- 1Infection and Innate Immunity Lab, Department of Biological Sciences, Institute for Biomedical Sciences, The George Washington University, Washington, DC, United States
- 2Department of Biochemistry and Molecular Pharmacology, New York University School of Medicine, New York, NY, United States
Background
Flaviviruses are enveloped single-stranded RNA viruses and major human pathogens. They are responsible for causing outbreaks and therefore they represent a serious health issue worldwide (1). Because of the clinical significance of flaviviruses and the severity of epidemics they cause globally, developing efficient vaccines and drugs is critical for the success of disease control measures. Importantly, many flaviviruses, including Dengue virus (DENV), Japanese Encephalitis virus (JEV), West Nile virus (WNV), Yellow Fever virus (YFV), and Zika virus (ZIKV), are vectored though arthropods (arboviruses), mainly mosquitoes and ticks (2). Although most previous efforts have primarily focused on advancing the design of therapeutic strategies for alleviating the disease symptoms caused by flaviviruses, it is equally significant to also be able to interpret the molecular nature of the interactions that take place between flaviviruses and the insect vector and determine whether these interactions affect pathophysiological processes during infection and transmission.
Interactions between mosquito vectors and flaviviruses have been studied in several occasions (3). For instance, ZIKV is mainly transmitted by Aedes aegypti mosquitoes and recent studies have begun to examine vector-virus relationships and transmission dynamics of this virus pathogen (4). Ae. aegypti mosquitoes infected with ZIKV activate the RNA interference (RNAi) mechanism by upregulating several virus-produced short interfering RNAs (siRNAs), piwi-interacting RNAs and microRNAs. Many of the latter are also regulated by DENV and WNVs, but not by the alphavirus Chikungunya, indicating conservation in the mosquito response to flavivirus infection (5). Flavivirus infection in mosquito vectors activates innate immune signaling, which promotes the induction of antiviral response through the production of effector molecules (6). Activation of JAK/STAT together with Toll signaling elevates the Ae. aegypti resistance to ZIKV infection, silencing the Toll pathway adaptor MyD88 increases DENV infection in the Ae. aegypti midgut, and DENV infection in this mosquito vector decreases the signaling activity of immune deficiency (Imd) pathway (7–9). Interestingly, the secreted protein Vago limits WNV replication in Culex mosquito cells through induction of JAK/STAT signaling (10). Studies in mosquitoes are expected to contribute toward developing efficient procedures for preventing the spread of flaviviruses.
Results from research with insect vectors further stress the need for insect immunologists studying antiviral immunity to draw more attention to the outcome of flavivirus infection in insects rather than focusing exclusively on the replication efficacy of the virus. Therefore, this opinion article aims at highlighting recent studies that have started to dissect the interaction between antiviral immune mechanisms and flavivirus tropism as well as host tissue homeostasis and pathophysiological defects in mosquitoes and the model insect Drosophila. Such information is critical because it has the potential to lead to the development of novel therapeutics that will be directed against the ability of flaviviruses to multiply in certain insect organs. This will be valuable knowledge for understanding and potentially predicting the severity and extent of flavivirus infection and the efficiency of transmission.
Flavivirus Interaction with Mosquito Immune and Metabolic Organs
The insect fat body is a diffused organ that functions similarly to the mammalian liver and is responsible for metabolism and storage of nutrients as well as the production and secretion of antimicrobial peptides and other immune factors (11). Flaviviruses present in the mosquito hemocoel (the insect body cavity) replicate in the abdominal and thoracic fat body before they disseminate to the salivary glands and other insect tissues (12). For instance, WNV replicates primarily in the fat body of Culex pipiens quinquefasciatus (13), but DENV replication has not been associated with this tissue in Aedes albopictus and Ae. aegypti mosquitoes (14, 15). However, more recent findings indicate that DENV replication in the fat body cells of A. albopictus alters the expression of Actin and alpha Tubulin (16), and downregulates the transcription of Toll pathway related genes in Ae. aegypti (8). In Ae. aegypti, YFV replicates in the fat body and other organs and feeding mosquitoes with a DENV-infected blood meal leads to activation of autophagy in the this tissue and the midgut and increases the expression of genes related to apoptosis such as the effector caspase Casps7 (17–19). Notably, activation of JAK/STAT signaling specifically in the fat body of Ae. aegypti restrains DENV efficiently but fails to restrict ZIKV infection, and priming of these mosquitoes with inactive DENV induces the activation of Notch signaling upon infection with active DENV and reduces viral propagation in the midgut and carcass (20, 21). Flaviviruses transmitted by mosquitoes are taken up through a blood meal and migrate to the mosquito gut before they move to the salivary glands in order to be passed on to another vertebrate host and maintain their lifecycle (22). Main barriers to systemic infection include the midgut infection barrier, midgut escape barrier, salivary gland infection barrier, and salivary gland escape barrier (23). In the gut, flavivirus infection triggers the induction of antiviral pathways through interaction with receptors in the midgut epithelial cells (24). Interfering with the expression of certain RNAi signaling components in Ae. aegypti adult mosquitoes reduces DENV titers in the midgut following oral infection (25). Although Toll signaling plays a crucial role in the anti-DENV response in the midgut of Ae. aegypti (26, 27), the involvement of this pathway in the mosquito immune response against WNV and YFV is not fully determined yet (28). Also, Imd signaling activity participates in the induction of anti-DENV immune functions in the mosquito gut, because inhibition of this pathway leads to higher DENV load in Ae. aegypti midgut (29). Similar role has further been demonstrated for two DENV restriction factors, which are regulated by the JAK/STAT pathway and their expression lowers viral titers in the Ae. aegypti midgut (30). Apoptosis events have been found to occur in the midgut of C. pipiens and Ae. aegypti refractory strains during infection with WNV or DENV, respectively, indicating a potential role in restricting virus propagation by these mosquito vectors (31, 32). Strikingly, the presence of gut microbiota in Ae. aegypti can have a direct or indirect influence on DENV infection and spread either by enhancing the mosquito innate immune response or suppressing virus replication through the secretion of unknown molecules [(24, 33) and references therein]. DENV infection in Ae. aegypti can be affected by the midgut-inhabiting bacterium Serratia odorifera or the fungus Talaromyces, which both increase mosquito sensitivity to this virus. This is achieved through the production of a bacterial polypeptide that interacts with the virus or the modification of trypsin enzyme activity by the fungus. Both microbially mediated effects lead to considerable changes in mosquito physiology (34, 35). Interestingly, variation in Wolbachia-mediated DENV blocking in Ae. aegypti has been previously attributed to the production of nitric oxide or other free radicals (36). Also, serum ion can be utilized by the Ae. aegypti iron metabolism pathway to strengthen reactive oxygen species activity in the gut epithelium in order to oppose DENV infection (37). In addition, expression of the redox-sensing gene nuclear factor erythroid-derived factor 2 (Nrf2) limits ZIKV infection by maintaining midgut homeostasis through modulation of reactive oxygen species in the midgut as well as microbiota growth and stem cell proliferation (38).
The association between flavivirus infection and lipid droplet regulation in mammalian cells has been previously reported (39–41). For example, infection of BHK-21, HepG2, and C6/36 cells by DENV increases markedly the number of lipid droplets per cell. This interaction possibly occurs between lipid droplets and various conserved residues in the core protein of the virus and probably indicates a link between viral replication and modulation of lipid metabolism (42). Also, during DENV infection of the hepatocyte derived cellular carcinoma cell line Huh7, HMG-CoA reductase activity increases, leading to higher cholesterol levels in the endoplasmic reticulum necessary for virus replication complex formation (43). In a similar fashion, infection of the Ae. aegypti cell line Aag2 with DENV increases lipid droplet accumulation (44). This phenotype in the DENV infected cells is associated with substantial upregulation of transcript levels of genes encoding factors related to lipid droplet biogenesis and lipid storage. These effects are connected with changes in immune signaling regulation, given that ectopic activation of Toll or Imd pathways further result in higher numbers of lipid droplets in the midgut.
Lessons From the Drosophila-Flavivirus Model
Due to ZIKV outbreaks in several countries over the past five years, recent research has used the Drosophila model for leveraging the powerful genetic and genomic tools in the fly in order to understand flavivirus pathogenesis (Figure 1). ZIKV possesses a positive-sense single-stranded RNA genome encoding three structural (capsid, pre-membrane, envelope) and seven non-structural proteins (NS1, NS2A, NS2B, NS3, NS4A, NS4B, and NS5) (45). Expression of the ZIKV non-structural protein NS4A in the brain of Drosophila larvae induces apoptosis and leads to microcephaly, while expression of human Ankyrin Repeat And LEM Domain Containing 2 (ANKLE2) gene, which is involved in brain development and has been previously implicated in hereditary microcephaly, in flies overexpressing ZIKV NS4A abolishes these defects. These results provide proof that ZIKV NS4A interacts physically with the ANKLE2 protein and causes microcephaly in an ANKLE2-dependent manner (46) (Figure 1A). More recently, these findings were extended by showing that mutations in ANKLE2 gene perturbs an asymmetric cell division pathway in Drosophila neuroblasts and causes neurological disease and microcephaly, whereas overexpression of ZIKV NS4A in neuroblasts produces a similar phenotype observed in Ankle2 mutants (47).
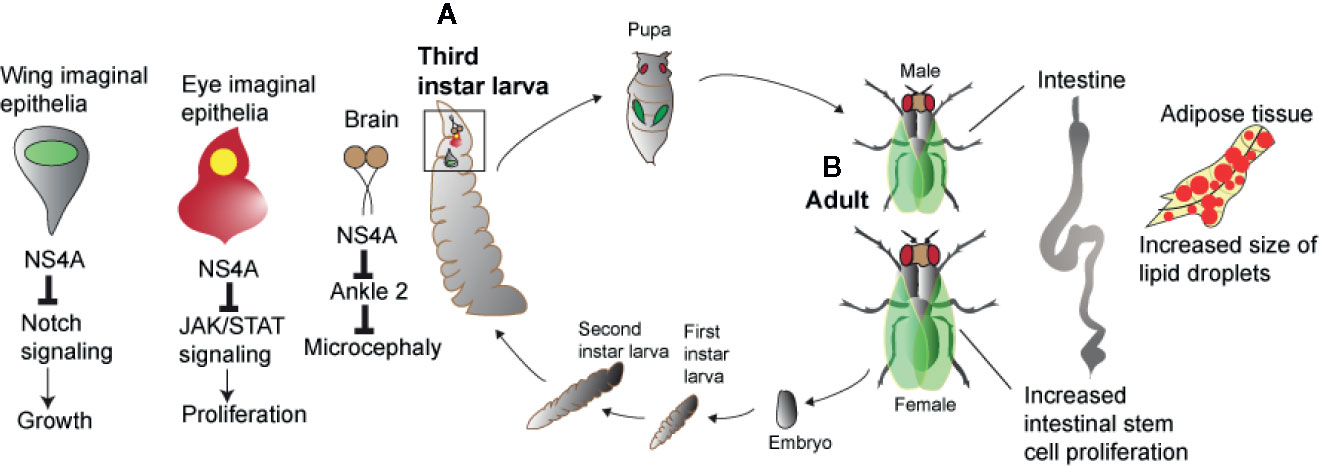
Figure 1 Zika virus and host pathologies in Drosophila. Zika virus results in perturbed homeostasis of different organs in Drosophila larva and adult. (A) Overexpression of Zika virus NS4A results in microcephaly in brain and restricted growth of the wing and eye imaginal epithelia. In the wing and eye imaginal epithelia, Zika virus NS4A interacts with Notch and JAK/STAT signaling pathways while in case of the brain, Zika virus NS4A targets ANKLE2, a highly conserved mitotic regulator. (B) Infection of Zika virus in Drosophila adult flies results in perturbed intestinal and adipose tissue homeostasis marked by increased intestinal stem cell proliferation and increased size of the lipid droplets.
Also, it has been shown that ZIKV infection induces antiviral autophagy in the brain of adult Drosophila and this process depends on the activity of the Imd transcription factor Relish (48). The fly ortholog of the mammalian polyubiquitin-binding scaffold protein p62, the autophagy cargo receptor Ref(2)P, is also directed against ZIKV in the brain and protection against this pathogen is not dependent on RNAi signaling activity (48). Interestingly, ZIKV also replicates in the fat body, crop and gut of the adult fly and this tissue tropism disrupts gut and fat body lipid droplet homeostasis (49) (Figure 1B). This tissue-specific phenotype is further intensified in loss-of-function flies mutant for Dicer-2, the RNase of the RNAi pathway and is accompanied by reduced insulin signaling activity that leads to increased ZIKV replication and fly sensitivity to the infection (49). A genetic screen using naturally derived Drosophila lines revealed the insulin-like receptor InR as essential for fly survival to arbovirus infection (50). Insulin signaling was further found to suppress RNAi activity, but priming with mammalian insulin enhances the immune response to control ZIKV and DENV infection through induction of genes regulated via the JAK/STAT pathway.
Recently, it was demonstrated that ZIKV infection in Drosophila adult flies upregulates several gene targets that act as negative regulators of the JAK/STAT pathway and expression of certain ZIKV structural and non-structural proteins in different tissues of transgenic flies results in restricted eye growth, which is due to reduced rate of proliferation in eye imaginal epithelia (45). In particular, overexpression of ZIKV NS4A, a dominant negative form of domeless, and co-expression of dominant negative form of domeless and NS4A driven under an eye-specific promoter induces restricted eye phenotype in a JAK/STAT dependent manner. Of note, overexpression of ZIKV NS4A in the wing reduces the size of the pouch domain, an effect that is associated with decreased Notch signaling. This information points toward a relationship between ZIKV gene expression, JAK/STAT and Notch signaling activity which is necessary for Drosophila growth and development, and induction of pathological defects in the fly (Figure 1A). In a similar manner, expression of the DENV NS3 protein (which promotes virus replication) in Drosophila transgenic flies reduces their survival response to bacterial infection, but not to abiotic stress, indicating a link between DENV NS3 activity and antimicrobial immune capacity (51). Finally, a genome-wide RNAi screen in Drosophila cells has identified a large number of genes encoding cellular factors, many of which are able to restrict WNV infection. Intriguingly, all these genes are conserved in mosquitoes and the majority have human orthologs. Furthermore, a subset of those genes (e.g., dRUVBL1 and dXPO1) reduces flavivirus infection in adult flies demonstrating the power of Drosophila for the discovery of novel host molecules with anti-flavivirus activity (52).
Conclusions and Perspectives
Flaviviruses have recently expanded globally by causing severe health impacts. Animal models are critical for understanding the molecular and physiological basis of host antiviral response and flavivirus pathogenesis. Because host innate immune responses are evolutionary conserved across many phyla, investigating the effect of flavivirus infection on the immune signaling and function of animal models is particularly informative because it can lead to the identification of anti-flavivirus immune processes in humans. Also, elucidating the scale of interactions between the host innate immune system and flaviviruses can lead to tissue-specific pathological deficits that modulate host functional changes associated with the disease. Probing the exact nature of interactions that take place during transmission of flaviviruses by mosquitoes and ticks and exploring the impact of the pathogens on tissue homeostasis during this process is considered a future research priority. Due to the close taxonomic relationship between mosquitoes and the common fruit fly (they are both members of the order Diptera), the use of Drosophila offers many advantages for studying these insect-borne viruses. Recent studies in Drosophila adult flies and larvae have been pivotal for the identification of fundamental mechanisms in insects that participate in the control of flaviviruses in the mosquito vector. If Drosophila factors interacting with flavivirus proteins are identified and characterized functionally, such findings could be extrapolated to mosquitoes after verification in the natural host (53). This approach could in turn put us in a better position to control the spread of flaviviruses in the mosquito vector, and thus enable us to prevent flavivirus dissemination to the human population.
Author Contributions
SH wrote the original draft of the manuscript and IE revised and edited the paper. All authors contributed to the article and approved the submitted version.
Conflict of Interest
The authors declare that the research was conducted in the absence of any commercial or financial relationships that could be construed as a potential conflict of interest.
Acknowledgments
We would like to thank the Department of Biological Sciences and Columbian College of Arts and Science at The George Washington University for providing support to our research.
References
1. Fernandez-Garcia M-D, Mazzon M, Jacobs M, Amara M. Pathogenesis of flavivirus infections: using and abusing the host cell. Cell Host Microbe (2009) 5:318–28. doi: 10.1016/j.chom.2009.04.001
2. Chong HY, Leow AB, Majeed ABA, Leow CH. Flavivirus infection-A review of immunopathogenesis, immunological response, and immunodiagnosis. Virus Res (2019) 274:197770. doi: 10.1016/j.virusres.2019.197770
3. Samuel GH, Adelman ZN, Myles KM. Antiviral Immunity and Virus-Mediated Antagonism in Disease Vector Mosquitoes. Trends Microbiol (2018) 26:447–61. doi: 10.1016/j.tim.2017.12.005
4. Plourde AR, Bloch EM. A Literature Review of Zika Virus. Emerg Infect Dis (2016) 22:1185–92. doi: 10.3201/eid2207.151990
5. Saldaña MA, Etebari K, Hart CE, Widen SG, Wood TG, Thangamani S, et al. Zika virus alters the microRNA expression profile and elicits an RNAi response in Aedes aegypti mosquitoes. PloS Negl Trop Dis (2017) 11:e0005760. doi: 10.1371/journal.pntd.0005760
6. Liu T, Xu Y, Wang X, Gu J, Yan G, Chen X-G. Antiviral systems in vector mosquitoes. Dev Comp Immunol (2018) 83:34–43. doi: 10.1016/j.dci.2017.12.025
7. Angleró-Rodríguez Y II, MacLeod HJ, Kang S, Carlson JS, Jupatanakul N, Dimopoulos G. Aedes aegypti Molecular Responses to Zika Virus: Modulation of Infection by the Toll and Jak/Stat Immune Pathways and Virus Host Factors. Front Microbiol (2017) 8:2050. doi: 10.3389/fmicb.2017.02050
8. Ramirez JL, Dimopoulos G. The Toll immune signaling pathway control conserved anti-dengue defenses across diverse Ae. aegypti strains and against multiple dengue virus serotypes. Dev Comp Immunol (2010) 34:625–9. doi: 10.1016/j.dci.2010.01.006
9. Sim S, Dimopoulos G. Dengue virus inhibits immune responses in Aedes aegypti cells. PloS One (2010) 5:e10678. doi: 10.1371/journal.pone.0010678
10. Paradkar PN, Trinidad L, Voysey R, Duchemin J-B, Walker PJ. Secreted Vago restricts West Nile virus infection in Culex mosquito cells by activating the Jak-STAT pathway. Proc Natl Acad Sci U S A (2012) 109:18915–20. doi: 10.1073/pnas.1205231109
11. Li S, Yu X, Feng Q. Fat Body Biology in the Last Decade. Annu Rev Entomol (2019) 64:315–33. doi: 10.1146/annurev-ento-011118-112007
12. Huang YJ, Higgs S, Horne KM, Vanlandingham DL. Flavivirus-mosquito interactions. Viruses (2014) 6:4703–30. doi: 10.3390/v6114703
13. Girard YA, Klingler KA, Higgs S. West Nile virus dissemination and tissue tropisms in orally infected Culex pipiens quinquefasciatus. Vector Borne Zoonotic Dis (2004) 4:109–22. doi: 10.1089/1530366041210729
14. Linthicum KJ, Platt K, Myint KS, Lerdthusnee K, Innis BL, Vaughn DW. Dengue 3 virus distribution in the mosquito Aedes aegypti: an immunocytochemical study. Med Vet Entomol (1996) 10:87–92. doi: 10.1111/j.1365-2915.1996.tb00086.x
15. Sriurairatna S, Bhamarapravati N. Replication of dengue-2 virus in Aedes albopictus mosquitoes. An electron microscopic study. Am J Trop Med Hyg (1977) 26:1199–205. doi: 10.4269/ajtmh.1977.26.1199
16. Zhang M, Zheng X, Wu Y, Gan M, He A, Li Z, et al. Differential proteomics of Aedes albopictus salivary gland, midgut and C6/36 cell induced by dengue virus infection. Virology (2013) 444:109–18. doi: 10.1016/j.virol.2013.06.001
17. Ocampo CB, Caicedo PA, Jaramillo G, Ursic Bedoya R, Baron O, Serrato IM, et al. Differential expression of apoptosis related genes in selected strains of Aedes aegypti with different susceptibilities to dengue virus. PloS One (2013) 8:e61187. doi: 10.1089/vbz.2007.0269
18. Eng MW, van Zuylen MN, Severson DW. Apoptosis-related genes control autophagy and influence DENV-2 infection in the mosquito vector, Aedes aegypti. Insect Biochem Mol Biol (2016) 76:70–83. doi: 10.1016/j.ibmb.2016.07.004
19. McElroy KL, Girard YA, McGee CE, Tsetsarkin KA, Vanlandingham DL, Higgs S. Characterization of the antigen distribution and tissue tropisms of three phenotypically distinct yellow fever virus variants in orally infected Aedes aegypti mosquitoes. Vector Borne Zoonotic Dis (2008) 8:675–87. doi: 10.1089/vbz.2007.0269
20. Jupatanakul N, Sim S, Angleró-Rodríguez Y II, Souza-Neto J, Das S, Poti KE, et al. Engineered Aedes aegypti JAK/STAT Pathway-Mediated Immunity to Dengue Virus. PloS Negl Trop Dis (2017) 11:e0005187. doi: 10.1371/journal.pntd.0005187
21. Serrato-Salas J, Izquierdo-Sánchez J, Argüello M, Conde R, Alvarado-Delgado A, Lanz-Mendoza H. Aedes aegypti antiviral adaptive response against DENV-2. Dev Comp Immunol (2018) 84:28–36. doi: 10.1016/j.dci.2018.01.022
22. Alonso-Palomares LA, Moreno-García M, Lanz-Mendoza H, Salazar M II. Molecular Basis for Arbovirus Transmission by Aedes aegypti Mosquitoes. Intervirology (2018) 61:255–64. doi: 10.1159/000499128
23. Franz AW, Kanor AM, Passarelli AL, Clem RJ. Tissue Barriers to Arbovirus Infection in Mosquitoes. Viruses (2015) 7:3741–67. doi: 10.3390/v7072795
24. Saraiva RG, Kang S, Simões ML, Angleró-Rodríguez Y II, Dimopoulos G. Mosquito gut antiparasitic and antiviral immunity. Dev Comp Immunol (2016) 64:53–64. doi: 10.1016/j.tim.2017.12.005
25. Sánchez-Vargas I, Scott JC, Poole-Smith BK, Franz AWE, Barbosa-Solomieu V, Wilusz J, et al. Dengue virus type 2 infections of Aedes aegypti are modulated by the mosquito’s RNA interference pathway. PloS Pathog (2009) 5:e1000299. doi: 10.1371/journal.ppat.1000299
26. Sim S, Ramirez JL, Dimopoulos G. Dengue virus infection of the Aedes aegypti salivary gland and chemosensory apparatus induces genes that modulate infection and blood-feeding behavior. PloS Pathog (2012) 8:e1002631. doi: 10.1371/journal.ppat.1002631
27. Xi Z, Ramirez JL, Dimopoulos G. The Aedes aegypti Toll pathway controls Dengue virus infection. PloS Pathog (2008) 4:e1000098. doi: 10.1371/journal.ppat.1000098
28. Colpitts TM, Cox J, Vanlandingham DL, Feitosa FM, Cheng G, Kurscheid S, et al. Alterations in the Aedes aegypti transcriptome during Infection with West Nile, Dengue and Yellow Fever viruses. PloS Pathog (2011) 7:e1002189. doi: 10.1371/journal.ppat.1002189
29. Sim S, Jupatanakul N, Ramirez JL, Kang S, Romero-Vivas CM, Mohammed H, et al. Transcriptomic profiling of diverse Aedes aegypti strains reveals increased basal-level immune activation in Dengue virus-refractory populations and identifies novel virus-vector molecular interactions. PloS Neglected Trop Dis (2013) 7:e2295. doi: 10.1371/journal.pntd.0002295
30. Souza-Neto JA, Sim S, Dimopoulos G. An evolutionary conserved function of the JAK-STAT pathway in anti-dengue defense. Proc Natl Acad Sci USA (2009) 106:17841–6. doi: 10.1073/pnas.0905006106
31. Liu B, Behura SK, Clem RJ, Schneemann A, Becnel J, Severson DW, et al. P53-mediated rapid induction of apoptosis conveys resistance to viral infection in Drosophila melanogaster. PloS Pathog (2013) 9:e1003137. doi: 10.1371/journal.ppat.1003137
32. Vaidyanathan R, Scott T. Apoptosis in mosquito midgut epithelia associated with West Nile virus infection. Apoptosis (2006) 11:1643–51. doi: 10.1007/s10495-006-8783-y
33. Wu P, Sun P, Nie K, Zhu Y, Shi M, Xiao C, et al. A Gut Commensal Bacterium Promotes Mosquito Permissiveness to Arboviruses. Cell Host Microbe (2019) 25:101–112.e5. doi: 10.1016/j.chom.2018.11.004
34. Angleró-Rodríguez Y II, Talyuli OA, Blumberg BJ, Kang S, Demby C, Shields A, et al. An Aedes aegypti-associated fungus increases susceptibility to dengue virus by modulating gut trypsin activity. Elife (2017) 6:e28844. doi: 10.7554/eLife.28844
35. Apte-Deshpande A, Paingankar M, Gokhale MD, Deobagkar DN. Serratia odorifera a midgut inhabitant of Aedes aegypti mosquito enhances its susceptibility to dengue-2 virus. PloS One (2012) 7:e40401. doi: 10.1371/journal.pone.0040401
36. Terradas G, Allen SL, Chenoweth SF, McGraw EA. Family level variation in Wolbachia-mediated dengue virus blocking in Aedes aegypti. Parasitol Vectors (2017) 10:622. doi: 10.1186/s13071-017-2589-3
37. Zhu Y, Tong L, Nie K, Wiwatanaratanabutr I, Sun P, Li Q, et al. Host serum iron modulates dengue virus acquisition by mosquitoes. Nat Microbiol (2019) 4:2405–15. doi: 10.1038/s41564-019-0555-x
38. Bottino-Rojas V, Talyuli OAC, Carrara L, Martins AJ, James AA, Oliveira PL, et al. The redox-sensing gene Nrf2 affects intestinal homeostasis, insecticide resistance, and Zika virus susceptibility in the mosquito Aedes aegypti. J Biol Chem (2018) 293:9053–63. doi: 10.1074/jbc.RA117.001589
39. Carvalho FA, Carneiro FA, Martins IC, Assunção-Miranda I, Faustino AF, Pereira RM, et al. Dengue virus capsid protein binding to hepatic lipid droplets (LD) is potassium ion dependent and is mediated by LD surface proteins. J Virol (2012) 86:2096–108. doi: 10.1128/JVI.06796-11
40. Martins IC, Gomes-Neto F, Faustino AF, Carvalho FA, Carneiro FA, Bozza PT, et al. The disordered N-terminal region of dengue virus capsid protein contains a lipid-droplet-binding motif. Biochem J (2012) 444:405–15. doi: 10.1042/BJ20112219
41. Teoh PG, Huang ZS, Pong WL, Chen PC, Wu HN. Maintenance of dimer conformation by the dengue virus core protein α4-α4’ helix pair is critical for nucleocapsid formation and virus production. J Virol (2014) 88:7998–8015. doi: 10.1128/JVI.00940-14
42. Samsa MM, Mondotte JA, Iglesias NG, Assunção-Miranda I, Barbosa-Lima G, Da Poian AT, et al. Dengue virus capsid protein usurps lipid droplets for viral particle formation. PloS Pathog (2009) 5:e1000632. doi: 10.1371/journal.ppat.1000632
43. Soto-Acosta R, Bautista-Carbajal P, Cervantes-Salazar M, Angel-Ambrocio AH, Del Angel RM. DENV up-regulates the HMG-CoA reductase activity through the impairment of AMPK phosphorylation: A potential antiviral target. PloS Pathog (2017) 13:e1006257. doi: 10.1371/journal.ppat.1006257
44. Barletta AB, Alves LR, Silva MC, Sim S, Dimopoulos G, Liechocki S, et al. Emerging role of lipid droplets in Aedes aegypti immune response against bacteria and Dengue virus. Sci Rep (2016) 6:19928. doi: 10.1038/srep19928
45. Harsh S, Fu Y, Kenney E, Han Z, Eleftherianos I. Zika virus non-structural protein NS4A restricts eye growth in Drosophila through regulation of JAK/STAT signaling. Dis Model Mech (2020) 13:dmm040816. doi: 10.1242/dmm.040816
46. Shah PS, Link N, Jang GM, Sharp PP, Zhu T, Swaney DL, et al. Comparative Flavivirus-Host Protein Interaction Mapping Reveals Mechanisms of Dengue and Zika Virus Pathogenesis. Cell (2018) 175:1931–45. doi: 10.1016/j.cell.2018.11.028
47. Link N, Chung H, Jolly A, Withers M, Tepe B, Arenkiel BR, et al. Mutations in ANKLE2, a ZIKA Virus Target, Disrupt an Asymmetric Cell Division Pathway in Drosophila Neuroblasts to Cause Microcephaly. Dev Cell (2019) 51:713–29. doi: 10.1016/j.devcel.2019.10.009
48. Liu Y, Gordesky-Gold B, Leney-Greene M, Weinbren NL, Tudor M, Cherry S. Inflammation-Induced, STING-Dependent Autophagy Restricts Zika Virus Infection in the Drosophila Brain. Cell Host Microbe (2018) 24:57–68e3. doi: 10.1016/j.chom.2018.05.022
49. Harsh S, Ozakman Y, Kitchen SM, Paquin-Proulx D, Nixon DF, Eleftherianos I. Dicer-2 Regulates Resistance and Maintains Homeostasis against Zika Virus Infection in Drosophila. J Immunol (2018) 201:3058–72. doi: 10.4049/jimmunol.1800597
50. Ahlers LRH, Trammell CE, Carrell GF, Mackinnon S, Torrevillas BK, Chow CY, et al. Insulin Potentiates JAK/STAT Signaling to Broadly Inhibit Flavivirus Replication in Insect Vectors. Cell Rep (2019) 29:1946–60. doi: 10.1016/j.celrep.2019.10.029
51. Querenet M, Danjoy ML, Mollereau B, Davoust N. Expression of dengue virus NS3 protein in Drosophila alters its susceptibility to infection. Fly (Austin) (2015) 9:1–6. doi: 10.1080/19336934.2015.1072662
52. Yasunaga A, Hanna SL, Li J, Cho H, Rose PP, Spiridigliozzi A, et al. Genome-wide RNAi screen identifies broadly-acting host factors that inhibit arbovirus infection. PloS Pathog (2014) 10:e1003914. doi: 10.1371/journal.ppat.1003914
Keywords: flavivirus, mosquito, Drosophila, immunity, homeostasis, pathophysiology
Citation: Harsh S and Eleftherianos I (2020) Flavivirus Infection and Regulation of Host Immune and Tissue Homeostasis in Insects. Front. Immunol. 11:618801. doi: 10.3389/fimmu.2020.618801
Received: 18 October 2020; Accepted: 16 November 2020;
Published: 30 November 2020.
Edited by:
Luc Swevers, National Centre of Scientific Research Demokritos, GreeceReviewed by:
Berlin L. Londono-Renteria, Kansas State University, United StatesDoug Brackney, Connecticut Agricultural Experiment Station, United States
Humberto Lanz-Mendoza, National Institute of Public Health, Mexico
Copyright © 2020 Harsh and Eleftherianos. This is an open-access article distributed under the terms of the Creative Commons Attribution License (CC BY). The use, distribution or reproduction in other forums is permitted, provided the original author(s) and the copyright owner(s) are credited and that the original publication in this journal is cited, in accordance with accepted academic practice. No use, distribution or reproduction is permitted which does not comply with these terms.
*Correspondence: Ioannis Eleftherianos, aW9hbm5pc2VAZ3d1LmVkdQ==