- 1Department of Psychiatry, The Second Affiliated Hospital, Zhejiang University School of Medicine, Hangzhou, China
- 2Department of Surgical Oncology, The Second Affiliated Hospital, Zhejiang University School of Medicine, Hangzhou, China
- 3Department of Student Affairs, Zhejiang University School of Medicine, Hangzhou, China
- 4Department of Gastroenterology, Sir Run Run Shaw Hospital, Zhejiang University School of Medicine, Hangzhou, China
- 5State Key Laboratory for Diagnosis and Treatment of Infectious Diseases, Collaborative Innovation Center for Diagnosis and Treatment of Infectious Diseases, The First Affiliated Hospital, College of Medicine, Zhejiang University, Hangzhou, China
- 6Department of Neurosurgery, The Second Affiliated Hospital, Zhejiang University School of Medicine, Hangzhou, China
Emerging evidence demonstrates the critical role of the immune response in the mechanisms relating to mood disorders, such as major depression (MDD) and bipolar disorder (BD). This has cast a spotlight on a specialized branch committed to the research of dynamics of the fine interaction between emotion (or affection) and immune response, which has been termed as “affective immunology.” Inflammatory cytokines and gut microbiota are actively involved in affective immunology. Furthermore, abnormalities of the astrocytes and microglia have been observed in mood disorders from both postmortem and molecular imaging studies; however, the underlying mechanisms remain elusive. Notably, the crosstalk between astrocyte and microglia acts as a mutual and pivotal intermediary factor modulating the immune response posed by inflammatory cytokines and gut microbiota. In this study, we propose the “altered astrocyte-microglia crosstalk (AAMC)” hypothesis which suggests that the astrocyte-microglia crosstalk regulates emotional alteration through mediating immune response, and thus, contributing to the development of mood disorders.
Introduction
Almost a century ago, Julius Wagner-Jauregg first reported the impact of immunological disturbance on psychological function. This finding stirred up researchers' enthusiasm about bidirectional communication between immunological dysfunction and mental disorders. To date, understanding the etiology of mental disorders, such as schizophrenia, autism spectrum disorder, anxiety disorder, as well as mood disorders, is one of the most actively explored topics in immunology (1, 2).
Mood disorders, including major depressive disorder (MDD) and bipolar disorder (BD), are a group of complex debilitating psychiatric illnesses identified by symptoms rather than biological markers. Both of these disorders remain serious health concerns worldwide, owing to their high prevalence, risk for recurrence, and suicide. Further, the mainstream pharmacological treatments—antidepressants and mood stabilizers—are unsatisfactory in treating such patients due to their delayed onset of action, limited efficacy, and vast array of adverse side effects (3, 4). The important reason behind this dilemma is frustratingly limited understanding of the pathological mechanism underlying mood disorders, including affective immunology (5, 6).
To understand the dynamics of the fine interaction between the emotion (or affection) and immune response, a specialized branch called “affective immunology” was recently introduced to distinguish it from “psychoneuroimmunology” which broadly studies the relationship between psychological processes, neuroendocrine activities, and immune systems (7). The high plasticity of the immune system significantly raises the exciting possibility of translational research. In the wake of rapidly accumulating evidence implying the critical role of affective immunity in the cellular and molecular mechanisms underlying the mood disorders, great efforts are ongoing to develop more immunomodulators targeting immune cells (especially microglia) (8, 9), inflammatory cytokines (10, 11), as well as gut microbiota (12, 13).
Microglia are widely known as innate sentinel immune cells that reside in the central nervous system (CNS). These cells respond dynamically to changes in the physical environment and are proven to be key players in affective immunology. Additionally, another common glia, astrocytes, also participate in neuroinflammation by re-sculpting blood-brain barrier (BBB) and releasing inflammatory cytokines. Interestingly, a unique bond between microglia and astrocytes exists, namely the astrocyte-microglia crosstalk, coordinate their functions in neuroinflammatory response (14). Collectively, the astrocyte-microglia crosstalk likely exerts an influence on emotion and affection by regulating the neuroinflammatory response. Hence, we speculate that the altered astrocyte-microglia crosstalk (AAMC) is a primary determinant of mood disorders, thereby a more specific and direct therapeutic target.
In this study, we integrate available data from both preclinical and translational studies regarding affective immunology and highlight the core role of the astrocyte-microglia crosstalk. Furthermore, we intend to discuss the dysregulated crosstalk between astrocytes and microglia and hope to shed some light on potential therapeutic opportunities for treating mood disorders.
Affective Immunology
The Interaction Between Emotion and Immune System
Researchers have revealed that emotion and immune system mirror each other. However, the effect and causality between the two are debatable.
Mounting evidence has shown the beneficial impact of positive emotion (e.g., humor, happiness, and hope) on the immune system. For instance, people with positive emotions showed lower susceptibility to infection (15, 16) and greater immune response (17, 18). This role of “immune enhancer” is mediated by higher levels of antibodies (18) and T cells (19–21), and increased activity of natural killer (NK) cells (19, 22), as well as reduced inflammatory markers including interleukin-6 (IL-6) and C-reactive protein (CRP) (22, 23). In contrast, negative emotions (e.g., sadness, nervousness, worry, loneliness, and fear) and psychological stress are associated with poorer immunological function with lower NK cell cytotoxicity (8), fewer T cells (24), and increased inflammatory markers (25). Importantly, these immune alterations can persist up to 2 h following brief emotional turbulence like stress exposure (26), and it might be stored as immunological memory, thus shedding light on the development of emotional interventions (27).
Contrastingly, some researchers suggest that a dysfunctional immune system can induce emotional changes. For instance, immunotherapy using interferon-alpha (IFN-α) and vaccination can lead to negative emotion along with increased levels of inflammatory cytokines (10, 28, 29). Particularly, these cytokines are postulated to regulate BBB permeability and activity of the hypothalamic-pituitary-adrenal (HPA) axis (30, 31). This thus influences various neuronal events related to emotions including glia-neuron communication, neurotransmission, and synaptic pruning (32, 33). This finding leads to an interesting hypothesis that emotions can act as an “infection defense” to various environmental pathogens (34).
Taken together, bidirectional communication between emotions and the immune system exists, suggesting a striking role of the immune response in the development of mood disorders.
The Compelling Role of Inflammation in Mood Disorders
Aberrant inflammatory processes exert an influence on the progression of mood disorders and also mediate the treatment response. High levels of peripheral inflammatory cytokines and chemokines, including IL-1β, IL-6, IFN-γ, tumor necrosis factor-alpha (TNF-α), and CRP, have been reported in patients with depression (35). In addition, the levels of these cytokines are reduced following an effective antidepressant therapy (36). Conversely, anti-inflammatory treatment results in improvement of depressive symptoms (37). Furthermore, immune-related genes encoding these cytokines have recently been found to be associated with depression (38), thus strongly supporting postulation that inflammatory cytokines are the active regulator of depression. Therefore, inflammatory cytokines are considered as “biomarkers” for diagnosis, susceptibility, and treatment responsiveness for MDD (36). With regards to BD, inflammatory cytokines have been found to be upregulated in both the depressive and manic episodes and return to normal levels in the euthymic state (9, 39). In addition to circulating cytokines, transmembrane TNF-α has also been shown to significantly increase in Brodmann area (BA) 46 for MDD and BA24 for BD (40).
Other than cytokines, the emerging evidence shows altered composition of gut microbiota in both MDD and BD (13, 41, 42). Specifically, Alistipes and Klebsiella are increased in MDD patients, while bacteria belonging to the Lachnospiraceae family are decreased (12, 43). Likewise, an abundance of Lachnospira was reported in the gut of BD patients (44). The altered gut microbiome can not only influence peripheral immune response (45) but can also regulate the neuroinflammation via the vagus nerve and microbial metabolites such as short-chain fatty acids (SCFA), secondary bile acids, serotonin, tryptophan metabolites (46), and neurotransmitter production (e.g., gamma-aminobutyric acid, noradrenaline, dopamine, and acetylcholine) (47, 48). However, since there is large variability in the composition and diversity of gut microbiota between individuals and emotional states, it remains difficult to identify an optimal microbiome profile. A major challenge lies in translating these observations into interventions that could be used to treat mood disorders.
Taken together, the inflammatory cytokines and gut microbiota play compelling roles in the etiology of mood disorders (Figure 1). Particularly, both have profound impacts on the neuroinflammatory response by boosting the activity of microglia and astrocytes (46, 49, 50). In the next section, we present pathological alterations of both microglia and astrocytes in depression and BD, respectively.
Dysfunction of Microglia and Astrocytes in Mood Disorders
Dysfunctional astrocytes and microglia are inextricably intertwined in mood disorders. To date, a growing body of evidence from human autopsy and serum/CSF/imaging biomarkers indicates their abnormalities underlying mood disorders (Table 1). Additionally, distinct gene profiling patterns of these cells have been reported. For instance, the CD206 gene expression pattern of microglia varies between depressive and manic states of BD, suggesting the genetic evidence of microglial dysfunction in BD and the potential of microglial CD206 as a state marker (73).
Dysfunction of Microglia and Astrocytes in Depression
Postmortem brain tissue of suicide victims has provided evidence suggesting enhanced microglial activation in the depressive episode (32, 71, 81). Steiner et al. observed greater human leukocyte antigen, D related (HLA-DR) staining in the dorsolateral prefrontal cortex (PFC) and anterior cingulate cortex (ACC) (71). Subsequently, an increased density of microglial quinolinic acid within ACC was reported in both unipolar and bipolar depression (32). Strikingly, it was found that primed microglia, rather than resting phenotype, were increased in ACC (81). These findings are consistent with the findings of recent molecular imaging studies using translocator protein (TSPO) as a marker of microglial activation (77, 78). Elevated TSPO density was found in PFC, ACC, and insula of patients experiencing major depressive episode (77, 79), especially in those with a long duration of untreated MDD (78). However, an earlier positron emission tomography (PET) imaging studies showed no significant difference in TSPO density between depressive patients and matched controls (76). The discrepancies may be related to the relatively small sample size and high heterogeneity of severity, onset age, and antidepressants used. Collectively, enhanced microglial activation in specific brain regions is a core constituent of depression pathology. Accordingly, inhibition of microglial activation by minocycline administration can lead to an improvement in depressive symptoms in various animal and human studies (31, 82–85). Similarly, blocking the adenosine triphosphate (ATP)-gated P2X7 ion channel of microglia was shown to be a potential, new, and effective antidepressant therapy (86).
Morphological and functional abnormalities of astrocytes have also been seen in patients with depressive episode. A histological study using Golgi-staining found hypertrophic astrocytes with more intricate processes and longer projections within ACC of depressed suicide cases (87), suggesting local low-grade inflammation with reactive astrocytosis. These findings were further confirmed by the observation of weakened BBB with reduced astrocytic endfeet (88) and gap junction proteins (89, 90), which facilitates the recruitment of immune cell and diffusion of pro-inflammatory cytokines (30). In parallel, mounting evidence of astrocyte-specific biomarkers demonstrates the dysfunction of astrocytes in depressive episode. Glial fibrillary acidic protein (GFAP), involved in astrocytic structure and movement, is thought to be upregulated during neuroinflammation (91). However, decreased density of GFAP-positive astrocytes was consistently found in depression-related brain regions, such as PFC, cingulate cortex (55, 58), hippocampus (54), amygdala (51), thalamus, and caudate nuclei (56). Although of less astrocyte-specificity, other markers such as calcium-binding protein S100β (92) and the water channel aquaporin-4 (AQP-4) (93) provided supporting evidence of astrocytic damage (especially neuroprotective phenotype) during dysregulated neuroinflammatory response induced by depressive episode (61, 88). Importantly, possibly due to epigenetic mechanism, maternal depression can result in a profound reduction of astrocyte density in the offspring, as shown in an animal model (94). In summary, the pathological alterations in astrocytes represent a prominent characteristic of depression, which can be reversed using effective antidepressant therapy. Fluoxetine (63, 95, 96), mirtazapine (97), ketamine (98, 99), as well as repetitive high-frequency transcranial magnetic stimulation (TMS) (100) have been shown to have a beneficial impact on astrocytes, paralleled by improvement of depressive symptoms. Additional support for the critical role of astrocytes in depression is derived from recent studies suggesting the therapeutic option for depression via the regulating the activity of astrocytes (101, 102).
Dysfunction of Microglia and Astrocytes in Bipolar Disorder
Although the dysfunction of microglia and astrocytes has also been implicated in the development of BD (103, 104), the picture appears to be more complicated compared to MDD. Human postmortem studies in BD have not yielded consistent results. The majority of them showed an unchanged density of astrocytes and microglia in the frontal cortex (40, 64, 105, 106), ACC (60), amygdala (51, 67, 69), hippocampus (107), entorhinal cortex (59, 67), basal ganglia (57), dorsal raphe nucleus (72), and cerebellum (52). On the contrary, several studies showed positive results. For instance, the level of GFAP has been reported to be increased in BA9 (66) and decreased in BA10 (53), BA24 (63), BA11, and 47 (62). The level of S100β has been reported to be increased in BA40 and decreased in BA9 (68). The expression of CD11b protein, a marker of astrocytic and microglial activation, has been reported to be upregulated in PFC (65) and downregulated in ACC (75).
Obviously, the heterogeneity in terms of brain regions studied (62, 68) and methodology used (40, 66, 107) contributes to the discrepancy in these findings. Additionally, the mixed perimortem states are conceivably confounding factors that cannot be neglected. Some brain tissues were from depressive suicide cases, while most were from patients that died due to physical disease including pneumonia, pulmonary embolism, myocardial infarction, and cerebral hemorrhage which might affect acid-base balance and neuroinflammatory response (64, 72, 107). Also, substance abuse is common in BD and it can influence microglial activity (75). For each subject, the diagnosis of BD was based on the retrospective review of medical records and extensive telephone interviews with relatives, but their comorbidity and phenotype (depressive episode, manic episode or remission state) remain unclear (108). Due to the complexity of BD, studies regarding its diverse phenotypes are requisite to identify trait- or state-related alterations of astrocytes and microglia (5). Similar to unipolar depression, bipolar depression has been found to be related to reduced S100β positive astrocytes in the bilateral hippocampus (CA1 subregion) (61). Nevertheless, no significant difference in GFPA positive astrocyte and HLA-DR positive microglia were found in bipolar depression, which might be ascribed to the relatively small sample size (61, 71). With regards to manic episode, higher levels of peripheral S100β have been observed, implying astrocytic activation (70). Likewise, astrocytic and microglial activation are involved in euthymic patients (74, 80). Jakobsson et al. found increased cerebrospinal fluid (CSF) and serum levels of MCP-1/CCL2 and YKL-40/chitinase-3-like protein 1 (CHI3L1) in patients with mood-stabilized BD (74). Moreover, a PET study revealed microglial activation in hippocampus (80), which is positively related to neuronal integrity (109). Frustratingly, it remains difficult to conclude an absolute statement based on these limited studies.
Dysfunctional astrocytes and microglia reflect abnormal neuroinflammatory response in mood disorders. Below, we will discuss the astrocyte-microglia crosstalk and its pivotal role in affective immunology.
The Astrocyte-Microglia Crosstalk in Neuroinflammation
Overview of the Astrocyte-Microglia Crosstalk
Although both microglia and astrocytes belong to glia cells, they have very different origins; the former are CNS resident macrophages, while the latter are derived from neuroepithelial progenitors and serve as stromal cells (110, 111). To date, numerous cellular and molecular mechanisms of bidirectional communication between them have been shown (49, 112).
Astrocyte-derived IL-1 could activate microglia via permeabilizing the BBB. Besides, astrocytes can release inflammatory cytokines and chemokines, such as IL-15 (113), IL-33 (114), migration inhibitory factor (MIF) (115), and ATP (116), to directly enhance microglial abilities like migration, engulfing apoptotic cells, phagocytosing extracellular matrix, and pruning synapses. Similarly, microglia could influence astrocytic activity by releasing ATP (33), complement factor C1q, IL-1α, TNF (49), transforming growth factor-beta (TGF-β), vascular endothelial growth factor-β (VEGF-β) (50), and insulin-like growth factor 1 (IGF-1) (14). Moreover, astrocytes and microglia communicate by coordinated response using common soluble factors [including norepinephrine (117, 118), purines (119), and circulating bacterial metabolites from gut microbiome (47, 50)], consistently impacting the neuronal activity. Last but not least, there is limited evidence to explain the relatively stable proportions of astrocytes and microglia. Researchers have hypothesized that communication between astrocytes and microglia performs an essential role in balancing their proportionate numbers (120, 121).
Taken together, the microglia and astrocytes function synchronously and complementarily during various physiological and pathological processes (14, 122), including synaptic formation and remolding, BBB regulation, homeostasis, and immune response (123). Once the astrocyte-microglia crosstalk is perturbed, pathological events occur.
The Astrocyte–Microglia Crosstalk During Innate Immune Response
Both astrocytes and microglia actively participate in neuroinflammation by regulating the innate immune system (124). When the microglia sense danger signals with their motile protrusions, they immediately release cytokines and chemokines that lead to reactive astrocytosis. Interestingly, the phenotypes of reactive astrocytes, whether they are neuroprotective or neurotoxic, are determined by microglia-derived pro-inflammatory cytokines according to diverse pathological conditions (49, 119, 125). The neuroprotective reactive astrocytes are induced via purinergic signaling (119). They can release neurotrophic factors and secret proteins, resulting in synaptogenesis and scar formation (119). The scar protects brain tissue from invading of excessive inflammation response. Conversely, the neurotoxic reactive astrocytes increase the expression of multiple genes that are related to tissue damage and induction of proinflammatory mediators (112, 126). Astrocyte-derived proinflammatory molecules can increase BBB permeability, which contributes to the recruitment of immune cells and increased migration and phagocytosis of microglia (30). This thereby amplifies the inflammatory response. Many researches have shown the pivotal nature of lipocalin-2 (LCN2) (127), as well as monocyte chemoattractant protein 1/C-C motif chemokine ligand 2 (MCP-1/CCL2), IFN-γ inducible protein 10/C-X-C motif chemokine ligand 10 (IP-10/CXCL10) (128), complement factor C3 (129), and plasminogen activator inhibitor type 1 (PAI-1) (130) in enhancing microglial activity. Contrarily, at the late stage of inflammation, the reactive astrocytes attenuate microglial activation by orosomucoid-2 (ORM2) (131), TGF-β (132), and glial cell line-derived neurotrophic factor (GDNF) (133), and inhibits the microglial phagocytosis by pentraxin 3 (PTX3) (134), thereby limiting the neuroinflammation. Above all, the astrocyte-microglia crosstalk is crucial for moderating innate immune response; otherwise, the neuroinflammatory response would get out of control (Figure 2).
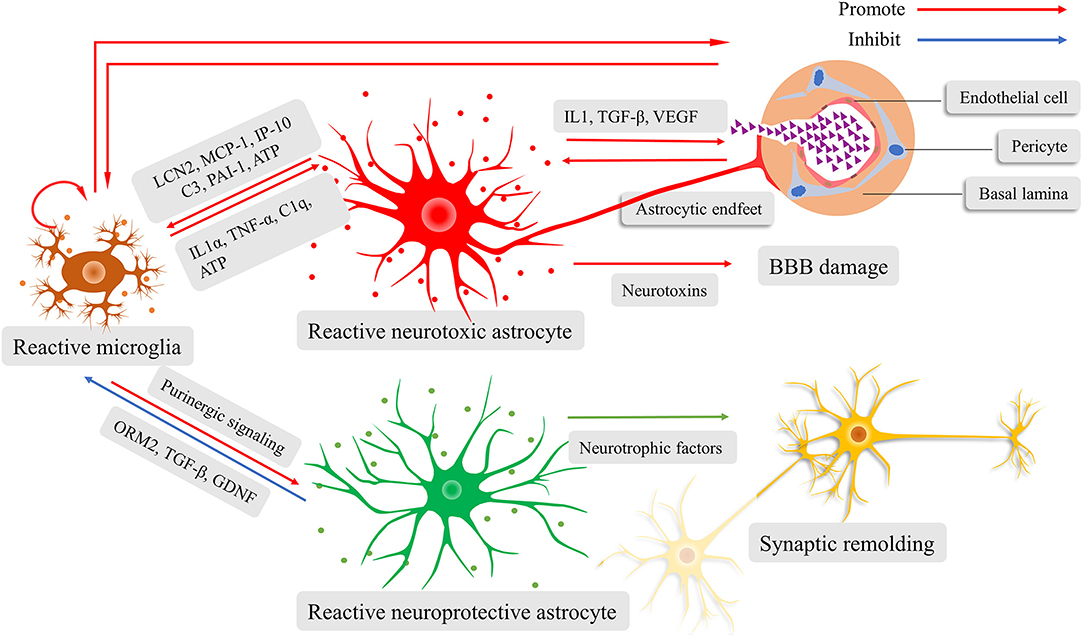
Figure 2. Schematic illustration of the fine interaction between astrocytes and microglia during neuroinflammation. Reactive microglia activate and determine the phenotypes of astrocytes, ranging from neurotoxic to neuroprotective. The reactive neurotoxic astrocytes promote the capacity of microglial activation, motility, and phagocytosis, while weakening the blood-brain barrier (BBB) and prune synapses. Increased BBB permeability facilitates the recruitment of immune cells and diffusion of inflammatory cytokines, amplifying neuroinflammatory response. The reactive neuroprotective astrocyte can lead to microglial inactivation, synaptogenesis, and scar formation.
Blood-Brain Barrier, Gut Microbiota, and the Astrocyte-Microglia Crosstalk
As stated earlier, the alteration in BBB permeability is a key mechanism of regulating neuroinflammatory response. This allows or restricts the entry of immune cells and peripheral inflammatory mediators into the parenchyma of CNS at different stages. As one of the essential components of BBB, astrocytes dynamically regulate its permeability through inflammatory cytokines and connexins on endfeet (135–137). For instance, reactive astrocytes become hypertrophic with a reduced number of connexins to weaken BBB during neuroinflammatory conditions (90). Besides, resting microglia is beneficial for relatively intact BBB, while reactive microglia tend to migrate and induce BBB breakdown (138). Meanwhile, the gut microbiota is essential for the integrity of BBB (139). Once barriers are breached, blood-derived signals, including circulating microbial metabolites from the gut microbiota, can enter the brain and act back on the astrocyte-microglia crosstalk (47, 50).
Specifically, both astrocytes and microglia express the aryl hydrocarbon receptors (AHRs) (50) and can sense tryptophan metabolites (46) and SCFA, respectively (47). The AHRs signaling creates an anti-inflammatory state by balancing the gene expression of TGF-α and VEGF-β (50). Moreover, the normal development of microglia is strongly associated with a full repertoire of gut microbiota in early life (47, 140, 141). Reciprocally, the astrocyte-microglia crosstalk has been found to influence intestinal permeability and microbiome profile (142).
As for mood disorders, overactive astrocyte-microglia crosstalk can increase the permeability of BBB and intestine, which facilitate the diffusion of various inflammatory cytokines and microbial metabolites, and further activate the astrocyte-microglia crosstalk. In this context, the feedforward mechanism amplifies the neuroinflammatory response. Therefore, we postulate AAMC at the heart of affective immunology.
Crosstalk Between Astrocytes and Microglia in the Affective Immunology: Does It Play Key Role?
Given the abundant evidence indicating the significant role of neuroinflammation in affective immunology, including inflammatory cytokines and gut microbiota (38, 42, 43), postmortem studies and molecular imaging researches have revealed that AAMC participates in the development of mood disorders (Figure 1) (56, 72, 78, 80). Despite recent progress, the underlying mechanisms remain elusive. Given that recent data has shown that AAMC serves as an essential mediator of both inflammatory cytokines and gut microbiota (114, 142, 143), we propose the hypothesis that astrocyte-microglia crosstalk triggers emotional alteration through regulating neuroinflammatory response, and thus contributes to mood disorders. In this section, we summarize data from present literature and discuss the crosstalk between astrocytes and microglia, aiming to support our hypothesis. However, the limitations of this hypothesis are also presented, and the suggestions for future research are offered.
As mentioned above, reactive astrocytes and microglia have been reported in mood disorders. Their presence is strongly suggesting of increased neuroinflammatory response (32, 81, 87). Reactive astrocytes become hypertrophic with reduced gap junction proteins, and they release cytokines and chemokines (such as IL-1, VEGF-A, TGF-β, and MIF), which drives BBB disruption and enhances microglial activation, migration, and phagocytosis (88, 90, 112, 135, 137). The reactive microglia make a significant impact on the astrocytic transformation (neuroprotective or neurotoxic phenotypes) and capacity mediating by purine signaling and inflammatory cytokines (40, 49, 119). Moreover, the increased BBB permeability facilitates the infiltration of peripheral immune cells, circulating cytokines and microbial metabolic (30). This can promote astrocytic and microglial activity, thereby amplifying the neuroinflammatory response (46, 50). Indeed, the studies regarding upregulated cortical inflammatory cytokine further support the neuroinflammatory cascade in mood disorders (2, 40). The excessive neuroinflammatory response in mood disorders reflects AAMC, which results in detrimental impacts on the downstream processes of the astrocyte-microglia crosstalk, such as neurotransmission and synaptic remolding (33, 123).
To our knowledge, astrocytes express glutamate receptors and regulate glutamate homeostasis through exocytosis (clearance of excess glutamate) and endocytosis (glutamate re-storage and transportation) (144). Dysfunctional astrocytes account for the imbalanced glutamatergic neurotransmission and hence excitotoxicity seen in mood disorders (65, 116). Arguably, microglia serve as a coordinator of astrocytes in regulating neurotransmission (33). Reactive microglia derive ATP and recruit astrocytes, resulting in an increase of glutamic release (33). In addition to ATP pathway, microglia-derived quinolinic acid known as an N-methyl-D-aspartate (NMDA) receptor agonist has been found to be upregulated, thereby contributing to the high-level glutamate in mood disorders (32). More importantly, inflammatory cytokines derived from AAMC (IL-1β, IL-6, and TNF-α) leads to downregulated expression and functionality of the excitatory amino-acid transporters 2 (EAAT2). This further attenuates the astrocytic ability of buffering and clearing the excessive glutamate (145). Conversely, the NMDA receptor antagonist ketamine can reverse the AAMC in mood disorders and alleviate excitotoxicity during the neuroinflammatory response, and hence exert rapid antidepressant effect (98, 99).
On the other hand, the anti-depressive effect of ketamine should ascribe to a reversal of another downstream process of AAMC-triggered neuroinflammation, the synaptic remolding in mood disorders (63, 123, 146). Inflammatory cytokines produced by astrocytes and microglia, especially IL-1β, TNF-α, and IFN-α, can detrimentally affect the synaptogenesis by regulating the expression of genes involved in synaptic plasticity (123). In addition, complement factors C1q and C3, as well as anti-inflammatory cytokine– TGF-β, are critical mediators of synaptic pruning and refinement (147). The neuroinflammation-driven synaptic remolding results in abnormal neurocircuits in mood disorders (14).
Taken together, these findings suggest the exciting possibility that AAMC can be a promising target for preventing and treating mood disorders. However, there are several limitations to the hypothesis. For example, the other components of BBB, such as endothelial cells and pericytes, are crucial for homeostatic brain and neuroinflammation (30). Besides, the fine interaction between neurons and the astrocyte-microglia crosstalk is vital for mental health (118, 148). Further discussion should take these factors into account to improve the hypothesis.
Conclusion
This study highlights the vital role of the astrocyte-microglia crosstalk in affective immunology and posits that AAMC triggers emotional changes by modulating neuroinflammatory response. This thus contributes to the development of mood disorders. Most of the supporting evidence discussed here comes from human studies. Few animal experiments are cited as proof to elucidate the cellular and molecular mechanism, bearing in mind that animal models are insufficient to reflect the pathophysiology of mood disorders. However, postmortem studies might omit transient pathological alterations of astrocyte-microglia crosstalk seen in mood disorders, due to small sample size and confounding factors including age, disease duration, phenotype, medication use, postmortem interval, and duration of tissue storage. Fortunately, molecular imaging can detect the transient abnormalities of the astrocyte-microglia crosstalk in vivo and hopefully be applied to the individualized treatment of disorders.
Data Availability Statement
The original contributions presented in the study are included in the article/supplementary material, further inquiries can be directed to the corresponding author/s.
Author Contributions
LY, YZ, HJ, and ST wrote the paper and made the original figures. AS, LY, and YQ critically revised the texts and figures. All authors read and approved the final manuscript.
Funding
This work was funded by China Postdoctoral Science Foundation (2017M612010), National Natural Science Foundation of China (81701144 and 81901319), and Zhejiang Province Natural Science Foundation (LQ17H090003).
Conflict of Interest
The authors declare that the research was conducted in the absence of any commercial or financial relationships that could be construed as a potential conflict of interest.
Abbreviations
AAMC, altered astrocyte-microglia crosstalk; CNS, central nervous system; BBB, blood-brain barrier; MCP, monocyte chemoattractant protein; IL, interleukin; TGF, transforming growth factor; NK, nature killer; CRP, C reactive protein; HPA, hypothalamic-pituitary-adrenal; MDD, major depression disorder; BD, bipolar disorder; IFN, interferon; TNF, tumor necrosis factor; SCFA, short-chain fatty acids; MIF, migration inhibitory factor; VEGF, vascular endothelial growth factor; CCL, C-C motif chemokine ligand; GDNF, glial cell line-derived neurotrophic factor; PTX3, Pentraxin 3; AHR, aryl hydrocarbon receptor; PFC, prefrontal cortex; ACC, anterior cingulate cortex; TSPO, translocator protein; GFAP, Glial fibrillary acidic protein; TMS, transcranial magnetic stimulation; CSF, cerebrospinal fluid; BA, Brodmann area; ATP, adenosine triphosphate; IGF-1, insulin-like growth factor 1; LCN2, lipocalin-2; ORM2, orosomucoid-2; IP-10, IFN-γ inducible protein 10; CXCL10, C-X-C motif chemokine ligand 10; PAI-1, plasminogen activator inhibitor type 1; HLA-DR, Human leukocyte antigen D-related; AQP-4, aquaporin-4; CHI3L1, chitinase-3-like protein 1; NMDA, N-methyl-D-aspartate; EAAT2, excitatory amino-acid transporters 2.
References
1. Drexhage RC, Knijff EM, Padmos RC, Heul-Nieuwenhuijzen LVD, Beumer W, Versnel MA, et al. The mononuclear phagocyte system and its cytokine inflammatory networks in schizophrenia and bipolar disorder. Expert Rev Neurother. (2010) 10:59–76. doi: 10.1586/ern.09.144
2. Kovacs D, Eszlari N, Petschner P, Pap D, Vas S, Kovacs P, et al. Effects of IL1B single nucleotide polymorphisms on depressive and anxiety symptoms are determined by severity and type of life stress. Brain Behav Immun. (2016) 56:96–104. doi: 10.1016/j.bbi.2016.02.012
3. O'Leary OF Dinan TG, Cryan JF. Faster, better, stronger: towards new antidepressant therapeutic strategies. Eur J Pharmacol. (2015) 753:32–50. doi: 10.1016/j.ejphar.2014.07.046
4. Grande I, Berk M, Birmaher B, Vieta E. Bipolar disorder. Lancet (London, England). (2016) 387:1561–72. doi: 10.1016/S0140-6736(15)00241-X
5. Harrison PJ, Geddes JR, Tunbridge EM. The emerging neurobiology of bipolar disorder. Trends Neurosci. (2018) 41:18–30. doi: 10.1016/j.tins.2017.10.006
6. Cruz-Pereira JS, Rea K, Nolan YM, O'Leary OF Dinan TG, Cryan JF. Depression's unholy trinity: dysregulated stress, immunity, and the microbiome. Annu Rev Psychol. (2020) 71:49–78. doi: 10.1146/annurev-psych-122216-011613
7. D'Acquisto F. Affective immunology: where emotions and the immune response converge. Dialogues Clin Neurosci. (2017) 19:9–19.
8. Lutgendorf SK, Reimer TT, Harvey JH, Marks G, Hong SY, Hillis SL, et al. Effects of housing relocation on immunocompetence and psychosocial functioning in older adults. J Gerontol A Biol Sci Med Sci. (2001) 56:M97–M105. doi: 10.1093/gerona/56.2.M97
9. Furlan R, Melloni E, Finardi A, Vai B, Di Toro S, Aggio V, et al. Natural killer cells protect white matter integrity in bipolar disorder. Brain Behav Immun. (2019) 81:410–21. doi: 10.1016/j.bbi.2019.06.037
10. Capuron L, Miller AH. Cytokines and psychopathology: lessons from interferon-alpha. Biol Psychiatry. (2004) 56:819–24. doi: 10.1016/j.biopsych.2004.02.009
11. Köhler CA, Freitas TH, Stubbs B, Maes M, Solmi M, Veronese N, et al. Peripheral alterations in cytokine and chemokine levels after antidepressant drug treatment for major depressive disorder: systematic review and meta-analysis. Mol Neurobiol. (2018) 55:4195–206. doi: 10.1007/s12035-017-0632-1
12. Naseribafrouei A, Hestad K, Avershina E, Sekelja M, Linløkken A, Wilson R, et al. Correlation between the human fecal microbiota and depression. Neurogastroenterol Moti. (2014) 26:1155–62. doi: 10.1111/nmo.12378
13. Painold A, Mörkl S, Kashofer K, Halwachs B, Dalkner N, Bengesser S, et al. A step ahead: Exploring the gut microbiota in inpatients with bipolar disorder during a depressive episode. Bipolar Disord. (2019) 21:40–9. doi: 10.1111/bdi.12682
14. Vainchtein ID, Molofsky AV. Astrocytes and microglia: in sickness and in health. Trends Neurosci. (2020) 43:144–54. doi: 10.1016/j.tins.2020.01.003
15. Cohen S, Doyle WJ, Turner RB, Alper CM, Skoner DP. Emotional style and susceptibility to the common cold. Psychosom Med. (2003) 65:652–7. doi: 10.1097/01.PSY.0000077508.57784.DA
16. Cohen S, Alper CM, Doyle WJ, Treanor JJ, Turner RB. Positive emotional style predicts resistance to illness after experimental exposure to rhinovirus or influenza a virus. Psychosom Med. (2006) 68:809–15. doi: 10.1097/01.psy.0000245867.92364.3c
17. Marsland AL, Cohen S, Rabin BS, Manuck SB. Trait positive affect and antibody response to hepatitis B vaccination. Brain Behav Immun. (2006) 20:261–9. doi: 10.1016/j.bbi.2005.08.009
18. Ayling K, Fairclough L, Tighe P, Todd I, Halliday V, Garibaldi J, et al. Positive mood on the day of influenza vaccination predicts vaccine effectiveness: a prospective observational cohort study. Brain Behav Immun. (2018) 67:314–23. doi: 10.1016/j.bbi.2017.09.008
19. Segerstrom SC, Taylor SE, Kemeny ME, Fahey JL. Optimism is associated with mood, coping, and immune change in response to stress. J Pers Soc Psychol. (1998) 74:1646–55. doi: 10.1037/0022-3514.74.6.1646
20. Major B, Rattazzi L, Brod S, Pilipović I, Leposavić G, D'Acquisto F. Massage-like stroking boosts the immune system in mice. Sci Rep. (2015) 5:10913. doi: 10.1038/srep10913
21. Rattazzi L, Piras G, Brod S, Smith K, Ono M, D'Acquisto F. Impact of enriched environment on murine T cell differentiation and gene expression profile. Front Immunol. (2016) 7:381. doi: 10.3389/fimmu.2016.00381
22. Fang CY, Reibel DK, Longacre ML, Rosenzweig S, Campbell DE, Douglas SD. Enhanced psychosocial well-being following participation in a mindfulness-based stress reduction program is associated with increased natural killer cell activity. J Altern Compl Med. (2010) 16:531–8. doi: 10.1089/acm.2009.0018
23. Steptoe A, O'Donnell K, Badrick E, Kumari M, Marmot M. Neuroendocrine and inflammatory factors associated with positive affect in healthy men and women: the Whitehall II study. Am J Epidemiol. (2008) 167:96–102. doi: 10.1093/aje/kwm252
24. Herbert TB, Cohen S. Stress and immunity in humans: a meta-analytic review. Psychosom Med. (1993) 55:364–79. doi: 10.1097/00006842-199307000-00004
25. Steptoe A, Hamer M, Chida Y. The effects of acute psychological stress on circulating inflammatory factors in humans: a review and meta-analysis. Brain Behav Immun. (2007) 21:901–12. doi: 10.1016/j.bbi.2007.03.011
26. Marsland AL, Walsh C, Lockwood K, John-Henderson NA. The effects of acute psychological stress on circulating and stimulated inflammatory markers: a systematic review and meta-analysis. Brain Behav Immun. (2017) 64:208–19. doi: 10.1016/j.bbi.2017.01.011
27. Lewitus GM, Schwartz M. Behavioral immunization: immunity to self-antigens contributes to psychological stress resilience. Mol Psychiatry. (2009) 14:532–6. doi: 10.1038/mp.2008.103
28. Brydon L, Harrison NA, Walker C, Steptoe A, Critchley HD. Peripheral inflammation is associated with altered substantia nigra activity and psychomotor slowing in humans. Biol Psychiatry. (2008) 63:1022–9. doi: 10.1016/j.biopsych.2007.12.007
29. Eisenberger NI, Berkman ET, Inagaki TK, Rameson LT, Mashal NM, Irwin MR. Inflammation-induced anhedonia: endotoxin reduces ventral striatum responses to reward. Biol Psychiatry. (2010) 68:748–54. doi: 10.1016/j.biopsych.2010.06.010
30. Abbott NJ, Patabendige AA, Dolman DE, Yusof SR, Begley DJ. Structure and function of the blood-brain barrier. Neurobiol Dis. (2010) 37:13–25. doi: 10.1016/j.nbd.2009.07.030
31. Majidi J, Kosari-Nasab M, Salari A-A. Developmental minocycline treatment reverses the effects of neonatal immune activation on anxiety- and depression-like behaviors, hippocampal inflammation, and HPA axis activity in adult mice. Brain Res Bull. (2016) 120:1–13. doi: 10.1016/j.brainresbull.2015.10.009
32. Steiner J, Walter M, Gos T, Guillemin GJ, Bernstein HG, Sarnyai Z, et al. Severe depression is associated with increased microglial quinolinic acid in subregions of the anterior cingulate gyrus: evidence for an immune-modulated glutamatergic neurotransmission? Neurol Psych Brain Res. (2011) 8:94. doi: 10.1016/j.npbr.2012.02.043
33. Pascual O, Ben Achour S, Rostaing P, Triller A, Bessis A. Microglia activation triggers astrocyte-mediated modulation of excitatory neurotransmission. Proc Natl Acad Sci USA. (2012) 109:E197–205. doi: 10.1073/pnas.1111098109
34. Anders S, Tanaka M, Kinney DK. Depression as an evolutionary strategy for defense against infection. Brain Behav Immun. (2013) 31:9–22. doi: 10.1016/j.bbi.2012.12.002
35. Dowlati Y, Herrmann N, Swardfager W, Liu H, Sham L, Reim EK, et al. A meta-analysis of cytokines in major depression. Biol Psychiatry. (2010) 67:446–57. doi: 10.1016/j.biopsych.2009.09.033
36. Wang L, Wang R, Liu L, Qiao D, Baldwin DS, Hou R. Effects of SSRIs on peripheral inflammatory markers in patients with major depressive disorder: a systematic review and meta-analysis. Brain Behav Immun. (2019) 79:24–38. doi: 10.1016/j.bbi.2019.02.021
37. Raison CL, Rutherford RE, Woolwine BJ, Shuo C, Schettler P, Drake DF, et al. A randomized controlled trial of the tumor necrosis factor antagonist infliximab for treatment-resistant depression: the role of baseline inflammatory biomarkers. JAMA Psychiatry. (2013) 70:31–41. doi: 10.1001/2013.jamapsychiatry.4
38. Barnes J, Mondelli V, Pariante CM. Genetic contributions of inflammation to depression. Neuropsychopharmacology. (2017) 42:81–98. doi: 10.1038/npp.2016.169
39. Maletic V, Raison C. Integrated neurobiology of bipolar disorder. Front Psychiatry. (2014) 5:98. doi: 10.3389/fpsyt.2014.00098
40. Dean B, Gibbons AS, Tawadros N, Brooks L, Everall IP, Scarr E. Different changes in cortical tumor necrosis factor-α-related pathways in schizophrenia and mood disorders. Mol Psychiatry. (2013) 18:767–73. doi: 10.1038/mp.2012.95
41. Aizawa E, Tsuji H, Asahara T, Takahashi T, Teraishi T, Yoshida S, et al. Possible association of Bifidobacterium and Lactobacillus in the gut microbiota of patients with major depressive disorder. J Affect Disord. (2016) 202:254–7. doi: 10.1016/j.jad.2016.05.038
42. Coello K, Hansen TH, Sørensen N, Munkholm K, Kessing LV, Pedersen O, et al. Gut microbiota composition in patients with newly diagnosed bipolar disorder and their unaffected first-degree relatives. Brain Behav Immun. (2019) 75:112–8. doi: 10.1016/j.bbi.2018.09.026
43. Jiang H, Ling Z, Zhang Y, Mao H, Ma Z, Yin Y, et al. Altered fecal microbiota composition in patients with major depressive disorder. Brain Behav Immun. (2015) 48:186–94. doi: 10.1016/j.bbi.2015.03.016
44. Flowers SA, Evans SJ, Ward KM, McInnis MG, Ellingrod VL. Interaction between atypical antipsychotics and the gut microbiome in a bipolar disease cohort. Pharmacotherapy. (2017) 37:261–7. doi: 10.1002/phar.1890
45. El Aidy S, Dinan TG, Cryan JF. Gut microbiota: the conductor in the orchestra of immune-neuroendocrine communication. Clin Ther. (2015) 37:954–67. doi: 10.1016/j.clinthera.2015.03.002
46. Rothhammer V, Mascanfroni ID, Bunse L, Takenaka MC, Kenison JE, Mayo L, et al. Type I interferons and microbial metabolites of tryptophan modulate astrocyte activity and central nervous system inflammation via the aryl hydrocarbon receptor. Nat Med. (2016) 22:586–97. doi: 10.1038/nm.4106
47. Erny D, Hrabe de Angelis AL, Jaitin D, Wieghofer P, Staszewski O, David E, et al. Host microbiota constantly control maturation function of microglia in the CNS. Nat Neurosci. (2015) 18:965–77. doi: 10.1038/nn.4030
48. Sarkar A, Lehto SM, Harty S, Dinan TG, Cryan JF, Burnet PWJ. Psychobiotics and the manipulation of bacteria-gut-brain signals. Trends Neurosci. (2016) 39:763–81. doi: 10.1016/j.tins.2016.09.002
49. Liddelow SA, Guttenplan KA, Clarke LE, Bennett FC, Bohlen CJ, Schirmer L, et al. Neurotoxic reactive astrocytes are induced by activated microglia. Nature. (2017) 541:481–7. doi: 10.1038/nature21029
50. Rothhammer V, Borucki DM, Tjon EC, Takenaka MC, Chao CC, Ardura-Fabregat A, et al. Microglial control of astrocytes in response to microbial metabolites. Nature. (2018) 557:724–8. doi: 10.1038/s41586-018-0119-x
51. Altshuler LL, Abulseoud OA, Foland-Ross L, Bartzokis G, Chang S, Mintz J, et al. Amygdala astrocyte reduction in subjects with major depressive disorder but not bipolar disorder. Bipolar Disord. (2010) 12:541–9. doi: 10.1111/j.1399-5618.2010.00838.x
52. Fatemi SH, Laurence JA, Araghi-Niknam M, Stary JM, Schulz SC, Lee S, et al. Glial fibrillary acidic protein is reduced in cerebellum of subjects with major depression, but not schizophrenia. Schizophr Res. (2004) 69:317–23. doi: 10.1016/j.schres.2003.08.014
53. Johnston-Wilson NL, Sims CD, Hofmann JP, Anderson L, Shore AD, Torrey EF, et al. Disease-specific alterations in frontal cortex brain proteins in schizophrenia, bipolar disorder, and major depressive disorder. Stanley Neuropathol Consortium Mol Psychiatry. (2000) 5:142–9. doi: 10.1038/sj.mp.4000696
54. Cobb JA, O'Neill K, Milner J, Mahajan GJ, Lawrence TJ, May WL, et al. Density of GFAP-immunoreactive astrocytes is decreased in left hippocampi in major depressive disorder. Neuroscience. (2016) 316:209–20. doi: 10.1016/j.neuroscience.2015.12.044
55. Gittins RA, Harrison PJ. A morphometric study of glia and neurons in the anterior cingulate cortex in mood disorder. J Affect Disord. (2011) 133:328–32. doi: 10.1016/j.jad.2011.03.042
56. Torres-Platas SG, Nagy C, Wakid M, Turecki G, Mechawar N. Glial fibrillary acidic protein is differentially expressed across cortical and subcortical regions in healthy brains and downregulated in the thalamus and caudate nucleus of depressed suicides. Mol Psychiatry. (2016) 21:509–15. doi: 10.1038/mp.2015.65
57. Barley K, Dracheva S, Byne W. Subcortical oligodendrocyte- and astrocyte-associated gene expression in subjects with schizophrenia, major depression and bipolar disorder. Schizophr Res. (2009) 112:54–64. doi: 10.1016/j.schres.2009.04.019
58. Webster MJ, O'Grady J, Kleinman JE, Weickert CS. Glial fibrillary acidic protein mRNA levels in the cingulate cortex of individuals with depression, bipolar disorder and schizophrenia. Neuroscience. (2005) 133:453–61. doi: 10.1016/j.neuroscience.2005.02.037
59. Damadzic R, Bigelow LB, Krimer LS, Goldenson DA, Saunders RC, Kleinman JE, et al. A quantitative immunohistochemical study of astrocytes in the entorhinal cortex in schizophrenia, bipolar disorder and major depression: absence of significant astrocytosis. Brain Res Bull. (2001) 55:611–8. doi: 10.1016/S0361-9230(01)00529-9
60. Williams MR, Hampton T, Pearce RKB, Hirsch SR, Ansorge O, Thom M, et al. Astrocyte decrease in the subgenual cingulate and callosal genu in schizophrenia. Eur Arch Psychiatry Clin Neurosci. (2013) 263:41–52. doi: 10.1007/s00406-012-0328-5
61. Gos T, Schroeter ML, Lessel W, Bernstein H-G, Dobrowolny H, Schiltz K, et al. S100B-immunopositive astrocytes and oligodendrocytes in the hippocampus are differentially afflicted in unipolar and bipolar depression: a postmortem study. J Psychiatr Res. (2013) 47:1694–9. doi: 10.1016/j.jpsychires.2013.07.005
62. Toro CT, Hallak JE, Dunham JS, Deakin JF. Glial fibrillary acidic protein and glutamine synthetase in subregions of prefrontal cortex in schizophrenia and mood disorder. Neurosci Lett. (2006) 404:276–81. doi: 10.1016/j.neulet.2006.05.067
63. Czéh B, Simon M, Schmelting B, Hiemke C, Fuchs E. Astroglial plasticity in the hippocampus is affected by chronic psychosocial stress and concomitant fluoxetine treatment. Neuropsychopharmacology. (2006) 31:1616–26. doi: 10.1038/sj.npp.1300982
64. Hercher C, Chopra V, Beasley CL. Evidence for morphological alterations in prefrontal white matter glia in schizophrenia and bipolar disorder. J Psychiatry Neurosc. (2014) 39:376–85. doi: 10.1503/jpn.130277
65. Rao JS, Harry GJ, Rapoport SI, Kim HW. Increased excitotoxicity and neuroinflammatory markers in postmortem frontal cortex from bipolar disorder patients. Mol Psychiatry. (2010) 15:384–92. doi: 10.1038/mp.2009.47
66. Feresten AH, Barakauskas V, Ypsilanti A, Barr AM, Beasley CL. Increased expression of glial fibrillary acidic protein in prefrontal cortex in psychotic illness. Schizophr Res. (2013) 150:252–7. doi: 10.1016/j.schres.2013.07.024
67. Pantazopoulos H, Woo T-UW, Lim MP, Lange N, Berretta S. Extracellular matrix-glial abnormalities in the amygdala and entorhinal cortex of subjects diagnosed with schizophrenia. Arch Gen Psychiatry. (2010) 67:155–66. doi: 10.1001/archgenpsychiatry.2009.196
68. Dean B, Gray L, Scarr E. Regionally specific changes in levels of cortical S100beta in bipolar 1 disorder but not schizophrenia. Aust N Z J Psychiatry. (2006) 40:217–24. doi: 10.1111/j.1440-1614.2006.01777.x
69. Hamidi M, Drevets WC, Price JL. Glial reduction in amygdala in major depressive disorder is due to oligodendrocytes. Biol Psychiatry. (2004) 55:563–9. doi: 10.1016/j.biopsych.2003.11.006
70. da Rosa MI, Simon C, Grande AJ, Barichello T, Oses JP, Quevedo J. Serum S100B in manic bipolar disorder patients: Systematic review and meta-analysis. J Affect Disord. (2016) 206:210–5. doi: 10.1016/j.jad.2016.07.030
71. Steiner J, Bielau H, Brisch R, Danos P, Ullrich O, Mawrin C, et al. Immunological aspects in the neurobiology of suicide: elevated microglial density in schizophrenia and depression is associated with suicide. J Psychiatr Res. (2008) 42:151–7. doi: 10.1016/j.jpsychires.2006.10.013
72. Brisch R, Steiner J, Mawrin C, Krzyzanowska M, Jankowski Z, Gos T. Microglia in the dorsal raphe nucleus plays a potential role in both suicide facilitation and prevention in affective disorders. Eur Arch Psychiatry Clin Neurosci. (2017) 267:403–15. doi: 10.1007/s00406-017-0774-1
73. Ohgidani M, Kato TA, Haraguchi Y, Matsushima T, Mizoguchi Y, Murakawa-Hirachi T, et al. Microglial CD206 gene has potential as a state marker of bipolar disorder. Front Immunol. (2017) 7:676–676. doi: 10.3389/fimmu.2016.00676
74. Jakobsson J, Bjerke M, Sahebi S, Isgren A, Ekman CJ, Sellgren C, et al. Monocyte and microglial activation in patients with mood-stabilized bipolar disorder. J Psychiatry Neurosci. (2015) 40:250–8. doi: 10.1503/jpn.140183
75. Seredenina T, Sorce S, Herrmann FR, Ma Mulone XJ, Plastre O, Aguzzi A, et al. Decreased NOX2 expression in the brain of patients with bipolar disorder: association with valproic acid prescription and substance abuse. Transl Psychiatry. (2017) 7:e1206. doi: 10.1038/tp.2017.175
76. Hannestad J, DellaGioia N, Gallezot J-D, Lim K, Nabulsi N, Esterlis I, et al. The neuroinflammation marker translocator protein is not elevated in individuals with mild-to-moderate depression: a [12C]PBR28 PET study. Brain Behav Immun. (2013) 33:131–8. doi: 10.1016/j.bbi.2013.06.010
77. Setiawan E, Wilson AA, Mizrahi R, Rusjan PM, Miler L, Rajkowska G, et al. Role of translocator protein density, a marker of neuroinflammation, in the brain during major depressive episodes. JAMA Psychiatry. (2015) 72:268–75. doi: 10.1001/jamapsychiatry.2014.2427
78. Setiawan E, Attwells S, Wilson AA, Mizrahi R, Rusjan PM, Miler L, et al. Association of translocator protein total distribution volume with duration of untreated major depressive disorder: a cross-sectional study. Lancet Psychiatry. (2018) 5:339–47. doi: 10.1016/S2215-0366(18)30048-8
79. Su L, Faluyi YO, Hong YT, Fryer TD, Mak E, Gabel S, et al. Neuroinflammatory and morphological changes in late-life depression: the NIMROD study. Br J Psychiatry. (2016) 209:525–6. doi: 10.1192/bjp.bp.116.190165
80. Haarman BCMB, Riemersma-Van der Lek RF, de Groot JC, Ruhé HGE, Klein HC, Zandstra TE, et al. Neuroinflammation in bipolar disorder - A [(11)C]-(R)-PK11195 positron emission tomography study. Brain Behav Immun. (2014) 40:219–25. doi: 10.1016/j.bbi.2014.03.016
81. Torres-Platas SG, Cruceanu C, Chen GG, Turecki G, Mechawar N. Evidence for increased microglial priming and macrophage recruitment in the dorsal anterior cingulate white matter of depressed suicides. Brain Behav Immun. (2014) 42:50–9. doi: 10.1016/j.bbi.2014.05.007
82. Henry CJ, Huang Y, Wynne A, Hanke M, Himler J, Bailey MT, et al. Minocycline attenuates lipopolysaccharide (LPS)-induced neuroinflammation, sickness behavior, and anhedonia. J Neuroinflammation. (2008) 5:15. doi: 10.1186/1742-2094-5-15
83. Husain MI, Chaudhry IB, Husain N, Khoso AB, Rahman RR, Hamirani MM, et al. Minocycline as an adjunct for treatment-resistant depressive symptoms: a pilot randomised placebo-controlled trial. J Psychopharmacol (Oxford). (2017) 31:1166–75. doi: 10.1177/0269881117724352
84. Wang H-T, Huang F-L, Hu Z-L, Zhang W-J, Qiao X-Q, Huang Y-Q, et al. Early-life social isolation-induced depressive-like behavior in rats results in microglial activation and neuronal histone methylation that are mitigated by minocycline. Neurotox Res. (2017) 31:505–20. doi: 10.1007/s12640-016-9696-3
85. Savitz JB, Teague TK, Misaki M, Macaluso M, Wurfel BE, Meyer M, et al. Treatment of bipolar depression with minocycline and/or aspirin: an adaptive, 2 × 2 double-blind, randomized, placebo-controlled, phase IIA clinical trial. Transl Psychiatry. (2018) 8:27. doi: 10.1038/s41398-017-0073-7
86. Bhattacharya A, Lord B, Grigoleit J-S, He Y, Fraser I, Campbell SN, et al. Neuropsychopharmacology of JNJ-55308942: evaluation of a clinical candidate targeting P2X7 ion channels in animal models of neuroinflammation and anhedonia. Neuropsychopharmacology. (2018) 43:2586–96. doi: 10.1038/s41386-018-0141-6
87. Torres-Platas SG, Hercher C, Davoli MA, Maussion G, Labont é B, Turecki G, et al. Astrocytic hypertrophy in anterior cingulate white matter of depressed suicides. Neuropsychopharmacology. (2011) 36:2650–8. doi: 10.1038/npp.2011.154
88. Rajkowska G, Hughes J, Stockmeier CA, Javier Miguel-Hidalgo J, Maciag D. Coverage of blood vessels by astrocytic endfeet is reduced in major depressive disorder. Biol Psychiatry. (2013) 73:613–21. doi: 10.1016/j.biopsych.2012.09.024
89. Ernst C, Nagy C, Kim S, Yang JP, Deng X, Hellstrom IC, et al. Dysfunction of astrocyte connexins 30 and 43 in dorsal lateral prefrontal cortex of suicide completers. Biol Psychiatry. (2011) 70:312–9. doi: 10.1016/j.biopsych.2011.03.038
90. Ezan P, André P, Cisternino S, Saubaméa B, Boulay A-C, Doutremer S, et al. Deletion of astroglial connexins weakens the blood-brain barrier. J Cerebral Blood Flow Metab.(2012) 32:1457–67. doi: 10.1038/jcbfm.2012.45
91. Middeldorp J, Hol EM. GFAP in health and disease. Progress Neurobiol. (2011) 93:421–43. doi: 10.1016/j.pneurobio.2011.01.005
92. Steiner J, Bernstein H-G, Bielau H, Berndt A, Brisch R, Mawrin C, et al. Evidence for a wide extra-astrocytic distribution of S100B in human brain. BMC Neurosci. (2007) 8:2–2. doi: 10.1186/1471-2202-8-2
93. Amiry-Moghaddam M, Ottersen OP. The molecular basis of water transport in the brain. Nat Rev Neurosci. (2003) 4:991–1001. doi: 10.1038/nrn1252
94. Gong Y, Sun X-L, Wu F-F, Su C-J, Ding J-H, Hu G. Female early adult depression results in detrimental impacts on the behavioral performance and brain development in offspring. CNS Neurosci Ther. (2012) 18:461–70. doi: 10.1111/j.1755-5949.2012.00324.x
95. Kinoshita M, Hirayama Y, Fujishita K, Shibata K, Shinozaki Y, Shigetomi E, et al. Anti-depressant fluoxetine reveals its therapeutic effect via astrocytes. EBioMedicine. (2018) 32:72–83. doi: 10.1016/j.ebiom.2018.05.036
96. Shu X, Sun Y, Sun X, Zhou Y, Bian Y, Shu Z, et al. The effect of fluoxetine on astrocyte autophagy flux and injured mitochondria clearance in a mouse model of depression. Cell Death Dis. (2019) 10:577. doi: 10.1038/s41419-019-1813-9
97. Hisaoka-Nakashima K, Taki S, Watanabe S, Nakamura Y, Nakata Y, Morioka N. Mirtazapine increases glial cell line-derived neurotrophic factor production through lysophosphatidic acid 1 receptor-mediated extracellular signal-regulated kinase signaling in astrocytes. Eur J Pharmacol. (2019) 860:172539. doi: 10.1016/j.ejphar.2019.172539
98. Wang Y, Xie L, Gao C, Zhai L, Zhang N, Guo L. Astrocytes activation contributes to the antidepressant-like effect of ketamine but not scopolamine. Pharmacol Biochem Behav. (2018) 170:1–8. doi: 10.1016/j.pbb.2018.05.001
99. Lasi č E, Lisjak M, Horvat A, BoŽić M, Šakanović A, Anderluh G, et al. Astrocyte specific remodeling of plasmalemmal cholesterol composition by ketamine indicates a new mechanism of antidepressant action. Sci Rep. (2019) 9:10957. doi: 10.1038/s41598-019-47459-z
100. Xue S-S, Xue F, Ma Q-R, Wang S-Q, Wang Y, Tan Q-R, et al. Repetitive high-frequency transcranial magnetic stimulation reverses depressive-like behaviors and protein expression at hippocampal synapses in chronic unpredictable stress-treated rats by enhancing endocannabinoid signaling. Pharmacol Biochem Behav. (2019) 184:172738–172738. doi: 10.1016/j.pbb.2019.172738
101. Wang Y, Ni J, Zhai L, Gao C, Xie L, Zhao L, et al. Inhibition of activated astrocyte ameliorates lipopolysaccharide- induced depressive-like behaviors. J Affect Disord. (2019) 242:52–9. doi: 10.1016/j.jad.2018.08.015
102. Portal B, Delcourte S, Rovera R, Lejards C, Bullich S, Malnou CE, et al. Genetic and pharmacological inactivation of astroglial connexin 43 differentially influences the acute response of antidepressant and anxiolytic drugs. Acta Physiol. (2020) e13440. doi: 10.1111/apha.13440
103. Réus GZ, Fries GR, Stertz L, Badawy M, Passos IC, Barichello T, et al. The role of inflammation and microglial activation in the pathophysiology of psychiatric disorders. Neuroscience. (2015) 300:141–54. doi: 10.1016/j.neuroscience.2015.05.018
104. Peng L, Li B, Verkhratsky A. Targeting astrocytes in bipolar disorder. Expert Rev Neurother. (2016) 16:649–57. doi: 10.1586/14737175.2016.1171144
105. Tkachev D, Mimmack ML, Ryan MM, Wayland M, Freeman T, Jones PB, et al. Oligodendrocyte dysfunction in schizophrenia and bipolar disorder. Lancet (London, England). (2003) 362:798–805. doi: 10.1016/S0140-6736(03)14289-4
106. Wesseling H, Gottschalk MG, Bahn S. Targeted multiplexed selected reaction monitoring analysis evaluates protein expression changes of molecular risk factors for major psychiatric disorders. Int J Neuropsychopharmacol. (2014) 18:pyu015. doi: 10.1093/ijnp/pyu015
107. Malchow B, Strocka S, Frank F, Bernstein H-G, Steiner J, Schneider-Axmann T, et al. Stereological investigation of the posterior hippocampus in affective disorders. J Neural Transmission. (2015) 122:1019–33. doi: 10.1007/s00702-014-1316-x
108. Woo T-UW, Walsh JP, Benes FM. Density of glutamic acid decarboxylase 67 messenger RNA-containing neurons that express the N-methyl-D-aspartate receptor subunit NR2A in the anterior cingulate cortex in schizophrenia and bipolar disorder. Arch Gen Psychiatry. (2004) 61:649–57. doi: 10.1001/archpsyc.61.7.649
109. Haarman BCMB, Burger H, Doorduin J, Renken RJ, Sibeijn-Kuiper AJ, Marsman J-BC, et al. Volume, metabolites and neuroinflammation of the hippocampus in bipolar disorder - a combined magnetic resonance imaging and positron emission tomography study. Brain Behav Immun. (2016) 56:21–33. doi: 10.1016/j.bbi.2015.09.004
110. Ginhoux F, Prinz M. Origin of microglia: current concepts and past controversies. Cold Spring Harb Perspect Biol. (2015) 7:a020537. doi: 10.1101/cshperspect.a020537
111. Molofsky AV, Deneen B. Astrocyte development: a guide for the perplexed. Glia. (2015) 63:1320–9. doi: 10.1002/glia.22836
112. Jha MK, Lee WH, Suk K. Functional polarization of neuroglia: implications in neuroinflammation and neurological disorders. Biochem Pharmacol. (2016) 103:1–16. doi: 10.1016/j.bcp.2015.11.003
113. Shi SX, Li YJ, Shi K, Wood K, Ducruet AF, Liu Q. IL (Interleukin)-15 Bridges Astrocyte-Microglia Crosstalk and Exacerbates Brain Injury Following Intracerebral Hemorrhage. Stroke, Strokeaha. (2020) 51, 967–74. doi: 10.1161/STROKEAHA.119.028638
114. Vainchtein ID, Chin G, Cho FS, Kelley KW, Miller JG, Chien EC, et al. Astrocyte-derived interleukin-33 promotes microglial synapse engulfment and neural circuit development. Science (New York, NY). (2018) 359:1269–73. doi: 10.1126/science.aal3589
115. Petralia MC, Mazzon E, Fagone P, Basile MS, Lenzo V, Quattropani MC, et al. Pathogenic contribution of the Macrophage migration inhibitory factor family to major depressive disorder and emerging tailored therapeutic approaches. J Affect Disord. (2020) 263:15–24. doi: 10.1016/j.jad.2019.11.127
116. Bennett MR, Farnell L, Gibson WG. P2X7 regenerative-loop potentiation of glutamate synaptic transmission by microglia and astrocytes. J Theor Biol. (2009) 261:1–16. doi: 10.1016/j.jtbi.2009.07.024
117. Paukert M, Agarwal A, Cha J, Doze VA, Kang JU, Bergles DE. Norepinephrine controls astroglial responsiveness to local circuit activity. Neuron. (2014) 82:1263–70. doi: 10.1016/j.neuron.2014.04.038
118. Liu Y, Li Y, Eyo U, Chen T, Umpierre A, Zhu J, et al. Neuronal network activity controls microglial process surveillance in awake mice via norepinephrine signaling. Nat Neurosci. (2019) 22:1771–81. doi: 10.1038/s41593-019-0511-3
119. Shinozaki Y, Shibata K, Yoshida K, Shigetomi E, Gachet C, Ikenaka K, et al. Transformation of astrocytes to a neuroprotective phenotype by microglia via P2Y1 receptor downregulation. Cell Rep. (2017) 19:1151–64. doi: 10.1016/j.celrep.2017.04.047
120. Zhou X, Franklin RA, Adler M, Jacox JB, Bailis W, Shyer JA, et al. Circuit design features of a stable two-cell system. Cell. (2018) 172:744–57.e717. doi: 10.1016/j.cell.2018.01.015
121. Puñal VM, Paisley CE, Brecha FS, Lee M, Perelli R, O'Koren E, et al. Large-scale death of retinal astrocytes during normal development mediated by microglia. PLoS Biol. (2019) 17:e3000492. doi: 10.1371/journal.pbio.3000492
122. Zhou Y, Pan Y, Shao A. Letter by zhou et al. regarding article, “brain cleanup as a potential target for poststroke recovery: the role of RXR (Retinoic X Receptor) in phagocytes”. Stroke. (2020) 51:e89. doi: 10.1161/STROKEAHA.120.029270
123. Innes S, Pariante CM, Borsini A. Microglial-driven changes in synaptic plasticity: a possible role in major depressive disorder. Psychoneuroendocrinology. (2019) 102:236–47. doi: 10.1016/j.psyneuen.2018.12.233
124. Zhou Y, Shao A, Yao Y, Tu S, Deng Y, Zhang J. Dual roles of astrocytes in plasticity and reconstruction after traumatic brain injury. Cell Commun Signal. (2020) 18:62. doi: 10.1186/s12964-020-00549-2
125. Jha MK, Kim JH, Song GJ, Lee WH, Lee IK, Lee HW, et al. Functional dissection of astrocyte-secreted proteins: implications in brain health and diseases. Prog Neurobiol. (2018) 162:37–69. doi: 10.1016/j.pneurobio.2017.12.003
126. Lan X, Han X, Li Q, Yang QW, Wang J. Modulators of microglial activation and polarization after intracerebral haemorrhage. Nat Rev Neurol. (2017) 13:420–33. doi: 10.1038/nrneurol.2017.69
127. Kim JH, Ko PW, Lee HW, Jeong JY, Lee MG, Kim JH, et al. Astrocyte-derived lipocalin-2 mediates hippocampal damage and cognitive deficits in experimental models of vascular dementia. Glia. (2017) 65:1471–90. doi: 10.1002/glia.23174
128. Tanuma N, Sakuma H, Sasaki A, Matsumoto Y. Chemokine expression by astrocytes plays a role in microglia/macrophage activation and subsequent neurodegeneration in secondary progressive multiple sclerosis. Acta Neuropathol. (2006) 112:195–204. doi: 10.1007/s00401-006-0083-7
129. Lian H, Litvinchuk A, Chiang AC, Aithmitti N, Jankowsky JL, Zheng H. Astrocyte-microglia cross talk through complement activation modulates amyloid pathology in mouse models of Alzheimer's disease. J Neurosci. (2016) 36:577–89. doi: 10.1523/JNEUROSCI.2117-15.2016
130. Jeon H, Kim JH, Kim JH, Lee WH, Lee MS, Suk K. Plasminogen activator inhibitor type 1 regulates microglial motility and phagocytic activity. J Neuroinflammation. (2012) 9:149. doi: 10.1186/1742-2094-9-149
131. Jo M, Kim J-H, Song GJ, Seo M, Hwang EM, Suk K. Astrocytic orosomucoid-2 modulates microglial activation and neuroinflammation. J Neurosci. (2017) 37:2878–94. doi: 10.1523/JNEUROSCI.2534-16.2017
132. Norden DM, Fenn AM, Dugan A, Godbout JP. TGFβ produced by IL-10 redirected astrocytes attenuates microglial activation. Glia. (2014) 62:881–95. doi: 10.1002/glia.22647
133. Rocha SM, Cristovão AC, Campos FL, Fonseca CP, Baltazar G. Astrocyte-derived GDNF is a potent inhibitor of microglial activation. Neurobiol Dis. (2012) 47:407–15. doi: 10.1016/j.nbd.2012.04.014
134. Jeon H, Lee S, Lee WH, Suk K. Analysis of glial secretome: the long pentraxin PTX3 modulates phagocytic activity of microglia. J Neuroimmunol. (2010) 229:63–72. doi: 10.1016/j.jneuroim.2010.07.001
135. Dohgu S, Yamauchi A, Takata F, Naito M, Tsuruo T, Higuchi S, et al. Transforming growth factor-beta1 upregulates the tight junction and P-glycoprotein of brain microvascular endothelial cells. Cell Mol Neurobiol. (2004) 24:491–7. doi: 10.1023/B:CEMN.0000022776.47302.ce
136. Wosik K, Cayrol R, Dodelet-Devillers A, Berthelet F, Bernard M, Moumdjian R, et al. Angiotensin II controls occludin function and is required for blood brain barrier maintenance: relevance to multiple sclerosis. J Neurosci. (2007) 27:9032–42. doi: 10.1523/JNEUROSCI.2088-07.2007
137. Argaw AT, Asp L, Zhang J, Navrazhina K, Pham T, Mariani JN, et al. Astrocyte-derived VEGF-A drives blood-brain barrier disruption in CNS inflammatory disease. J Clin Invest. (2012) 122:2454–68. doi: 10.1172/JCI60842
138. Lou N, Takano T, Pei Y, Xavier AL, Goldman SA, Nedergaard M. Purinergic receptor P2RY12-dependent microglial closure of the injured blood-brain barrier. Proc Natl Acad Sci USA. (2016) 113:1074–9. doi: 10.1073/pnas.1520398113
139. Braniste V, Al-Asmakh M, Kowal C, Anuar F, Abbaspour A, Tóth M, et al. The gut microbiota influences blood-brain barrier permeability in mice. Sci Transl Med. (2014) 6:263ra158. doi: 10.1126/scitranslmed.3009759
140. Fung TC, Olson CA, Hsiao EY. Interactions between the microbiota, immune and nervous systems in health and disease. Nat Neurosci. (2017) 20:145–55. doi: 10.1038/nn.4476
141. Thion MS, Low D, Silvin A, Chen J, Grisel P, Schulte-Schrepping J, et al. Microbiome influences prenatal and adult microglia in a sex-specific manner. Cell. (2018) 172:500–16.e516. doi: 10.1016/j.cell.2017.11.042
142. Sharma RK, Yang T, Oliveira AC, Lobaton GO, Aquino V, Kim S, et al. Microglial cells impact gut microbiota and gut pathology in angiotensin II-induced hypertension. Circ Res. (2019) 124:727–36. doi: 10.1161/CIRCRESAHA.118.313882
143. Norden DM, Trojanowski PJ, Villanueva E, Navarro E, Godbout JP. Sequential activation of microglia and astrocyte cytokine expression precedes increased Iba-1 or GFAP immunoreactivity following systemic immune challenge. Glia. (2016) 64:300–16. doi: 10.1002/glia.22930
144. Verkhratsky A, Nedergaard M. Physiology of Astroglia. Physiol Rev. (2018) 98:239–389. doi: 10.1152/physrev.00042.2016
145. Haroon E, Miller AH, Sanacora G. Inflammation, glutamate, and glia: a trio of trouble in mood disorders. Neuropsychopharmacology. (2017) 42:193–215. doi: 10.1038/npp.2016.199
146. Li N, Lee B, Liu R-J, Banasr M, Dwyer JM, Iwata M, et al. mTOR-dependent synapse formation underlies the rapid antidepressant effects of NMDA antagonists. Science (New York, NY). (2010) 329:959–64. doi: 10.1126/science.1190287
147. Bialas AR, Stevens B. TGF-β signaling regulates neuronal C1q expression and developmental synaptic refinement. Nat Neurosci. (2013) 16:1773–82. doi: 10.1038/nn.3560
Keywords: astrocyte-microglia crosstalk, neuroinflammation, mood disorders, depression, bipolar disorder
Citation: Yang L, Zhou Y, Jia H, Qi Y, Tu S and Shao A (2020) Affective Immunology: The Crosstalk Between Microglia and Astrocytes Plays Key Role? Front. Immunol. 11:1818. doi: 10.3389/fimmu.2020.01818
Received: 21 March 2020; Accepted: 07 July 2020;
Published: 20 August 2020.
Edited by:
Reinhild Klein, University of Tübingen, GermanyReviewed by:
Esther Melamed, University of Texas at Austin, United StatesClara Ballerini, University of Florence, Italy
Copyright © 2020 Yang, Zhou, Jia, Qi, Tu and Shao. This is an open-access article distributed under the terms of the Creative Commons Attribution License (CC BY). The use, distribution or reproduction in other forums is permitted, provided the original author(s) and the copyright owner(s) are credited and that the original publication in this journal is cited, in accordance with accepted academic practice. No use, distribution or reproduction is permitted which does not comply with these terms.
*Correspondence: Anwen Shao, MjExMTgxMTZAemp1LmVkdS5jbg==; YW53ZW5zaGFvQHNpbmEuY29t
†These authors have contributed equally to this work