- Department of Pathology, Case Western Reserve University School of Medicine, Cleveland, OH, United States
Mucosal barriers are active sites that encounter a bombardment of antigenic stimuli derived from both the commensal flora and a variety of pathogens, as well as from environmental insults. As such, the ability to mount appropriate innate immune responses is an important first line of defense that confers protection to the host. Central to innate immunity are innate lymphoid cells (ILCs), which were first described a decade ago, and represent a family of heterogeneous cells driven by specific transcription factors and exhibit distinct cytokine profiles that are shared with their CD4+ T-helper cell counterparts. ILCs are particularly enriched at mucosal surfaces, and the tissue microenvironment and cytokine milieu in which ILCs reside are critical factors that drive the behavior and overall function of these cells. In fact, ILCs situated at mucosal barriers must be able to temper their response to a constant exposure of environmental antigens, but also promptly react to pathogens or signals that are potentially harmful to the host. In this context, the ability of ILCs to readily transdifferentiate in response to their dynamic surroundings has become a vigorous area of research, and defining specific mechanism(s) of ILC plasticity is at the advent of discovery. This review will summarize what is currently known regarding the network of cytokines and regulatory elements that enable ILCs to readily transform, based on the range of diverse signals and signal gradients they encounter that lead to either protective or pathogenic function(s), with focus on the gut mucosal immune system.
Introduction
Innate lymphoid cells (ILCs) are a diverse family of developmentally-related immune cells that are heterogeneous in their tissue location, cytokine secretion, and effector functions. The term “ILC” has been widely used since 2010, with distinct subsets formally proposed in 2013, based on the transcription factors and specific cytokines regulating their development and function (1). Initially, three groups of ILCs were described, representing innate counterparts that functionally mirror CD4+ T-helper cell subsets. More recently, NK and lymphoid tissue inducer (LTi) cells have expanded this family, thereby representing five different ILC groups (2). Group 1 ILCs (ILC1s) express IFNγ and rely on the transcription factor, T-bet, but unlike closely-related NK cells, they do not depend on Eomesodermin (Eomes) and are, in general, non-cytotoxic; however, a phenotypic hybrid of ILC1s and NK cells also exists (3). ILC2s produce the Th2 cytokines, IL-4, IL-5, and IL-13, as well as IL-9 and amphiregulin, and are functionally influenced by the transcription factors, GATA3 and RORα. ILC3s utilize RORγt to drive production of IL-22, but also IL-17, and are distinguished into further subsets based on expression of the natural cytotoxicity receptors (NCRs), NKp46 and NKp44. LTi cells are also dependent on RORγt, but produce lymphotoxin and are critical for secondary lymphoid organ formation, including Peyer’s patches. Although organizing ILCs into these five subgroups provides a basic infrastructure to understand the ILC family in regards to development and function, it is also important to consider whether this diversity is discrete, or reflects a given subset’s adaptability to the changing tissue microenvironment, such as what occurs during disease pathogenesis, and for these cells to undergo transformation.
In this context, several significant questions remain unanswered regarding the mechanisms underlying ILC development, divergence, and differentiation. What kind of environmental cues and other regulatory factors are necessary to determine cell fate? How do these processes relate to that of the CD4+ T cell helper population? Which ILC subgroups are terminally-differentiated, or like their corresponding CD4+ T helper cells, can convert from one ILC type to another? Although investigation of ILC plasticity is still in its infancy, these questions are now being answered, particularly with the emergence of more advanced techniques, such as single-cell transcriptomics, which facilitates a better understanding of the complexity and heterogeneity of ILCs (4–7).
In general, ILC2s and ILC3s have the ability to transdifferentiate into ILC1s, which is a reversible process, and highly dependent on the cytokine milieu and tissue microenvironment in which these cells reside. Transformation of ILC2s to ILC3s has also been reported, while plasticity between “natural” and “inflammatory” ILC2s, as well as of NK cells to ILC1s, may represent transient stages or terminally-differentiated events during disease pathogenesis, respectively. The following sections will summarize current findings regarding ILC plasticity and what signals (e.g., cytokines) control these processes, with particular focus on the gut mucosal immune system. We will also discuss the more recently coined regulatory ILC (ILCreg) subset and its contribution to mucosal immunity.
Cytokines that Regulate Plasticity of LTi and ILC3s
Plasticity within ILC subgroups was first observed in LTi cells, in which a gradient of RORγt expression is stabilized by IL-7 and the gut microbiome, while IL-12 and IL-15 accelerate its loss. Specifically, RORγt+ LTi cells produce IL-22 and are functionally protective, whereas RORγt− LTi cells secrete IFNγ and can induce colitis (8, 9). Similarly, ILC3s that are NKp46-CCR6-/low are able to differentiate into NKp46+ ILC3s, depending on upregulation of the prototypic ILC1 transcription factor, T-bet, which stimulates IFNγ and IL-22 production that are important for protection against Salmonella infection (10). In addition, Notch signaling mediated by T-bet guides the development of NKp46+ ILC3s (11). Interestingly, this upregulation of T-bet in a subpopulation of ILC3s is concurrent with loss of RORγt expression (8, 12).
How these “ex-ILC3s” are generated is of great interest and the focus of recent investigation. Although different mechanisms have been implicated, the tissue-specific microenvironment appears to be essential to skew ILC3 identity, as the frequency of these subsets differs depending on their residence. Most abundant within the intestines are ILC3s, wherein CCR6+ ILC3s and/or LTi-like cells are most prominently found in cryptopatches, whereas NKp46+/-CCR6-/low ILC3s are located within the lamina propria. Interestingly, Pearson et al., recently demonstrated that ILC3s can mobilize from intestinal cryptopatches to the lamina propria, and this migration is dependent on GM-CSF (13). Another mechanism for ILC3 switching was revealed through the use of RORγt-GFP reporter mice (14), which have been instrumental for lineage-tracing experiments (15); these mice retain GFP expression in their RORγt+ cells, even after loss of RORγt expression. Studies show that within the ILC1 subset, some cells have the ability to produce IFNγ, a typical ILC1 cytokine, and still have traceable GFP expression, suggesting that they were likely once RORγt+ ILC3s (i.e., ex-ILC3s) (8–10, 16–18). Although ILC3s rely on IL-7 signaling for proper development and maintenance, ex-ILC3s downregulate CD127 and c-Kit, and are more responsive to IL-15; in contrast, all other ILC3 subsets are not entirely dependent on IL-15 signaling (18, 19). Furthermore, upon IL-23 stimulation, STAT4/T-bet-dependent regulation of NCR+ ILC3s can promote IFNγ production and plasticity towards type 1 fate (20).
More recently, an intermediate population has been identified in human tonsils and small intestines, with characteristics of both ILC3s and intraepithelial CD103+ ILC1s (21). This population expresses CD103, CCR6, and CD300LF to different degrees, with scRNA-seq analysis providing evidence that ILC3s are able to convert in vivo into CD103+ ILC1s. Furthermore, Aiolos (encoded by IKZF3), a member of the Ikaros family of transcription factors, and expressed mainly by ILC1s and NK cells, is critical for skewing ILC3s into CD103- (22) and intraepithelial CD103+ ILC1s (21). The transcription factor, c-Maf, is also implicated in controlling the homeostatic balance of ILC3s by directly inhibiting T-bet, and therefore transdifferentiation to ILC1s (23, 24); in the absence of c-Maf, CD196- ILC3s transdifferentiate to an ILC1 phenotype (23). Another factor, BCL6, regulates ILC3-to-ILC1 plasticity by repressing ILC3-promoting pathways, such as IL-23-induced signaling (24), resulting in reduced ILC1 frequency.
In humans, the transition from ILC3s to ILC1s is contingent on downstream signals directed by IL-12 that induce the expression of T-bet (12). This is the case in inflamed intestinal tissues from Crohn’s disease patients, wherein increased ILC1s, at the expense of ILC3s, is observed, highlighting the prevalence of ILC3s during homeostasis, possibly by supporting T regulatory cell (Treg) activity via IL-2 (25), and ILC1s during (pathogenic) inflammatory events (26). Interestingly, differentiation from NKp46- to NKp46+ ILC3s, and then to NKp46+ ex-ILC3s (i.e., “ILC1s”), modulated by low-to-high T-bet expression, is reversible both in vivo and in vitro, and is dependent on IL-23, IL-2, and IL-1β, and is further enhanced by retinoic acid (12). This ILC3-to-ILC1 polarization also depends on the presence of CD14+ and CD14- dendritic cells, wherein an increase in the former promotes ILC1 differentiation, and an increase in the latter, induces ILC3 skewing. These findings indicate that, as much as the cytokine milieu and activation of transcription factors can affect ILC subset composition, so can environmental signals from local immune cells. In other studies, fate-mapping experiments have established that NKp46+RORγt+ ILC3s can downregulate in vivo expression of NKp46, generating NKp46-RORγt+ ILC3s (11, 27).
As mentioned earlier, T-bet itself can direct the development of NKp46+ ILC3s, which is mediated through Notch signaling (10). Confounding this finding, however, Notch is also reported to regulate plasticity within ILC3 subsets by controlling the fate of NKp46+ ILC3s (28). Furthermore, interconversion within the ILC3 subset occurs in response to TGFβ (29). Transcripts for the two subunits of the TGFβ receptor, TGFβ receptor I and II, can be detected in ILCs (30). Deletion of TGFβ receptor II leads to expansion of NKp46+ ILC3s, indicating that TGFβ impairs the development of NKp46+ ILC3s. Additionally, TGFβ antagonizes Notch signaling, implying that the ILC3 phenotype depends on fine-tuning the divergent effects of both TGFβ and Notch signaling that may be required to preserve homeostasis, in vivo (29).
Taken together, these studies indicate that ILC3s exhibit bi-directional differentiation that can be modulated by T-bet and RORγt gradients within the ILC3 lineage (Figure 1A). Are ex-ILC3s generated from NCR+ ILC3s, and are they all dependent on Notch and TGF-β signaling? How does T-bet and RORγt detect the extent of inflammation and deliver equivalent immune responses? To determine how these transcription factors readily impose various ILC3 effector programs, it is imperative to recognize the underlying molecular mechanisms for ILC3 plasticity. How this balance is maintained could reflect blunted inflammatory responses (i.e., in presence of commensal flora during homeostasis), while being poised to mount a vigorous immune reaction when challenge or insult occurs, particularly at mucosal barriers. Various ILC3 subsets may be actively modulated by the temporal degree of inflammation that then directs T-bet and RORγt expression.
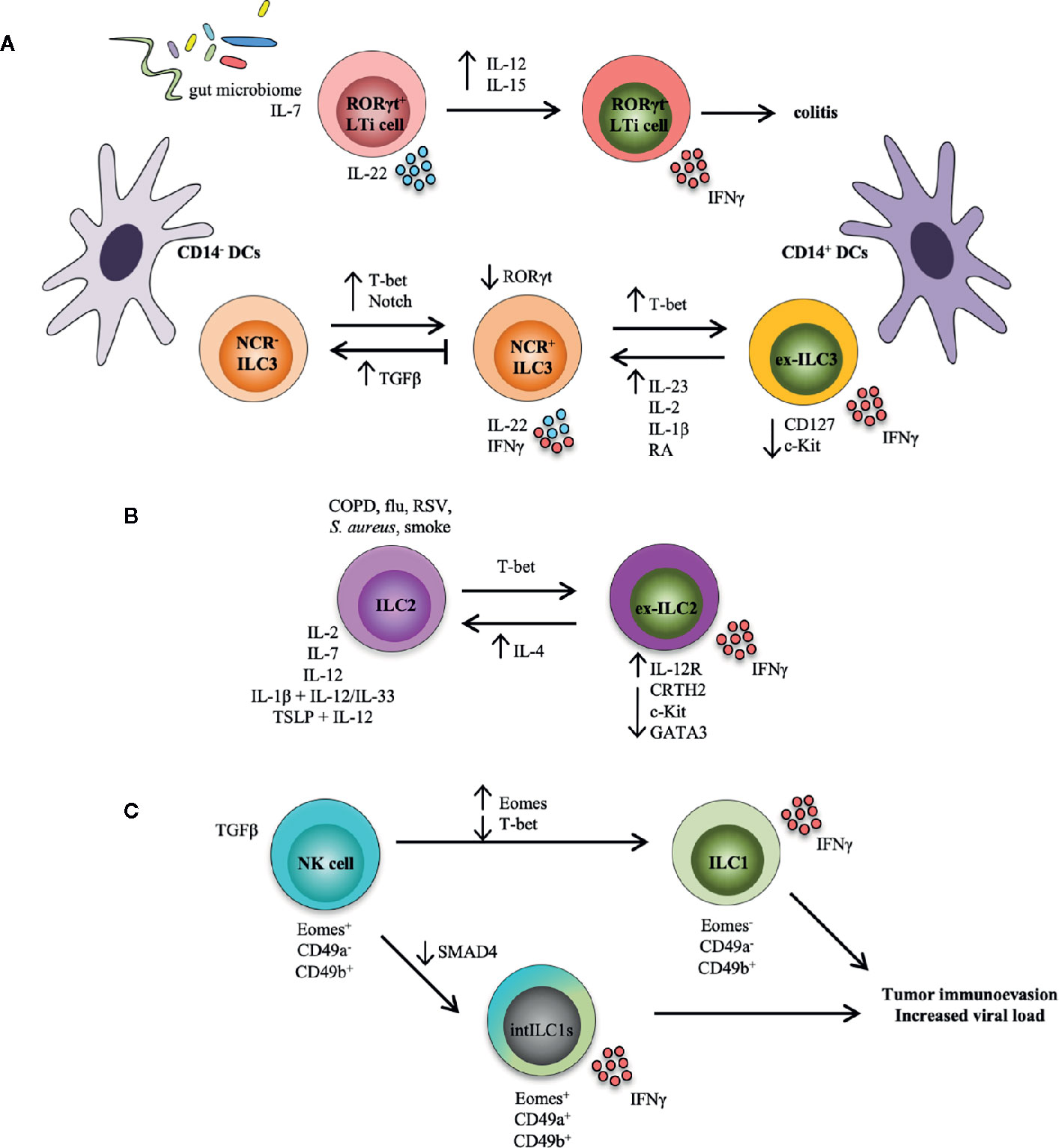
Figure 1 ILC transdifferentiation occurs in response to cytokine and/or pathogen stimulation. Schematic representation summarizing plasticity among ILC populations reported both in mouse models and in human settings. (A) Plasticity within the LTi and ILC3 populations. RORγt in LTi cells is stabilized by IL-7 and the gut microbiome; these cells produce IL-22 and are functionally protective. Conversely, IL-12 and IL-15 accelerate loss of RORγt in LTi cells, which secrete IFNγ and induce colitis. NCR- ILC3s can convert into NCR+ ILC3s under the influence of T-bet and Notch. T-bet upregulation of NCR+ ILC3s can transform ILC3s into ILC1-like cells (i.e., ex-ILC3s) that downregulate expression of CD127 and c-Kit, and secrete IFNγ. This process is reversed by IL-23, IL-1β, IL-2, and retinoic acid (RA), while TGFβ inhibits NCR+ ILC3 development from NCR- ILC3s and possibly drives reversion of these cells. Increased presence of CD14+ dendritic cells (DCs) drives an ex-ILC3 phenotype, while CD14- DCs promote the generation of NCR- ILC3s. (B) Plasticity within the ILC2 population. In the presence of an inflammatory environment typical of COPD (chronic obstructive pulmonary disease), smoke, and bacterial or viral infection, or after stimulation with IL-2, IL-7, IL-12, IL-1β, and IL-12, as well as TSLP and IL-12, ILC2s can assume an ILC1-like phenotype (i.e., ex-ILC2) that upregulates expression of the IL-12 receptor, downregulates ILC2-associated proteins (CRTH2, c-Kit, GATA3), and produces IFNγ. GATA3, a critical transcription factor for maintaining ILC2 identity, is repressed by T-bet. This route can also be reversed by eosinophil-derived IL-4. (C) Plasticity within the NK and ILC1 populations. In the context of a tumor microenvironment, NK cells (Eomes+CD49a-CD49b+) can convert to intermediate ILC1s (Eomes+CD49a+CD49b+), or intILC1s, upon stimulation with TGFβ. Similarly, NK cells can also transform to real ILC1s (Eomes-CD49a-CD49b+). SMAD4 promotes TGFβ signaling, but the lack of SMAD4 in NK cells transforms them into intILC1s. These ex-NK cells are not functional however, and provide a mechanism for tumors to escape surveillance, and may allow for increased viral burden in infection.
Cytokines that Regulate Plasticity of ILC2s
ILC2s have also been reported to alter their functional and transcriptional programs. ILC2s are capable of converting to IFNγ-producing ILC1s in both mice and humans (31–33). Specifically, ILC2s derived from human blood proliferate in vitro in the presence of IL-2 and IL-7, upregulate T-bet, and secrete IFNγ via IL-12 (34). Other studies show that, in the presence of IL-1β, human ILC2s not only express T-bet, but also the IL-12 receptor subunits, IL-12RB1 and IL-12RB2, enabling ILC2s to respond to IL-12 (32). Interestingly, patients with defects in IL-12Rβ1 suffer from a syndrome referred to as Mendelian susceptibility to mycobacterial disease; these individuals are not capable of generating ILC2s that potentially can convert to ILC1s (34). IL-12 is also important in inducing genomic modifications in the IFNγ locus, allowing for IFNγ secretion; these IFNγ-expressing “ex-ILC2s” are also able to secrete IL-13 (32). Similarly, Bal et al. showed in an inflammatory environment (i.e., lung tissues of patients with chronic obstructive pulmonary disease) that ILC2s can convert into IFNγ-producing ILC1s by exposure to either combination IL-1β and IL-12, IL-33, or TSLP (thymic stromal lymphopoietin) and IL-12 (31). After adoptive transfer into humanized mice, these ILC2s downregulate chemoattractant receptor-homologous molecule expressed on T-helper type 2 cells (CRTH2) and c-Kit expression, which are typical markers for ILC2s (31). These CRTH2-Kit- ex-ILC2s also express increased Tbx21 (T-bet) compared to CRTH2+Kit+ ILC2s. In humans, two subsets of CRTH2+ ILC2s were identified in peripheral blood, based on CD117 expression: CD117- ILC2s, indicating mature ILC2s, and CD117+ ILC2s, showing some features of ILC3s, such as RORγt expression (35, 36). Upon IL-33 stimulation, CD117+ ILC2s produce Th2 cytokines, whereas IL1β and IL-23 induce these cells to produce IL-17 (35, 36) and CCR6, which is also expressed by IL-17-producing ILC3s (37). ILC2s have also been reported to display phenotypic plasticity in response to infectious agents, such as influenza virus, respiratory syncytial virus, Staphylococcus aureus, and interestingly, cigarette smoke (33). GATA3 expression is downregulated in ILC2s in response to exposure to these agents, with a subsequent increase in IL-12Rβ2, IL-18Rα, and T-bet. This effect was confirmed in vivo by adoptive transfer of ILC2s from ST2/IL-33R reporter mice into recipients lacking T cells and ILCs (Rag2-/-Il2rg-/- mice) that were then infected with influenza. Although donor ILC2s downregulate GATA3 and upregulate IL-18Rα and IL-12Rβ2, they do not express T-bet; however, upon stimulation with IL-12 and IL-18, a portion of these ex-ILC2s are capable of IFNγ secretion, suggesting skewing towards ILC1s (33).
Interestingly, similar to ILC3-to-ILC1 conversion, ILC2-to-ILC1 transdifferentiation is also reversible. Although the mechanism for this process is not entirely clear, eosinophil-derived IL-4 appears to prevent IL-12-mediated ILC2 differentiation to ILC1 in an inflammatory milieu, such as within nasal polyps of patients with chronic rhinosinusitis (31). Furthermore, expression of the receptors for IL-1β, IL-12, IL-18, and IL-33 influence ILC2 expansion and T-bet induction, facilitating ILC2 conversion towards an ILC1 phenotype (31–33).
TGFβ from pulmonary epithelial cells has been observed to promote allergic immune responses by expanding IL-13–secreting ILC2s (30). IL-33 induces proliferation of ILC2s and stimulates TGFβ secretion in lung airways to activate ILC2 function and migration (30). The possibility therefore exists that TGFβ, in line with other inflammatory mediators, acts as a modulator of ILC2 and ILC3 fate. In fact, it has been proposed that murine ILC2s can be categorized into two subsets: a transient “inflammatory” subpopulation whose fate and function is dependent on the transcription factor, BATF, and expresses more IL-25R (thereby responding preferentially to IL-25), and a tissue resident “natural” ST2+ ILC2 subpopulation induced by IL-33 (38, 39). Inflammatory (i)ILC2s are not responsive to IL-33, but can convert into natural (n)ILC2s, both in vivo and in vitro, and express low quantities of RORγt and upon stimulation, IL-17 and IL-13, indicating plasticity between iILC2s and ILC3-like cells (38).
Although this phenomenon has not been recapitulated in humans, human clones of ILC2s and ILC3s that secrete both IL-22 and IL-13 have been characterized (40, 41). Similarly, ILC2s can respond to inflammation in skin and lungs by transdifferentiating into IL-17-producing ILC3s; specifically, ILC2s co-cultured with dermal cells and the fungus, Candida albicans, produce IL-17 and acquire a phenotype similar to that of NKp44- ILC3s (35). Finally, patients with more severe non-allergic asthma, with blunted Th2 responses, display both IL-5- and IL-13-producing ILC2s, and IL-17-producing ILC3s and ILC2s (42).
Together, these studies provide support for the existence of ILC2 phenotypic flexibility (Figure 1B). Future studies will benefit from experiments that can trace genetic lineages to clarify issues related to ILC2 plasticity. Discriminating between in vivo expansion of already low numbers of ILC subsets vs. transdifferentiation is challenging. Identifying extracellular influences and why lineage flexibility is necessary, will facilitate knowledge as to how pathogenic responses can be controlled and skewed toward protective function(s), leading to potential therapeutic interventions (8, 43).
Cytokines That Regulate Plasticity of ILC1s and NK Cells
Previous sections of this review have highlighted interconversion of ILC3s and ILC2s into ILC1s, and vice-versa. This section will examine ILC plasticity of the closely-related subgroups, NK cells and ILC1s. Although both subsets possess identical cell surface markers (NKp46, NKG2D), parallel transcriptional programming, and similar cytokine profiles (IFNγ, TNF), they are now considered distinct populations. For instance, NK cells developmentally do not need GATA3 (44), but some ILC1s do (45–48). The proposed cellular basis of this divergence is due to the transcription factors, inhibitor of DNA binding protein-2 (ID2) and promyelocytic leukemia zinc finger protein (PLZF), which are expressed in ILC precursors, but not in NK cells (18, 49). Recent studies, however, using polychromic reporter mice, show that ILC precursors actually have considerable NK precursor activity, challenging the existing paradigm that considers ILC1 and NK cells as two different subsets (50, 51). Moreover, conversion of NK cells into ILC1s is observed in mice with non-alcoholic fatty liver disease, and likely mediated, in part, by TGFβ (52). NK cells also express Eomes that, together with T-bet, modulate production of lysis-associated granules containing perforin and granzyme (53). Therefore, conceptually, if ILCs are innate counterparts of CD4+ T helper cells, then NK cells represent the innate version of CD8+ T cells.
Current investigation, however, suggests that mature NK cells and ILC1s can also undergo plasticity between themselves. Forced Eomes expression in T-bet+ ILC1s is, in fact, sufficient for transformation to CD49b+ NK cells (54). Conversely, IL-12 can convert Eomes+ NK cells into Eomes- ILC1-like cells following infection with Toxoplasma gondii (55). Intriguingly, in humans, an Eomes+Tbetlo liver-resident NK cell subset has been identified (56, 57). More recently, two reports show the requirement for TGFβ in driving NK cell-to-ILC1 conversion in a tumor microenvironment (58, 59) and during virus infection (59). Gao et al., recently identified NK cells that convert into intermediate ILC1s (intILC1s), also via TGFβ (58). Surprisingly, even though it is well-established that SMAD4 promotes signaling by TGFβ family members, and TGFβ encourages skewing towards the ILC1 lineage, Cortez, et al. demonstrated that SMAD4 deficiency in ILC1s does not affect their differentiation, but instead converts SMAD4-deficient NK cells into ILC1-like cells (59). This suggests that SMAD4 acts as a negative regulator of TGFβ signaling in NK cells, and inhibits typical TGFβ imprinting that is characteristic of ILC1s. More importantly, these intILC1s possess gene expression profiles that are intermediate between ILC1s and NK cells, and are incapable of limiting tumor burden or viral load. Whether these ILC1-like cells can actually revert back to NK cells is uncertain, but these studies reveal a mechanism by which the ILC continuum is controlled by a rheostat that includes a cytokine milieu driven by the local tissue microenvironment (Figure 1C).
New ILC on the Block: ILCregs
Like Tregs, ILCs are reported to have a corresponding population, aptly-called ILCregs (60). Wang, et al. identified ILCregs in mouse and human intestines that are induced upon inflammatory stimuli, such as DSS, anti-CD40 antibody, as well as Salmonella typhimurium and Citrobacter rodentium infection (60). ILCregs purportedly originate from common helper ILC precursors (CHILP), and not ILC precursors, and express the transcription factors, ID3 and Sox4. Although ILCregs do not express CD4 or Foxp3, they possess a gene identity distinct from other ILC subsets and Tregs. Importantly, ILCregs produce IL-10 and TGFβ, and suppress activation of ILC1s and ILC3s, but not ILC2s, in an IL-10-dependent manner. TGFβ is necessary for maintenance of ILCregs and autocrine TGFβ is required for its expansion during intestinal inflammation. The role of ILCregs in colorectal cancer (CRC) show that these cells transdifferentiate from ILC3s during CRC progression via TGFβ, indicating potential pro-tumorigenic function during ILC3-to-ILCreg plasticity (61). Furthermore, retinoic acid is reported to induce transdifferentiation of ILC2s into IL-10-producing ILCregs during airway inflammation (62), while ILC2s provide a predominant and inducible source of IL-10 in the GI tract (63). The existence of this novel ILC subset is, however, controversial and the topic of current investigation that elicits several open-ended questions. How do the transcription factors, ID3 and Sox4, synergize to modulate ILCreg development? Since ILCregs constitutively express Tgfbr1, Tgfbr2, Il2rb, and Il2rg, can they be stimulated directly by IL-2 and TGFβ? Why are ILC2s unresponsive to the effects of IL-10-producing ILCregs? It is interesting to note that a subset of IL-10-secreting ILC2s, detected in murine lungs after IL-33 treatment or papain stimulation, associates with reduced lung eosinophilia (64). Comparable to ILCregs, these IL-10+ ILC2s also express the anti-inflammatory gene, Retnla, thereby suggesting anti-inflammatory properties, yet warrants further investigation in other pathogenic inflammatory states.
Conclusions and Future Directions
ILCs are particularly enriched at mucosal surfaces, and studies over the last decade highlight various functions that are important at the intestinal barrier (65), both in maintaining gut homeostasis, but also during chronic inflammation. ILC plasticity allows adaptability in response to changes in the local tissue microenvironment that are critical to appropriately respond to pathogenic challenge, without the need for de novo ILC generation and recruitment. Investigation into the precise mechanisms that control plasticity of specific ILC subsets, particularly at mucosal surfaces, is in its infancy and will aid in further understanding disease pathogenesis and designing targeted therapies in the future.
Author Contributions
CS and K-AB contributed equally to researching, writing of initial drafts, and assembling manuscript. TP conceptualized, edited, and assembled the final submitted manuscript. All authors contributed to the article and approved the submitted version.
Funding
This work was supported by grants from the National Institutes of Health: DK091222, DK042191 (TTP), and DK097948 Pilot and Feasibility Award (CDS); and the Crohn’s & Colitis Foundation: CDA-581292 (CDS) and RFA-410354 (KGB).
Conflict of Interest
The authors declare that the research was conducted in the absence of any commercial or financial relationships that could be construed as a potential conflict of interest.
Abbreviations
c-Maf, musculoaponeurotic fibrosarcoma oncogene homolog; Eomes, Eomesodermin; GATA3, GATA binding protein-3; GFP, green fluorescent protein; GM-CSF, granulocyte macrophage colony stimulating factor; IFNγ, interferon-γ; IL-, interleukin-; ILC, innate lymphoid cell; ILCreg, regulatory innate lymphoid cell; LTi, lymphoid tissue inducer; NCR, natural cytotoxicity receptor; NK, natural killer; TGFβ, transforming growth factor-beta; TSLP, thymic stromal lymphopoietin; Th, T-helper; Treg, T-regulatory cell.
References
1. Spits H, Artis D, Colonna M, Diefenbach A, Di Santo JP, Eberl G, et al. Innate lymphoid cells–a proposal for uniform nomenclature. Nat Rev Immunol (2013) 13(2):145–9. doi: 10.1038/nri3365
2. Vivier E, Artis D, Colonna M, Diefenbach A, Di Santo JP, Eberl G, et al. Innate Lymphoid Cells: 10 Years On. Cell (2018) 174(5):1054–66. doi: 10.1016/j.cell.2018.07.017
3. Spits H, Bernink JH, Lanier L. NK cells and type 1 innate lymphoid cells: partners in host defense. Nat Immunol (2016) 17(7):758–64. doi: 10.1038/ni.3482
4. Gury-BenAri M, Thaiss CA, Serafini N, Winter DR, Giladi A, Lara-Astiaso D, et al. The Spectrum and Regulatory Landscape of Intestinal Innate Lymphoid Cells Are Shaped by the Microbiome. Cell (2016) 166(5):1231–46.e13. doi: 10.1016/j.cell.2016.07.043
5. Yu Y, Tsang JC, Wang C, Clare S, Wang J, Chen X, et al. Single-cell RNA-seq identifies a PD-1hi ILC progenitor and defines its development pathway. Nature (2016) 539(7627):102–6. doi: 10.1038/nature20105
6. Ishizuka IE, Chea S, Gudjonson H, Constantinides MG, Dinner AR, Bendelac A, et al. Single-cell analysis defines the divergence between the innate lymphoid cell lineage and lymphoid tissue-inducer cell lineage. Nat Immunol (2016) 17(3):269–76. doi: 10.1038/ni.3344
7. Bjorklund AK, Forkel M, Picelli S, Konya V, Theorell J, Friberg D, et al. The heterogeneity of human CD127(+) innate lymphoid cells revealed by single-cell RNA sequencing. Nat Immunol (2016) 17(4):451–60. doi: 10.1038/ni.3368
8. Vonarbourg C, Mortha A, Bui VL, Hernandez PP, Kiss EA, Hoyler T, et al. Regulated expression of nuclear receptor RORgammat confers distinct functional fates to NK cell receptor-expressing RORgammat(+) innate lymphocytes. Immunity (2010) 33(5):736–51. doi: 10.1016/j.immuni.2010.10.017
9. Cella M, Otero K, Colonna M. Expansion of human NK-22 cells with IL-7, IL-2, and IL-1beta reveals intrinsic functional plasticity. Proc Natl Acad Sci U S A (2010) 107(24):10961–6. doi: 10.1073/pnas.1005641107
10. Klose CS, Kiss EA, Schwierzeck V, Ebert K, Hoyler T, d’Hargues Y, et al. A T-bet gradient controls the fate and function of CCR6-RORgammat+ innate lymphoid cells. Nature (2013) 494(7436):261–5. doi: 10.1038/nature11813
11. Rankin LC, Groom JR, Chopin M, Herold MJ, Walker JA, Mielke LA, et al. The transcription factor T-bet is essential for the development of NKp46+ innate lymphocytes via the Notch pathway. Nat Immunol (2013) 14(4):389–95. doi: 10.1038/ni.2545
12. Bernink JH, Krabbendam L, Germar K, de Jong E, Gronke K, Kofoed-Nielsen M, et al. Interleukin-12 and -23 Control Plasticity of CD127(+) Group 1 and Group 3 Innate Lymphoid Cells in the Intestinal Lamina Propria. Immunity (2015) 43(1):146–60. doi: 10.1016/j.immuni.2015.06.019
13. Pearson C, Thornton EE, McKenzie B, Schaupp AL, Huskens N, Griseri T, et al. ILC3 GM-CSF production and mobilisation orchestrate acute intestinal inflammation. Elife (2016) 5:e10066. doi: 10.7554/eLife.10066
14. Eberl G, Marmon S, Sunshine MJ, Rennert PD, Choi Y, Littman DR. An essential function for the nuclear receptor RORgamma(t) in the generation of fetal lymphoid tissue inducer cells. Nat Immunol (2004) 5(1):64–73. doi: 10.1038/ni1022
15. Sawa S, Cherrier M, Lochner M, Satoh-Takayama N, Fehling HJ, Langa F, et al. Lineage relationship analysis of RORgammat+ innate lymphoid cells. Science (2010) 330(6004):665–9. doi: 10.1126/science.1194597
16. Fuchs A, Vermi W, Lee JS, Lonardi S, Gilfillan S, Newberry RD, et al. Intraepithelial type 1 innate lymphoid cells are a unique subset of IL-12- and IL-15-responsive IFN-gamma-producing cells. Immunity (2013) 38(4):769–81. doi: 10.1016/j.immuni.2013.02.010
17. Bernink JH, Peters CP, Munneke M, te Velde AA, Meijer SL, Weijer K, et al. Human type 1 innate lymphoid cells accumulate in inflamed mucosal tissues. Nat Immunol (2013) 14(3):221–9. doi: 10.1038/ni.2534
18. Klose CS, Flach M, Mohle L, Rogell L, Hoyler T, Ebert K, et al. Differentiation of type 1 ILCs from a common progenitor to all helper-like innate lymphoid cell lineages. Cell (2014) 157(2):340–56. doi: 10.1016/j.cell.2014.03.030
19. Satoh-Takayama N, Lesjean-Pottier S, Vieira P, Sawa S, Eberl G, Vosshenrich CA, et al. IL-7 and IL-15 independently program the differentiation of intestinal CD3-NKp46+ cell subsets from Id2-dependent precursors. J Exp Med (2010) 207(2):273–80. doi: 10.1084/jem.20092029
20. Mikami Y, Scarno G, Zitti B, Shih HY, Kanno Y, Santoni A, et al. NCR(+) ILC3 maintain larger STAT4 reservoir via T-BET to regulate type 1 features upon IL-23 stimulation in mice. Eur J Immunol (2018) 48(7):1174–80. doi: 10.1002/eji.201847480
21. Cella M, Gamini R, Secca C, Collins PL, Zhao S, Peng V, et al. Subsets of ILC3-ILC1-like cells generate a diversity spectrum of innate lymphoid cells in human mucosal tissues. Nat Immunol (2019) 20(8):980–91. doi: 10.1038/s41590-019-0425-y
22. Mazzurana L, Forkel M, Rao A, Van Acker A, Kokkinou E, Ichiya T, et al. Suppression of Aiolos and Ikaros expression by lenalidomide reduces human ILC3-ILC1/NK cell transdifferentiation. Eur J Immunol (2019) 49(9):1344–55. doi: 10.1002/eji.201848075
23. Parker ME, Barrera A, Wheaton JD, Zuberbuehler MK, Allan DSJ, Carlyle JR, et al. c-Maf regulates the plasticity of group 3 innate lymphoid cells by restraining the type 1 program. J Exp Med (2020) 217(1). doi: 10.1084/jem.20191030
24. Pokrovskii M, Hall JA, Ochayon DE, Yi R, Chaimowitz NS, Seelamneni H, et al. Characterization of Transcriptional Regulatory Networks that Promote and Restrict Identities and Functions of Intestinal Innate Lymphoid Cells. Immunity (2019) 51(1):185–97.e6. doi: 10.1016/j.immuni.2019.06.001
25. Zhou L, Chu C, Teng F, Bessman NJ, Goc J, Santosa EK, et al. Innate lymphoid cells support regulatory T cells in the intestine through interleukin-2. Nature (2019) 568(7752):405–9. doi: 10.1038/s41586-019-1082-x
26. Bal SM, Golebski K, Spits H. Plasticity of innate lymphoid cell subsets. Nat Rev Immunol (2020). doi: 10.1038/s41577-020-0282-9
27. Rankin LC, Girard-Madoux MJ, Seillet C, Mielke LA, Kerdiles Y, Fenis A, et al. Complementarity and redundancy of IL-22-producing innate lymphoid cells. Nat Immunol (2016) 17(2):179–86. doi: 10.1038/ni.3332
28. Chea S, Perchet T, Petit M, Verrier T, Guy-Grand D, Banchi EG, et al. Notch signaling in group 3 innate lymphoid cells modulates their plasticity. Sci Signaling (2016) 9(426):ra45. doi: 10.1126/scisignal.aaf2223
29. Viant C, Rankin LC, Girard-Madoux MJH, Seillet C, Shi W, Smyth MJ, et al. Transforming growth factor-beta and Notch ligands act as opposing environmental cues in regulating the plasticity of type 3 innate lymphoid cells. Sci Signaling (2016) 9(426):ra46. doi: 10.1126/scisignal.aaf2176
30. Denney L, Byrne AJ, Shea TJ, Buckley JS, Pease JE, Herledan GM, et al. Pulmonary Epithelial Cell-Derived Cytokine TGF-beta1 Is a Critical Cofactor for Enhanced Innate Lymphoid Cell Function. Immunity (2015) 43(5):945–58. doi: 10.1016/j.immuni.2015.10.012
31. Bal SM, Bernink JH, Nagasawa M, Groot J, Shikhagaie MM, Golebski K, et al. IL-1beta, IL-4 and IL-12 control the fate of group 2 innate lymphoid cells in human airway inflammation in the lungs. Nat Immunol (2016) 17(6):636–45. doi: 10.1038/ni.3444
32. Ohne Y, Silver JS, Thompson-Snipes L, Collet MA, Blanck JP, Cantarel BL, et al. IL-1 is a critical regulator of group 2 innate lymphoid cell function and plasticity. Nat Immunol (2016) 17(6):646–55. doi: 10.1038/ni.3447
33. Silver JS, Kearley J, Copenhaver AM, Sanden C, Mori M, Yu L, et al. Inflammatory triggers associated with exacerbations of COPD orchestrate plasticity of group 2 innate lymphoid cells in the lungs. Nat Immunol (2016) 17(6):626–35. doi: 10.1038/ni.3443
34. Lim AI, Menegatti S, Bustamante J, Le Bourhis L, Allez M, Rogge L, et al. IL-12 drives functional plasticity of human group 2 innate lymphoid cells. J Exp Med (2016) 213(4):569–83. doi: 10.1084/jem.20151750
35. Bernink JH, Ohne Y, Teunissen MBM, Wang J, Wu J, Krabbendam L, et al. c-Kit-positive ILC2s exhibit an ILC3-like signature that may contribute to IL-17-mediated pathologies. Nat Immunol (2019) 20(8):992–1003. doi: 10.1038/s41590-019-0423-0
36. Hochdorfer T, Winkler C, Pardali K, Mjosberg J. Expression of c-Kit discriminates between two functionally distinct subsets of human type 2 innate lymphoid cells. Eur J Immunol (2019) 49(6):884–93. doi: 10.1002/eji.201848006
37. Golebski K, Ros XR, Nagasawa M, van Tol S, Heesters BA, Aglmous H, et al. IL-1beta, IL-23, and TGF-beta drive plasticity of human ILC2s towards IL-17-producing ILCs in nasal inflammation. Nat Commun (2019) 10(1):2162. doi: 10.1038/s41467-019-09883-7
38. Huang Y, Guo L, Qiu J, Chen X, Hu-Li J, Siebenlist U, et al. IL-25-responsive, lineage-negative KLRG1(hi) cells are multipotential ‘inflammatory’ type 2 innate lymphoid cells. Nat Immunol (2015) 16(2):161–9. doi: 10.1038/ni.3078
39. Miller MM, Patel PS, Bao K, Danhorn T, O’Connor BP, Reinhardt RL. BATF acts as an essential regulator of IL-25-responsive migratory ILC2 cell fate and function. Sci Immunol (2020) 5(43). doi: 10.1126/sciimmunol.aay3994
40. Mjosberg JM, Trifari S, Crellin NK, Peters CP, van Drunen CM, Piet B, et al. Human IL-25- and IL-33-responsive type 2 innate lymphoid cells are defined by expression of CRTH2 and CD161. Nat Immunol (2011) 12(11):1055–62. doi: 10.1038/ni.2104
41. Crellin NK, Trifari S, Kaplan CD, Satoh-Takayama N, Di Santo JP, Spits H. Regulation of cytokine secretion in human CD127(+) LTi-like innate lymphoid cells by Toll-like receptor 2. Immunity (2010) 33(5):752–64. doi: 10.1016/j.immuni.2010.10.012
42. Cai T, Qiu J, Ji Y, Li W, Ding Z, Suo C, et al. IL-17-producing ST2(+) group 2 innate lymphoid cells play a pathogenic role in lung inflammation. J Allergy Clin Immunol (2019) 143(1):229–44.e9. doi: 10.1016/j.jaci.2018.03.007
43. Buonocore S, Ahern PP, Uhlig HH, Ivanov II, Littman DR, Maloy KJ, et al. Innate lymphoid cells drive interleukin-23-dependent innate intestinal pathology. Nature (2010) 464(7293):1371–5. doi: 10.1038/nature08949
44. Samson SI, Richard O, Tavian M, Ranson T, Vosshenrich CA, Colucci F, et al. GATA-3 promotes maturation, IFN-gamma production, and liver-specific homing of NK cells. Immunity (2003) 19(5):701–11. doi: 10.1016/S1074-7613(03)00294-2
45. Hoyler T, Klose CS, Souabni A, Turqueti-Neves A, Pfeifer D, Rawlins EL, et al. The transcription factor GATA-3 controls cell fate and maintenance of type 2 innate lymphoid cells. Immunity (2012) 37(4):634–48. doi: 10.1016/j.immuni.2012.06.020
46. Yagi R, Zhong C, Northrup DL, Yu F, Bouladoux N, Spencer S, et al. The transcription factor GATA3 is critical for the development of all IL-7Ralpha-expressing innate lymphoid cells. Immunity (2014) 40(3):378–88. doi: 10.1016/j.immuni.2014.01.012
47. Klein Wolterink RG, Serafini N, van Nimwegen M, Vosshenrich CA, de Bruijn MJ, Fonseca Pereira D, et al. Essential, dose-dependent role for the transcription factor Gata3 in the development of IL-5+ and IL-13+ type 2 innate lymphoid cells. Proc Natl Acad Sci U S A (2013) 110(25):10240–5. doi: 10.1073/pnas.1217158110
48. Serafini N, Klein Wolterink RG, Satoh-Takayama N, Xu W, Vosshenrich CA, Hendriks RW, et al. Gata3 drives development of RORgammat+ group 3 innate lymphoid cells. J Exp Med (2014) 211(2):199–208. doi: 10.1084/jem.20131038
49. Constantinides MG, McDonald BD, Verhoef PA, Bendelac A. A committed precursor to innate lymphoid cells. Nature (2014) 508(7496):397–401. doi: 10.1038/nature13047
50. Xu W, Cherrier DE, Chea S, Vosshenrich C, Serafini N, Petit M, et al. An Id2(RFP)-Reporter Mouse Redefines Innate Lymphoid Cell Precursor Potentials. Immunity (2019) 50(4):1054–68.e3. doi: 10.1016/j.immuni.2019.02.022
51. Walker JA, Clark PA, Crisp A, Barlow JL, Szeto A, Ferreira ACF, et al. Polychromic Reporter Mice Reveal Unappreciated Innate Lymphoid Cell Progenitor Heterogeneity and Elusive ILC3 Progenitors in Bone Marrow. Immunity (2019) 51(1):104–18.e7. doi: 10.1016/j.immuni.2019.05.002
52. Cuff AO, Sillito F, Dertschnig S, Hall A, Luong TV, Chakraverty R, et al. The Obese Liver Environment Mediates Conversion of NK Cells to a Less Cytotoxic ILC1-Like Phenotype. Front Immunol (2019) 10:2180. doi: 10.3389/fimmu.2019.02180
53. Gordon SM, Chaix J, Rupp LJ, Wu J, Madera S, Sun JC, et al. The transcription factors T-bet and Eomes control key checkpoints of natural killer cell maturation. Immunity (2012) 36(1):55–67. doi: 10.1016/j.immuni.2011.11.016
54. Pikovskaya O, Chaix J, Rothman NJ, Collins A, Chen YH, Scipioni AM, et al. Cutting Edge: Eomesodermin Is Sufficient To Direct Type 1 Innate Lymphocyte Development into the Conventional NK Lineage. J Immunol (2016) 196(4):1449–54. doi: 10.4049/jimmunol.1502396
55. Park E, Patel S, Wang Q, Andhey P, Zaitsev K, Porter S, et al. Toxoplasma gondii infection drives conversion of NK cells into ILC1-like cells. Elife (2019) 8. doi: 10.7554/eLife.47605
56. Harmon C, Robinson MW, Fahey R, Whelan S, Houlihan DD, Geoghegan J, et al. Tissue-resident Eomes(hi) T-bet(lo) CD56(bright) NK cells with reduced proinflammatory potential are enriched in the adult human liver. Eur J Immunol (2016) 46(9):2111–20. doi: 10.1002/eji.201646559
57. Stegmann KA, Robertson F, Hansi N, Gill U, Pallant C, Christophides T, et al. CXCR6 marks a novel subset of T-bet(lo)Eomes(hi) natural killer cells residing in human liver. Sci Rep (2016) 6:26157. doi: 10.1038/srep26157
58. Gao Y, Souza-Fonseca-Guimaraes F, Bald T, Ng SS, Young A, Ngiow SF, et al. Tumor immunoevasion by the conversion of effector NK cells into type 1 innate lymphoid cells. Nat Immunol (2017) 18(9):1004–15. doi: 10.1038/ni.3800
59. Cortez VS, Ulland TK, Cervantes-Barragan L, Bando JK, Robinette ML, Wang Q, et al. SMAD4 impedes the conversion of NK cells into ILC1-like cells by curtailing non-canonical TGF-beta signaling. Nat Immunol (2017) 18(9):995–1003. doi: 10.1038/ni.3809
60. Wang S, Xia P, Chen Y, Qu Y, Xiong Z, Ye B, et al. Regulatory Innate Lymphoid Cells Control Innate Intestinal Inflammation. Cell (2017) 171(1):201–16.e18. doi: 10.1016/j.cell.2017.07.027
61. Wang S, Qu Y, Xia P, Chen Y, Zhu X, Zhang J, et al. Transdifferentiation of tumor infiltrating innate lymphoid cells during progression of colorectal cancer. Cell Res (2020). doi: 10.1038/s41422-020-0312-y
62. Morita H, Kubo T, Ruckert B, Ravindran A, Soyka MB, Rinaldi AO, et al. Induction of human regulatory innate lymphoid cells from group 2 innate lymphoid cells by retinoic acid. J Allergy Clin Immunol (2019) 143(6):2190–201.e9. doi: 10.1016/j.jaci.2018.12.1018
63. Bando JK, Gilfillan S, Di Luccia B, Fachi JL, Secca C, Cella M, et al. ILC2s are the predominant source of intestinal ILC-derived IL-10. J Exp Med (2020) 217(2). doi: 10.1084/jem.20191520
64. Seehus CR, Kadavallore A, Torre B, Yeckes AR, Wang Y, Tang J, et al. Alternative activation generates IL-10 producing type 2 innate lymphoid cells. Nat Commun (2017) 8(1):1900. doi: 10.1038/s41467-017-02023-z
Keywords: innate lymphoid cells (ILCs), cytokines, plasticity, gut mucosal immunity, innate immunity
Citation: De Salvo C, Buela K-A and Pizarro TT (2020) Cytokine-Mediated Regulation of Innate Lymphoid Cell Plasticity in Gut Mucosal Immunity. Front. Immunol. 11:585319. doi: 10.3389/fimmu.2020.585319
Received: 20 July 2020; Accepted: 03 November 2020;
Published: 04 December 2020.
Edited by:
Annalisa Del Prete, University of Brescia, ItalyReviewed by:
Cyril Seillet, Walter and Eliza Hall Institute of Medical Research, AustraliaGiuseppe Sciumè, Sapienza University of Rome, Italy
Copyright © 2020 De Salvo, Buela and Pizarro. This is an open-access article distributed under the terms of the Creative Commons Attribution License (CC BY). The use, distribution or reproduction in other forums is permitted, provided the original author(s) and the copyright owner(s) are credited and that the original publication in this journal is cited, in accordance with accepted academic practice. No use, distribution or reproduction is permitted which does not comply with these terms.
*Correspondence: Theresa T. Pizarro, theresa.pizarro@case.edu
†These authors have contributed equally to this work