- 1Department of Population Medicine and Diagnostic Sciences, Animal Health Diagnostic Center, College of Veterinary Medicine, Cornell University, Ithaca, NY, United States
- 2Department of Veterinary and Biomedical Sciences, Animal Disease Research And Diagnostic Laboratory, South Dakota State University, Brookings, SD, United States
- 3Department of Veterinary Diagnostic and Production Animal Medicine, Iowa State University, Ames, IA, United States
- 4Department of Veterinary Preventive Medicine, Center for Food Animal Health, Ohio State University, Wooster, OH, United States
Swine influenza is a highly contagious respiratory disease of pigs caused by influenza A viruses (IAV-S). IAV-S causes significant economic losses to the swine industry and poses challenges to public health given its zoonotic potential. Thus effective IAV-S vaccines are needed and highly desirable and would benefit both animal and human health. Here, we developed two recombinant orf viruses, expressing the hemagglutinin (HA) gene (OV-HA) or the HA and the nucleoprotein (NP) genes of IAV-S (OV-HA-NP). The immunogenicity and protective efficacy of these two recombinant viruses were evaluated in pigs. Both OV-HA and OV-HA-NP recombinants elicited robust virus neutralizing antibody response in pigs, with higher levels of neutralizing antibodies (NA) being detected in OV-HA-NP-immunized animals pre-challenge infection. Although both recombinant viruses elicited IAV-S-specific T-cell responses, the frequency of IAV-S-specific proliferating CD8+ T cells upon re-stimulation was higher in OV-HA-NP-immunized animals than in the OV-HA group. Importantly, IgG1/IgG2 isotype ELISAs revealed that immunization with OV-HA induced Th2-biased immune responses, whereas immunization with OV-HA-NP virus resulted in a Th1-biased immune response. While pigs immunized with either OV-HA or OV-HA-NP were protected when compared to non-immunized controls, immunization with OV-HA-NP resulted in incremental protection against challenge infection as evidenced by a reduced secondary antibody response (NA and HI antibodies) following IAV-S challenge and reduced virus shedding in nasal secretions (lower viral RNA loads and frequency of animals shedding viral RNA and infectious virus), when compared to animals in the OV-HA group. Interestingly, broader cross neutralization activity was also observed in serum of OV-HA-NP-immunized animals against a panel of contemporary IAV-S isolates representing the major genetic clades circulating in swine. This study demonstrates the potential of ORFV-based vector for control of swine influenza virus in swine.
Introduction
Swine influenza is a highly contagious respiratory disease of pigs caused by influenza A viruses in swine (IAV-S). IAV-S is an enveloped, single stranded RNA virus of the family Orthomyxoviridae. The IAV-S genome consists of eight single-stranded negative-sense RNA segments encoding 11 proteins (PB1, PB2, PB1-F2, PA, HA, NP, NA, M1, M2, NS1, NS2/NEP) (1). There are three recognized subtypes of IAV-S that are currently circulating in the US: H1N1, H1N2 and H3N2 (2). The H1N1 subtype is the major subtype that has been prevalent in the US swine population for several decades; however, recent epidemiological data suggests an increasing incidence of H1N2 and H3N2 IAV-S subtypes (3, 4). IAV-S causes acute respiratory disease in pigs resulting in high morbidity (up to 100%). The mortality rate is usually low (1-4%) with most infected animals recovering within 3-7 days of infection (5, 6). The median yearly herd prevalence of IAV-S reported in the US is approximately 28%, but it can reach up to 57% in winter and spring months (7). IAV-S results in significant economic losses to the swine industry mainly due to weight loss, increased time to market, costs associated with treatment of secondary bacterial infections and mortality. This makes IAV-S one of the top three health challenges to the swine industry affecting pigs in all phases of production (8, 9). In addition to IAV-S, pigs are also susceptible to infection with avian and human IAVs thereby providing a niche for genetic reassortment between avian/human or swine influenza viruses. This poses a major threat for emergence of new subtypes as well as increases the risk of zoonotic transmission of IAVs. Therefore, effective prevention and control measures for IAV infections in swine have direct impacts on both animal and human health.
Currently, most available IAV-S vaccines are based on whole inactivated virus (WIV). However, these vaccines have not been able to effectively control IAV in swine and in some cases vaccine associated enhanced respiratory disease has been observed when there is an antigenic mismatch between vaccine strain and infecting strain (10). A live-attenuated influenza virus (LAIV) vaccine based on a virus containing a deletion of the NS1 gene, has been recently licensed for use in pigs in the US and may overcome some of the drawbacks of WIV vaccines (11). However, LAIV vaccines have the potential to reassort with the endemic viruses which could result in new influenza virus variants. Indeed, novel variants that arose from reassortment between the vaccine virus and endemic field strains have been recently reported (12). These observations highlight the need for safer and more efficacious IAV-S vaccine candidates. Here we investigated the potential of vectored vaccine candidates based on the parapox orf virus (ORFV) in controlling IAV-S infection in pigs.
Orf virus (ORFV) belongs to genus Parapoxvirus within the family Poxviridae (13) and is a ubiquitous virus that primarily causes a self-limiting mucocutaneous infection in sheep, goats and wild ruminants (14, 15). ORFV contains a double-stranded DNA genome with approximately 138 kbp in length and encodes 131 putative genes, including several with immunomodulatory (IMP) functions (16). Given ORFV IMP properties, the virus has long been used as a preventive and therapeutic agent in veterinary medicine (17, 18). Additionally, the potential of ORFV as a vaccine delivery platform against several viral diseases in permissive and non-permissive animal species has been explored by us and others (19–25). ORFV based vectored-vaccine candidates have been shown to induce protective immunity against pseudorabies virus (PRV), classical swine fever virus (CSFV) and porcine epidemic diarrhea virus (PEDV) (23, 24, 26, 27). Among the features that make ORFV a promising viral vector for vaccine delivery in swine are: (i) its restricted host range, (ii) its ability to induce both humoral and cellular immune response (23, 28), (iii) its tropism which is restricted to skin keratinocytes with no evidence of systemic dissemination, (iv) lack of vector-specific neutralizing antibodies which allows efficient prime-boost strategies using the same vector constructs (29, 30), and (v) its large genome size with the presence of several non-essential genes, which can be manipulated without severely impacting virus replication. Additionally, ORFV encodes several genes with well-characterized immunomodulatory properties. These include a homologue of interleukin 10 (IL-10) (31), a chemokine binding protein (CBP) (32), an inhibitor of granulocyte-monocyte colony stimulating factor (GM-CSF) (33), an interferon resistance gene (VIR) (34), a homologue of vascular endothelial growth factor (VEGF) (35), and inhibitors of nuclear-factor kappa-B (NF-ƙB) signaling pathway (36–39). The presence of these well-characterized immunomodulatory proteins allowed us to rationally engineer ORFV-based vectors with enhanced safety and immunogenicity profile for use in livestock species, including swine (23–25).
Here we assessed the immunogenicity and protective efficacy of recombinant ORFV vectors expressing the HA protein alone or the HA and the nucleoprotein (NP) of IAV-S. While the HA protein contains immunodominant epitopes recognized by neutralizing antibodies (40, 41), the NP protein contains highly conserved immunodominant T-cell epitopes (42). We performed a side-by-side comparison of the immunogenicity and protective efficacy of the recombinant OV vectors expressing the HA or the HA and the NP proteins in pigs.
Material and Methods
Cells and Viruses
Primary ovine turbinate cells (OFTu), Madin-Darby canine kidney cells (MDCK) and swine turbinate cells (STU) were cultured at 37°C with 5% CO2 in minimum essential medium (MEM) supplemented with 10% FBS, 2 mM L-glutamine and containing streptomycin (100 µg/mL), penicillin (100 U/mL) and gentamycin (50 µg/mL).
The ORFV strain IA82 (OV-IA82; kindly provided by Dr. Daniel Rock at University of Illinois Urbana-Champaign), was used as the parental virus to construct the recombinant viruses and in all the experiments involving the use of wild-type ORFV. Wild-type and recombinant ORFV viruses were amplified in OFTu cells. Swine influenza virus H1N1 A/Swine/OH/24366/2007 (H1N1), kindly provided by Gourapura Lab was used for virus challenge, virus neutralization assay, hemagglutination inhibition (HI), and as a coating antigen for whole virus ELISA. The H1N1 A/Swine/OH/24366/2007 (H1N1) virus was propagated in MDCK cells using DMEM containing TPCK-treated trypsin (2 µg/mL) and 25 mM HEPES buffer. All the IAV-S isolates used in cross-neutralization assay were obtained from National Veterinary Services Laboratory (NVSL) (Ames, Iowa). These isolates included: A/Swine/Iowa/A02424852/2020, A/Swine/South Dakota/A02156993/2018, A/Swine/Missouri/A02479312/2020, A/Swine/Michigan/A02524810/2020, A/Swine/Texas/A02245632/2020, A/Swine/Oklahoma/A02245707/2020, A/Swine/Minnesota/A01785306/2017, A/Swine/Iowa/A02479151/2020, A/Swine/Oklahoma/A02214419/2017, and A/Swine/South Dakota/A02524887/2020.
Generation of Recombination Plasmids
To insert the heterologous IAV-S gene in the ORFV121 locus, a recombination plasmid containing right and left flanking sequences of the ORFV121 gene were inserted into pUC57 plasmid. The HA gene of swine influenza virus, A/SW/OH/511445/2007 (OH7) (GenBank: EU604689) (43) was inserted between the ORFV121 flanking sequences in the pUC57 plasmid. The HA gene was condon optimized for swine species (GenScript). The HA gene was cloned under the vaccinia virus (VACV) I1L promoter (5’-TATTTAAAAGTTGTTTGGTGAACTTAAATGG – 3’) (44) and a flag-tag epitope (DYKDDDK) was fused to the amino terminus of the HA gene to detect its expression. The gene encoding green fluorescent protein (GFP) was inserted downstream of HA gene and used as a selection marker for recombinant virus purification. The GFP sequence was flanked by loxp sequences 5’-ATAACTTCGTATAATGTATACTATACGAAGTTAT-3’ to allow for removal of GFP by Cre recombinase following recombinant virus purification. This recombination cassette was named pUC57-121LR-SIV-HA-loxp-GFP (Figure 1A).
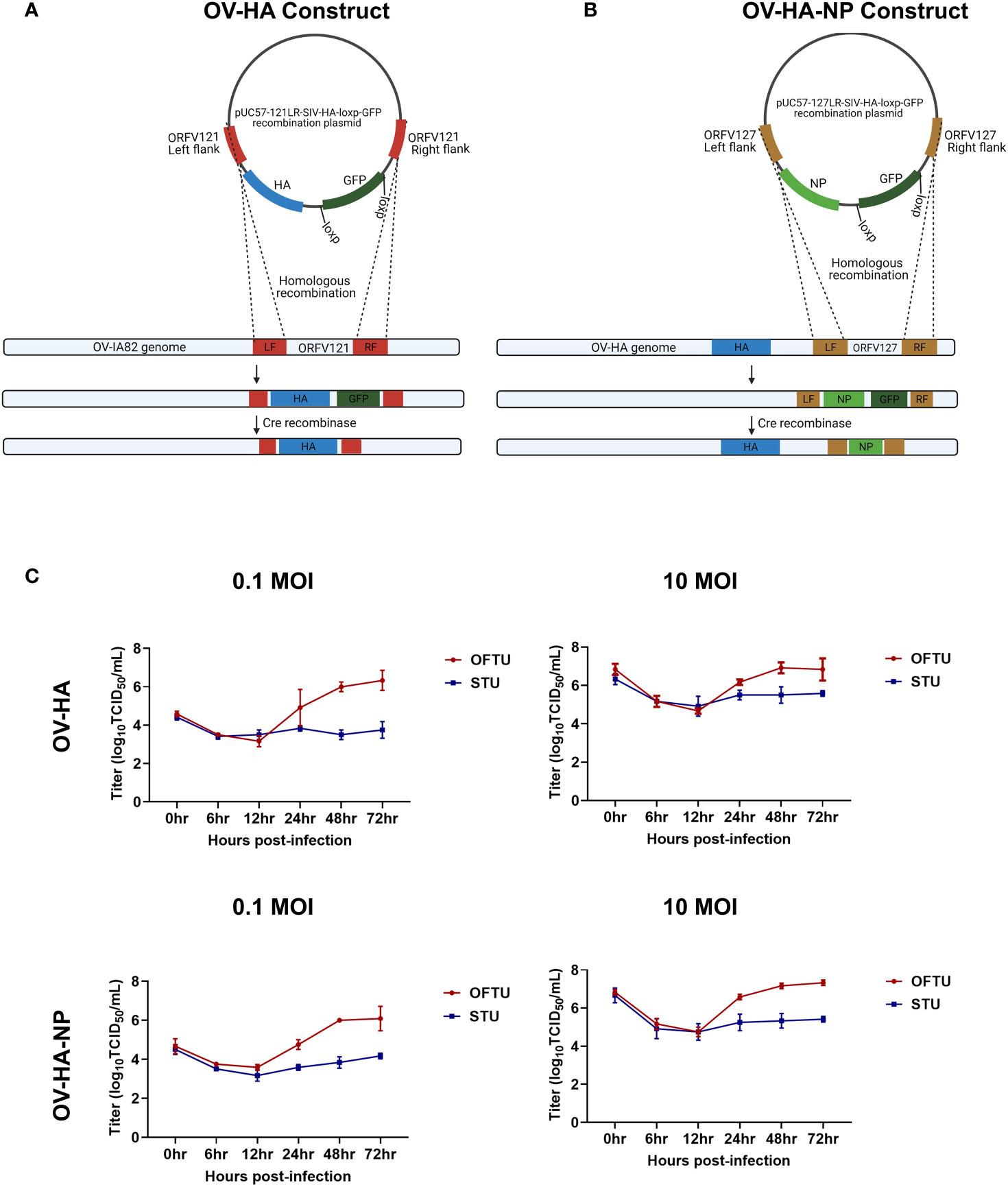
Figure 1 Construction of ORFV recombinants and their replication kinetics. (A) Schematic representation of homologous recombination between pUC57-121LR-SIV-HA-loxp-GFP plasmid and ORFV-IA82 genome. The recombinant virus was treated with Cre recombinase to remove GFP marker gene to obtain markerless OV-HA construct. (B) Schematic representation of homologous recombination between pUC57-127LR-SIV-NP-loxp-GFP plasmid and OV-HA genome. The recombinant virus was treated with Cre recombinase to obtain markerless OV-HA-NP construct. (C) Multi-step (0.1 MOI) and single step (10 MOI) growth curve of OV-HA and OV-HA-NP. OFTu or STU cells were infected with OV-HA and-HA-NP recombinants and virus titers were calculated at 0, 6, 12, 24, 48 and 72 hours post-infection. Error bars represent SEM calculated based on three independent experiments.
Similarly, another recombination cassette was generated to insert the NP gene of IAV-S into the ORFV ORFV127 locus. A recombination cassette for ORFV127 was constructed as described above with the ORFV127 left and right flanking regions being cloned into the pUC57-LoxP-GFP plasmid (pUC57-127LR-LoxP-GFP. The nucleoprotein (NP) gene of swine influenza virus, A/SW/OH/511445/2007 (OH7) (GenBank: EU604694) (43) was inserted between ORFV127 left and right flanks. The NP gene was cloned under the VACV vv7.5 promoter (45) and the HA epitope tag sequence (YPYDVPDYA) was fused at the amino terminus of the NP protein to detect its expression by the recombinant virus. In addition, an eukaryotic Kozak consensus sequence 5’-gccaaccATGg-3’ (46), where ATG refers to the start codon of the NP gene, was added immediately downstream of vv7.5 promoter. This recombination cassette was named pUC57-127LR-SIV-NP-loxp-GFP (Figure 1B).
Generation of Recombinant OV-HA and OV-HA-NP Viruses
The HA gene of IAV-S was inserted into the ORFV121 locus of the ORFV genome by homologous recombination. Briefly, OFTu cells cultured in 6-well plates were infected with OV-IA82 with a multiplicity of infection (MOI) of 1. Three hours later, the infected cells were transfected with 2 µg of pUC57-121LR-SIV-HA-loxp-GFP using Lipofectamine 3000 according to the manufacturer’s instruction (Invitrogen, catalog no: L3000-075). At 48 hours post- infection/transfection cell cultured were harvested, subjected to three freeze-and-thaw cycles. The ORFV recombinant expressing IAV-S HA was purified using plaque assay by selecting viral foci expressing GFP. After several rounds of plaque purification, the presence of HA gene and absence of ORFV121 gene was confirmed by PCR as described before (23, 25) and the insertion and integrity of the virus genome sequence was confirmed by whole genome sequencing using the Nextera XT DNA library preparation kit followed by sequencing on the Illumina MiSeq sequencing platform. Once the purified recombinant virus was obtained, the GFP selection gene was removed by using Cre recombinase treatment as described below. This recombinant is referred to as OV-HA throughout this manuscript.
Similarly, double gene expression vector containing the IAV-S HA and NP genes in ORFV121 and the ORFV127 gene loci (47), respectively, was generated by homologous recombination. Both ORFV121 and ORFV127 are virulence determinants that contribute to ORFV IA-82 virulence in the natural host (39, 47). For this, infection/transfection was performed by infecting OFTu cells with the OV-HA recombinant virus and transfecting with pUC57-127LR-SIV-NP-loxp-GFP plasmid. The recombinant virus was purified using plaque assay as described above and the GFP reporter gene was removed using the Cre recombinase system as described below. The resulting recombinant ORFV vector expressing the HA and NP gene is referred to as OV-HA-NP in this manuscript.
The Cre/loxP recombination system was used to remove the GFP reporter gene from the OV-HA or OV-HA-NP recombinants. A plasmid pBS185 CMV-Cre, carrying the cre gene under the hCMV promoter was a kind gift from Brian Sauer (48) (Addgene catalog number: 11916). OFTu cells were plated in a 24- well plate and 24h later transfected with 500 ng of the pBS185-CMV-Cre plasmid using Lipofectamine 3000 (Invitrogen, catalog num: L3000-075) according to the manufacturer’s instructions. Approximately 24h after transfections, cells were infected with ~ 1 MOI of the plaque purified recombinant viruses (OV-HA-GFP or OV-HA-NP-GFP). Approximately 48 h post-infection, recombinant viruses were harvested and subjected to a second passage in Cre-expressing cells. Next, two to three rounds of plaque assays were performed to select foci lacking GFP expression and to obtain reporterless OV-HA or OV-HA-NP recombinant viruses. Following markerless virus selection complete genome sequencing was performed to determine the integrity of ORFV and IAV-S sequences in the recombinant OV-HA and OV-HA-NP viruses.
Growth Curves
Replication kinetics of OV-HA and OV-HA-NP recombinant viruses were assessed in vitro in OFTu and STU cells. Briefly, OFTu and STU cells cultured in 12-well plates were inoculated with OV-HA or OV-HA-NP with an MOI of 0.1 (multistep growth curve) or 10 (single-step growth curve) and harvested at 6, 12, 24, 48, 72 hours post-infection (hpi). Virus titers in cell lysates were determined on each time point using Sperman and Karber’s method and expressed as tissue culture infectious dose 50 (TCID50) per milliliter (49).
Immunofluorescence
Immunofluorescence assay (IFA) was used to assess expression of the heterologous proteins by the OV-HA or the OV-HA-NP viruses as described previously (23). Briefly, OFTu cells were inoculated with each recombinant virus (MOI of 1) and fixed with 3.7% formaldehyde at 48 hours pi. Then, cells were permeabilized with 0.2% PBS-Triton X-100 for 10 min at room temperature. Another set of samples which were not permealized were also tested side-by-side to compare the expression pattern between permeabilized and non-permeabilized cells. Flag-tag specific mouse antibody (Genscript, catalog no: A100187) and HA-tag specific rabbit antibody (Cell Signaling, catalog no: 3724S) were used as primary antibodies to detect HA and NP protein respectively. Then, cells were incubated with Alexa fluor 594 goat anti-mouse IgG (H+L) secondary antibodies (Invitrogen, catalog no: A11005) or Alexa fluor 488 goat anti-rabbit IgG antibody and cells were observed under a fluorescence microscope.
Animal Immunization and Challenge Studies
Animal immunization challenge studies were conducted at South Dakota State University (SDSU) Animal Resource Wing (ARW), following the guidelines and protocols approved by the SDSU Institutional Animal Care and Use Committee (IACUC approval no. 17-018A). The immunogenicity of the two recombinant viruses (OV-HA and OV-HA-NP) was evaluated in 3-week old high-health pigs. A summary of experimental design is presented in Table 1. Twenty-four pigs, seronegative for IAV-S, were randomly allocated into three experimental groups as follows: Group 1, sham immunized (n=8); Group 2, OV-HA immunized (n=8); Group 3, OV-HA-NP immunized (n=8). The animals were acclimatized for one week before immunization. Immunization was performed by intramuscular injection of 2 ml of a virus suspension containing 107 TCID50/mL in MEM. All animals were immunized on day 0 and received a booster immunization on day 21 post-immunization. All animals were challenged intranasally on day 35 post-immunization with 5 mL virus inoculum containing 6 × 106 TCID50 of H1N1 A/Swine/OH/24366/2007 (H1N1) (50) per animal. Animals were monitored daily for clinical signs of IAV-S. Blood samples were taken from external jugular vein of pigs and the blood samples were processed to obtain serum and PBMC on days 0, 7, 14, 21, 28, 35, 38 and 42 days post-immunization. Nasal swabs were collected on days 0, 1, 3, 7 post-challenge. The experiment was terminated on day 42 post-immunization or 7 days post-challenge. The lungs were collected from euthanized animals during necropsy and examined grossly for pathologic changes by a pathologist blinded to study groups.
Virus Neutralization (VN) Assay
Virus neutralization titers in the serum samples were determined as described previously (51). Briefly, serum samples were heat inactivated for 30 minutes at 56°C. Two-fold serial dilutions of serum were incubated with 200 TCID50 of each IAV-S H1N1 isolate (A/Swine/OH/24366/2007, A/Swine/Iowa/A02424852/2020, A/Swine/South Dakota/A02156993/2018, A/Swine/Missouri/A02479312/2020, A/Swine/Michigan/A02524810/2020, A/Swine/Texas/A02245632/2020, A/Swine/Oklahoma/A02245707/2020, A/Swine/Minnesota/A01785306/2017, A/Swine/Iowa/A02479151/2020, A/Swine/Oklahoma/A02214419/2017, and A/Swine/South Dakota/A02524887/2020), at 37°C for 1 hour. The virus-serum mixture was then transferred to a 96-well plate pre-seeded with MDCK cells 24 h earlier. After 1 hour of adsorption, virus-serum mixture was removed and fresh DMEM containing 2 µg/mL of TPCK-treated trypsin was added to the cells. After 48-hour incubation at 37°C, cells were fixed with 80% acetone. Virus positive MDCK cells were detected by immunofluorescence assay using a mouse monoclonal antibody targeting nucleoprotein (NP) of influenza virus (IAV-NP HB-65 mAb; kindly provided by Drs. Eric Nelson and Steve Lawson, SDSU). The virus neutralization titer was defined as the reciprocal of the highest dilution of serum where there was complete inhibition of infection/replication as evidenced by absence of fluorescent foci. Appropriate positive and negative control samples were included in all the plates.
Hemagglutination Inhibition (HI) Assay
HI assay was performed according to the method described previously (51). Briefly, 2-fold serial dilutions (starting dilution 1:4) were prepared in PBS. Then 4 HA units of H1N1 A/Swine/OH/24366/2007 virus was added to the serum dilutions and incubated at room temperature for 1 hour. A solution of turkey red blood cells (0.5% RBCs in PBS) were added to the wells and incubated for 30 min at RT. The HI titer was calculated as the reciprocal of the highest dilution of sera that inhibited hemagglutination of turkey RBC.
Real-Time Reverse Transcriptase PCR (rRT-PCR)
Virus shedding in nasal secretions and viral load in lungs was evaluated by rRT-PCR. Lung tissues were homogenized using tissue homogenizer by adding 10 mL of DMEM in 1 g of lung tissue. Viral nucleic acid was extracted from the nasal swabs and lung tissue homogenates using the MagMax Viral RNA/DNA isolation Kit (Life Technologies). The rRT-PCR tests were performed at Animal Disease Research and Diagnostic Lab (ADRDL), SDSU, SD. Genome copy numbers per milliliter were determined based on the relative standard curve derived from four-parameter logistic regression analysis (R-square=0.9928, Root mean square error (RMSE)=1.0012).
Virus Isolation
Virus isolation was performed on the nasal swabs collected on day 0, 1, 3, and 7 post-challenge. Nasal swabs were filtered through a 0.22-micron filter and mixed with DMEM containing 2 µg/mL of TPCK-treated trypsin in 1:1 ratio. Then, 250 µL of this inoculum was added to 24-well plate containing MDCK cells. The cells were incubated for 1 hour at 37°C. After 1 hour adsorption, 250 µL of DMEM was added to the wells and plate was incubated for 48 hours. After 48 hours, cell lysate was harvested, and two more blind serial passages were performed. After the third passage, the supernatant was collected, and the cells were fixed with 80% acetone. Immunofluorescence assay (IFA) was performed using IAV-NP mAb (IAV-NP HB-65) as primary antibody and Alexa fluor 594 goat anti-mouse antibody as secondary antibody (Invitrogen, catalog no: A11005). IAV-S infected cells were identified based on the presence of fluorescent foci.
ELISA
IAV-S-specific IgG, IgG1a and IgG2a antibodies elicited by immunization with OV-HA or OV-HA-NP were assessed by whole virus ELISA. The antigen for coating the ELISA plates was prepared as described previously (52) with some modifications. Briefly, ultra-centrifugation of virus culture supernatant was performed through a 30% sucrose cushion using Optima-L 100K ultracentrifuge (Beckman Coulter) at 18,000 RPM for 1.5 hours. The virus pellet was resuspended in DMEM and subjected to UV inactivation using the CL1000 UV crosslinker. Determination of the optimal antigen concentration and dilution of secondary antibodies were carried out by checkerboard titration.
To detect IAV-S specific total IgG, Immulon 1B ELISA plates (ThermoFisher Scientific, catalog no: 3355) were coated with 250 ng/well of concentrated and UV inactivated IAV-S virus and incubated at 37°C for 2 hours. Then plates were washed three times with PBST (1X PBS with 0.5% Tween-20) and blocked with 200 µL/well of blocking solution (5% milk in PBST) and incubated overnight at 4°C. Then, the plates were washed three times with PBST. Serum samples diluted in blocking solution at the dilution of 1:100 was added, and the plates were incubated for 1 hr at room temperature (RT). After, three washes with PBST, 100 µL of biotinylated anti-pig IgG antibody (Bethyl, catalog no: A100-104) diluted in blocking buffer (1:4000) was added to the plate and incubated for 1 hr at RT. Following three washes, HRP-conjugated streptavidin (Thermo Scientific, catalog no: 21136) diluted in blocking solution (1:4000) was added to plates and incubated for 1 hr at RT. Plates were washed again for three times with PBST and 100 µL/well of 3,3ʹ,5,5ʹ-tetramethylbenzidine (TMB) substrate was added to the plates (KPL, catalog no: 5120-0047). Finally, the colorimetric reaction was stopped by adding 100 µL 1N HCl solution per well. Optical density (OD) values were measured at 450 nm using a microplate reader. Cut-off value was determined as mean OD of negative serum samples plus three times of standard deviation (mean + 3SD).
The levels of IgG1 and IgG2 in the serum samples were determined by end-point isotype ELISA. Serum samples collected on day 35 post-immunization were used for isotype ELISA. Briefly, Immulon 1B plates were coated with 250 ng/well of concentrated and UV inactivated IAV-S virus and then blocked with 5% milk prepared in PBST. The plates were incubated overnight at 4°C. Next day, two fold serial dilution of serum samples from 1:100 to 1:12800 were made in 5% milk and diluted serum samples were transferred to the plates coated with the whole virus SIV. After 1 hour of incubation, mouse anti-pig IgG1 (Biorad, catalog no: MCA635GA) or mouse anti-pig IgG2 antibody (Biorad, catalog no: MCA636GA) were added to the plates. Next, biotinylated anti-mouse antibody (KPL, catalog no: 5260-0048) was added and plates were further incubated for 1 hour at room temperature. Then, HRP-conjugated streptavidin (Thermo Scientific, catalog no: 21136) was added to the plates and incubated for 1 hr at RT. Finally, TMB substrate was added and then reaction was stopped by using 1N HCL as described above. Cut-off OD value was determined as mean OD of negative samples + 3SD. The highest dilution of the serum sample having the OD value higher than the cutoff OD value was defined as the end-point IgG1 or IgG2 titer of the serum sample.
Flow Cytometry
IAV-S-specific T-cell response elicited by ORFV recombinants was evaluated by an intracellular cytokine staining (ICS) assay for interferon gamma (IFN-γ) and T-cell proliferation assay. For IFN-γ expression assay, cryopreserved PBMCs collected on day 35 post-immunization (0 dpc) were thawed and seeded at a density of 5 x 105 cells/well in 96-well plate. Cells were stimulated with UV inactivated IAV-S at MOI of 1. Additionally, cells were stimulated with concanavalin (ConA: 2 μg/ml) (Sigma, catalog no: C0412) plus phytohemagglutinin (PHA: 5 μg/ml) (Sigma, catalog no: 61764) as positive control and cRPMI (RPMI with 10% FBS) was added to the negative control wells. Protein transport inhibitor, Brefeldin A (BD Biosciences, catalog no: 555029), was added 6 hours after stimulation and the cells were incubated for 12 hours prior to flow cytometric analysis. For the proliferation assay, PBMCs (35 dpi) were stained with 2.5 μM carboxyfluorescin succinimidyl ester (CFSE; in PBS) (BD Horizon, catalog no: 565082). CFSE stained cells were seeded at a density of 5 x 105 cells/well in 96-well plate. The cells were stimulated as described above. After stimulation, the cells were incubated for 4 days at 37°C with 5% CO2 prior to staining. Antibodies used for immunostaining the cells were: CD3+ (Mouse anti-pig CD3ε; Alexa Fluor 647; BD Pharmingen, catalog no: 561476), CD4+ (Primary antibody: Mouse anti-pig CD4, Monoclonal Antibody Center (WSU), catalog no: 74-12-4; secondary antibody: Goat anti-mouse IgG2b PE/Cy7, Southern Biotech, catalog no: 1090-17), CD8+ (Primary antibody: Mouse anti-pig CD8α, Monoclonal Antibody Center (WSU), catalog no: 76-2-11; secondary antibody: Goat anti-mouse IgG2a FitC, Southern Biotech, catalog no: 1080-02), IFN-γ (Anti-pig IFN-γ PE, BD Pharmingen, catalog no: 559812. The stained cells were analyzed using Attune NxT flow-cytometer. Results were corrected for background proliferation by subtracting mock-stimulated proliferation from the frequency of cells that responded under inactivated SIV stimulation. The percentage of responding cells was calculated as the percentage of total T cells (live CD3+ cells).
Statistical Analysis
Statistical analysis was performed using Graphpad Prism software. The normality of the data was tested using Shapiro-Wilk test. Comparison of means between the groups was done using two-way ANOVA for normal data or Kruskal Wallis test for non-normal data. Pairwise comparison was performed using Tukey multiple comparison test. P value of less than 0.05 was considered significant. Flow cytometry data was analyzed using Flow Jo software.
Results
Construction of ORFV Recombinants
The OV-HA recombinant virus was obtained by inserting the full-length HA gene of IAV-S (H1N1) into ORFV121 locus by homologous recombination between a transfer plasmid pUC57-121LR-SIV-HA-loxp-GFP and the parental ORFV strain IA82 (Figure 1A). The OV-HA-NP recombinant virus was obtained by inserting the full-length HA gene into ORFV121 locus and the NP gene into ORFV127 locus. The wild type ORFV strain IA82 was used to generate the OV-HA virus which served as a parental virus for generation of the OV-HA-NP recombinant (Figure 1B). Expression of HA was driven by the vaccina virus (VACV) I1L promoter (44) and expression of the NP gene was driven by the VACV vv7.5 promoter (45). After infection with the parental virus and transfection with the recombination plasmid, the recombinant viruses were obtained and selected. Several rounds of plaque assays were performed to obtain purified recombinant viruses. Once the recombinant viruses were purified and verified by PCR, the marker gene encoding for the green fluorescent protein (GFP) was removed by using the Cre recombinase system. Whole-genome sequencing of plaque purified recombinant viruses was performed after Cre recombinase treatment. Sequence analysis confirmed the integrity and identity of ORFV sequences, demonstrated the presence of HA gene, deletion of ORFV121 in OV-HA construct, the presence of HA and NP genes and deletion of ORFV121 and ORFV127 genes in OV-HA-NP construct (data not shown).
Replication Kinetics of OV-HA and OV-HA-NP Viruses In Vitro
Replication properties of both recombinant viruses (OV-HA and OV-HA-NP) were assessed in vitro in primary ovine fetal turbinate cells (OFTu) and primary swine turbinate cells (STU) using one-step and multi-step growth curves (Figure 1C). Cells were infected with an MOI of 0.1 or 10 and cell lysates were harvested at 6, 12, 24, 48, 72 hours post-infection. Both recombinants replicated efficiently in natural host OFTu cells. However, replication of OV-HA and OV-HA-NP viruses was markedly impaired in the STU cells (Figure 1C).
Expression of Heterologous Proteins by OV-HA and OV-HA-NP Recombinant Viruses
Expression of the HA protein and NP protein by OV-HA and/or OV-HA-NP viruses was confirmed by immunofluorescence assay (IFA) and flow-cytometry. As shown in the Figure 2A, OV-HA recombinant expressed high levels of HA and OV-HA-NP recombinant expressed high levels of HA and NP proteins (Figure 2A). Expression of HA and NP were also confirmed by flow cytometry (Figure 2C). The IFA was also performed in non-permeabilized cells. Both HA and NP proteins were detected in non-permeabilized cells; however, the levels of protein detected were slightly lower than in permeabilized cells (Figure 2B). As expected, this decrease was more evident for NP protein than for the HA protein. These findings suggest that while a great proportion of the HA protein expressed by both OV-HA and OV-HA-NP recombinant viruses localizes to the cell surface, and expression of the NP protein is mostly confined to the intracellular compartment.
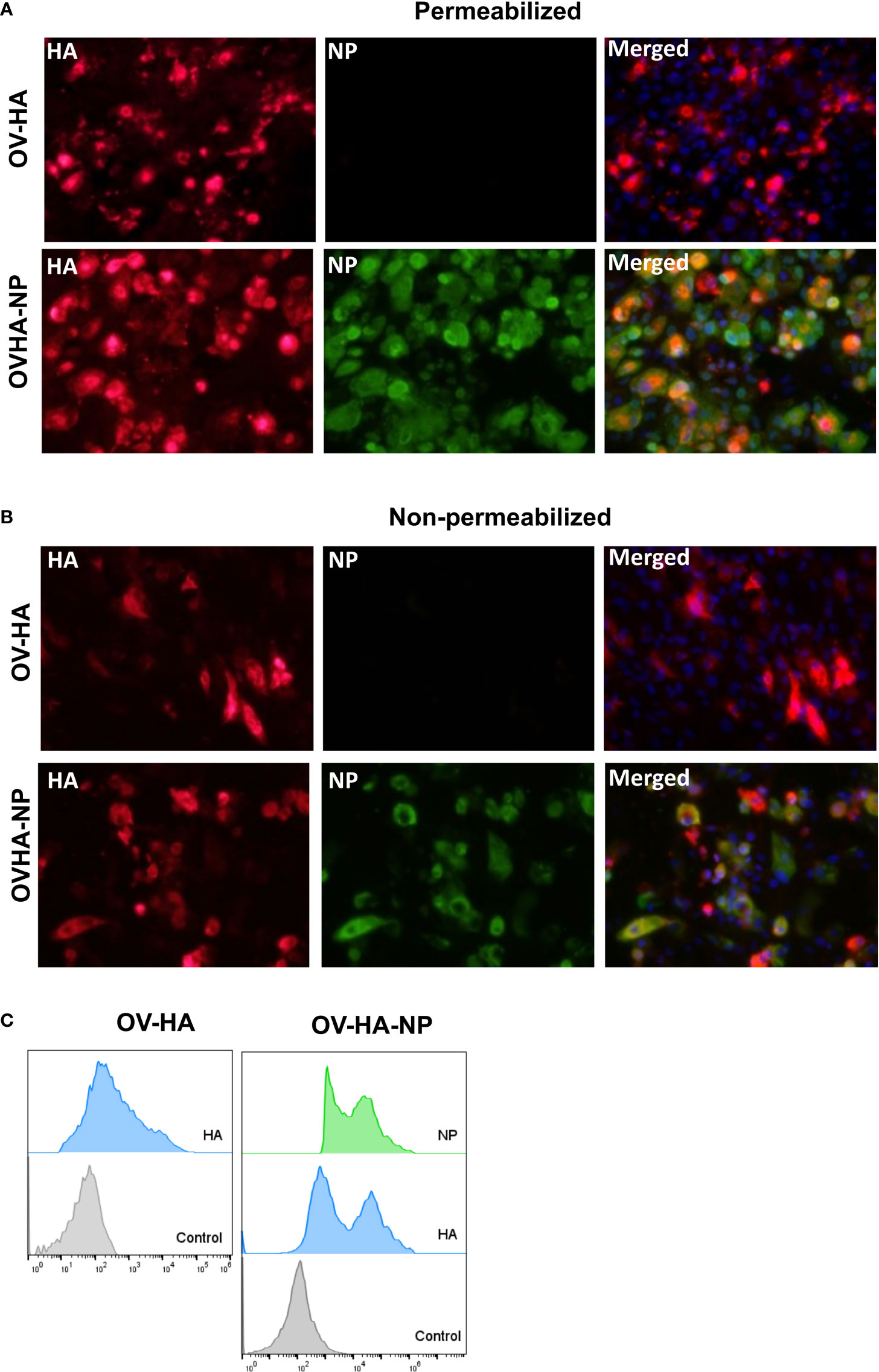
Figure 2 Expression of heterologous proteins by ORFV recombinants. (A) Immunofluorescence assay in permeabilized OFTu cells. Upper panel shows expression of HA protein and absence of NP protein in OV-HA recombinant. Lower panel shows expression of HA and NP protein by OV-HA-NP recombinant. (B) Immunofluorescence assay performed in non-permeabilized OFTu cells. Upper panel shows expression of HA by OV-HA recombinant and lower panel shows expression of HA and NP by OV-HA-NP recombinant. Blue fluorescence in merged images in panel A and B indicates nuclear staining by DAPI. (C) Expression of heterologous proteins by ORFV recombinants assessed by flow-cytometry. OFTu cells were infected with OV-HA, OV-HA-NP or Wild-type OV-IA82 as negative control. Infected cells were collected 48 hours post-infection, fixed and then stained with appropriate antibodies for flow cytometric analysis.
Immunogenicity of OV-HA and OV-HA-NP in Pigs
To assess the immunogenicity of OV-HA and OV-HA-NP, 4-week old, IAV-S negative, weaned piglets were immunized intramuscularly with two doses of OV-HA and OV-HA-NP at a 21 day interval (Figure 3A and Table 1). Antibody responses were evaluated using virus neutralization (VN) and hemagglutination inhibition (HI) assays. One week after the first immunization, neutralizing antibodies were detected primarily in OV-HA-NP group, however only one animal in OV-HA vaccinated group had detectable level of neutralizing antibodies at this time point (Figure 3B). An anamnestic increase in neutralizing antibody titers was seen in both vaccinated groups one week after the boost immunization (28 days post-immunization). After booster immunization, animals from both OV-HA and OV-HA-NP immunized groups maintained high level of neutralizing antibodies until the end of the experiment. (42 dpi, Figure 3B). Following challenge infection (day 35 pi) anamnestic neutralizing responses were observed in the OV-HA-immunized group, whereas neutralizing antibody titers remained unaltered in OV-HA-NP-immunized animals (Figure 3C and Table 3).
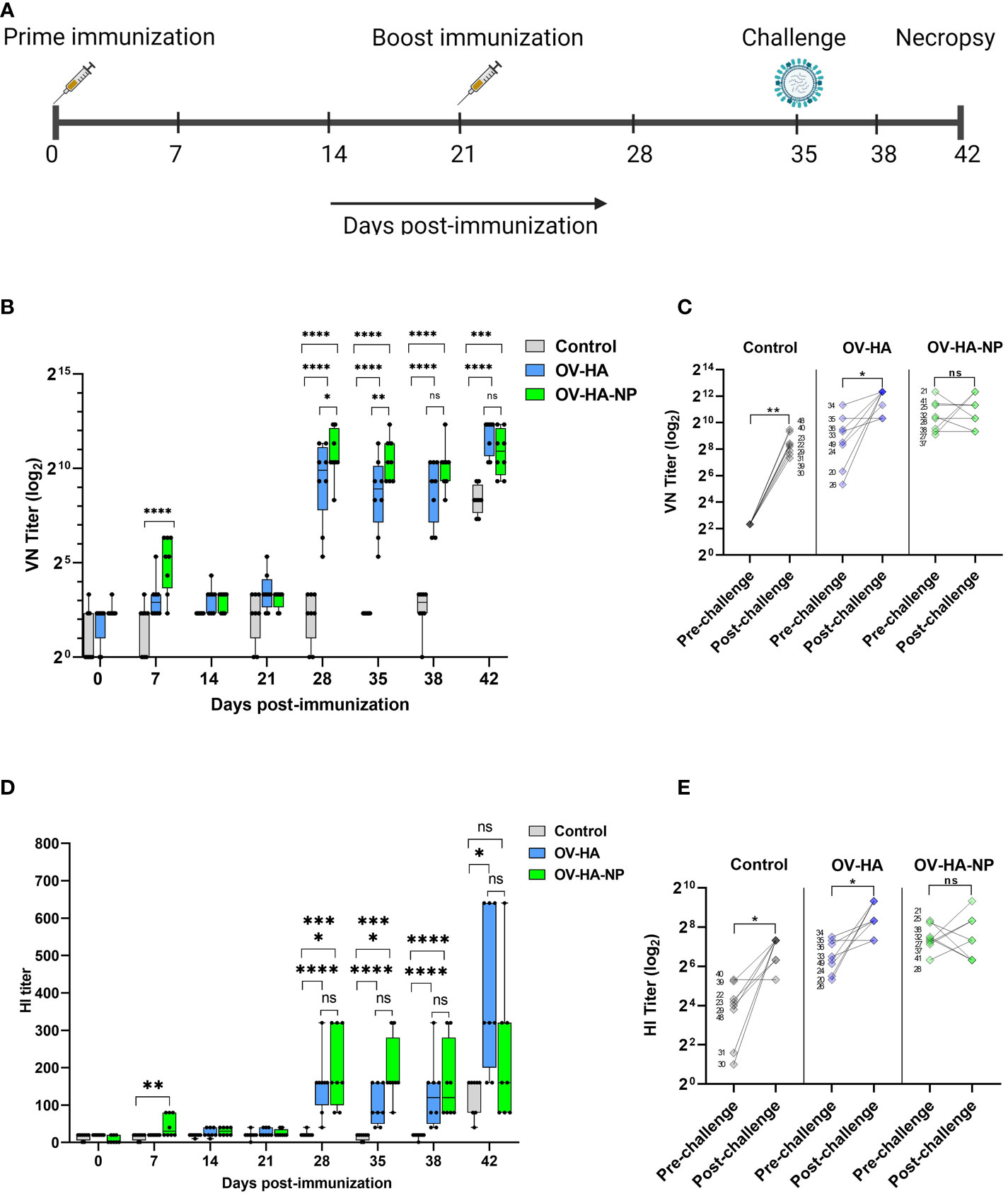
Figure 3 Immunization-challenge experiment design and humoral response to immunization. (A) A timeline of immunization-challenge experiment. (B) IAV-S specific neutralizing antibody response elicited by immunization with OV-HA and OV-HA-NP. (C) VN titer of individual animal measured on 0 dpc (pre-challenge) and 7 dpc (post-challenge). (D) IAV-S specific humoral immune response induced by OV-HA and OV-HA-NP assessed by hemagglutination inhibition (HI) assay. Red arrow heads represent the day of challenge. The error bars represent SEM. VN titer shown in logarithmic scale for effective visualization. HI titer shown in liner scale. P-values: *P < 0.05, **P < 0.01, ***P < 0.001, ****P < 0.0001. (E) HI titer of individual animal measured on 0 dpc (pre-challenge) and 7 dpc (post-challenge). Wilcoxson rank sum test was used for comparing means (pre-challenge vs. post-challenge) within the groups. *P < 0.05; **P < 0.01; ns, non-significant.
Serological responses were also measured using an hemagglutination inhibition (HI) assay. The presence of HI antibodies was detected in OV-HA-NP group on day 7 pi. Similar to the VN results, an anamnestic increase in HI antibody titers was observed one week after the booster immunization in both groups (Figure 3D). Interestingly, the HI titers in the OV-HA group increased significantly after challenge, which is more evident a week after challenge (42 dpi). Similar to the NA responses, such anamnestic increase in HI titers was not seen in OV-HA-NP-immunized animals (Figure 3E and Table 3). Overall, these results demonstrate that immunization with OV-HA and OV-HA-NP viruses elicited high IAV-S specific neutralizing and HI antibody responses in immunized pigs.
IAV-S-Specific IgG Isotype Responses Elicited by Immunization With OV-HA and OV-HA-NP Viruses
IAV-S specific IgG responses were measured using a whole virus ELISA. Low levels of IAV-S-specific total IgG antibodies were detected in OV-HA and OV-HA-NP immunized groups on day 21 pi (Figure 4A). Similar to VN and HI assay, significantly higher levels of IgG antibodies were observed a week following the booster immunization (day 28 pi). Thereafter consistently higher levels of IgG were detected in serum of both OV-HA and OV-HA-NP immunized groups until the end of the experiment (Figure 4A). The expression and delivery of the NP by the OV-HA-NP recombinant virus elicited higher levels of IgG antibodies in immunized pigs after the booster immunization on day 21 pi when compared to those observed in OV-HA-immunized animals (P < 0.0001, Figure 4A).
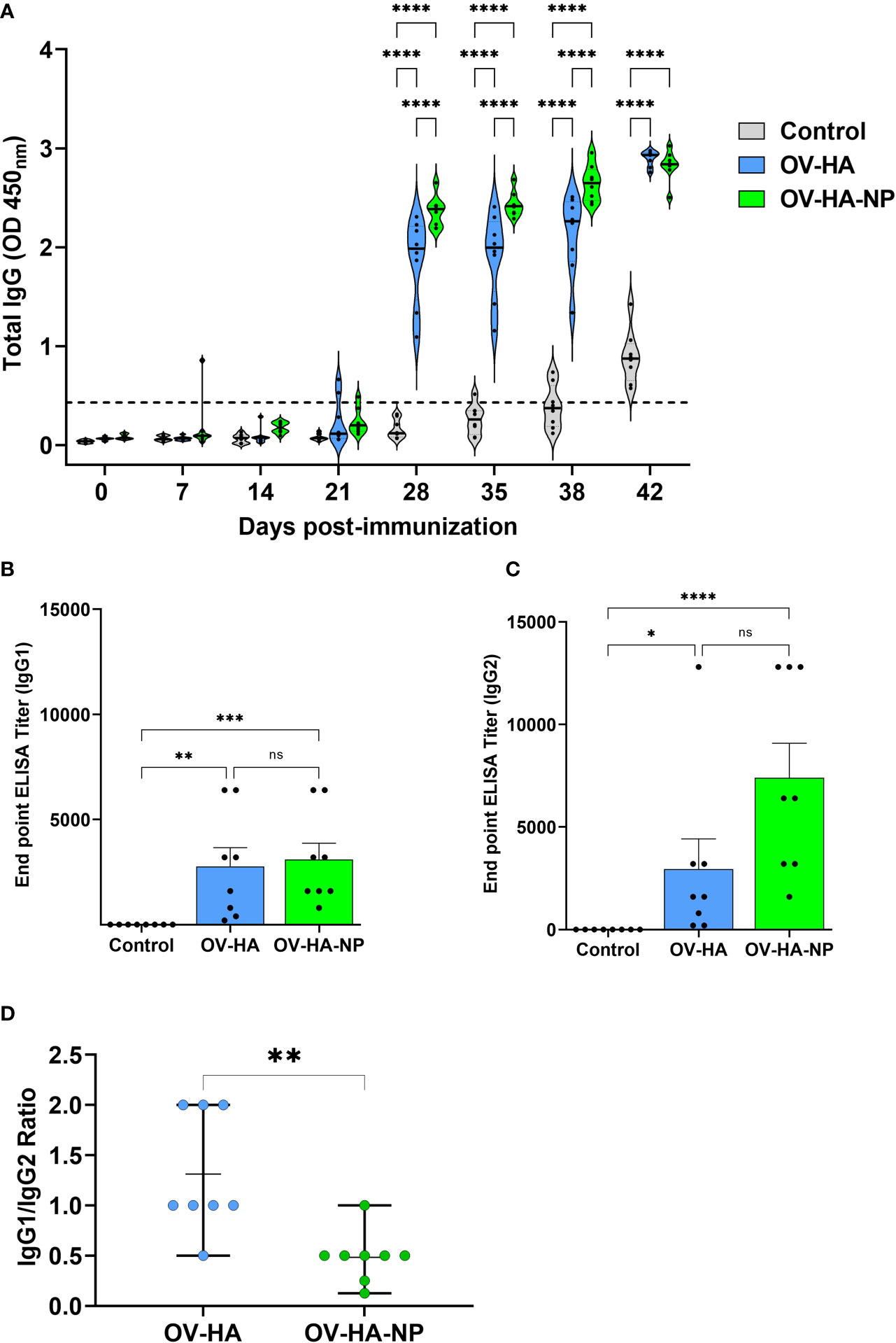
Figure 4 IAV-S specific IgG responses to immunization. (A) Total serum IgG level elicited by OV-HA and OV-HA-NP immunization at various time points were assessed by ELISA. Isotype ELISA demonstrating endpoint titers elicited by immunization at 35 days pi in serum was assessed for detecting specific (B) IgG1 and (C) IgG2 antibodies. (D) IgG1/IgG2 ratio in immunized animals. Each dot represents IgG1/IgG2 ratio of an individual animal. Middle bar represents mean ratio and upper and lower bars represent range. P-values: *P < 0.05, **P < 0.01, ***P < 0.001, ****P < 0.0001; ns, non-significant.
The endpoint titer of IgG1 and IgG2 isotype antibodies elicited by immunization with OV-HA and OV-HA-NP were determined by an isotype ELISA performed on serum samples collected on 35 days pi. Immunization with OV-HA and OV-HA-NP viruses elicited similar levels of IgG1 response, however, significantly higher titers of IgG2 antibodies were detected in OV-HA-NP-immunized animals when compared to IgG2 titers detected in OV-HA-immunized animals (Figures 4B, C). The ratio of Th2-associated IgG1 isotype and Th1-associated IgG2 isotype (IgG1/IgG2 ratio) calculated based on the endpoint titers detected in each group was 1.31 (i.e. > 1) for the OV-HA group and 0. 48 (i.e. <1) for the OV-HA-NP group (Figure 4D). The IgG1/IgG2 ratio in OV-HA-NP group was significantly lower than in the OV-HA group (P = 0.0048, Mann-Whitney test). Together these results suggest that the immune response in OV-HA group is mostly Th2 biased. In contrast, the immune response was Th1 biased on the OV-HA-NP group as indicated by higher levels of IgG2 antibodies in the serum of OV-HA-NP immunized animals.
Cellular Immune Responses Elicited by Immunization With OV-HA and OV-HA-NP
IAV-S-specific T-cell responses elicited by immunization with OV-HA and OV-HA-NP viruses was assessed on peripheral blood mononuclear cells (PBMCs) collected on day 35 pi (pre-challenge infection). The frequency of T-cell subsets secreting IFN-γ following re-stimulation with IAV-S was measured using intracellular cytokine staining (ICS) assays. Upon singlet selection, live/dead cell discrimination, IFN-γ expression by different T-cell subsets including total T-cells (CD3+), CD4+ T-cells (CD3+/CD4+), CD8+ T-cells (CD3+/CD4-CD8+), CD3+ cells expressing CD4 and CD8 marker (CD3+/CD4+/CD8+) and CD3+ cells lacking CD4 and CD8 marker (CD3+/CD4-/CD8-) were assessed. Animals immunized with either OV-HA or OV-HA-NP had significantly higher percentage of CD3+ T-cells secreting IFN-γ when compared to the non-immunized control animals (Figure 5A). Notably, within the vaccinated animals, OV-HA-NP group presented a significantly higher frequency of IFN-γ secreting CD3+ T-cells than the OV-HA group (P=0.0055). The animals in the OV-HA-NP group presented higher frequency of IFN-γ secreting CD3+/CD4+ T-cells, however, the differences between the groups were not statistically significant. Both immunized groups presented increased frequencies of CD3+/CD8+, CD3+/CD4-/CD8-, CD3+/CD4+/CD8+ IFN-γ secreting T-cell subsets when compared to the control sham-immunized group (Figure 5A).
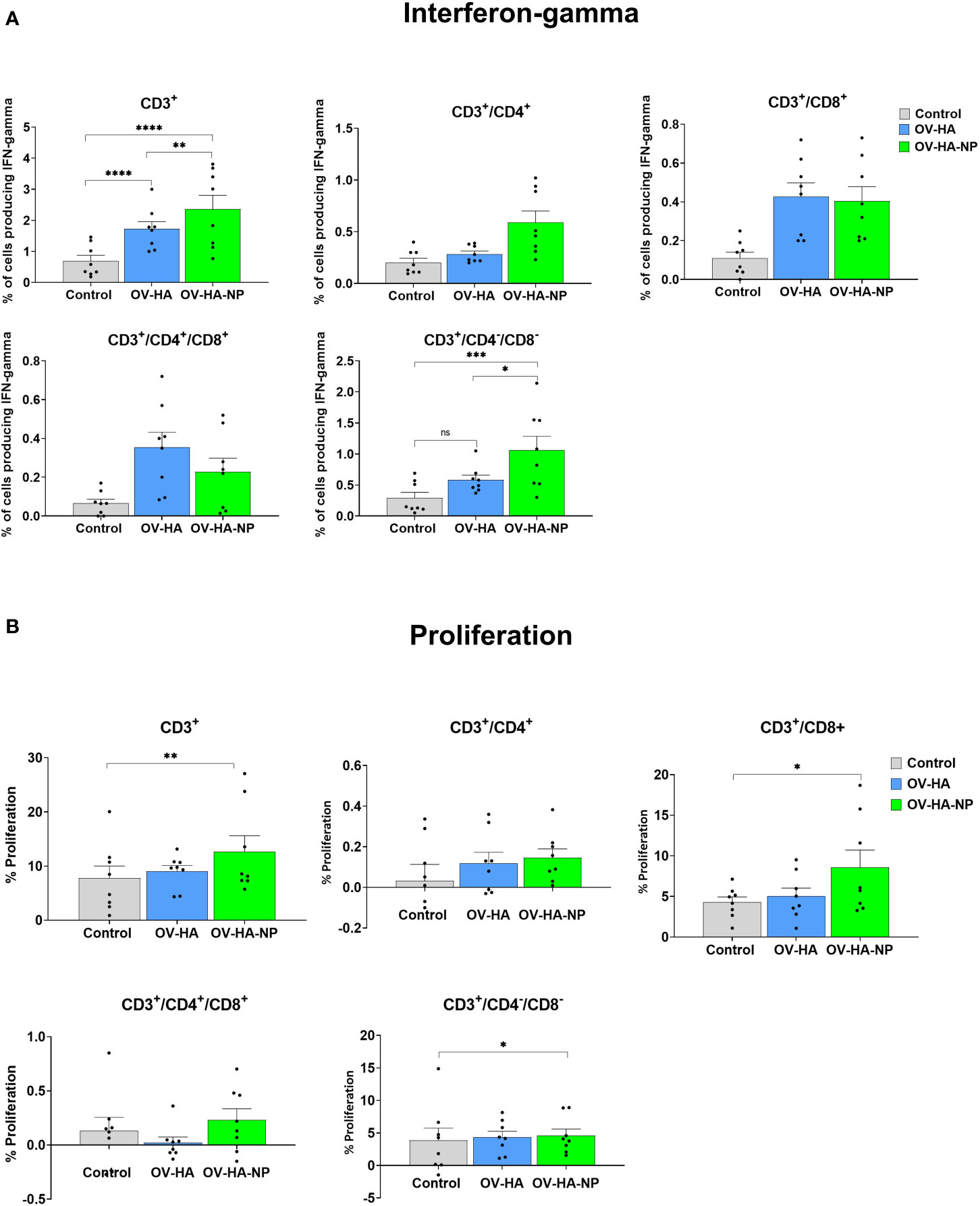
Figure 5 T-cell immune response to immunization. PBMCs isolated from pigs at 35 dpi following recall stimulation with inactivated IAV-S were analyzed for: (A) IFN-γ production by different T-cell subsets measured by flow cytometry assay; and (B) T-cells proliferation by CFSE dilution assay. Data represents group means and error bars represent SEM. P-values: *P < 0.05, **P < 0.01, ***P < 0.001, ****P < 0.0001; ns, non-significant.
IAV-S-specific T-cell responses were also evaluated by the carboxyfluorescein succinimidyl ester (CFSE) dilution assay to determine the specific T-cell subsets proliferating upon re-stimulation of PBMCs with inactivated IAV-S. As described above for the IFN-γ ICS, upon singlet selection and dead cell exclusion, proliferation by the major swine T-cell subsets was evaluated (Figure 5B). While proliferation of CD3+ T-cell subset was observed in animals immunized with OV-HA or OV-HA-NP, significant proliferation of CD3+ T-cells was observed in OV-HA-NP group upon recall stimulation (P=0.0095; Figure 5B). Additionally, a significant increase in the proliferation of CD3+/CD8+ T-cell subset was observed in the OV-HA-NP group (P=0.0217, Figure 5B). An increase in proliferation of CD3+/CD4+ T-cells was also observed (Figure 5B); however, the differences between the treatment groups were not statistically significant (Figure 5B). Overall, these results show that both OV-HA and OV-HA-NP group were able to induce IAV-S-specific T-cell responses in the immunized animals. As expected, CD3+ T-cell responses elicited by immunization with the OV-HA-NP construct was higher than those observed in animals immunized with the single gene OV-HA construct as evidenced by the increased number of IFN-γ secreting CD3+/CD4-/CD8- cells and increased proliferation of CD3+/CD4+/CD8+ and CD3+/CD4-/CD8- T-cell populations.
Protective Efficacy of OV-HA and OV-HA-NP Viruses Intranasal IAV-S Challenge
The protective efficacy of OV-HA and OV-HA-NP were evaluated upon intranasal challenge with IAV-S (after day 35 pi). Virus shedding was assessed in nasal secretions and viral load and pathology were evaluated in the lung. Nasal swabs were collected on days 0, 1, 3, and 7 post-challenge (pc) and IAV-S RNA levels were investigated in nasal secretions using real-time reverse transcriptase PCR (rRT-PCR). On day 1 pc, significantly lower IAV-S genome copy numbers – indicating reduced virus RNA shedding – was detected in both OV-HA and OV-HA-NP immunized groups when compared to the control sham immunized group (Figure 6A). Only two animals (2/8) in the OV-HA-NP group were positive for viral RNA on day 1 pc. On 3 dpc, while all animals in control group (8/8) were positive and presented high genome copy numbers of IAV-S in nasal secretions, only three animals (3/8) in OV-HA-NP were positive for viral RNA (Figure 6A). Notably, the amount of IAV-S RNA shed by OV-HA-NP-immunized animals were significantly lower than the amount shed by control or OV-HA immunized animals (Figure 6A). It is also important to note that animals in OV-HA group had significantly lower level of viral RNA than control group on day 3 pi (Figure 6A). On day 7 post-challenge, all animals (8/8) in the control sham-immunized group were still shedding IAV-S RNA in nasal secretions, while only two animals (2/8) in the OV-HA-immunized group were positive presenting low viral RNA copy numbers in nasal secretions. Notably, none of the animals in the OV-HA-NP-immunized group were shedding IAV-S in nasal secretions on day 7 pi (Figure 6A). These results demonstrate that immunization with OV-HA and OV-HA-NP resulted in decreased virus shedding and shorter duration of virus shedding in nasal secretions following intranasal IAV-S challenge. It is important to note that an incremental reduction in virus shedding was observed in OV-HA-NP-immunized animals when compared to OV-HA-immunized animals
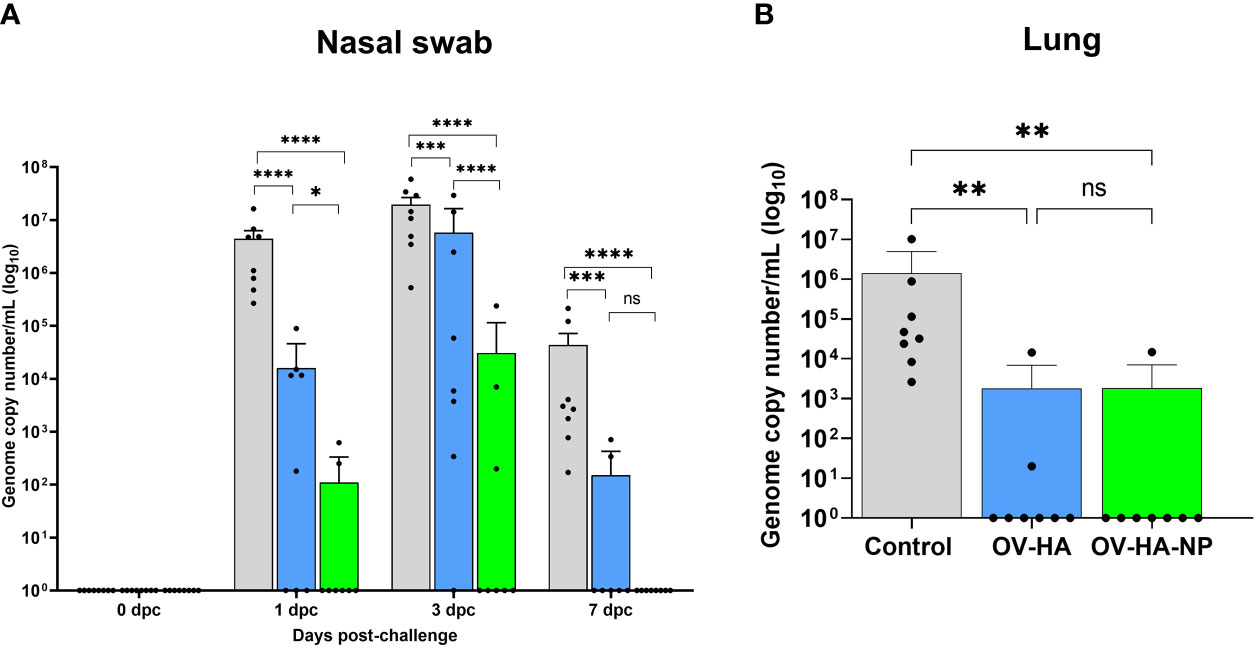
Figure 6 Protective efficacy of OV-HA and OV-HA-NP against IAV-S challenge. (A) IAV-S viral RNA shedding in the nasal swab was determined by RT-qPCR and expressed as log10 genome copy number per milliliter. (B) IAV-S viral load in the lung determined by RT-qPCR and expressed as log10 genome copy number per milliliter. Data represents group mean and error bars represent SEM. P-values: *P < 0.05, **P < 0.01, ***P < 0.001, ****P < 0.0001; ns, non-significant.
Shedding of infectious IAV-S was also assessed in nasal secretions collected on days 0, 1, 3, and 7 post-challenge. Each sample was subjected to three blind passages in MDCK cells. An immunofluorescence assay using an IAV-S NP-specific monoclonal antibody was performed on the third passage to confirm isolation of IAV-S. On day 1 pc, 4 (50%) animals in the control group were positive for IAV-S, while none of the animals from the OV-HA and OV-HA-NP group were positive on VI (Table 2). On day 3 pc, 7 (87.5%) animals were positive in the sham-immunized control group; 3 (37.5%) animals were positive in OV-HA immunized group and 1 (12.5%) animal was positive in OV-HA-NP-immunized group. Statistical analysis confirmed that there was a significant difference in the number of IAV-S positive animals between control group and OV-HA-NP group on 3 dpc (P= 0.0101 Fisher’s exact test) (Table 2). IAV-S was not isolated from any of the animals on day 7 post-challenge (Table 2). These results indicate that both OV-HA and OV-HA-NP recombinants were able to reduce virus replication and shedding in the immunized animals. Importantly, detection of infectious virus in only one out of eight animals in OV-HA-NP groups highlights the incremental protection provided by immunization of pigs this recombinant virus.
Viral load was assessed in the lung of control and immunized pigs on day 7 dpc by using rRT-PCR. While high amounts of IAV-S RNA were detected in the lung of animals in the control sham-immunized group, immunization with OV-HA or OV-HA-NP led to a marked decrease in viral load in the lung (Figure 6B). Notably, only one animal (1/8) in the OV-HA-NP group and two animals (2/8) in OV-HA group presented IAV-S RNA in lung, whereas all the animals in control group (8/8) were positive for IAV-S RNA. Significantly lower IAV-S RNA loads were detected in the lung of immunized animals when compared to control animals (Figure 6B).
In addition to viral loads, pathological changes were also evaluated in the lung of all animals in the study. At necropsy, macroscopic lesions in the lung were characterized by a pathologist who was blinded to the experimental groups. A summary of the gross lung lesions is provided on Table 3. All animals in the control group presented characteristic plum-colored consolidated areas mostly on the cranioventral areas and interstitial pneumonia. Mild lobular consolidation and interstitial pneumonia was present in 2 animals in OV-HA group and 2 animals in OV-HA-NP group. Importantly, these lesions were observed in animals presenting lower levels of neutralizing antibody titers (Table 3). Two animals that showed lung lesions in OV-HA group had the lowest VN titers on day 35 post-immunization (Titers 1:40 and 1:80). Similarly, two animal that showed lung lesions in OV-HA-NP group also had the lower VN titers when compared to the VN titers of other animals within that group (Table 3). Together these results indicate that immunization with ORFV-based vectors, provided good protection against intranasal homologous IAV-S challenge in pigs.
Presence of Cross-Reactive Antibodies in OV-HA or OV-HA-NP Immunized Pigs
We further asked if the antibodies induced by OV-HA or OV-HA-NP were able to neutralize recent IAV-S (H1N1) isolates belonging to different genetic clades. Ten different H1N1 strains isolated between 2017-2020 representing different IAV-S clades that are circulating globally (Table 4) were used in cross-neutralization assays. Phylogenetic trees were constructed based on amino acid sequences of HA (Figure 7A) and NP (Figure 7B) proteins to evaluate genetic relatedness of these ten viruses with vaccine and challenge virus used in this study. The HA and NP sequence used to construct OV-HA or OV-HA-NP was based on A/Swine/OH/511445/2007 and this HA/NP sequence share 100% identity with HA/NP sequence of the challenge virus (A/Swine/OH/24366/2007) and cluster together in the phylogenetic trees (Figures 7A, B and Table 4). The amino acid difference between vaccine HA sequence and ten isolates used for cross-neutralization assay ranges from 3.1% to 27%, whereas the amino acid sequence of NP is more conserved and differences between the isolates ranges from 2.2% to 3.4% (Table 4).
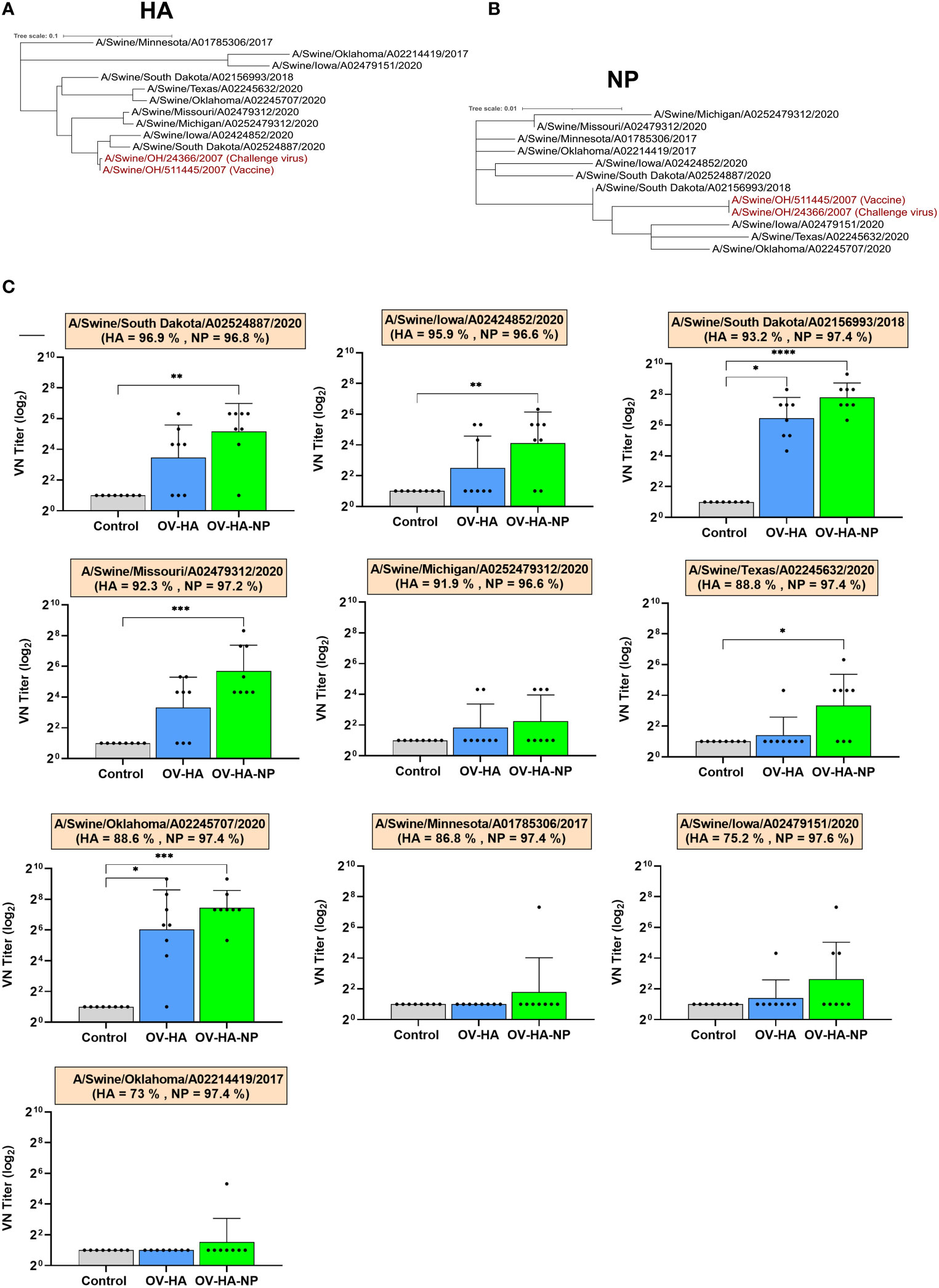
Figure 7 Cross-neutralization assay. (A) Phylogenetic tree based on HA amino acid sequence. Animo acid sequences were aligned using MUSCLE and trees were constructed using Maximum Likelihood method implemented in MEGA-X (53). (B) Phylogenetic tree based on amino acid sequence of NP protein. Sequences were aligned using MUSCLE and trees were constructed using Maximum Likelihood method. (C) Cross-neutralizing antibody titers against different IAV-S viruses in the serum samples collected on 35 days post-immunization. Each panel represents one virus isolate and the percentage of its HA/NP similarity with that of vaccine HA/NP is provided under the name of each isolate. Bar represent mean and error bar represent SEM. P-values: *P < 0.05, **P < 0.01, ***P < 0.001, ****P < 0.0001.
To further profile the breath of neutralizing activity, serum samples collected on day 35 day post-immunization was used in the cross-neutralization assay using the ten isolates described above. The serum from OV-HA-NP group presented neutralizing activity against six out of ten isolates, whereas the serum from OV-HA immunized animals neutralized two isolates (Figure 7C). The viruses that were neutralized by the serum from OV-HA-NP serum belong to gamma, gamma-2-beta-like, pdm and beta clades (Figure 7C and Table 4). The serum from OV-HA or OV-HA-NP group were able to neutralize H1N1 isolates that share more than 92% HA sequence similarity with the vaccine HA sequence. An exception to this is A/Swine/Oklahoma/A02245707/2020, which shares only 88.6% amino acid identity to the vaccine HA sequence but is still neutralized by the serum from both OV-HA and OV-HA-NP group. It is important to note that the cross-neutralizing antibody titers were low to moderate when compared to the titers against the challenge virus. Overall, these results show that antibody induced by OV-HA or OV-HA-NP have cross-neutralizing activities which can neutralize viruses that differ antigenically from the vaccine or challenge virus used in this study.
Discussion
In this study we explored the potential of ORFV recombinants expressing the HA or both HA and NP proteins of IAV-S in providing protection against intranasal challenge infection in swine. Previous work from our group have shown that rational vector design by deleting well-characterized immunomodulatory genes of ORFV is useful in developing highly effective vaccine delivery platforms resulting in safe and highly immunogenic vaccine candidates. One of the well characterized ORFV IMPs is ORFV121, which encodes an NF-κB inhibitor that determines ORFV virulence and pathogenesis in the natural host (39). We have developed vaccine candidates for porcine epidemic diarrhea virus (PEDV) and rabies virus (RabV) by inserting appropriate protective antigens (spike glycoprotein for PEDV; rabies glycoprotein for RabV) in the ORFV121 gene locus. Given the immunogenicity and safety profile of the OV-PEDV-S and OV-RABV-G recombinant virus in swine, here we constructed an OV-HA recombinant by inserting the HA gene of IAV-S virus in ORFV121 locus. Moreover, to potentially enhance the protective responses elicited by the vaccine candidate we generated a second recombinant virus expressing both IAV-S NP and HA proteins. For this, another well-characterized ORFV IMP, the ORFV127 was selected as an insertion site for the NP gene. ORFV127 encodes a viral IL-10 homolog (16, 54), which is known to have anti-inflammatory and immunosuppressive activities that may favor immune evasion of orf virus (55, 56). Most importantly, the protein encoded by ORFV127 is known to contribute to ORFV virulence in the natural host (47). Using this approach we tested the hypothesis that simultaneous deletion of two ORFV IMP genes ORFV121 and ORFV127 and concurrent insertion of two highly immunogenic protective antigens of IAV-S (HA and NP) would enhance the immunogenicity of the recombinant virus in swine and provide higher protective efficacy from IAV-S challenge. While the data presented here show that both recombinants OV-HA and OV-HA-NP induced robust immune response against IAV-S in pigs, the insertion of the NP gene in the OV-HA-NP recombinant virus resulted in a slight increase in the immunogenicity of the OV vector. This is likely due to antibody and/or T cell responses directed to the NP gene, however, deletion of ORFV127 from the vector backbone may also have contributed to these results.
Following challenge infection, we observed interesting differences in the antibody response elicited by OV-HA and OV-HA-NP immunization. As expected, intranasal challenge with IAV-S in sham immunized pigs resulted in anamnestic VN response. Notably, in the immunized groups the VN antibody titers increased by a greater magnitude in the OV-HA group than in the OV-HA-NP group. In the OV-HA group, the geometric mean VN titers were 380.5 and 3319.9 on days 0 and 7 pc respectively, indicating a 9-fold increase in the VN titer after challenge. Whereas in OV-HA-NP group, the geometric mean VN titers were 1395.8 and 1810.19 on days 0 and 7 pc respectively, which is only a modest 1.2-fold increase in VN titers pc. Importantly, the VN titers in OV-HA-NP immunized group increased only in the two animals that had the lowest VN titer on day 0 pc. The VN titers in the remaining 6 animals in the OV-HA-NP group remained constant following challenge infection. These results demonstrate that immunization with the OV-HA-NP recombinant virus provided robust immune protection against intranasal IAV-S challenge, and most animals did not present a secondary antibody response to the to the challenge virus.
The importance of T-cells in influenza virus clearance and their cross-reactive potential has been well documented (57, 58). In this context, CD4+ T-cells help with activation, differentiation and antibody production by virus-specific B cells (59). Additionally, CD4+ helper cells also play an important role in CD8+ cytotoxic T cell activation. Activated CD8+ cytotoxic T-cells function in virus clearance by killing infected cells (60). The NP protein of influenza virus is known to contain several immunologically dominant T-cell epitopes and it is the main antigen recognized by cytotoxic T- lymphocytes (CTL) during influenza A virus infections (42, 61–64). The Immune Epitope Database and Analysis Resource, a manually curated database of experimentally characterized immune epitopes, has recorded 248 T-cells epitopes for nucleoprotein (NP) of influenza virus. Given that NP is relatively conserved among influenza viruses, including in IAV-S, this protein has been one of the target viral antigens for the development of universal influenza vaccine candidates. Because of these important immunological properties, we have developed and evaluated the OV-HA-NP construct expressing both HA and NP proteins. We found that cell mediated immune responses were slightly increased by co-delivery and expression of IAV-S HA and NP by OV-HA-NP in pigs when compared to OV-HA group. A significantly higher frequency of CD3+ T-cells proliferated and expressed IFN-γ upon re-stimulation with IAV-S in the OV-HA-NP-immunized group. Importantly, immunization with OV-HA-NP resulted in an increased frequency of CD3+/CD8+ T cells upon restimulation with IAV-S. While overall T-cell responses were higher in OV-HA-NP group, an increase in T-cell response was also seen in OV-HA group when compared to the sham immunized group, as evidenced by increase in IFN-γ secreting CD3+ T-cell population following antigen stimulation. This can be explained by the presence of several T-cells epitopes in the IAV HA protein, the majority of which have been identified as CD4+ T-cell epitopes (65, 66).
Depending upon the type of antigenic stimulation, CD4+ helper T-cell precursors (Tho) can either differentiate into Th1- or Th2- helper cells. Th1 cells secrete several cytokines including IFN-γ and IL-12 which help in cell mediated immunity, whereas Th2 cells secrete cytokines like IL-4, IL-6 which contribute to antibody mediated immunity (40, 67). Importantly, IgG isotype expression is also controlled by the different cytokines (68, 69). In pigs, IFN-γ enhances production of IgG2 isotype and hence this IgG isotype is considered to be associated with Th1 immune response. On the other hand, cytokines like IL-4, IL-10 induce secretion of IgG1 and are known to be associated with Th2 immune response (70). Thus, the ratio of IgG1:IgG2 can be used to infer Th1/Th2 bias in response to vaccination. In this study, we found a higher level of IgG1 in pigs immunized with OV-HA recombinant (IgG1:IgG2 >1, Th2 bias), which suggests a bias towards antibody-mediated immunity in this group. Conversely, in OV-HA-NP group, the levels of IgG2 were higher (IgG1:IgG2 <1, Th1 bias), which suggests a bias towards cell-mediated immunity in this group. Because of the significant role of IAV-S HA on immunity and the incremental benefit observed here after the inclusion of NP in the OV vector, future multivalent OV vector constructions should also consider inclusion of the NP and, perhaps, of other internal IAV-S genes.
This study further demonstrates the utility of ORFV as a vaccine delivery platform in swine. The study also shows that two ORFV IMP encoding genes (ORFV121 and ORFV127) can be deleted simultaneously from the virus genome to efficiently delivery at least two viral antigens in swine. One of the advantages of ORFV-based vectors is that the same vector can be used repeatedly for prime-boost regimens. This is important because pre-existing immunity precludes the use of many vector platforms for vaccine delivery. The humoral immune response data presented here shows that a boost effect was induced after second immunization. In fact, previous findings from our lab show that similar effect can be observed even after three immunizations with ORFV. Additionally, presence of cross-neutralizing antibodies predominantly in OV-HA-NP group that can neutralize IAV-S belonging to different clades has an important implication for future development of broadly protective vaccine candidate. In the future, we plan to use the HA gene from other IAV-S subtypes (H1N2, H3N2) to develop multivalent vaccine candidates and evaluate heterosubtypic protection. The analysis of secretory IgA immune response, which play an important role in providing mucosal immune response is lacking in this study. Future studies involving detailed analysis of mucosal immune response elicited by ORFV-based constructs and challenge infection with heterologous IAV strains are warranted. Nonetheless, results presented here demonstrate that ORFV-based vectors can be important tools to develop improved vaccine candidates to effectively control IAV-S infections in swine.
Data Availability Statement
All data is presented in the manuscript. Further inquiries can be directed to the corresponding author.
Ethics Statement
The immunization challenge animal study was conducted at South Dakota State University (SDSU) Animal Resource Wing (ARW), following the guidelines and protocols approved by the SDSU Institutional Animal Care and Use Committee (IACUC approval no. 17-018A).
Author Contributions
LJ conducted the experiments, performed data analysis, generated figures and tables, and helped writing the manuscript. DK and PP helped with histopathology. SD and GR contributed to the materials and reagents. DD conceived, designed, supervised the study, helped with data interpretation, and writing of the manuscript. All authors contributed to the article and approved the submitted version.
Funding
This work was supported by AFRI Foundational and Applied Science Program (grant no. 2017-67015-32034/project accession no. NYCV478904) from the USDA National Institute of Food and Agriculture.
Conflict of Interest
DD has patent on orf virus vector (patent no. 11013798).
The remaining authors declare that the research was conducted in the absence of any commercial or financial relationships that could be construed as a potential conflict of interest.
Publisher’s Note
All claims expressed in this article are solely those of the authors and do not necessarily represent those of their affiliated organizations, or those of the publisher, the editors and the reviewers. Any product that may be evaluated in this article, or claim that may be made by its manufacturer, is not guaranteed or endorsed by the publisher.
Acknowledgments
We acknowledge Animal Resource Wing (ARW) SDSU for their assistance in animal experiments. We thank the Cornell BRC Flow Cytometry Facility at the Cornell Institute of Biotechnology for the use of flow cytometers for data acquisition. We also would like to thank Bishwas Sharma, Maureen Hoch Vieira Fernandes, Jessica Caroline Gomes Noll, Gabriela Mansano do Nascimento, and Steve Lawson for their help with sample collection.
References
2. Ma W. Swine Influenza Virus: Current Status and Challenge. Virus Res (2020) 288:1–8. Elsevier B.V. doi: 10.1016/j.virusres.2020.198118
3. Anderson TK, Chang J, Arendsee ZW, Venkatesh D, Souza CK, Kimble JB, et al. Swine Influenza A Viruses and the Tangled Relationship With Humans. Cold Spring Harb Perspect Med (2020) 11(3):a038737. doi: 10.1101/cshperspect.a038737
4. USDA. Influenza A Virus in Swine Surveillance. United States Department of Agriculture (2020). Accessed from: https://www.aphis.usda.gov/animal_health/animal_dis_spec/swine/downloads/fy2020quarter4swinereport.pdf.
5. OIE. Swine Influenza. World Organization for Animal Health (2019). Accessed from: https://www.oie.int/app/uploads/2021/03/swine-influenza.pdf.
6. Vincent AL, Ma W, Lager KM, Janke BH, Richt JA. Swine Influenza Viruses. A North American Perspective. Adv Virus Res (2008) 72:127–54. Academic Press. doi: 10.1016/S0065-3527(08)00403-X
7. Chamba Pardo FO, Alba-Casals A, Nerem J, Morrison RB, Puig P, Torremorell M. Influenza Herd-Level Prevalence and Seasonality in Breed-to-Wean Pig Farms in the Midwestern United States. Front Vet Sci (2017) 4:11. doi: 10.3389/fvets.2017.00167
8. CFSH. Swine Influenza. The Center for Food Security and Public Health (2016). Accessed from: https://www.cfsph.iastate.edu/Factsheets/pdfs/swine_influenza.pdf.
9. Vincent AL, Perez DR, Rajao D, Anderson TK, Abente EJ, Walia RR, et al. Influenza A Virus Vaccines for Swine. Vet Microbiol (2017) 206:35–44. doi: 10.1016/j.vetmic.2016.11.026
10. Vincent AL, Lager KM, Janke BH, Gramer MR, Richt JA. Failure of Protection and Enhanced Pneumonia With a US H1N2 Swine Influenza Virus in Pigs Vaccinated With an Inactivated Classical Swine H1N1 Vaccine. Vet Microbiol (2008) 126:310–23. doi: 10.1016/j.vetmic.2007.07.011
11. Genzow M, Goodell C, Kaiser TJ, Johnson W, Eichmeyer M. Live Attenuated Influenza Virus Vaccine Reduces Virus Shedding of Newborn Piglets in the Presence of Maternal Antibody. Influenza Other Respir Viruses (2018) 12:353–9. doi: 10.1111/irv.12531
12. Sharma A, Zeller MA, Li G, Harmon KM, Zhang J, Hoang H, et al. Detection of Live Attenuated Influenza Vaccine Virus and Evidence of Reassortment in the U.S. Swine Population. J Vet Diagn Investig (2020) 32:301–11. doi: 10.1177/1040638720907918
13. ICTV. Virus Taxonomy: Online (10th) Report of the International Committee on Taxonomy of Viruses. International Committee on Taxonomy of Viruses (2017).
14. Spyrou V, Valiakos G. Orf Virus Infection in Sheep or Goats. Vet Microbiol (2015) 181:178–82. doi: 10.1016/j.vetmic.2015.08.010
16. Delhon G, Tulman ER, Afonso CL, Lu Z, de la Concha-Bermejillo A, Lehmkuhl HD, et al. Genomes of the Parapoxviruses Orf Virus and Bovine Papular Stomatitis Virus. J Virol (2004) 78:168–77. doi: 10.1128/JVI.78.1.168-177.2004
17. Weber O, Knolle P, Volk H-D, Weber O, Knolle P, Volk H-D, et al. Immunomodulation by Inactivated Orf Virus (ORFV) - Therapeutic Potential. In: Mercer A, Schmidt A, Weber O, editors. Basel: PoxvirusesFirst. Birkhäuser Basel (2007). p. 297–310.
18. Weber O, Mercer AA, Friebe A, Knolle P, Volk H-D. Therapeutic Immunomodulation Using a Virus–the Potential of Inactivated Orf Virus. Eur J Clin Microbiol Infect Dis (2013) 32:451–60. doi: 10.1007/s10096-012-1780-x
19. Amann R, Rohde J, Wulle U, Conlee D, Raue R, Martinon O, et al. A New Rabies Vaccine Based on a Recombinant Orf Virus (Parapoxvirus) Expressing the Rabies Virus Glycoprotein. J Virol (2012) 87:1618–30. doi: 10.1128/JVI.02470-12
20. Henkel M, Planz O, Fischer T, Stitz L, Rziha H-J. Prevention of Virus Persistence and Protection Against Immunopathology After Borna Disease Virus Infection of the Brain by a Novel Orf Virus Recombinant. J Virol (2005) 79:314–25. doi: 10.1128/JVI.79.1.314-325.2005
21. Rohde J, Amann R, Rziha H-J. New Orf Virus (Parapoxvirus) Recombinant Expressing H5 Hemagglutinin Protects Mice Against H5N1 and H1N1 Influenza A Virus) New Orf Virus (Parapoxvirus) Recombinant Expressing H5 Hemagglutinin Protects Mice Against H5N1 and H1N1 Influenza A Virus. PloS One (2013) 8:83802. doi: 10.1371/journal.pone.0083802
22. Rohde J, Schirrmeier H, Granzow H, Rziha H-J. A New Recombinant Orf Virus (ORFV, Parapoxvirus) Protects Rabbits Against Lethal Infection With Rabbit Hemorrhagic Disease Virus (RHDV). Vaccine (2011) 29:9256–64. doi: 10.1016/j.vaccine.2011.09.121
23. Hain KS, Joshi LR, Okda F, Nelson J, Singrey A, Lawson S, et al. Immunogenicity of a Recombinant Parapoxvirus Expressing the Spike Protein of Porcine Epidemic Diarrhea Virus. J Gen Virol (2016) 97:2719–31. doi: 10.1099/jgv.0.000586
24. Joshi LR, Okda FA, Singrey A, Maggioli MF, Faccin TC, Fernandes MHV, et al. Passive Immunity to Porcine Epidemic Diarrhea Virus Following Immunization of Pregnant Gilts With a Recombinant Orf Virus Vector Expressing the Spike Protein. Arch Virol (2018) 163:2327–35. doi: 10.1007/s00705-018-3855-1
25. Martins M, Joshi LR, Rodrigues FS, Anziliero D, Frandoloso R, Kutish GF, et al. Immunogenicity of ORFV-Based Vectors Expressing the Rabies Virus Glycoprotein in Livestock Species. Virology (2017) 511:229–39. doi: 10.1016/j.virol.2017.08.027
26. Dory D, Fischer T, Béven V, Cariolet R, Rziha H-J, Jestin A. Prime-Boost Immunization Using DNA Vaccine and Recombinant Orf Virus Protects Pigs Against Pseudorabies Virus (Herpes Suid 1). Vaccine (2006) 24:6256–63. doi: 10.1016/j.vaccine.2006.05.078
27. Voigt H, Merant C, Wienhold D, Braun A, Hutet E, Le Potier MF, et al. Efficient Priming Against Classical Swine Fever With a Safe Glycoprotein E2 Expressing Orf Virus Recombinant (ORFV VrV-E2). Vaccine (2007) 25:5915–26. doi: 10.1016/j.vaccine.2007.05.035
28. Fischer T, Planz O, Stitz L, Rziha H-J. Novel Recombinant Parapoxvirus Vectors Induce Protective Humoral and Cellular Immunity Against Lethal Herpesvirus Challenge Infection in Mice. J Virol (2003) 77:9312–23. doi: 10.1128/JVI.77.17.9312-9323.2003
29. Haig DM, McInnes CJ. Immunity and Counter-Immunity During Infection With the Parapoxvirus Orf Virus. Virus Res (2002) 88:3–16. doi: 10.1016/S0168-1702(02)00117-X
30. Mercer AA, Yirrell DL, Reid HW, Robinson AJ. Lack of Cross-Protection Between Vaccinia Virus and Orf Virus in Hysterectomy-Procured, Barrier-Maintained Lambs. Vet Microbiol (1994) 41:373–82. doi: 10.1016/0378-1135(94)90033-7
31. Fleming SB, Anderson IE, Thomson J, Deane DL, McInnes CJ, McCaughan CA, et al. Infection With Recombinant Orf Viruses Demonstrates That the Viral Interleukin-10 Is a Virulence Factor. J Gen Virol (2007) 88:1922–7. doi: 10.1099/vir.0.82833-0
32. Seet BT, McCaughan CA, Handel TM, Mercer A, Brunetti C, McFadden G, et al. Analysis of an Orf Virus Chemokine-Binding Protein: Shifting Ligand Specificities Among a Family of Poxvirus Viroceptors. Proc Natl Acad Sci USA (2003) 100:15137–42. doi: 10.1073/pnas.2336648100
33. Deane D, McInnes CJ, Percival A, Wood A, Thomson J, Lear A, et al. Orf Virus Encodes a Novel Secreted Protein Inhibitor of Granulocyte-Macrophage Colony-Stimulating Factor and Interleukin-2. J Virol (2000) 74:1313–20. doi: 10.1128/JVI.74.3.1313-1320.2000
34. McInnes CJ, Wood AR, Mercer AA. Orf Virus Encodes a Homolog of the Vaccinia Virus Interferon-Resistance Gene E3L. Virus Genes (1998) 17:107–15. doi: 10.1023/A:1026431704679
35. Westphal D, Ledgerwood EC, Hibma MH, Fleming SB, Whelan EM, Mercer AA. A Novel Bcl-2-Like Inhibitor of Apoptosis Is Encoded by the Parapoxvirus ORF Virus. J Virol (2007) 81:7178–88. doi: 10.1128/JVI.00404-07
36. Diel DG, Delhon G, Luo S, Flores EF, Rock DL. A Novel Inhibitor of the NF-{Kappa}B Signaling Pathway Encoded by the Parapoxvirus Orf Virus. J Virol (2010) 84:3962–73. doi: 10.1128/JVI.02291-09
37. Diel DG, Luo S, Delhon G, Peng Y, Flores EF, Rock DL. A Nuclear Inhibitor of NF-kappaB Encoded by a Poxvirus. J Virol (2011) 85:264–75. doi: 10.1128/JVI.01149-10
38. Khatiwada S, Delhon G, Nagendraprabhu P, Chaulagain S, Luo S, Diel DG, et al. A Parapoxviral Virion Protein Inhibits NF-κb Signaling Early in Infection. PloS Pathog (2017) 13:e1006561. doi: 10.1371/journal.ppat.1006561
39. Diel DG, Luo S, Delhon G, Peng Y, Flores EF, Rock DL. Orf Virus ORFV121 Encodes a Novel Inhibitor of NF-kappaB That Contributes to Virus Virulence. J Virol (2011) 85:2037–49. doi: 10.1128/JVI.02236-10
40. Stanekov Z, Varekov E. Conserved Epitopes of Influenza A Virus Inducing Protective Immunity and Their Prospects for Universal Vaccine Development. Virol J (2010) 7:1–13 BioMed Central. doi: 10.1186/1743-422X-7-351
41. Knossow M, Gaudier M, Douglas A, Barrère B, Bizebard T, Barbey C, et al. Mechanism of Neutralization of Influenza Virus Infectivity by Antibodies. Virology (2002) 302:294–8. doi: 10.1006/viro.2002.1625
42. Grant E, Wu C, Chan KF, Eckle S, Bharadwaj M, Zou QM, et al. Nucleoprotein of Influenza A Virus Is a Major Target of Immunodominant CD8 + T-Cell Responses. Immunol Cell Biol (2013) 91:184–94. doi: 10.1038/icb.2012.78
43. Vincent AL, Swenson SL, Lager KM, Gauger PC, Loiacono C, Zhang Y. Characterization of an Influenza A Virus Isolated From Pigs During an Outbreak of Respiratory Disease in Swine and People During a County Fair in the United States. Vet Microbiol (2009) 137:51–9. doi: 10.1016/j.vetmic.2009.01.003
44. Liu X, Kremer M, Broyles SS. A Natural Vaccinia Virus Promoter With Exceptional Capacity to Direct Protein Synthesis. J Virol Methods (2004) 122:141–5. doi: 10.1016/j.jviromet.2004.08.009
45. Chakrabarti S, Sisler JR, Moss B. Compact, Synthetic, Vaccinia Virus Early/Late Promoter for Protein Expression. Biotechniques (1997) 23:1094–7. doi: 10.2144/97236st07
46. Kozak M. An Analysis of 5’-Noncoding Sequences From 699 Vertebrate Messenger rNAS. Nucleic Acids Res (1987) 15:8125–48. doi: 10.1093/nar/15.20.8125
47. Martins M, Rodrigues FS, Joshi LR, Jos´ J, Jardim JC, Flores MM, et al. Orf Virus ORFV112, ORFV117 and ORFV127 Contribute to ORFV IA82 Virulence in Sheep. Vet Microbiol (2021) 257:1–8. doi: 10.1016/j.vetmic.2021.109066
48. Sauer B, Handerson N. Targeted Insertion of Exogenous DNA Into the Eukaryotic Genome by the Cre Recombinase. New Biol (1990) 2:441–9. PubMed.
49. Hierholzer JC, Killington RA. Virus Isolation and Quantitation. In: Virology Methods Manual. Academic Press Inc. San Diego, CA 92101: Elsevier (1996). p. 25–46.
50. Yassine HM, Khatri M, Zhang YJ, Lee CW, Byrum BA, O’Quin J, et al. Characterization of Triple Reassortant H1N1 Influenza A Viruses From Swine in Ohio. Vet Microbiol (2009) 139:132–9. doi: 10.1016/j.vetmic.2009.04.028
51. WHO. Manual for the Laboratory Diagnosis and Virological Surveillance of Influenza. World Health Organization Press. Geneva, Switzerland: WHO Global Influenza Surveillance Network (2011).
52. Dhakal S, Hiremath J, Bondra K, Lakshmanappa YS, Shyu DL, Ouyang K, et al. Biodegradable Nanoparticle Delivery of Inactivated Swine Influenza Virus Vaccine Provides Heterologous Cell-Mediated Immune Response in Pigs. J Control Release (2017) 247:194–205. doi: 10.1016/j.jconrel.2016.12.039
53. Kumar S, Stecher G, Li M, Knyaz C, Tamura K. MEGA X: Molecular Evolutionary Genetics Analysis Across Computing Platforms. Mol Biol Evol (2018) 35:1547–9. doi: 10.1093/molbev/msy096
54. Fleming SB, McCaughan CA, Andrews AE, Nash AD, Mercer AA. A Homolog of Interleukin-10 Is Encoded by the Poxvirus Orf Virus. J Virol (1997) 71:4857–61. doi: 10.1128/jvi.71.6.4857-4861.1997
55. Dumont FJ. Therapeutic Potential of IL-10 and its Viral Homologues: An Update. Expert Opin Ther Pat (2003) 13:1551–77. doi: 10.1517/13543776.13.10.1551
56. Moore KW, de Waal Malefyt R, Coffman RL, O’Garra A. Interleukin-10 and the Interleukin-10 Receptor. Annu Rev Immunol (2001) 19:683–765. doi: 10.1146/annurev.immunol.19.1.683
57. Topham DJ, Tripp RA, Doherty PC. CD8+ T Cells Clear Influenza Virus by Perforin or Fas-Dependent Processes. J Immunol (1997) 159:5197–200.
58. Soema PC, Van Riet E, Kersten G, Amorij JP. Development of Cross-Protective Influenza A Vaccines Based on Cellular Responses. Front Immunol (2015) 6:1–9. Frontiers Media S.A. doi: 10.3389/fimmu.2015.00237
59. Sun J, Braciale TJ. Role of T Cell Immunity in Recovery From Influenza Virus Infection. Curr Opin Virol (2013) 3:425–9. Elsevier B.V. doi: 10.1016/j.coviro.2013.05.001
60. Hamada H, Bassity E, Flies A, Strutt TM, Garcia-Hernandez M de L, McKinstry KK, et al. Multiple Redundant Effector Mechanisms of CD8 + T Cells Protect Against Influenza Infection. J Immunol (2013) 190:296–306. doi: 10.4049/jimmunol.1200571
61. Chen L, Zanker D, Xiao K, Wu C, Zou Q, Chen W. Immunodominant CD4+ T-Cell Responses to Influenza A Virus in Healthy Individuals Focus on Matrix 1 and Nucleoprotein. J Virol (2014) 88(20):11760–73. doi: 10.1128/JVI.01631-14
62. Gao XM, Liew FY, Tite JP. Identification and Characterization of T Helper Epitopes in the Nucleoprotein of Influenza A Virus. J Immunol (1989) 143:3007–14.
63. Wu C, Zanker D, Valkenburg S, Tan B, Kedzierska K, Ming Zou Q, et al. Systematic Identification of Immunodominant CD8 + T-Cell Responses to Influenza A Virus in HLA-A2 Individuals. PNAS (2011) 108:9178–83. doi: 10.1073/pnas.1105624108
64. Yewdell JW, Bennink JR, Smith GL, Moss B. Influenza A Virus Nucleoprotein Is a Major Target Antigen for Cross-Reactive Anti-Influenza A Virus Cytotoxic T Lymphocytes. Proc Natl Acad Sci USA (1985) 82:1785–9. doi: 10.1073/pnas.82.6.1785
65. Cassotta A, Paparoditis P, Geiger R, Mettu RR, Landry SJ, Donati A, et al. Deciphering and Predicting CD4+ T Cell Immunodominance of Influenza Virus Hemagglutinin. J Exp Med (2020) 217:1–15. doi: 10.1084/jem.20200206
66. Bui HH, Peters B, Assarsson E, Mbawuike I, Sette A. Ab and T Cell Epitopes of Influenza A Virus, Knowledge and Opportunities. Proc Natl Acad Sci USA (2007) 104:246–51. doi: 10.1073/pnas.0609330104
67. Stevens TL, Bossie A, Sanders VM, Fernandez-Botran R, Coffman RL, Mosmann TR, et al. Regulation of Antibody Isotype Secretion by Subsets of Antigen-Specific Helper T Cells. Nature (1988) 334:255–8. doi: 10.1038/334255a0
68. Crawley A, Wilkie BN. Porcine Ig Isotypes: Function and Molecular Characteristics. Vaccine (2003) 21:2911–22. doi: 10.1016/S0264-410X(03)00142-7
69. Bretscher PA. On the Mechanism Determining the Th1/Th2 Phenotype of an Immune Response, and its Pertinence to Strategies for the Prevention, and Treatment, of Certain Infectious Diseases. Scand J Immunol (2014) 79:361–76. doi: 10.1111/sji.12175
Keywords: orf virus, swine influenza virus, vectored-vaccine, neutralizing antibodies, cell-mediated immunity
Citation: Joshi LR, Knudsen D, Piñeyro P, Dhakal S, Renukaradhya GJ and Diel DG (2021) Protective Efficacy of an Orf Virus-Vector Encoding the Hemagglutinin and the Nucleoprotein of Influenza A Virus in Swine. Front. Immunol. 12:747574. doi: 10.3389/fimmu.2021.747574
Received: 26 July 2021; Accepted: 30 September 2021;
Published: 05 November 2021.
Edited by:
Rong Hai, University of California, Riverside, United StatesReviewed by:
Victor Leyva-Grado, AuroVaccines, United StatesWenjun Ma, University of Missouri, United States
Copyright © 2021 Joshi, Knudsen, Piñeyro, Dhakal, Renukaradhya and Diel. This is an open-access article distributed under the terms of the Creative Commons Attribution License (CC BY). The use, distribution or reproduction in other forums is permitted, provided the original author(s) and the copyright owner(s) are credited and that the original publication in this journal is cited, in accordance with accepted academic practice. No use, distribution or reproduction is permitted which does not comply with these terms.
*Correspondence: Diego G. Diel, ZGdkaWVsQGNvcm5lbGwuZWR1