelial cell lines, where the intracellular Ca2+ concentration is increased compared to non-CF cells (150) due to: (I) intrinsic defects associated with CFTR deficiency, (II) chronic exposure to bacterial infection, and (III) persistent stimulation by pro-inflammatory mediators.
Intrinsic Defects Associated to CFTR Deficiency
In 1961, Donnell et al. published the first evidence of alterations of Ca2+ homeostasis in CF patients (151), which was then confirmed by Feigal et al., through direct measurement of the increased intracellular Ca2+ concentration in fibroblasts derived from CF patients (152, 153) and by Cabrini and De Togni as increased cytosolic Ca2+ concentration in CF neutrophils (154). In 1982, an increased mitochondrial Ca2+ uptake was observed in CF skin fibroblast, due to altered respiratory system activity (155). In 2009, Antigny et al. measured a decreased mitochondrial Ca2+ uptake in F508delCFTR airway epithelial cell lines as a consequence of depolarized and fragmented mitochondria (156). The debate is presently lessened because it has been demonstrated that a functional CFTR channel reduces the basal intracellular Ca2+ concentration in human airway epithelial cell lines and donor-derived primary airway epithelial cells, influencing the mitochondrial Ca2+ signals evoked by physiological and pathological stimuli (132). This abnormal intracellular Ca2+ increment in CF epithelial airway cells is in part justified by the reduced activity of Plasma Membrane (PM) Ca2+ ATPase (PMCA), which limits the Ca2+ efflux through the PM, and increased SERCA activity, which favors the endoplasmic reticulum (ER) Ca2+ accumulation (132). This increment in intracellular Ca2+ concentration in human epithelial airway cells is normalized by the administration of the corrector agent, VX809 (157). Several other pieces of evidence suggest that the increased intracellular Ca2+ concentration observed in CF airway cells depends on multifactorial aspects associated with defective CFTR, involving many Ca2+ channels expressed in the PM, including: (I) the Transient Receptor Potential Canonical channel 6 (TRPC6), normally expressed in human primary CF epithelial cells. Its Ca2+ influx capacity is enhanced in F508delCFTR and G551D-CFTR cells (158, 159); (II) the Store Operated Ca2+ Entry (SOCE) resulted in significantly increased CF airway cell lines and primary cells, due to an enhanced Orai1 channel insertion to PM, with consequent exacerbation of IL-8 secretion (160); and (III) the TRP channel TRPA1, expressed in bronchial columnar epithelial cells. Its direct activation by P. aeruginosa increases Ca2+ entry, mediating the release of cytokines such as IL-8, IL-1β, and TNF-α (81).
Chronic Exposure to Bacterial Infection and Persistent Stimulation by Pro-inflammatory Mediators
The chronic infection of CF airways amplifies the altered intracellular Ca2+ homeostasis of CF epithelial cells, predisposing the airway cells to a hyperinflammatory profile, which contributes to producing an excess of cytokines. Bacterial constituents and pro-inflammatory mediators cooperate, inducing an abnormal intracellular Ca2+ signaling in CF airway epithelia due to increased activation of apical G protein-coupled receptors (GPCRs) and a sustained ER Ca2+-release (161). The higher intracellular Ca2+ concentration in CF cells contributes to a greater and more prolonged NF-κB activation with consequent effects on the expression and release of pro-inflammatory cytokines, such as IL-8 and IL-1β (82, 161). The persistent NF-κB activation in human CF airway cells is the consequence of the synergistic effects of bacterial components, such as flagellin, where flagellin interacting with asialoGM1 receptor favors the release of ATP from CF airway cell lines, which mediates purinergic receptors and activates downstream intracellular Ca2+ signaling that synergizes with the TLR5-dependent signaling to activate NF-κB (see section host-pathogen interactions and intracellular signaling modulating IL-8/CXCL8 expression) (92). The release of nucleotides from bronchial epithelial cells targeting P2Y2 purinergic receptors has been proposed to intervene on different aspects of CFTR regulation and lung pathophysiology (162–165). Bacterial constituents, pili and flagellin, interact with TLRs and TLR-associated glycolipid in airway cells (85, 166). In particular, TLR2 or asialoGM1 linked to TLR2 express both on the apical surface of airway cells and recognize bacterial constituents to induce the pro-inflammatory transcription of CXCL8 or MUC-2 gene via NF-κB activation, through the recruitment of PI3K and phospholipase C gamma (PLCγ), which in turn stimulate the release of Ca2+ through Inositol Triphosphate Receptors (IP3R) channels (167, 168). The generation of cytosolic Ca2+ transients activates classical Protein Kinase C (PKC) α and β isoforms, which through a phosphorylation cascade mediate the activation of NF-κB (169). In a similar molecular pathway, PLC beta 3 (PLCB3) also plays a relevant role in triggering cytosolic Ca2+ transients induced by P. aeruginosa, regulating the activation of PKCα and PKCβ to induce an NF-κB-dependent transcription of CXCL8 gene in human airway epithelial cell lines and in patient-derived primary cells (78). β-sitosterol (BSS) was used to inhibit the active form of PKCs involved in the transduction of P. aeruginosa-dependent pro-inflammatory Ca2+-dependent signaling in CF patient-derived airway epithelial cells, leading to a significant reduction in expression of IL-8, growth-related oncogene (GRO)-α, and GRO-β (170). The role of PLCB3 in amplifying the expression and release of IL-8 during pathogen infection, through the regulation of intracellular Ca2+ transients, is associated with the severity and progression of CF lung disease. Single Nucleotide Polymorphisms (SNP) genetic study with the progression of CF lung disease severity, identify from a panel of 135 genes of immune response the association of c.2534C>T (p.S845L) variant of PLCB3 with a mild progression of pulmonary disease in CF (93). PLCB3-S845L results in a loss-of-function variant, where defective intracellular Ca2+ redistribution and PKCs' activation limited the IL-8, IL-1β, and MUC5 expression in CF patient-derived airway epithelial cells exposed to P. aeruginosa or CF patient-derived mucopurulent material. The IP3R-mediated ER Ca2+-release is significantly augmented in CF epithelial cell lines (171), a consequence also of ER Ca2+ store expansion observed in CF cells (150). The ER expansion is not dependent on ER retention of misfolded CFTR, but reflects an airway epithelial response acquired following persistent bacterial infection, resulting in ER unfolded protein response (UPR) activation mediated by the IRE1/XBP-1 pathway and in a larger intracellular Ca2+ mobilization in response to abnormal GPCRs activation (172).
The sustained ER Ca2+-release in CF airway cells conditions the mitochondria to a direct involvement in the pro-inflammatory response. CF airway cell lines and CF patient-derived airway primary cells are prone to P. aeruginosa-dependent mitochondrial perturbations, in which the mitochondrial Ca2+ uniporter (MCU) is a signal-integrating organelle that mediates mitochondrial ROS-dependent NLRP3 inflammasome activation and recruitment of both NLRP3 and NLRC4 inflammasome (132). The degree and quality of the inflammatory response in CF airway cells is also sustained by P. aeruginosa-dependent mitochondrial perturbations, initiated by flagellin, such as mitochondrial membrane potential loss, ROS production, and mitochondrial fragmentation (132). Rimessi et al. have characterized the role of mitochondria as drivers of the P. aeruginosa-triggered inflammatory exacerbation in CF airway cells, demonstrating that mitochondrial Ca2+ signaling plays a critical role in inflammasome NLRP3 recruitment and inflammasome-dependent IL-1β and IL-18 release in CF airway cell lines and in CF patient-derived airway primary cells (132). By modulating the MCU-dependent mitochondrial Ca2+-uptake, genetically or mediating pharmacological inhibition with KB-R7943, it is possible to control the pathogen-dependent mitochondrial dysfunction preventing the integration of pro-inflammatory signals from mitochondria into CF patient-derived airway primary cells and in vivo mouse models (131, 132).
Although whether the Ca2+-dependent activation of chloride channels in CF bronchial epithelial cells could partially vary the defects of CFTR ion transport is presently under scrutiny (173), the results recalled above support the concept that the up-regulation of intracellular Ca2+ signaling is a key amplifier of the inflammatory response and lung pathogenesis in CF, which opens the issue of new potential molecular therapeutic targets.
Targeting Neutrophil Chemotaxis in CF: Novel Molecules and Drug Repurposing
Preliminary observations in rat lung models (59) suggested the repurposing of ibuprofen, a non-steroideal anti-inflammatory drug used in conditions like osteoarthritis, rheumatoid arthritis, juvenile idiopathic arthritis, and acutely painful musculoskeletal conditions, to clinical use for CF patients (174, 175). Long-term application of this drug, as reported by a Cochrane analysis, has proved the concept that strategies to modulate lung inflammation can be beneficial for people with CF (176). As ibuprofen inhibits prostaglandin synthesis (177), a very broad anti-inflammatory mechanism that is not closely specific to the pathophysiology of CF lung inflammation, innovative approaches to target the adverse effects produced by the huge amount of neutrophils in the CF conductive airways have been recently tested. Some of these approaches have been launched upon the knowledge of the specificity of CF lung inflammation, whereas others were just pure empirical testing. The first attempts were focused on antagonizing neutrophil proteases elastase, one of the main deleterious effect of neutrophil inflammation in CF (28, 29, 178–186). Different elastase inhibitors have also been recently tested in clinical trial, with promising results in terms of safety and tolerability (187, 188), maintaining the high levels of interest in the rationale of targeting neutrophil elastase in CF lung inflammation (186).
Early signaling evoked by bacteria has been tested by inhibition of TLR2 or by inducing extracellular calcium entry through calcium ionophores (81, 189). More downstream the production of IL-8, different antagonists of its receptors that block its action on cell targets have been challenged (190–192). More recently, different molecular approaches targeting the intracellular signaling in bronchial epithelial cells have been developed. In consideration of the key role of NF-κB in the transcriptional regulation of IL-8 and other pro-inflammatory genes, several studies have been focused on pharmacological alteration of NF-κB activity. The transcription factor (TF) decoy strategy was applied by Bezzerri et al., using TF oligodeoxynucleotides (ODNs) to NF-κB able to inhibit transcription of IL-8 in bronchial cells (89). The TF decoy approach was based on the intracellular delivery of double-stranded ODNs causing inhibition of the binding of TF-related proteins (as determined in vitro using EMSA assays) to the different consensus sequences in the promoter of specific genes. When CF cells were transfected with double-stranded TF “decoy” ODNs, mimicking different NF-κB consensus sequences, partial inhibition of P. aeruginosa-dependent transcription of IL-8 was obtained. In addition, other NF-κB regulated genes were inhibited, such as GRO-gamma and IL-6. In order to demonstrate that TFD against NF-κB interferes with the NF-κB pathway, Finotti et al. demonstrated mediating chromatin immunoprecipitation (ChIP) treatment with TFD oligodeoxyribonucleotides of IB3-1 cells infected with P. aeruginosa leads to a decreased occupancy of the IL-8 gene promoter by NF-κB factors (193). Further studies were focused on the development of more stable therapeutic molecules and on the delivery strategy for TFD molecules. Among stable ODN analogs, peptide nucleic acids (PNAs)-based agents were found to be promising for CF. In this respect, PNA-DNA-PNA (PDP) chimeras are molecules of great interest from several points of view: (a) they can be complexed with liposomes and microspheres; (b) they are resistant to DNases, serum, and cytoplasmic extracts; and (c) they are potent decoy molecules (194, 195). By using electrophoretic mobility shift assay and RT-PCR analysis, it was demonstrated that: (a) the effects of PDP/PDP NF-κB decoy chimera on the accumulation of pro-inflammatory mRNAs in P. aeruginosa-infected IB3-1 cells in particular; (b) the PDP/PDP chimera is a strong inhibitor of IL-8 gene expression; and (c) the effect of PDP/PDP chimeras, unlike those of ODN-based decoys, are observed even in the absence of protection with lipofectamine (193–196). In another study, NF-κB decoys were employed with the hypothesis that they may limit lung inflammation in CF. In the study by De Stefano et al. (197), the effects of decoy ODN targeting NF-κB and delivered through biodegradable and respirable poly(D,L-lactide-co-glycolide) large porous particles (LPP) were determined on IL-6 and IL-8 mRNA expression in CF cells stimulated with lipopolysaccharide (LPS) from P. aeruginosa. The conclusion was that respirable biodegradable decoy ODN LPP may represent a promising strategy for inhibiting NF-κB transcriptional activity and related gene expression. This treatment, in vivo, was expected to reduce lung chronic inflammation in CF patients. Interestingly, De Stefano et al. (198) investigated the effects of NF-kB decoys delivered with inhalable nanoparticles in a rat model of lung inflammation induced by intratracheal aerosolization of LPS from Pseudomonas aeruginosa. A single intratracheal insufflation of the decoy ODNs reduced the bronchoalveolar neutrophil infiltration induced by LPS. This reduction was associated with decreased NF-κB/DNA binding activity, and decreased the content of IL-6, IL-8, and mucin-2 in lung homogenates.
In consideration of the involvement of microRNAs in the post-transcriptional regulation of IL-8 and other pro-inflammatory genes, both antagomiR and miRNA replacement approaches have been proposed (199–202). This confirmed that, in addition to relevance for the theoretical point of view, the studies on epigenetic regulation of chemokines (described in Chapter 5) might be important for the development of therapeutic protocols. For instance, transfection of CF cells with miR-93 (105) and miR-636 mimics (107) leads to an IL-8 decrease (105) and to a reduction of NF-κB activity, causing decreased secretion of IL-8 and IL-6 (200, 201). Another miRNA target to be considered is miR-199a-3p, whose expression is inversely correlated with increases in the expression of IKKβ and IL-8 (200). On the other hand, targeting miR-155 with antagomir might also be considered for IL-8 reduction (104). In fact, down-regulation of miR-155 was found to suppress the IL-8-associated pro-inflammatory phenotype in CF cells. In order to reduce the miR-155 levels in CF cells, antagomiR molecules against miR-155 were employed, modified with cholesterol to permit efficient entry into cells. Incubation of IB3-1 cells with antagomir-155 effectively down-regulated miR-155 expression, together with a sharp decrease in IL-8 mRNA and protein levels (104). On the other hand, as expected, miRNAs were also demonstrated to directly target the 3′UTR of IL-8 mRNA, such as miR-93 (105) and miR-17 (105). Therefore, modulating the expression of miRNAs that target IL-8 mRNA in CF bronchial epithelial cells is likely to represent a new therapeutic strategy for CF (199–201).
These studies have conclusively demonstrated that the pro-inflammatory status in CF is under the control of a complex network constituted by transcription factors and non-coding RNAs, responsible for transcriptional and post-transcriptional regulation of the expression of genes, such as IL-8, belonging to the pro-inflammatory CF network. These studies allowed the identification of novel targets for pharmacological interventions based on newly designed therapeutic approaches.
In addition to the development of new experimental approaches, recent efforts have been undertaken on drug repurposing, in order to bring new therapies based on drugs already used for other indications. This is expected to bring to the market several treatments at a lower risk, reduced cost, and less development time when compared to conventional drug development programs (202–204). One of the most interesting classes of molecules are psoralens, extensively studied as molecules to be employed in PUVA (Psoralen and Ultraviolet A)-therapy, a treatment extensively used in a variety of pathological conditions, including eczema, psoriasis, graft-vs.-host disease, vitiligo, mycosis fungoides, large-plaque parapsoriasis, and cutaneous T-cell lymphoma (205). It was in a study that found that 5-methoxypsoralen reduces P. aeruginosa-dependent IL-8 transcription in bronchial epithelial cell lines (206). When the analysis was extended to analogs of 5-methoxypsoralen (207, 208), a potent effect was observed with 4,6,4′-trimethyl-angelicin (TMA), which inhibited P. aeruginosa-dependent IL-8 transcription at a nanomolar concentration in IB3-1, CuFi-1, CFBE41o-, and Calu-3 bronchial epithelial cell lines. Analysis of phosphoproteins involved in pro-inflammatory transmembrane signaling evidenced that TMA reduces the phosphorylation of ribosomal S6 kinase-1 and AKT2/3, which were found to be involved in P. aeruginosa-dependent activation of IL-8 gene transcription (208). In addition, to understand whether the NF-κB pathway should be considered a target of TMA, chromatin immunoprecipitation was performed, demonstrating that TMA (100 nM) preincubated in whole living cells reduced the interaction of NF-κB with the promoter of IL-8 gene. These results suggest that TMA could inhibit IL-8 gene transcription mainly by intervening on driving the recruitment of activated transcription factors on the IL-8 gene promoter, as demonstrated in NF-κB (208). Recently, TMA was also shown to exhibit, in addition to anti-inflammatory activity, potentiation and correction of the CFTR. In conclusion, TMA is a triple-acting compound that reduces excessive IL-8 expression and potentiating/correcting CFTR function (209, 210). Another repurposed drug proposed for possible anti-inflammatory effects is azithromycin (AZM). IL-8 expression and DNA binding activity of two key pro-inflammatory transcription factors, NF-κB and AP-1, were investigated in CF and isogenic non-CF airway epithelial cell lines. AZM reduced both IL-8 mRNA and protein expression in CF cells reaching the levels of non-CF cells. In the presence of AZM reduction of NF-κB and AP-1, DNA binding was also observed (211). Regarding anti-inflammatory approaches, in vitro studies have tested the effects of genistein, fluvastatin, and corilagin, amongst others (212–214). The isoflavonoid genistein [5,7-Dihydroxy-3-(4-hydroxyphenyl)chromen-4-one] reduces IL-8 production in cultured CF bronchial gland cells by increasing cytosolic IκBα protein levels, thereby inhibiting NF-kB activation (212). The statin fluvastatin [(±)-(3R′,5S′,6E)-7-[3-(4-Fluorophenyl)-1-isopropylindol-2-yl]-3,5-dihydroxy-6-heptenoate] decreased IL-8 production in whole blood in response to Pseudomonas or Aspergillus antigens, by preventing the prenylation of molecules, such as rho-A, ras, or rac, implicated in IL-8 signaling (213). Corilagin [beta-1-O-galloyl-3,6-(R)-hexahydroxydiphenoyl-d-glucose], a gallotannin identified in several plants, including Phyllanthus urinaria, binds to NF-κB, thus inhibiting NF-κB/DNA interactions and decreasing IL-8 gene expression in CF bronchial IB3-1 cells (214).
As for drug repurposing, corilagin, already shown to exhibit versatile medicinal activities, was found to be of potential use as a possible therapeutic molecule for CF. Interestingly, in addition of IL-8 inhibition, corilagin inhibits TNF-α-induced secretion of MCP-1 and RANTES (214).
The possible identification of repurposed drugs was also tackled by alternative approaches, such as connectivity mapping (ssCMap) to predict A20-inducing drugs and their anti-inflammatory action in CF. A20 is a NF-κB down-regulator that is expressed at low levels in CF and it is hypothesized to be a key target to normalize the inflammatory response (215). Publicly available gene array expression data, together with a statistically significant connections' map (sscMap), were employed. The objective was to predict drugs already licensed for therapeutic use in human pathologies to induce A20 mRNA and protein expression and thereby reduce inflammation. Ikarugamycin and quercetin have been identified as possible candidates for anti-inflammatory approaches, analyzing their effects on A20 and NF-κB(p65) expression (mRNA) as well as IL-8 pro-inflammatory cytokine release in the presence and absence of bacterial LPS in bronchial epithelial cells lines and in primary nasal epithelial cells from patients with CF and non-CF controls (215). Despite the very interesting results obtained from studies of drug repurposing in CF, the safety assessment in a new disease indication (CF in this case) is still an important concern in the regulatory process. While the safety assessment is based on drug label information, the drug repurposing approach may involve different formulations, changes in dosage that should be given great attention in the different patient populations considered.
Concluding Remarks and Perspectives
The present review outlines different specific pathophysiological aspects of CF lung inflammation in which the bronchial epithelial cells represent a “crossroad of signaling” in this disease. Particular emphasis has been given to the role of bronchial epithelial cells in driving the process of neutrophil chemotaxis, with special regard to intracellular signaling, that could be considered a therapeutic target to reduce the lung tissue damage dependent on the byproducts released by hyper-activated neutrophils in the CF bronchial mucosa. To translate into therapy, a special focus was placed on innovative molecules or on drug repurposing to target pathways that are specific of the CF lung pathophysiology, instead of testing broad range anti-inflammatory molecules. As CF lung inflammation is a clearly secondary effect of altered CFTR protein defective ion transport, a question arises on whether in the era of CFTR modulators, with increasing efficacy in CFTR rescue, gating potentiation, and PM stabilization, anti-inflammatory drugs maintain a specific therapeutic rationale. It has already been shown that rescue of F508del CFTR in CF in experimental model systems can partly reduce the release of pro-inflammatory mediators, including IL-8 (216–218). However, the CFTR correctors and potentiators are not available for all the classes of CFTR molecular defects, leaving a significant fraction of CF patients without this treatment option. The clinical response has been shown to be variable within patients with the same class of mutations; the advanced inflammatory disease in adolescent and adult CF patients is unlikely to be completely halted using only CFTR correctors and potentiators, leaving the development of novel anti-inflammatory drugs a rational unmet need for CF treatment (219, 220).
Author Contributions
GC, AR, PP, and RG initiated the concept and wrote the manuscript together with MB, IL, and AF. All authors contributed to the article and approved the submitted version.
Funding
The research was funded by grants from Italian Cystic Fibrosis Research Foundation grant FFC #18/2009, FFC #19/2011, and FFC #17/2014 to GC, FFC #20/2015 to AR, FFC #12/2010 and #19/2014 to PP, grant FFC #7/2018 to RG, grant FFC #22/2019 to IL, and Institutional funds from the University of Ferrara supporting the Center for Innovative Therapies for Cystic Fibrosis. AR was supported by local founds from the University of Ferrara, FIR-2017, the Italian Ministry of Health (GR-2016-02364602), and the Italian Ministry of Education, University and Research (PRIN Grant 2017XA5J5N). The Italian Association for Cancer Research (AIRC, IG-23670), Telethon (GGP11139B), local funds from the University of Ferrara, and the Italian Ministry of Education, University, and Research (PRIN Grant 2017E5L5P3) to PP.
Conflict of Interest
The authors declare that the research was conducted in the absence of any commercial or financial relationships that could be construed as a potential conflict of interest.
Acknowledgments
We are grateful to Mirko Pinotti, Marco Cipolli, Adriana Chilin, Marco Prosdocimi, Maria Cristina Dechecchi, Anna Tamanini, Valentino Bezzerri, Paola Prandini, Lisa Provezza, Alessandra Santangelo, Silvia Munari, and Simone Patergnani for helpful discussions. PP is grateful to Camilla degli Scrovegni for continuous support.
References
1. Riordan JR, Rommens JM, Kerem B, Alon N, Rozmahel R, Grzelczak Z, et al. Identification of the cystic fibrosis gene: cloning and characterization of complementary DNA. Science. (1989) 245:1066–73. doi: 10.1126/science.2475911
2. Rommens JM, Iannuzzi MC, Kerem B, Drumm ML, Melmer G, Dean M, et al. Identification of the cystic fibrosis gene: chromosome walking and jumping. Science. (1989) 245:1059–65. doi: 10.1126/science.2772657
3. Kerem B, Rommens JM, Buchanan JA, Markiewicz D, Cox TK, Chakravarti A, et al. Identification of the cystic fibrosis gene: genetic analysis. Science. (1989) 245:1073–80. doi: 10.1126/science.2570460
4. Khan TZ, Wagener JS, Bost T, Martinez J, Accurso FJ, Riches DW. Early pulmonary inflammation in infants with cystic fibrosis. Am J Respir Crit Care Med. (1995) 151:1075–82. doi: 10.1164/ajrccm.151.4.7697234
5. Noah TL, Black HR, Cheng PW, Wood RE, Leigh MW. Nasal and bronchoalveolar lavage fluid cytokines in early cystic fibrosis. J Infect Dis. (1997) 175:638–47. doi: 10.1093/infdis/175.3.638
6. Armstrong DS, Grimwood K, Carlin JB, Carzino R, Gutièrrez JP, Hull J, et al. Lower airway inflammation in infants and young children with cystic fibrosis. Am J Respir Crit Care Med. (1997) 156:1197–204. doi: 10.1164/ajrccm.156.4.96-11058
7. Stoltz DA, Meyerholz DK, Welsh MJ. Origins of cystic fibrosis lung disease. N Engl J Med. (2015) 372:351–62. doi: 10.1056/NEJMra1300109
8. Boucher RC. Evidence for airway surface dehydration as the initiating event in CF airway disease. J Intern Med. (2007) 261:5–16. doi: 10.1111/j.1365-2796.2006.01744.x
9. Tingpej P, Smith L, Rose B, Zhu H, Conibear T, Al Nassafi K, et al. Phenotypic characterization of clonal and non-clonal Pseudomonas aeruginosa strains isolated from lungs of adults with cystic fibrosis. J Clin Microbiol. (2007) 45:1697–704. doi: 10.1128/JCM.02364-06
10. Boucher RC. Airway surface dehydration in cystic fibrosis: pathogenesis and therapy. Annu Rev Med. (2007) 58:157–70. doi: 10.1146/annurev.med.58.071905.105316
11. Derichs N, Jin BJ, Song Y, Finkbeiner WE, Verkman AS. Hyperviscous airway periciliary and mucous liquid layers in cystic fibrosis measured by confocal fluorescence photobleaching. FASEB J. (2011) 25:2325–32. doi: 10.1096/fj.10-179549
12. Hayes E, Pohl K, McElvaney NG, Reeves EP. The cystic fibrosis neutrophil: a specialized yet potentially defective cell. Arch Immunol Ther Exp. (2011) 59:97–112. doi: 10.1007/s00005-011-0113-6
13. Laval J, Ralhan A, Hartl D. Neutrophils in cystic fibrosis. Biol Chem. (2016) 397:485–96. doi: 10.1515/hsz-2015-0271
14. Painter RG, Valentine VG, Lanson NA Jr, Leidal K, Zhang Q, Lombard G, et al. CFTR Expression in human neutrophils and the phagolysosomal chlorination defect in cystic fibrosis. Biochemistry. (2006) 45:10260–9. doi: 10.1021/bi060490t
15. Painter RG, Bonvillain RW, Valentine VG, Lombard GA, LaPlace SG, Nauseef WM, et al. The role of chloride anion and CFTR in killing of Pseudomonas aeruginosa by normal and CF neutrophils. J Leukoc Biol. (2008) 83:1345–53. doi: 10.1189/jlb.0907658
16. Painter RG, Marrero L, Lombard GA, Valentine VG, Nauseef WM, Wang G. CFTR-mediated halide transport in phagosomes of human neutrophils. J Leukoc Biol. (2010) 87:933–42. doi: 10.1189/jlb.1009655
17. Bonfield TL, Hodges CA, Cotton CU, Drumm ML. Absence of the cystic fibrosis transmembrane regulator (Cftr) from myeloid-derived cells slows resolution of inflammation and infection. J Leukoc Biol. (2012) 92:1111–22. doi: 10.1189/jlb.0412188
18. Ng HP, Zhou Y, Song K, Hodges CA, Drumm ML, Wang G. Neutrophil-mediated phagocytic host defense defect in myeloid Cftr-inactivated mice. PLoS ONE. (2014) 9:e106813. doi: 10.1371/journal.pone.0106813
19. Zhou Y, Song K, Painter RG, Aiken M, Reiser J, Stanton BA, et al. Cystic fibrosis transmembrane conductance regulator recruitment to phagosomes in neutrophils. J Innate Immun. (2013) 5:219–30. doi: 10.1159/000346568
20. Pohl K, Hayes E, Keenan J, Henry M, Meleady P, Molloy K, et al. A neutrophil intrinsic impairment affecting Rab27a and degranulation in cystic fibrosis is corrected by CFTR potentiator therapy. Blood. (2014) 124:999–1009. doi: 10.1182/blood-2014-02-555268
21. Hartl D, Latzin P, Hordijk P, Marcos V, Rudolph C, Woischnik M, et al. Cleavage of CXCR1 on neutrophils disables bacterial killing in cystic fibrosis lung disease. Nat Med. (2007) 13:1423–30. doi: 10.1038/nm1690
22. Döring G. Polymorphonuclear leukocyte elastase: its effects on the pathogenesis of Pseudomonas aeruginosa infection in cystic fibrosis. Antibiot Chemother. (1989) 42:169–76. doi: 10.1159/000417617
23. Nadel JA. Protease actions on airway secretions. Relevance to cystic fibrosis. Ann N Y Acad Sci. (1991) 624:286–96. doi: 10.1111/j.1749-6632.1991.tb17027.x
24. Birrer P, McElvaney NG, Rüdeberg A, Sommer CW, Liechti-Gallati S, Kraemer R, et al. Protease-antiprotease imbalance in the lungs of children with cystic fibrosis. Am J Respir Crit Care Med. (1994) 150:207–13. doi: 10.1164/ajrccm.150.1.7912987
25. Döring G. The role of neutrophil elastase in chronic inflammation. Am J Respir Crit Care Med. (1994) 150:S114–7. doi: 10.1164/ajrccm/150.6_Pt_2.S114
26. Suter S. The role of bacterial proteases in the pathogenesis of cystic fibrosis. Am J Respir Crit Care Med. (1994) 150:S118–22. doi: 10.1164/ajrccm/150.6_Pt_2.S118
27. Venaille TJ, Ryan G, Robinson BW. Epithelial cell damage is induced by neutrophil-derived, not pseudomonas-derived, proteases in cystic fibrosis sputum. Respir Med. (1998) 92:233–40. doi: 10.1016/S0954-6111(98)90101-9
28. Kelly E, Greene CM, McElvaney NG. Targeting neutrophil elastase in cystic fibrosis. Expert Opin Ther Targets. (2008) 12:145–57. doi: 10.1517/14728222.12.2.145
29. Griese M, Kappler M, Gaggar A, Hartl D. Inhibition of airway proteases in cystic fibrosis lung disease. Eur Respir J. (2008) 32:783–95. doi: 10.1183/09031936.00146807
30. Dittrich AS, Kühbandner I, Gehrig S, Rickert-Zacharias V, Twigg M, Wege S, et al. Elastase activity on sputum neutrophils correlates with severity of lung disease in cystic fibrosis. Eur Respir J. (2018) 51:1701910. doi: 10.1183/13993003.01910-2017
31. McKelvey MC, Weldon S, McAuley DF, Mall MA, Taggart CC. Targeting proteases in cystic fibrosis lung disease: paradigms, progress, and potential. Am J Respir Crit Care Med. (2019). 201:141–7. doi: 10.1164/rccm.201906-1190PP
32. Le Gars M, Descamps D, Roussel D, Saussereau E, Guillot L, Ruffin M, et al. Neutrophil elastase degrades cystic fibrosis transmembrane conductance regulator via calpains and disables channel function in vitro and in vivo. Am J Respir Crit Care Med. (2013) 187:170–9. doi: 10.1164/rccm.201205-0875OC
33. Taggart C, Coakley RJ, Greally P, Canny G, O'Neill SJ, McElvaney NG. Increased elastase release by CF neutrophils is mediated by tumor necrosis factor-alpha and interleukin-8. Am J Physiol Lung Cell Mol Physiol. (2000) 278:L33–41. doi: 10.1152/ajplung.2000.278.1.L33
34. Nakamura H, Yoshimura K, McElvaney NG, Crystal RG. Neutrophil elastase in respiratory epithelial lining fluid of individuals with cystic fibrosis induces interleukin-8 gene expression in a human bronchial epithelial cell line. J Clin Invest. (1992) 89:1478–84. doi: 10.1172/JCI115738
35. Walsh DE, Greene CM, Carroll TP, Taggart CC, Gallagher PM, O'Neill SJ, et al. Interleukin-8 up-regulation by neutrophil elastase is mediated by MyD88/IRAK/TRAF-6 in human bronchial epithelium. J Biol Chem. (2001) 276:35494–9. doi: 10.1074/jbc.M103543200
36. Devaney JM, Greene CM, Taggart CC, Carroll TP, O'Neill SJ, McElvaney NG. Neutrophil elastase up-regulates interleukin-8 via toll-like receptor 4. FEBS Lett. (2003) 544:129–32. doi: 10.1016/S0014-5793(03)00482-4
37. Galli F, Battistoni A, Gambari R, Pompella A, Bragonzi A, Pilolli F, et al. Oxidative stress and antioxidant therapy in cystic fibrosis. Biochim Biophys Acta. (2012) 1822:690–713. doi: 10.1016/j.bbadis.2011.12.012
38. Cantin AM, North SL, Fells GA, Hubbard RC, Crystal RG. Oxidant-mediated epithelial cell injury in idiopathic pulmonary fibrosis. J Clin Invest. (1987) 79:1665–73. doi: 10.1172/JCI113005
39. Cantin A, Woods DE. Protection by antibiotics against myeloperoxidase-dependent cytotoxicity to lung epithelial cells in vitro. J Clin Invest. (1993) 91:38–45. doi: 10.1172/JCI116196
40. Witko-Sarsat V, Delacourt C, Rabier D, Bardet J, Nguyen AT, Descamps-Latscha B. Neutrophil-derived long-lived oxidants in cystic fibrosis sputum. Am J Respir Crit Care Med. (1995) 152:1910–6. doi: 10.1164/ajrccm.152.6.8520754
41. Worlitzsch D, Herberth G, Ulrich M, Döring G. Catalase, myeloperoxidase and hydrogen peroxide in cystic fibrosis. Eur Respir J. (1998) 11:377–83. doi: 10.1183/09031936.98.11020377
42. Marcos V, Zhou-Suckow Z, Önder Yildirim A, Bohla A, Hector A, Vitkov L, et al. Free DNA in cystic fibrosis airway fluids correlates with airflow obstruction. Mediators Inflamm. (2015) 2015:408935. doi: 10.1155/2015/408935
43. Lethem MI, James SL, Marriott C, Burke JF. The origin of DNA associated with mucus glycoproteins in cystic fibrosis sputum. Eur Respir J. (1990) 3:19–23.
44. Marcos V, Zhou Z, Yildirim AO, Bohla A, Hector A, Vitkov L, et al. CXCR2 mediates NADPH oxidase-independent neutrophil extracellular trap formation in cystic fibrosis airway inflammation. Nat Med. (2010) 16:1018–23. doi: 10.1038/nm.2209
45. Cheng OZ, Palaniyar N. NET balancing: a problem in inflammatory lung diseases. Front Immunol. (2013) 4:1. doi: 10.3389/fimmu.2013.00001
46. Porto BN, Stein RT. Neutrophil extracellular traps in pulmonary diseases: too much of a good thing? Front Immunol. (2016) 7:311. doi: 10.3389/fimmu.2016.00311
47. Schultz A, Stick S. Early pulmonary inflammation and lung damage in children with cystic fibrosis. Respirology. (2015) 20:569–78. doi: 10.1111/resp.12521
48. Montgomery ST, Mall MA, Kicic A, Stick SM, AREST CF. Hypoxia and sterile inflammation in cystic fibrosis airways: mechanisms and potential therapies. Eur Respir J. (2017) 49:1600903. doi: 10.1183/13993003.00903-2016
49. Esther CR Jr, Muhlebach MS, Ehre C, Hill DB, Wolfgang MC, Kesimer M, et al. Mucus accumulation in the lungs precedes structural changes and infection in children with cystic fibrosis. Sci Transl Med. (2019) 11:eaav3488. doi: 10.1126/scitranslmed.aav3488
50. Höpken UE, Lu B, Gerard NP, Gerard C. The C5a chemoattractant receptor mediates mucosal defence to infection. Nature. (1996) 383:86–9. doi: 10.1038/383086a0
51. Gerard NP, Lu B, Liu P, Craig S, Fujiwara Y, Okinaga S, et al. An anti- inflammatory function for the complement anaphylatoxin C5a-binding protein, C5L2. J Biol Chem. (2005) 280:39677–80. doi: 10.1074/jbc.C500287200
52. van den Berg CW, Tambourgi DV, Clark HW, Hoong SJ, Spiller OB, McGreal EP. Mechanism of neutrophil dysfunction: neutrophil serine proteases cleave and inactivate the C5a receptor. J Immunol. (2014) 192:1787–95. doi: 10.4049/jimmunol.1301920
53. Sass LA, Hair PS, Perkins AM, Shah TA, Krishna NK, Cunnion KM. Complement effectors of inflammation in cystic fibrosis lung fluid correlate with clinical measures of disease. PLoS ONE. (2015) 10:e0144723. doi: 10.1371/journal.pone.0144723
54. Hair PS, Sass LA, Vazifedan T, Shah TA, Krishna NK, Cunnion KM. Complement effectors, C5a and C3a, in cystic fibrosis lung fluid correlate with disease severity. PLoS ONE. (2017) 12:e0173257. doi: 10.1371/journal.pone.0173257
55. Ford-Hutchinson AW, Bray MA, Doig MV, Shipley ME, Smith MJ. Leukotriene B, a potent chemokinetic and aggregating substance released from polymorphonuclear leukocytes. Nature. (1980) 286:264–5. doi: 10.1038/286264a0
56. Sampson AP, Spencer DA, Green CP, Piper PJ, Price JF. Leukotrienes in the sputum and urine of cystic fibrosis children. Br J Clin Pharmacol. (1990) 30:861–9. doi: 10.1111/j.1365-2125.1990.tb05452.x
57. Konstan MW, Walenga RW, Hilliard KA, Hilliard JB. Leukotriene B4 markedly elevated in the epithelial lining fluid of patients with cystic fibrosis. Am Rev Respir Dis. (1993) 148:896–901. doi: 10.1164/ajrccm/148.4_Pt_1.896
58. Carpagnano GE, Barnes PJ, Geddes DM, Hodson ME, Kharitonov SA. Increased leukotriene B4 and interleukin-6 in exhaled breath condensate in cystic fibrosis. Am J Respir Crit Care Med. (2003) 167:1109–12. doi: 10.1164/rccm.200203-179OC
59. Konstan MW, Vargo KM, Davis PB. Ibuprofen attenuates the inflammator response to Pseudomonas aeruginosa in a rat model of chronic pulmonary infection. Implications for antiinflammatory therapy in cystic fibrosis. Am Rev Respir Dis. (1990) 141:186–92. doi: 10.1164/ajrccm/141.1.186
60. Konstan MW, Davis PB. Pharmacological approaches for the discovery and development of new anti-inflammatory agents for the treatment of cystic fibrosis. Adv Drug Deliv Rev. (2002) 54:1409–23. doi: 10.1016/S0169-409X(02)00146-1
61. Konstan MW, Döring G, Heltshe SL, Lands LC, Hilliard KA, Koker P, et al. A randomized double blind, placebo controlled phase 2 trial of BIIL 284BS (an LTB4 receptor antagonist) for the treatment of lung disease in children and adults with cystic fibrosis. J Cyst Fibros. (2014) 13:148–55. doi: 10.1016/j.jcf.2013.12.009
62. Döring G, Bragonzi A, Paroni M, Aktürk FF, Cigana C, Schmidt A, et al. BIIL 284 reduces neutrophil numbers but increases P. aeruginosa bacteremia and inflammation in mouse lungs. J Cyst Fibros. (2014) 13:156–63. doi: 10.1016/j.jcf.2013.10.007
63. Forrest OA, Ingersoll SA, Preininger MK, Laval J, Limoli DH, Brown MR, et al. Frontline Science: pathological conditioning of human neutrophils recruited to the airway milieu in cystic fibrosis. J Leukoc Biol. (2018) 104:665–75. doi: 10.1002/JLB.5HI1117-454RR
64. Elborn JS, Ahuja S, Springman E, Mershon J, Grosswald R, Rowe SM. EMPIRE-CF: a phase II randomized placebo-controlled trial of once-daily, oral acebilustat in adult patients with cystic fibrosis–study design and patient demographics. Contemp Clin Trials. (2018) 72:86–94. doi: 10.1016/j.cct.2018.07.014
65. Saiman L, Prince A. Pseudomonas aeruginosa pili bind to asialoGM1 which is increased on the surface of cystic fibrosis epithelial cells. J Clin Invest. (1993) 92:1875–80. doi: 10.1172/JCI116779
66. Imundo L, Barasch J, Prince A, Al-Awqati Q. Cystic fibrosis epithelial cells have a receptor for pathogenic bacteria on their apical surface. Proc Natl Acad Sci USA. (1995) 92:3019–23. doi: 10.1073/pnas.92.7.3019
67. DiMango E, Zar HJ, Bryan R, Prince A. Diverse Pseudomonas aeruginosa gene products stimulate respiratory epithelial cells to produce interleukin-8. J Clin Invest. (1995) 96:2204–10. doi: 10.1172/JCI118275
68. Feldman M, Bryan R, Rajan S, Scheffler L, Brunnert S, Tang H, et al. Role of flagella in pathogenesis of Pseudomonas aeruginosa pulmonary infection. Infect Immun. (1998) 66:43–51. doi: 10.1128/IAI.66.1.43-51.1998
69. DiMango E, Ratner AJ, Bryan R, Tabibi S, Prince A. Activation of NF-kappaB by adherent Pseudomonas aeruginosa in normal and cystic fibrosis respiratory epithelial cells. J Clin Invest. (1998) 101:2598–605. doi: 10.1172/JCI2865
70. Bryan R, Prince A. Bacterial-epithelial interactions. Mol Med. (2002) 70:465–78. doi: 10.1385/1-59259-187-6:465
71. Rastogi D, Ratner AJ, Prince A. Host-bacterial interactions in the initiation of inflammation. Paediatr Respir Rev. (2001) 2:245–52. doi: 10.1053/prrv.2001.0147
72. Parker D, Prince A. Epithelial uptake of flagella initiates pro-inflammatory signaling. PLoS ONE. (2013) 8:e59932. doi: 10.1371/journal.pone.0059932
73. Massion PP, Inoue H, Richman-Eisenstat J, Grunberger D, Jorens PG, Housset B, et al. Novel Pseudomonas product stimulates interleukin-8 production in airway epithelial cells in vitro. J Clin Invest. (1994) 93:26–32. doi: 10.1172/JCI116954
74. Jorens PG, Richman-Eisenstat JB, Housset BP, Massion PP, Ueki I, Nadel JA. Pseudomonas-induced neutrophil recruitment in the dog airway in vivo is mediated in part by IL-8 and inhibited by a leumedin. Eur Respir J. (1994) 7:1925–31.
75. Moura JA, Cristina de Assis M, Ventura GC, Saliba AM, Gonzaga L Jr, Si-Tahar M, et al. Differential interaction of bacterial species from the Burkholderia cepacia complex with human airway epithelial cells. Microbes Infect. (2008) 10:52–9. doi: 10.1016/j.micinf.2007.10.002
76. Zhao X, Town JR, Li F, Zhang X, Cockcroft DW, Gordon JR. ELR-CXC chemokine receptor antagonism targets inflammatory responses at multiple levels. J Immunol. (2009) 182:3213–22. doi: 10.4049/jimmunol.0800551
77. Kaza SK, McClean S, Callaghan M. IL-8 released from human lung epithelial cells induced by cystic fibrosis pathogens Burkholderia cepacia complex affects the growth and intracellular survival of bacteria. Int J Med Microbiol. (2011) 301:26–33. doi: 10.1016/j.ijmm.2010.06.005
78. Bezzerri V, d'Adamo P, Rimessi A, Lanzara C, Crovella S, Nicolis E, et al. Phospholipase C-β3 is a key modulator of IL-8 expression in cystic fibrosis bronchial epithelial cells. J Immunol. (2011) 186:4946–58. doi: 10.4049/jimmunol.1003535
79. Dechecchi MC, Nicolis E, Mazzi P, Cioffi F, Bezzerri V, Lampronti I, et al. Modulators of sphingolipid metabolism reduce lung inflammation. Am J Respir Cell Mol Biol. (2011) 45:825–33. doi: 10.1165/rcmb.2010-0457OC
80. Mizunoe S, Shuto T, Suzuki S, Matsumoto C, Watanabe K, Ueno-Shuto K, et al. Synergism between interleukin (IL)-17 and Toll-like receptor 2 and 4 signals to induce IL-8 expression in cystic fibrosis airway epithelial cells. J Pharmacol Sci. (2012) 118:512–20. doi: 10.1254/jphs.11240FP
81. Prandini P, De Logu F, Fusi C, Provezza L, Nassini R, Montagner G, et al. Transient receptor potential ankyrin 1 channels modulate inflammatory response in respiratory cells from patients with cystic fibrosis. Am J Respir Cell Mol Biol. (2016) 55:645–56. doi: 10.1165/rcmb.2016-0089OC
82. Ribeiro CM, Paradiso AM, Schwab U, Perez-Vilar J, Jones L, O'neal W, et al. Chronic airway infection/inflammation induces a Ca2+i-dependent hyperinflammatory response in human cystic fibrosis airway epithelia. J Biol Chem. (2005) 280:17798–806. doi: 10.1074/jbc.M410618200
83. Rosenow T, Mok LC, Turkovic L, Berry LJ, Sly PD, Ranganathan S, et al. The cumulative effect of inflammation and infection on structural lung disease in early cystic fibrosis. Eur Respir J. (2019) 54:1801771. doi: 10.1183/13993003.01771-2018
84. Balázs A, Mall MA. Mucus obstruction and inflammation in early cystic fibrosis lung disease: emerging role of the IL-1 signaling pathway. Pediatr Pulmonol. (2019) 54(suppl.3):S5–12. doi: 10.1002/ppul.24462
85. Adamo R, Sokol S, Soong G, Gomez MI, Prince A. Pseudomonas aeruginosa flagella activate airway epithelial cells through asialoGM1 and toll-like receptor 2 as well as toll-like receptor 5. Am J Respir Cell Mol Biol. (2004) 30:627–34. doi: 10.1165/rcmb.2003-0260OC
86. Sadikot RT, Blackwell TS, Christman JW, Prince AS. Pathogen-host interactions in Pseudomonas aeruginosa pneumonia. Am J Respir Crit Care Med. (2005) 171:1209–23. doi: 10.1164/rccm.200408-1044SO
87. Prince A. Flagellar activation of epithelial signaling. Am J Respir Cell Mol Biol. (2006) 34:548–51. doi: 10.1165/rcmb.2006-0022SF
88. Ratner AJ, Bryan R, Weber A, Nguyen S, Barnes D, Pitt A, et al. Cystic fibrosis pathogens activate Ca2+-dependent mitogen-activated protein kinase signaling pathways in airway epithelial cells. J Biol Chem. (2001) 276:19267–75. doi: 10.1074/jbc.M007703200
89. Bezzerri V, Borgatti M, Finotti A, Tamanini A, Gambari R, Cabrini G. Mapping the transcriptional machinery of the IL-8 gene in human bronchial epithelial cells. J Immunol. (2011) 187:6069–81. doi: 10.4049/jimmunol.1100821
90. Illek B, Fu Z, Schwarzer C, Banzon T, Jalickee S, Miller SS, et al. Flagellin-stimulated Cl- secretion and innate immune responses in airway epithelia: role for p38. Am J Physiol Lung Cell Mol Physiol. (2008) 295:L531–42. doi: 10.1152/ajplung.90292.2008
91. McNamara N, Khong A, McKemy D, Caterina M, Boyer J, Julius D, et al. ATP transduces signals from ASGM1, a glycolipid that functions as a bacterial receptor. Proc Natl Acad Sci USA. (2001) 98:9086–91. doi: 10.1073/pnas.161290898
92. McNamara N, Gallup M, Sucher A, Maltseva I, McKemy D, Basbaum C. AsialoGM1 and TLR5 cooperate in flagellin-induced nucleotide signaling to activate Erk1/2. Am J Respir Cell Mol Biol. (2006) 34:653–60. doi: 10.1165/rcmb.2005-0441OC
93. Rimessi A, Bezzerri V, Salvatori F, Tamanini A, Nigro F, Dechecchi MC, et al. PLCB3 loss of function reduces Pseudomonas aeruginosa-dependent IL-8 release in cystic fibrosis. Am J Respir Cell Mol Biol. (2018) 59:428–36. doi: 10.1165/rcmb.2017-0267OC
94. Bezzerri V, Borgatti M, Nicolis E, Lampronti I, Dechecchi MC, Mancini I, et al. Transcription factor oligodeoxynucleotides to NF-kappaB inhibit transcription of IL-8 in bronchial cells. Am J Respir Cell Mol Biol. (2008) 39:86–96. doi: 10.1165/rcmb.2007-0176OC
95. Jundi K, Greene CM. Transcription of interleukin-8: how altered regulation can affect cystic fibrosis lung disease. Biomolecules. (2015) 5:1386–98. doi: 10.3390/biom5031386
96. Ashburner BP, Westerheide SD, Baldwin AS Jr. The p65 (RelA) subunit of NF-κB interacts with the histone deacetylase (HDAC) corepressors HDAC1 and HDAC2 to negatively regulate gene expression. Mol Cell Biol. (2001) 21:7065–77. doi: 10.1128/MCB.21.20.7065-7077.2001
97. Vanden Berghe W, de Bosscher K, Boone E, Plaisance S, Haegeman G. The nuclear factor-κB engages CBP/p300 and histone acetyltransferase activity for transcriptional activation of the interleukin-6 gene promoter. J Biol Chem. (1999) 274:32091–8. doi: 10.1074/jbc.274.45.32091
98. Wu GD, Lai EJ, Huang N, Wen X. Oct-1 and CCAAT/enhancer-binding protein (C/EBP) bind to overlapping elements within the interleukin-8 promoter. The role of Oct-1 as a transcriptional repressor. J Biol Chem. (1997) 272:2396–403. doi: 10.1074/jbc.272.4.2396
99. Nourbakhsh M, Kalble S, Dorrie A, Hauser H, Resch K, Kracht M. The NF-κB repressing factor is involved in basal repression and interleukin (IL)-1-induced activation of IL-8 transcription by binding to a conserved NF-κB-flanking sequence element. Biol Chem. (2001) 276:4501–8. doi: 10.1074/jbc.M007532200
100. Farahmand L, Darvishi B, Majidzadeh AK. Suppression of chronic inflammation with engineered nanomaterials delivering nuclear factor κB transcription factor decoy oligodeoxynucleotides. Drug Deliv. (2017) 24:1249–61. doi: 10.1080/10717544.2017.1370511
101. Wardwell PR, Bader RA. Immunomodulation of cystic fibrosis epithelial cells via NF-κB decoy oligonucleotide-coated polysaccharide nanoparticles. J Biomed Mater Res A. (2015) 103:1622–31. doi: 10.1002/jbm.a.35296
102. Bartling TR, Drumm ML. Loss of CFTR results in reduction of histone deacetylase 2 in airway epithelial cells. Am J Physiol Lung Cell Mol Physiol. (2009) 297:L35–43. doi: 10.1152/ajplung.90399.2008
103. Poghosyan A, Patel JK, Clifford RL, Knox AJ. Epigenetic dysregulation of interleukin 8 (CXCL8) hypersecretion in cystic fibrosis airway epithelial cells. Biochem Biophys Res Commun. (2016) 476:431–7. doi: 10.1016/j.bbrc.2016.05.140
104. Bhattacharyya S, Kumar P, Tsuchiya M, Bhattacharyya A, Biswas R. Regulation of miR-155 biogenesis in cystic fibrosis lung epithelial cells: antagonistic role of two mRNA-destabilizing proteins, KSRP and TTP. Biochem Biophys Res Commun. (2013) 433:484–8. doi: 10.1016/j.bbrc.2013.03.025
105. Fabbri E, Borgatti M, Montagner G, Bianchi N, Finotti A, Lampronti I, et al. Expression of microRNA-93 and Interleukin-8 during Pseudomonas aeruginosa-mediated induction of proinflammatory responses. Am J Respir Cell Mol Biol. (2014) 50:1144–55. doi: 10.1165/rcmb.2013-0160OC
106. Oglesby IK, Vencken SF, Agrawal R, Gaughan K, Molloy K, Higgins G, et al. miR-17 overexpression in cystic fibrosis airway epithelial cells decreases interleukin-8 production. Eur Respir J. (2015) 46:1350–60. doi: 10.1183/09031936.00163414
107. Bardin P, Foussignière T, Rousselet N, Rebeyrol C, Porter JC, Corvol H, et al. miR-636: a newly-identified actor for the regulation of pulmonary inflammation in cystic fibrosis. Front Immunol. (2019) 10:2643. doi: 10.3389/fimmu.2019.02643
108. Muir A, Soong G, Sokol S, Reddy B, Gomez MI, Van Heeckeren A, et al. Toll-like receptors in normal and cystic fibrosis airway epithelial cells. Am J Respir Cell Mol Biol. (2004) 30:777–83. doi: 10.1165/rcmb.2003-0329OC
109. Greene CM, Carroll TP, Smith SG, Taggart CC, Devaney J, Griffin S, et al. TLR-induced inflammation in cystic fibrosis and non-cystic fibrosis airway epithelial cells. J Immunol. (2005) 174:1638–46. doi: 10.4049/jimmunol.174.3.1638
110. Brown RK, Kelly FJ. Evidence for increased oxidative damage in patients with cystic fibrosis. Pediatr Res. (1994) 36:487–93. doi: 10.1203/00006450-199410000-00013
111. Boncoeur E, Criq VS, Bonvin E, Roque T, Henrion-Caude A, Gruenert DC, et al. Oxidative stress induces extracellular signal-regulated kinase 1/2 mitogen-activated protein kinase in cystic fibrosis lung epithelial cells: potential mechanism for excessive IL-8 expression. Int J Biochem Cell Biol. (2008) 40:432–46. doi: 10.1016/j.biocel.2007.08.013
112. Livnat G, Bentur L, Kuzmisnsky E, Nagler RM. Salivary profile and oxidative stress in children and adolescents with cystic fibrosis. J Oral Pathol Med. (2010) 39:16–21. doi: 10.1111/j.1600-0714.2009.00813.x
113. Dickerhof N, Pearson JF, Hoskin TS, Berry LJ, Turner R, Sly PD, et al. Oxidative stress in early cystic fibrosis lung disease is exacerbated by airway glutathione deficiency. Free Radic Biol Med. (2017) 113:236–43. doi: 10.1016/j.freeradbiomed.2017.09.028
114. Tucker MA, Fox BM, Seigler N, Rodriguez-Miguelez P, Looney J, Thomas J, et al. Endothelial dysfunction in cystic fibrosis: role of oxidative stress. Oxid Med Cell Longev. (2019) 2019:1629638. doi: 10.1155/2019/1629638
115. Favia M, de Bari L, Bobba A, Atlante A. An intriguing involvement of mitochondria in cystic fibrosis. J Clin Med. (2019) 8:1890. doi: 10.3390/jcm8111890
116. Scholte BJ, Horati H, Veltman M, Vreeken RJ, Garratt LW, Tiddens HAWM, et al. Oxidative stress and abnormal bioactive lipids in early cystic fibrosis lung disease. J Cyst Fibros. (2019) 18:781–9. doi: 10.1016/j.jcf.2019.04.011
117. Velsor LW, Kariya C, Kachadourian R, Day BJ. Mitochondrial oxidative stress in the lungs of cystic fibrosis transmembrane conductance regulator protein mutant mice. Am J Respir Cell Mol Biol. (2006) 35:579–86. doi: 10.1165/rcmb.2005-0473OC
118. Kelly-Aubert M, Trudel S, Fritsch J, Nguyen-Khoa T, Baudouin-Legros M, Moriceau S, et al. GSH monoethyl ester rescues mitochondrial defects in cystic fibrosis models. Hum Mol Genet. (2011) 20:2745–59. doi: 10.1093/hmg/ddr173
119. Rottner M, Tual-Chalot S, Mostefai HA, Andriantsitohaina R, Freyssinet JM, Martínez MC. Increased oxidative stress induces apoptosis in human cystic fibrosis cells. PLoS ONE. (2011) 6:e24880. doi: 10.1371/journal.pone.0024880
120. Cantin AM, Bilodeau G, Ouellet C, Liao J, Hanrahan JW. Oxidant stress suppresses CFTR expression. Am J Physiol Cell Physiol. (2006) 290:C262–70. doi: 10.1152/ajpcell.00070.2005
121. Luciani A, Villella VR, Esposito S, Brunetti-Pierri N, Medina D, Settembre C, et al. Defective CFTR induces aggresome formation and lung inflammation in cystic fibrosis through ROS-mediated autophagy inhibition. Nat Cell Biol. (2010) 12:863–75. doi: 10.1038/ncb2090
122. Rimessi A, Previati M, Nigro F, Wieckowski MR, Pinton P. Mitochondrial reactive oxygen species and inflammation: molecular mechanisms, diseases and promising therapies. Int J Biochem Cell Biol. (2016) 81:281–93. doi: 10.1016/j.biocel.2016.06.015
123. Escames G, López LC, García JA, García-Corzo L, Ortiz F, Acuña-Castroviejo D. Mitochondrial DNA and inflammatory diseases. Hum Genet. (2012) 131:161–73. doi: 10.1007/s00439-011-1057-y
124. Nakahira K, Haspel JA, Rathinam VA, Lee SJ, Dolinay T, Lam HC, et al. Autophagy proteins regulate innate immune responses by inhibiting the release of mitochondrial DNA mediated by the NALP3 inflammasome. Nat Immunol. (2011) 12:222–30. doi: 10.1038/ni.1980
125. Junkins RD, Shen A, Rosen K, McCormick C, Lin TJ. Autophagy enhances bacterial clearance during P. aeruginosa lung infection. PLoS ONE. (2013) 8:e72263. doi: 10.1371/journal.pone.0072263
126. Maiuri L, Kroemer G. Autophagy delays progression of the two most frequent human monogenetic lethal diseases: cystic fibrosis and Wilson disease. Aging. (2018) 10:3657–61. doi: 10.18632/aging.101736
127. Abdulrahman BA, Khweek AA, Akhter A, Caution K, Kotrange S, Abdelaziz DH, et al. Autophagy stimulation by rapamycin suppresses lung inflammation and infection by Burkholderia cenocepacia in a model of cystic fibrosis. Autophagy. (2011) 7:1359–70. doi: 10.4161/auto.7.11.17660
128. Rubinsztein DC, Codogno P, Levine B. Autophagy modulation as a potential therapeutic target for diverse diseases. Nat Rev Drug Discov. (2012) 11:709–30. doi: 10.1038/nrd3802
129. De Stefano D, Villella VR, Esposito S, Tosco A, Sepe A, De Gregorio F, et al. Restoration of CFTR function in patients with cystic fibrosis carrying the F508del-CFTR mutation. Autophagy. (2014) 10:2053–74. doi: 10.4161/15548627.2014.973737
130. Villella VR, Esposito S, Ferrari E, Monzani R, Tosco A, Rossin F, et al. Autophagy suppresses the pathogenic immune response to dietary antigens in cystic fibrosis. Cell Death Dis. (2019) 10:258. doi: 10.1038/s41419-019-1500-x
131. Rimessi A, Pozzato C, Carparelli L, Rossi A, Ranucci S, De Fino I, et al. Pharmacological modulation of Mitochondrial Calcium Uniporter controls lung inflammation in cystic fibrosis. Sci Adv. (2020) 16:aax9093. doi: 10.1126/sciadv.aax9093
132. Rimessi A, Bezzerri V, Patergnani S, Marchi S, Cabrini G, Pinton P. Mitochondrial Ca2+-dependent NLRP3 activation exacerbates the Pseudomonas aeruginosa-driven inflammatory response in cystic fibrosis. Nat Commun. (2015) 6:6201. doi: 10.1038/ncomms7201
133. Feigal RJ, Shapiro BL. Mitochondrial calcium uptake and oxygen consumption in cystic fibrosis. Nature. (1979) 278:276–7. doi: 10.1038/278276a0
134. Shapiro BL, Feigal RJ, Lam LF. Mitrochondrial NADH dehydrogenase in cystic fibrosis. Proc Natl Acad Sci USA. (1979) 76:2979–83. doi: 10.1073/pnas.76.6.2979
135. Congdon PJ, Littlewood JM, Aggarwal RK, Shapiro H. Glucose 6-phosphate dehydrogenase deficiency and cystic fibrosis. Postgrad Med J. (1981) 57:453–4. doi: 10.1136/pgmj.57.669.453
136. Valdivieso AG, Marcucci F, Taminelli G, Guerrico AG, Alvarez S, Teiber ML, et al. The expression of the mitochondrial gene MT-ND4 is downregulated in cystic fibrosis. Biochem Biophys Res Commun. (2007) 356:805–9. doi: 10.1016/j.bbrc.2007.03.057
137. Atlante A, Favia M, Bobba A, Guerra L, Casavola V, Reshkin SJ. Characterization of mitochondrial function in cells with impaired cystic fibrosis transmembrane conductance regulator (CFTR) function. J Bioenerg Biomembr. (2016) 48:197–210. doi: 10.1007/s10863-016-9663-y
138. Kushwah R, Gagnon S, Sweezey NB. Intrinsic predisposition of naïve cystic fibrosis T cells to differentiate towards a Th17 phenotype. Respir Res. (2013) 14:138. doi: 10.1186/1465-9921-14-138
139. Tarique AA, Sly PD, Holt PG, Bosco A, Ware RS, Logan J, et al. CFTR-dependent defect in alternatively-activated macrophages in cystic fibrosis. J Cyst Fibros. (2017) 16:475–82. doi: 10.1016/j.jcf.2017.03.011
140. Assani K, Tazi MF, Amer AO, Kopp BT. IFN-γ stimulates autophagy-mediated clearance of Burkholderia cenocepacia in human cystic fibrosis macrophages. PLoS ONE. (2014) 9:e96681. doi: 10.1371/journal.pone.0096681
141. Assani K, Shrestha CL, Robledo-Avila F, Rajaram MV, Partida-Sanchez S, Schlesinger LS, et al. Human cystic fibrosis macrophages have defective calcium-dependent protein kinase C activation of the NADPH oxidase, an effect augmented by Burkholderia cenocepacia. J Immunol. (2017) 198:1985–94. doi: 10.4049/jimmunol.1502609
142. Lamothe J, Valvano MA. Burkholderia cenocepacia-induced delay of acidification and phagolysosomal fusion in cystic fibrosis transmembrane conductance regulator (CFTR)-defective macrophages. Microbiology. (2008) 154:3825–34. doi: 10.1099/mic.0.2008/023200-0
143. Kopp BT, Abdulrahman BA, Khweek AA, Kumar SB, Akhter A, Montione R, et al. Exaggerated inflammatory responses mediated by Burkholderia cenocepacia in human macrophages derived from Cystic fibrosis patients. Biochem Biophys Res Commun. (2012) 424:221–7. doi: 10.1016/j.bbrc.2012.06.066
144. Mould KJ, Jackson ND, Henson PM, Seibold M, Janssen WJ. Single cell RNA sequencing identifies unique inflammatory airspace macrophage subsets. JCI Insight. (2019) 4:126556. doi: 10.1172/jci.insight.126556
145. Hisert KB, Liles WC, Manicone AM. A flow cytometric method for isolating cystic fibrosis airway macrophages from expectorated sputum. Am J Respir Cell Mol Biol. (2019) 61:42–50. doi: 10.1165/rcmb.2018-0236MA
146. Ramji R, Alexander AF, Muñoz-Rojas AR, Kellman LN, Miller-Jensen K. Microfluidic platform enables live-cell imaging of signaling and transcription combined with multiplexed secretion measurements in the same single cells. Integr Biol. (2019) 11:142–53. doi: 10.1093/intbio/zyz013
147. Irimia D, Wang X. Inflammation-on-a-chip: probing the immune system ex vivo. Trends Biotechnol. (2018) 36:923–37. doi: 10.1016/j.tibtech.2018.03.011
148. Kongsuphol P, Liu Y, Ramadan Q. On-chip immune cell activation and subsequent time-resolved magnetic bead-based cytokine detection. Biomed Microdevices. (2016) 18:93. doi: 10.1007/s10544-016-0117-4
149. Fu Z, Bettega K, Carroll S, Buchholz KR, Machen TE. Role of Ca2+ in responses of airway epithelia to Pseudomonas aeruginosa, flagellin, ATP, and thapsigargin. Am J Physiol Lung Cell Mol Physiol. (2007) 292:L353–64. doi: 10.1152/ajplung.00042.2006
150. Ribeiro CM. The role of intracellular calcium signals in inflammatory responses of polarised cystic fibrosis human airway epithelia. Drugs R D. (2006) 7:17–31. doi: 10.2165/00126839-200607010-00002
151. Donnell GN, Cleland RS. Intestinal atresia or stenosis in the newborn associated with fibrocystic disease of the pancreas. Calif Med. (1961) 94:165–70.
152. Feigal RJ, Shapiro BL. Altered intracellular calcium in fibroblasts from patients with cystic fibrosis and heterozygotes. Pediatr Res. (1979) 13:764–8. doi: 10.1203/00006450-197906000-00009
153. Shapiro BL, Lam LF. Intracellular calcium in cystic fibrosis heterozygotes. Life Sci. (1987) 40:2361–6. doi: 10.1016/0024-3205(87)90510-8
154. Cabrini G, De Togni P. Increased cytosolic calcium in cystic fibrosis neutrophils. Effect on stimulus-secretion coupling. Life Sci. (1985) 36:1561–7. doi: 10.1016/0024-3205(85)90380-7
155. Feigal RJ, Tomczyk MS, Shapiro BL. The calcium abnormality in cystic fibrosis mitochondria: relative role of respiration and ATP hydrolysis. Life Sci. (1982) 30:93–8. doi: 10.1016/0024-3205(82)90640-3
156. Antigny F, Girardin N, Raveau D, Frieden M, Becq F, Vandebrouck C. Dysfunction of mitochondria Ca2+ uptake in cystic fibrosis airway epithelial cells. Mitochondrion. (2009) 9:232–41. doi: 10.1016/j.mito.2009.02.003
157. Philippe R, Antigny F, Buscaglia P, Norez C, Becq F, Frieden M, et al. SERCA and PMCA pumps contribute to the deregulation of Ca2+ homeostasis in human CF epithelial cells. Biochim Biophys Acta. (2015) 1853:892–903. doi: 10.1016/j.bbamcr.2015.01.010
158. Antigny F, Norez C, Dannhoffer L, Bertrand J, Raveau D, Corbi P, et al. Transient receptor potential canonical channel 6 links Ca2+ mishandling to cystic fibrosis transmembrane conductance regulator channel dysfunction in cystic fibrosis. Am J Respir Cell Mol Biol. (2011) 44:83–90. doi: 10.1165/rcmb.2009-0347OC
159. Vachel L, Norez C, Becq F, Vandebrouck C. Effect of VX-770 (ivacaftor) and OAG on Ca2+ influx and CFTR activity in G551D and F508del-CFTR expressing cells. J Cyst Fibros. (2013) 12:584–91. doi: 10.1016/j.jcf.2013.05.008
160. Balghi H, Robert R, Rappaz B, Zhang X, Wohlhuter-Haddad A, Evagelidis A, et al. Enhanced Ca2+ entry due to Orai1 plasma membrane insertion increases IL-8 secretion by cystic fibrosis airways. FASEB J. (2011) 25:4274–91. doi: 10.1096/fj.11-187682
161. Tabary O, Boncoeur E, de Martin R, Pepperkok R, Clément A, Schultz C, et al. Calcium-dependent regulation of NF-(kappa)B activation in cystic fibrosis airway epithelial cells. Cell Signal. (2006) 18:652–60. doi: 10.1016/j.cellsig.2005.06.004
162. Lazarowski ER, Boucher RC. Purinergic receptors in airway epithelia. Curr Opin Pharmacol. (2009) 9:262–7. doi: 10.1016/j.coph.2009.02.004
163. Huang P, Gilmore E, Kultgen P, Barnes P, Milgram S, Stutts MJ. Local regulation of cystic fibrosis transmembrane regulator and epithelial sodium channel in airway epithelium. Proc Am Thorac Soc. (2004) 1:33–7. doi: 10.1513/pats.2306012
164. Faria D, Schreiber R, Kunzelmann K. CFTR is activated through stimulation of purinergic P2Y2 receptors. Pflugers Arch. (2009) 457:1373–80. doi: 10.1007/s00424-008-0606-2
165. Billet A, Hanrahan JW. The secret life of CFTR as a calcium-activated chloride channel. J Physiol. (2013) 591:5273–8. doi: 10.1113/jphysiol.2013.261909
166. Triantafilou M, Morath S, Mackie A, Hartung T, Triantafilou K. Lateral diffusion of Toll-like receptors reveals that they are transiently confined within lipid rafts on the plasma membrane. J Cell Sci. (2004) 117:4007–14. doi: 10.1242/jcs.01270
167. Chun J, Prince A. Activation of Ca2+-dependent signaling by TLR2. J Immunol. (2006) 177:1330–7. doi: 10.4049/jimmunol.177.2.1330
168. Dohrman A, Miyata S, Gallup M, Li JD, Chapelin C, Coste A, et al. Mucin gene (MUC 2 and MUC 5AC) upregulation by Gram-positive and Gram-negative bacteria. Biochim Biophys Acta. (1998) 1406:251–9. doi: 10.1016/S0925-4439(98)00010-6
169. Asehnoune K, Strassheim D, Mitra S, Yeol Kim J, Abraham E. Involvement of PKCalpha/beta in TLR4 and TLR2 dependent activation of NF-kappaB. Cell Signal. (2005) 17:385–94. doi: 10.1016/j.cellsig.2004.08.005
170. Lampronti I, Dechecchi MC, Rimessi A, Bezzerri V, Nicolis E, Guerrini A, et al. β-sitosterol reduces the expression of chemotactic cytokine genes in cystic fibrosis bronchial epithelial cells. Front Pharmacol. (2017) 8:236. doi: 10.3389/fphar.2017.00236
171. Martins JR, Kongsuphol P, Sammels E, Dahimène S, Aldehni F, Clarke LA, et al. F508del-CFTR increases intracellular Ca(2+) signaling that causes enhanced calcium-dependent Cl(-) conductance in cystic fibrosis. Biochim Biophys Acta. (2011) 1812:1385–92. doi: 10.1016/j.bbadis.2011.08.008
172. Ribeiro CM, Lubamba BA. Role of IRE1α/XBP-1 in cystic fibrosis airway inflammation. Int J Mol Sci. (2017) 18:E118. doi: 10.3390/ijms18010118
173. Kunzelmann K, Kongsuphol P, Chootip K, Toledo C, Martins JR, Almaca J, et al. Role of the Ca2+ -activated Cl- channels bestrophin and anoctamin in epithelial cells. Biol Chem. (2011) 392:125–34. doi: 10.1515/bc.2011.010
174. Konstan MW, Hoppel CL, Chai BL, Davis PB. Ibuprofen in children with cystic fibrosis: pharmacokinetics and adverse effects. J Pediatr. (1991) 118:956–64. doi: 10.1016/S0022-3476(05)82218-8
175. Konstan MW, Byard PJ, Hoppel CL, Davis PB. Effect of high-dose ibuprofen in patients with cystic fibrosis. N Engl J Med. (1995) 332:848–54. doi: 10.1056/NEJM199503303321303
176. Lands LC, Stanojevic S. Oral non-steroidal anti-inflammatory drug therapy for lung disease in cystic fibrosis. Cochrane Database Syst Rev. (2019) 9:CD001505. doi: 10.1002/14651858.CD001505.pub5
178. McElvaney NG, Nakamura H, Birrer P, Hébert CA, Wong WL, Alphonso M, et al. Modulation of airway inflammation in cystic fibrosis. In vivo suppression of interleukin-8 levels on the respiratory epithelial surface by aerosolization of recombinant secretory leukoprotease inhibitor. J Clin Invest. (1992) 90:1296–301. doi: 10.1172/JCI115994
179. Allen ED. Opportunities for the use of aerosolized alpha 1-antitrypsin for the treatment of cystic fibrosis. Chest. (1996) 110:256S−60S. doi: 10.1378/chest.110.6_Supplement.256S
180. Vogelmeier C, Gillissen A, Buhl R. Use of secretory leukoprotease inhibitor to augment lung antineutrophil elastase activity. Chest. (1996) 110(6 suppl.):261S−6S. doi: 10.1378/chest.110.6_Supplement.261S
181. Bingle L, Tetley TD. Secretory leukoprotease inhibitor: partnering alpha 1-proteinase inhibitor to combat pulmonary inflammation. Thorax. (1996) 51:1273–4. doi: 10.1136/thx.51.12.1273
182. Tremblay GM, Janelle MF, Bourbonnais Y. Anti-inflammatory activity of neutrophil elastase inhibitors. Curr Opin Investig Drugs. (2003) 4:556–65.
183. Roghanian A, Sallenave JM. Neutrophil elastase (NE) and NE inhibitors: canonical and non-canonical functions in lung chronic inflammatory diseases (cystic fibrosis and chronic obstructive pulmonary disease). J Aerosol Med Pulm Drug Deliv. (2008) 21:125–44. doi: 10.1089/jamp.2007.0653
184. Zani ML, Tanga A, Saidi A, Serrano H, Dallet-Choisy S, Baranger K, et al. SLPI and trappin-2 as therapeutic agents to target airway serine proteases in inflammatory lung diseases: current and future directions. Biochem Soc Trans. (2011) 39:1441–6. doi: 10.1042/BST0391441
185. Dunlevy FK, Martin SL, de Courcey F, Elborn JS, Ennis M. Anti-inflammatory effects of DX-890, a human neutrophil elastase inhibitor. J Cyst Fibros. (2012) 11:300–4. doi: 10.1016/j.jcf.2012.02.003
186. McElvaney NG. Alpha-1 antitrypsin therapy in cystic fibrosis and the lung disease associated with alpha-1 antitrypsin deficiency. Ann Am Thorac Soc. (2016) 13(suppl.2):S191–6. doi: 10.1513/AnnalsATS.201504-245KV
187. Keir HR, Fong CJ, Crichton ML, Barth P, Chevalier E, Brady G, et al. Personalised anti- inflammatory therapy for bronchiectasis and cystic fibrosis: selecting patients for controlled trials of neutrophil elastase inhibition. ERJ Open Res. (2019) 5:00252–2018. doi: 10.1183/23120541.00252-2018
188. Watz H, Nagelschmitz J, Kirsten A, Pedersen F, van der Mey D, Schwers S, et al. Safety and efficacy of the human neutrophil elastase inhibitor BAY 85-8501 for the treatment of non-cystic fibrosis bronchiectasis: a randomized controlled trial. Pulm Pharmacol Ther. (2019) 56:86–93. doi: 10.1016/j.pupt.2019.03.009
189. Greene CM, Ramsay H, Wells RJ, O'Neill SJ, McElvaney NG. Inhibition of Toll-like receptor 2-mediated interleukin-8 production in Cystic Fibrosis airway epithelial cells via the alpha7-nicotinic acetylcholine receptor. Mediators Inflamm. (2010) 2010:423241. doi: 10.1155/2010/423241
190. Ha H, Debnath B, Odde S, Bensman T, Ho H, Beringer PM, et al. Discovery of novel CXCR2 inhibitors using ligand-based pharmacophore models. J Chem Inf Model. (2015) 55:1720–38. doi: 10.1021/acs.jcim.5b00181
191. Leaker BR, Barnes PJ, O'Connor B. Inhibition of LPS-induced airway neutrophilic inflammation in healthy volunteers with an oral CXCR2 antagonist. Respir Res. (2013) 14:137. doi: 10.1186/1465-9921-14-137
192. Jackson PL, Noerager BD, Jablonsky MJ, Hardison MT, Cox BD, Patterson JC, et al. A CXCL8 receptor antagonist based on the structure of N-acetyl-proline-glycine-proline. Eur J Pharmacol. (2011) 668:435–42. doi: 10.1016/j.ejphar.2011.02.045
193. Finotti A, Borgatti M, Bezzerri V, Nicolis E, Lampronti I, Dechecchi M, et al. Effects of decoy molecules targeting NF-kappaB transcription factors in Cystic fibrosis IB3-1 cells: recruitment of NF-kappaB to the IL-8 gene promoter and transcription of the IL-8 gene. Artif DNA PNA XNA. (2012) 3:97–296. doi: 10.4161/adna.21061
194. Romanelli A, Pedone C, Saviano M, Bianchi N, Borgatti M, Mischiati C, et al. Molecular interactions with nuclear factor kappaB (NF-kappaB) transcription factors of a PNA-DNA chimera mimicking NF-kappaB binding sites. Eur J Biochem. (2001) 268:6066–75. doi: 10.1046/j.0014-2956.2001.02549.x
195. Borgatti M, Lampronti I, Romanelli A, Pedone C, Saviano M, Bianchi N, et al. Transcription factor decoy molecules based on a peptide nucleic acid (PNA)-DNA chimera mimicking Sp1 binding sites. J Biol Chem. (2003) 278:7500–9. doi: 10.1074/jbc.M206780200
196. Borgatti M, Finotti A, Romanelli A, Saviano M, Bianchi N, Lampronti I, et al. Peptide nucleic acids (PNA)-DNA chimeras targeting transcription factors as a tool to modify gene expression. Curr Drug Targets. (2004) 5:735–44. doi: 10.2174/1389450043345155
197. De Stefano D, Ungaro F, Giovino C, Polimeno A, Quaglia F, Carnuccio R. Sustained inhibition of IL-6 and IL-8 expression by decoy ODN to NF-κB delivered through respirable large porous particles in LPS-stimulated cystic fibrosis bronchial cells. J Gene Med. (2011) 13:200–8. doi: 10.1002/jgm.1546
198. De Stefano D, Coletta C, Bianca Rd, Falcone L, d'Angelo I, Ungaro F, et al. A decoy oligonucleotide to NF-κB delivered through inhalable particles prevents LPS-induced rat airway inflammation. Am J Respir Cell Mol Biol. (2013) 49:288–95. doi: 10.1165/rcmb.2012-0473OC
199. Bardin P, Sonneville F, Corvol H, Tabary O. Emerging microRNA therapeutic approaches for cystic fibrosis. Front Pharmacol. (2018) 9:1113. doi: 10.3389/fphar.2018.01113
200. Bardin P, Marchal-Duval E, Sonneville F, Blouquit-Laye S, Rousselet N, Le Rouzic P, et al. Small RNA and transcriptome sequencing reveal the role of miR-199a-3p in inflammatory processes in cystic fibrosis airways. J Pathol. (2018) 245:410–20. doi: 10.1002/path.5095
201. Luly FR, Lévêque M, Licursi V, Cimino G, Martin-Chouly C, Théret N, et al. MiR-146a is over-expressed and controls IL-6 production in cystic fibrosis macrophages. Sci Rep. (2019) 9:16259. doi: 10.1038/s41598-019-52770-w
202. Tambuyzer E, Vandendriessche B, Austin CP, Brooks PJ, Larsson K, Miller Needleman KI, et al. Therapies for rare diseases: therapeutic modalities, progress and challenges ahead. Nat Rev Drug Discov. (2019) 19:93–111. doi: 10.1038/s41573-019-0049-9
203. Liu Z, Borlak J, Tong W. Deciphering miRNA transcription factor feed-forward loops to identify drug repurposing candidates for cystic fibrosis. Genome Med. (2014) 6:94. doi: 10.1186/s13073-014-0094-2
204. Newman SP. Delivering drugs to the lungs: the history of repurposing in the treatment of respiratory diseases. Adv Drug Deliv Rev. (2018) 133:5–18. doi: 10.1016/j.addr.2018.04.010
205. Marka A, Carter JB., Phototherapy for cutaneous T-cell lymphoma. Dermatol Clin. (2020) 38:127–35. doi: 10.1016/j.det.2019.08.013
206. Nicolis E, Lampronti I, Dechecchi MC, Borgatti M, Tamanini A, Bezzerri V, et al. Modulation of expression of IL-8 gene in bronchial epithelial cells by 5-methoxypsoralen. Int Immunopharmacol. (2009) 9:1411–22. doi: 10.1016/j.intimp.2009.08.013
207. Borgatti M, Chilin A, Piccagli L, Lampronti I, Bianchi N, Mancini I, et al. Development of a novel furocoumarin derivative inhibiting NF-κB dependent biological functions: design, synthesis and biological effects. Eur J Med Chem. (2011) 46:4870–7. doi: 10.1016/j.ejmech.2011.07.032
208. Tamanini A, Borgatti M, Finotti A, Piccagli L, Bezzerri V, Favia M, et al. Trimethylangelicin reduces IL-8 transcription and potentiates CFTR function. Am J Physiol Lung Cell Mol Physiol. (2011) 300:L380–90. doi: 10.1152/ajplung.00129.2010
209. Favia M, Mancini MT, Bezzerri V, Guerra L, Laselva O, Abbattiscianni AC, et al. Trimethylangelicin promotes the functional rescue of mutant F508del CFTR protein in cystic fibrosis airway cells. Am J Physiol Lung Cell Mol Physiol. (2014) 307:L48–61. doi: 10.1152/ajplung.00305.2013
210. Laselva O, Marzaro G, Vaccarin C, Lampronti I, Tamanini A, Lippi G, et al. Molecular mechanism of action of trimethylangelicin derivatives as CFTR modulators. Front Pharmacol. (2018) 9:719. doi: 10.3389/fphar.2018.00719
211. Cigana C, Nicolis E, Pasetto M, Assael BM, Melotti P. Anti-inflammatory effects of azithromycin in cystic fibrosis airway epithelial cells. Biochem Biophys Res Commun. (2006) 350:977–82. doi: 10.1016/j.bbrc.2006.09.132
212. Tabary O, Escotte S, Couetil JP, Hubert D, Dusser D, Puchelle E, et al. Genistein inhibits constitutive and inducible NF-κB activation and decreases IL-8 production by human cystic fibrosis bronchial gland cells. Am J Pathol. (1999) 155:473–81. doi: 10.1016/S0002-9440(10)65143-7
213. Jouneau S, Bonizec M, Belleguic C, Desrues B, Brinchault G, Galaine J, et al. Anti-inflammatory effect of fluvastatin on IL-8 production induced by Pseudomonas aeruginosa and Aspergillus fumigatus antigens in cystic fibrosis. PLoS ONE. (2011) 6:e22655. doi: 10.1371/journal.pone.0022655
214. Gambari R, Borgatti M, Lampronti I, Fabbri E, Brognara E, Bianchi N, et al. Corilagin is a potent inhibitor of NF-κB activity and downregulates TNF-α induced expression of IL-8 gene in cystic fibrosis IB3-1 cells. Int Immunopharmacol. (2012) 13:308–15. doi: 10.1016/j.intimp.2012.04.010
215. Malcomson B, Wilson H, Veglia E, Thillaiyampalam G, Barsden R, Donegan S, et al. Connectivity mapping (ssCMap) to predict A20-inducing drugs and their antiinflammatory action in cystic fibrosis. Proc Natl Acad Sci USA. (2016) 113:E3725–34. doi: 10.1073/pnas.1520289113
216. Veit G, Bossard F, Goepp J, Verkman AS, Galietta LJ, Hanrahan JW, et al. Proinflammatory cytokine secretion is suppressed by TMEM16A or CFTR channel activity in human cystic fibrosis bronchial epithelia. Mol Biol Cell. (2012) 23:4188–202. doi: 10.1091/mbc.e12-06-0424
217. Ruffin M, Roussel L, Maillé É, Rousseau S, Brochiero E. Vx-809/Vx-770 treatment reduces inflammatory response to Pseudomonas aeruginosa in primary differentiated cystic fibrosis bronchial epithelial cells. Am J Physiol Lung Cell Mol Physiol. (2018) 314:L635–41. doi: 10.1152/ajplung.00198.2017
218. Zeng M, Szymczak M, Ahuja M, Zheng C, Yin H, Swaim W, et al. Restoration of CFTR activity in ducts rescues acinar cell function and reduces inflammation in pancreatic and salivary glands of mice. Gastroenterology. (2017) 153:1148–59. doi: 10.1053/j.gastro.2017.06.011
219. Roesch EA, Nichols DP, Chmiel JF. Inflammation in cystic fibrosis: an update. Pediatr Pulmonol. (2018) 53:S30–50. doi: 10.1002/ppul.24129
Keywords: cystic fibrosis, epithelium, lung, chemotaxis, neutrophil, inflammation
Citation: Cabrini G, Rimessi A, Borgatti M, Lampronti I, Finotti A, Pinton P and Gambari R (2020) Role of Cystic Fibrosis Bronchial Epithelium in Neutrophil Chemotaxis. Front. Immunol. 11:1438. doi: 10.3389/fimmu.2020.01438
Received: 13 January 2020; Accepted: 03 June 2020;
Published: 04 August 2020.
Edited by:
Loic Guillot, Institut National de la Santé et de la Recherche Médicale (INSERM), FranceReviewed by:
Noel Gerard McElvaney, Royal College of Surgeons in Ireland, IrelandAlessandra Livraghi-Butrico, University of North Carolina at Chapel Hill, United States
Copyright © 2020 Cabrini, Rimessi, Borgatti, Lampronti, Finotti, Pinton and Gambari. This is an open-access article distributed under the terms of the Creative Commons Attribution License (CC BY). The use, distribution or reproduction in other forums is permitted, provided the original author(s) and the copyright owner(s) are credited and that the original publication in this journal is cited, in accordance with accepted academic practice. No use, distribution or reproduction is permitted which does not comply with these terms.
*Correspondence: Giulio Cabrini, Z2l1bGlvLmNhYnJpbmlAdW5pZmUuaXQ=; Roberto Gambari, cm9iZXJ0by5nYW1iYXJpQHVuaWZlLml0