- 1Department of Pathophysiology and Immunology, National Institute of Geriatrics, Rheumatology, and Rehabilitation, Warsaw, Poland
- 2Department of Pharmacy, Kepler University Hospital, Linz, Austria
The origin and the global spread of severe acute respiratory syndrome coronavirus 2 (SARS-CoV-2) causing coronavirus disease 2019 (COVID-19) in early 2020 was accompanied by high rates of mortality in regions belonging to the ancient silk road, such as the south of China, Iran, Turkey and the northern parts of Italy. However, children seem to be spared in the epidemic as very small percentage worldwide being ill. The protection of children and neonates suggests the involvement of a specific component of adaptive immunity present at early development. Native immunoglobulin belonging to the class of IgM is abundantly present in neonates and children and is known for its recognition of self- and altered self-antigens. Native IgM may be able to neutralize virus by the recognition of endogenous “danger signal” encoded in the viral envelope and originally imprinted in the membranes of infected and stressed cells. Noteworthy, thrombosis and vasculitis, two symptoms in severely affected adult and pediatric patients are shared between COVID-19 and patients with Behcet’s disease, an autoimmune disorder exhibiting a region-specific prevalence in countries of the former silk road. Molecular mechanisms and clinical indicators suggest reactive oxygen species as trigger factor for severe progression of COVID-19 and establish a link to the innate immune defense against bacteria. The selective pressure exerted by bacterial pathogens may have shaped the genetics of inhabitants at this ancient trade route in favor of bacterial defense, to the detriment of severe COVID-19 progression in the 21th century.
Introduction
On March 11, 2020, the World Health Organization (WHO) has declared COVID-19 as a pandemic a disease resulting from the infection by SARS-CoV-2, a novel member of the coronoviridae family (1). In severe cases, the disease manifests by interstitial pneumonia and alveolar damage within approximately 7 days of symptom onset, which can lead to acute respiratory distress syndrome (ARDS) (2, 3). ARDS is associated with an uncontrolled immune activation, characterized by a massive upregulation of pro-inflammatory cytokines, chemokines, and hematopoietic growth factors (IFN-γ, IL-1β, IL-6, IL-7, IL-8, IL-2, TNF, CXCL10, CCL2, GM-CSF) called cytokine storm (3–5), culminating in hyperinflammation and multi-organ disease (6), which is the leading cause of mortality (7). Septic shock and multiorgan failure were the most common immediate cause of death, often due to suppurative pulmonary infection as shown by Elezkurtaj et al. (8).
As of December 23, 2020, SARS-CoV-2 has been the cause of infection in more than 78 million people resulting in more than 1.7 million deaths worldwide; however, comparatively, few infections have been described in children. In contrast to infected adults, most children with confirmed SARS-CoV-2 infection seems to have a mild clinical course, and almost 16% of them did not show any symptoms of infection (9). By March 25, 2020, Italy had the second highest number of COVID-19 infections and the highest number of deaths worldwide, but only 1% of the total number of patients were children younger than 18 years of age, although children comprise 10% of the total population. Only 11% of those affected children required hospitalization, and none of them died (10).
Two distinct mechanistic checkpoints seem to determine the disease outcome following infection with SARS-CoV-2. First, the differential in host susceptibility to viral infection leading to clinical symptoms, and second, the severe deterioration of disease associated with cytokine storm and mortality. Thereby, susceptibility and mortality were found to be a matter of age and geographic location, respectively.
The immunity against COVID-19 in children and the differences in mortality between geographic regions during the initial spread of SARS-CoV-2 in the spring of 2020, prompted us to address those questions from several perspectives, encompassing immunology, virology, cell biology, historical, and linguistic sciences. The synopsis across different viewpoints culminated in two hypotheses, as proposed in this review.
First, we summarize the current knowledge on COVID-19 in children. The following two sections deal with less recognized facts on the cell biology of SARS-CoV infection and the difficulty to explain protection by an IgG-mediated mechanism directed against spike protein. Finally, we conclude our hypothesis based on a danger model of adaptive immunity. In the next two chapters, we elucidate the current knowledge on B1 B cells and natural IgM in viral defense and how these may apply to the SARS-CoV-2 pandemic.
Our two last sections are devoted to understanding the ethnic and global differences in COVID-19–related mortality. In section entitled Mortality: Why Some Patients End Up on Ventilators and Cytokine Storm in COVID-19?, we discuss the role of receptors, signaling proteins, and enzymes involved in the generation of reactive oxygen species and their association with the cytokine storm. In last section (Genetics of ROS Generation and Human Sociographic and Linguistic Evolution), we explain our hypothesis on polymorphisms associated with bacterial defense and simultaneously determine the outcome of COVID-19 disease.
Susceptibility—Covid-19 In Children And Specifics In Pediatric Immunity
Children with SARS-Cov-2 infection present milder symptoms and show less laboratory and radiologic abnormalities compared to adults (11). The same observation was reported during the SARS and MERS-CoV epidemics in 2003 and 2012, respectively (12, 13). Depending on the study, up to 35% of pediatric patients with COVID-19 are asymptomatic. It remains uncertain whether asymptomatic children transmit the virus; however, it was shown that even asymptomatic children can have high viral loads of SARS-CoV-2 (14).
Although most of the children infected with SARS-Cov-2 present a less severe form of COVID-19, in April 2020, the UK reported a growing number of cases with features similar to atypical Kawasaki disease (15). Among the first eight hospitalized children reported, six were of Afro-Caribbean, one of Asian and Middle-Eastern descent each, and five of them were boys mostly overweight, suggesting that the ethnic characteristic prone to COVID-19 infection in children resembles the group of adults with high risk for COVID-19 mortality (16). Interestingly, no pathological organism was identified in seven from eight children during hospital treatment (15), indicating a self-preserving inflammatory disease triggered by viral exposure and persistent even after the immunologic clearance of the virus.
Recently, a novel multisystem inflammatory syndrome in children (MIS-C) belonging to the spectrum of Kawasaki disease-like pathology have been reported in regions with receding SARS-CoV-2 epidemics (17). Inflammatory shock, gastrointestinal symptoms, and coagulopathy, which are rarely seen in classic Kawasaki disease, are prominent features of this syndrome, affecting mostly older children (mean age was 12 in that study) with Black or Hispanic ethnicity. All children harbored antibodies against SARS-CoV-2 of class IgG and IgA, with absence of circulating IgM in most cases (18). Detection of autoantibodies of IgG and IgA class against endothelial, mucosal, and immune antigens together with neutrophil and monocyte upregulation of CD54 and CD64 suggest that autoreactivity together with inflammatory innate immune response may be critical to the pathogenesis of MIS-C (18). The two syndromes connected with SARS-Cov-2 infection and described in children, Kawasaki-like disease and MIS-C, preferentially affects children which share an ethnic background shown to be one of the factors predisposing for severe outcome of COVID-19 infection in adults. The cohort study in the UK, investigating the association between patient individual factors and risk for COVID-19 hospital death, revealed as the main risk factors in adults the male gender, advanced age, obesity, and ethnicity (substantially higher risk for people of Asian and African origin) (16).
It is not known why SARS-CoV-2 infection in children overall appears to be mild; however, recent data suggest that children younger than 10 to 14 years are less susceptible to infection of SARS-CoV-2 than adults, while adolescents appeared to have similar susceptibility to adults (19). Although the frequency of asymptomatic SARS-CoV-2 infections among children is unknown, it is possible that the role of children as drivers of pathogen transmission is real (20).
Despite the fact that the proportion of COVID-19 cases that are pediatric has risen substantially from 3% of the reported cases in April to 12% in August and 15% in September in USA (while testing children remained at the same level since April 2020), hospitalization and death due to COVID-19 is still rare (21). Children made up 0.07% of total deaths, and these rates remained stable across the study period (21).
Differences in activity of immune function between children and adults may explain their protection from development of the life-threatening pneumonia. Children have physiologically elevated lymphocyte number, which persist under SARS-Cov-2 infection (22). In addition, children display a reduced production of pro-inflammatory cytokines, such as IL-6 (the main cytokine involved in cytokine storm induction) and a higher production of anti-inflammatory IL-10, as compared to adults upon immune activation (23). Because of that, children may be less prone to develop ARDS. Frequent viral infections during childhood and the scheduled active vaccinations may constantly trigger their innate and adaptive immune system into a state of activation and thus more effectively fight subsequent infections (24). Importantly, over 75% of children seroconvert in response to seasonal coronaviruses before their fourth birthday (20). Although cross-reactivity of antibodies against seasonal coronaviruses and SARS is restricted, it helps to fight SARS-CoV-2 infection in children and young people (20).
However, none of those explanations convincingly elucidate the riddle of the mild COVID-19 presentation in children. Since even infants of affected mothers are protected from the development of COVID-19, we started to believe that an adaptive immune mechanism beyond IgG, IgA, and cytotoxic CD8+ T cells is responsible. In order to elucidate this mechanism, we first need to understand the cell biology of coronavirus infection.
Cell Biology Of Sars-Cov-2 Infection
Coronaviruses (Covs) are enveloped viruses with a positive sense, single-stranded RNA genome (25). Three of them (SARS-CoV, MERS-CoV, and SARS-CoV-2) are known to induce zoonotic disease in men (26). The viral genome encodes four major structural proteins: the spike (S) protein, nucleocapsid (N) protein, membrane (M) protein, and the envelope (E) protein, all required to produce structurally complete viral particles. Within S protein, there is a receptor-binding domain (RBD), which binds to host target receptors, allowing the virus to entry into the host. Previous investigations on SARS-CoV revealed that the virus targets airway and alveolar epithelial cells, vascular endothelial cells and alveolar macrophages for the initial entry into host cells (27).
Coronaviruses are different from other well-studied enveloped viruses in that they bud into the endoplasmic reticulum Golgi intermediate compartment (ERGIC), from where they acquire their membrane envelope (25). Although, the endoplasmic reticulum (ER) is able to degrade misfolded protein in a process called ER-associated degradation (ERAD), if the capacity of ERAD is exceeded, those misfolded proteins trigger an ER stress- and unfolded-protein response (UPR). Further prolongation of UPR results in initiation of apoptosis. Viral infections can also trigger the UPR, adopted by host cells as antiviral strategy (28). Interestingly, CoV E protein exert anti-apoptotic function in infected cells by suppressing the UPR during infection in order to continue viral propagation (25).
The origin of viral envelope from host’s ER in conjunction with the ER stress-response, prompted us to compare between the classical self-nonself and an alternative model of antigen recognition, based on danger signals.
Limitations Of The Classical View On Adaptive Immunity
Glycosylation is one of the most important post-translational modification of proteins and is characteristic for eukaryotic organisms. Viruses often utilize the host glycosylation machinery during replication and assembly in the host cells (29). Glycans present an overall image of “self” to the immune surveillance of the host organism and may act as protective shell for viral peptide epitopes (30). SARS-CoV does not seem to utilize such a mechanism in order to escape from neutralizing immunoglobulins, like glycan shielding of the receptor-binding site. Instead, a recombinant spike protein fragment with RBD of SARS-CoV presented the highest immunogenicity among other recombinant spike protein fragments tested (31, 32). If SARS-CoV-2 would have similar properties like investigated SARS-CoV, it would help for the successful development of neutralizing monoclonal Abs as therapy. However, alterations in spike proteins, which are emerging as virus spreads and mutates, may render SARS-CoV-2 resistant to monoclonal Abs. Even more surprising is the report that patients who died of SARS-CoV infection achieved their peak levels of neutralizing Abs activity earlier (at 15 days post infection) as compared with those who survived (at 20 days post infection) (33). Adding to this contradiction is the observation that 80% of those patients who progress to ARDS exhibit a successful seroconversion to antiviral IgG (34). Atyeo et al. have correlated disease outcome with the level of specific viral antibodies in patient serum (35). Hereby, they reported that a humoral response specific for viral spike-protein was characteristic for convalescent individuals, while antibodies against nucleocapsid proteins were elevated in deceased individuals. Similarly, children with SARS-CoV-2–associated MIS-C are spared from severe COVID-19 turnover and produce specific IgG antibodies for spike-protein but not against the nucleocapsid-protein (36).
The Putative Role Of Natural Igm In Sars-Cov-2 Clearance
Due to the fact that young children and even neonates are protected from development of COVID-19, and in view of their naivety in terms of adaptive immunity, such as memory cells and IgG production, we are considering IgM as possible factor responsible for the protection. In particular, natural IgM may play the key role in defense against SARS-CoV-2. Natural IgM constitutes the most abundant immunoglobulin in neonates and exhibits a high potential for self-antigen recognition. The ability to recognize self- or altered self-antigens by the adaptive arm of the immune system is the basic principle of the “danger hypothesis” postulated by Polly Matzinger (37). Consequently, SARS-CoV-2 particles budding from the infected cell are wrapped by the host`s membrane previously modified in the UPR process and thus, are recognized by natural IgM as “altered self”. The same way as apoptotic debris or exosomes are recognized by natural IgM and subsequently prepared for phagocytosis, SARS-CoV-2 as viral particle or as infected cell could be recognized and effectively cleared (Figure 1).
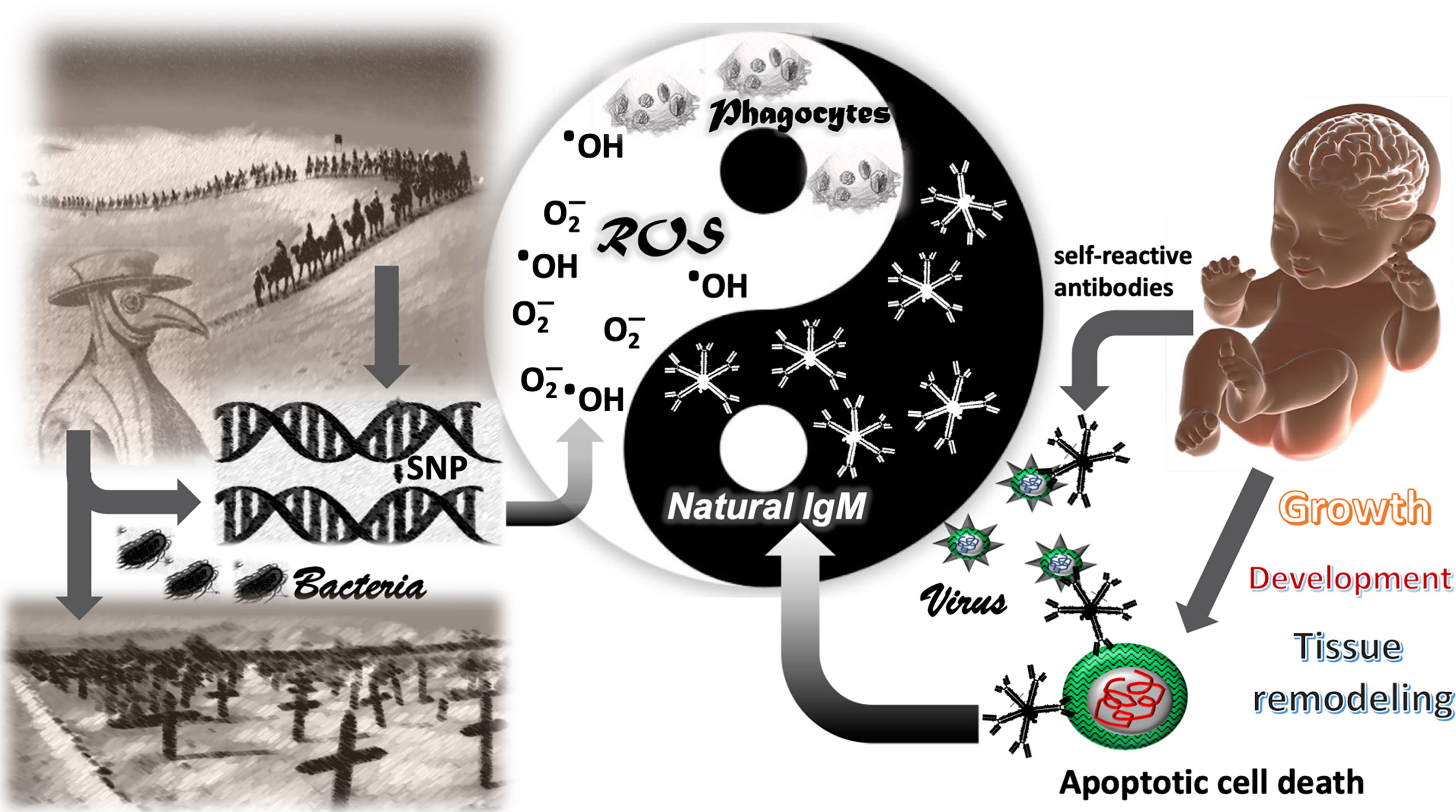
Figure 1 The Yang-Yin of immune defense. The genetic traits favoring the defense against bacterial pathogens in the past (ROS and IgM) appeared to be vulnerable for COVID-19–related mortality in the 21th century. The left side demonstrates how bacterial pathogens shaped the genome in human populations by selective survival of individuals with single nucleotide polymorphisms (SNPs) in genes facilitating bacterial defense, such as the generation of reactive oxygen species (ROS) in phagocytes. The right side shows a newborn with the inherent high polyclonality of its natural IgM. Natural IgM recognize altered self-antigens and are important for removal of apoptotic cells in the developing neonate. Altered self-antigens are also present in the viral envelope of corona viridae. Such as natural IgM removes apoptotic cells in absence of inflammation, the virus is neutralized without inflammatory symptoms and disease. Reactive oxygen species represent the inflammatory and anti-bacterial part of immune defense, associated with Yang in traditional Chinese medicine (TCM). The natural IgM represents the anti-inflammatory and self-recognizing part of immune system involved in tissue remodeling, associated with Yin in TCM.
In the next sections, we will review on natural IgM and their corresponding B1 B cells, summarize their known functions in viral defense, and discuss their putative role in protection against SARS-CoV-2 fatal infection.
B1 B Cells In Viral Defense
B1 B cells are generated earlier in ontogeny than conventional B cells, namely B2 B cells. The human analog to murine B1 cells is a CD45+CD27+CD70- B cells subset, reactive with non-protein and self-antigens, such as phosphorylcholine (PC) and dsDNA and detected both in human umbilical cord blood and in the adult peripheral immune system (38). B1 B cells are vastly generated before birth in the fetal liver, are present at high percentage (70–90% of B cells are CD5+) in early childhood, drop to a percentage below 10% of all peripheral B cells during adulthood, and further decrease in numbers with age (39).
B1 cells are long-lived and self-renewing and produce more than 90% of the natural antibodies (NAbs), thus providing an important link between innate and adaptive immune systems (40, 41). A key property of NAbs is to maintain immune homeostasis by the clearance of apoptotic debris, the suppression of autoimmune and inflammatory responses and the regulation of B cell development (41). They form the first line of defense against micro-organisms by recognition of structural conserved antigens (both self and non-self).
Since the first study in 1999, aiming to identify natural antibodies specific for viral antigens, many studies have confirmed a role of natural IgM in the protection against viral infections (42, 43). The presence of early neutralizing antibodies during infections with several cytopathic viruses (such as polio, influenza, and rabies) is essential for protection against lethal disease, which often correlates with viral replication in neural tissues (43). As B1 cells arise from fetal liver, they are functionally active in newborns and are crucial for immune protection throughout the time of adaptive immune maturation (41) as IgM production by B1 cells is independent of T cell activation (44). It seems that very early B cells are responsible for the first protection of the newborn organism and that this defense is basically composed of polyreactive IgM (45). Because IgM is not able to cross the placental barrier, the presence of respiratory syncytial virus (RSV)-IgM may reflect the existence of broadly reactive NAbs in newborns (46).
We assume that a similar mechanism in viral immune defense will apply to SARS-Cov-2 in neonates. In contrast to MERS-Cov and the previous SARS-CoV, pregnant women infected with SARS-Cov-2 do not seem to be prone to unfavorable pregnancy outcome (47). Furthermore, SARS-CoV-2 was not detected in products of conception, breast milk, or in neonatal nasopharyngeal swab samples at birth (48). Among mothers with confirmed COVID-19, the virus was not detected in the serum or throat swab of their newborns (48, 49). Interestingly, SARS-CoV-2 IgG concentrations were elevated in five of six infants while increased SARS-CoV-2 IgM levels were observed in two infants (49). Although none of the infants presented any symptoms of COVID-19, significant increase of inflammatory cytokine IL-6 together with increased Ab levels support the possibility of vertical transmission (49). In contrast to IgG Ab, which is passively transmitted from mother to the fetus through the placenta, IgM is not transferred due to its large macromolecular size. While the elevated IgG level may reflect maternal or infant infection, we believe that the increased level of IgM Abs in those neonates suggests that IgM was produced by the infant after the virus crossed the placenta similarly to RSV infection.
Thus, the presence of IgM antibodies in neonates, even in the absence of an elevation, may be sufficient to protect them against COVID-19, when SARS-Cov-2 is present in their mother. The clonal repertoire of B cells producing NAbs is likely shaped by the early life antigenic history of an individual, as neonatal exposure to bacterial antigens result in striking changes in composition of antigen specific NAbs. Thus NAbs reactivity and its potential for antiviral activity is closely related to the species of the viral origin, which is especially important in zoonotic viral infections (29).
The Natural Igm Antibody (Ab) Repertoire And Its Impact On Defense Against SARS-Cov-2 Infection
Natural antibodies are mostly of IgM isotype, followed by a much smaller fractions of IgG, IgA or IgE isotypes (41). Polyreactive NAbs recognize and bind phylogenetically conserved structures like phospholipids, oxidized lipids, glycolipids, and glycoproteins (41, 50). Current evidence suggests that the pool of B1 cells secreting IgM results from positive selection upon recognition of self-antigens during development. Several IgM and IgA NAbs, recognizing autoantigens, such as phosphatidylcholine determinants in oxidized lipid layers on apoptotic cells, were found binding to homologous molecules in the cell wall of pneumococci (38). Thus, it is likely that composition of the NAbs repertoire is modulated since early childhood by continuous interactions with the microbiome, which is the community of microbial commensals present in our bodies. The cross-reactivity of NAbs between self and microbial antigens also suggests, that vaccination may enhance Ab production and modulate their specificity. Indeed, the protective level of cross-reactive antibodies against oxidized low-density lipoprotein (anti-oxLDL Ab) appeared to be significantly higher after pneumococcal vaccination (51). Similar mechanism may support observations that Calmette-Guérin (BCG) vaccination against tuberculosis is negatively associated with prevalence and mortality of COVID-19 (52).
Several distinct properties of IgM, such as its antigen-binding polyreactivity, the high avidity assured by the ten binding sites, the ability to access cryptic antigens hidden for IgG, and its capacity to promote the removal of apoptotic cells support the specific role of IgM in health and disease (42). Those unique properties allow the recognition and removal of “altered” self-antigens, an ability which is linked to the binding of shared molecules on pathogens, thereby contributing to immune homeostasis controlling inflammation and tissue damage. Indeed, there are several known autoantigens exposed on cells during the process of apoptotic death, which are recognized by subsets of natural IgM (38). Interestingly, IgM that specifically recognize oxidation-associated determinants exposed on apoptotic cells are highly abundant in human newborns (53, 54). Having in mind that SARS-CoV-2 particles leaving the infected cell are covered by the host’s membrane previously modified in the UPR process and thus resembling modifications in apoptotic cells, it is very probable that those particles might be recognized by natural IgM as “altered self”. The same way as apoptotic debris or modified self-particles are recognized by natural IgM and subsequently prepared for phagocytosis, SARS-CoV-2 as viral particle or as infected cell could be recognized and cleared by IgM, highly represented in children’s immune system.
Both natural and induced IgM are polymeric (pentameric) and due to its large size of 970 kDa, IgM does not traverse easily between vascular and extravascular compartments, and therefore, demonstrate specific retention at sites of production or transportation by poly-Ig receptors, such as the mucosal surface, breast milk, or serum (55). That allows natural and induced IgM to act as an early defense mechanism against not only systemic but also mucosal pathogens (56). Those antibodies are conserved in humans and recognize specialized oligosaccharides integrated into the bacterial cell wall and capsule (57). Interestingly, both germ-free and normal conventional-housed mice have similar serum IgM levels, which support the concept that serum IgM concentrations are independent from foreign antigen exposure (42, 58). Additionally, female mice show significantly higher level of serum IgM than male mice (57). The presence of higher levels of oligosaccharide-specific serum IgM results in selective survival of female mice and their offspring, compared to males during bacterial infections. IgM secreting cells seem to be regulated by estrogens, and estrogen-driven antibodies are maternally transferrable to offspring and conferred protection during infancy (57). That might be a reason for the lower fatality rate of severe COVID-19 in females as well as lower incidence of more severe COVID-related diseases in girls in comparison to boys (10, 59).
Mortality: why some patients end up on ventilators and cytokine storm in covid-19?
The respiratory system is most of all organs involved in continuous environmental exchange and exposed to microbial organisms, due to its large alveolar and mucosal surface, and due to the liters of air moving with every breath. Its essential role in gas exchange and oxygen delivery made it necessary for the lung to evolve strategies to control the inflammation accompanied by antimicrobial defense. Multiple receptors expressed on epithelial and mucosal immune cells directly interact with microbial pathogens, in order to orchestrate the balance between immune defense and inflammation. One of those receptors early associated with SARS-CoV infection is ACE-2, a type I membrane protein proven to be the functional receptor of SARS-CoV and SARS-CoV-2 in the lung (60). ACE-2 is a component of the renin-angiotensin-aldosterone system (RAAS), which represents a cascade of vasoactive peptides, controlling main processes in human physiology like blood pressure, wound healing, and inflammation (61). The key role of ACE-2 is to covert angiotensin II (AngII) into angiotensin-(1-7), which was shown to have anti-oxidant and anti-inflammatory (including antithrombotic and anti-fibrotic) effects (62, 63).
Although the role of ACE-2 in SARS-CoV-2 infection is conflicting, there is increasing evidence in published literature that a high level of ACE-2 expression is rather beneficial than harmful in patients with lung injury (61). ACE-2 expression is high in infants, reaching a plateau in adolescence, and decrease during adulthood, in males faster than in females (64). It was speculated, that the decline in sex hormones contribute to the observed decrease in expression of ACE-2 with age, as estrogen and androgens activate ACE-2 expression (64). The analysis of ACE-2 expression across age, gender, and ethnicity showed that ACE-2 expression level is high in Asian females and young people, suggesting a negative correlation between ACE-2 expression and SARS-CoV-2 severe outcome (64). Compared to children, older patients present reduced the number of ACE-2–expressing cells and lung progenitor cells, which made those patients more vulnerable to develop severe pneumonia with poor recovery potential from COVID-19 (65).
Thus, children having high expression of ACE-2 seem to be protected against angiotensin II accumulation and its harmful cytotoxic effects and benefit from the anti-inflammatory properties exerted by angiotensin-(1-7).
Another receptor playing a key role in pulmonary viral defense and inflammation is retinoic acid-inducible gene (RIG)-I which recognize RNA of a variety of virus families (66). Upon viral RNA recognition, RIG-I together with adapter molecule mitochondrial antiviral-signaling (MAVS) protein triggers a cascade leading to the activation of the inflammasome, culminating in the expression of antiviral interferons (67). Most recent evidence suggests that overactivation of the inflammasome by SARS-CoV-2 is the responsible factor for the cytokine storm (68). Interestingly, inflammasome and certain adaptor molecules for RIG-I signaling are located at the mitochondrial outer membrane (67). Mitochondria serve a key role in the supply of Krebs cycle and lipid metabolites and are the main responsible organelles for maintenance of the cellular redox equilibrium. The level of inflammasome activation via RIG-I and viral RNA is dependent on ROS (69) through upregulation of the mitochondria-associated adapter MAVS (70). The activity of complex multiunit enzymes belonging to the NADPH oxidase (NOX)- and the dual oxidase (DUOX) families, both expressed in airway- and alveolar epithelial cells, is catalyzing the local generation of ROS subsequent to viral challenges (71).
In this review, we hypothesize that a massive increase in production of ROS triggered by assisted ventilation under high oxygen pressure and facilitated by the downregulation of ACE-2 and the viral load leads to a vicious cycle between RIG-I signaling, exacerbated inflammasome activation and ROS production, ending up in a cytokine storm. There is accumulating evidence that the assisted ventilation in patients with COVID-19 does not change the disease course (2, 72). In addition, iron as limiting element for the continuous activity of NOX and DUOX is reduced in its availability by the upregulation of ferritin, in order to avoid accumulation of ROS and thereof cellular toxicity. Indeed, patients presenting a severe form of COVID exhibit high levels of serum ferritin (73–75), suggesting an inflammatory process accompanied by high levels of ROS.
Genetics of ROS Generation and Human Sociographic and Linguistic Evolution
The key regulatory checkpoints in ROS production are determined by activity and localization of the multiunit enzymes NOX and DUOX. While epithelial cells of the upper respiratory tract express several isoforms of NOX and DUOX, alveolar epithelial cells, macrophages, and vascular endothelial cells express only two isoforms of NOX, namely NOX2 and NOX4 (76). Macrophages and granulocytes require NOX2 for generation of sufficient ROS in lysosomal compartments for the elimination of bacterial pathogens. The significance of NOX2 function for bacterial defense is apparent in patients with chronic granulomatous disease (CGD), where several identified mutations in any of the five subunits of NOX2 lead to high susceptibility for bacterial infection, giving rise to a low life expectancy (77). Since the first description of a genetic mutation in a NOX subunit as cause for CGD in the 1980s, single nucleotide polymorphisms (SNPs) in several NOX subunits and in enzymes involved in neutralization of ROS were identified and associated with atherosclerosis (78), type II diabetes mellitus (79), diabetic nephropathy (79), and thrombosis (80). However, besides the relevant SNPs, the genetic background of patients in those studies, as reflected by their ethnicity, seems to have a significant impact on the outcome. Interestingly, the small p22(phox) subunit, shared between NOX 1- 4 enzymes, serves a crucial role in the assembly and intracellular localization of all other enzymatic subunits and was found to be highly polymorphic with functional differences in ROS generation (81).
Consistent with the role of ACE-2 in neutralizing ROS and decreasing angiotensin II, there is evidence that a persistent high activity of angiotensin II is at least in part responsible for the organ injury observed in COVID-19 (82, 83). SARS-CoV-2 downregulates ACE-2 expression after using it for cellular entry, resulting in unopposed angiotensin II accumulation and local RAAS activation (84, 85). The level of plasma angiotensin II correlates with the degree of lung injury and total viral load in COVID-19 patients (83).
Before the invention and wide applications of antibiotics in the 1950s, bacterial infection represented the major cause of death in the human population (86). Bacteria and its ability for host adaption has shaped human evolution for thousands of years by maintaining selective pressure on immune function in young individuals before attaining their reproductive age. Polymorphisms in genes associated with functional differences in generation or neutralization of ROS, or its subcellular localization in phagocytes, may had a profound impact on survival and subsequent genetic inheritance during the pre-antibiotic times (Figure 1). In human history, populations migrated and settled in regions supporting and improving their livelihood. Ancient trade routes, such as the silk route, provided an economic belt for settlement, connecting Southeast Asia with Southern Europe and spanning a period of 1,500 years. Along this route, the movement of people with diverse ethnic and genetic background was accompanied by rodents, parasites, and pathogens. Thus, ancient trade routes, such as the silk route, may have generated and selected genetic variants for bacterial immune defense, facilitated by merging the genetic background of multiple ethnic groups under the challenge of an aggravated bacterial exposition. Until today, the prevalence of Behcet’s disease, an autoimmune vasculitis of unknown etiology, is located along the countries of silk road (87), suggesting a selection process for distinct immunological traits along this route. Remarkably, a predisposition for distinct symptoms, such as vasculitis and thrombosis, is shared between Behcet’s disease and severe COVID-19. Interestingly, mutation of GM-CSF—one of the major cytokine initiating and perpetuating inflammatory diseases was shown to be also one of the risk alleles in developing Behcet-like disease (88). Thrombosis is frequently observed in COVID-19 patients suffering an aggravated disease course (89), and vasculitis resembling Kawasaki disease was specifically observed in children, subsequently to a suspected contact with SARS-CoV-2 (90). When connecting countries and regions with high mortality due to COVID-19 or persistent high infection rate of SARS-CoV-2 on a map in the early 2020, those countries are part of the former silk road, such as Iran, Turkey, northern Italy and southern China. Wuhan, the epicenter of COVID-19 is in less than 1,000 km distance from Guangzhou, the beginning of the ancient silk road, both cities were haunted by an extraordinary high rate of mortality during the epidemics (2, 91). On the other end, France and south of Switzerland sharing linguistic, cultural, and economic connections to northern Italy over a long-lasting period in human history. Both regions were heavily affected by COVID-19 related mortality (92). A similar indication is found in Canada, where exclusively the French-speaking Quebec is severely affected by COVID-19 mortality.
Thus, we propose the hypothesis, that during ancient times, over a period of 1,500 years along the silk road, distinct genetic traits affecting the redox equilibrium were shaped in the local inhabitants. As much those genetic traits favored the defense against bacterial pathogens in the past by an appropriate production of bactericidal ROS, the same genetic traits prove them vulnerable for COVID-19 related mortality in the 21st century as presented in Figure 1.
Discussion
The pathology of COVID-19 extends far beyond a pulmonary disease and does not resemble any other viral pneumonia reported so far (23). In order to search for therapeutic approaches, COVID-19 needs to be viewed as complex interaction between pulmonary, hematologic, and endothelial dysfunction in the context of an inflammatory condition. To solve such an intertwined mechanism, it is of great value that medical scientists investigate children and young adults due to their vastly different clinical outcomes.
Although a vast amount of clinical data and results in the basic science on COVID-19 are published up to date, the mechanism of severe disease progression, the differential in the rates of mortality depending on geographic location or ethnicity, and the reason why children and young adults are mostly protected from development of disease are unknown. In this review, we performed an approach to answer those questions by integrating historical circumstances, current clinical data and little noticed elements of immunity. Hereby, we propose that infants, children and young adults are protected from SARS-CoV-2 due to the polyclonality of natural IgM, able to recognize viral particles or infected cells by endogenous self-antigens, according to the danger model of immunity. The differences in severity of COVID-19 observed in adults, may have their roots in the genetics of bacterial defense mechanisms, revealing themselves as harmful in COVID-19 when combined with mechanical ventilation under high oxygen pressure.
In order to test our hypotheses, we propose the genetic analysis of children with Kawasaki-like disease and MIS-C with attention to genes involved in IgM Ab response and reactive oxygen metabolism. Furthermore, retrospective analysis and prospective studies on the use of nutritional supplements with antioxidant properties, such as vitamin C, are required to assess their potential impact on disease outcome in COVID-19. The search for correlations between the severity of SARS-CoV-2 infection and single nucleotide polymorphisms in genes involved in ROS metabolism would test our hypothesis.
Recent clinical observations support our hypothesis. Although it is currently uncertain whether immunomodulating agents, such as biological disease-modifying anti-rheumatic drugs (bDMARDs), affect the outcome of SARS-CoV-2 infections, the individual cases reported that bDMARDs treatment does not correlate with worse COVID-19 outcome (93). Also according to the guidelines of European League Against Rheumatism (EULAR), the immunomodulatory treatment advised to rheumatic patients should be continued (94). However, due to recently reported two fatal cases, some caution should be applied when using rituximab (RTX), a B-cell depleting bDMARD in patients with immune-mediated diseases (95). This observation is supported by a report on persistent SARS-CoV-2 viremia in two rituximab-treated patients with severe COVID-19 pneumonia and death without any sign of viral clearance (96). Alarming data from the German National Registry for patients with IRD infected with SARS-CoV-2 show that 61% patients treated with RTX required hospitalization while 50% needed ventilation (97). Indeed, the consequence of the RTX administration is very low IgM concentration, which may support our hypothesis of an important role of IgM in protective immunity against COVID-19 (98). Additionally, the mild clinical course of COVID-19 in patients with agammaglobulinemia suggest that it is not IgG that protect from severe or fatal COVID-19 course (99).
Conclusions And Suggestions For Potential Therapeutic Interventions
Concluding from our hypothesis on reactive oxygen species as key factor for COVID-19 related mortality, we suggest the use of an amphiphilic antioxidant for targeting ROS in lysosomal compartments. The mild sedative drug melatonin shares antioxidant and amphiphilic properties, along with a low potential for adverse effects (100). Another alternative to COVID-19 treatment due to its antioxidant activity and cytoprotective effects may be the most abundant free amino acid in humans, taurine (101). Taurine regulates inflammatory processes associated with oxidative stress, such as the detoxification of hypochlorous acid, which results in formation of less toxic and anti-inflammatory taurine chloramine. We suggest the identification of polymorphisms in genes related to reactive oxygen metabolism to estimate the potential susceptibility of infected patients for severe disease progression and clinical trials with intravenous immunoglobulin preparations, enriched for IgM in the early stage of disease.
Data Availability Statement
The original contributions presented in the study are included in the article/supplementary material. Further inquiries can be directed to the corresponding author.
Author Contributions
MM and H-JG wrote the manuscript. H-JG revised the manuscript for important intellectual content. All authors contributed to the article and approved the submitted version.
Funding
This work was supported by core grant S/9 to the National Institute of Geriatrics, Rheumatology, and Rehabilitation from Polish Ministry of Science and Higher Education.
Conflict of Interest
The authors declare that the research was conducted in the absence of any commercial or financial relationships that could be construed as a potential conflict of interest.
References
1. Tay MZ, Poh CM, Renia L, MacAry PA, Ng LFP. The Trinity of COVID-19: Immunity, Inflammation and Intervention. Nat Rev Immunol (2020) 20(6):363–74. doi: 10.1038/s41577-020-0311-8
2. Yang X, Yu Y, Xu J, Shu H, Xia J, Liu H, et al. Clinical Course and Outcomes of Critically Ill Patients With SARS-CoV-2 Pneumonia in Wuhan, China: A Single-Centered, Retrospective, Observational Study. Lancet Respir Med (2020) 8(5):475–81. doi: 10.1016/S2213-2600(20)30079-5
3. Favalli EG, Ingegnoli F, De Lucia O, Cincinelli G, Cimaz R, Caporali R. Covid-19 Infection and Rheumatoid Arthritis: Faraway, So Close! Autoimmun Rev (2020) 19(5):102523. doi: 10.1016/j.autrev.2020.102523
4. Richardson PJ, Corbellino M, Stebbing J. Baricitinib for COVID-19: A Suitable Treatment? - Authors’ Reply. Lancet Infect Dis (2020) 20(9):1013–4. doi: 10.1016/S1473-3099(20)30270-X
5. Mehta P, Porter JC, Manson JJ, Isaacs JD, Openshaw PJM, McInnes IB, et al. Therapeutic Blockade of Granulocyte Macrophage Colony-Stimulating Factor in COVID-19-Associated Hyperinflammation: Challenges and Opportunities. Lancet Respir Med (2020) 8(8):822–30. doi: 10.1016/S2213-2600(20)30267-8
6. Henderson LA, Canna SW, Schulert GS, Volpi S, Lee PY, Kernan KF, et al. On the Alert for Cytokine Storm: Immunopathology in COVID-19. Arthritis Rheumatol (2020) 72(7):1059–63. doi: 10.1002/art.41285
7. Mehta P, McAuley DF, Brown M, Sanchez E, Tattersall RS, Manson JJ, et al. Covid-19: Consider Cytokine Storm Syndromes and Immunosuppression. Lancet (2020) 395(10229):1033–4. doi: 10.1016/S0140-6736(20)30628-0
8. Elezkurtaj S, Greuel S, Ihlow J, Michaelis EG, Bischoff P, Kunze CA, et al. Causes of Death and Comorbidities in Hospitalized Patients With COVID-19. Sci Rep (2021) 11(1):4263. doi: 10.1038/s41598-021-82862-5
9. Lu X, Zhang L, Du H, Zhang J, Li YY, Qu J, et al. SARS-Cov-2 Infection in Children. N Engl J Med (2020) 382(17):1663–5. doi: 10.1056/NEJMc2005073
10. Parri N, Lenge M, Buonsenso D, G. Coronavirus Infection in Pediatric Emergency Departments Research. Children With Covid-19 in Pediatric Emergency Departments in Italy. N Engl J Med (2020) 383(2):187–90. doi: 10.1056/NEJMc2007617
11. Zimmermann P, Curtis N. Covid-19 in Children, Pregnancy and Neonates: A Review of Epidemiologic and Clinical Features. Pediatr Infect Dis J (2020) 39(6):469–77. doi: 10.1097/INF.0000000000002700
12. Leung CW, Kwan YW, Ko PW, Chiu SS, Loung PY, Fong NC, et al. Severe Acute Respiratory Syndrome Among Children. Pediatrics (2004) 113(6):e535–43. doi: 10.1542/peds.113.6.e535
13. Alfaraj SH, Al-Tawfiq JA, Altuwaijri TA, Memish ZA. Middle East Respiratory Syndrome Coronavirus in Pediatrics: A Report of Seven Cases From Saudi Arabia. Front Med (2019) 13(1):126–30. doi: 10.1007/s11684-017-0603-y
14. Kam KQ, Yung CF, Cui L, Tzer Pin Lin R, Mak TM, Maiwald M, et al. A Well Infant With Coronavirus Disease 2019 With High Viral Load. Clin Infect Dis (2020) 71(15):847–9. doi: 10.1093/cid/ciaa201
15. Riphagen S, Gomez X, Gonzalez-Martinez C, Wilkinson N, Theocharis P. Hyperinflammatory Shock in Children During COVID-19 Pandemic. Lancet (2020) 395(10237):1607–8. doi: 10.1016/S0140-6736(20)31094-1
16. Williamson EJ, Walker AJ, Bhaskaran K, Bacon S, Bates C, Morton CE, et al. Factors Associated With COVID-19-Related Death Using Opensafely. Nature (2020) 584(7821):430–6. doi: 10.1038/s41586-020-2521-4
17. Cheung EW, Zachariah P, Gorelik M, Boneparth A, Kernie SG, Orange JS, et al. Multisystem Inflammatory Syndrome Related to COVID-19 in Previously Healthy Children and Adolescents in New York City. JAMA (2020) 324(3):294–6. doi: 10.1001/jama.2020.10374
18. Gruber CN, Patel RS, Trachtman R, Lepow L, Amanat F, Krammer F, et al. Mapping Systemic Inflammation and Antibody Responses in Multisystem Inflammatory Syndrome in Children (Mis-C). Cell (2020) 183(4):982–95.e14. doi: 10.1016/j.cell.2020.09.034
19. Viner RM, Mytton OT, Bonell C, Melendez-Torres GJ, Ward J, Hudson L, et al. Susceptibility to SARS-CoV-2 Infection Among Children and Adolescents Compared With Adults: A Systematic Review and Meta-Analysis. JAMA Pediatr (2020) 175(2):143–56. doi: 10.1001/jamapediatrics.2020.4573
20. Felsenstein S, Hedrich CM. Covid-19 in Children and Young People. Lancet Rheumatol (2020) 2(9):e514–6. doi: 10.1016/S2665-9913(20)30212-5
21. Sisk B, Cull W, Harris JM, Rothenburger A, Olson L. National Trends of Cases of COVID-19 in Children Based on US State Health Department Data. Pediatrics (2020) 146(6):e2020027425. doi: 10.1542/peds.2020-027425
22. Cristiani L, Mancino E, Matera L, Nenna R, Pierangeli A, Scagnolari C, et al. Will Children Reveal Their Secret? The Coronavirus Dilemma. Eur Respir J (2020) 55(4):2000749. doi: 10.1183/13993003.00749-2020
23. Lingappan K, Karmouty-Quintana H, Davies J, Akkanti B, Harting MT. Understanding the Age Divide in COVID-19: Why are Children Overwhelmingly Spared? Am J Physiol Lung Cell Mol Physiol (2020) 319(1):L39–44. doi: 10.1152/ajplung.00183.2020
24. Benn CS, Netea MG, Selin LK, Aaby P. A Small Jab - a Big Effect: Nonspecific Immunomodulation by Vaccines. Trends Immunol (2013) 34(9):431–9. doi: 10.1016/j.it.2013.04.004
25. Schoeman D, Fielding BC. Coronavirus Envelope Protein: Current Knowledge. Virol J (2019) 16(1):69. doi: 10.1186/s12985-019-1182-0
26. Ye ZW, Yuan S, Yuen KS, Fung SY, Chan CP, Jin DY. Zoonotic Origins of Human Coronaviruses. Int J Biol Sci (2020) 16(10):1686–97. doi: 10.7150/ijbs.45472
27. Hamming I, Timens W, Bulthuis ML, Lely AT, Navis G, van Goor H. Tissue Distribution of ACE2 Protein, the Functional Receptor for SARS Coronavirus. A First Step in Understanding SARS Pathogenesis. J Pathol (2004) 203(2):631–7. doi: 10.1002/path.1570
28. Fung TS, Liu DX. Coronavirus Infection, ER Stress, Apoptosis and Innate Immunity. Front Microbiol (2014) 5:296. doi: 10.3389/fmicb.2014.00296
29. New JS, King RG, Kearney JF. Glycan Reactive Natural Antibodies and Viral Immunity. Viral Immunol (2020) 33(4):266–76. doi: 10.1089/vim.2019.0136
30. Gerencer M, Barrett PN, Kistner O, Mitterer A, Dorner F. Natural IgM Antibodies in Baby Rabbit Serum Bind High-Mannose Glycans on HIV Type 1 Glycoprotein 120/160 and Activate Classic Complement Pathway. AIDS Res Hum Retroviruses (1998) 14(7):599–605. doi: 10.1089/aid.1998.14.599
31. Berry JD, Hay K, Rini JM, Yu M, Wang L, Plummer FA, et al. Neutralizing Epitopes of the SARS-CoV s-Protein Cluster Independent of Repertoire, Antigen Structure or mAb Technology. MAbs (2010) 2(1):53–66. doi: 10.4161/mabs.2.1.10788
32. Qiu M, Shi Y, Guo Z, Chen Z, He R, Chen R, et al. Antibody Responses to Individual Proteins of SARS Coronavirus and Their Neutralization Activities. Microbes Infect (2005) 7(5-6):882–9. doi: 10.1016/j.micinf.2005.02.006
33. Zhang L, Zhang F, Yu W, He T, Yu J, Yi CE, et al. Antibody Responses Against SARS Coronavirus Are Correlated With Disease Outcome of Infected Individuals. J Med Virol (2006) 78(1):1–8. doi: 10.1002/jmv.20499
34. Peiris JS, Chu CM, Cheng VC, Chan KS, Hung IF, Poon LL, et al. Clinical Progression and Viral Load in a Community Outbreak of Coronavirus-Associated SARS Pneumonia: A Prospective Study. Lancet (2003) 361(9371):1767–72. doi: 10.1016/S0140-6736(03)13412-5
35. Atyeo C, Fischinger S, Zohar T, Slein MD, Burke J, Loos C, et al. Distinct Early Serological Signatures Track With SARS-CoV-2 Survival. Immunity (2020) 53(3):524–32.e4. doi: 10.1016/j.immuni.2020.07.020
36. Weisberg SP, Connors T, Zhu Y, Baldwin M, Lin WH, Wontakal S, et al. Antibody Responses to SARS-CoV2 Are Distinct in Children With MIS-C Compared to Adults With COVID-19. medRxiv (2020) 2020.07.12.20151068. doi: 10.1101/2020.07.12.20151068
37. Matzinger P. The Danger Model: A Renewed Sense of Self. Science (2002) 296(5566):301–5. doi: 10.1126/science.1071059
38. Grönwall C, Silverman GJ. Natural IgM: Beneficial Autoantibodies for the Control of Inflammatory and Autoimmune Disease. J Clin Immunol (2014) 34(Suppl 1):S12–21. doi: 10.1007/s10875-014-0025-4
39. Prieto JMB, Felippe MJB. Development, Phenotype, and Function of Non-Conventional B Cells. Comp Immunol Microbiol Infect Dis (2017) 54:38–44. doi: 10.1016/j.cimid.2017.08.002
40. Clarke AJ, Riffelmacher T, Braas D, Cornall RJ, Simon AK. B1a B Cells Require Autophagy for Metabolic Homeostasis and Self-Renewal. J Exp Med (2018) 215(2):399–413. doi: 10.1084/jem.20170771
41. Kumar D, Romero Y, Schuck KN, Smalley H, Subedi B, Fleming SD. Drivers and Regulators of Humoral Innate Immune Responses to Infection and Cancer. Mol Immunol (2020) 121:99–110. doi: 10.1016/j.molimm.2020.03.005
42. Ehrenstein MR, Notley CA. The Importance of Natural IgM: Scavenger, Protector and Regulator. Nat Rev Immunol (2010) 10(11):778–86. doi: 10.1038/nri2849
43. Ochsenbein AF, Fehr T, Lutz C, Suter M, Brombacher F, Hengartner H, et al. Control of Early Viral and Bacterial Distribution and Disease by Natural Antibodies. Science (1999) 286(5447):2156–9. doi: 10.1126/science.286.5447.2156
44. Fehr T, Naim HY, Bachmann MF, Ochsenbein AF, Spielhofer P, Bucher E, et al. T-Cell Independent IgM and Enduring Protective IgG Antibodies Induced by Chimeric Measles Viruses. Nat Med (1998) 4(8):945–8. doi: 10.1038/nm0898-945
45. Lydyard PM, Quartey-Papafio R, Broker B, Mackenzie L, Jouquan J, Blaschek MA, et al. The Antibody Repertoire of Early Human B Cells. I. High Frequency of Autoreactivity and Polyreactivity. Scand J Immunol (1990) 31(1):33–43. doi: 10.1111/j.1365-3083.1990.tb02740.x
46. Jans J, Pettengill M, Kim D, van der Made C, de Groot R, Henriet S, et al. Human Newborn B Cells Mount an Interferon-α/β Receptor-Dependent Humoral Response to Respiratory Syncytial Virus. J Allergy Clin Immunol (2017) 139(6):1997–2000.e4. doi: 10.1016/j.jaci.2016.10.032
47. Simões E Silva AC, Leal CRV. Is SARS-CoV-2 Vertically Transmitted? Front Pediatr (2020) 8:276. doi: 10.3389/fped.2020.00276
48. Dong L, Tian J, He S, Zhu C, Wang J, Liu C, et al. Possible Vertical Transmission of SARS-CoV-2 From an Infected Mother to Her Newborn. JAMA (2020) 323(18):1846–8. doi: 10.1001/jama.2020.4621
49. Zeng H, Xu C, Fan J, Tang Y, Deng Q, Zhang W, et al. Antibodies in Infants Born to Mothers With COVID-19 Pneumonia. JAMA (2020) 323(18):1848–9. doi: 10.1001/jama.2020.4861
50. Holodick NE, Rodríguez-Zhurbenko N, Hernández AM. Defining Natural Antibodies. Front Immunol (2017) 8:872. doi: 10.3389/fimmu.2017.00872
51. Suthers B, Hansbro P, Thambar S, McEvoy M, Peel R, Attia J. Pneumococcal Vaccination may Induce Anti-Oxidized Low-Density Lipoprotein Antibodies That Have Potentially Protective Effects Against Cardiovascular Disease. Vaccine (2012) 30(27):3983–5. doi: 10.1016/j.vaccine.2012.03.084
52. Escobar LE, Molina-Cruz A, Barillas-Mury C. BCG Vaccine Protection From Severe Coronavirus Disease 2019 (COVID-19). Proc Natl Acad Sci USA (2020) 117(30):17720–6. doi: 10.1073/pnas.2008410117
53. Chou MY, Fogelstrand L, Hartvigsen K, Hansen LF, Woelkers D, Shaw PX, et al. Oxidation-Specific Epitopes Are Dominant Targets of Innate Natural Antibodies in Mice and Humans. J Clin Invest (2009) 119(5):1335–49. doi: 10.1172/JCI36800
54. Wang C, Turunen SP, Kummu O, Veneskoski M, Lehtimäki J, Nissinen AE, et al. Natural Antibodies of Newborns Recognize Oxidative Stress-Related Malondialdehyde Acetaldehyde Adducts on Apoptotic Cells and Atherosclerotic Plaques. Int Immunol (2013) 25(10):575–87. doi: 10.1093/intimm/dxt022
55. Yong-Rui Z, Christine G, Betty D. Chapter 13: B Cells. In: Kelley and Firestein’s Textbook of Rheumatology, Tenth Edition. Elsevier (2017). p. 207–30.
56. Baumgarth N, Herman OC, Jager GC, Brown LE, Herzenberg LA, Chen J. B-1 and B-2 Cell-Derived Immunoglobulin M Antibodies Are Nonredundant Components of the Protective Response to Influenza Virus Infection. J Exp Med (2000) 192(2):271–80. doi: 10.1084/jem.192.2.271
57. Zeng Z, Surewaard BGJ, Wong CHY, Guettler C, Petri B, Burkhard R, et al. Sex-Hormone-Driven Innate Antibodies Protect Females and Infants Against EPEC Infection. Nat Immunol (2018) 19(10):1100–11. doi: 10.1038/s41590-018-0211-2
58. Haury M, Sundblad A, Grandien A, Barreau C, Coutinho A, Nobrega A. The Repertoire of Serum IgM in Normal Mice Is Largely Independent of External Antigenic Contact. Eur J Immunol (1997) 27(6):1557–63. doi: 10.1002/eji.1830270635
59. Verdoni L, Mazza A, Gervasoni A, Martelli L, Ruggeri M, Ciuffreda M, et al. An Outbreak of Severe Kawasaki-Like Disease at the Italian Epicentre of the SARS-CoV-2 Epidemic: An Observational Cohort Study. Lancet (2020) 395(10239):1771–8. doi: 10.1016/S0140-6736(20)31103-X
60. Yan R, Zhang Y, Li Y, Xia L, Guo Y, Zhou Q. Structural Basis for the Recognition of SARS-CoV-2 by Full-Length Human ACE2. Science (2020) 367(6485):1444–8. doi: 10.1126/science.abb2762
61. Vaduganathan M, Vardeny O, Michel T, McMurray JJV, Pfeffer MA, Solomon SD. Renin-Angiotensin-Aldosterone System Inhibitors in Patients With Covid-19. N Engl J Med (2020) 382(17):1653–9. doi: 10.1056/NEJMsr2005760
62. Benter IF, Yousif MH, Dhaunsi GS, Kaur J, Chappell MC, Diz DI. Angiotensin-(1-7) Prevents Activation of NADPH Oxidase and Renal Vascular Dysfunction in Diabetic Hypertensive Rats. Am J Nephrol (2008) 28(1):25–33. doi: 10.1159/000108758
63. Andrzejczak D. [Angiotensin (1-7): A New Link in the Renin-Angiotensin-Aldosterone System (RAAS)]. Postepy Hig Med Dosw (Online) (2009) 63:23–9.
64. Chen J, Jiang Q, Xia X, Liu K, Yu Z, Tao W, et al. Individual Variation of the SARS-CoV-2 Receptor ACE2 Gene Expression and Regulation. Aging Cell (2020) 19(7):e13168. doi: 10.1111/acel.13168
65. Zhang Z, Guo L, Huang L, Zhang C, Luo R, Zeng L, et al. Distinct Disease Severity Between Children and Older Adults With COVID-19: Impacts of ACE2 Expression, Distribution, and Lung Progenitor Cells. Clin Infect Dis (2021) 3:ciaa1911. doi: 10.1093/cid/ciaa1911
66. Kell AM, Gale M. RIG-I in RNA Virus Recognition. Virology (2015) 479-480:110–21. doi: 10.1016/j.virol.2015.02.017
67. Mills EL, Kelly B, O’Neill LAJ. Mitochondria Are the Powerhouses of Immunity. Nat Immunol (2017) 18(5):488–98. doi: 10.1038/ni.3704
68. Ratajczak MZ, Kucia M. Sars-CoV-2 Infection and Overactivation of Nlrp3 Inflammasome as a Trigger of Cytokine “Storm” and Risk Factor for Damage of Hematopoietic Stem Cells. Leukemia (2020) 34(7):1726–9. doi: 10.1038/s41375-020-0887-9
69. Jeon YJ, Kim HJ. Duox2-induced Innate Immune Responses in the Respiratory Epithelium and Intranasal Delivery of Duox2 DNA Using Polymer That Mediates Immunization. Appl Microbiol Biotechnol (2018) 102(10):4339–43. doi: 10.1007/s00253-018-8956-y
70. Soucy-Faulkner A, Mukawera E, Fink K, Martel A, Jouan L, Nzengue Y, et al. Requirement of NOX2 and Reactive Oxygen Species for Efficient RIG-I-mediated Antiviral Response Through Regulation of MAVS Expression. PloS Pathog (2010) 6(6):e1000930. doi: 10.1371/journal.ppat.1000930
71. van der Vliet A. NADPH Oxidases in Lung Biology and Pathology: Host Defense Enzymes, and More. Free Radic Biol Med (2008) 44(6):938–55. doi: 10.1016/j.freeradbiomed.2007.11.016
72. Ñamendys-Silva SA. Respiratory Support for Patients With COVID-19 Infection. Lancet Respir Med (2020) 8(4):e18. doi: 10.1016/S2213-2600(20)30110-7
73. Liao D, Zhou F, Luo L, Xu M, Wang H, Xia J, et al. Haematological Characteristics and Risk Factors in the Classification and Prognosis Evaluation of COVID-19: A Retrospective Cohort Study. Lancet Haematol (2020) 7(9):e671–e8. doi: 10.1016/S2352-3026(20)30217-9
74. Henry BM, de Oliveira MHS, Benoit S, Plebani M, Lippi G. Hematologic, Biochemical and Immune Biomarker Abnormalities Associated With Severe Illness and Mortality in Coronavirus Disease 2019 (COVID-19): A Meta-Analysis. Clin Chem Lab Med (2020) 58(7):1021–8. doi: 10.1515/cclm-2020-0369
75. Velavan TP, Meyer CG. Mild Versus Severe COVID-19: Laboratory Markers. Int J Infect Dis (2020) 95:304–7. doi: 10.1016/j.ijid.2020.04.061
76. Bernard K, Hecker L, Luckhardt TR, Cheng G, Thannickal VJ. NADPH Oxidases in Lung Health and Disease. Antioxid Redox Signal (2014) 20(17):2838–53. doi: 10.1089/ars.2013.5608
77. Arnold DE, Heimall JR. A Review of Chronic Granulomatous Disease. Adv Ther (2017) 34(12):2543–57. doi: 10.1007/s12325-017-0636-2
78. Yeh HL, Kuo LT, Sung FC, Yeh CC. Association Between Polymorphisms of Antioxidant Gene (MnSOD, CAT, and GPx1) and Risk of Coronary Artery Disease. BioMed Res Int (2018) p:5086869. doi: 10.1155/2018/5086869
79. Li T, Xi HF, Luo HM, Liu WX, Gao X, Liu DW, et al. Association of the NAD(P)H Oxidase p22 Phox Gene C242T Polymorphism With Type 2 Diabetes Mellitus, Diabetic Nephropathy, and Carotid Atherosclerosis With Type 2 Diabetes Mellitus: A Meta-Analysis. Meta Gene (2015) 6:1–8. doi: 10.1016/j.mgene.2015.07.009
80. Ito D, Murata M, Watanabe K, Yoshida T, Saito I, Tanahashi N, et al. C242T Polymorphism of NADPH Oxidase P22 PHOX Gene and Ischemic Cerebrovascular Disease in the Japanese Population. Stroke (2000) 31(4):936–9. doi: 10.1161/01.STR.31.4.936
81. Bedard K, Attar H, Bonnefont J, Jaquet V, Borel C, Plastre O, et al. Three Common Polymorphisms in the CYBA Gene Form a Haplotype Associated With Decreased ROS Generation. Hum Mutat (2009) 30(7):1123–33. doi: 10.1002/humu.21029
82. Gurwitz D. Angiotensin Receptor Blockers as Tentative SARS-CoV-2 Therapeutics. Drug Dev Res (2020) 81(5):537–40. doi: 10.1002/ddr.21656
83. Liu Y, Yang Y, Zhang C, Huang F, Wang F, Yuan J, et al. Clinical and Biochemical Indexes From 2019-nCoV Infected Patients Linked to Viral Loads and Lung Injury. Sci China Life Sci (2020) 63(3):364–74. doi: 10.1007/s11427-020-1643-8
84. Kuba K, Imai Y, Rao S, Gao H, Guo F, Guan B, et al. A Crucial Role of Angiotensin Converting Enzyme 2 (ACE2) in SARS Coronavirus-Induced Lung Injury. Nat Med (2005) 11(8):875–9. doi: 10.1038/nm1267
85. Dijkman R, Jebbink MF, Deijs M, Milewska A, Pyrc K, Buelow E, et al. Replication-Dependent Downregulation of Cellular Angiotensin-Converting Enzyme 2 Protein Expression by Human Coronavirus NL63. J Gen Virol (2012) 93(Pt 9):1924–9. doi: 10.1099/vir.0.043919-0
86. Zaffiri L, Gardner J, Toledo-Pereyra LH. History of Antibiotics. From Salvarsan to Cephalosporins. J Invest Surg (2012) 25(2):67–77. doi: 10.3109/08941939.2012.664099
87. Davatchi F, Chams-Davatchi C, Shams H, Shahram F, Nadji A, Akhlaghi M, et al. Behcet’s Disease: Epidemiology, Clinical Manifestations, and Diagnosis. Expert Rev Clin Immunol (2017) 13(1):57–65. doi: 10.1080/1744666X.2016.1205486
88. Rosler B, Heinhuis B, Wang X, Silvestre R, Joosten LAB, Netea MG, et al. Mimicking Behcet’s Disease: GM-CSF Gain of Function Mutation in a Family Suffering From a Behcet’s Disease-Like Disorder Marked by Extreme Pathergy. Clin Exp Immunol (2021) 204(2):189–98. doi: 10.1111/cei.13568
89. Price LC, McCabe C, Garfield B, Wort SJ. Thrombosis and COVID-19 Pneumonia: The Clot Thickens! Eur Respir J (2020) 56(1):2001608. doi: 10.1183/13993003.01608-2020
90. Moreira A. Kawasaki Disease Linked to COVID-19 in Children. Nat Rev Immunol (2020) 20(7):407. doi: 10.1038/s41577-020-0350-1
91. Li XQ, Cai WF, Huang LF, Chen C, Liu YF, Zhang ZB, et al. [Comparison of Epidemic Characteristics Between SARS in 2003 and COVID-19 in 2020 in Guangzhou]. Zhonghua Liu Xing Bing Xue Za Zhi (2020) 41(5):634–7. doi: 10.3760/cma.j.cn112338-20200228-00209
92. Ceylan Z. Estimation of COVID-19 Prevalence in Italy, Spain, and France. Sci Total Environ (2020) 729:138817. doi: 10.1016/j.scitotenv.2020.138817
93. Monti S, Balduzzi S, Delvino P, Bellis E, Quadrelli VS, Montecucco C. Clinical Course of COVID-19 in a Series of Patients With Chronic Arthritis Treated With Immunosuppressive Targeted Therapies. Ann Rheum Dis (2020) 79(5):667–8. doi: 10.1136/annrheumdis-2020-217424
94. Eular Guidance for Patients COVID-19 Outbreak. Available at: https://www.eular.org/eular_guidance_for_patients_covid19_outbreak.cfm.
95. Schulze-Koops H, Krueger K, Vallbracht I, Hasseli R, Skapenko A. Increased Risk for Severe COVID-19 in Patients With Inflammatory Rheumatic Diseases Treated With Rituximab. Ann Rheum Dis (2020) annrheumdis-2020-218075. doi: 10.1136/annrheumdis-2020-218075
96. Tepasse PR, Hafezi W, Lutz M, Kuhn J, Wilms C, Wiewrodt R, et al. Persisting SARS-CoV-2 Viraemia After Rituximab Therapy: Two Cases With Fatal Outcome and a Review of the Literature. Br J Haematol (2020) 190(2):185–8. doi: 10.1111/bjh.16896
97. Schulze-Koops H, Krueger K, Vallbracht IV, Hasseli R, Skapenko A. Treatment of Patients With Inflammatory Rheumatic Diseases With Rituximab Should be Carefully Considered During the SARS-CoV-2/COVID-19 Pandemic. Response to: ‘Persistence of rT-PCR-SARS-CoV-2 Infection and Delayed Serological Response, as a Possible Effect of Rituximab According to the Hypothesis of Schulze-Koops Et Al’ by Benucci. Ann Rheum Dis (2020) annrheumdis-2020-218686. doi: 10.1136/annrheumdis-2020-218686
98. Kridin K, Ahmed AR. Post-Rituximab Immunoglobulin M (IgM) Hypogammaglobulinemia. Autoimmun Rev (2020) 19(3):102466. doi: 10.1016/j.autrev.2020.102466
99. Quinti I, Lougaris V, Milito C, Cinetto F, Pecoraro A, Mezzaroma I, et al. A Possible Role for B Cells in COVID-19? Lesson From Patients With Agammaglobulinemia. J Allergy Clin Immunol (2020) 146(1):211–213.e4. doi: 10.1016/j.jaci.2020.04.013
100. Zhang R, Wang X, Ni L, Di X, Ma B, Niu S, et al. Covid-19: Melatonin as a Potential Adjuvant Treatment. Life Sci (2020) 250:117583. doi: 10.1016/j.lfs.2020.117583
Keywords: COVID-19, natural IgM, children, Kawasaki-like disease, ACE-2 receptor, reactive oxygen species, silk road, B1 B cells
Citation: Massalska MA and Gober H-J (2021) How Children Are Protected From COVID-19? A Historical, Clinical, and Pathophysiological Approach to Address COVID-19 Susceptibility. Front. Immunol. 12:646894. doi: 10.3389/fimmu.2021.646894
Received: 28 December 2020; Accepted: 21 May 2021;
Published: 11 June 2021.
Edited by:
Eduardo P. Amaral, National Institute of Allergy and Infectious Diseases (NIH), United StatesReviewed by:
Puja Mehta, University College London, United KingdomAline Sardinha-Silva, National Institute of Allergy and Infectious Diseases (NIH), United States
Copyright © 2021 Massalska and Gober. This is an open-access article distributed under the terms of the Creative Commons Attribution License (CC BY). The use, distribution or reproduction in other forums is permitted, provided the original author(s) and the copyright owner(s) are credited and that the original publication in this journal is cited, in accordance with accepted academic practice. No use, distribution or reproduction is permitted which does not comply with these terms.
*Correspondence: Magdalena Anna Massalska, bWFnZGFsZW5hLm1hc3NhbHNrYUBzcGFydGFuc2thLnBs