- 1College of Life Sciences and Medicine, Zhejiang Sci-Tech University, Hangzhou, China
- 2IND Center, Chongqing Institute of Precision Medicine and Biotechnology Co., Ltd., Chongqing, China
- 3IND Center, Chongqing Precision Biotech Co., Ltd., Chongqing, China
- 4Center for Precision Medicine of Cancer, Chongqing Key Laboratory of Translational Research for Cancer Metastasis and Individualized Treatment, Chongqing University Cancer Hospital, Chongqing, China
Bispecific antibodies (BsAbs) are antibodies with two binding sites directed at two different antigens or two different epitopes on the same antigen. The clinical therapeutic effects of BsAbs are superior to those of monoclonal antibodies (MoAbs), with broad applications for tumor immunotherapy as well as for the treatment of other diseases. Recently, with progress in antibody or protein engineering and recombinant DNA technology, various platforms for generating different types of BsAbs based on novel strategies, for various uses, have been established. More than 30 mature commercial technology platforms have been used to create and develop BsAbs based on the heterologous recombination of heavy chains and matching of light chains. The detailed mechanisms of clinical/therapeutic action have been demonstrated with these different types of BsAbs. Three kinds of BsAbs have received market approval, and more than 110 types of BsAbs are at various stages of clinical trials. In this paper, we elaborate on the classic platforms, mechanisms, and applications of BsAbs. We hope that this review can stimulate new ideas for the development of BsAbs and improve current clinical strategies.
Introduction
Various factors and multiple signaling pathways are involved in cancers and other complex diseases. Therefore, it is difficult to obtain satisfactory with MoAbs for drug resistance, and most studies on MoAb combination therapies are still in the early stage (1). Compared with MoAbs, BsAbs offer more advantages. In terms of superior cytotoxic effects, under tumorigenic conditions and infections, there is a lower rate of resistance to them due to the matched targeting of two different antigens (2). Since the original concept of BsAbs was first proposed by Nisonoff and his collaborators in the 1960s (3, 4), the first insight into antibody architecture, followed by numerous other finding, have been reported. In 1975, the invention of hybridoma technology finally solved the problem of producing pure antibodies, which marked the arrival of a new era of MoAbs therapy (5). In 1983, hybrid-hybridoma (quadroma) technology was pioneered by Milstein and Cuello (6). Then, in 1988, the Huston team invented the single-chain variable fragment (scFv), which has minimized the refolding problems, such as incorrect domain pairing or aggregation, of two-chain species (7). However, it was not until the knobs-into-holes technology emerged in 1996 that BsAbs became more developed (8). Subsequently, with progress in antibody engineering and antibody biology, the concept and technology of constructing BsAbs continued to evolve. In addition to the development of various platforms, the applications of BsAbs are diverse and the potential combination of targets is flexible. This review provides a comprehensive and systematic summary of the classic platforms, mechanisms, and applications of BsAbs.
BsAb Molecular Platforms
The main challenge encountered in the development of BsAbs is that there are two types of chains, heavy and light, that when mismatched may produce a variety of side products (9–11). Therefore, several strategies are used to achieve correct matching of heavy and light chains. BsAbs are usually divided into two types: IgG-like and non-IgG-like. The relatively large molecular weight of IgG-like BsAbs helps to purify and improve solubility and stability, increase the serum half-life and affinity, and thereby enhance biological activity (12). Non-IgG-like BsAbs only have therapeutic effects through antigen binding because of the lack of Fc fragments. They are easy to produce and have low immunogenicity (13). Further studies on the correct matching of heavy chains are needed for IgG-like BsAbs than for non-IgG-like BsAbs because of the presence of Fc. The following sections introduce some of the BsAb molecular platforms.
Platforms of IgG-Like BsAbs
The heavy chain in IgG-like BsAbs has been modified to promote heterologous Fc matching. For example, knobs-into-holes changes the local spatial structure of Fc (14, 15). The strand-exchange engineered domain (SEED) platform uses complementary sequences to promote heterodimer assembly (16). Whereas the DEKK platform uses mutations to form salt bridges (17). Orthogonal Fab (18), ART-Ig (19), and FAST-Ig depends on electrostatic manipulation for interactions. The DuoBody platform controls Fab dynamic recombination exchange (20). Dual variable domain immunoglobulin (DVD-Ig) and Fabs-in-tandem immunoglobulin (FIT-Ig) platforms are symmetrical structures (21–23). Two-in-one and other molecular platforms rely on phage display and use Crossmab and Wuxibody molecular platforms for light chains to promote correct matching (24–27).
Knobs-Into-Holes
The knobs-into-holes model is a novel and effective design for engineering antibody heavy chain homodimers for heterodimerization. The principle behind knobs-into-holes is based on replacing a smaller amino acid with a larger amino acid (T336Y) in the CH3 region of an antibody chain to form a “knobs” structure, and at the same time substituting a larger amino acid in the other chain with a smaller amino acid to form a “holes” structure (Y407T). This structure has a recombination efficiency of 57% (15). Subsequently, Merchant et al. developed a tactic for constructing human BsIgG that greatly eliminates mispairing between light chains and heavy chains. Moreover, they evaluated the effect of v1–v16 variants on the yield of Ab/IA hybrid. The mutations S354C/Y349C and Y349C/E356C are critical factors affecting the production of Ab/IA for variant v8. In addition, v11, v13, and v14 variants, which are cysteine replacement mutations, further increase Ab/IA yield. As the most potent variant, v11 (S354C: T366W/Y349C: T366S: L368A: Y407V) greatly improved the heterodimerization ratio up to a maximum of approximately 95% (8).
SEED
Heterologous recombination of the Fc segment can also be achieved via the SEED molecular platform. The alternating sequence of human IgA and IgG in the SEED CH3 domain produces two asymmetric but complementary domains called AG and GA. The SEED design allows efficient generation of AG/GA heterodimers and is not conducive to the formation of homodimers between AG and GA. Simultaneously, SEED can be combined with Fab, ScFv, and VHH structures (28). SEED fusion retains complete antibody binding affinity and has excellent biochemical and biophysical stability (29). Intravenous injection in mice showed that SEED produces pharmacokinetics with a longer serum half-life (16).
DEKK
The DEKK platform generates both L351D and L368E mutations in one of the heavy chains and simultaneously generates L351K and T366K mutations in the other heavy chain. The IgG main chain can accommodate the introduction of lysine side chains to form a stable salt bridge interaction with the opposite chain (30). MCLA-128 is based on a DEKK platform that targets HER2 and HER3 (17). MCLA-128 has shown good efficacy in clinical trial (phase II: NCT03321981) in patients with breast cancer metastasis and in another clinical trial (phase I/II: NCT02912949) in patients with pancreatic cancer and non-small cell lung cancer(NSCLC) fused with NRG1 (31). The pharmacokinetics of MCLA-128 exhibit similar disposition characteristics to those of other therapeutic MoAbs, and the impact of body-size parameters on the disposition of MCLA-128 has been found to be minimal (32).
ART-Ig
The ART-Ig platform promotes heterodimer recombination by introducing different charges in the Fc region (33). If one chain introduces (D360K, D403K) mutations, then the other chain introduces (K402D, K419D) mutations. Or if one chain introduces (T394D, P395D, P396D) mutations, then the other chain introduces (P395K, P396K, V397K) mutations. ERY947 is a BsAb based on the ART-Ig molecular platform with a common light chain targeting GPC3 and CD3. It shows high effectiveness against GPC3-expressing tumors with controllable and reversible cytokine release (34). Emicizumab is also based on this platform. A non-common light chain FAST-Ig molecular platform that introduces different charges in CH1 and CL has been developed on the basis of this platform. Based on this FAST-Ig platform, NXT007 targets FIXa/FX to exert more significant effects in treating hemophilia than emicizumab does (35).
Orthogonal Fab
The principle of this platform is the introduction of mutations to generate an “orthogonal interface” that enables preferential alignment of the different Fab domains with correct assembly (12). VRD1 (VL-Q38D VH-Q39K/VL-D1R VH-R62E) mutations and CRD2 (CL-L135Y S176W/CH1-H172A F174G) mutations are introduced in the variable region of one antibody, and the VRD2 (VL-Q38R VH-Q39Y) mutation is introduced into the region of another antibody to reduce light chain mismatches. By using this platform, BsAbs can be stably expressed in mammalian cells (18). LY3164530, which targets EGFR and c-mesenchymal-epithelial transition factor (c-MET) also uses this platform and a phase I (NCT02221882) study in patients with advanced and metastasis cancer is on-going (36). In cell-based assays, LY3164530 has been shown to exhibit superior activity in overcoming HGF-mediated resistance to erlotinib, gefitinib, lapatinib, or vemurafenib compared with the combination of individual monoclonal antibodies targeting these receptors (37).
DuoBody
The Fab arm of the IgG4 antibody can be dynamically recombined and exchanged in vivo to formed BsAbs, in a process referred to as named Fab-arm exchange (FAE) (38–40). Controlled Fab-arm exchange (cFAE) is the core technology of the Duobody platform. The Duobody platform is only necessary for promoting the completion of the Fab-arm exchange between the two antibodies to form a BsAb by introducing K409R and F405L mutation sites in the CH3 regions (41). The current BsAbs produced by this molecular platform are JNJ-63709178 (phase I: NCT02715011), which targets CD3 and CD123 for AML and JNJ-61186372 (phase I: NCT02609776), which targets EGFR and c-MET for NSCLC. JNJ-61186372 blocks ligand-induced EGFR and c-MET phosphorylation, and more effectively inhibits ERK and AKT phosphorylation and downstream signal activation (42, 43). DuoBody-CD3xCD20 has shown specific and highly potent preclinical anti-tumor activity in lymphoma models in vitro and in vivo (44).
DVD-Ig and FIT-Ig
DVD-Ig and FIT-Ig molecular platforms are symmetrical with four antigen binding sites that can target two different targets at the same time. The difference is that DVD-Ig only contains a pair of Fab domains while the FIT-Ig structure contains two pairs of Fab domains. The DVD-Ig platform contains the Fc region, and each antibody arm uses flexible short peptides to connect two variable regions (45, 46). Antibodies that use the DVD-Ig platform are ABT122 (IL-7×TNF-α), which is used to treat patients with rheumatoid arthritis (RA) who have experienced an inadequate response to methotrexate (47); and ABT165 (Delta-like ligand 4, DLL4×VEGF) which can effectively and significantly inhibit tumor growth in U87-MG human glioblastoma and SW480 human colon cancer xenograft models. ABT-165 induces a stronger anti-tumor response in combination with chemotherapeutics, and has been proven to show good efficacy and safety in vivo (48). The FIT-Ig platform was developed in 2017 (22). EpimAb has developed EMB01, using the FIT-Ig platform, to concurrently target both EGFR and c-MET in order to treat patients with advanced/metastatic solid tumors, including but not limited to NSCLC, colorectal cancer (without RAS positive mutations), gastric cancer, and liver cancer. Compared with anti EGFR mAb or anti c-MET mAb alone, EMB-01 induces significantly stronger anti-tumor activity in PDX and CDX tumor models (49).
DAF
This platform uses phage display technology to optimize the herceptin antibody with changes to 11 residues in VL CDRs to specifically bind to VEGF while retaining the ability to bind to HER2, thus achieving the binding on a single antibody can simultaneously to two targets (24). Therefore, this platform is also called the two-in-one platform or dual action fab (DAF) platform (21). MEHD7945A simultaneously targets HER3 and EGFR, and inhibits their downstream signal transduction. Compared with the monospecific anti-HER3 antibody, MEHD7945A has a wider range of efficacy in a variety of tumor models, such as pancreatic cancer, NSCLC and carcinoma of the head and neck (50, 51).
CrossMab and Wuxibody
Crossmab and Wuxibody are both used for resolving the BsAb light chain mismatch. The difference between them is that Crossmab resolves the light chain mismatch by exchanging one side of CL and CH1, while WuxiBody introduces a T cell receptor (cα and cβ) replace CL and CH1 on one side.
The CrossMab platform is mainly optimized for light chains. By swapping the regions of one side heavy chain and light chain, the BsAb light chain can be assembled correctly. It is usually combined with other platforms such as knobs-into-holes, DEEK, and ART-Ig. The process of light and heavy chain exchange can be divided into Fab exchange, variable region exchanges, and constant region exchange. Fab regions exchange produces no functional side products. Variable regions exchange produces Bence-Jones-like side products (52, 53). It has been shown that exchanging the CH1 of the heavy chain with the CL of the light chain is more effective, and this is currently used as a common method (54–56).
With the utilization of the CrossMab platform, in addition to bivalent BsAbs structures, trivalent and tetravalent BsAbs structures can also be generated. A large variety of customized BsAbs based on the CrossMab platform have entered the clinic research (55). Vanucizumab (RG7221) targets ANG-2/VEGF, in which the correct matching of the heavy chain region is achieved via the knobs-into-holes platform (57). VEGF/Ang-2 (RG7716) is the first BsAb to be used in an ophthalmology clinical study. CD3×CEA (RG7802) targets CEA positive solid tumors (58), and FAP-DR5 (RG7386) recognizes fibroblast activation protein α (FAP) and death receptor 5 (DR5) on tumor cells (59).
The Wuxibody platform is created by WuXi Biologics. It is based on the heterodimer of TCR, which replaces the CL and CH1 of the antibody with the constant region of the TCR, so that the light chain of the antibody can be matched correctly (60). The tethered-variable CL BsIgG (TcBsIgG) platform, which was developed by Genentech, also promotes the correct matching of the light chain. The antibodies VL and VH are connected with (G4S)4; then the CL and the two heavy chains are expressed together to obtain the correct BsAb (61).
Platforms of Non-IgG-Like BsAbs
The design of non-IgG-like antibodies is relatively simple because of the lack of the Fc segment. For example, bispecific T-cell engager (BiTE) uses linker to connect two ScFvs (62). The Fv of dual affinity retargeting (DART) is formed by the association of a VL partner on one chain with a VH partner on the second chain (63, 64). Other platforms involve tetravalent antiparallel structure (TandAbs) and VH only (Bi-Nanobody) (65–68). Although non-IgG-like BsAbs have high tumor tissue permeability because of their low molecular weight, they a relatively short half-life and require multiple doses (10, 13, 69).
BiTE
BiTE connects the CD3 antibody ScFv and the tumor-associated antigen (TAA) or tumor-specific antigen ScFv with G4S linker to redirect T cells to cancer cells and perform targeted killing (70, 71). In addition to blinatumomab, other BsAbs use the BiTE platform; these include CD3 × BCMA (B-cell maturation antigen) (AMG420) (72, 73), CD3 × CD33 (AMG330) (74, 75), CD3 × PSMA (prostate-specific membrane antigen) (AMG212) (76), and CD3 × EGFRvIII (epithelial growth factor receptor variant III) (AMG596) (77, 78). BsAbs on the BiTE platform are small and have a short half-life of only about 2 h which means necessitates administration with continuous intravenous infusion is needed (79, 80). Based on the BiTE platform, Amgen developed the second-generation BiTE platform, known as half-life extended BiTE (HLE-BiTE), which connects Fc to the end of ScFv extends its half-life to 7 days (81). CD3×CD19(AMG562), CD3×CD33(AMG673) (82), CD3×FLT3(FMS-like tyrosine kinase 3)(AMG427) (83), and CD3×BCMA(AMG701) all utilize the HLE-BiTE platform (84).
The combination of BiTE and chimeric antigen receptor T cell (CAR-T) immunotherapy can be applied in cancer treatment, there is an example of using BiTE platform BsAbs and CAR-T immunotherapy. EGFRvIII is overexpressed in glioblastoma cells. Elimination of EGFRvIII-positive tumor cells by CAR-T cells results in a loss of the target EGFRvIII, which consequently leads to relapse. When the BsAb sequence is added after the EGFRvIII CAR-T plasmid sequence, CAR-T cells can target EGFRvIII-positive tumor cells, and the released BsAb can recruit T cells to form a bridge, thereby killing EGFR-positive tumor cells that have lost the EGFRvIII antigen. The combination of CAR-T immunotherapy and BsAb therapy can lead to a better tumor remission than the use of either therapy alone (85).
DART
The DART platform is formed by linking VH and VL sequences with other antibody VL and VH sequences, respectively. In addition, cysteine is introduced at the C-terminus of the two polypeptide chains to form an interchain disulfide bond. Flotetuzumab (MGD006), which targets CD3 and CD123, is based on a DART platform for relapsed or refractory acute myeloid leukemia and myelodysplastic syndrome (86, 87). An cynomolgus monkey model showed that flotetuzumab was well tolerated. Of 14 patients treated in clinical trials (phase I/II: NCT02152956), 28% achieved CR. The most common side effects were infusion-related reactions and cytokine release syndrome (CRS) (87, 88). MGD007, another DART platform BsAb, targets CD3 and GPA33 for GPA-positive colorectal cancer (89). In comparison with the BiTE designs, DART achieves a higher magnitude of T cell activation (63).
TandAbs
The TandAbs platform is a tetravalent antibody molecule with two binding sites for each of two antigens (67). A homodimer molecule is formed by the reverse pairing of two peptide chains. AFM11, which targets CD3 and CD19, is based on the TandAbs platform and has more significant and marked therapeutic effects. In a xenograft model, AFM11 showed dose-dependent inhibition of the growth of Raji tumors in vivo as well as good tumor localization (66).
Bi-Nanobody
The bi-Nanobody platform connects the VH regions of two or more antibody molecules to achieve multi-specific binding. The products of this platform are small molecules that possess the advantage of high stability and better tissue permeability in vivo (90). Ozoralizumab (ATN-103, TS-152), developed by Ablynx, is a trivalent BsAb targeting albumin (ALB) and tumor necrosis factor (TNF) for RA. Currently, ozoralizumab is being test in five clinical trials, one of which has reached phase III (NCT04077567). Ablynx has also developed another BsAb to treat RA, vobarilizumab (ALX-0061), which targets ALB and IL6R. After a single intravenous injection of ALX-0061 in cynomolgus monkeys, the half-life in plasma was 6.6 days, which was similar to the expected half-life of serum albumin. In nonhuman primate models, serum c-reactive protein levels were significantly reduced (91). Figure 1 summarizes the structure of BsAbs on different platforms.
Mechanisms of Action of BsAbs
Because BsAbs have two binding sites directed at different antigens or recognize two different epitopes of one antigen simultaneously, the functioning pathways are quite flexible. Some BsAbs play the role of immune cell connector, connecting immune cells to tumor cells and enabling immune cells to exert their killing effect of immune cells. Most BsAbs target CD3, CD16, and CD47, and some release immune cells from an immunosuppressed state for dual-targeted immune checkpoints, such as PD1, CTLA-4, LAG-3 (92, 93). Some BsAbs target the immune checkpoint and TAAs, while other BsAbs target CD28 and CD137 to activate immune cell activity (94). In addition to targeting immune cells, BsAbs target tumor antigens, blocking dual signaling pathways. The main targets are PSMA, HER2, HER3, EGFR, DLL1, and ANG-2. Besides targeting dual tumor targets, some target inflammatory factors in the tumor microenvironment to reduce inflammation and CRS. Most BsAbs target tumors, although some are used to treat hemophilia A, neovascular age-related macular degeneration, diabetes, pneumonia caused by bacteria, and Alzheimer’s disease.
Recruitment and Activation of Immune Cells
CD3 is a characteristic antigen expressed on the surface of T cells. By targeting the CD3 antigen and a TAA, BsAbs can redirect T cells to cancer cells to exert a killing effect (95). Catumaxomab and blinatumomab are examples of antibodies in the market that recruit and activate immune cells. However, because catumaxomab is a murine antibody as well as an overly high-affinity antibody of CD3 (4.4 nM), T cells are overactivated, leading to CRS. AMG 424, designed by Amgen, was based on the XmAb platform which heterologous recombination from ScFv-Fc of CD3 and Fab-Fc of CD38 (96). CD38 is highly expressed in malignant multiple myeloma cells, but weakly expressed in lymphocytes and NK cells (97). Therefore, while CD38 is not an ideal target for CAR-T immunotherapy, AMG 424 has shown great therapeutic potential in animal models. AMG 424 uses the low-affinity CD3 antibody instead of the high-affinity CD3 antibody; the release of TNF-α and IFN-γ is reduced, but the effect is not changed. Furthermore, AMG 424 inhibits tumor growth in bone marrow, and thus it is the only BsAb drug approved by the FDA to treat multiple myeloma (98).
In addition to T cells, specific antigens on the surface of NK cells and macrophages are often used as targets for BsAbs. AFM13, which targets CD16A and CD30, is a tetravalent BsAb based on the TandAbs platform used to treat malignant tumors that express CD30. AFM13 recruits NK cells to exert killing effects against CD30-positive Hodgkin’s lymphoma and has low cytokine release (99). AFM13 is used to treat Hodgkin’s lymphoma. Data from a phase I study (NCT01221571) of severe relapsed or refractory Hodgkin’s lymphoma showed that AFM13 has a half-life up to 19 h; three of 26 evaluable patients achieved partial remission (11.5%) and 13 patients achieved stable disease progression (50%), the overall disease control rate was 61.5% (100). In a phase 1b study (NCT02665650), the remission rate for AFM13 combined with pembrolizumab reached 88% at the highest therapeutic dose. The ORR was 83%. A pharmacokinetic evaluation of AFM13 in combination therapy showed that its half-life extended to 20.6 h (101), demonstrating an excellent clinical effect. CD47 is often expressed on the surface of tumor cells; CD47-SIRPα releases a “don’t eat me” signal to DC cells, thus evading recognition by DC cells. NI-1801 targets CD47 and mesothelin, and NI-1701 targets CD47 and CD19 to enhance the phagocytotic effect of DC cells. NI-1801/NI-1701 depends on the κλ-body platform, which uses the same heavy chain, two different light chains, one κ chain, and one λ chain. It is a fully human bispecific IgG without any modification, with high stability and a long half-life (102, 103).
Blocking of Immune Checkpoints
Immune checkpoints, such as PD1, CTLA-4, LAG-3, have an inhibitory effect on the activity of immune cells (104). When these checkpoints are blocked with antibodies, immune cells can be reactivated. BsAbs targeting immune checkpoints are mostly indicated for solid tumors, such as AK104, MGD019, XmAb20717, MEDI5752, MGD013, RO7121661, which are dual targeting immune checkpoints. The functions of lymphocytes co-expressed by PD-1 and LAG-3 are mostly dysregulated, and combined treatment with PD-1 and LAG-3 can effectively restore T cell function (105). MGD013 is a tetravalent DART BsAb. As of April 25, 2020, the overall safety of MGD013 (including the incidence of immune-mediated adverse events) was generally consistent with PD-1 antibody monotherapy in terms of event type and frequency, whereas MGD013 had better efficacy. BsAbs of immune checkpoints combined with TAAs, such as AK112 (PD1×VEGF) and IBI315 (PD1×HER2), have also been developed.
Blocking of Inflammatory Factors
RA is a chronic inflammatory autoimmune disease of the joints. Synovial inflammation, cartilage damage, and bone erosion are the main symptoms of RA (106). Antibodies targeting proinflammatory factors TNF, IL-1β, IL-23, IL-17, IL-6, can treat RA (107). However, some patients do not respond to TNF-α. In these patients, IL-17A concentration increases and is positively correlated with the severity of the disease. Preclinical and clinical studies have shown that blocking two or more cytokines can be more efficacious than using MoAbs alone (108). ABT122, based on the DVD-Ig platform, targets TNF-α and IL-17 dual factors to treat RA. In addition, ABT-122 can quickly alter the potential pathophysiological pathways of RA patients (including reducing the chemokines CXCL9, CXCL10, and CCL23) and completely inhibit the production of IL-6 induced by TNF-α and IL-17A (109–111). RO7040547 targets IL-17 and IL-13. SAR156597 targets IL-4 and IL-13. In the CD40-induced colitis model, BsAbs target IL-23 and TNF-α shows a synergistic effect relative to antibodies against TNF-α and IL-23 alone in inflammatory bowel disease. Targeting IL-23 and IL-6, AZ17 as a BsAb in the preclinical development stage, is theoretically possible to treat inflammatory bowel disease (112, 113).
Blocking of Dual Signaling Pathways
BsAbs can target two specific antigens or different areas of the same antigen at the same time (114). Blocking signal transmission along signal pathways can affect the occurrence and development of tumors, target the tumor microenvironment, and inhibit the regeneration of tumor blood vessels. For example, BI 836880 and vanucizumab target EGFR and ANG2; ABT-165 targets DLL4×VEGF, OMP-305B83, and TR009; EMB01 targets EGFR and c-MET; and MCLA-158 targets EGFR×LGR5. ZW25 simultaneously targets different epitopes of HER2.
HER2, also known as neu, ErbB-2, CD340, and p185, is a member of the ERBB family of receptor tyrosine kinases. This family also includes ERBB1, ERBB3, and ERBB4. HER2 does not bind directly to ligands but can achieve heterodimerization with any other EGFR family receptor (115). It is the preferred heterodimerization partner for all ERBB proteins (116). Heterodimerization enhances signal transmission and initiates several downstream signaling pathways (117), including MAPK, PI3K/AKT/mTOR, SRC, and STAT signal transduction cascades, leading to cell proliferation, migration, and differentiation (118–120). The structure of HER2 includes an N-terminal extracellular domain, a transmembrane domain, and an intracellular domain. The extracellular domain comprises four domains. Trastuzumab, which targets HER2 extracellular domain IV, is used to treat metastatic breast cancer in patients overexpressing HER2 (121). Pertuzumab targets HER2 extracellular domain II, thereby inhibiting dimerization between HER receptors (122). ZW25 targets HER2 extracellular domains II and IV simultaneously. ZW25 blocks the downstream signal transmission of HER2 and has good tolerability and considerable single-dose activity (123). In one study, the ORR of patients (most with gastroesophageal cancer or colorectal cancer) was 41%, and the side effects were mild (124).
EMB-01 is generated from FIT-Ig, targeting both c-MET and EGFR to treat NSCLC (49). The EGFR antibody inhibits activation of the EGFR signaling pathway by blocking the binding of ligands and receptors. However, EGFR gene mutations and c-MET gene amplification cause EGFR antibody resistance. On the one hand, EMB-01 can prevent ligands from activating the EGFR and c-MET pathways; on the other hand, EMB-01 molecules can bind two EGFR molecules and c-MET molecules at the same time to form a complex structure on the cell surface, thereby inducing endocytosis. This process, which is irreversible, can fundamentally eliminate these two receptors on the surface of tumor cells, and achieve long-term effects. Table 1 summarizes some clinical BsAbs and their mechanisms of action.
Applications of BsAbs
BsAbs are widely used in both diagnosis and therapy. In terms of diagnosis, BsAbs can be combined with HRPO; be used in pre-targeting strategies to assist in clinical diagnosis; and provide better imaging for the early detection, diagnosis, and treatment of cancer. In terms of therapy, the main strategy is to precisely target and reactivate immune cells, help regulate the activation of immune cells, fine-tune the fate and function of immune cells, improve the tolerance of immune cells, and promote a return to immune homeostasis. In addition to tumors, BsAbs can treat other diseases, such as hemophilia A, diabetes, Alzheimer’s disease, and ophthalmological diseases.
Applications in Diagnostics
Clinical Diagnosis
Because BsAbs can bind to specific antigens and specific detection sites simultaneously, their design is flexible, sensitive, and convenient. They can reduce the harmful effects of chemical modification of enzymes or antibodies, simplify detection, and help detect infectious bacterial and viral diseases and diagnose cancer (125). Lipoarabinomannan (LAM), an important non-protein antigen of the cell wall of tuberculosis bacteria, is found in various bodily fluids of infected patients. BsAbs that target LAM and HRPO can be used for the rapid detection of tuberculosis bacteria. An evaluation of 21 stored clinical serum samples showed that the BsAb assay has 100% specificity and 64% sensitivity, furthermore, results can be obtained in 2 h, in comparison traditional laboratory culture requires 2 to 6 weeks (126). Another BsAb targeting human red blood cells (RBCs) and hepatitis B virus surface antigen (HBsAg) is used to detect HBsAg in blood samples. In an enzyme-linked immunosorbent assay, this BsAb showed specific binding to RBCs and HBsAg. Compared with conventional immunoassays, this method has a specificity of 100% and sensitivity of 97.7% and is simple and quick; no special equipment or training is needed (127, 128). Escherichia coli O157:H7 is a serious human pathogen that causes hemorrhagic colitis and hemolytic uremic syndrome. Quadroma P126 targets O157 and HRPO. E. coli O157:H7 can be quickly detected by ELISA with Quadroma P126. As above, the principal action of this BsAb is to target Bordetella pertussis LPS and HRPO with a detection limit of 5 CFU (129). Staphylococcus aureus causes wound infection. BsAbs target HRPO and S. aureus thermal nuclease (TNase) to enable detection of the presence of S. aureus (130). Finally, BsAb targeting of the nuclear plasmid protein of the SARS coronavirus immunoassay antigen is superior to that by MoAb in terms of specificity, sensitivity, and signal strength while maintaining a minimal signal background (131).
Medical Imaging
TF2 is constructed via the dock-and-lock platform, which can simultaneously recognize the histamine succinate glycine (HSG) motif and CEA (132). IMP288 is a divalent HSG hapten that can be labeled with a variety of radionuclides (90Y, 177Lu, 111In, 124I, 18F, 68Ga) (133). In clinical diagnosis, 18F fluorodeoxyglucose positron emission tomography (FDG-PET) can identify cancer and new potential lesions. However, FDG-PET has limited sensitivity to tumors smaller than 1 cm and lacks specificity for inflammatory or infectious lesions (134). Therefore, pre-targeted detection based on BsAbs is used in clinic. First, a BsAb that specifically recognizes both tumor antigens and small molecule polypeptides is injected into patient. Then, a small radiolabeled molecule is injected into the tumor location and combined with the pre-targeted BsAb when the concentration of BsAb in the blood increased to achieve blood clearance. The tumor is assessed based on radioactive radiation, and the excess radiolabeled small molecule is excreted. This method has high binding specificity, sensitivity, and safety. Pre-targeting often uses the positron nuclide 68Ga, which has a short half-life (135). In one study, the sensitivity of pre-targeting treatment with TF2 for tumor detection was 67% in a pre-targeting group but only 31% in a control group. For tumors smaller than 200 mg, sensitivity was 44% in the pre-targeting group and 0% in the control group (136). A TF2 immuno-PET and 68Ga phase II trial (NCT02587247) showed that pre-target immunization with anti-TF2 and 68Ga-labeled IMP288 was safe and feasible; 11 patients had no adverse reactions. Based on lesion analysis, the sensitivity, specificity, positive predictive value, and negative predictive value were 76%, 67%, 87%, and 33%, respectively, for the control group (FDG-PET) and 88%, 100%, 100%, and 67% for the pre-target immune group, which shows that pre-targeting with BsAbs has good diagnostic value (137). Early detection and diagnosis of pancreatic cancer remains a major clinical challenge. The anti-MUC1 MoAb PAM4 is highly specific for pancreatic adenocarcinoma. Comparison of TF10 (PAM4×HRPO) pre-targeting with that of directly radiolabeled PAM4, via imaging examination of the TF10 pre-targeting group 3 h after the radiolabeled peptide was injected showed a large amount of uptake in the tumor; however, none was detected in normal tissue. Therefore, TF10 pre-targeting can provide better imaging for early detection, diagnosis, and treatment of pancreatic cancer (138, 139).
Applications in Therapy
Tumors
Over 86% of the BsAbs developed to date are used in cancer treatment (140). During targeted cancer therapy, the half-life of BsAbs changes with their molecular size. For some BsAbs of small molecular size, such as those on the BiTE platform, it is necessary to increase their half-life, for example by adding a section of Fc to avoid multiple infusions. Some nanobodies extend the half-life by conjugation. For example, adding a human serum albumin binding domain can enlarge the molecular size and promote the localization of BsAbs (141).
BsAbs that use multivalent states targeting CD3 demonstrate a better tumor killing effect than 1 + 1, such as the TCB platform. Targeting different positions of antigens and different affinities can also cause huge differences in effect (142). The high CD3 affinity of BsAb is not necessary, because the low-affinity BsAb of CD3 shows the same killing effect in vivo and has better safety and good tolerance than the high-affinity BsAb (CD3×CLL1) of CD3 (143). Therefore, BsAbs often use a low-affinity antibody for CD3 and a high-affinity antibody for TAA (144, 145). TAAs with different levels of expression required the selection of antibodies with different affinities. Different platforms have different effects: for example, compared with scFv-based BsAbs, Fab-based BsAbs have better biophysical properties. In addition, Fab-based BsAbs can not only duplicate the activity of their scFv counterparts but also have unique biological activity (146). Figure 2 shows the structures of BsAbs with CD3 as one of their targets
Diabetes
RG7992 (BFKB8488A) targets fibroblast growth factor receptor 1 (FGFR1) and coreceptor β klotho (KLB) (147, 148). FGFR1 improves insulin sensitivity, ameliorates liver steatosis, and accelerates weight loss (149, 150). Although FGFR1 is widely expressed in many cells, β-klotho is restricted to the liver and adipose tissues (151, 152). Therefore, RG7992 can avoid extensive FGFR activation and provide clinical benefits for patients with obesity and diabetes. A clinical trial (phase I: NCT02593331) evaluated the safety, tolerability, and pharmacokinetics of BFKB8488A in overweight and obese participants who may have had insulin resistance.
Alzheimer’s Disease
BI 1034020 targets two different epitopes of Aβ (Aβ40 and Aβ42) with high affinity (153). Its purpose is to reduce the level of free Aβ peptide in plasma and thus prevent the formation of new Aβ plaques and clear existing plaques (153). Furthermore, because the antibody targeting the transferrin receptor (TfR) can cross the blood-brain barrier(BBB) of mice through TfR receptor-mediated cell transduction, BsAbs that target TfR and β-secretase 1(BACE1) can cross the BBB and reduce brain Aβ in a TfR affinity-dependent manner (154, 155). Injecting anti-TfR/BACE1 BsAb into monkeys has been shown to reduce Aβ levels in cerebrospinal fluid and brain tissue in a concentration-dependent manner (156).
In terms of crossing the BBB, ANG4043 provides a new strategy to target Angiopep-2 (An2) and HER2. An2 can bind to low-density lipoprotein-like receptor 1 (LRP1), which effectively crosses the BBB through receptor-mediated endocytosis and is taken up by cells expressing LRP1 (157). Preclinical evidence on ANG4043 shows that it not only treats HER2-positive brain metastasis but is also helpful in treating various brain tumors and other diseases of the central nervous system.
Pneumonia and Eye Diseases
The abuse of antibiotics, has led to an increase in bacterial resistance, and Pseudomonas aeruginosa has emerged as the main culprit behind clinical lung infections (158). In the rabbit lung infection model, MEDI3902 against the type 3 secreted protein (PcrV) and Psl exopolysaccharide has a protective effect in prevention and treatment. In animals treated with MEDI3902, Pseudomonas aeruginosa was found to be greatly reduced, and the organ burden and the expression of pro-inflammatory mediators in lung tissue were also significantly decrease (159, 160). A phase I (NCT02255760) dose escalation study of MEDI3902 showed that the severity of treatment-emergent adverse events (TEAE) was mild or moderate at all doses, and the serum anti-cytotoxicity and opsonizing phagocytic cell killing activity were found to be related to the serum concentration of MEDI3902. The results of the phase I study of MEDI3902 in healthy people proved its safety and effectiveness in Pseudomonas aeruginosa pneumonia (161).
Neovascular age-related macular degeneration (nAMD) is the main cause of vision loss in the elderly (162). The current standard of care for the treatment of nAMD is to inhibit VEGF (163). ANG-2 is a growth factor that plays a key role in vascular homeostasis, angiogenesis and vascular permeability. Faricimab (RG7716) targets at VEGF-A and ANG-2 shows greater efficacy than anti-VEGF-A alone in a laser-induced non-human primate model (164). The results of a phase I trial of RG7716 for nAMD showed no dose-limiting toxicity in single or multiple dose groups. In the combined single-dose groups and in the 6-mg multiple-dose group, best-corrected visual acuity (BCVA) increased from baseline to 28 days after the last dose administration by a median of 7 letters (range, 0–18 letters; n = 11) and 7.5 letters (range, 3–18 letters; n = 6). RG7716 is well tolerated and shows overall good safety (165).
BsAbs ON THE MARKET
Currently, there are only three commercially available BsAbs. Catumaxomab, which was first approved in 2009, treats malignant ascites caused by malignant solid tumors by targeting both EpCAM and CD3. Then in 2014, Amgen’s blinatumomab was approved by the FDA to treat relapsed or refractory precursor B-cell acute lymphoblastic leukemia(ALL) with CD3/CD19 dual targets (166). Finally, Roche’s emicizumab, which was approved in 2017, acts on factor FIXa/FX through the formation of a protein complex to treat bleeding due to hemophilia A (167–169). Because of the company’s strategy, catumaxomab was withdrawn from the European Union market in 2017. Thus, only blinatumomab and emicizumab have been marketed globally. Clinical trials for these three antibodies are ongoing. A total of 77 clinical research projects focuses on blinatumomab; most clinical trials are currently in phase II, while most clinical trials of emicizumab are in phase III. Remarketing of catumaxomab has also begun. Structural diagrams of the three BsAbs and related clinical information are shown in Figure 3.
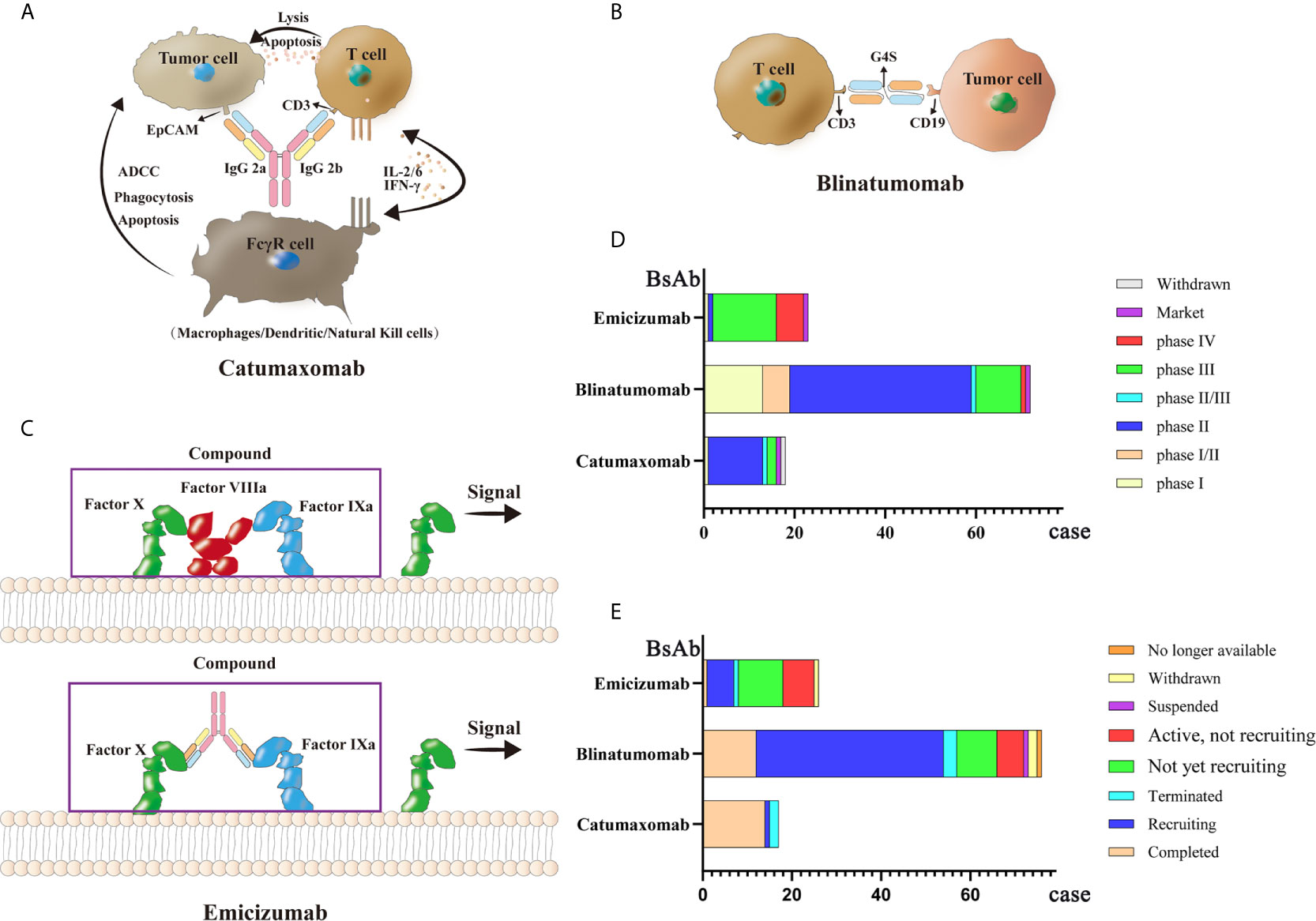
Figure 3 Mechanisms and clinical study of three market BsAbs: (A) Catumaxomab (B) Blinatumomab (C) Emicizumab (D) phase of three market BsAbs (E) status of three market BsAbs.
Catumaxomab
Catumaxomab is a Mus musculus hybrid Rattus norvegicus Sprague Dawley trifunctional BsAb. The variable region of catumaxomab specifically targets the EpCAM antigen on tumor cells and the CD3 antigen on T cells. Targeting the CD3 antigen can stimulate T cells to secrete cytokines to eliminate tumor cells (170). The Fc segment can recruit Fc receptor-containing immune cells such as macrophages, dendritic cells (DCs), and natural killer cells (NKs) (171, 172). Catumaxomab shows good clinical efficacy with relatively safe and controllable side effects in patients with malignant ascites. A phase II (NCT00326885) clinical trial was conducted to assess the effectiveness and safety of catumaxomab in patients with chemotherapy-refractory ovarian cancer and recurrent malignant ascites. Among 40 screened patients, 32 treated patients (80%) (28 cases of ovarian cancer, and four cases of primary peritoneal cancer) received four 3-h intraperitoneal catumaxomab infusions (10, 20, 50, and 150 μg within 10 days). Seven patients (23%) achieved the primary end point of at least a four-fold increase in the puncture-free interval (PuFI) relative to the pretreatment interval. The median PuFI was prolonged two-fold while the median TTPu was prolonged four-fold. An effective reduction in the formation of ascites was also observed (173, 174). The most frequent adverse reactions during catumaxomab treatment are fever, nausea, fatigue, chills, abdominal pain, elevated liver enzymes and lymphopenia. However, these adverse events are predictable, relatively controllable, and mostly reversible (175–177).
Blinatumomab
Blinatumomab acts on CD3/CD19 dual targets to treat Philadelphia chromosome-negative precursor B-cell ALL (178, 179). It is the first BsAbs drug approved by the FDA to treat leukemia cells through human T cells. Patients with ALL who are cured always have some measurable residual disease (MRD), which often causes a relapse (180, 181). After blinatumomab treatment, a certain degree of complete remission (CR) can be achieved (182–184). The main side effects of blinatumomab are fever, headache, edema, nausea, tremor, neutropenia, thrombocytopenia, and elevated transaminase (168, 185). A phase II (NCT01209286) trial was performed to determine clinical activity in patients with relapsed or refractory B precursor ALL. Thirty-six patients were treated with blinatumomab in cycles of 4-week continuous infusion; this single-arm exploratory study reported a CR or CR with partial hematological (CRh) recovery rate of 69%, with 88% of the responded patients achieving MRD response. Median overall survival (OS) was 9.8 months, and the median recurrence-free survival (RFS) was 7.6 months. The most frequent adverse reactions were fever (81%), fatigue (50%), headache (47%), tremor (36%), and leukopenia (19%), These medical events were resolved clinically (186). In another phase II clinical trial (NCT01466179), 63 out of 189 (33%) patients achieved CR and 18 (10%) patients achieved CRh. The most common adverse events were febrile neutropenia (48 cases, 25%) and anemia (27 cases, 14%) (187). Further, a phase I/II study of blinatumomab (NCT01471782) showed that of 70 patients, 27 (39%) achieved CR within the first two cycles and 14 (52%) achieved complete MRD response (188). In sum, blinatumomab has shown encouraging clinical effects.
Emicizumab
Emicizumab is used to treat patients with congenital factor VIII deficiency (189). When healthy people bleed, factor FIXa and factor FX are gathered by factor VIII to form a coagulation complex. However, because they lack coagulation factor VIII, patients with hemophilia cannot form this coagulation protein (190, 191). Emicizumab acts as a bridge, connecting factor FIXa and factor FX together, and has a hemostatic effect (192). The original factor VIII drug has low subcutaneous bioavailability, a short half-life, and poor pharmacokinetics; therefore, frequent intravenous injection is required. Emicizumab has high subcutaneous bioavailability and a half-life of more than two weeks (167), hence its use as the main drug for treating hemophilia A. In a phase III clinical trial (NCT02622321), participants who had received episodic treatment with bypassing agents (BPA) before trial were divided into group A, receiving subcutaneous emicizumab prophylaxis (35 participants) and group B, without receiving emicizumab prophylaxis (18 participants). Patients in group A showed an annualized bleeding rate of 2.9 events, while those in group B reported 23.3 events (193). Another clinical trial (NCT02847637) showed that the bleeding rate of hemophilia A patients who received a subcutaneous injection of emicizumab per week (group A) or every 2 weeks (group B) decreased significantly compared with that of patients who received no preventive measures (group C). According to this study, emicizumab therapy led to a significantly lower bleeding rate than the previously used factor VIII prophylaxis (194).
Conclusions
Progress in the field of antibody or protein engineering and recombinant DNA technology have led to the establishment of different platforms to generate BsAbs. These platforms have been applied to generate different structures of BsAbs that target different molecules or different epitopes of the same molecule. These different molecules could be either specifically from tumor cells, or from immunocytes, which enhance the anti-tumor efficacy of BsAbs. These different structures of BsAbs exert differing activity according to the status of different diseases. Therefore, the most optimal structures of BsAbs can be selected for the treatment of particular diseases. Cancers are the most common diseases for which BsAbs are developed. BsAbs have been also used in other kinds of diseases, as well as in molecular imaging for diagnosis. Three BsAbs have been approved for marketing in treatment of cancers and hemophilia A. Furthermore, 110 BsAbs have been tested in clinical trials at different stages. The future development of BsAbs will involve the development and use of new targets, new combinations, new platforms, and new geometric configurations as well as combined treatments with traditional biological drugs, other forms of immunotherapy, physical and chemical therapies. BsAbs may become a new focus of future medical and biological research and development.
Author Contributions
JM, YM, YX, YQ, WZ, YH, and JS wrote the manuscript. MT, YX, and CQ reviewed and edited the manuscript before submission. JM prepared the figures and tables. All authors contributed to the article and approved the submitted version.
Funding
This work was supported by National Key Research and Development Program (2016YFC1303405), (SQ2020YFF0401839), and National Natural Science Foundation of China (91959206).
Conflict of Interest
Authors JS and YH were employed by the company Chongqing Precision Biotech Co., Ltd. Authors YQ, WZ, MT, and YX were employed by the company Chongqing Institute of Precision Medicine and Biotechnology Co., Ltd.
The remaining authors declare that the research was conducted in the absence of any commercial or financial relationships that could be construed as a potential conflict of interest.
Acknowledgments
We thank miss Mengzu Wang for language editing.
References
1. Henricks LM, Schellens JH, Huitema AD, Beijnen JH. The Use of Combinations of Monoclonal Antibodies in Clinical Oncology. Cancer Treat Rev (2015) 41(10):859–67. doi: 10.1016/j.ctrv.2015.10.008
2. Schmid AS, Neri D. Advances in Antibody Engineering for Rheumatic Diseases. Nat Rev Rheumatol (2019) 15(4):197–207. doi: 10.1038/s41584-019-0188-8
3. Nisonoff A, Wissler FC, Lipman LN. Properties of the Major Component of a Peptic Digest of Rabbit Antibody. Science (1960) 132(3441):1770–1. doi: 10.1126/science.132.3441.1770
4. Nisonoff A, Rivers MM. Recombination of a Mixture of Univalent Antibody Fragments of Different Specificity. Arch Biochem Biophys (1961) 93:460–2. doi: 10.1016/0003-9861(61)90296-x
5. Köhler G, Milstein C. Continuous Cultures of Fused Cells Secreting Antibody of Predefined Specificity. Nature (1975) 256(5517):495–7. doi: 10.1038/256495a0
6. Milstein C, Cuello AC. Hybrid Hybridomas and Their Use in Immunohistochemistry. Nature (1983) 305(5934):537–40. doi: 10.1038/305537a0
7. Huston JS, Levinson D, Mudgett-Hunter M, Tai MS, Novotný J, Margolies MN, et al. Protein Engineering of Antibody Binding Sites: Recovery of Specific Activity in an Anti-Digoxin Single-Chain Fv Analogue Produced in Escherichia Coli. Proc Natl Acad Sci U S A (1988) 85(16):5879–83. doi: 10.1073/pnas.85.16.5879
8. Merchant AM, Zhu Z, Yuan JQ, Goddard A, Adams CW, Presta LG, et al. An Efficient Route to Human Bispecific IgG. Nat Biotechnol (1998) 16(7):677–81. doi: 10.1038/nbt0798-677
9. Wang Q, Chen Y, Park J, Liu X, Hu Y, Wang T, et al. Design and Production of Bispecific Antibodies. Antibodies (Basel) (2019) 8(3):43. doi: 10.3390/antib8030043
10. Brinkmann U, Kontermann RE. The Making of Bispecific Antibodies. MAbs (2017) 9(2):182–212. doi: 10.1080/19420862.2016.1268307
11. Krah S, Kolmar H, Becker S, Zielonka S. Engineering IgG-Like Bispecific Antibodies-An Overview. Antibodies (Basel) (2018) 7(3):28. doi: 10.3390/antib7030028
12. Kontermann RE, Brinkmann U. Bispecific Antibodies. Drug Discov Today (2015) 20(7):838–47. doi: 10.1016/j.drudis.2015.02.008
13. Fan G, Wang Z, Hao M, Li J. Bispecific Antibodies and Their Applications. J Hematol Oncol (2015) 8:130. doi: 10.1186/s13045-015-0227-0
14. Xu Y, Lee J, Tran C, Heibeck TH, Wang WD, Yang J, et al. Production of Bispecific Antibodies in “Knobs-Into-Holes” Using a Cell-Free Expression System. MAbs (2015) 7(1):231–42. doi: 10.4161/19420862.2015.989013
15. Ridgway JB, Presta LG, Carter P. ‘Knobs-Into-Holes’ Engineering of Antibody CH3 Domains for Heavy Chain Heterodimerization. Protein Eng (1996) 9(7):617–21. doi: 10.1093/protein/9.7.617
16. Davis JH, Aperlo C, Li Y, Kurosawa E, Lan Y, Lo K-M, et al. Seedbodies: Fusion Proteins Based on Strand-Exchange Engineered Domain (SEED) CH3 Heterodimers in an Fc Analogue Platform for Asymmetric Binders or Immunofusions and Bispecific Antibodies. Protein Eng Des Sel (2010) 23(4):195–202. doi: 10.1093/protein/gzp094
17. De Nardis C, Hendriks LJA, Poirier E, Arvinte T, Gros P, Bakker ABH, et al. A New Approach for Generating Bispecific Antibodies Based on a Common Light Chain Format and the Stable Architecture of Human Immunoglobulin G. J Biol Chem (2017) 292(35):14706–17. doi: 10.1074/jbc.M117.793497
18. Lewis SM, Wu X, Pustilnik A, Sereno A, Huang F, Rick HL, et al. Generation of Bispecific IgG Antibodies by Structure-Based Design of an Orthogonal Fab Interface. Nat Biotechnol (2014) 32(2):191–8. doi: 10.1038/nbt.2797
19. Igawa T. [Next Generation Antibody Therapeutics Using Bispecific Antibody Technology]. Yakugaku Zasshi (2017) 137(7):831–6. doi: 10.1248/yakushi.16-00252-3
20. Labrijn AF, Meesters JI, de Goeij BE, van den Bremer ET, Neijssen J, van Kampen MD, et al. Efficient Generation of Stable Bispecific IgG1 by Controlled Fab-arm Exchange. Proc Natl Acad Sci U S A (2013) 110(13):5145–50. doi: 10.1073/pnas.1220145110
21. Bostrom J, Yu SF, Kan D, Appleton BA, Lee CV, Billeci K, et al. Variants of the Antibody Herceptin That Interact With HER2 and VEGF At the Antigen Binding Site. Science (2009) 323(5921):1610–4. doi: 10.1126/science.1165480
22. Gong S, Ren F, Wu D, Wu X, Wu C. Fabs-in-Tandem Immunoglobulin is a Novel and Versatile Bispecific Design for Engaging Multiple Therapeutic Targets. mAbs (2017) 9(7):1118–28. doi: 10.1080/19420862.2017.1345401
23. Gong S, Wu C. Generation of Fabs-in-tandem Immunoglobulin Molecules for Dual-Specific Targeting. Methods (2019) 154:87–92. doi: 10.1016/j.ymeth.2018.07.014
24. Eigenbrot C, Fuh G. Two-in-One Antibodies With Dual Action Fabs. Curr Opin Chem Biol (2013) 17(3):400–5. doi: 10.1016/j.cbpa.2013.04.015
25. Lee CV, Koenig P, Fuh G. A Two-in-One Antibody Engineered From a Humanized Interleukin 4 Antibody Through Mutation in Heavy Chain Complementarity-Determining Regions. MAbs (2014) 6(3):622–7. doi: 10.4161/mabs.28483
26. Sustmann C, Dickopf S, Regula JT, Kettenberger H, Mølhøj M, Gassner C, et al. DuoMab: A Novel CrossMab-based IgG-derived Antibody Format for Enhanced Antibody-Dependent Cell-Mediated Cytotoxicity. MAbs (2019) 11(8):1402–14. doi: 10.1080/19420862.2019.1661736
27. Wan Y, Zhang T, Wang Y, Wang Y, Li Y. Removing Light Chain-Missing Byproducts and Aggregates by Capto Mmc ImpRes Mixed-Mode Chromatography During the Purification of Two WuXiBody-based Bispecific Antibodies. Protein Expr Purif (2020) 175:105712. doi: 10.1016/j.pep.2020.105712
28. Dietrich S, Gross AW, Becker S, Hock B, Stadlmayr G, Rüker F, et al. Constant Domain-Exchanged Fab Enables Specific Light Chain Pairing in Heterodimeric Bispecific SEED-Antibodies. Biochim Biophys Acta Proteins Proteom (2020) 1868(1):140250. doi: 10.1016/j.bbapap.2019.07.003
29. Muda M, Gross AW, Dawson JP, He C, Kurosawa E, Schweickhardt R, et al. Therapeutic Assessment of SEED: A New Engineered Antibody Platform Designed to Generate Mono- and Bispecific Antibodies. Protein Eng Des Sel (2011) 24(5):447–54. doi: 10.1093/protein/gzq123
30. De Nardis C, Hendriks LJA, Poirier E, Arvinte T, Gros P, Bakker ABH, et al. A New Approach for Generating Bispecific Antibodies Based on a Common Light Chain Format and the Stable Architecture of Human Immunoglobulin G(1). J Biol Chem (2017) 292(35):14706–17. doi: 10.1074/jbc.M117.793497
31. Rose S. MCLA-128 Fights NRG1 Fusion-Positive Cancers. Cancer Discov (2019) 9(12):1636. doi: 10.1158/2159-8290.Cd-nb2019-128
32. de Vries Schultink AHM, Bol K, Doornbos RP, Murat A, Wasserman E, Dorlo TPC, et al. Population Pharmacokinetics of MCLA-128, a HER2/HER3 Bispecific Monoclonal Antibody, in Patients With Solid Tumors. Clin Pharmacokinet (2020) 59(7):875–84. doi: 10.1007/s40262-020-00858-2
33. Shiraiwa H, Narita A, Kamata-Sakurai M, Ishiguro T, Sano Y, Hironiwa N, et al. Engineering a Bispecific Antibody With a Common Light Chain: Identification and Optimization of an anti-CD3 Epsilon and anti-GPC3 Bispecific Antibody, ERY974. Methods (2019) 154:10–20. doi: 10.1016/j.ymeth.2018.10.005
34. Ishiguro T, Sano Y, Komatsu SI, Kamata-Sakurai M, Kaneko A, Kinoshita Y, et al. An Anti-Glypican 3/CD3 Bispecific T Cell-Redirecting Antibody for Treatment of Solid Tumors. Sci Transl Med (2017) 9(410):eaal4291. doi: 10.1126/scitranslmed.aal4291
35. Yamaguchi K, Soeda T, Sato M, Shibahara N, Koga H, Ichiki M, et al. Pharmacology and Pharmacokinetics of NXT007; Emicizumab-Based Engineered Fixa/Fx Bispecific Antibody With Improved Properties. Blood (2020) 136(Supplement 1):19. doi: 10.1182/blood-2020-138958
36. Patnaik A, Gordon M, Tsai F, Papadopoulos KP, Rasco D, Beeram M, et al. A Phase I Study of LY3164530, a Bispecific Antibody Targeting MET and EGFR, in Patients With Advanced or Metastatic Cancer. Cancer Chemother Pharmacol (2018) 82(3):407–18. doi: 10.1007/s00280-018-3623-7
37. Liu L, Zeng W, Chedid M, Zeng Y, Tschang S-H, Tian Y, et al. A Novel MET-EGFR Bispecific Antibody LY3164530 Shows Advantage Over Combining MET and EGFR Antibodies in Tumor Inhibition and Overcome Resistance. Cancer Res (2016) 76(14 suppl). doi: 10.1158/1538-7445.Am2016-873
38. van der Neut Kolfschoten M, Schuurman J, Losen M, Bleeker WK, Martínez-Martínez P, Vermeulen E, et al. Anti-Inflammatory Activity of Human IgG4 Antibodies by Dynamic Fab Arm Exchange. Science (2007) 317(5844):1554–7. doi: 10.1126/science.1144603
39. Chen Z, Qian Y, Song Y, Xu X, Tao L, Mussa N, et al. Design of Next-Generation Therapeutic IgG4 With Improved Manufacturability and Bioanalytical Characteristics. MAbs (2020) 12(1):1829338. doi: 10.1080/19420862.2020.1829338
40. Schuurman J, Van Ree R, Perdok GJ, Van Doorn HR, Tan KY, Aalberse RC. Normal Human Immunoglobulin G4 is Bispecific: it has Two Different Antigen-Combining Sites. Immunology (1999) 97(4):693–8. doi: 10.1046/j.1365-2567.1999.00845.x
41. Labrijn AF, Meesters JI, Priem P, de Jong RN, van den Bremer ETJ, van Kampen MD, et al. Controlled Fab-arm Exchange for the Generation of Stable Bispecific IgG1. Nat Protoc (2014) 9(10):2450–63. doi: 10.1038/nprot.2014.169
42. Grugan KD, Dorn K, Jarantow SW, Bushey BS, Pardinas JR, Laquerre S, et al. Fc-Mediated Activity of EGFR X c-Met Bispecific Antibody JNJ-61186372 Enhanced Killing of Lung Cancer Cells. MAbs (2017) 9(1):114–26. doi: 10.1080/19420862.2016.1249079
43. Moores SL, Chiu ML, Bushey BS, Chevalier K, Luistro L, Dorn K, et al. A Novel Bispecific Antibody Targeting EGFR and cMet Is Effective Against EGFR Inhibitor-Resistant Lung Tumors. Cancer Res (2016) 76(13):3942–53. doi: 10.1158/0008-5472.Can-15-2833
44. Engelberts PJ, Hiemstra IH, de Jong B, Schuurhuis DH, Meesters J, Beltran Hernandez I, et al. DuoBody-CD3xCD20 Induces Potent T-cell-mediated Killing of Malignant B Cells in Preclinical Models and Provides Opportunities for Subcutaneous Dosing. EBioMedicine (2020) 52:102625. doi: 10.1016/j.ebiom.2019.102625
45. Wu C, Ying H, Grinnell C, Bryant S, Miller R, Clabbers A, et al. Simultaneous Targeting of Multiple Disease Mediators by a Dual-Variable-Domain Immunoglobulin. Nat Biotechnol (2007) 25(11):1290–7. doi: 10.1038/nbt1345
46. DiGiammarino E, Ghayur T, Liu J. Design and Generation of DVD-Ig™ Molecules for Dual-Specific Targeting. Methods Mol Biol (2012) 899:145–56. doi: 10.1007/978-1-61779-921-1_9
47. Miossec P, Kolls JK. Targeting IL-17 and TH17 Cells in Chronic Inflammation. Nat Rev Drug Discov (2012) 11(10):763–76. doi: 10.1038/nrd3794
48. Hou W, Yuan Q, Yuan X, Wang Y, Mo W, Wang H, et al. A Novel Tetravalent Bispecific Antibody Targeting Programmed Death 1 and Tyrosine-Protein Kinase Met for Treatment of Gastric Cancer. Invest New Drugs (2019) 37(5):876–89. doi: 10.1007/s10637-018-0689-3
49. Ren F, Wu X, Yang D, Wu D, Gong S, Zhang Y, et al. Abstract 528: EMB-01: An Innovative Bispecific Antibody Targeting EGFR and cMet on Tumor Cells Mediates a Novel Mechanism to Improve Anti-Tumor Efficacy. Cancer Res (2020) 80(16 Supplement):528. doi: 10.1158/1538-7445.Am2020-528
50. Schaefer G, Haber L, Crocker LM, Shia S, Shao L, Dowbenko D, et al. A Two-in-One Antibody Against HER3 and EGFR has Superior Inhibitory Activity Compared With Monospecific Antibodies. Cancer Cell (2011) 20(4):472–86. doi: 10.1016/j.ccr.2011.09.003
51. Jimeno A, Machiels JP, Wirth L, Specenier P, Seiwert TY, Mardjuadi F, et al. Phase Ib Study of Duligotuzumab (MEHD7945A) Plus Cisplatin/5-Fluorouracil or Carboplatin/Paclitaxel for First-Line Treatment of Recurrent/Metastatic Squamous Cell Carcinoma of the Head and Neck. Cancer (2016) 122(24):3803–11. doi: 10.1002/cncr.30256
52. Gally JA, Edelman GM. Protein-Protein Interactions Among L Polypeptide Chains of Bence-Jones Proteins and Human Gamma-Globulins. J Exp Med (1964) 119(5):817–36. doi: 10.1084/jem.119.5.817
53. Schiffer M, Hardman KD, Wood MK, Edmundson AB, Hood ME, Ely KR, et al. A Preliminary Crystallographic Investigation of Human L-type Bence-Jones Protein. J Biol Chem (1970) 245(4):728–30. doi: 10.1016/S0021-9258(18)63324-1
54. Schaefer W, Regula JT, Bähner M, Schanzer J, Croasdale R, Dürr H, et al. Immunoglobulin Domain Crossover as a Generic Approach for the Production of Bispecific IgG Antibodies. Proc Natl Acad Sci U S A (2011) 108(27):11187–92. doi: 10.1073/pnas.1019002108
55. Klein C, Schaefer W, Regula JT. The Use of CrossMAb Technology for the Generation of Bi- and Multispecific Antibodies. MAbs (2016) 8(6):1010–20. doi: 10.1080/19420862.2016.1197457
56. Regula JT, Imhof-Jung S, Mølhøj M, Benz J, Ehler A, Bujotzek A, et al. Variable Heavy–Variable Light Domain and Fab-arm CrossMabs With Charged Residue Exchanges to Enforce Correct Light Chain Assembly. Protein Eng Des Sel (2018) 31(7-8):289–99. doi: 10.1093/protein/gzy021
57. Kienast Y, Klein C, Scheuer W, Raemsch R, Lorenzon E, Bernicke D, et al. Ang-2-VEGF-a CrossMab, a Novel Bispecific Human IgG1 Antibody Blocking VEGF-A and Ang-2 Functions Simultaneously, Mediates Potent Antitumor, Antiangiogenic, and Antimetastatic Efficacy. Clin Cancer Res (2013) 19(24):6730–40. doi: 10.1158/1078-0432.Ccr-13-0081
58. Lehmann S, Perera R, Grimm HP, Sam J, Colombetti S, Fauti T, et al. In Vivo Fluorescence Imaging of the Activity of CEA TCB, a Novel T-Cell Bispecific Antibody, Reveals Highly Specific Tumor Targeting and Fast Induction of T-Cell-Mediated Tumor Killing. Clin Cancer Res (2016) 22(17):4417–27. doi: 10.1158/1078-0432.Ccr-15-2622
59. Brünker P, Wartha K, Friess T, Grau-Richards S, Waldhauer I, Koller CF, et al. RG7386, a Novel Tetravalent FAP-DR5 Antibody, Effectively Triggers FAP-Dependent, Avidity-Driven DR5 Hyperclustering and Tumor Cell Apoptosis. Mol Cancer Ther (2016) 15(5):946–57. doi: 10.1158/1535-7163.Mct-15-0647
60. Guo G, Han J, Wang Y, Li Y. A Potential Downstream Platform Approach for WuXiBody-based IgG-like Bispecific Antibodies. Protein Expr Purif (2020) 173:105647. doi: 10.1016/j.pep.2020.105647
61. Kim HS, Dunshee DR, Yee A, Tong RK, Kim I, Farahi F, et al. Tethered-Variable CL Bispecific IgG: An Antibody Platform for Rapid Bispecific Antibody Screening. Protein Eng Des Sel (2017) 30(9):627–37. doi: 10.1093/protein/gzx034
62. Huehls AM, Coupet TA, Sentman CL. Bispecific T-cell Engagers for Cancer Immunotherapy. Immunol Cell Biol (2015) 93(3):290–6. doi: 10.1038/icb.2014.93
63. Moore PA, Zhang W, Rainey GJ, Burke S, Li H, Huang L, et al. Application of Dual Affinity Retargeting Molecules to Achieve Optimal Redirected T-cell Killing of B-cell Lymphoma. Blood (2011) 117(17):4542–51. doi: 10.1182/blood-2010-09-306449
64. Johnson S, Burke S, Huang L, Gorlatov S, Li H, Wang W, et al. Effector Cell Recruitment With Novel Fv-based Dual-Affinity Re-Targeting Protein Leads to Potent Tumor Cytolysis and In Vivo B-cell Depletion. J Mol Biol (2010) 399(3):436–49. doi: 10.1016/j.jmb.2010.04.001
65. Reusch U, Harrington KH, Gudgeon CJ, Fucek I, Ellwanger K, Weichel M, et al. Characterization of CD33/CD3 Tetravalent Bispecific Tandem Diabodies (TandAbs) for the Treatment of Acute Myeloid Leukemia. Clin Cancer Res (2016) 22(23):5829–38. doi: 10.1158/1078-0432.Ccr-16-0350
66. Reusch U, Duell J, Ellwanger K, Herbrecht C, Knackmuss SH, Fucek I, et al. A Tetravalent Bispecific TandAb (CD19/CD3), AFM11, Efficiently Recruits T Cells for the Potent Lysis of CD19(+) Tumor Cells. MAbs (2015) 7(3):584–604. doi: 10.1080/19420862.2015.1029216
67. Kipriyanov SM, Moldenhauer G, Schuhmacher J, Cochlovius B, Von der Lieth CW, Matys ER, et al. Bispecific Tandem Diabody for Tumor Therapy With Improved Antigen Binding and Pharmacokinetics. J Mol Biol (1999) 293(1):41–56. doi: 10.1006/jmbi.1999.3156
68. Els Conrath K, Lauwereys M, Wyns L, Muyldermans S. Camel Single-Domain Antibodies as Modular Building Units in Bispecific and Bivalent Antibody Constructs. J Biol Chem (2001) 276(10):7346–50. doi: 10.1074/jbc.M007734200
69. Ahamadi-Fesharaki R, Fateh A, Vaziri F, Solgi G, Siadat SD, Mahboudi F, et al. Single-Chain Variable Fragment-Based Bispecific Antibodies: Hitting Two Targets With One Sophisticated Arrow. Mol Ther Oncolytics (2019) 14:38–56. doi: 10.1016/j.omto.2019.02.004
70. Ross SL, Sherman M, McElroy PL, Lofgren JA, Moody G, Baeuerle PA, et al. Bispecific T Cell Engager (BiTE®) Antibody Constructs can Mediate Bystander Tumor Cell Killing. PloS One (2017) 12(8):e0183390. doi: 10.1371/journal.pone.0183390
71. Wolf E, Hofmeister R, Kufer P, Schlereth B, Baeuerle PA. BiTEs: Bispecific Antibody Constructs With Unique Anti-Tumor Activity. Drug Discov Today (2005) 10(18):1237–44. doi: 10.1016/s1359-6446(05)03554-3
72. Verkleij CPM, Frerichs KA, Broekmans M, Absalah S, Maas-Bosman PWC, Kruyswijk S, et al. T-Cell Redirecting Bispecific Antibodies Targeting BCMA for the Treatment of Multiple Myeloma. Oncotarget (2020) 11(45):4076–81. doi: 10.18632/oncotarget.27792
73. Topp MS, Duell J, Zugmaier G, Attal M, Moreau P, Langer C, et al. Evaluation of AMG 420, an anti-BCMA Bispecific T-cell Engager (BiTE) Immunotherapy, in R/R Multiple Myeloma (MM) Patients: Updated Results of a First-in-Human (FIH) Phase I Dose Escalation Study. J Clin Oncol (2019) 37(15_suppl):8007. doi: 10.1200/JCO.2019.37.15_suppl.8007
74. Aigner M, Feulner J, Schaffer S, Kischel R, Kufer P, Schneider K, et al. T Lymphocytes can be Effectively Recruited for Ex Vivo and In Vivo Lysis of AML Blasts by a Novel CD33/CD3-bispecific BiTE Antibody Construct. Leukemia (2013) 27(5):1107–15. doi: 10.1038/leu.2012.341
75. Ravandi F, Stein AS, Kantarjian HM, Walter RB, Paschka P, Jongen-Lavrencic M, et al. A Phase 1 First-in-Human Study of AMG 330, an Anti-CD33 Bispecific T-Cell Engager (Bite®) Antibody Construct, in Relapsed/Refractory Acute Myeloid Leukemia (R/R AML). Blood (2018) 132(Supplement 1):25–. doi: 10.1182/blood-2018-99-109762
76. Friedrich M, Raum T, Lutterbuese R, Voelkel M, Deegen P, Rau D, et al. Regression of Human Prostate Cancer Xenografts in Mice by AMG 212/BAY2010112, a Novel PSMA/CD3-Bispecific BiTE Antibody Cross-Reactive With non-Human Primate Antigens. Mol Cancer Ther (2012) 11(12):2664–73. doi: 10.1158/1535-7163.Mct-12-0042
77. Rosenthal MA, Balana C, van Linde ME, Sayehli C, Fiedler WM, Wermke M, et al. Atim-49 (Ltbk-01). AMG 596, A Novel Anti-EGFRvIII Bispecific T Cell Engager (Bite®) Molecule for the treatment of glioblastoma (GBM): Planned Interim Analysis in recurrent GBM (rGBM). Neuro Oncol (2019) 21(Supplement_6):vi283–vi. doi: 10.1093/neuonc/noz219.1195
78. Rosenthal M, Balana C, Linde MEV, Sayehli C, Fiedler WM, Wermke M, et al. Novel anti-EGFRvIII Bispecific T Cell Engager (BiTE) Antibody Construct in Glioblastoma (GBM): Trial in Progress of AMG 596 in Patients With Recurrent or Newly Diagnosed Disease. J Clin Oncol (2019) 37(15_suppl):TPS2071–TPS. doi: 10.1200/JCO.2019.37.15_suppl.TPS2071
79. Arvedson T, Balazs M, Bogner P, Black K, Graham K, Henn A, et al. Abstract 55: Generation of Half-Life Extended Anti-CD33 BiTE® Antibody Constructs Compatible With Once-Weekly Dosing. Cancer Res (2017) 77:55–. doi: 10.1158/1538-7445.AM2017-55
80. Einsele H, Borghaei H, Orlowski RZ, Subklewe M, Roboz GJ, Zugmaier G, et al. The BiTE (Bispecific T-cell Engager) Platform: Development and Future Potential of a Targeted Immuno-Oncology Therapy Across Tumor Types. Cancer (2020) 126(14):3192–201. doi: 10.1002/cncr.32909
81. Lorenczewski G, Friedrich M, Kischel R, Dahlhoff C, Anlahr J, Balazs M, et al. Generation of a Half-Life Extended Anti-CD19 Bite® Antibody Construct Compatible With Once-Weekly Dosing for Treatment of CD19-Positive Malignancies. Blood (2017) 130(Supplement 1):2815–. doi: 10.1182/blood.V130.Suppl_1.2815.2815
82. Subklewe M, Stein A, Walter RB, Bhatia R, Wei AH, Ritchie D, et al. Preliminary Results From a Phase 1 First-in-Human Study of AMG 673, a Novel Half-Life Extended (HLE) Anti-CD33/CD3 BiTE® (Bispecific T-Cell Engager) in Patients With Relapsed/Refractory (R/R) Acute Myeloid Leukemia (AML). Blood (2019) 134(Supplement_1):833–. doi: 10.1182/blood-2019-127977
83. Brauchle B, Goldstein RL, Karbowski CM, Henn A, Li C-M, Bücklein VL, et al. Characterization of a Novel FLT3 BiTE Molecule for the Treatment of Acute Myeloid Leukemia. Mol Cancer Ther (2020) 19(9):1875–88. doi: 10.1158/1535-7163.Mct-19-1093
84. Cho S-F, Lin L, Xing L, Wen K, Yu T, Wahl J, et al. AMG 701, a Half-Life Extended Anti-BCMA BiTE®, Potently Induces T Cell-Redirected Lysis of Human Multiple Myeloma Cells and can be Combined With IMiDs to Overcome the Immunosuppressive Bone Marrow Microenvironment. Clin Lymphoma Myeloma Leuk (2019) 19(10):e54. doi: 10.1016/j.clml.2019.09.082
85. Choi BD, Yu X, Castano AP, Bouffard AA, Schmidts A, Larson RC, et al. CAR-T Cells Secreting BiTEs Circumvent Antigen Escape Without Detectable Toxicity. Nat Biotechnol (2019) 37(9):1049–58. doi: 10.1038/s41587-019-0192-1
86. Campagne O, Delmas A, Fouliard S, Chenel M, Chichili GR, Li H, et al. Integrated Pharmacokinetic/Pharmacodynamic Model of a Bispecific CD3xCD123 DART Molecule in Nonhuman Primates: Evaluation of Activity and Impact of Immunogenicity. Clin Cancer Res (2018) 24(11):2631–41. doi: 10.1158/1078-0432.Ccr-17-2265
87. Chichili GR, Huang L, Li H, Burke S, He L, Tang Q, et al. A CD3xCD123 Bispecific DART for Redirecting Host T Cells to Myelogenous Leukemia: Preclinical Activity and Safety in Nonhuman Primates. Sci Transl Med (2015) 7(289):289ra82. doi: 10.1126/scitranslmed.aaa5693
88. Guy DG, Uy GL. Bispecific Antibodies for the Treatment of Acute Myeloid Leukemia. Curr Hematol Malig Rep (2018) 13(6):417–25. doi: 10.1007/s11899-018-0472-8
89. Moore PA, Shah K, Yang Y, Alderson R, Roberts P, Long V, et al. Development of MGD007, a gpA33 X CD3-Bispecific Dart Protein for T-Cell Immunotherapy of Metastatic Colorectal Cancer. Mol Cancer Ther (2018) 17(8):1761–72. doi: 10.1158/1535-7163.Mct-17-1086
90. Bannas P, Hambach J, Koch-Nolte F. Nanobodies and Nanobody-Based Human Heavy Chain Antibodies as Antitumor Therapeutics. Front Immunol (2017) 8:1603. doi: 10.3389/fimmu.2017.01603
91. Van Roy M, Ververken C, Beirnaert E, Hoefman S, Kolkman J, Vierboom M, et al. The Preclinical Pharmacology of the High Affinity Anti-IL-6R Nanobody® ALX-0061 Supports its Clinical Development in Rheumatoid Arthritis. Arthritis Res Ther (2015) 17(1):135. doi: 10.1186/s13075-015-0651-0
92. Anderson AC, Joller N, Kuchroo VK. Lag-3, Tim-3, and TIGIT: Co-Inhibitory Receptors With Specialized Functions in Immune Regulation. Immunity (2016) 44(5):989–1004. doi: 10.1016/j.immuni.2016.05.001
93. Deak LLC, Seeber S, Perro M, Weber P, Lauener L, Chen S, et al. Abstract 2270: RG7769 (PD1-TIM3), a Novel Heterodimeric Avidity-Driven T Cell Specific PD-1/TIM-3 Bispecific Antibody Lacking Fc-mediated Effector Functions for Dual Checkpoint Inhibition to Reactivate Dysfunctional T Cells. Cancer Res (2020) 80(16 Supplement):2270–. doi: 10.1158/1538-7445.Am2020-2270
94. Liu L, Lam C-YK, Long V, Widjaja L, Yang Y, Shah K, et al. Abstract 3642: Tumor-antigen Expression-Dependent Activation of the CD137 Costimulatory Pathway by Bispecific DART® Proteins. Cancer Res (2017) 77(13 Supplement):3642–. doi: 10.1158/1538-7445.Am2017-3642
95. Zhou Y, Zong H, Han L, Xie Y, Jiang H, Gilly J, et al. A Novel Bispecific Antibody Targeting CD3 and Prolactin Receptor (PRLR) Against PRLR-expression Breast Cancer. J Exp Clin Cancer Res (2020) 39(1):87. doi: 10.1186/s13046-020-01564-4
96. de Zafra C, Balazs M, Fajardo F, Liang L, Zhong W, Henn A, et al. Preclinical Characterization of AMG 424, a Novel Humanized T Cell-Recruiting Bispecific Anti-CD3/CD38 Antibody. Blood (2017) 130(Supplement 1):500–. doi: 10.1182/blood.V130.Suppl_1.500.500
97. Lin P, Owens R, Tricot G, Wilson CS. Flow Cytometric Immunophenotypic Analysis of 306 Cases of Multiple Myeloma. Am J Clin Pathol (2004) 121(4):482–8. doi: 10.1309/74R4TB90BUWH27JX
98. Zuch de Zafra CL, Fajardo F, Zhong W, Bernett MJ, Muchhal US, Moore GL, et al. Targeting Multiple Myeloma With AMG 424, a Novel Anti-CD38/CD3 Bispecific T-Cell-Recruiting Antibody Optimized for Cytotoxicity and Cytokine Release. Clin Cancer Res (2019) 25(13):3921–33. doi: 10.1158/1078-0432.Ccr-18-2752
99. Reusch U, Burkhardt C, Fucek I, Le Gall F, Le Gall M, Hoffmann K, et al. A Novel Tetravalent Bispecific TandAb (CD30/CD16A) Efficiently Recruits NK Cells for the Lysis of CD30+ Tumor Cells. MAbs (2014) 6(3):728–39. doi: 10.4161/mabs.28591
100. Rothe A, Sasse S, Topp MS, Eichenauer DA, Hummel H, Reiners KS, et al. A Phase 1 Study of the Bispecific anti-CD30/CD16A Antibody Construct AFM13 in Patients With Relapsed or Refractory Hodgkin Lymphoma. Blood (2015) 125(26):4024–31. doi: 10.1182/blood-2014-12-614636
101. Bartlett NL, Herrera AF, Domingo-Domenech E, Mehta A, Forero-Torres A, Garcia-Sanz R, et al. A Phase 1b Study of AFM13 in Combination With Pembrolizumab in Patients With Relapsed or Refractory Hodgkin Lymphoma. Blood (2020) 136(21):2401–9. doi: 10.1182/blood.2019004701
102. Fischer N, Elson G, Magistrelli G, Dheilly E, Fouque N, Laurendon A, et al. Exploiting Light Chains for the Scalable Generation and Platform Purification of Native Human Bispecific IgG. Nat Commun (2015) 6:6113. doi: 10.1038/ncomms7113
103. Hatterer E, Barba L, Noraz N, Daubeuf B, Aubry-Lachainaye JP, von der Weid B, et al. Co-Engaging CD47 and CD19 With a Bispecific Antibody Abrogates B-cell Receptor/CD19 Association Leading to Impaired B-cell Proliferation. MAbs (2019) 11(2):322–34. doi: 10.1080/19420862.2018.1558698
104. Pardoll DM. The Blockade of Immune Checkpoints in Cancer Immunotherapy. Nat Rev Cancer (2012) 12(4):252–64. doi: 10.1038/nrc3239
105. Woo SR, Turnis ME, Goldberg MV, Bankoti J, Selby M, Nirschl CJ, et al. Immune Inhibitory Molecules LAG-3 and PD-1 Synergistically Regulate T-cell Function to Promote Tumoral Immune Escape. Cancer Res (2012) 72(4):917–27. doi: 10.1158/0008-5472.Can-11-1620
107. Furst DE, Emery P. Rheumatoid Arthritis Pathophysiology: Update on Emerging Cytokine and Cytokine-Associated Cell Targets. Rheumatology (Oxford) (2014) 53(9):1560–9. doi: 10.1093/rheumatology/ket414
108. Sands BE, Kozarek R, Spainhour J, Barish CF, Becker S, Goldberg L, et al. Safety and Tolerability of Concurrent Natalizumab Treatment for Patients With Crohn’s Disease Not in Remission While Receiving Infliximab. Inflamm Bowel Dis (2007) 13(1):2–11. doi: 10.1002/ibd.20014
109. Mansikka H, Ruzek M, Hugunin M, Ivanov A, Brito A, Clabbers A, et al. FRI0164 Safety, Tolerability, And Functional Activity of ABT-122, A Dual TNF-and IL-17a–Targeted DVD-IG™, Following Single-Dose Administration in Healthy Subjects. Ann Rheum Dis (2015) 74(suppl2):482.2–3. doi: 10.1136/annrheumdis-2015-eular.1541
110. Hsieh C-M, Cuff C, Tarcsa E, Hugunin M. FRI0303 Discovery and Characterization of ABT-122, an Anti-TNF/IL-17 DVD-Ig™ Molecule as a Potential Therapeutic Candidate for Rheumatoid Arthritis. Ann Rheum Dis (2014) 73(Suppl 2):495–. doi: 10.1136/annrheumdis-2014-eular.4676
111. Genovese MC, Weinblatt ME, Aelion JA, Mansikka HT, Peloso PM, Chen K, et al. ABT-122, a Bispecific Dual Variable Domain Immunoglobulin Targeting Tumor Necrosis Factor and Interleukin-17A, in Patients With Rheumatoid Arthritis With an Inadequate Response to Methotrexate: A Randomized, Double-Blind Study. Arthritis Rheumatol (2018) 70(11):1710–20. doi: 10.1002/art.40580
112. Mudter J, Neurath MF. Il-6 Signaling in Inflammatory Bowel Disease: Pathophysiological Role and Clinical Relevance. Inflamm Bowel Dis (2007) 13(8):1016–23. doi: 10.1002/ibd.20148
113. Peyrin-Biroulet L, Demarest S, Nirula A. Bispecific Antibodies: The Next Generation of Targeted Inflammatory Bowel Disease Therapies. Autoimmun Rev (2019) 18(2):123–8. doi: 10.1016/j.autrev.2018.07.014
114. Huang S, van Duijnhoven SMJ, Sijts AJAM, van Elsas A. Bispecific Antibodies Targeting Dual Tumor-Associated Antigens in Cancer Therapy. J Cancer Res Clin Oncol (2020) 146(12):3111–22. doi: 10.1007/s00432-020-03404-6
115. Garrett TPJ, McKern NM, Lou M, Elleman TC, Adams TE, Lovrecz GO, et al. The Crystal Structure of a Truncated ErbB2 Ectodomain Reveals an Active Conformation, Poised to Interact With Other ErbB Receptors. Mol Cell (2003) 11(2):495–505. doi: 10.1016/S1097-2765(03)00048-0
116. Graus-Porta D, Beerli RR, Daly JM, Hynes NE. ErbB-2, the Preferred Heterodimerization Partner of All ErbB Receptors, is a Mediator of Lateral Signaling. EMBO J (1997) 16(7):1647–55. doi: 10.1093/emboj/16.7.1647
117. Klapper LN, Glathe S, Vaisman N, Hynes NE, Andrews GC, Sela M, et al. The ErbB-2/HER2 Oncoprotein of Human Carcinomas may Function Solely as a Shared Coreceptor for Multiple Stroma-Derived Growth Factors. Proc Natl Acad Sci U S A (1999) 96(9):4995–5000. doi: 10.1073/pnas.96.9.4995
118. Seshacharyulu P, Ponnusamy MP, Haridas D, Jain M, Ganti AK, Batra SK. Targeting the EGFR Signaling Pathway in Cancer Therapy. Expert Opin Ther Targets (2012) 16(1):15–31. doi: 10.1517/14728222.2011.648617
119. Yarden Y, Pines G. The ERBB Network: At Last, Cancer Therapy Meets Systems Biology. Nat Rev Cancer (2012) 12(8):553–63. doi: 10.1038/nrc3309
120. Yarden Y, Shilo B-Z. Snapshot: EGFR Signaling Pathway. Cell (2007) 131(5):1018. doi: 10.1016/j.cell.2007.11.013
121. Albanell J, Baselga J. Trastuzumab, a Humanized anti-HER2 Monoclonal Antibody, for the Treatment of Breast Cancer. Drugs Today (Barcelona Spain 1998) (1999) 35(12):931–46.
122. Adams CW, Allison DE, Flagella K, Presta L, Clarke J, Dybdal N, et al. Humanization of a Recombinant Monoclonal Antibody to Produce a Therapeutic HER Dimerization Inhibitor, Pertuzumab. Cancer Immunol Immunother (2006) 55(6):717–27. doi: 10.1007/s00262-005-0058-x
123. Bartsch R, Bergen E. ASCO 2018: Highlights in HER2-positive Metastatic Breast Cancer. Memo (2018) 11(4):280–3. doi: 10.1007/s12254-018-0441-x
124. Caruso C. ZW25 Effective in HER2-Positive Cancers. Cancer Discov (2019) 9(1):8. doi: 10.1158/2159-8290.CD-NB2018-162
125. Byrne H, Conroy PJ, Whisstock JC, O’Kennedy RJ. A Tale of Two Specificities: Bispecific Antibodies for Therapeutic and Diagnostic Applications. Trends Biotechnol (2013) 31(11):621–32. doi: 10.1016/j.tibtech.2013.08.007
126. Sarkar S, Tang XL, Das D, Spencer JS, Lowary TL, Suresh MR. A Bispecific Antibody Based Assay Shows Potential for Detecting Tuberculosis in Resource Constrained Laboratory Settings. PloS One (2012) 7(2):e32340. doi: 10.1371/journal.pone.0032340
127. Chen YP, Qiao YY, Zhao XH, Chen HS, Wang Y, Wang Z. Rapid Detection of Hepatitis B Virus Surface Antigen by an Agglutination Assay Mediated by a Bispecific Diabody Against Both Human Erythrocytes and Hepatitis B Virus Surface Antigen. Clin Vaccine Immunol (2007) 14(6):720–5. doi: 10.1128/cvi.00310-06
128. Sedykh SE, Prinz VV, Buneva VN, Nevinsky GA. Bispecific Antibodies: Design, Therapy, Perspectives. Drug Des Devel Ther (2018) 12:195–208. doi: 10.2147/dddt.S151282
129. Tang XL, Peppler MS, Irvin RT, Suresh MR. Use of Bispecific Antibodies in Molecular Velcro Assays Whose Specificity Approaches the Theoretical Limit of Immunodetection for Bordetella Pertussis. Clin Diagn Lab Immunol (2004) 11(4):752–7. doi: 10.1128/cdli.11.4.752-757.2004
130. Wagstaffe SJ, Hill KE, Williams DW, Randle BJ, Thomas DW, Stephens P, et al. Bispecific Antibody-Mediated Detection of the Staphylococcus Aureus Thermonuclease. Anal Chem (2012) 84(14):5876–84. doi: 10.1021/ac203403d
131. Kammila S, Das D, Bhatnagar PK, Sunwoo HH, Zayas-Zamora G, King M, et al. A Rapid Point of Care Immunoswab Assay for SARS-CoV Detection. J Virol Methods (2008) 152(1-2):77–84. doi: 10.1016/j.jviromet.2008.05.023
132. Rossi EA, Goldenberg DM, Cardillo TM, McBride WJ, Sharkey RM, Chang C-H. Stably Tethered Multifunctional Structures of Defined Composition Made by the Dock and Lock Method for Use in Cancer Targeting. Proc Natl Acad Sci U S A (2006) 103(18):6841–6. doi: 10.1073/pnas.0600982103
133. McBride WJ, Zanzonico P, Sharkey RM, Norén C, Karacay H, Rossi EA, et al. Bispecific Antibody Pretargeting PET (immunoPET) With an 124I-Labeled Hapten-Peptide. J Nucl Med (2006) 47(10):1678–88.
134. Mainenti PP, Mancini M, Mainolfi C, Camera L, Maurea S, Manchia A, et al. Detection of Colo-Rectal Liver Metastases: Prospective Comparison of Contrast Enhanced US, Multidetector CT, PET/CT, and 1.5 Tesla MR With Extracellular and Reticulo-Endothelial Cell Specific Contrast Agents. Abdom Imaging (2010) 35(5):511–21. doi: 10.1007/s00261-009-9555-2
135. Kraeber-Bodéré F, Rousseau C, Bodet-Milin C, Frampas E, Faivre-Chauvet A, Rauscher A, et al. A Pretargeting System for Tumor PET Imaging and Radioimmunotherapy. Front Pharmacol (2015) 6:54. doi: 10.3389/fphar.2015.00054
136. Foubert F, Gouard S, Saï-Maurel C, Chérel M, Faivre-Chauvet A, Goldenberg DM, et al. Sensitivity of Pretargeted immunoPET Using (68)Ga-Peptide to Detect Colonic Carcinoma Liver Metastases in a Murine Xenograft Model: Comparison With (18)FDG PET-CT. Oncotarget (2018) 9(44):27502–13. doi: 10.18632/oncotarget.25514
137. Touchefeu Y, Bailly C, Frampas E, Eugène T, Rousseau C, Bourgeois M, et al. Promising Clinical Performance of Pretargeted immuno-PET With anti-CEA Bispecific Antibody and Gallium-68-Labelled IMP-288 Peptide for Imaging Colorectal Cancer Metastases: A Pilot Study. Eur J Nucl Med Mol Imaging (2020) 48(3):874–82. doi: 10.1007/s00259-020-04989-3
138. Gold DV, Goldenberg DM, Karacay H, Rossi EA, Chang CH, Cardillo TM, et al. A Novel Bispecific, Trivalent Antibody Construct for Targeting Pancreatic Carcinoma. Cancer Res (2008) 68(12):4819–26. doi: 10.1158/0008-5472.Can-08-0232
139. Cardillo TM, Karacay H, Goldenberg DM, Yeldell D, Chang CH, Modrak DE, et al. Improved Targeting of Pancreatic Cancer: Experimental Studies of a New Bispecific Antibody, Pretargeting Enhancement System for Immunoscintigraphy. Clin Cancer Res (2004) 10(10):3552–61. doi: 10.1158/1078-0432.Ccr-03-0340
140. Labrijn AF, Janmaat ML, Reichert JM, Parren P. Bispecific Antibodies: A Mechanistic Review of the Pipeline. Nat Rev Drug Discov (2019) 18(8):585–608. doi: 10.1038/s41573-019-0028-1
141. Dennis MS, Zhang M, Meng YG, Kadkhodayan M, Kirchhofer D, Combs D, et al. Albumin Binding as a General Strategy for Improving the Pharmacokinetics of Proteins. J Biol Chem (2002) 277(38):35035–43. doi: 10.1074/jbc.M205854200
142. Bluemel C, Hausmann S, Fluhr P, Sriskandarajah M, Stallcup WB, Baeuerle PA, et al. Epitope Distance to the Target Cell Membrane and Antigen Size Determine the Potency of T Cell-Mediated Lysis by BiTE Antibodies Specific for a Large Melanoma Surface Antigen. Cancer Immunol Immunother (2010) 59(8):1197–209. doi: 10.1007/s00262-010-0844-y
143. Leong SR, Sukumaran S, Hristopoulos M, Totpal K, Stainton S, Lu E, et al. An anti-CD3/anti-CLL-1 Bispecific Antibody for the Treatment of Acute Myeloid Leukemia. Blood (2017) 129(5):609–18. doi: 10.1182/blood-2016-08-735365
144. Betts A, van der Graaf PH. Mechanistic Quantitative Pharmacology Strategies for the Early Clinical Development of Bispecific Antibodies in Oncology. Clin Pharmacol Ther (2020) 108(3):528–41. doi: 10.1002/cpt.1961
145. Bacac M, Fauti T, Sam J, Colombetti S, Weinzierl T, Ouaret D, et al. A Novel Carcinoembryonic Antigen T-Cell Bispecific Antibody (CEA TCB) for the Treatment of Solid Tumors. Clin Cancer Res (2016) 22(13):3286–97. doi: 10.1158/1078-0432.Ccr-15-1696
146. Wu X, Sereno AJ, Huang F, Lewis SM, Lieu RL, Weldon C, et al. Fab-Based Bispecific Antibody Formats With Robust Biophysical Properties and Biological Activity. MAbs (2015) 7(3):470–82. doi: 10.1080/19420862.2015.1022694
147. Luo Y, Ye S, Li X, Lu W. Emerging Structure–Function Paradigm of Endocrine FGFs in Metabolic Diseases. Trends Pharmacol Sci (2019) 40(2):142–53. doi: 10.1016/j.tips.2018.12.002
148. Baruch A, Wong C, Chinn LW, Vaze A, Sonoda J, Gelzleichter T, et al. Antibody-Mediated Activation of the FGFR1/Klothoβ Complex Corrects Metabolic Dysfunction and Alters Food Preference in Obese Humans. Proc Natl Acad Sci (2020) 117(46):28992–9000. doi: 10.1073/pnas.2012073117
149. Kliewer SA, Mangelsdorf DJ. A Dozen Years of Discovery: Insights Into the Physiology and Pharmacology of FGF21. Cell Metab (2019) 29(2):246–53. doi: 10.1016/j.cmet.2019.01.004
150. Suh JM, Jonker JW, Ahmadian M, Goetz R, Lackey D, Osborn O, et al. Endocrinization of FGF1 Produces a Neomorphic and Potent Insulin Sensitizer. Nature (2014) 513(7518):436–9. doi: 10.1038/nature13540
151. Fon Tacer K, Bookout AL, Ding X, Kurosu H, John GB, Wang L, et al. Research Resource: Comprehensive Expression Atlas of the Fibroblast Growth Factor System in Adult Mouse. Mol Endocrinol (2010) 24(10):2050–64. doi: 10.1210/me.2010-0142
152. Kolumam G, Chen MZ, Tong R, Zavala-Solorio J, Kates L, van Bruggen N, et al. Sustained Brown Fat Stimulation and Insulin Sensitization by a Humanized Bispecific Antibody Agonist for Fibroblast Growth Factor Receptor 1/βklotho Complex. EBioMedicine (2015) 2(7):730–43. doi: 10.1016/j.ebiom.2015.05.028
153. Steeland S, Vandenbroucke RE, Libert C. Nanobodies as Therapeutics: Big Opportunities for Small Antibodies. Drug Discov Today (2016) 21(7):1076–113. doi: 10.1016/j.drudis.2016.04.003
154. Gadkar K, Yadav DB, Zuchero JY, Couch JA, Kanodia J, Kenrick MK, et al. Mathematical PKPD and Safety Model of Bispecific TfR/BACE1 Antibodies for the Optimization of Antibody Uptake in Brain. Eur J Pharm Biopharm (2016) 101:53–61. doi: 10.1016/j.ejpb.2016.01.009
155. Couch JA, Yu YJ, Zhang Y, Tarrant JM, Fuji RN, Meilandt WJ, et al. Addressing Safety Liabilities of TfR Bispecific Antibodies That Cross the Blood-Brain Barrier. Sci Transl Med (2013) 5(183):183ra57, 1–12. doi: 10.1126/scitranslmed.3005338
156. Yu YJ, Atwal JK, Zhang Y, Tong RK, Wildsmith KR, Tan C, et al. Therapeutic Bispecific Antibodies Cross the Blood-Brain Barrier in Nonhuman Primates. Sci Transl Med (2014) 6(261):261ra154. doi: 10.1126/scitranslmed.3009835
157. Regina A, Demeule M, Tripathy S, Lord-Dufour S, Currie JC, Iddir M, et al. ANG4043, a Novel Brain-Penetrant peptide-mAb Conjugate, is Efficacious Against HER2-positive Intracranial Tumors in Mice. Mol Cancer Ther (2015) 14(1):129–40. doi: 10.1158/1535-7163.Mct-14-0399
158. Gellatly SL, Hancock REW. Pseudomonas Aeruginosa: New Insights Into Pathogenesis and Host Defenses. Pathog Dis (2013) 67(3):159–73. doi: 10.1111/2049-632x.12033
159. Le HN, Quetz JS, Tran VG, Le VTM, Aguiar-Alves F, Pinheiro MG, et al. MEDI3902 Correlates of Protection Against Severe Pseudomonas Aeruginosa Pneumonia in a Rabbit Acute Pneumonia Model. Antimicrob Agents Chemother (2018) 62(5):e02565-17. doi: 10.1128/aac.02565-17
160. Le HN, Tran VG, Vu TTT, Gras E, Le VTM, Pinheiro MG, et al. Treatment Efficacy of MEDI3902 in Pseudomonas Aeruginosa Bloodstream Infection and Acute Pneumonia Rabbit Models. Antimicrob Agents Chemother (2019) 63(8):e00710-19. doi: 10.1128/aac.00710-19
161. Ali SO, Yu XQ, Robbie GJ, Wu Y, Shoemaker K, Yu L, et al. Phase 1 Study of MEDI3902, an Investigational anti-Pseudomonas Aeruginosa PcrV and Psl Bispecific Human Monoclonal Antibody, in Healthy Adults. Clin Microbiol Infect (2019) 25(5):629.e1–.e6. doi: 10.1016/j.cmi.2018.08.004
162. Klein R, Klein BE, Knudtson MD, Cotch MF, Wong TY, Liu K, et al. Subclinical Atherosclerotic Cardiovascular Disease and Early Age-Related Macular Degeneration in a Multiracial Cohort: The Multiethnic Study of Atherosclerosis. Arch Ophthalmol (2007) 125(4):534–43. doi: 10.1001/archopht.125.4.534
163. Gahn GM, Khanani AM. New Therapies of Neovascular AMD Beyond Anti-VEGF Injections. Vision (Basel) (2018) 2(1):15. doi: 10.3390/vision2010015
164. Regula JT, Lundh von Leithner P, Foxton R, Barathi VA, Cheung CM, Bo Tun SB, et al. Targeting Key Angiogenic Pathways With a Bispecific CrossMAb Optimized for Neovascular Eye Diseases. EMBO Mol Med (2016) 8(11):1265–88. doi: 10.15252/emmm.201505889
165. Chakravarthy U, Bailey C, Brown D, Campochiaro P, Chittum M, Csaky K, et al. Phase I Trial of Anti-Vascular Endothelial Growth Factor/Anti-Angiopoietin 2 Bispecific Antibody RG7716 for Neovascular Age-Related Macular Degeneration. Ophthalmol Retina (2017) 1(6):474–85. doi: 10.1016/j.oret.2017.03.003
166. Benjamin JE, Stein AS. The Role of Blinatumomab in Patients With Relapsed/Refractory Acute Lymphoblastic Leukemia. Ther Adv Hematol (2016) 7(3):142–56. doi: 10.1177/2040620716640422
167. Kitazawa T, Igawa T, Sampei Z, Muto A, Kojima T, Soeda T, et al. A Bispecific Antibody to Factors IXa and X Restores Factor VIII Hemostatic Activity in a Hemophilia A Model. Nat Med (2012) 18(10):1570–4. doi: 10.1038/nm.2942
168. Przepiorka D, Ko CW, Deisseroth A, Yancey CL, Candau-Chacon R, Chiu HJ, et al. FDA Approval: Blinatumomab. Clin Cancer Res (2015) 21(18):4035–9. doi: 10.1158/1078-0432.Ccr-15-0612
169. Scott LJ, Kim ES. Emicizumab-Kxwh: First Global Approval. Drugs (2018) 78(2):269–74. doi: 10.1007/s40265-018-0861-2
170. Seimetz D. Novel Monoclonal Antibodies for Cancer Treatment: The Trifunctional Antibody Catumaxomab (Removab). J Cancer (2011) 2:309–16. doi: 10.7150/jca.2.309
171. Takai T. Roles of Fc Receptors in Autoimmunity. Nat Rev Immunol (2002) 2(8):580–92. doi: 10.1038/nri856
172. Nimmerjahn F, Ravetch JV. Fcgamma Receptors as Regulators of Immune Responses. Nat Rev Immunol (2008) 8(1):34–47. doi: 10.1038/nri2206
173. Frampton JE. Catumaxomab: in Malignant Ascites. Drugs (2012) 72(10):1399–410. doi: 10.2165/11209040-000000000-00000
174. Linke R, Klein A, Seimetz D. Catumaxomab: Clinical Development and Future Directions. MAbs (2010) 2(2):129–36. doi: 10.4161/mabs.2.2.11221
175. Heiss MM, Murawa P, Koralewski P, Kutarska E, Kolesnik OO, Ivanchenko VV, et al. The Trifunctional Antibody Catumaxomab for the Treatment of Malignant Ascites Due to Epithelial Cancer: Results of a Prospective Randomized Phase II/III Trial. Int J Cancer (2010) 127(9):2209–21. doi: 10.1002/ijc.25423
176. Sebastian M, Kiewe P, Schuette W, Brust D, Peschel C, Schneller F, et al. Treatment of Malignant Pleural Effusion With the Trifunctional Antibody Catumaxomab (Removab) (anti-EpCAM X Anti-CD3): Results of a Phase 1/2 Study. J Immunother (2009) 32(2):195–202. doi: 10.1097/CJI.0b013e318195b5bb
177. Berek JS, Edwards RP, Parker LP, DeMars LR, Herzog TJ, Lentz SS, et al. Catumaxomab for the Treatment of Malignant Ascites in Patients With Chemotherapy-Refractory Ovarian Cancer: A Phase II Study. Int J Gynecol Cancer (2014) 24(9):1583–9. doi: 10.1097/igc.0000000000000286
178. May MB, Glode A. Blinatumomab: A Novel, Bispecific, T-cell Engaging Antibody. Am J Health Syst Pharm (2016) 73(1):e6–e13. doi: 10.2146/ajhp150134
179. Wilke AC, Gökbuget N. Clinical Applications and Safety Evaluation of the New CD19 Specific T-cell Engager Antibody Construct Blinatumomab. Expert Opin Drug Saf (2017) 16(10):1191–202. doi: 10.1080/14740338.2017.1338270
180. Combariza JF, Arango M, Díaz L, Agudelo C, Hernandez S, Madera AM, et al. Measurable Residual Disease Assessment and Allogeneic Transplantation as Consolidation Therapy in Adult Acute Lymphoblastic Leukemia in Colombia. Clin Lymphoma Myeloma Leuk (2020) 21(4):e365–72. doi: 10.1016/j.clml.2020.11.010
181. Potter MN, Steward CG, Oakhill A. The Significance of Detection of Minimal Residual Disease in Childhood Acute Lymphoblastic Leukaemia. Br J Haematol (1993) 83(3):412–8. doi: 10.1111/j.1365-2141.1993.tb04665.x
182. Gökbuget N, Dombret H, Bonifacio M, Reichle A, Graux C, Faul C, et al. Blinatumomab for Minimal Residual Disease in Adults With B-cell Precursor Acute Lymphoblastic Leukemia. Blood (2018) 131(14):1522–31. doi: 10.1182/blood-2017-08-798322
183. Pulte ED, Vallejo J, Przepiorka D, Nie L, Farrell AT, Goldberg KB, et al. FDA Supplemental Approval: Blinatumomab for Treatment of Relapsed and Refractory Precursor B-Cell Acute Lymphoblastic Leukemia. Oncologist (2018) 23(11):1366–71. doi: 10.1634/theoncologist.2018-0179
184. Sigmund AM, Sahasrabudhe KD, Bhatnagar B. Evaluating Blinatumomab for the Treatment of Relapsed/Refractory All: Design, Development, and Place in Therapy. Blood Lymphat Cancer (2020) 10:7–20. doi: 10.2147/blctt.S223894
185. Queudeville M, Schlegel P, Heinz AT, Lenz T, Döring M, Holzer U, et al. Blinatumomab in Pediatric Patients With Relapsed/Refractory B-cell Precursor Acute Lymphoblastic Leukemia. Eur J Haematol (2020) 106(4):473–83. doi: 10.1111/ejh.13569
186. Topp MS, Gökbuget N, Zugmaier G, Klappers P, Stelljes M, Neumann S, et al. Phase II Trial of the Anti-CD19 Bispecific T Cell-Engager Blinatumomab Shows Hematologic and Molecular Remissions in Patients With Relapsed or Refractory B-precursor Acute Lymphoblastic Leukemia. J Clin Oncol (2014) 32(36):4134–40. doi: 10.1200/JCO.2014.56.3247
187. Topp MS, Gökbuget N, Stein AS, Zugmaier G, O’Brien S, Bargou RC, et al. Safety and Activity of Blinatumomab for Adult Patients With Relapsed or Refractory B-precursor Acute Lymphoblastic Leukaemia: A Multicentre, Single-Arm, Phase 2 Study. Lancet Oncol (2015) 16(1):57–66. doi: 10.1016/S1470-2045(14)71170-2
188. von Stackelberg A, Locatelli F, Zugmaier G, Handgretinger R, Trippett TM, Rizzari C, et al. Phase I/Phase Ii Study of Blinatumomab in Pediatric Patients With Relapsed/Refractory Acute Lymphoblastic Leukemia. J Clin Oncol (2016) 34(36):4381–9. doi: 10.1200/JCO.2016.67.3301
189. Schmitt C, Adamkewicz JI, Xu J, Petry C, Catalani O, Young G, et al. Pharmacokinetics and Pharmacodynamics of Emicizumab in Persons With Hemophilia A With Factor VIII Inhibitors: HAVEN 1 Study. Thromb Haemost (2020) 121(3):351–60. doi: 10.1055/s-0040-1717114
190. Knöbl P. Prevention and Management of Bleeding Episodes in Patients With Acquired Hemophilia a. Drugs (2018) 78(18):1861–72. doi: 10.1007/s40265-018-1027-y
191. Kessler CM, Knöbl P. Acquired Haemophilia: An Overview for Clinical Practice. Eur J Haematol (2015) 95 Suppl 81:36–44. doi: 10.1111/ejh.12689
192. Knoebl P, Thaler J, Jilma P, Quehenberger P, Gleixner KV, Sperr WR. Emicizumab for the Treatment of Acquired Hemophilia a. Blood (2020) 137(3):410–9. doi: 10.1182/blood.2020006315
193. Oldenburg J, Mahlangu JN, Kim B, Schmitt C, Callaghan MU, Young G, et al. Emicizumab Prophylaxis in Hemophilia A With Inhibitors. N Engl J Med (2017) 377(9):809–18. doi: 10.1056/NEJMoa1703068
Keywords: BsAbs, development, platform, mechanism, application
Citation: Ma J, Mo Y, Tang M, Shen J, Qi Y, Zhao W, Huang Y, Xu Y and Qian C (2021) Bispecific Antibodies: From Research to Clinical Application. Front. Immunol. 12:626616. doi: 10.3389/fimmu.2021.626616
Received: 06 November 2020; Accepted: 16 April 2021;
Published: 05 May 2021.
Edited by:
Catherine Sautes-Fridman, INSERM U1138 Centre de Recherche des Cordeliers (CRC), FranceReviewed by:
Martina Spiljar, University of Geneva, SwitzerlandFabio Malavasi, University of Turin, Italy
Copyright © 2021 Ma, Mo, Tang, Shen, Qi, Zhao, Huang, Xu and Qian. This is an open-access article distributed under the terms of the Creative Commons Attribution License (CC BY). The use, distribution or reproduction in other forums is permitted, provided the original author(s) and the copyright owner(s) are credited and that the original publication in this journal is cited, in accordance with accepted academic practice. No use, distribution or reproduction is permitted which does not comply with these terms.
*Correspondence: Cheng Qian, Y3FpYW44NjM0QGdtYWlsLmNvbQ==; Yanmin Xu, QWxpY2VfeHVAcHJlY2lzaW9uLWJpb3RlY2guY29t