- 1Basic Medical Research Centre, Medical School, Nantong University, Nantong, China
- 2Nantong-Leicester Joint Institute of Kidney Science, Nephrology, Affiliated Hospital of Nantong University, Nantong, China
- 3Department of Cardiovascular Sciences, College of Life Sciences, University of Leicester, Leicester, United Kingdom
Acute kidney injury (AKI) is a health problem worldwide, but there is a lack of early diagnostic biomarkers and target-specific treatments. Ischemia-reperfusion (IR), a major cause of AKI, not only induces kidney injury, but also stimulates the self-defense system including innate immune responses to limit injury. One of these responses is the production of erythropoietin (EPO) by adjacent normal tissue, which is simultaneously triggered, but behind the action of its receptors, either by the homodimer EPO receptor (EPOR)2 mainly involved in erythropoiesis or the heterodimer EPOR/β common receptor (EPOR/βcR) which has a broad range of biological protections. EPOR/βcR is expressed in several cell types including tubular epithelial cells at low levels or absent in normal kidneys, but is swiftly upregulated by hypoxia and inflammation and also translocated to cellular membrane post IR. EPOR/βcR mediates anti-apoptosis, anti-inflammation, pro-regeneration, and remodeling via the PI3K/Akt, STAT3, and MAPK signaling pathways in AKI. However, the precise roles of EPOR/βcR in the pathogenesis and progression of AKI have not been well defined, and its potential as an earlier biomarker for AKI diagnosis and monitoring repair or chronic progression requires further investigation. Here, we review biological functions and mechanistic signaling pathways of EPOR/βcR in AKI, and discuss its potential clinical applications as a biomarker for effective diagnosis and predicting prognosis, as well as directing cell target drug delivery.
Introduction
Acute kidney injury (AKI) is a critical syndrome characterized by a sudden decline of renal function, with high morbidity and mortality (1–3). The poor prognosis of AKI is also evidenced by the high risk of progression to chronic kidney disease and end-stage renal disease over time, characterized by tubulointerstitial fibrosis (4, 5). Effective management of AKI is urgently needed including early diagnosis as well as target-specific treatment.
Renal ischemia-reperfusion (IR) injury is a common contributor to AKI, which could result from various clinical settings such as kidney transplantation, cardiac surgery, shock, vomiting, diarrhea, and burns (6). Parallel to injury, the kidney also develops defensive responses to restrict cellular damage and promote repair, one of which is associated with the classical hormone erythropoietin (EPO). Recombinant EPO, 5000 U/L in hemoperfusate, markedly decreased apoptotic cells in tubular areas, but increased the apoptotic cell death of inflammatory cells and their clearance in isolated porcine kidneys (n = 6) (7). In vivo, EPO at 1000 U/kg significantly improved renal function and structure at the acute stage (24 h) of IR-induced AKI in rats (n = 6) (8). These observations suggest a therapeutic opportunity of using EPO to improve the outcome of AKI. However, a large dose of EPO is needed for tissue protection, so it often results in side effects such as hypertension and thrombosis due to its high affinity to the classical homodimer (EPOR)2. The renoprotective receptor of EPO was a complex composed of the EPO receptor and β common receptor (EPOR/βcR), also known as innate repair receptor (9), but EPO has a low affinity to EPOR/βcR. Therefore, EPO-derived helix B surface peptide (HBSP) or its cyclic form CHBP was designed, which recognizes only EPOR/βcR, but not (EPOR)2, thus dissociating tissue protection and erythropoiesis (10).
Our previous work demonstrated the renoprotective effects of HBSP and CHBP in a series study using both animal and cellular models. It was shown that the expression of EPOR/βcR was greatly triggered by IR injury in kidneys, and in particular located in tubular epithelial cells (TECs) (11), while its ligand HBSP or CHBP protected the kidney against IR-induced AKI, as well as cyclosporine A-induced fibrotic damage in kidneys (12). Mechanically, HBSP or CHBP was found to regulate the activation of transient receptor potential melastatin 7 ion channels and endoplasmic reticulum stress in tubular epithelia (13, 14). Exploring the role of EPOR/βcR in renal IR-induced AKI could help researchers to better understand its self-defense mechanisms, its signaling pathways and associated outcomes, as well as assessing its role as a potential biomarker to facilitate timely diagnosis, monitor the progression of kidney injury, and direct cell target drug delivery.
EPO and its Derivatives
EPO is a highly-glycosylated protein with a molecular weight of around 30.4 kDa (15). In adults, interstitial fibroblasts in the kidneys are the major cells of EPO production, which maintain the level of EPO in the circulation by feedback signals (16). The main function of EPO is enabling the terminal differentiation of erythroid progenitor cells by inhibiting apoptosis and activating pro-survival signaling pathways (17–19). However, the action of EPO is not restricted to the hematopoietic system. Initial research observed a neuroprotective effect of recombinant EPO against ischemia-induced neuron damage in the brain (20). A similar effect was also described in other organs including the kidney, heart, and liver against ischemia-related injuries, by improving autophagy, anti-apoptosis, and anti-inflammation (21–23). It indicates that these organs have a receptor of EPO, which mediates the protection of tissues. Accumulated evidence suggests that heterodimer EPOR/βcR is responsible for the tissue protective effects (24–26). Upon injury, the expression of EPOR/βcR is instantly elevated, but EPO production in the site of injury is usually postponed, for example in astrocytes of the brain (27). Thus, there is a therapeutic window for exogenous supplement of EPO and its derivatives (9). Due to the much lower binding affinity of EPO to (1-20 nM) EPOR/βcR than to classic homodimer (EPOR)2 (100-200 pM) (28), a significantly higher dose of EPO is required to induce cytoprotection compared to erythropoiesis. Nevertheless, a high dose of EPO showed no significant tissue-protective effect in clinical trials, including four trails of renal transplantation (29–32) and one trail of AKI (33). Additionally, a number of studies indicated a high risk of mortality and adverse events related to the cardiovascular system by raising hematocrit and enhancing the activation of platelets and endothelia after EPO administration (34–37). Therefore, the application of EPO is restricted due to significant risk compromising its benefit. Looking into the 3D structure of EPO, helices A, C, and D binding with (EPOR)2 are associated with erythropoiesis, but only aqueous helix B binding with EPOR/βcR is related to tissue protection. Therefore, EPO was modified in a variety of ways to generate molecules retaining tissue protection and avoiding hematopoiesis, which included desialated EPO, carbamylated EPO (CEPO), and glutaraldehyde EPO. However, these EPO derivatives have different pitfalls including either short half-life, immune stimulation, or tissue permeability and compatibility (38–42).
Nevertheless, a small peptide derived from EPO, helix B surface peptide (HBSP), was designed and synthesized. It is a linear peptide formed by 11 amino acids, derived from the exterior aqueous surface of helix B, also known as ARA290 (10). HBSP possesses specific and powerful roles in tissue protection but without the side effect of erythropoiesis (43). Unfortunately, the plasma half-life of HBSP is short, around 2 min, which might affect its function in vivo. Scientists from the Chinese Academy of Sciences optimized the metabolic stabilization of HBSP in plasma by the conformational constraining of the head-to-tail connection that forms cyclic HBSP (CHBP) via thioether. CHBP gained proteolytic resistance significantly and prolonged its half-life time to at least 300 min in vivo (44). Preclinical studies proved that CHBP was renoprotective against IR injury in rodents (45) and large animal porcine models (3). The clinical application of CHBP, in particular HBSP, is promising as it is easily obtained, is an ambient dry powder for transportation, has low cost, and potential high efficacy for organ protection (45). However, metabolic characteristics such as serum stability, safety, tissue permeability, and cytoprotective efficacy in humans still need to be further investigated.
The effectiveness of a ligand drug is not only dependent on its specificity and tissue permeability, but also relies on the responsiveness of the receptor type. EPOR was reported to be associated with chronic fibrosis of the kidney by receiving persistent signals from EPO or excessive expression (46, 47). It was also reported that recombinant EPO provided renoprotection against fibrosis in adenine-induced CKD (48). However, EPO protected the IR kidney at the acute stage but promoted renal fibrosis in the long term. It is necessary to note that recombinant human EPO greatly increased myofibroblast numbers and collagen deposition in kidneys at 28 days post IR (46). The same study revealed that EPO stimulated profibrotic transforming growth factor β, oxidative stress and phosphorylation of ERK in IR kidneys, and also promoted epithelial-to-mesenchymal transition and activated fibroblasts under oxidative stress in vitro. Through overexpression of EPOR in tubules, evidence also demonstrated that higher EPO/EPOR signaling caused fibrosis in the IR-injured kidney at 14 days post IR by suppressing basal autophagy activity and upregulating apoptotic events through modifying microtubule-associated protein 1A/1B-light chain 3 and active caspase-3 expression, respectively (47). This evidence indicates that the homodimer (EPOR)2 could contribute to the fibrotic development of IR kidneys, as the heterodimer EPOR/βcR was demonstrated to have an anti-fibrotic role in the kidney after IR through its specific ligands such as CHBP (45). Thus, an advantage of EPO derivatives is that they only specifically bind to EPOR/βcR, avoiding the side effects of EPO caused by not only erythropoiesis but also pro-fibrosis. Understanding the expression, regulation, and function of (EPOR)2 and EPOR/βcR under different diseases would be beneficial to increase the effectiveness of their respective ligand drugs, reduce side effects, and avoid invalid use.
Physical Interaction Between EPOR and βCR
The protective role of EPO revealed in different organs indicates that a variety of non-hematopoietic tissues have the receptor of EPO. EPOR, a member of the type I cytokine receptor family, is present on the surface of erythroid progenitor cells at a high level, and assembly of the homodimer (EPOR)2 mediates erythropoiesis upon receiving signals from EPO (49). During the developmental stages of embryos, EPOR was found broadly expressed in many organs and cells, such as the kidney, brain, and heart (50–52). It appeared predominantly in the kidney after the first two trimesters. Knockout of EPOR in a mouse fetus resulted in death of the mouse, which may be partially due to erythropoiesis and the development of organs including the kidney (53). Thus, the signaling of EPO/EPOR is crucial in the normal development of mouse fetuses, showing its potentially broader roles apart from erythropoiesis. However, EPOR expression was gradually decreased in the kidney cortex and became stable until the maturation of the kidney postnatally (54). In adult rats, EPOR expression in kidneys remains very low or absent under normal conditions, mainly in TECs (55). This expressional change of EPOR indicates its multiple functions that may be involved in the different periods of life.
Alike EPOR, βcR also belongs to the type I cytokine receptor family. βcR is a shared common β chain with a receptor of interleukin (IL)-3, IL-5, and granulocyte-macrophage colony stimulating factor (GMCSF), which binds to a corresponding α chain (56–58). βcR mainly locates on the surface of hematopoietic cells and exerts immune regulation (59). In 1995, βcR was found to be responsive to EPO as the tyrosine phosphorylation of βcR was observed upon EPO treatment to cultured cells (60). Paul and his colleagues (61) transfected B lymphocytes with vectors expressing both EPOR and βcR genes and then obtained their heterologous expression in these cells. The two receptors associated with each other as EPOR/βcR were demonstrated in transfected cells by the detection of co-immunoprecipitation. In 2004, Brines and his colleagues demonstrated that EPOR and βcR were physically linked via cysteine residues (24), which might be already assembled in the absence of the EPO molecule (61). Co-expression of EPOR and βcR has been detected in various organs, such as the kidney, heart, and nervous system (62, 63).
Signaling Pathways Mediated By EPOR/βCR
EPOR and βcR do not contain an intrinsic kinase domain for downstream signaling but could induce Janus kinase 2 (JAK2) auto-phosphorylation by spatial conformational change upon receptor occupancy (60, 64, 65). As EPO signals go through both homodimer and heteromer receptors, molecular pathways specifically associated with EPOR/βcR were demonstrated by non-erythropoietic tissue-protective compounds, primarily HBSP and CEPO (42). Three dominant pathways followed by JAK2 phosphorylation have been identified, which were similar to those used in the hematopoietic system followed by homodimer receptor (EPOR)2 binding (Figure 1).
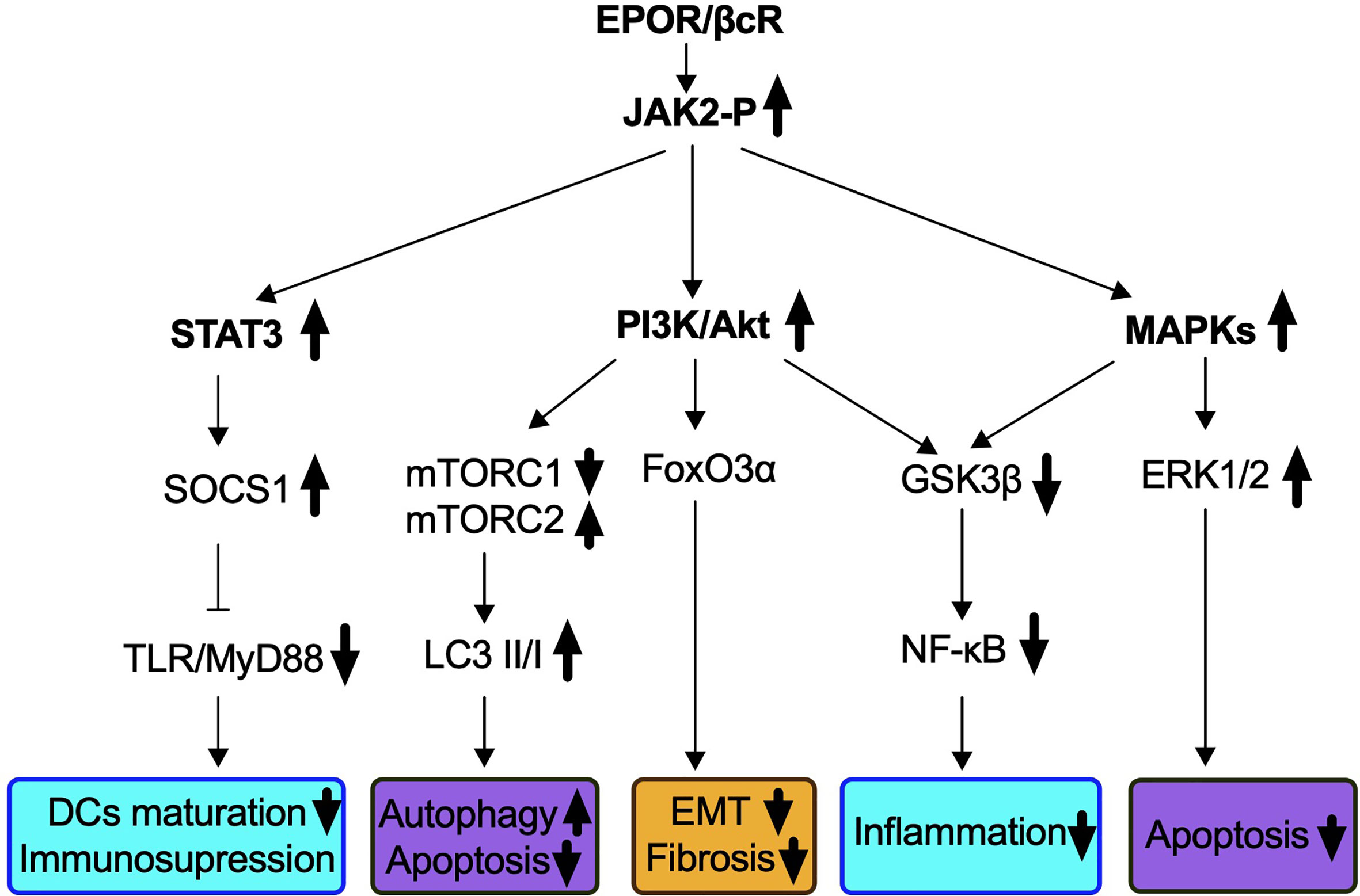
Figure 1 Signaling pathways identified in IR-induced AKI via EPOR/βcR activation. EPO-derived tissue protective components such as CEPO, HBSP, and CHBP lead to the phosphorylation of EPOR/βcR-linked JAK2, and subsequently activates several downstream cascades. Biological processes that were influenced through EPOR/βcR signaling included immune response (blue box), cell death (purple box), and kidney repair/preventing renal fibrosis (yellow box).
Firstly, the phosphatidylinositol 3-kinase (PI3K)/Akt (also known as protein kinase B) pathway was involved through EPOR/βcR in the kidney, liver, and heart (66–70). In a mouse renal IR model, autophagy was induced by CHBP via inhibition of mammalian target of rapamycin complex 1 (mTORC1), but activation of mTORC2 led to renoprotective effects (44). Forkhead box O 3α (FoxO3α), a downstream effector of Akt, was activated by CHBP and linked to anti-fibrotic effect in a kidney IR model (45). Nevertheless, upon CEPO treatment, FoxO3α was dephosphorylated and consequently stimulated p27 expression, which was a key factor responsible for the negative regulation of cell cycle and cell proliferation demonstrated in human acute myeloid leukemia cell line UT-7 (71).
The second major molecular pathway involves signal transducer and activator of transcription 3 (STAT3) signaling. Phosphorylation of STAT3 in murine kidneys was found upregulated by IR insult, along with decreased apoptotic events (44). The activation of the STAT3 pathway was reported to occur in a cardiovascular system insulted by IR by the blockade of apoptosis and inflammation (68, 69, 72). In addition, in a rat renal allograft model, JAK2/STAT3 signaling induced the downstream pathway of the suppressor of cytokine signaling 1 expression for inhibiting toll-like receptor-induced dendritic cell maturation, as well as pro-inflammatory cytokine production of these cells (73). Most recently, the same group discovered that the JAK2/STAT3 pathway was also essential to enhance the immunosuppressive capability of myeloid-derived suppressor cells (74).
A third pathway refers to mitogen-activated protein kinases (MAPKs) identified in the heart, liver as well as the kidney via EPOR/βcR signaling (67–69). Extracellular signal-regulated kinase (ERK)1/2, a downstream pathway of MAPK, plays an important role in the protection of EPO from IR-induced renal apoptosis (75). Activation of ERK signals also accelerates repair of TECs and inhibits progression of interstitial fibrosis, and its correlation with EPOR/βcR needs investigation (76).
Additionally, both the PI3K and MAPK pathways inhibit glycogen synthase kinase 3β (GSK3β), which leads to stabilization of the mitochondrion, reduces oxidative stress damage, and subsequently inhibits death signals (72, 77). HBSP enhanced the phosphorylation of GSK3β in the rat kidney post IR and ultimately reduced inflammation by inhibiting nuclear factor-κB (NF-κB) (39). Moreover, an oxidative pathway including NADH-ubiquinone oxidoreductase Fe-S protein 6, alpha-aminoadipic semialdehyde synthase, and ATP-binding cassette subfamily D member 3 was also elicited by EPOR/βcR, leading to the renoprotective effect of CHBP (78).
Renoprotection Mediated By EPOR/βCR
The signaling pathways of EPOR/βcR bring multiple favorable effects on IR-injured kidney, not only against the injury, but also promotes repair. Upon IR insult, kidney cells were stressed with energy exploitation, waste retention, and accumulation of reactive oxygen species, then underwent sublethal, lethal damage, resulting in the release of alarm signals and evoking a sterile inflammatory reaction (7). Although the kidney has the capability to self-renew, maladaptive repair characterized as the deposition of collagen in tubulointerstitial areas cannot be avoided completely (79). As expected, the instant injury at an early stage of IR injury was greatly reversed by EPO and its derivatives through ameliorating kidney apoptosis and inflammatory response. Moreover, early intervention of EPO derivatives seems to turn on the switch of long-lasting biological actions as it can also inhibit chronic renal fibrosis. It was thought that EPOR/βcR mediated the balance of the microenvironment at injury sites, which benefited the recovery of the injured organ. However, the exact mechanism is still not very clear. The cell types in the kidney possessing EPOR/βcR were summarized (Figure 2), with detailed discussion below.
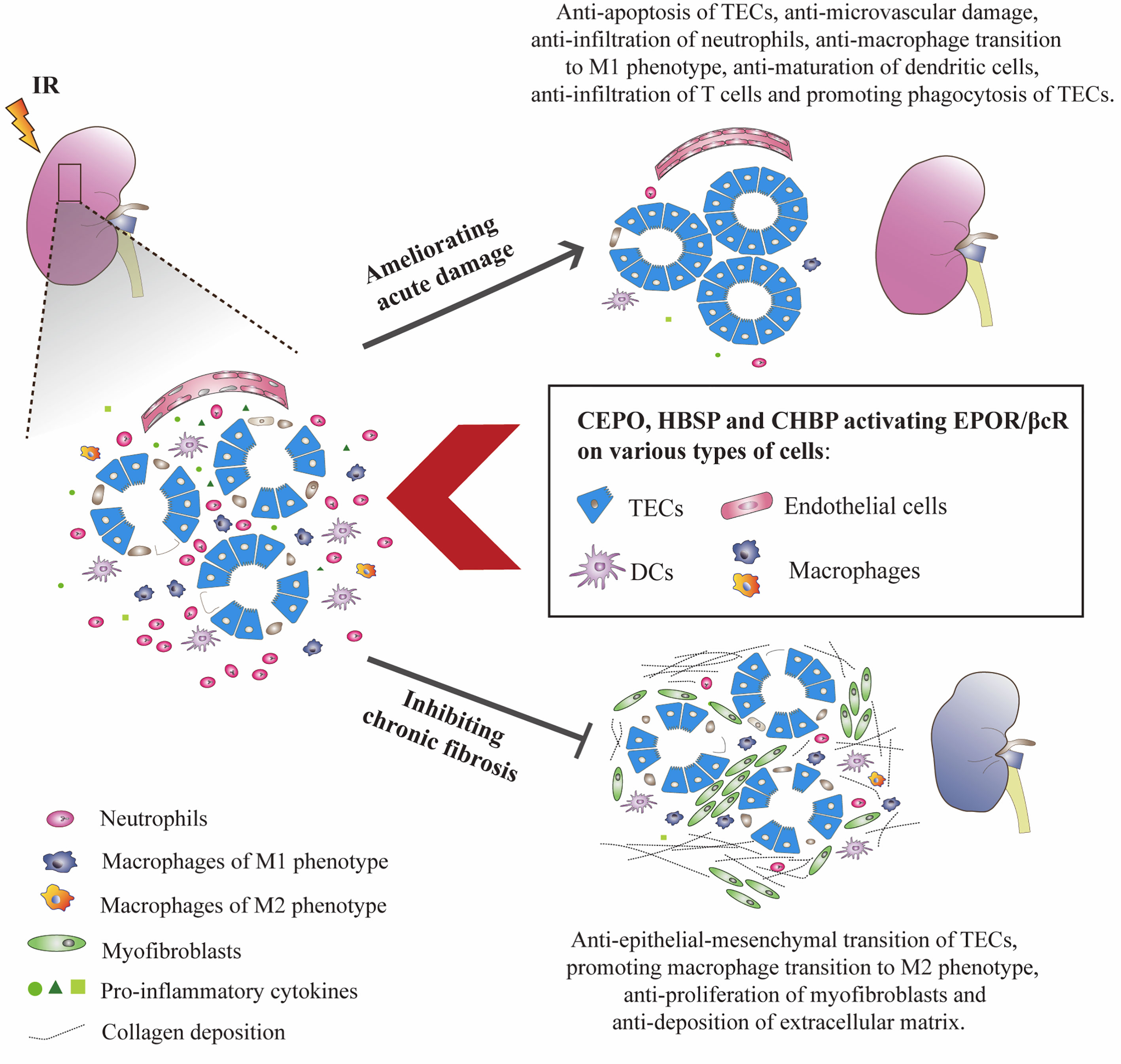
Figure 2 EPO derivatives with tissue protective features benefited IR-injured kidneys at both acute and chronic stages. CEPO, HBSP and CHBP occupied EPOR/βcR on identified cells, reduced the apoptotic death of TECs, and the infiltration, pro-inflammatory transformation and maturation of inflammatory cells at the acute injury stage. The potential role of EPOR/βcR on the phagocytic function of TECs needs further study. These EPO derivatives also promoted the proliferation of TECs and transformation of macrophages to the M2 phenotype (anti-inflammatory) and reversed the proliferation of myofibroblasts and deposition of extracellular matrix proteins including collagen in interstitial areas at the chronic repair stage.
Ameliorating Apoptotic Cell Death
Injury of renal TECs was the most predominant pathological change of renal IR injury. These cells demand a high amount of oxygen and ATP, and thus are susceptible to ischemic insult. Severe stress to TECs could lead the cells to a variety of cell death modes including apoptosis (80). In both rodent and large animal experiments, inhibiting apoptotic pathways demonstrated substantial benefits to the recovery of IR kidneys (81, 82). Anti-apoptotic effect through EPOR/βcR in IR-induced AKI was also evidenced in animal models (3, 39, 83). The underlying mechanism could be manifested by the instant expression of EPOR/βcR on kidney TECs, which helps to construct an image that HBSP directly binds to EPOR/βcR on these cells and transmits protective signals inhibiting apoptosis (11). Evidence also suggested that the anti-apoptotic effect of EPOR/βcR activation would also contribute to the reduced level of oxidative (78) and enhanced autophagy via inhibition of mTORC1 and activation of mTORC2 (44), which rescues the TECs from apoptotic cell death. In addition, sustained dysfunction of the endothelium post reperfusion results in the obstruction of capillaries and a local ‘no-reflow’ phenomenon, which could lead to continuous hypoxia and cumulative apoptosis (84). Sautina et al. defined the association of vascular endothelial growth factor receptor 2 with EPOR/βcR to elicit downstream signals in the endothelium and attenuated microvascular damage (85, 86). It is indicated that EPOR/βcR would also mediate the maintenance of microvasculature and reduce further damage to tubules.
Regulating Immune Response
Neutrophils, Macrophages, Dendritic Cells, and T Cells
Inflammatory cells accumulated at the site of damage are usually a double-edged sword. These cells cause further damage at the early stage of AKI, and also initiate the clearance of injured cells and inflammation to limit damage and promote tissue repair. However, sustained inflammatory cell infiltration leads to uncontrolled inflammation in the kidney, further tissue injury, and maladaptive repair (87). Others and our previous work demonstrated that HBSP reduced infiltration of neutrophils and production of pro-inflammatory cytokines such as IL-1, IL-6, and tumor necrosis factor α by inhibiting the NF-κB pathway in rodent renal IR injury models (39, 44). Furthermore, in a large animal model, CHBP protected isolated porcine kidney and restored renal function by reducing interstitial neutrophils and decreasing pro-inflammatory cytokine transcripts 3 h after reperfusion (3). It has been found that EPOR/βcR presented or functioned in several types of immune cells, such as macrophages and dendritic cells (25). HBSP inhibited the transformation of macrophages to the pro-inflammatory M1 phenotype (88) but markedly increased the ratio of the anti-inflammatory M2 phenotype (89), of which the latter supports the transition from tubule injury to tubule repair in IR kidneys (90). In addition, CHBP also inhibited the maturation of dendritic cells through the JAK2/STAT3 signaling pathway (73), suggesting the expression of EPOR/βcR on dendritic cells. This effect of CHBP also suppressed the infiltration of T cells, which would reduce tissue damage caused by excessive immune activation (91). The expressional and functional profile of EPOR/βcR on dendritic cells, antigen-presenting cells, and T cells should be further defined, which may influence the outcome of diseases.
Phagocytosis of TECs
Additionally, timely clearance of damaged cells also benefits the injured kidney from secondary inflammatory injury (92). Bangwei Luo et al. reported that apoptotic cell-released sphingosine 1-phosphate activated EPO/EPOR signaling in macrophages, which enhanced dying cell clearance (93). However, whether the phagocytic function of macrophages was enhanced through EPOR homodimer or hetero-receptor EPOR/βcR upon EPO binding remains unclear. Nevertheless, surviving TECs are the main (semi-professional) phagocytes to uptake dead cells in the kidneys subjected to IR injury by expressing kidney injury molecule-1 (KIM-1) (94). KIM-1, a specific molecule expressed by TECs upon injury, recognizes phosphatidylserine on apoptotic cells and transforms TECs to phagocytes (94). The mechanism of EPOR/βcR ameliorating IR injury in kidneys may also relate to the phagocytic function of TECs and facilitate clearance of apoptotic cells. Using a bioinformatics method, STAT3 was identified as an upstream regulator of KIM-1 expression (95), which could also be activated via EPOR/βcR. It is suggesting that ligands to EPOR/βcR might trigger signals for TECs turning into phagocytes.
Promoting Regeneration/Remodeling
The kidney could recover from IR injury that resulted in substantial loss of TECs (96). Nevertheless, kidney repair is often maladaptive when the injury is severe or mild but frequent (97). The sources of cells that contribute to the replenishment of the population of TECs after injury mainly originate from endogenous surviving TECs (98). Many injury factors, especially long-term hypoxia resulted from sustained loss of peritubular microvessels (99), and disturbance of immune-respondent components such as chronic activation of macrophages of the M1 phenotype (100, 101) have been suggested to contribute to post-ischemic fibrosis. These factors may then induce epigenetic changes in resident myofibroblasts, which result in prolonged fibroblast activation and fibrogenesis (102). Imbalanced regeneration of renal parenchymal cells and repair were characterized by interstitial fibrosis that might be the key determiner for the fate of the injured kidney in terms of its long-term outcome.
The activation of EPOR/βcR was observed to decrease the expression of α-smooth muscle actin (α-SMA), a specific marker for myofibroblasts, and deposition of the extracellular matrix in rodent IR injury and unilateral ureteral obstruction models (44, 83). EPOR/βcR signaling also compromised the pro-fibrotic effect of transforming growth factor-β in cultured TECs by maintaining the level of E-cadherin and lowering vimentin, attenuating their epithelial-mesenchymal transition in vitro (3, 45). This action would contribute to the reduced expression of pro-fibrotic FoxO3α on TECs through EPOR/βcR signaling. In addition, CEPO, which specifically recognizes EPOR/βcR, decreased α-SMA expression, a marker of myofibroblasts, in a 14-day rat AKI model induced by unilateral ureteral obstruction (103). These findings suggest that signals after EPOR/βcR activation would block the way of fibrogenesis by modifying the behavior of both parenchymal cells and interstitial cells, however, the exact mechanism needs further investigation. Evidence has shown that EPOR/βcR mediated the proliferation of endothelial cells and neural progenitor cells (26, 104). However, whether EPOR/βcR mediates the dedifferentiation and proliferation of surviving TECs is unknown. Moreover, communication among cells is critical to kidney repair, such as crosstalk between TECs and myofibroblasts, pericytes, or macrophages, which would lead to regeneration of kidney parenchymal cells or the bulk production of the extracellular matrix. Investigation of EPOR/βcR on cell-to-cell communication would not only lead to further understanding of its pro-repair and anti-fibrotic action but also benefit the determination of the pathogenesis of renal IR injury.
EPOR/βCR as Potential Biomarker
Regulation and Modulation of EPOR/βcR
On a genomic level, the upstream regulation of EPOR expression has been studied in various studies involving the kidney. Hypoxia inducible factor-1α (HIF-α) is a major transcription factor mediating the cellular response to hypoxia and plays a pivotal role in the resolution of AKI (105). Exogenous HIF-α improved the survival of rat AKI induced by IR insult, as well as increased the downstream effector EPOR, indicating the association between EPOR and HIF-α (106). In addition, overexpression of transmembrane Klotho or administration of secreted Klotho exerted protective effects against IR-induced AKI (107, 108). Hu and colleagues reported that EPOR was a downstream effector of Klotho (55, 109). It was also suggested that tumor necrotic factor α and its receptor were required for the upregulation of EPOR and the therapeutic effects of EPO (110, 111). Furthermore, Youn-Soo Lee et al. found that deficiency of the Von Hippel-Lindau gene resulted in the development of tumors in the kidney, characterized by consistent upregulation of EPO and EPOR in renal carcinoma (112). Thus, it indicated that Von Hippel-Lindau might be an upstream inhibitor of EPO or EPOR, of which the latter genes are responsible for the proliferation of immature mesenchymal cells (113). Besides, EPO induces the ubiquitination and internalization of EPOR via clathrin-mediated endocytosis, following by the degradation of its intracellular part by the proteasome, preventing further signal transduction (114, 115). Nevertheless, the regulation of βcR expression in kidney parenchymal cells at the genomic level remains unreported, for which studies might be limited by the lack of specific ligands for (βcR)2. The potential effect of upstream genes on EPOR regulation and EPOR/βcR formation for enhancing protection needs to be further explored.
Both EPOR and βcR belong to the type I cytokine superfamily, of which one family member usually creates a complex with the other in the same family as stimulated by the ligand. In addition, EPOR could be translocated from the nucleus to the cytoplasm and cellular membrane under hypoxia (116). In addition, it was claimed that EPOR could be recruited into membrane lipid raft fractions within 1 min post the exposure of the growth factor, reaching a peak at 10 min (117). There is also evidence regarding the βcR migration to lipid rafts under stimulation of the ligand GMCSF in hematopoietic cells (118). Similarly, the altered cell surface expression of βcR in fibroblasts and macrophages that immediately occurred under stress indicated the swift translocation of the receptor (119). It was assumed that homodimer (EPOR)2 and (βcR)2 translocated from the cytoplasm to the plasma membrane quickly upon stimulation and assembled a heteroreceptor at the molecular level. Additionally, the regulation of heteroreceptor EPOR/βcR expression has been also shown by its ligand treatment in different disease models. CEPO promoted the complex association of EPOR and βcR on myocardium at 4 h post-reperfusion in a mouse myocardial IR injury model (70). However, the level of EPOR/βcR was decreased by HBSP in a 48-h mouse renal IR model, which may contribute to the decreased degree of kidney injury (11). These regulatory observations indicated potential differences in the underlying mechanisms of EPOR/βcR expression.
Potential Diagnostic Biomarker
Up to date, the diagnosis for AKI in the clinic mainly relies on the change of serum creatinine level and/or urine output (120). However, the functional alteration of the kidney always results from massive structural disorder, so the supreme timing for targeted therapeutics is often missed, which is crucial for the long-term outcome. Minor damage in the kidney might result in the poor prognosis without timely diagnosis and intervention (97). An ideal biomarker is one that can diagnose AKI at the early stage, monitor the development of the injury, direct the therapeutic interventions, and predict the prognosis of disease (33). The instant expression of EPOR/βcR in the kidney upon renal stress or insult suggested that EPOR, βcR, and/or EPOR/βcR might be a potential early biomarker(s) for IR-induced AKI. However, kidney biopsy is not a routine examination for AKI patients. Dropped off damage parenchymal cells and/or apoptotic inflammatory cells to the tubular lumen provided an opportunity for detecting these receptors in the urine, which might also reflect the level of these receptors in the kidney and the occurrence of renal injury. However, there is no available report regarding EPOR/βcR in plasma and urine so far, which may be due to the challenge of EPOR/βcR detection. Nevertheless, EPOR was found as a soluble protein in plasma that corresponds to the extracellular domain of EPOR on the cellular membrane. Biologically, the soluble EPOR exists in human plasma to bind excessive EPO and maintains the latter at a constant level (121). It has been reported that the level of soluble EPOR may contribute to erythropoietin resistance in end stage renal disease, and that its production may be mediated by pro-inflammatory cytokines such as TNF-α and IL-6 (122), indicating soluble EPOR as a potential biomarker of cell injury. However, the regulation of soluble EPOR in the plasma of animals or humans with IR-injured kidneys remains unclear. Understanding the expression and regulation of EPOR in different forms, as well as EPOR/βcR could benefit its potential application in AKI diagnosis and progression.
Therefore, exploration of the dynamic expressional profile of EPOR and potentially EPOR/βcR in the serum and urine post renal IR at different stages of injury would provide essential evidence to assess the diagnostic potential of these receptors. The association between the expression of these receptors and the development of renal IR injury might give information on the monitoring of disease. Early intervention using EPO derivatives that specifically activate EPOR/βcR will be beneficial for not only the short-term but also long-term outcome of IR-induced AKI. The dynamic change of EPOR/βcR in the kidney post insults such as IR at the initiation and the development of injury, as well as repair stage, might direct the application of specific ligand drugs.
Challenges and Opportunities
Apart from the availability of the clinical specimens, the sensitivity and specificity of detection for the level of EPO and its receptors including EPOR, βcR, and EPOR/βcR in liquid specimens should be optimized. Especially for the heterocomplex, a co-immunoprecipitation assay needs a large sample with adequate expression. Whether EPOR or βcR protein, as well as the mRNA level of these receptors, could represent the level of heteroreceptor remains unclear and requires further study. Additionally, the complexity of disease may compromise the sensitivity of detection as various diseases may increase the expression of this protective receptor especially in patients with problems in other organs except the kidney or possessing complex kidney problems. Besides, there is also crosstalk between the kidney and other organs such as the brain, liver, and lung post renal IR (123). The level of EPOR, βcR, or EPOR/βcR in urine might not reflect the exact injury in the kidney, making the assessment of renal injury difficult. However, the functions, underlining mechanisms, and diagnostic potential regarding EPOR/βcR in AKI would also be applicable to other organs with acute injury. There are clinical trials using HBSP (also named ARA290) on neural systems (NCT02070783, NCT02039687) and the endocrine system (NCT01933529) indicating that HBSP possesses a wide range and great potential for clinical applications in different organ systems. EPOR/βcR has been found widely expressed in many kinds of cells including progenitor cells. The potential side effect of in vivo administration of EPOR/βcR ligands such as HBSP/CHBP in the clinic shall consider the potential that the repair signal of the receptor might lead to excessive proliferation of progenitor cells, resulting in tumorigenesis or fibrosis. In addition, EPOR/βcR might also be a natural guider for specific cell target drug delivery such as small interfering RNA conjugated with its ligand HBSP or CHBP, as TECs are most vulnerable to IR injury and highly expressed EPOR/βcR.
Conclusion
The timely and spatial expression of EPOR/βcR in the injured kidney upon IR suggests its potential for early diagnosis and monitoring progression, as well as cell target drug delivering such as siRNA conjugated to its ligand. The key facts and messages are summarized as follows:
1. Hypoxia and inflammatory cytokines stimulate the swift translocation of EPOR and βcR to the cellular membrane and assemble the heterodimer.
2. EPO-derived peptide HBSP or CHBP only activates EPOR/βcR, possessing great renoprotection against IR injury. The metabolic profile and potential side effect of EPO derivatives should be further investigated before their clinical applications.
3. The signaling pathways of PI3K/Akt, STAT3, and MAPK were involved in the renoprotective effects of EPOR/βcR such as anti-apoptosis of TECs and endothelial cells and anti-inflammation at the acute stage, promoting proliferation of parenchymal cells and anti-fibrosis at the chronic stage.
4. Highly expressed EPOR/βcR in damaged cells provides a great opportunity for cell target delivery of drugs that was conjugated with its ligand such as siRNA conjugated with HBSP to precisely treat IR-induced AKI, promote repair/remodeling, and prevent its chronic progression to fibrosis.
Author Contributions
BY and YW contributed to the conception and design of the article. The review was written by YW and revised by BY. Both authors approved the final version of the manuscript.
Funding
This work was supported by the grants from the National Natural Science Foundation of China (81873622) and the Leicester Kidney Care Appeal (2018); the Research and Innovation Department, the University Hospitals of Leicester NHS Trust, and the University of Leicester.
Conflict of Interest
The authors declare that the research was conducted in the absence of any commercial or financial relationships that could be construed as a potential conflict of interest.
The reviewer CS declared a shared affiliation with one of the authors BY to the handling editor at the time of review.
References
1. Yang L, Xing G, Wang L, Wu Y, Li S, Xu G, et al. Acute Kidney Injury in China: A Cross-Sectional Survey. Lancet (2015) 386(10002):1465–71. doi: 10.1016/S0140-6736(15)00344-X
2. Coca SG, Yusuf B, Shlipak MG, Garg AX, Parikh CR. Long-Term Risk of Mortality and Other Adverse Outcomes After Acute Kidney Injury: A Systematic Review and Meta-Analysis. Am J Kidney Dis (2009) 53(6):961–73. doi: 10.1053/j.ajkd.2008.11.034
3. Yang C, Hosgood SA, Meeta P, Long Y, Zhu T, Nicholson ML, et al. Cyclic Helix B Peptide in Preservation Solution and Autologous Blood Perfusate Ameliorates Ischemia-Reperfusion Injury in Isolated Porcine Kidneys. Transplant Direct (2015) 1(2):e6. doi: 10.1097/TXD.0000000000000515
4. Hsu CY, Chertow GM, McCulloch CE, Fan D, Ordonez JD, Go AS. Nonrecovery of Kidney Function and Death After Acute on Chronic Renal Failure. Clin J Am Soc Nephrol (2009) 4(5):891–98. doi: 10.2215/CJN.05571008
5. Ishani A, Xue JL, Himmelfarb J, Eggers PW, Kimmel PL, Molitoris BA, et al. Acute Kidney Injury Increases Risk of ESRD Among Elderly. J Am Soc Nephrol (2009) 20(1):223–28. doi: 10.1681/ASN.2007080837
6. Mehta RL, Burdmann EA, Cerda J, Feehally J, Finkelstein F, Garcia-Garcia G, et al. Recognition and Management of Acute Kidney Injury in the International Society of Nephrology 0by25 Global Snapshot: A Multinational Cross-Sectional Study. Lancet (2016) 387(10032):2017–25. doi: 10.1016/S0140-6736(16)30240-9
7. Yang B, Hosgood SA, Bagul A, Waller HL, Nicholson ML. Erythropoietin Regulates Apoptosis, Inflammation and Tissue Remodelling Via Caspase-3 and IL-1β in Isolated Hemoperfused Kidneys. Eur J Pharmacol (2011) 660(2-3):420–30. doi: 10.1016/j.ejphar.2011.03.044
8. Golmohammadi MG, Banaei S, Nejati K, Chinifroush-Asl MM. Vitamin D3 and Erythropoietin Protect Against Renal Ischemia-Reperfusion Injury Via Heat Shock Protein 70 and microRNA-21 Expression. Sci Rep (2020) 10(1):20906. doi: 10.1038/s41598-020-78045-3
9. Brines M, Cerami A. The Receptor That Tames the Innate Immune Response. Mol Med (2012) 18:486–96. doi: 10.2119/molmed.2011.00414
10. Brines M, Patel NS, Villa P, Brines C, Mennini T, De Paola M, et al. Nonerythropoietic, Tissue-Protective Peptides Derived From the Tertiary Structure of Erythropoietin. Proc Natl Acad Sci USA (2008) 105(31):10925–30. doi: 10.1073/pnas.0805594105
11. Yang C, Zhao T, Lin M, Zhao Z, Hu L, Jia Y, et al. Helix B Surface Peptide Administered After Insult of Ischemia Reperfusion Improved Renal Function, Structure and Apoptosis Through Beta Common Receptor/Erythropoietin Receptor and PI3K/Akt Pathway in a Murine Model. Exp Biol Med (Maywood) (2013) 238(1):111–19. doi: 10.1258/ebm.2012.012185
12. Wu Y, Zhang J, Liu F, Yang C, Zhang Y, Liu A, et al. Protective Effects of HBSP on Ischemia Reperfusion and Cyclosporine a Induced Renal Injury. Clin Dev Immunol (2013) 2013(758159). doi: 10.1155/2013/758159
13. Liu A, Wu J, Yang C, Wu Y, Zhang Y, Zhao F, et al. TRPM7 in CHBP-Induced Renoprotection Upon Ischemia Reperfusion-Related Injury. Sci Rep (2018) 8(1):5510. doi: 10.1038/s41598-018-22852-2
14. Zhang Y, Wang Q, Liu A, Wu Y, Liu F, Wang H, et al. Erythropoietin Derived Peptide Improved Endoplasmic Reticulum Stress and Ischemia-Reperfusion Related Cellular and Renal Injury. Front Med (Lausanne) (2020) 7:5(5). doi: 10.3389/fmed.2020.00005
15. Jelkmann W. Molecular Biology of Erythropoietin. Intern Med (2004) 43(8):649–59. doi: 10.1038/ki.1993.327
16. Bondurant MC, Koury MJ. Anemia Induces Accumulation of Erythropoietin mRNA in the Kidney and Liver. Mol Cell Biol (1986) 6(7):2731–33. doi: 10.1128/mcb.6.7.2731
17. Lin CS, Lim SK, D’Agati V, Costantini F. Differential Effects of an Erythropoietin Receptor Gene Disruption on Primitive and Definitive Erythropoiesis. Genes Dev (1996) 10(2):154–64. doi: 10.1101/gad.10.2.154
18. Wu H, Liu X, Jaenisch R, Lodish HF. Generation of Committed Erythroid BFU-E and CFU-E Progenitors Does Not Require Erythropoietin or the Erythropoietin Receptor. Cell (1995) 83(1):59–67. doi: 10.1016/0092-8674(95)90234-1
19. Nairz M, Sonnweber T, Schroll A, Theurl I, Weiss G. The Pleiotropic Effects of Erythropoietin in Infection and Inflammation. Microbes Infect (2012) 14(3):238–46. doi: 10.1016/j.micinf.2011.10.005
20. Byts N, Sirén AL. Erythropoietin: A Multimodal Neuroprotective Agent. Exp Transl Stroke Med (2009) 1(4). doi: 10.1186/2040-7378-1-4
21. Kwak J, Kim JH, Jang HN, Jung MH, Cho HS, Chang SH, et al. Erythropoietin Ameliorates Ischemia/Reperfusion-Induced Acute Kidney Injury Via Inflammasome Suppression in Mice. Int J Mol Sci (2020) 21(10). doi: 10.3390/ijms21103453
22. Li GQ, Chen M. Cardioprotective Effect of Erythropoietin in Rats With Acute Myocardial Infarction Through JNK Pathway. Eur Rev Med Pharmacol Sci (2019) 23(3 Suppl):153–60. doi: 10.26355/eurrev_201901_18642
23. Tan R, Tian H, Yang B, Zhang B, Dai C, Han Z, et al. Autophagy and Akt in the Protective Effect of Erythropoietin Helix B Surface Peptide Against Hepatic Ischaemia/Reperfusion Injury in Mice. Sci Rep (2018) 8(1):14703. doi: 10.1038/s41598-018-33028-3
24. Brines M, Grasso G, Fiordaliso F, Sfacteria A, Ghezzi P, Fratelli M, et al. Erythropoietin Mediates Tissue Protection Through an Erythropoietin and Common Beta-Subunit Heteroreceptor. Proc Natl Acad Sci USA (2004) 101(41):14907–12. doi: 10.1073/pnas.0406491101
25. Lee TS, Lu KY, Yu YB, Lee HT, Tsai FC. Beta Common Receptor Mediates Erythropoietin-Conferred Protection on OxLDL-Induced Lipid Accumulation and Inflammation in Macrophages. Mediators Inflamm (2015) 2015(439759). doi: 10.1155/2015/439759
26. Su KH, Shyue SK, Kou YR, Ching LC, Chiang AN, Yu YB, et al. β Common Receptor Integrates the Erythropoietin Signaling in Activation of Endothelial Nitric Oxide Synthase. J Cell Physiol (2011) 226(12):3330–39. doi: 10.1002/jcp.22678
27. Masuda S, Okano M, Yamagishi K, Nagao M, Ueda M, Sasaki R. A Novel Site of Erythropoietin Production. Oxygen-Dependent Production in Cultured Rat Astrocytes. J Biol Chem (1994) 269(30):19488–93. doi: 10.1016/S0021-9258(17)32195-6
28. Brines M, Cerami A. Erythropoietin-Mediated Tissue Protection: Reducing Collateral Damage From the Primary Injury Response. J Intern Med (2008) 264(5):405–32. doi: 10.1111/j.1365-2796.2008.02024.x
29. Aydin Z, Mallat MJ, Schaapherder AF, van Zonneveld AJ, van Kooten C, Rabelink TJ, et al. Randomized Trial of Short-Course High-Dose Erythropoietin in Donation After Cardiac Death Kidney Transplant Recipients. Am J Transplant (2012) 12(7):1793–800. doi: 10.1111/j.1600-6143.2012.04019.x
30. Hafer C, Becker T, Kielstein JT, Bahlmann E, Schwarz A, Grinzoff N, et al. High-Dose Erythropoietin has No Effect on Short- or Long-Term Graft Function Following Deceased Donor Kidney Transplantation. Kidney Int (2012) 81(3):314–20. doi: 10.1038/ki.2011.349
31. Martinez F, Kamar N, Pallet N, Lang P, Durrbach A, Lebranchu Y, et al. High Dose Epoetin Beta in the First Weeks Following Renal Transplantation and Delayed Graft Function: Results of the Neo-PDGF Study. Am J Transplant (2010) 10(7):1695–700. doi: 10.1111/j.1600-6143.2010.03142.x
32. Sureshkumar KK, Hussain SM, Ko TY, Thai NL, Marcus RJ. Effect of High-Dose Erythropoietin on Graft Function After Kidney Transplantation: A Randomized, Double-Blind Clinical Trial. Clin J Am Soc Nephrol (2012) 7(9):1498–506. doi: 10.2215/CJN.01360212
33. Endre ZH, Walker RJ, Pickering JW, Shaw GM, Frampton CM, Henderson SJ, et al. Early Intervention With Erythropoietin Does Not Affect the Outcome of Acute Kidney Injury (the EARLYARF Trial). Kidney Int (2010) 77(11):1020–30. doi: 10.1038/ki.2010.25
34. Lund A, Lundby C, Olsen NV. High-Dose Erythropoietin for Tissue Protection. Eur J Clin Invest (2014) 44(12):1230–8. doi: 10.1111/eci.12357
35. Ludman AJ, Yellon DM, Hasleton J, Ariti C, Babu GG, Boston-Griffiths E, et al. Effect of Erythropoietin as an Adjunct to Primary Percutaneous Coronary Intervention: A Randomised Controlled Clinical Trial. Heart (2011) 97(19):1560–65. doi: 10.1136/hrt.2011.223867
36. Najjar SS, Rao SV, Melloni C, Raman SV, Povsic TJ, Melton L, et al. Intravenous Erythropoietin in Patients With ST-Segment Elevation Myocardial Infarction: REVEAL: A Randomized Controlled Trial. JAMA (2011) 305(18):1863–72. doi: 10.1001/jama.2011.592
37. Ott I, Schulz S, Mehilli J, Fichtner S, Hadamitzky M, Hoppe K, et al. Erythropoietin in Patients With Acute ST-Segment Elevation Myocardial Infarction Undergoing Primary Percutaneous Coronary Intervention: A Randomized, Double-Blind Trial. Circ Cardiovasc Interv (2010) 3(5):408–13. doi: 10.1161/CIRCINTERVENTIONS.109.904425
38. Okada T, Sawada T, Kubota K. Asialoerythropoietin has Strong Renoprotective Effects Against Ischemia-Reperfusion Injury in a Murine Model. Transplantation (2007) 84(4):504–10. doi: 10.1097/01.tp.0000277672.02783.33
39. Patel NS, Kerr-Peterson HL, Brines M, Collino M, Rogazzo M, Fantozzi R, et al. Delayed Administration of Pyroglutamate Helix B Surface Peptide (pHBSP), a Novel Nonerythropoietic Analog of Erythropoietin, Attenuates Acute Kidney Injury. Mol Med (2012) 18(1):719–27. doi: 10.2119/molmed.2012.00093
40. Cassis P, Azzollini N, Solini S, Mister M, Aiello S, Cugini D, et al. Both Darbepoetin Alfa and Carbamylated Erythropoietin Prevent Kidney Graft Dysfunction Due to Ischemia/Reperfusion in Rats. Transplantation (2011) 92(3):271–9. doi: 10.1097/TP.0b013e3182241106
41. Chattong S, Tanamai J, Kiatsomchai P, Nakatsu M, Sereemaspun A, Pimpha N, et al. Glutaraldehyde Erythropoietin Protects Kidney in Ischaemia/Reperfusion Injury Without Increasing Red Blood Cell Production. Br J Pharmacol (2013) 168(1):189–99. doi: 10.1111/j.1476-5381.2012.02123.x
42. Leist M, Ghezzi P, Grasso G, Bianchi R, Villa P, Fratelli M, et al. Derivatives of Erythropoietin That Are Tissue Protective But Not Erythropoietic. Science (2004) 305(5681):239–42. doi: 10.1126/science.1098313
43. Zhang C, Yang C, Zhu T. From Erythropoietin to Its Peptide Derivatives: Smaller But Stronger. Curr Protein Pept Sci (2017) 18(12):1191–4. doi: 10.2174/1389203717666160909130006
44. Yang C, Xu Z, Zhao Z, Li L, Zhao T, Peng D, et al. A Novel Proteolysis-Resistant Cyclic Helix B Peptide Ameliorates Kidney Ischemia Reperfusion Injury. Biochim Biophys Acta (2014) 1842(11):2306–17. doi: 10.1016/j.bbadis.2014.09.001
45. Yang C, Cao Y, Zhang Y, Li L, Xu M, Long Y, et al. Cyclic Helix B Peptide Inhibits Ischemia Reperfusion-Induced Renal Fibrosis Via the PI3K/Akt/FoxO3a Pathway. J Transl Med (2015) 13(355). doi: 10.1186/s12967-015-0699-2
46. Gobe GC, Bennett NC, West M, Colditz P, Brown L, Vesey DA, et al. Increased Progression to Kidney Fibrosis After Erythropoietin Is Used as a Treatment for Acute Kidney Injury. Am J Physiol Renal Physiol (2014) 306(6):F681–92. doi: 10.1152/ajprenal.00241.2013
47. Shi M, Flores B, Li P, Gillings N, McMillan KL, Ye J, et al. Effects of Erythropoietin Receptor Activity on Angiogenesis, Tubular Injury, and Fibrosis in Acute Kidney Injury: A “U-Shaped” Relationship. Am J Physiol Renal Physiol (2018) 314(4):F501–16. doi: 10.1152/ajprenal.00306.2017
48. Vázquez-Méndez E, Gutiérrez-Mercado Y, Mendieta-Condado E, Gálvez-Gastélum FJ, Esquivel-Solís H, Sánchez-Toscano Y, et al. Recombinant Erythropoietin Provides Protection Against Renal Fibrosis in Adenine-Induced Chronic Kidney Disease. Mediators Inflamm (2020) 2020(8937657). doi: 10.1155/2020/8937657
49. Jelkmann W, Bohlius J, Hallek M, Sytkowski AJ. The Erythropoietin Receptor in Normal and Cancer Tissues. Crit Rev Oncol Hematol (2008) 67(1):39–61. doi: 10.1016/j.critrevonc.2008.03.006
50. Echigoya MH, Obikane K, Nakashima T, Sasaki S. Glomerular Localization of Erythropoietin Receptor mRNA and Protein in Neonatal and Mature Mouse Kidney. Nephron Exp Nephrol (2005) 100(1):e21–9. doi: 10.1159/000084109
51. Juul SE, Yachnis AT, Rojiani AM, Christensen RD. Immunohistochemical Localization of Erythropoietin and Its Receptor in the Developing Human Brain. Pediatr Dev Pathol (1999) 2(2):148–58. doi: 10.1007/s100249900103
52. Zafiriou MP, Noack C, Unsold B, Didie M, Pavlova E, Fischer HJ, et al. Erythropoietin Responsive Cardiomyogenic Cells Contribute to Heart Repair Post Myocardial Infarction. Stem Cells (2014) 32(9):2480–91. doi: 10.1002/stem.1741
53. Suzuki N, Ohneda O, Takahashi S, Higuchi M, Mukai HY, Nakahata T, et al. Erythroid-Specific Expression of the Erythropoietin Receptor Rescued Its Null Mutant Mice From Lethality. Blood (2002) 100(7):2279–88. doi: 10.1182/blood-2002-01-0124
54. Xiao L, Li Z, Xu P, Li Z, Xu J, Yang Z. The Expression of EPOR in Renal Cortex During Postnatal Development. PloS One (2012) 7(7):e41993. doi: 10.1371/journal.pone.0041993
55. Hu MC, Shi M, Cho HJ, Zhang J, Pavlenco A, Liu S, et al. The Erythropoietin Receptor Is a Downstream Effector of Klotho-Induced Cytoprotection. Kidney Int (2013) 84(3):468–81. doi: 10.1038/ki.2013.149
56. Brines M. The Therapeutic Potential of Erythropoiesis-Stimulating Agents for Tissue Protection: A Tale of Two Receptors. Blood Purif (2010) 29(2):86–92. doi: 10.1159/000245630
57. D’Andrea RJ, Gonda TJ. A Model for Assembly and Activation of the GM-CSF, IL-3 and IL-5 Receptors: Insights From Activated Mutants of the Common Beta Subunit. Exp Hematol (2000) 28(3):231–43. doi: 10.1016/S0301-472X(99)00159-9
58. Murphy JM, Young IG. IL-3, IL-5, and GM-CSF Signaling: Crystal Structure of the Human Beta-Common Receptor. Vitam Horm (2006) 74:1–30. doi: 10.1016/S0083-6729(06)74001-8
59. Metcalf D. Hematopoietic Regulators: Redundancy or Subtlety? Blood (1993) 82(12):3515–23. doi: 10.1182/blood.V82.12.3515.3515
60. Hanazono Y, Sasaki K, Nitta H, Yazaki Y, Hirai H. Erythropoietin Induces Tyrosine Phosphorylation of the Beta Chain of the GM-CSF Receptor. Biochem Biophys Res Commun (1995) 208(3):1060–6. doi: 10.1006/bbrc.1995.1442
61. Jubinsky PT, Krijanovski OI, Nathan DG, Tavernier J, Sieff CA. The Beta Chain of the Interleukin-3 Receptor Functionally Associates With the Erythropoietin Receptor. Blood (1997) 90(5):1867–73. doi: 10.1182/blood.V90.5.1867
62. Uversky VN, Redwan EM. Erythropoietin and Co.: Intrinsic Structure and Functional Disorder. Mol Biosyst (2016) 13(1):56–72. doi: 10.1039/c6mb00657d
63. Collino M, Thiemermann C, Cerami A, Brines M. Flipping the Molecular Switch for Innate Protection and Repair of Tissues: Long-Lasting Effects of a Non-Erythropoietic Small Peptide Engineered From Erythropoietin. Pharmacol Ther (2015) 151:32–40. doi: 10.1016/j.pharmthera.2015.02.005
64. Funakoshi-Tago M, Pelletier S, Moritake H, Parganas E, Ihle JN. Jak2 FERM Domain Interaction With the Erythropoietin Receptor Regulates Jak2 Kinase Activity. Mol Cell Biol (2008) 28(5):1792–801. doi: 10.1128/MCB.01447-07
65. Chin H, Wakao H, Miyajima A, Kamiyama R, Miyasaka N, Miura O. Erythropoietin Induces Tyrosine Phosphorylation of the Interleukin-3 Receptor Beta Subunit (betaIL3) and Recruitment of Stat5 to Possible Stat5-docking Sites in Betail3. Blood (1997) 89(12):4327–36. doi: 10.1182/blood.V89.12.4327
66. Zhang J, Zou YR, Zhong X, Deng HD, Pu L, Peng K, et al. Erythropoietin Pretreatment Ameliorates Renal Ischaemia-Reperfusion Injury by Activating PI3K/Akt Signalling. Nephrology (Carlton) (2015) 20(4):266–72. doi: 10.1111/nep.12384
67. Patel NS, Nandra KK, Brines M, Collino M, Wong WF, Kapoor A, et al. A Nonerythropoietic Peptide That Mimics the 3D Structure of Erythropoietin Reduces Organ Injury/Dysfunction and Inflammation in Experimental Hemorrhagic Shock. Mol Med (2011) 17(9-10):883–92. doi: 10.2119/molmed.2011.00053
68. Ahmet I, Tae HJ, Juhaszova M, Riordon DR, Boheler KR, Sollott SJ, et al. A Small Nonerythropoietic Helix B Surface Peptide Based Upon Erythropoietin Structure Is Cardioprotective Against Ischemic Myocardial Damage. Mol Med (2011) 17(3-4):194–200. doi: 10.2119/molmed.2010.00235
69. Ueba H, Brines M, Yamin M, Umemoto T, Ako J, Momomura S, et al. Cardioprotection by a Nonerythropoietic, Tissue-Protective Peptide Mimicking the 3D Structure of Erythropoietin. Proc Natl Acad Sci USA (2010) 107(32):14357–62. doi: 10.1073/pnas.1003019107
70. Xu X, Cao Z, Cao B, Li J, Guo L, Que L, et al. Carbamylated Erythropoietin Protects the Myocardium From Acute Ischemia/Reperfusion Injury Through a PI3K/Akt-Dependent Mechanism. Surgery (2009) 146(3):506–14. doi: 10.1016/j.surg.2009.03.022
71. Chamorro ME, Maltaneri RE, Vittori DC, Nesse AB. Protein Tyrosine Phosphatase 1B (PTP1B) Is Involved in the Defective Erythropoietic Function of Carbamylated Erythropoietin. Int J Biochem Cell Biol (2015) 61:63–71. doi: 10.1016/j.biocel.2015.01.019
72. Liu P, You W, Lin L, Lin Y, Tang X, Liu Y, et al. Helix B Surface Peptide Protects Against Acute Myocardial Ischemia-Reperfusion Injury Via the RISK and SAFE Pathways in a Mouse Model. Cardiology (2016) 134(2):109–17. doi: 10.1159/000443680
73. Yang C, Zhang Y, Wang J, Li L, Wang L, Hu M, et al. A Novel Cyclic Helix B Peptide Inhibits Dendritic Cell Maturation During Amelioration of Acute Kidney Graft Rejection Through Jak-2/STAT3/SOCS1. Cell Death Dis (2015) 6:e1993. doi: 10.1038/cddis.2015.338
74. Li J, Zhang G, Zhang W, Zhang Y, Zhang X, Qiu Y, et al. CHBP Induces Stronger ImmunosuppressiveCD127 + M-MDSC Via Erythropoietin Receptor. Cell Death Dis (2021) 12(2):177. doi: 10.1038/s41419-021-03448-7
75. Zou YR, Zhang J, Wang J, Peng L, Li GS, Wang L. Erythropoietin Receptor Activation Protects the Kidney From Ischemia/Reperfusion-Induced Apoptosis by Activating ERK/P53 Signal Pathway. Transplant Proc (2016) 48(1):217–21. doi: 10.1016/j.transproceed.2016.01.009
76. Jang HS, Han SJ, Kim JI, Lee S, Lipschutz JH, Park KM. Activation of ERK Accelerates Repair of Renal Tubular Epithelial Cells, Whereas it Inhibits Progression of Fibrosis Following Ischemia/Reperfusion Injury. Biochim Biophys Acta (2013) 1832(12):1998–2008. doi: 10.1016/j.bbadis.2013.07.001
77. Moon C, Krawczyk M, Paik D, Coleman T, Brines M, Juhaszova M, et al. Erythropoietin, Modified to Not Stimulate Red Blood Cell Production, Retains Its Cardioprotective Properties. J Pharmacol Exp Ther (2006) 316(3):999–1005. doi: 10.1124/jpet.105.094854
78. Yang C, Liu J, Li L, Hu M, Long Y, Liu X, et al. Proteome Analysis of Renoprotection Mediated by a Novel Cyclic Helix B Peptide in Acute Kidney Injury. Sci Rep (2015) 5:18045. doi: 10.1038/srep18045
79. Kumar S. Cellular and Molecular Pathways of Renal Repair After Acute Kidney Injury. Kidney Int (2018) 93(1):27–40. doi: 10.1016/j.kint.2017.07.030
80. Bonventre JV, Weinberg JM. Recent Advances in the Pathophysiology of Ischemic Acute Renal Failure. J Am Soc Nephrol (2003) 14(8):2199–210. doi: 10.1097/01.asn.0000079785.13922.f6
81. Yang C, Zhao T, Zhao Z, Jia Y, Li L, Zhang Y, et al. Serum-Stabilized Naked Caspase-3 siRNA Protects Autotransplant Kidneys in a Porcine Model. Mol Ther (2014) 22(10):1817–28. doi: 10.1038/mt.2014.111
82. Wu Y, Chen W, Zhang Y, Liu A, Yang C, Wang H, et al. Potent Therapy and Transcriptional Profile of Combined Erythropoietin-Derived Peptide Cyclic Helix B Surface Peptide and Caspase-3 siRNA Against Kidney Ischemia/Reperfusion Injury in Mice. J Pharmacol Exp Ther (2020) 375(1):92–103. doi: 10.1124/jpet.120.000092
83. Kitamura H, Isaka Y, Takabatake Y, Imamura R, Suzuki C, Takahara S, et al. Nonerythropoietic Derivative of Erythropoietin Protects Against Tubulointerstitial Injury in a Unilateral Ureteral Obstruction Model. Nephrol Dial Transplant (2008) 23(5):1521–8. doi: 10.1093/ndt/gfm842
84. Linkermann A, Chen G, Dong G, Kunzendorf U, Krautwald S, Dong Z. Regulated Cell Death in AKI. J Am Soc Nephrol (2014) 25(12):2689–701. doi: 10.1681/ASN.2014030262
85. Sautina L, Sautin Y, Beem E, Zhou Z, Schuler A, Brennan J, et al. Induction of Nitric Oxide by Erythropoietin Is Mediated by the {Beta} Common Receptor and Requires Interaction With VEGF Receptor 2. Blood (2010) 115(4):896–905. doi: 10.1182/blood-2009-04-216432
86. Stoyanoff TR, Rodriguez JP, Todaro JS, Colavita JPM, Torres AM, Aguirre MV. Erythropoietin Attenuates LPS-Induced Microvascular Damage in a Murine Model of Septic Acute Kidney Injury. BioMed Pharmacother (2018) 107:1046–55. doi: 10.1016/j.biopha.2018.08.087
87. Kreisel D, Sugimoto S, Tietjens J, Zhu J, Yamamoto S, Krupnick AS, et al. Bcl3 Prevents Acute Inflammatory Lung Injury in Mice by Restraining Emergency Granulopoiesis. J Clin Invest (2011) 121(1):265–76. doi: 10.1172/JCI42596
88. Watanabe M, Lundgren T, Saito Y, Cerami A, Brines M, Ostenson CG, et al. A Nonhematopoietic Erythropoietin Analogue, ARA 290, Inhibits Macrophage Activation and Prevents Damage to Transplanted Islets. Transplantation (2016) 100(3):554–62. doi: 10.1097/TP.0000000000001026
89. Ueba H, Shiomi M, Brines M, Yamin M, Kobayashi T, Ako J, et al. Suppression of Coronary Atherosclerosis by Helix B Surface Peptide, a Nonerythropoietic, Tissue-Protective Compound Derived From Erythropoietin. Mol Med (2013) 19:195–202. doi: 10.2119/molmed.2013.00037
90. Lee S, Huen S, Nishio H, Nishio S, Lee HK, Choi BS, et al. Distinct Macrophage Phenotypes Contribute to Kidney Injury and Repair. J Am Soc Nephrol (2011) 22(2):317–26. doi: 10.1681/ASN.2009060615
91. Purroy C, Fairchild RL, Tanaka T, Baldwin WM 3rd, Manrique J, Madsen JC, et al. Erythropoietin Receptor-Mediated Molecular Crosstalk Promotes T Cell Immunoregulation and Transplant Survival. J Am Soc Nephrol (2017) 28(8):2377–92. doi: 10.1681/ASN.2016101100
92. Henson PM, Bratton DL, Fadok VA. Apoptotic Cell Removal. Curr Biol (2001) 11(19):R795–805. doi: 10.1016/s0960-9822(01)00474-2
93. Luo B, Gan W, Liu Z, Shen Z, Wang J, Shi R, et al. Erythropoeitin Signaling in Macrophages Promotes Dying Cell Clearance and Immune Tolerance. Immunity (2016) 44(2):287–302. doi: 10.1016/j.immuni.2016.01.002
94. Yang L, Brooks CR, Xiao S, Sabbisetti V, Yeung MY, Hsiao LL, et al. KIM-1-Mediated Phagocytosis Reduces Acute Injury to the Kidney. J Clin Invest (2015) 125(4):1620–36. doi: 10.1172/JCI75417
95. Ajay AK, Kim TM, Ramirez-Gonzalez V, Park PJ, Frank DA, Vaidya VS. A Bioinformatics Approach Identifies Signal Transducer and Activator of Transcription-3 and Checkpoint Kinase 1 as Upstream Regulators of Kidney Injury Molecule-1 After Kidney Injury. J Am Soc Nephrol (2014) 25(1):105–18. doi: 10.1681/ASN.2013020161
96. Witzgall R, Brown D, Schwarz C, Bonventre JV. Localization of Proliferating Cell Nuclear Antigen, Vimentin, c-Fos, and Clusterin in the Postischemic Kidney. Evidence for a Heterogenous Genetic Response Among Nephron Segments, and a Large Pool of Mitotically Active and Dedifferentiated Cells. J Clin Invest (1994) 93(5):2175–88. doi: 10.1172/JCI117214
97. Takaori K, Nakamura J, Yamamoto S, Nakata H, Sato Y, Takase M, et al. Severity and Frequency of Proximal Tubule Injury Determines Renal Prognosis. J Am Soc Nephrol (2016) 27(8):2393–406. doi: 10.1681/ASN.2015060647
98. Humphreys BD, Valerius MT, Kobayashi A, Mugford JW, Soeung S, Duffield JS, et al. Intrinsic Epithelial Cells Repair the Kidney After Injury. Cell Stem Cell (2008) 2(3):284–91. doi: 10.1016/j.stem.2008.01.014
99. Basile DP. The Endothelial Cell in Ischemic Acute Kidney Injury: Implications for Acute and Chronic Function. Kidney Int (2007) 72(2):151–56. doi: 10.1038/sj.ki.5002312
100. Duffield JS. Macrophages and Immunologic Inflammation of the Kidney. Semin Nephrol (2010) 30(3):234–54. doi: 10.1016/j.semnephrol.2010.03.003
101. Ko GJ, Boo CS, Jo SK, Cho WY, Kim HK. Macrophages Contribute to the Development of Renal Fibrosis Following Ischaemia/Reperfusion-Induced Acute Kidney Injury. Nephrol Dial Transplant (2008) 23(3):842–52. doi: 10.1093/ndt/gfm694
102. Bechtel W, McGoohan S, Zeisberg EM, Müller GA, Kalbacher H, Salant DJ, et al. Methylation Determines Fibroblast Activation and Fibrogenesis in the Kidney. Nat Med (2010) 16(5):544–50. doi: 10.1038/nm.2135
103. Srisawat N, Manotham K, Eiam-Ong S, Katavetin P, Praditpornsilpa K. Erythropoietin and Its Non-Erythropoietic Derivative: Do They Ameliorate Renal Tubulointerstitial Injury in Ureteral Obstruction? Int J Urol (2008) 15(11):1011–17. doi: 10.1111/j.1442-2042.2008.02149.x
104. Wang L, Zhang ZG, Gregg SR, Zhang RL, Jiao Z, LeTourneau Y, et al. The Sonic Hedgehog Pathway Mediates Carbamylated Erythropoietin-Enhanced Proliferation and Differentiation of Adult Neural Progenitor Cells. J Biol Chem (2007) 282(44):32462–70. doi: 10.1074/jbc.M706880200
105. Kolyada AY, Tighiouart H, Perianayagam MC, Liangos O, Madias NE, Jaber BL. A Genetic Variant of Hypoxia-Inducible Factor-1alpha Is Associated With Adverse Outcomes in Acute Kidney Injury. Kidney Int (2009) 75(12):1322–9. doi: 10.1038/ki.2009.68
106. Wang H, Liu N, Li R, Tian J, Hu W, Zhang J. Nephropreventing Effect of Hypoxia-Inducible Factor 1alpha in a Rat Model of Ischaemic/Reperfusion Acute Kidney Injury. Clin Exp Pharmacol Physiol (2018) 45(10):1076–82. doi: 10.1111/1440-1681.12947
107. Hu MC, Shi M, Zhang J, Quiñones H, Kuro-o M, Moe OW. Klotho Deficiency Is an Early Biomarker of Renal Ischemia-Reperfusion Injury and Its Replacement Is Protective. Kidney Int (2010) 78(12):1240–51. doi: 10.1038/ki.2010.328
108. Sugiura H, Yoshida T, Mitobe M, Yoshida S, Shiohira S, Nitta K, et al. Klotho Reduces Apoptosis in Experimental Ischaemic Acute Kidney Injury via HSP-70. Nephrol Dial Transplant (2010) 25(1):60–8. doi: 10.1093/ndt/gfp451
109. Nangaku M. Tissue Protection by Erythropoietin: New Findings in a Moving Field. Kidney Int (2013) 84(3):427–9. doi: 10.1038/ki.2013.140
110. Taoufik E, Petit E, Divoux D, Tseveleki V, Mengozzi M, Roberts ML, et al. TNF Receptor I Sensitizes Neurons to Erythropoietin- and VEGF-Mediated Neuroprotection After Ischemic and Excitotoxic Injury. Proc Natl Acad Sci USA (2008) 105(16):6185–90. doi: 10.1073/pnas.0801447105
111. Wang L, Chopp M, Teng H, Bolz M, Francisco MA, Aluigi DM, et al. Tumor Necrosis Factor Alpha Primes Cerebral Endothelial Cells for Erythropoietin-Induced Angiogenesis. J Cereb Blood Flow Metab (2011) 31(2):640–7. doi: 10.1038/jcbfm.2010.138
112. Lee YS, Vortmeyer AO, Lubensky IA, Vogel TW, Ikejiri B, Ferlicot S, et al. Coexpression of Erythropoietin and Erythropoietin Receptor in Von Hippel-Lindau Disease-Associated Renal Cysts and Renal Cell Carcinoma. Clin Cancer Res (2005) 11(3):1059–64. doi: 10.1182/blood.V92.9.3388
113. Liu N, Tian J, Wang W, Cheng J, Hu D, Zhang J. Effect and Mechanism of Erythropoietin on Mesenchymal Stem Cell Proliferation In Vitro Under the Acute Kidney Injury Microenvironment. Exp Biol Med (Maywood) (2011) 236(9):1093–99. doi: 10.1258/ebm.2011.011001
114. Meyer L, Deau B, Forejtníková H, Duménil D, Margottin-Goguet F, Lacombe C, et al. beta-Trcp Mediates Ubiquitination and Degradation of the Erythropoietin Receptor and Controls Cell Proliferation. Blood (2007) 109(12):5215–22. doi: 10.1182/blood-2006-10-055350
115. Bulut GB, Sulahian R, Ma Y, Chi NW, Huang LJ. Ubiquitination Regulates the Internalization, Endolysosomal Sorting, and Signaling of the Erythropoietin Receptor. J Biol Chem (2011) 286(8):6449–57. doi: 10.1074/jbc.M110.186890
116. Miao S, Wang SM, Cheng X, Li YF, Zhang QS, Li G, et al. Erythropoietin Promoted the Proliferation of Hepatocellular Carcinoma Through Hypoxia Induced Translocation of Its Specific Receptor. Cancer Cell Int (2017) 17(119). doi: 10.1186/s12935-017-0494-7
117. McGraw KL, Fuhler GM, Johnson JO, Clark JA, Caceres GC, Sokol L, et al. Erythropoietin Receptor Signaling Is Membrane Raft Dependent. PloS One (2012) 7(4):e34477. doi: 10.1371/journal.pone.0034477
118. Saulle E, Riccioni R, Coppola S, Parolini I, Diverio D, Riti V, et al. Colocalization of the VEGF-R2 and the Common IL-3/GM-CSF Receptor Beta Chain to Lipid Rafts Leads to Enhanced p38 Activation. Br J Haematol (2009) 145(3):399–411. doi: 10.1111/j.1365-2141.2009.07627.x
119. Bohr S, Patel SJ, Vasko R, Shen K, Iracheta-Vellve A, Lee J, et al. Modulation of Cellular Stress Response Via the Erythropoietin/CD131 Heteroreceptor Complex in Mouse Mesenchymal-Derived Cells. J Mol Med (Berl) (2015) 93(2):199–210. doi: 10.1007/s00109-014-1218-2
120. Khwaja A. KDIGO Clinical Practice Guidelines for Acute Kidney Injury. Nephron Clin Pract (2012) 120(4):c179–84. doi: 10.1159/000339789
121. Yet MG, Jones SS. The Extracytoplasmic Domain of the Erythropoietin Receptor Forms a Monomeric Complex With Erythropoietin. Blood (1993) 82(6):1713–19. doi: 10.1182/blood.V82.6.1713.1713
122. Khankin EV, Mutter WP, Tamez H, Yuan HT, Karumanchi SA, Thadhani R. Soluble Erythropoietin Receptor Contributes to Erythropoietin Resistance in End-Stage Renal Disease. PloS One (2010) 5(2):e9246. doi: 10.1371/journal.pone.0009246
Keywords: acute kidney injury, apoptosis, EPOR/βcR, fibrosis, inflammation, ischemia-reperfusion
Citation: Wu Y and Yang B (2021) Erythropoietin Receptor/β Common Receptor: A Shining Light on Acute Kidney Injury Induced by Ischemia-Reperfusion. Front. Immunol. 12:697796. doi: 10.3389/fimmu.2021.697796
Received: 20 April 2021; Accepted: 17 May 2021;
Published: 30 June 2021.
Edited by:
Cheng Yang, Fudan University, ChinaReviewed by:
Dong Zhu, Fudan University, ChinaCordula M. Stover, University of Leicester, United Kingdom
Copyright © 2021 Wu and Yang. This is an open-access article distributed under the terms of the Creative Commons Attribution License (CC BY). The use, distribution or reproduction in other forums is permitted, provided the original author(s) and the copyright owner(s) are credited and that the original publication in this journal is cited, in accordance with accepted academic practice. No use, distribution or reproduction is permitted which does not comply with these terms.
*Correspondence: Bin Yang, Ynk1QGxlLmFjLnVr