- 1Department of Internal Medicine 5, Haematology and Oncology, Friedrich Alexander University Erlangen-Nuremberg (FAU), University Hospital Erlangen, Erlangen, Germany
- 2Children’s Hospital, Goethe-University Frankfurt, Frankfurt, Germany
- 3Experimental Immunology, Goethe University Frankfurt , Frankfurt, Germany
- 4 Frankfurt Cancer Institute, Goethe University, Frankfurt, Germany
- 5Department of Dermatology, Laboratory of Dendritic Cell Biology, University Hospital Erlangen and Friedrich-Alexander University Erlangen-Nuremberg (FAU), Erlangen, Germany
- 6Institute of Immunology, University Medical Center Mainz, Mainz, Germany
- 7Research Centre for Immunotherapy, University Medical Center Mainz, Mainz, Germany
Cellular therapy has entered the daily clinical life with the approval of CAR T cell therapeutics and dendritic cell (DCs) vaccines in the US and the EU. In addition, numerous other adoptive cellular products, including natural killer (NK) cells, are currently evaluated in early phase I/ II clinical trials for the treatment of cancer patients. Despite these promising accomplishments, various challenges remain to be mastered in order to ensure sustained therapeutic success. These include the identification of strategies by which tumor cells escape the immune system or establish an immunosuppressive tumor microenvironment (TME). As part of the innate immune system, DCs and NK cells are both present within the TME of various tumor entities. While NK cells are well known for their intrinsic anti-tumor activity by their cytotoxicity capacities and the secretion of pro-inflammatory cytokines, the role of DCs within the TME is a double-edged sword as different DC subsets have been described with either tumor-promoting or -inhibiting characteristics. In this review, we will discuss recent findings on the interaction of DCs and NK cells under physiological conditions and within the TME. One focus is the crosstalk of various DC subsets with NK cells and their impact on the progression or inhibition of tumor growth. In addition, we will provide suggestions to overcome the immunosuppressive outcome of the interaction of DCs and NK cells within the TME.
Introduction
Tumor surveillance is achieved by a complex interplay of the components of the innate and adaptive immune system. Here, we summarize our current knowledge on the role and interaction of dendritic cells (DCs) and natural killer (NK) cells in the tumor microenvironment (TME). While circulating NK cells are efficient at identifying and eliminating tumor cells, DCs bridge the innate and adaptive immune system via the uptake of tumor cell debris and the subsequent presentation of tumor-specific antigens to T cells (1). NK cells and DCs are currently used for immunotherapies to treat tumor patients, for NK cells exhibit the ability to directly eliminate tumor cells without prior sensitization, while DCs are able to initiate an immune response by presenting antigens and inducing tumor-antigen specific CD8+ T cell (2). Moreover, when DCs and NK cells encounter each other, they are able to promote each other's activation, maturation, and functional activity (3). Within the TME, conventional type 1 DCs (cDC1s) show a bidirectional crosstalk with NK cells, increasing the selective recruitment of cDC1 together with their differentiation, and maturation as well as NK cell activation. This allows a better tumor control, patient survival and improved therapeutic responses (4, 5). However, it is known that tumor cells develop highly efficient mechanisms to impair the functionality of NK cells and DCs to escape immune surveillance in order to ensure their own survival. The tumor establishes an immune suppressive environment by the secretion of immune suppressive cytokines and chemokines, by metabolic acidification (6), by the recruitment of immune-regulatory cells, such as regulatory T cells (T regs) and myeloid-derived suppressor cells (MDSCs), by affecting the polarization of macrophages as well as by up-regulation of immunosuppressive proteins including ligands for immune checkpoint (ICP) receptors, such as PD-L1. The latter have the ability to suppress the anti-tumor activity of DCs and NK cells as well as their ability to promote each other. Moreover, DCs may even switch from a tumor-inhibiting to a tumor-promoting subtype within the TME (7). In order to overcome the suppressive effect of the TME on NK cells and DCs, the bidirectional crosstalk of NK cells and DC1 plays a very important role in the coordination of immune responses against cancer. That is why the presence of both DC1s and NK cells within the TME is primordial, and their interaction represents a potential target to improve the efficacy of cancer immunotherapy (2).
In this review, we will first give a short overview about the biology of DCs and NK cells. Next, we will describe their interaction under physiological conditions and within the TME. Finally, we will discuss possibilities to overcome the suppressive impact of the TME and to restore efficient tumor immune surveillance.
NK Cell Biology
As part of the innate immune system, NK cells have the ability to lyse virally infected and malignant cells. They play an important role at eradicating cancer cells and in shaping the response of adaptive immune cells. NK cells develop and differentiate in the bone marrow and represent approximately 10% of the lymphocytes in the peripheral blood (PB). NK cells express CD56 on their surface and lack CD3 expression (CD56+CD3−). Human NK cells are separated into two subsets, with an immune regulatory CD56brightCD16− population harboring potent cytokine production capability and a highly cytotoxic CD56dimCD16+ population, representing the major NK cell population within the peripheral blood (90%). CD56dimCD16+ express the Fc gamma receptor III CD16 recognizing the Fc portion of antigen-bound antibodies allowing them to lyse the opsonized cell, a mechanism called antibody dependent cell-mediated cytotoxicity (ADCC) (8).
In contrast to T or B cells, NK cells recognize their target cells in an antigen-independent manner regulated by a variety of activating and inhibiting receptors. NK cell cytotoxicity is regulated by balanced signals of these activating and inhibitory receptors (9, 10).
Major histocompatibility (MHC) class I molecules, expressed on all healthy nucleated cells, act as ligands for the killer-immunoglobulin-like receptor-group (KIR) or natural killer group 2A (NKG2A), which are mainly responsible for providing NK cell inhibiting signals. These mechanisms ensure that NK cells become selectively activated by cells that lack MHC-I-expression, such as tumor cells, but tolerate healthy tissue in their immune response, the so called “missing-self”-theory. If MHC-I expression is absent, the inhibitory receptor signal is missing, and NK cells can be activated (11). Usually MHC-I-expression is reduced or completely down-regulated on virally infected or malignantly transformed cells as an escape mechanism from cytotoxic T cell recognition, which on the other hand renders them sensitive to NK cell killing (8, 12).
The most important signals for activation of NK cells are mediated by the family of the natural cytotoxicity receptors (NCRs), the C-type lectin-like receptor NKG2D and also certain KIR-subtypes. NKp30, NKp44 and NKp46 that belong to the group of NCRs are expressed on all activated NK cells and bind non-HLA-specific ligands like virus-derived molecules (HA/HN for NKp46, NKp44) or intracellular proteins (BAT3/BAG6 for NKp30), which are released following cell stress or transformation (13). Furthermore, MICA-, MICB- and ULPB (or RAETI)-encoded proteins bind to the NKG2D-receptor with their MHC-I-like extracellular domain. Those encoded proteins belong to a group of at least 100 different ligands which are selectively expressed on non-healthy cells for the induction of NK cell activation (14).
NK cell killing includes a variety of mechanisms. Upon engagement an immunological synapse is established between NK cells and their target cells. Subsequently, they release the content of their cytotoxic granules, including perforin and granzyme b, into the synaptic gap. While perforin penetrates the target cell’s membrane, granzyme b gets inside the cell and induce programmed cell death (2, 8). Moreover, NK cells are able to induce apoptotic cell death via expression of death receptor ligands like FasL and TNF-related apoptosis-inducing ligand (TRAIL) on their surface (8, 12).
DC Biology
DCs are important regulators of immune responses (15). They are sentinels of the immune system and settle all lymphoid as well as peripheral tissues. Here, they constantly take up antigens from the surrounding and process these antigens. After migration to the local draining lymph node, they present these processed antigens as peptide MHC complexes to T cells. Depending on the environment during encounter of the antigen, DCs either induce peripheral tolerance (steady state) or T cell immunity (inflammation) (16–18). In steady state, DCs induce peripheral tolerance by presenting antigens without costimulatory molecules leading to anergy or the deletion of antigen-specific T cells. However, in inflammatory settings and instructed by microbiota-derived cues resulting in tonic IFNAR signaling DCs can be activated by danger signals or pathogens via pattern recognition receptors such as Toll-like receptors (TLR) or C-type lectin receptors (CLR) (1, 19, 20). This leads to enhanced expression of peptide MHC complexes as well as costimulatory molecules (e.g. CD40, CD86) and the secretion of T cell polarizing cytokines such as IL-12 (17). Thereby, DCs are capable to induce and direct T cell responses. However, DCs are also able to interact with cells of the innate immune system such as NK cells (4, 21–24).
As all immune cells, except for yolk sac-derived macrophages, including Langerhans cells, DCs originate from hematopoietic stem cells (HSC) in the bone marrow (25). After differentiation of HSCs into common myeloid progenitor (MPP) cells via the steps of multipotent progenitor (MPP) and lymphoid-primed multipotent progenitor (LMPP) cells, the common DC progenitor (CDP) separates from the common monocyte progenitor (cMoP). The CDP can give rise to all DC subsets including plasmacytoid (pDC) as well as conventional DCs (cDC). Depending on the expression of specific transcription factors such as IRF8 and IRF4, cDCs further differentiate in cDC1 and cDC2, respectively (18). In contrast to cDCs, pDCs can also arise from a common lymphoid progenitor (CLP). Terminal-differentiated as well as preDCs are able to leave the bone marrow and settle all lymphoid as well as peripheral tissues.
Based on ontogeny, surface receptor expression as well as functions, DCs are subdivided into different subsets, which are mainly conserved between human and mice (17, 18, 25–27). pDCs share high transcriptional similarities between humans and mice and are identified by the expression of Siglec-H, CD45R (B220), and CD317 (PDCA-1) in mice, and by CD303a (BDCA-2), CD304 (BDCA-4) as well as CD123 (IL-3Rα chain) in humans. In both species, they share the expression of TLR7 and TLR9 and strongly react to viral infections with the secretion of vast amounts of type I IFN. However, their true role in T cell stimulation is a matter of ongoing discussions (28–31).
cDCs can be further separated into cDC1 and cDC2 in both, mice, and humans. Human and murine cDC1 share the specific expression of CLEC9A, XCR1, and CADM1 (17, 32–36). While murine cDC1 further express CD8α in lymphoid and CD103 in non-lymphoid tissues, human cDC1 are identified by a high expression of CD141 (BDCA-3) (1). In both species, cDC1 highly express TLR3 and respond to stimulation of TLR3 with production of type III IFN (37). They further produce IL-12, which is crucial for interaction with T cells as well as with NK cells. In mice, cDC1 are the only cross-presenting DC subpopulation for the activation of cytotoxic CD8+ T cells. This highlights their vital role in the immune response in the context of tumors as well as viral infections (4, 38–40). Furthermore, they polarize CD4+ T cells preferentially into Th1 cells. However, recent studies on human DCs suggest that all DC subpopulations are able to cross-present antigens and cDC1 excel only in the cross-presentation of necrotic cell-derived antigens, presumably due to the expression of the F-actin receptor CLEC9A (41–44).
Human and murine cDC2 transcriptionally rely on IRF4 and share the expression of CD11c and SIRPα (18). Human cDC2 can be identified by the expression of CD1c (BDCA-1), CD301 (CLEC10A) as well as the FcϵR1α, while murine cDC2 express CD11b as well as CD4 and DCIR2 in lymphoid tissues (27, 45, 46). In general, cDC2 are a more heterogeneous DC subpopulation than cDC1. Recent approaches, using single cell transcriptomics, showed that both human and murine cDC2 harbor different subpopulations termed DC2/DC3 and cDC2a/b, respectively (25, 31, 46–49). In the murine system, cDC2 have a lower capacity to cross-present antigens to CD8+ T cells, however they are efficient to polarize naïve T cells into Th2 and Th17 cells (50–52). In contrast, in comparison to human cDC1, human cDC2 have been suggested to harbor similar capacity to cross-present antigens to CD8+ T cells. Furthermore, they have been shown to polarize CD4+ T cells into Th1 cells, which can be linked to the ability of human cDC2 to secrete IL-12p70 under inflammatory conditions (27). Both, cDC1 and cDC2 have a detrimental role in the preservation of peripheral tolerance as murine cDC1 convert naïve CD4+ T cells into FoxP3+ T reg cells, whereas cDC2 expand already existing FoxP3+ T reg cells in the periphery (53).
NK-DC Interaction Under Physiological Conditions
The first key report on the interaction between DCs and NK cells to control tumor growth was published in 1999, describing the coordinated control of mesothelioma tumors in mice by both subsets (54). Since then, several groups have explored their complex relationship revealing a bidirectional crosstalk between DCs and NK cells. Human NK cells are able to induce DC maturation and IL-12 production upon co-culture with immature monocyte-derived DCs (moDCs), which was suggested to be contact-dependent and relies on the secretion of NK cell-derived cytokines like TNF-α (55, 56). In contrast, freshly isolated human NK cells are activated by lipopolysaccharide (LPS)-treated mature moDCs (55). NK cell activation by moDCs was shown to be mediated mainly by cytokines of the IL-12 family. Initial reports demonstrated that IL-12 secretion by LPS-matured human moDCs stimulates IFN-γ production in NK cells (57), and similar results have been reported for other members of this cytokine family. Further, IL-23 has been suggested to directly stimulate IFN-γ production in human CD56bright NK cells, and in cooperation with IL-18, in CD56bright and CD56dim NK cells (58). Additionally, LPS-matured moDC-derived IL-27 increased IFN-γ production and upregulation of NKp46- and ADCC-dependent NK cell cytotoxicity (59). The importance of DC-derived IL-12 for NK cell activation has been illustrated within an in vivo model of mouse cytomegalovirus (MCMV) infection. Upon MCMV infection cDCs secrete IL-12p40 and IL-15 in a TLR9/MyD88-dependent manner leading to NK cell activation and IFN-γ production which ultimately results in MCMV clearance (60).
Moreover, other cytokines such as IL-15 or IFN-α were able to increase NK cell proliferation and survival as well as cytotoxicity, respectively (21, 61–64). Both cytokines are involved within the NK cell-mediated rejection of CD8+ T cell-resistant tumors within various tumor mouse models upon treatment with the STING agonist cyclic dinucleotide (CDN). CDN activated NK cells directly via type I IFN signaling and indirectly via IL-15Rα up-regulation in DCs, which also depended on type I IFN (65). Moreover, co-incubation of human peripheral blood NK cells with autologous lung DCs from smokers suffering from chronic obstructive pulmonary disease (COPD) resulted in an IL-15-dependent increased killing of epithelial lungs cells compared to DCs from smokers without COPD. Similar results were obtained when using lung DCs from mice who were exposed to cigarette smoke compared to pure air exposed ones (66).
In addition, activated NK cells were reported to specifically kill immature moDCs (56) via NKp30 (67) and DNAM-1 (68). This process was executed mainly by NKG2A+KIR- NK cells. This observation can be explained due to the week expression of the inhibitory NKG2A receptor ligand HLA-E on immature moDCs compared to LPS-matured moDCs, whereas HLA class I molecules, the ligands for the inhibitory KIRs, are expressed on both DCs populations (69). Similar, NK cells have been shown to interfere with the induction of Th2-biased T cell responses by killing Th2 immune response inducing moDCs in a NKp30 and DNAM-1 dependent manner (70).
Interestingly, apart from its involvement in the killing of immature moDCs, engagement of NKp30 resulted in increased secretion of TNF-α and IFN-γ by NK cells, which were able to induce moDC maturation (71). Recently a study revealed that the interaction between CD155+ DCs and laquinimod-activated NK cells, which up regulated their DNAM-1 expression (recognizing CD155), led to suppression of experimental autoimmune encephalomyelitis (EAE). Interestingly, the immunoregulatory effect was not due to increased NK cell cytotoxicity against DCs, but rather due to induced reduction of HLA class II expression on DCs (72). Moreover, another group highlighted the role of the NK cell receptor NKG2D and its ligands during NK-DC interaction. They demonstrated that upon footpad injection of ectromelia virus, virus-infected murine skin-derived migratory DCs up regulated NKG2D ligands on their surface and migrated to the draining lymph nodes (dLN), where they stimulated IFNγ production by NK cells. IFNγ secretion then stimulated CXCL9 production in inflammatory monocytes resulting in an increased recruitment of circulating CXCR3+ NK cells to the dLN (73).
Interaction of NK cells and DCs was also suggested to control tissue-specific autoimmunity through an innate IFN-γ–IL-27 axis resulting in the generation if IL-10-producing Tr1-like cells (74) and to promote the tumor surveillance of other immune cells, a process described as the NK cell helper function. Activated human NK cells play a role in the induction of cDC1, which are producers of high amounts of IL-12p70. In this process, CD4+ T cells are primed to produce increased amounts of IFN-γ and less IL-4 favoring the induction of antigen-specific CD8+ T cells (75). Furthermore, mouse NK cells, recruited via CXCR3 into local lymph nodes, provide an early IFN-γ source to induce a Th1 immune response (76). In accordance with this report, only stimuli associated with the Th1 response are able to induce IFN-γ production in mouse NK cells, but not those, which are associated with the Th2 response (77).
NK-DC Interaction Within the TME
The TME is a complex network comprising T reg cells, tumor-associated macrophages (TAMs), regulatory gamma-delta cells, myeloid-derived suppressor cells (MDSCs), soluble factors, the extracellular matrix and suppressive molecules expressed on tumor cells (78).
The interaction between DCs, NK cells and CD8+ T cells within the TME is diverse (Figure 1). Here, it was shown that the maturation of DCs is depending on NK cell derived HMGB1. Further, the chemokines CCL5 and XCL1/2 mediate the recruitment of cDCs to the TME, while the NK cell derived growth factor Flt3L ensures their survival. In return, IL-15 and IL-18 released by DCs active NK cells whereas DCs derived chemokines like CXCL9 and CXCL10 recruits NK cells and CD8+ T cells into the TME. Moreover, NK cells’ cytotoxic abilities and cytokine production, especially IFN-γ, is enhanced by cDCs in a cell-contact dependent and independent way (79, 80).
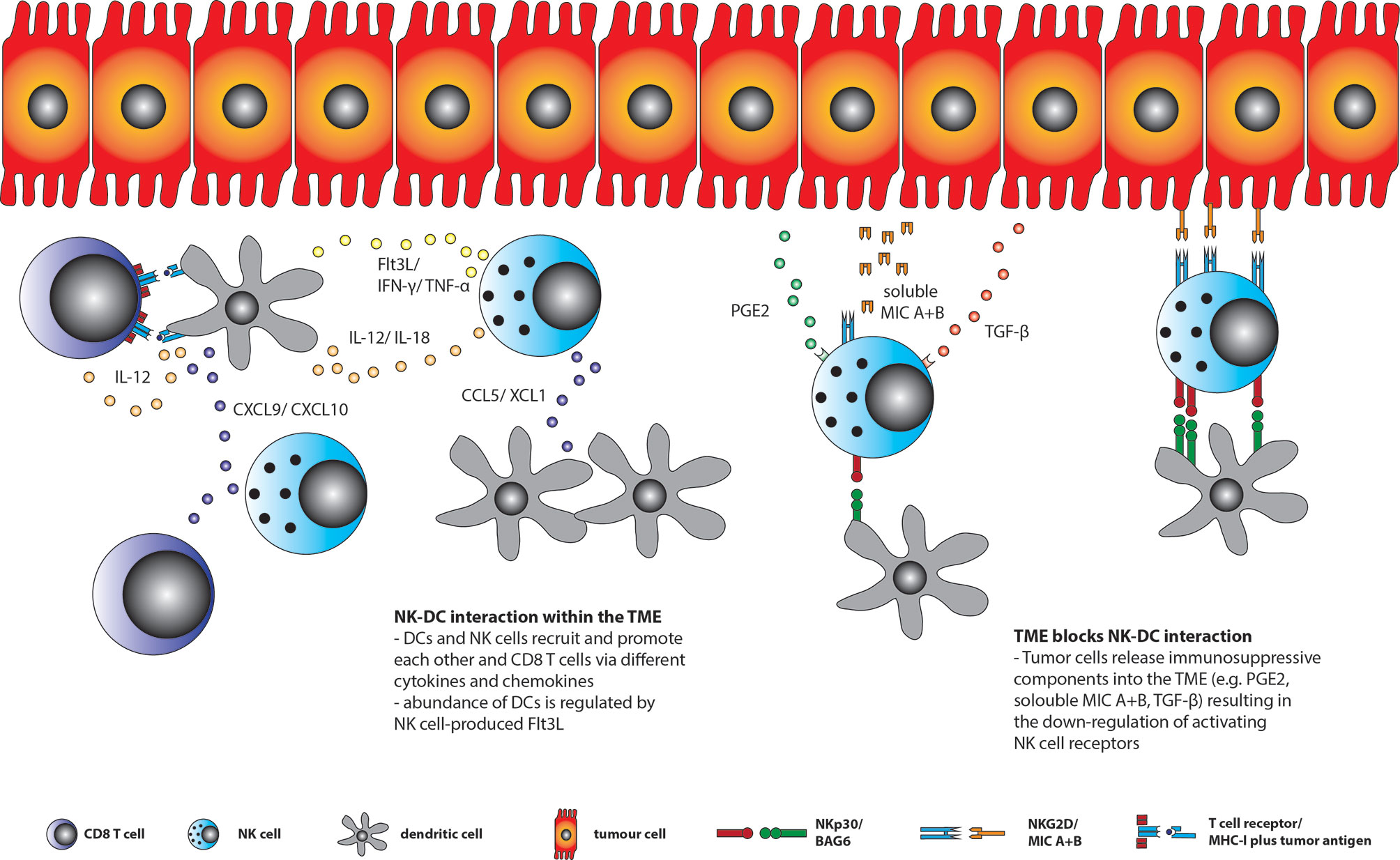
Figure 1 DC, CD8+ T and NK cell interaction within the TME. DCs, CD8+ T cells and NK cells may recruit each other into the TME by secreting various kinds of chemokines, like CCL5/XCL1 by NK cells to recruit DCs or CXCL9/10 by DCs to recruit NK and CD8+ T cells. Moreover, DCs activates CD8+ T cells via tumor antigen presentation and IL-12 production as well as TNFα and IFNγ secretion by NK cells through their own production of IL-12 and IL-18. In return, NK cell derived TNFα and IFNγ induce DC maturation. In addition, NK cells are the major source of the DC growth factor Flt3L. However, tumor cells are able to secret different compounds into the TME, like TGF-ß, soluble MIC A+B or PGE2, which are all able to down-regulate activating NK cell receptors (e.g. NKG2D and NKp30) resulting in reduced NK cell function and DC-NK cell interaction within the TME.
In a murine model, Allen et al. showed that tumor-derived CCL3 contributes to attract NK cells into the TME, leading to IFN-γ, DCs accumulation and T-cell recruitment (81). Also, in mouse, Wculek et al. demonstrated the capacity of cDCs bearing dead tumor cell antigens to highly induce CD8+ T cell response (82).
cDC1 express different chemokine receptors on their surface including the only receptor for XCL1, XCR1 (35) as well as receptors for CCL5 including CCR1 and CCR5 (83). Bottcher et al. demonstrated a reduced abundancy of cDC1, within tumors, when CCL5 and XCL1 was blocked in mice in vivo. Their data indicated that NK cell derived CCL5 and XCL1 recruits cDC1 into the TME of mouse tumors (4).
The localization of DCs within tumors is not only due to NK cell-derived chemokines but also depends on the local abundancy of growth factors like Flt3L. By depleting NK cells in tumor-bearing mice Barry et al. demonstrated that NK cell derived Flt3L regulates the amount of DCs within the tumor. These findings were further supported by the establishment of DC-NK cell conjugates within the TME (84).
It is well known that the TME induces tolerance and immunosuppression via various mechanism by dampening the functional activity of various immune cells, including NK cells and DCs (3). The TME alter the activation and cytokines production of pDCs and these pDCs are involved in tumor growth (85). Breast cancer or melanoma cells may reduce the expression of TLR or engage ILT7 (immunoglobulin like transcript 7) on pDCs due to their BST2 expression leading to reduced IFN-α productions and release. They further induce the production of immunosuppressive TGF-β, resulting in reduced expression of activating NK cell receptors, including NKp30 and NKG2D, but not NKp46. Reduced NKp30 expression decrease NK cell cytotoxicity against immature DCs, subsequently leading to the accumulation of immature immunosuppressive DCs, probably supporting tumor growth. The release of soluble NKG2D ligands from tumor cells has been described to cause reduced expression of NKG2D on NK cells, reducing NK-DC-interaction upon IFN-stimulation. Moreover, data indicated that lower NKG2D levels on NK cells not only decreased their cytotoxic activity against NKG2D ligand-expressing tumor cells but also negatively influence IFN-α-mediated NK-DC-interactions, since NKG2D ligands are up regulated upon IFN-α stimulation on DCs. In addition, PGE2 was demonstrated to reduce NKG2D and 2B4 expression on NK cells, which not only inhibits IFN-γ production and cytotoxicity, but potentially effect NK-DC-interactions as well. When DCs are matured with PGE2s they were unable to recruit NK cells and were less effective in inducing IFN-γ production in NK cells. Tumor cell induced T reg cells inhibit the IL-15Rα-expression on DCs and thereby play a role in preventing the formation of a NK-DC immuno-synapse. The release of PGE2 by tumor cells was suggested to inhibit IL-18-secretion by DCs, which was associated with a reduction of HMGB1-production by NK cells leading to reduced DC maturation (3, 86).
Discussion
As pointed out the interaction between NK cells and DCs is negatively influenced by the TME in various ways. Therefore, strategies are needed to overcome these obstacles in order to restore anti-tumor surveillance within the TME (Figure 2).
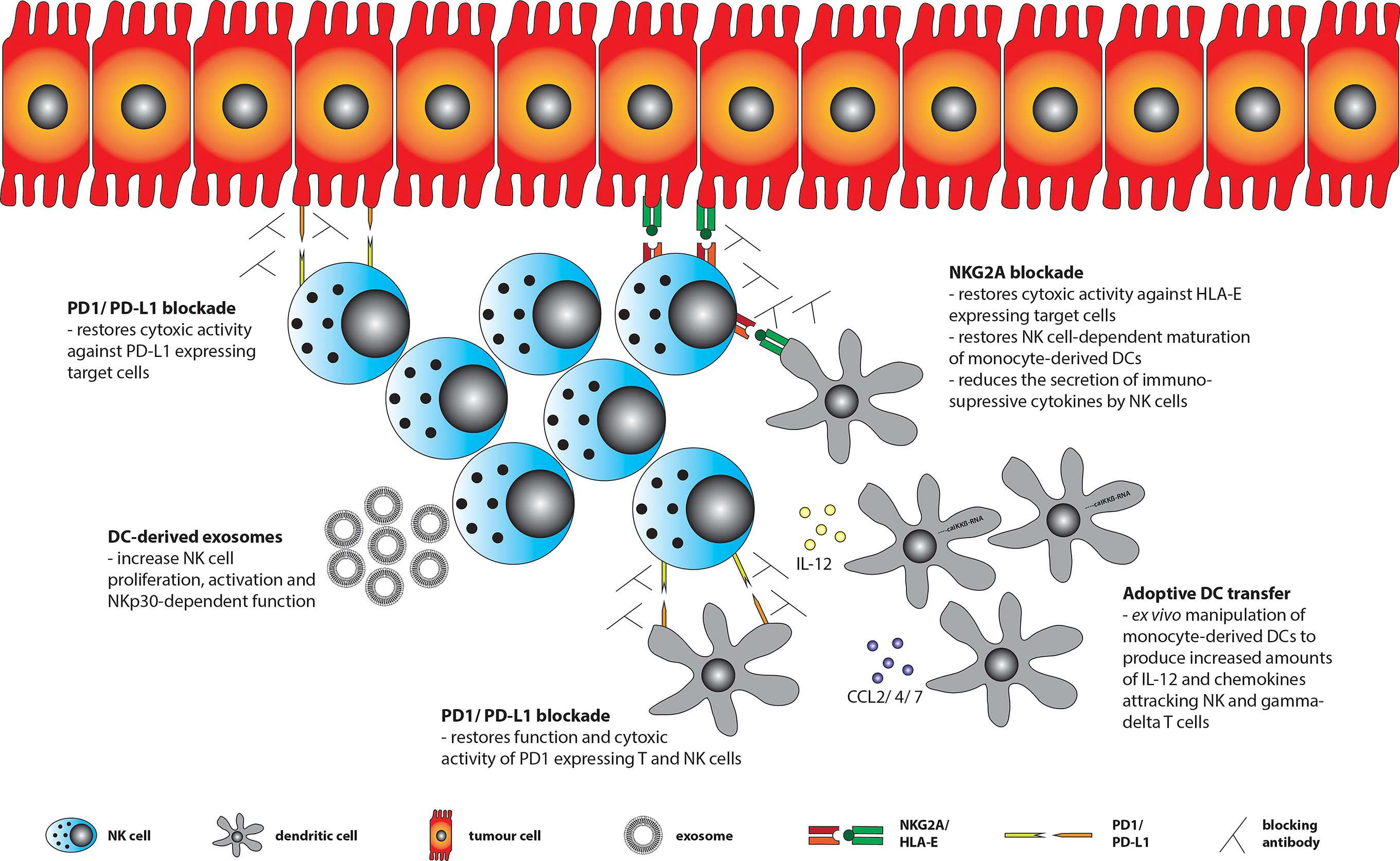
Figure 2 Treatment strategies to restore anti-tumor surveillance within the TME. Tumor cells can express ligands for various immune checkpoint (ICP) receptors including HLA-E (ligand for NKG2A) and PD-L1 (ligand for PD1), of which the latter one may also be expressed by tumor-infiltrating DCs. Using blocking antibodies against ICP receptors or their ligands restores NK cell function and DC-NK cell interaction. NK cells may further be stimulated by ex vivo generated DC-derived exosomes (Dex). In addition, adoptive transfer of ex vivo generated and engineered DCs (e.g. by transfecting them with a constitutively active IKKß (caIKKß) of the NF-kB pathway to enhance IL-12 production) could improve DC-NK cell interaction and eventually lift immunosuppression within the TME.
Optimization of DC Vaccination Products
One approach to optimize DC vaccination products might be to generate large amounts of pro-inflammatory DCs ex vivo, pulsed with tumor antigens, to lift immunosuppression within the TME. Until today various clinical trials using different DC products to treat cancer patients have been performed. Despite encouraging clinical responses in glioblastoma, pancreatic and prostate tumor patients (87–89), only sipuleucel-T has been approved by the FDA for the treatment of metastatic hormone refractory prostate cancer so far (90). This demonstrates that further improvement of the DC products is needed. For example, many trials used moDCs, which are quite convenient to be generated in vitro, but have the disadvantage, that they do not secrete IL12p70, when matured with a standard DC maturation cocktail (91, 92). This could be overcome by using mRNA electroporation to transfect moDCs with a caIKKß-RNA (constitutively active IKKß of the NF-kB pathway) enabling the transfected cells to enhance cytotoxic activity and IFN-γ production of autologous NK cells (92, 93). Another group used mRNA electroporation to engineer moDCs to produce IL-15/IL-15Rα or IFN-α leading to increased NK cell activation and cytotoxic activity against Burkitt lymphoma cells in vitro (94, 95). Increased NK cell cytotoxicity in vitro against Burkitt lymphoma cells was also achieved by co-culturing human NK cells together with TLR-activated CD1c+ myeloid and plasmacytoid DCs together instead of culturing them with only one specific DC subset alone (96). An additional approach is to optimize the DCs’ capacity to attract other immune cells, such as NK cells. Here, it would be interesting to understand the influence of IL-15 addition in the generation of ex vivo moDCs instead of IL-4. While IL-4 generated DCs secrete Th2 and T reg cell attracting chemokines such as CCL17 und CCL22, IL-15 rather induces the secretion of chemokines such as CCL2, CCL4, CCL7, CXCL9, CXCL10 and CXCL11, with CCL4 being the most important one to recruit NK and γδ T cells (97). Of note, IL-15 derived moDCs induced a stronger NK cell activation than IL-4 generated ones did (98). Moreover, IL-15 generated moDCs, that were matured with a human papilloma virus (HPV) vaccine, demonstrated a Th1-polarized cytokine profile, and increased the cytotoxicity of NK cells against cervical cancer cell lines (99).
DC-Derived Exosomes a Vaccination Product
Nevertheless, certain obstacles remain when using DC vaccination with the aim to modulate the TME. DCs are still under the influence of the immunosuppressive milieu of the TME, need chemotactic signaling to reach their destination and a high amount of work is needed to produce and properly store them. One solution to overcome these obstacles could be the use of DC-derived exosomes (so called Dex) instead of whole DCs. Exosomes are nano-sized membrane vesicles, which can be secreted by various cell types. These exosomes are not only coated with HLA and costimulatory molecules on their surface, they also contain cytosolic proteins such as heat shock proteins and in addition mRNAs and small RNAs (100, 101). DC-derived exosomes have already been used within clinical phase I/II trials (102–104). Within an initial phase 1 trial, 15 melanoma patients were treated with DC-derived exosomes from tumor-antigen pulsed moDCs (102). A subsequent analysis demonstrated that the used DC-derived exosomes were able to stimulate proliferation and activation of NK cells in vitro, due to their expression of NKG2D ligands and IL-15Rα. Moreover, DC-derived exosomes treatment increased the absolute number of NK cells in vivo and restored NKG2D expression levels as well as K562-specific cytotoxicity in 7 out of 14 melanoma patients (105). Similar results were observed during a phase II trial treating inoperable non-small cell lung cancer (NSCLC) patients with DC-derived exosomes from IFNγ-matured moDCs as maintenance therapy after induction chemotherapy. Here, an increase of NKp30-dependent NK cell function was observed, although NKp30 expression itself was not increased on patients’ NK cells. Interestingly, NKp30-dependent function was associated with longer progression-free survival and with BAG6 expression, the ligand for NKp30, on the final DC-derived exosomes product (104). Taken together, DC-derived exosomes have the potential to induce NK cell proliferation and activation in vivo and in vitro. In addition, they have the unique property to resist the immunosuppressive milieu of the TME and to easily reach various compartments without the need of chemokine-dependent recruitment, making them an interesting option to manipulate NK-DC interaction within the TME.
Blockade of Immune Checkpoint (ICP) Receptors
One of the major breakthrough in the treatment of tumor patients during the last decade was the introduction of blocking antibodies against immune checkpoint (ICP) receptors, especially against CTLA-4 and PD1, leading to long-term tumor control in melanoma, NSCLC and renal cell carcinoma patients. An important role for ICP receptors during the interaction between NK cells and DCs has been described for PD1, NKG2A and TIGIT.
PD-L1, the ligand for the ICP receptor PD1, is expressed on murine cDC1 and cDC2 (106) as well as on tumor and peripheral cDC and pDC of lung cancer patients (107). Its expression may limit the efficacy of DC vaccination trials, since PD-L1 inhibits proliferation and cytotoxic activity of PD1+ T and NK cells, respectively (108). The importance of PD1 expression on NK cells for tumor surveillance was demonstrated within the RMA-S lymphoma mouse model, which rather depends on NK cells controlling tumor growth than T cells. PD1 was strongly upregulated on NK cells within the TME and in draining lymph nodes. PD1 blockade led to a significantly reduced rate of tumor progression, which was not observed when NK cells were previously depleted. Interestingly, only a fraction of the NK cells expressed PD1. These cells were mostly activated NK cells and demonstrated higher functional activity than PD1 negative ones (109). PD1 expression on NK cells has been demonstrated as well in other tumor entities like Kaposi sarcoma (110), digestive cancers (111) and multiple myeloma (112). Using GM-CSF/IL-4 bone marrow-derived DCs (BMDCs) for vaccination in combination with pomalidomide and PD-L1 blockade, a significant tumor growth inhibition within a multiple myeloma mouse model could be achieved (113). Similar results were reported when using vaccination with GM-CSF/IL-4-derived BMDCs together with lenalidomide and PD1 blockade, which resulted in an increased functional activity of T and NK cells as well as a reduction of immunosuppressive cytokines within the TME (114). In addition, pDCs derived from the bone marrow of multiple myeloma patients expressed increased levels of PD-L1 and in vitro blockade of PD1 was able to enhance T cell proliferation and NK cell cytotoxicity during co-culture with myeloma-derived pDCs (115).
NKG2A is one of the major ICP receptors on NK cells recognizing the non-classical HLA molecule E (HLA-E) resulting in reduced cytotoxic activity against HLA-E positive target cells. MoDCs are able to upregulate NKG2A expression on NK cells in an IL12p70-dependent manner (116, 117). In addition, upon interaction with HLA-E expressing cells, NKG2A+ NK cells secreted increased amounts of IL-10 and TGF-ß resulting in reduced activation of moDCs and DC-mediated induction of CD4+CD25+ T reg cells. However, blockade of NKG2A was able to restore the activation of moDCs (118, 119). Currently, various clinical trials are testing NKG2A blockade within tumor patients. The first published and completed phase I/II trial in patients with advanced gynecologic malignancies demonstrated that the treatment was well tolerated, but only achieved a stable disease as maximal response (120). However, in patients with refractory/recurrent squamous cell carcinoma of the head and neck, the combination of an anti-NKG2A blocking antibody (monalizumab) together with an approved anti-EGFR antibody (cetuximab) demonstrated a confirmed RECIST (Response Evaluation Criteria in Solid Tumors) partial response in 8 of 26 (31%) and a stable disease in 14 of 26 (54%) patients during the interim’s analysis (121).
In addition, TIGIT is an inhibitory receptor recognizing the poliovirus receptor (PVR, CD155), such as the activating NK cell receptor DNAM1, but with a higher affinity. Importantly, binding of CD155 on moDCs by TIGIT+ T cells resulted in increased IL-10 and reduced IL-12p40 production (122). Interestingly, Flt3L-derived BMDCs derived from CD155-/- mice were more sensitive towards NK cell killing than Flt3L-derived BMDCs from WT mice indicating a potential role for the CD155-TIGIT signaling pathway during the interaction of NK cells and DCs (123). Currently, various clinical trials are testing the potential of TIGIT blockade in tumor patients.
Conclusion and Outlook
We have delineated that functions of DCs and NK cells as well as their interaction with each other are severely compromised within the TME. For this reason, various strategies to counteract immunosuppression within the TME have been discussed here. Although only PD1-PD-L1 blockade and sipuleucel-T have entered daily clinical life until today, further promising treatment options are currently being tested within clinical trials. Importantly, due to the complex and multiple facets of the TME, a combination of distinct treatment approaches will be inevitable to successfully lift immunosuppression from the TME without causing an immediate counter measurement by the tumor.
Author Contributions
BJ and EU designed and coordinated the review. BJ, EU, VGe, LH, VGr, HS, and DD wrote the manuscript. All authors agree to be accountable for the content of the work. All authors contributed to the article and approved the submitted version.
Funding
The laboratory of EU has been supported by the Frankfurt Cancer Institute FCI/DKTK (to EU), by the German Research Foundation DFG (CRC 1292, UL316/5-1), by the German Cancer Aid, the “Alfred & Angelika Gutermuth-Stiftung” and by “Menschen für Kinder e.V.”. HS was supported by the DFG (CRC 1292). DD was supported by funding from the German Research Foundation DFG (DU548/5-1, TRR305/1 project B05) and Agency national research (ANR)/German Research Foundation program (DU548/6-1) as well as intramural funds by the IZKF (A80).
Conflict of Interest
The authors declare that the research was conducted in the absence of any commercial or financial relationships that could be construed as a potential conflict of interest.
Acknowledgments
First of all, we thank all the patients who participated in the cited clinical trials. In addition, we apologize to all investigators whose scientific contribution and/or clinical trials could not be cited in this review article due to space limitations.
References
1. Amon L, Hatscher L, Heger L, Dudziak D, Lehmann CHK. Harnessing the Complete Repertoire of Conventional Dendritic Cell Functions for Cancer Immunotherapy. Pharmaceutics (2020) 12(7):1–80. doi: 10.3390/pharmaceutics12070663
2. Bodder J, Zahan T, van Slooten R, Schreibelt G, de Vries IJM, Florez-Grau G. Harnessing the Cdc1-NK Cross-Talk in the Tumor Microenvironment to Battle Cancer. Front Immunol (2020) 11:631713. doi: 10.3389/fimmu.2020.631713
3. Jacobs B, Ullrich E. The Interaction of NK Cells and Dendritic Cells in the Tumor Environment: How to Enforce NK Cell & DC Action Under Immunosuppressive Conditions? Curr Med Chem (2012) 19(12):1771–9. doi: 10.2174/092986712800099857
4. Bottcher JP, Bonavita E, Chakravarty P, Blees H, Cabeza-Cabrerizo M, Sammicheli S, et al. Nk Cells Stimulate Recruitment of cDC1 Into the Tumor Microenvironment Promoting Cancer Immune Control. Cell (2018) 172(5):1022–1037 e1014. doi: 10.1016/j.cell.2018.01.004
5. Peterson EE, Barry KC. The Natural Killer-Dendritic Cell Immune Axis in Anti-Cancer Immunity and Immunotherapy. Front Immunol (2020) 11:621254. doi: 10.3389/fimmu.2020.621254
6. Bohn T, Rapp S, Luther N, Klein M, Bruehl TJ, Kojima N, et al. Tumor Immunoevasion Via Acidosis-Dependent Induction of Regulatory Tumor-Associated Macrophages. Nat Immunol (2018) 19(12):1319–29. doi: 10.1038/s41590-018-0226-8
7. Hinshaw DC, Shevde LA. The Tumor Microenvironment Innately Modulates Cancer Progression. Cancer Res (2019) 79(18):4557–66. doi: 10.1158/0008-5472.CAN-18-3962
8. Meza Guzman LG, Keating N, Nicholson SE. Natural Killer Cells: Tumor Surveillance and Signaling. Cancers (Basel) (2020) 12(4):952. doi: 10.3390/cancers12040952
9. Terme M, Ullrich E, Delahaye NF, Chaput N, Zitvogel L. Natural Killer Cell-Directed Therapies: Moving From Unexpected Results to Successful Strategies. Nat Immunol (2008) 9(5):486–94. doi: 10.1038/ni1580
10. Ullrich E, Koch J, Cerwenka A, Steinle A. New Prospects on the NKG2D/NKG2DL System for Oncology. Oncoimmunology (2013) 2(10):e26097. doi: 10.4161/onci.26097
11. Ljunggren HG, Karre K. In Search of the ‘Missing Self’: MHC Molecules and NK Cell Recognition. Immunol Today (1990) 11(7):237–44. doi: 10.1016/0167-5699(90)90097-s
12. Reindl LM, Albinger N, Bexte T, Muller S, Hartmann J, Ullrich E. Immunotherapy With NK Cells: Recent Developments in Gene Modification Open Up New Avenues. Oncoimmunology (2020) 9(1):1777651. doi: 10.1080/2162402X.2020.1777651
13. Sivori S, Vacca P, Del Zotto G, Munari E, Mingari MC, Moretta L. Human NK Cells: Surface Receptors, Inhibitory Checkpoints, and Translational Applications. Cell Mol Immunol (2019) 16(5):430–41. doi: 10.1038/s41423-019-0206-4
14. Lanier LL. Nkg2d Receptor and Its Ligands in Host Defense. Cancer Immunol Res (2015) 3(6):575–82. doi: 10.1158/2326-6066.CIR-15-0098
15. Banchereau J, Steinman RM. Dendritic Cells and the Control of Immunity. Nature (1998) 392(6673):245–52. doi: 10.1038/32588
16. Steinman RM, Nussenzweig MC. Avoiding Horror Autotoxicus: The Importance of Dendritic Cells in Peripheral T Cell Tolerance. Proc Natl Acad Sci USA (2002) 99(1):351–8. doi: 10.1073/pnas.231606698
17. Heidkamp GF LC, Heger L, Baransk A, Hoffmann A, Lühr J, Dudziak D. Functional Specialization of Dendritic Cell Subsets. Encyclopedia Cell Biol (Elsevier) (2016) 3:588–604. doi: 10.1016/B978-0-12-394447-4.30076-1
18. Amon L, Lehmann CHK, Baranska A, Schoen J, Heger L, Dudziak D. Transcriptional Control of Dendritic Cell Development and Functions. Int Rev Cell Mol Biol (2019) 349:55–151. doi: 10.1016/bs.ircmb.2019.10.001
19. Merad M, Sathe P, Helft J, Miller J, Mortha A. The Dendritic Cell Lineage: Ontogeny and Function of Dendritic Cells and Their Subsets in the Steady State and the Inflamed Setting. Annu Rev Immunol (2013) 31:563–604. doi: 10.1146/annurev-immunol-020711-074950
20. Schaupp L, Muth S, Rogell L, Kofoed-Branzk M, Melchior F, Lienenklaus S, et al. Microbiota-Induced Type I Interferons Instruct a Poised Basal State of Dendritic Cells. Cell (2020) 181(5):1080–96.e1019. doi: 10.1016/j.cell.2020.04.022
21. Ferlazzo G, Pack M, Thomas D, Paludan C, Schmid D, Strowig T, et al. Distinct Roles of IL-12 and IL-15 in Human Natural Killer Cell Activation by Dendritic Cells From Secondary Lymphoid Organs. Proc Natl Acad Sci USA (2004) 101(47):16606–11. doi: 10.1073/pnas.0407522101
22. Piqueras B, Connolly J, Freitas H, Palucka AK, Banchereau J. Upon Viral Exposure, Myeloid and Plasmacytoid Dendritic Cells Produce 3 Waves of Distinct Chemokines to Recruit Immune Effectors. Blood (2006) 107(7):2613–8. doi: 10.1182/blood-2005-07-2965
23. Holmes TD, Wilson EB, Black EV, Benest AV, Vaz C, Tan B, et al. Licensed Human Natural Killer Cells Aid Dendritic Cell Maturation Via TNFSF14/LIGHT. Proc Natl Acad Sci USA (2014) 111(52):E5688–5696. doi: 10.1073/pnas.1411072112
24. Messlinger H, Sebald H, Heger L, Dudziak D, Bogdan C, Schleicher U. Monocyte-Derived Signals Activate Human Natural Killer Cells in Response to Leishmania Parasites. Front Immunol (2018) 9:24. doi: 10.3389/fimmu.2018.00024
25. Amon L, Lehmann CHK, Heger L, Heidkamp GF, Dudziak D. The Ontogenetic Path of Human Dendritic Cells. Mol Immunol (2020) 120:122–9. doi: 10.1016/j.molimm.2020.02.010
26. Heger L, Amon L, Lehmann CHK, Dudziak D. Systems Immunology Approaches for Understanding of Primary Dendritic Cell Subpopulations in the Past , Presence and Future. Elsevier Inc (2021) 2:501–10. doi: 10.1016/B978-0-12-801238-3.11609-5
27. Heidkamp GF, Sander J, Lehmann CHK, Heger L, Eissing N, Baranska A, et al. Human Lymphoid Organ Dendritic Cell Identity is Predominantly Dictated by Ontogeny, Not Tissue Microenvironment. Sci Immunol (2016) 1(6):1–17. doi: 10.1126/sciimmunol.aai7677
28. Villadangos JA, Young L. Antigen-Presentation Properties of Plasmacytoid Dendritic Cells. Immunity (2008) 29(3):352–61. doi: 10.1016/j.immuni.2008.09.002
29. Kaisho T. Molecular Mechanisms for Plasmacytoid Dendritic Cell Function and Development. Vaccine (2010) 28(50):8046–7. doi: 10.1016/j.vaccine.2010.09.025
30. Reizis B, Bunin A, Ghosh HS, Lewis KL, Sisirak V. Plasmacytoid Dendritic Cells: Recent Progress and Open Questions. Annu Rev Immunol (2011) 29:163–83. doi: 10.1146/annurev-immunol-031210-101345
31. Villani AC, Satija R, Reynolds G, Sarkizova S, Shekhar K, Fletcher J, et al. Single-Cell RNA-seq Reveals New Types of Human Blood Dendritic Cells, Monocytes, and Progenitors. Science (2017) 356(6335):eaah4573. doi: 10.1126/science.aah4573
32. Caminschi I, Proietto AI, Ahmet F, Kitsoulis S, Shin Teh J, Lo JC, et al. The Dendritic Cell Subtype-Restricted C-type Lectin Clec9A is a Target for Vaccine Enhancement. Blood (2008) 112(8):3264–73. doi: 10.1182/blood-2008-05-155176
33. Huysamen C, Willment JA, Dennehy KM, Brown GD. CLEC9A is a Novel Activation C-type Lectin-Like Receptor Expressed on BDCA3+ Dendritic Cells and a Subset of Monocytes. J Biol Chem (2008) 283(24):16693–701. doi: 10.1074/jbc.M709923200
34. Sancho D, Mourao-Sa D, Joffre OP, Schulz O, Rogers NC, Pennington DJ, et al. Tumor Therapy in Mice Via Antigen Targeting to a Novel, DC-restricted C-Type Lectin. J Clin Invest (2008) 118(6):2098–110. doi: 10.1172/JCI34584
35. Dorner BG, Dorner MB, Zhou X, Opitz C, Mora A, Guttler S, et al. Selective Expression of the Chemokine Receptor XCR1 on Cross-Presenting Dendritic Cells Determines Cooperation With CD8+ T Cells. Immunity (2009) 31(5):823–33. doi: 10.1016/j.immuni.2009.08.027
36. Contreras V, Urien C, Guiton R, Alexandre Y, Vu Manh TP, Andrieu T, et al. Existence of CD8alpha-like Dendritic Cells With a Conserved Functional Specialization and a Common Molecular Signature in Distant Mammalian Species. J Immunol (2010) 185(6):3313–25. doi: 10.4049/jimmunol.1000824
37. Lauterbach H, Bathke B, Gilles S, Traidl-Hoffmann C, Luber CA, Fejer G, et al. Mouse CD8alpha+ Dcs and Human BDCA3+ Dcs are Major Producers of IFN-lambda in Response to Poly IC. J Exp Med (2010) 207(12):2703–17. doi: 10.1084/jem.20092720
38. den Haan JM, Lehar SM, Bevan MJ. Cd8(+. But Not CD8(-) Dendritic Cells Cross-Prime Cytotoxic T Cells In Vivo. J Exp Med (2000) 192(12):1685–96. doi: 10.1084/jem.192.12.1685
39. Coussens LM, Zitvogel L, Palucka AK. Neutralizing Tumor-Promoting Chronic Inflammation: A Magic Bullet? Science (2013) 339(6117):286–91. doi: 10.1126/science.1232227
40. Maier B, Leader AM, Chen ST, Tung N, Chang C, LeBerichel J, et al. A Conserved Dendritic-Cell Regulatory Program Limits Antitumour Immunity. Nature (2020) 580(7802):257–62. doi: 10.1038/s41586-020-2134-y
41. Bachem A, Guttler S, Hartung E, Ebstein F, Schaefer M, Tannert A, et al. Superior Antigen Cross-Presentation and XCR1 Expression Define Human CD11c+CD141+ Cells as Homologues of Mouse CD8+ Dendritic Cells. J Exp Med (2010) 207(6):1273–81. doi: 10.1084/jem.20100348
42. Jongbloed SL, Kassianos AJ, McDonald KJ, Clark GJ, Ju X, Angel CE, et al. Human CD141+ (Bdca-3)+ Dendritic Cells (Dcs) Represent a Unique Myeloid DC Subset That Cross-Presents Necrotic Cell Antigens. J Exp Med (2010) 207(6):1247–60. doi: 10.1084/jem.20092140
43. Poulin LF, Salio M, Griessinger E, Anjos-Afonso F, Craciun L, Chen JL, et al. Characterization of Human DNGR-1+ BDCA3+ Leukocytes as Putative Equivalents of Mouse CD8alpha+ Dendritic Cells. J Exp Med (2010) 207(6):1261–71. doi: 10.1084/jem.20092618
44. Segura E, Durand M, Amigorena S. Similar Antigen Cross-Presentation Capacity and Phagocytic Functions in All Freshly Isolated Human Lymphoid Organ-Resident Dendritic Cells. J Exp Med (2013) 210(5):1035–47. doi: 10.1084/jem.20121103
45. Heger L, Balk S, Luhr JJ, Heidkamp GF, Lehmann CHK, Hatscher L, et al. Clec10a Is a Specific Marker for Human Cd1c(+) Dendritic Cells and Enhances Their Toll-Like Receptor 7/8-Induced Cytokine Secretion. Front Immunol (2018) 9:744. doi: 10.3389/fimmu.2018.00744
46. Heger L, Hofer TP, Bigley V, de Vries IJM, Dalod M, Dudziak D, et al. Subsets of CD1c(+) Dcs: Dendritic Cell Versus Monocyte Lineage. Front Immunol (2020) 11:559166. doi: 10.3389/fimmu.2020.559166
47. Brown CC, Gudjonson H, Pritykin Y, Deep D, Lavallee VP, Mendoza A, et al. Transcriptional Basis of Mouse and Human Dendritic Cell Heterogeneity. Cell (2019) 179(4):846–63.e824. doi: 10.1016/j.cell.2019.09.035
48. Dutertre CA, Becht E, Irac SE, Khalilnezhad A, Narang V, Khalilnezhad S, et al. Single-Cell Analysis of Human Mononuclear Phagocytes Reveals Subset-Defining Markers and Identifies Circulating Inflammatory Dendritic Cells. Immunity (2019) 51(3):573–589 e578. doi: 10.1016/j.immuni.2019.08.008
49. Bourdely P, Anselmi G, Vaivode K, Ramos RN, Missolo-Koussou Y, Hidalgo S, et al. Transcriptional and Functional Analysis of CD1c(+) Human Dendritic Cells Identifies a CD163(+) Subset Priming Cd8(+)Cd103(+) T Cells. Immunity (2020) 53(2):335–352 e338. doi: 10.1016/j.immuni.2020.06.002
50. Gao Y, Nish SA, Jiang R, Hou L, Licona-Limon P, Weinstein JS, et al. Control of T Helper 2 Responses by Transcription Factor IRF4-dependent Dendritic Cells. Immunity (2013) 39(4):722–32. doi: 10.1016/j.immuni.2013.08.028
51. Persson EK, Uronen-Hansson H, Semmrich M, Rivollier A, Hagerbrand K, Marsal J, et al. IRF4 Transcription-Factor-Dependent CD103(+)CD11b(+) Dendritic Cells Drive Mucosal T Helper 17 Cell Differentiation. Immunity (2013) 38(5):958–69. doi: 10.1016/j.immuni.2013.03.009
52. Schlitzer A, McGovern N, Teo P, Zelante T, Atarashi K, Low D, et al. IRF4 Transcription Factor-Dependent CD11b+ Dendritic Cells in Human and Mouse Control Mucosal IL-17 Cytokine Responses. Immunity (2013) 38(5):970–83. doi: 10.1016/j.immuni.2013.04.011
53. Yamazaki S, Dudziak D, Heidkamp GF, Fiorese C, Bonito AJ, Inaba K, et al. Cd8+ CD205+ Splenic Dendritic Cells are Specialized to Induce Foxp3+ Regulatory T Cells. J Immunol (2008) 181(10):6923–33. doi: 10.4049/jimmunol.181.10.6923
54. Fernandez NC, Lozier A, Flament C, Ricciardi-Castagnoli P, Bellet D, Suter M, et al. Dendritic Cells Directly Trigger NK Cell Functions: Cross-Talk Relevant in Innate Anti-Tumor Immune Responses In Vivo. Nat Med (1999) 5(4):405–11. doi: 10.1038/7403
55. Gerosa F, Baldani-Guerra B, Nisii C, Marchesini V, Carra G, Trinchieri G. Reciprocal Activating Interaction Between Natural Killer Cells and Dendritic Cells. J Exp Med (2002) 195(3):327–33. doi: 10.1084/jem.20010938
56. Piccioli D, Sbrana S, Melandri E, Valiante NM. Contact-Dependent Stimulation and Inhibition of Dendritic Cells by Natural Killer Cells. J Exp Med (2002) 195(3):335–41. doi: 10.1084/jem.20010934
57. Borg C, Jalil A, Laderach D, Maruyama K, Wakasugi H, Charrier S, et al. NK Cell Activation by Dendritic Cells (Dcs) Requires the Formation of a Synapse Leading to IL-12 Polarization in Dcs. Blood (2004) 104(10):3267–75. doi: 10.1182/blood-2004-01-0380
58. Ziblat A, Nunez SY, Raffo Iraolagoitia XL, Spallanzani RG, Torres NI, Sierra JM, et al. Interleukin (IL)-23 Stimulates IFN-Gamma Secretion by CD56(bright) Natural Killer Cells and Enhances Il-18-Driven Dendritic Cells Activation. Front Immunol (2017) 8:1959. doi: 10.3389/fimmu.2017.01959
59. Ziblat A, Domaica CI, Spallanzani RG, Iraolagoitia XL, Rossi LE, Avila DE, et al. Il-27 Stimulates Human NK-cell Effector Functions and Primes NK Cells for IL-18 Responsiveness. Eur J Immunol (2015) 45(1):192–202. doi: 10.1002/eji.201444699
60. Puttur F, Francozo M, Solmaz G, Bueno C, Lindenberg M, Gohmert M, et al. Conventional Dendritic Cells Confer Protection Against Mouse Cytomegalovirus Infection Via TLR9 and MyD88 Signaling. Cell Rep (2016) 17(4):1113–27. doi: 10.1016/j.celrep.2016.09.055
61. Della Chiesa M, Romagnani C, Thiel A, Moretta L, Moretta A. Multidirectional Interactions are Bridging Human NK Cells With Plasmacytoid and Monocyte-Derived Dendritic Cells During Innate Immune Responses. Blood (2006) 108(12):3851–8. doi: 10.1182/blood-2006-02-004028
62. Lucas M, Schachterle W, Oberle K, Aichele P, Diefenbach A. Dendritic Cells Prime Natural Killer Cells by Trans-Presenting Interleukin 15. Immunity (2007) 26(4):503–17. doi: 10.1016/j.immuni.2007.03.006
63. Luu TT, Ganesan S, Wagner AK, Sarhan D, Meinke S, Garbi N, et al. Independent Control of Natural Killer Cell Responsiveness and Homeostasis At Steady-State by CD11c+ Dendritic Cells. Sci Rep (2016) 6:37996. doi: 10.1038/srep37996
64. Gorvel L, Korenfeld D, Tung T, Klechevsky E. Dendritic Cell-Derived IL-32alpha: A Novel Inhibitory Cytokine of NK Cell Function. J Immunol (2017) 199(4):1290–300. doi: 10.4049/jimmunol.1601477
65. Nicolai CJ, Wolf N, Chang IC, Kirn G, Marcus A, Ndubaku CO, et al. NK Cells Mediate Clearance of CD8(+) T Cell-Resistant Tumors in Response to STING Agonists. Sci Immunol (2020) 5(45):eaaz2738. doi: 10.1126/sciimmunol.aaz2738
66. Finch DK, Stolberg VR, Ferguson J, Alikaj H, Kady MR, Richmond BW, et al. Lung Dendritic Cells Drive Natural Killer Cytotoxicity in Chronic Obstructive Pulmonary Disease Via IL-15Ralpha. Am J Respir Crit Care Med (2018) 198(9):1140–50. doi: 10.1164/rccm.201712-2513OC
67. Ferlazzo G, Tsang ML, Moretta L, Melioli G, Steinman RM, Munz C. Human Dendritic Cells Activate Resting Natural Killer (NK) Cells and are Recognized Via the NKp30 Receptor by Activated NK Cells. J Exp Med (2002) 195(3):343–51. doi: 10.1084/jem.20011149
68. Pende D, Castriconi R, Romagnani P, Spaggiari GM, Marcenaro S, Dondero A, et al. Expression of the DNAM-1 Ligands, Nectin-2 (CD112) and Poliovirus Receptor (CD155), on Dendritic Cells: Relevance for Natural Killer-Dendritic Cell Interaction. Blood (2006) 107(5):2030–6. doi: 10.1182/blood-2005-07-2696
69. Della Chiesa M, Vitale M, Carlomagno S, Ferlazzo G, Moretta L, Moretta A. The Natural Killer Cell-Mediated Killing of Autologous Dendritic Cells is Confined to a Cell Subset Expressing CD94/NKG2A, But Lacking Inhibitory Killer Ig-like Receptors. Eur J Immunol (2003) 33(6):1657–66. doi: 10.1002/eji.200323986
70. Walwyn-Brown K, Guldevall K, Saeed M, Pende D, Onfelt B, MacDonald AS, et al. Human NK Cells Lyse Th2-Polarizing Dendritic Cells Via NKp30 and DNAM-1. J Immunol (2018) 201(7):2028–41. doi: 10.4049/jimmunol.1800475
71. Vitale M, Della Chiesa M, Carlomagno S, Pende D, Arico M, Moretta L, et al. NK-Dependent DC Maturation is Mediated by TNFalpha and IFNgamma Released Upon Engagement of the NKp30 Triggering Receptor. Blood (2005) 106(2):566–71. doi: 10.1182/blood-2004-10-4035
72. Ott M, Avendano-Guzman E, Ullrich E, Dreyer C, Strauss J, Harden M, et al. Laquinimod, a Prototypic quinoline-3-carboxamide and Aryl Hydrocarbon Receptor Agonist, Utilizes a CD155-mediated Natural Killer/Dendritic Cell Interaction to Suppress CNS Autoimmunity. J Neuroinflamm (2019) 16(1):49. doi: 10.1186/s12974-019-1437-0
73. Wong E, Xu RH, Rubio D, Lev A, Stotesbury C, Fang M, et al. Migratory Dendritic Cells, Group 1 Innate Lymphoid Cells, and Inflammatory Monocytes Collaborate to Recruit Nk Cells to the Virus-Infected Lymph Node. Cell Rep (2018) 24(1):142–54. doi: 10.1016/j.celrep.2018.06.004
74. Chong WP, van Panhuys N, Chen J, Silver PB, Jittayasothorn Y, Mattapallil MJ, et al. Nk-DC Crosstalk Controls the Autopathogenic Th17 Response Through an Innate IFN-gamma-IL-27 Axis. J Exp Med (2015) 212(10):1739–52. doi: 10.1084/jem.20141678
75. Mailliard RB, Son YI, Redlinger R, Coates PT, Giermasz A, Morel PA, et al. Dendritic Cells Mediate NK Cell Help for Th1 and CTL Responses: Two-Signal Requirement for the Induction of NK Cell Helper Function. J Immunol (2003) 171(5):2366–73. doi: 10.4049/jimmunol.171.5.2366
76. Martin-Fontecha A, Thomsen LL, Brett S, Gerard C, Lipp M, Lanzavecchia A, et al. Induced Recruitment of NK Cells to Lymph Nodes Provides IFN-gamma for T(H)1 Priming. Nat Immunol (2004) 5(12):1260–5. doi: 10.1038/ni1138
77. Zanoni I, Foti M, Ricciardi-Castagnoli P, Granucci F. TLR-Dependent Activation Stimuli Associated With Th1 Responses Confer NK Cell Stimulatory Capacity to Mouse Dendritic Cells. J Immunol (2005) 175(1):286–92. doi: 10.4049/jimmunol.175.1.286
78. Zhang C, Liu Y. Targeting NK Cell Checkpoint Receptors or Molecules for Cancer Immunotherapy. Front Immunol (2020) 11:1295. doi: 10.3389/fimmu.2020.01295
79. Fu C, Jiang A. Dendritic Cells and CD8 T Cell Immunity in Tumor Microenvironment. Front Immunol (2018) 9:3059. doi: 10.3389/fimmu.2018.03059
80. Lucarini V, Melaiu O, Tempora P, D’Amico S, Locatelli F, Fruci D. Dendritic Cells: Behind the Scenes of T-Cell Infiltration Into the Tumor Microenvironment. Cancers (Basel) (2021) 13(3):433. doi: 10.3390/cancers13030433
81. Allen F, Bobanga ID, Rauhe P, Barkauskas D, Teich N, Tong C, et al. CCL3 Augments Tumor Rejection and Enhances CD8(+) T Cell Infiltration Through NK and CD103(+) Dendritic Cell Recruitment Via Ifngamma. Oncoimmunology (2018) 7(3):e1393598. doi: 10.1080/2162402X.2017.1393598
82. Wculek SK, Amores-Iniesta J, Conde-Garrosa R, Khouili SC, Melero I, Sancho D. Effective Cancer Immunotherapy by Natural Mouse Conventional Type-1 Dendritic Cells Bearing Dead Tumor Antigen. J Immunother Cancer (2019) 7(1):100. doi: 10.1186/s40425-019-0565-5
83. McColl SR. Chemokines and Dendritic Cells: A Crucial Alliance. Immunol Cell Biol (2002) 80(5):489–96. doi: 10.1046/j.1440-1711.2002.01113.x
84. Barry KC, Hsu J, Broz ML, Cueto FJ, Binnewies M, Combes AJ, et al. A Natural Killer-Dendritic Cell Axis Defines Checkpoint Therapy-Responsive Tumor Microenvironments. Nat Med (2018) 24(8):1178–91. doi: 10.1038/s41591-018-0085-8
85. Terra M, Oberkampf M, Fayolle C, Rosenbaum P, Guillerey C, Dadaglio G, et al. Tumor-Derived TGFbeta Alters the Ability of Plasmacytoid Dendritic Cells to Respond to Innate Immune Signaling. Cancer Res (2018) 78(11):3014–26. doi: 10.1158/0008-5472.CAN-17-2719
86. Van Elssen CH, Vanderlocht J, Oth T, Senden-Gijsbers BL, Germeraad WT, Bos GM. Inflammation-Restraining Effects of Prostaglandin E2 on Natural Killer-Dendritic Cell (NK-DC) Interaction are Imprinted During DC Maturation. Blood (2011) 118(9):2473–82. doi: 10.1182/blood-2010-09-307835
87. Jiang N, Qiao G, Wang X, Morse MA, Gwin WR, Zhou L, et al. Dendritic Cell/Cytokine-Induced Killer Cell Immunotherapy Combined With S-1 in Patients With Advanced Pancreatic Cancer: A Prospective Study. Clin Cancer Res (2017) 23(17):5066–73. doi: 10.1158/1078-0432.CCR-17-0492
88. Fucikova J, Podrazil M, Jarolim L, Bilkova P, Hensler M, Becht E, et al. Phase I/II Trial of Dendritic Cell-Based Active Cellular Immunotherapy With DCVAC/PCa in Patients With Rising PSA After Primary Prostatectomy or Salvage Radiotherapy for the Treatment of Prostate Cancer. Cancer Immunol Immunother (2018) 67(1):89–100. doi: 10.1007/s00262-017-2068-x
89. Liau LM, Ashkan K, Tran DD, Campian JL, Trusheim JE, Cobbs CS, et al. Correction to: First Results on Survival From a Large Phase 3 Clinical Trial of an Autologous Dendritic Cell Vaccine in Newly Diagnosed Glioblastoma. J Transl Med (2018) 16(1):179. doi: 10.1186/s12967-018-1552-1
90. Higano CS, Schellhammer PF, Small EJ, Burch PA, Nemunaitis J, Yuh L, et al. Integrated Data From 2 Randomized, Double-Blind, Placebo-Controlled, Phase 3 Trials of Active Cellular Immunotherapy With Sipuleucel-T in Advanced Prostate Cancer. Cancer (2009) 115(16):3670–9. doi: 10.1002/cncr.24429
91. Kalinski P, Hilkens CM, Snijders A, Snijdewint FG, Kapsenberg ML. Il-12-deficient Dendritic Cells, Generated in the Presence of Prostaglandin E2, Promote Type 2 Cytokine Production in Maturing Human Naive T Helper Cells. J Immunol (1997) 159(1):28–35.
92. Pfeiffer IA, Hoyer S, Gerer KF, Voll RE, Knippertz I, Guckel E, et al. Triggering of NF-kappaB in Cytokine-Matured Human DCs Generates Superior DCs for T-cell Priming in Cancer Immunotherapy. Eur J Immunol (2014) 44(11):3413–28. doi: 10.1002/eji.201344417
93. Bosch NC, Voll RE, Voskens CJ, Gross S, Seliger B, Schuler G, et al. NF-Kappab Activation Triggers NK-cell Stimulation by Monocyte-Derived Dendritic Cells. Ther Adv Med Oncol (2019) 11:1758835919891622. doi: 10.1177/1758835919891622
94. Van den Bergh J, Willemen Y, Lion E, Van Acker H, De Reu H, Anguille S, et al. Transpresentation of interleukin-15 by IL-15/IL-15Ralpha mRNA-engineered Human Dendritic Cells Boosts Antitumoral Natural Killer Cell Activity. Oncotarget (2015) 6(42):44123–33. doi: 10.18632/oncotarget.6536
95. Willemen Y, Van den Bergh JM, Lion E, Anguille S, Roelandts VA, Van Acker HH, et al. Engineering Monocyte-Derived Dendritic Cells to Secrete Interferon-Alpha Enhances Their Ability to Promote Adaptive and Innate Anti-Tumor Immune Effector Functions. Cancer Immunol Immunother (2015) 64(7):831–42. doi: 10.1007/s00262-015-1688-2
96. van Beek JJ, Gorris MA, Skold AE, Hatipoglu I, Van Acker HH, Smits EL, et al. Human Blood Myeloid and Plasmacytoid Dendritic Cells Cross Activate Each Other and Synergize in Inducing NK Cell Cytotoxicity. Oncoimmunology (2016) 5(10):e1227902. doi: 10.1080/2162402X.2016.1227902
97. Van Acker HH, Beretta O, Anguille S, De Caluwe L, Papagna A, Van den Bergh JM, et al. Desirable Cytolytic Immune Effector Cell Recruitment by interleukin-15 Dendritic Cells. Oncotarget (2017) 8(8):13652–65. doi: 10.18632/oncotarget.14622
98. Anguille S, Van Acker HH, Van den Bergh J, Willemen Y, Goossens H, Van Tendeloo VF, et al. Interleukin-15 Dendritic Cells Harness Nk Cell Cytotoxic Effector Function in a Contact- and IL-15-Dependent Manner. PloS One (2015) 10(5):e0123340. doi: 10.1371/journal.pone.0123340
99. Van den Bergh JM, Guerti K, Willemen Y, Lion E, Cools N, Goossens H, et al. HPV Vaccine Stimulates Cytotoxic Activity of Killer Dendritic Cells and Natural Killer Cells Against HPV-positive Tumour Cells. J Cell Mol Med (2014) 18(7):1372–80. doi: 10.1111/jcmm.12284
100. Pitt JM, Andre F, Amigorena S, Soria JC, Eggermont A, Kroemer G, et al. Dendritic Cell-Derived Exosomes for Cancer Therapy. J Clin Invest (2016) 126(4):1224–32. doi: 10.1172/JCI81137
101. Pitt JM, Kroemer G, Zitvogel L. Extracellular Vesicles: Masters of Intercellular Communication and Potential Clinical Interventions. J Clin Invest (2016) 126(4):1139–43. doi: 10.1172/JCI87316
102. Escudier B, Dorval T, Chaput N, Andre F, Caby MP, Novault S, et al. Vaccination of Metastatic Melanoma Patients With Autologous Dendritic Cell (DC) Derived-Exosomes: Results of Thefirst Phase I Clinical Trial. J Transl Med (2005) 3(1):10. doi: 10.1186/1479-5876-3-10
103. Morse MA, Garst J, Osada T, Khan S, Hobeika A, Clay TM, et al. A Phase I Study of Dexosome Immunotherapy in Patients With Advanced non-Small Cell Lung Cancer. J Transl Med (2005) 3(1):9. doi: 10.1186/1479-5876-3-9
104. Besse B, Charrier M, Lapierre V, Dansin E, Lantz O, Planchard D, et al. Dendritic Cell-Derived Exosomes as Maintenance Immunotherapy After First Line Chemotherapy in NSCLC. Oncoimmunology (2016) 5(4):e1071008. doi: 10.1080/2162402X.2015.1071008
105. Viaud S, Terme M, Flament C, Taieb J, Andre F, Novault S, et al. Dendritic Cell-Derived Exosomes Promote Natural Killer Cell Activation and Proliferation: A Role for NKG2D Ligands and IL-15Ralpha. PloS One (2009) 4(3):e4942. doi: 10.1371/journal.pone.0004942
106. Peng Q, Qiu X, Zhang Z, Zhang S, Zhang Y, Liang Y, et al. Pd-L1 on Dendritic Cells Attenuates T Cell Activation and Regulates Response to Immune Checkpoint Blockade. Nat Commun (2020) 11(1):4835. doi: 10.1038/s41467-020-18570-x
107. Mayoux M, Roller A, Pulko V, Sammicheli S, Chen S, Sum E, et al. Dendritic Cells Dictate Responses to PD-L1 Blockade Cancer Immunotherapy. Sci Transl Med (2020) 12(534):eaav7431. doi: 10.1126/scitranslmed.aav7431
108. Martinez VG, Hidalgo L, Valencia J, Hernandez-Lopez C, Entrena A, del Amo BG, et al. Autocrine Activation of Canonical BMP Signaling Regulates PD-L1 and PD-L2 Expression in Human Dendritic Cells. Eur J Immunol (2014) 44(4):1031–8. doi: 10.1002/eji.201343693
109. Hsu J, Hodgins JJ, Marathe M, Nicolai CJ, Bourgeois-Daigneault MC, Trevino TN, et al. Contribution of NK Cells to Immunotherapy Mediated by PD-1/PD-L1 Blockade. J Clin Invest (2018) 128(10):4654–68. doi: 10.1172/JCI99317
110. Beldi-Ferchiou A, Lambert M, Dogniaux S, Vely F, Vivier E, Olive D, et al. PD-1 Mediates Functional Exhaustion of Activated NK Cells in Patients With Kaposi Sarcoma. Oncotarget (2016) 7(45):72961–77. doi: 10.18632/oncotarget.12150
111. Liu Y, Cheng Y, Xu Y, Wang Z, Du X, Li C, et al. Increased Expression of Programmed Cell Death Protein 1 on NK Cells Inhibits NK-cell-mediated Anti-Tumor Function and Indicates Poor Prognosis in Digestive Cancers. Oncogene (2017) 36(44):6143–53. doi: 10.1038/onc.2017.209
112. Benson DM Jr., Bakan CE, Mishra A, Hofmeister CC, Efebera Y, Becknell B, et al. The PD-1/PD-L1 Axis Modulates the Natural Killer Cell Versus Multiple Myeloma Effect: A Therapeutic Target for CT-011, a Novel Monoclonal anti-PD-1 Antibody. Blood (2010) 116(13):2286–94. doi: 10.1182/blood-2010-02-271874
113. Chu TH, Vo MC, Park HS, Lakshmi TJ, Jung SH, Kim HJ, et al. Potent Anti-Myeloma Efficacy of Dendritic Cell Therapy in Combination With Pomalidomide and Programmed Death-Ligand 1 Blockade in a Preclinical Model of Multiple Myeloma. Cancer Immunol Immunother (2021) 70(1):31–45. doi: 10.1007/s00262-020-02654-0
114. Vo MC, Jung SH, Chu TH, Lee HJ, Lakshmi TJ, Park HS, et al. Lenalidomide and Programmed Death-1 Blockade Synergistically Enhances the Effects of Dendritic Cell Vaccination in a Model of Murine Myeloma. Front Immunol (2018) 9:1370. doi: 10.3389/fimmu.2018.01370
115. Ray A, Das DS, Song Y, Richardson P, Munshi NC, Chauhan D, et al. Targeting PD1-PDL1 Immune Checkpoint in Plasmacytoid Dendritic Cell Interactions With T Cells, Natural Killer Cells and Multiple Myeloma Cells. Leukemia (2015) 29(6):1441–4. doi: 10.1038/leu.2015.11
116. Curran SA, Romano E, Kennedy MG, Hsu KC, Young JW. Phenotypic and Functional Activation of Hyporesponsive KIRnegNKG2Aneg Human NK-cell Precursors Requires IL12p70 Provided by Poly(I:C)-matured Monocyte-Derived Dendritic Cells. Cancer Immunol Res (2014) 2(10):1000–10. doi: 10.1158/2326-6066.CIR-14-0054-T
117. Tognarelli S, Wirsching S, von Metzler I, Rais B, Jacobs B, Serve H, et al. Enhancing the Activation and Releasing the Brakes: A Double Hit Strategy to Improve Nk Cell Cytotoxicity Against Multiple Myeloma. Front Immunol (2018) 9:2743. doi: 10.3389/fimmu.2018.02743
118. Jinushi M, Takehara T, Tatsumi T, Kanto T, Miyagi T, Suzuki T, et al. Negative Regulation of NK Cell Activities by Inhibitory Receptor CD94/NKG2A Leads to Altered NK Cell-Induced Modulation of Dendritic Cell Functions in Chronic Hepatitis C Virus Infection. J Immunol (2004) 173(10):6072–81. doi: 10.4049/jimmunol.173.10.6072
119. Jinushi M, Takehara T, Tatsumi T, Yamaguchi S, Sakamori R, Hiramatsu N, et al. Natural Killer Cell and Hepatic Cell Interaction Via NKG2A Leads to Dendritic Cell-Mediated Induction of CD4 Cd25 T Cells With PD-1-dependent Regulatory Activities. Immunology (2007) 120(1):73–82. doi: 10.1111/j.1365-2567.2006.02479.x
120. Tinker AV, Hirte HW, Provencher D, Butler M, Ritter H, Tu D, et al. Dose-Ranging and Cohort-Expansion Study of Monalizumab (IPH2201) in Patients With Advanced Gynecologic Malignancies: A Trial of the Canadian Cancer Trials Group (Cctg): Ind221. Clin Cancer Res (2019) 25(20):6052–60. doi: 10.1158/1078-0432.CCR-19-0298
121. Andre P, Denis C, Soulas C, Bourbon-Caillet C, Lopez J, Arnoux T, et al. Anti-NKG2A Mab Is a Checkpoint Inhibitor That Promotes Anti-Tumor Immunity by Unleashing Both T and NK Cells. Cell (2018) 175(7):1731–43.e1713. doi: 10.1016/j.cell.2018.10.014
122. Yu X, Harden K, Gonzalez LC, Francesco M, Chiang E, Irving B, et al. The Surface Protein TIGIT Suppresses T Cell Activation by Promoting the Generation of Mature Immunoregulatory Dendritic Cells. Nat Immunol (2009) 10(1):48–57. doi: 10.1038/ni.1674
Keywords: NK cells, dendritic cells, DC-NK cell interaction, tumor microenvironment, cellular therapies
Citation: Jacobs B, Gebel V, Heger L, Grèze V, Schild H, Dudziak D and Ullrich E (2021) Characterization and Manipulation of the Crosstalk Between Dendritic and Natural Killer Cells Within the Tumor Microenvironment. Front. Immunol. 12:670540. doi: 10.3389/fimmu.2021.670540
Received: 21 February 2021; Accepted: 19 April 2021;
Published: 14 May 2021.
Edited by:
Erik Wennerberg, Institute of Cancer Research (ICR), United KingdomReviewed by:
Evelien Smits, University of Antwerp, BelgiumJurjen Tel, Eindhoven University of Technology, Netherlands
Copyright © 2021 Jacobs, Gebel, Heger, Grèze, Schild, Dudziak and Ullrich. This is an open-access article distributed under the terms of the Creative Commons Attribution License (CC BY). The use, distribution or reproduction in other forums is permitted, provided the original author(s) and the copyright owner(s) are credited and that the original publication in this journal is cited, in accordance with accepted academic practice. No use, distribution or reproduction is permitted which does not comply with these terms.
*Correspondence: Benedikt Jacobs, QmVuZWRpa3QuSmFjb2JzQHVrLWVybGFuZ2VuLmRl; Evelyn Ullrich, RXZlbHluLlVsbHJpY2hAa2d1LmRl