- 1Key Laboratory of Cell Engineering of Guizhou Province, Affiliated Hospital of Zunyi Medical University, Zunyi, Guizhou, China
- 2Department of Orthopaedic Surgery, Affiliated Hospital of Zunyi Medical University, Zunyi, Guizhou, China
- 3Collaborative Innovation Center of Chinese Ministry of Education, Zunyi Medical University, Zunyi, Guizhou, China
- 4The Clinical Stem Cell Research Institute, Affiliated Hospital of Zunyi Medical University, Zunyi, Guizhou, China
It is often difficult to regain neurological function following spinal cord injury (SCI). Neuroinflammation is thought to be responsible for this failure. Regulating the inflammatory response post-SCI may contribute to the recovery of neurological function. Over the past few decades, studies have found that macrophages/microglia are one of the primary effector cells in the inflammatory response following SCI. Growing evidence has documented that macrophages/microglia are plastic cells that can polarize in response to microenvironmental signals into M1 and M2 macrophages/microglia. M1 produces pro-inflammatory cytokines to induce inflammation and worsen tissue damage, while M2 has anti-inflammatory activities in wound healing and tissue regeneration. Recent studies have indicated that the transition from the M1 to the M2 phenotype of macrophage/microglia supports the regression of inflammation and tissue repair. Here, we will review the role of the inflammatory response and macrophages/microglia in SCI and repair. In addition, we will discuss potential molecular mechanisms that induce macrophage/microglia polarization, with emphasis on neuroprotective therapies that modulate macrophage/microglia polarization, which will provide new insights into therapeutic strategies for SCI.
Introduction.
Spinal cord injury (SCI) usually leads to permanent loss of motor, sensory and autonomic function below the site of injury (1). Unfortunately, about 1.3 million people worldwide suffer from SCI, with almost 180,000 new cases occurring yearly (2). Traumatic spinal cord injuries devastate the physical, financial, and psychological well-being of the injured person and their caregivers (3). The pathophysiological process of SCI can divide into primary and secondary injuries (4). Primary injury is irreversible, and the treatment of SCI is focused on minimizing the inflammatory response to secondary injuries (5, 6). To date, there is still a lack of effective treatment for neuroinflammatory conditions. Important neuroprotective measures presently applied in clinical practice include early surgical decompression, blood pressure augmentation, and intravenous glucocorticoids (GCs) (7, 8). However, these methods do not fundamentally improve the function of the injured spinal cord, and there is an urgent need to develop new therapeutic approaches to treat SCI.
As an important player in the immune response process, macrophages/microglia dominate the inflammatory process following SCI (9). After the injury, macrophages initially originated from resident activated-microglia and later primarily from circulating monocytes (10). As both infiltrating macrophages and resident microglia are derived from bone marrow mononuclear cells, they share similar molecular markers and functional characteristics, making it difficult to distinguish them at present (11). As a consequence, we use the term “macrophage/microglia” to describe these cells collectively. Studies have found that macrophage/microglia may have detrimental and beneficial roles in the injured spinal cord (12, 13). These seemingly contradictory functions of macrophages/microglia reflect the different phenotypes they acquire in response to different microenvironmental cues (14). Conventionally, macrophages/microglia are divided into two significant phenotypes: M1 and M2 (15). Classically activated macrophages/microglia (M1) is primed by Th1 cytokines, such as tumor necrosis factor-alpha (TNF-α) and interferon (IFN)-γ, and produce high levels of pro-inflammatory cytokines interleukin -6(IL-6), IL-23, IL-1β, and TNF-α (16, 17), which are pro-inflammation, induce axonal degeneration and cause neurotoxicity (18, 19). In contrast, alternatively activated macrophages/microglia (M2) are induced by Th2 cytokines such as IL-4 and IL-13 (17, 20). These cells secrete transforming growth factor-beta (TGF-β), IL-4, and IL-10 to suppress inflammation, induce angiogenesis, and promote tissue repair (20, 21). Growing evidence suggests that the transition of inflammatory macrophages/microglia to the M2 phenotype leads to reduced secondary damage and improved locomotor recovery after SCI (22, 23).
The current review will present and discuss the role of the inflammatory response and macrophages/microglia in SCI and repair. In addition, we will discuss potential molecular mechanisms that induce macrophage/microglia polarization, with emphasis on neuroprotective therapies that modulate macrophage/microglia polarization. It is hoped to provide direction and basis for future research on the mechanism of macrophage/microglia polarization and the treatment of SCI via regulating macrophages/microglia polarization.
The inflammatory response after SCI.
Primary injury results from initial trauma to the spinal cord, which can cause rupture of the blood-spinal cord barrier (BSCB), bleeding, edema and oxidative damage (24, 25). This process initiates a secondary injury cascade that begins only a few hours after injury, leading to an inflammatory response, ischemia and progressive neuronal death (Figure 1) (25, 26). The BSCB consists of continuous endothelial cells, pericytes and glial cells with molecular junctions (27). Similar to the blood-brain barrier, the BSCB is critical for the exclusion of peripheral immune cells, various inflammatory and toxic metabolites from the CNS, which maintains microenvironmental stability (28, 29). Barrier integrity is compromised following SCI by disruption of interendothelial tight junctions (TJs) and adherent junctions (AJs) and by overall mechanical damage to the vasculature (30). The sustained permeability of the BSCB allows infiltration of peripheral inflammatory cells and factors into the spinal cord and leads to secondary injury (24). During the recovery stage, BSCB repair and glial scar formation limit the recruitment of “fresh” M2 macrophages/microglia to the injury site (14). This could also contribute to the persistence of inflammation after SCI.
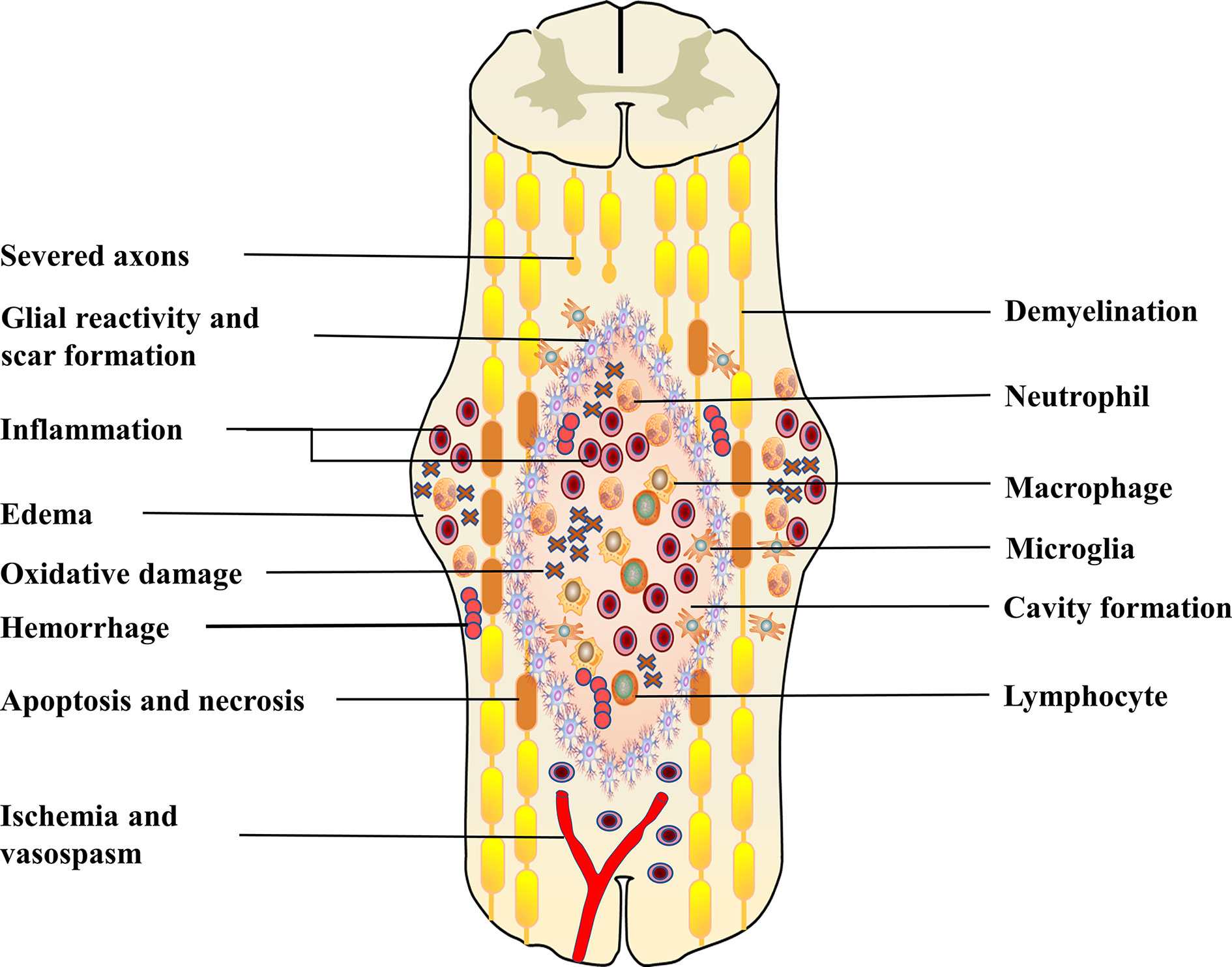
Figure 1 This figure shows the combination of pathophysiological events that occur post-SCI. This includes hemorrhage, oedema, inflammation, apoptosis, necrosis, oxidative damage, ischemia and vasospasm. Following primary injury, resident cells (astrocytes, microglia) are immediately activated and migrate to the site of injury. Subsequently, peripheral inflammatory cells, including neutrophils, macrophages and lymphocytes, infiltrate into the center of the damaged spinal cord. These activated immune cells can exacerbate the injury, causing neuronal death, which leads to axonal demyelination and disruption of synaptic transmission. In the subacute phase, fluid-filled cavities form in the center of the spinal cord. Astrocytes form a glial scar to isolate the damaged area. These sustained pathophysiological changes eventually lead to severe dysfunction below the damaged segment.
An inflammatory response is critical in the secondary injury cascade after SCI. It involves activated resident cells (microglia, astrocytes) and macrophages, neutrophils, and lymphocytes recruited from the peripheral blood to the site of injury (31, 32). These cells secrete pro-inflammatory cytokines, such as IL-1, IL-6, and TNF-α, all of which enhance the magnitude of the inflammatory response (4, 33). Damaged cells release damage-associated molecular patterns (DAMPs), such as small molecules and proteins, that induce sterile neuroinflammation after SCI (34, 35). DAMPs are perceived by pattern recognition receptors (PRRs), leading to the rapid activation of resident cells, such as microglia and astrocytes (36, 37). Activated microglia secrete various pro-inflammatory cytokines, including INF-γ, IL-6, TNF-α, and other cytotoxic factors (38, 39). At the same time, they were inducing vascular endothelial cells to express a variety of chemokines and cell adhesion molecules that contribute to the recruitment of peripheral immune cells to the site of injury (40, 41). As resident immune cells in the CNS, microglia also possess positive qualities. Fu et al. found that microglia may play a protective role after SCI by regulating astroglia scar formation, which insulates peripheral inflammatory cells in the core of the lesion, thus avoiding inflammation-mediated tissue damage (42). Similar to microglia, astrocyte membranes express a variety of PRRs, which can interact with DAMPs to initiate inflammatory responses (37, 43). Reactive astrocyte-derived permeability factor thymidine phosphorylase, which interacts with vascular endothelial growth factor A to induce blood-brain barrier disruption (44), alters the local microenvironment and facilitates the entry of peripheral immune cells into the CNS parenchyma. However, astrocytes are essential for regulating and alleviating inflammation after SCI and restoring microenvironmental homeostasis (45). First, the reactive astrocytes of the formed glial scar can constrain the harmful microenvironment (46). Second, astrocytes specifically eliminate glutamate, thereby reducing excitotoxic damage (46, 47). Neutrophils are the first peripheral immune cells to be recruited to a site of SCI (24, 48). These cells can promote phagocytosis and clearance of cellular debris and facilitate repair by secreting protease inhibitory factors (49, 50). However, infiltrating neutrophils release neutrophil extracellular traps (NETs), which subsequently promote BSCB disruption and neuroinflammation to exacerbate neuronal apoptosis and spinal cord edema following SCI (51). The endocytosis effect of macrophages on apoptotic neutrophils after tissue injury is the basis for eliminating inflammation and initiating tissue remodeling, which also plays an essential role in modulating neutrophil production (52, 53). Several studies have shown that macrophages are neurotoxic. For example, in the SCI model, macrophage depletion and lack of CX3CR1 signaling contributed to improved neurological function and tissue repair (54–56). In particular, macrophage depletion promoted recovery of TJ between vascular endothelial cells and reduced leakage of BSCB from the injured core post-SCI (57). These harmful or protective effects of macrophages on tissue regeneration are primarily owed to their different cellular phenotypes and the activation of specific intracellular signaling pathways. Like macrophages, activated lymphocytes have conflicting effects on SCI. On the one hand, T lymphocytes secrete various pro-inflammatory cytokines, such as IL-1β and INF-γ, resulting in neurological tissue damage (58). On the other hand, they could play an essential role in SCI and repair by regulating the function and recruitment of both innate and acquired immune cells following SCI (59). The conflicting results concerning the effects of T lymphocytes in restoration from SCI may be attributed to differences in the role of helper T-cell subsets (60).
Inflammation has both damaging and repairing effects. While the inflammatory response to SCI is beneficial and necessary for tissue repair following damage, sustained inflammation can be detrimental to tissue repair. As a result, effective suppression of excessive inflammation in SCI can improve the prognosis of SCI and promote nerve function recovery and tissue regeneration.
Role of macrophages/microglia in SCI.
The inflammatory response following SCI leads to the infiltration of many inflammatory cells, and macrophages/microglia play a critical role in the evolution and development of inflammation.
Role of M1 macrophages/microglia in SCI.
M1 macrophages/microglia can be induced by the Th1 cytokines such as TNF-α, IFN-γ, and lipopolysaccharide (LPS), which secrete destructive factors such as TNF-α, IL-1β, IL-6, and ROS (17, 61, 62), and can initiate the development of inflammation. Several studies have shown that the disruptive effects of activated macrophages/microglia are mainly ascribed to their M1 phenotype. For example, an increase in M1 polarized macrophages/microglia was observed in the context of SCI, which may inhibit M2 cells from repairing damaged tissue (63). Similarly, another study reported that Quercetin inhibits macrophages/microglia polarization towards the M1 phenotype via inhibition of the STAT1/NF-κB pathway, reduces neural tissue damage, and suppresses inflammatory response with a neuroprotective effect on SCI (64). macrophage/microglia have been demonstrated to contribute to spinal cord cavity formation and expansion after SCI. Fan et al. found that M1 macrophages/microglia may induce necroptosis in astrocytes following SCI through activation of TLR4/MyD88 signaling, which leads to enlargement of the spinal cord cavity (Figure 2) (65). These investigations suggest that M1 hinders neurogenesis and exacerbates secondary injury. However, M1 macrophages/microglia also possess positive qualities. These cells secrete soluble protein mediators such as vascular endothelial growth factor and enzymes that modify the extracellular matrix, which promote the proliferation of vascular endothelial cells (34). These seemingly conflicting results suggest that the M1 phenotype exerts some pro-tissue repair functions after all, such as clearance of cell debris soon after SCI. These findings suggest that future therapeutic strategies will need to inhibit M1 polarization at the correct time to improve the results of SCI.
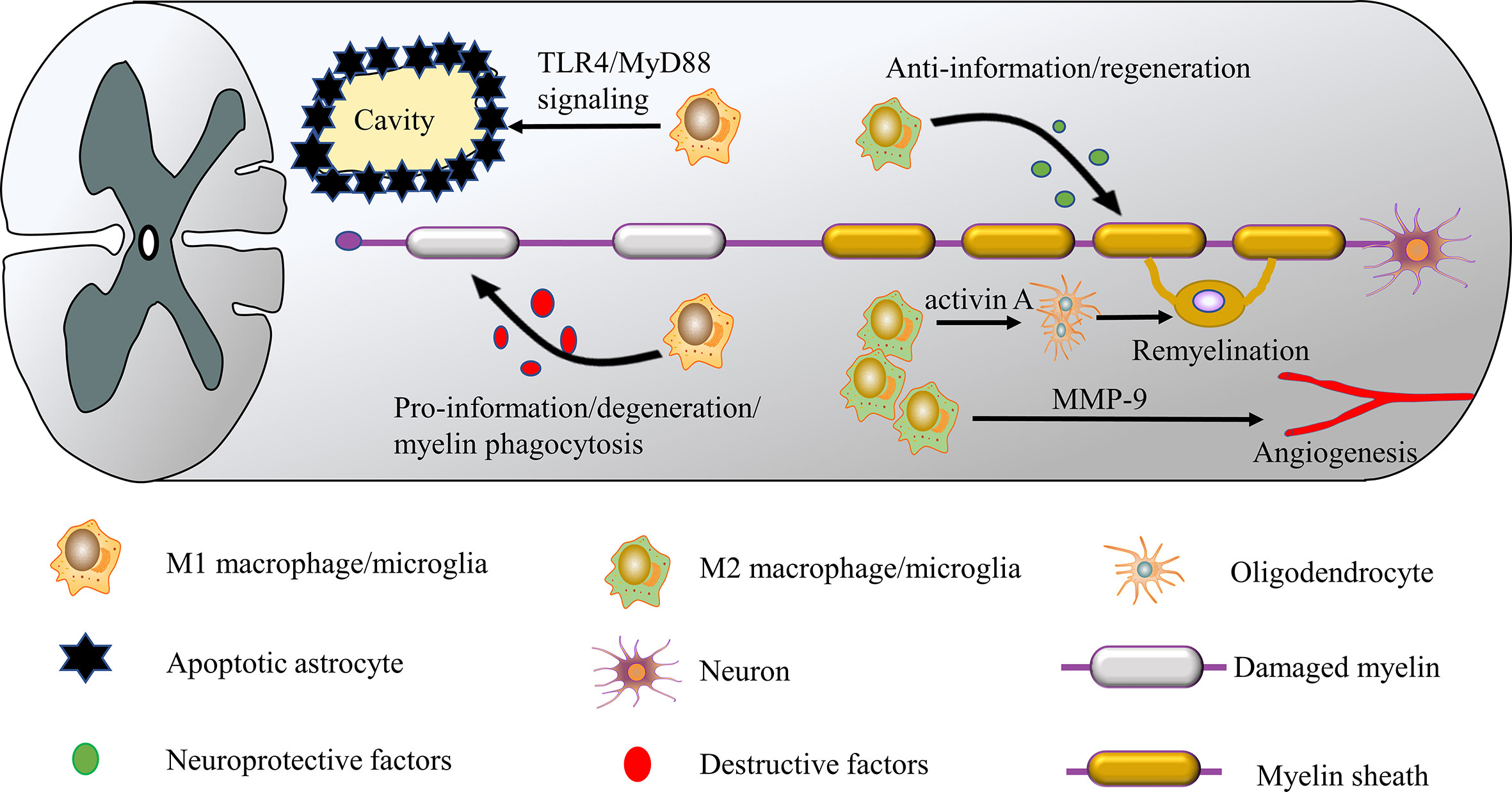
Figure 2 M1 and M2 macrophages/microglia have different roles in SCI. M1 macrophages/microglia are neurotoxic and release destructive factors that impair axon repair/regeneration. These cells can induce the necroptosis of astrocytes and promote the formation of the spinal cavity. In contrast, M2 macrophages/microglia have anti-inflammatory and neuroprotective effects, releasing neuroprotective factors such as IL-10 and TGF-β to improve SCI repair/regeneration. In addition, the M2 phenotype facilitates axon regeneration and angiogenesis by secreting activin A and matrix metalloproteinase 9 (MMP-9).
Role of M2 macrophages/microglia in SCI.
M2 macrophages/microglia have anti-inflammatory functions and regulate tissue repair and remodeling, express CD206 (mannose receptor) and arginase (Arg)-1, and secrete anti-inflammatory cytokines such as IL-4 and TGF-β (62, 66). M2 macrophages/microglia remove cellular debris through phagocytosis, release many neuroprotective and trophic factors (14, 67), and produce anti-inflammatory cytokine IL-10, inducing the conversion of M1 macrophages/microglia to the M2 phenotype (68, 69), which plays a role in wound healing. In addition, M2 macrophages/microglia secret matrix metalloproteinase 9 (MMP-9) as a single tissue metalloproteinase 1 inhibitor, increasing their angiogenic potential (14, 70). Several studies have confirmed the neuroprotective role of the M2 phenotype in SCI. For example, in an SCI model, transplantation of M2 macrophages was effective in suppressing neuroinflammation and promoting functional recovery (71). Wang et al (22). Found that Butylphthalide plays an essential role in the anti-inflammatory response to SCI by promoting macrophages/microglia M2 polarization and inhibiting macrophages/microglia M1 polarization by activating the p38 pathway. In another study, azithromycin (AZM) was found to alter SCI macrophages/microglia polarization towards a beneficial M2 phenotype, thereby inhibiting the secondary injury process (23). Furthermore, M2 macrophages/microglia are an essential component of the CNS regenerative response, facilitating myelin regeneration by generating activin A to drive oligodendrocyte differentiation (Figure 2) (72). These studies suggest that M2 is crucial in suppressing inflammation and tissue repair. But it should be noted that excessive or prolonged M2 polarization may contribute to scar formation and fibrotic reactions that will hinder axonal regeneration and recovery of neurological function (73). Because M2 macrophages/microglia involved in wound healing also secrete large amounts of TGF-β, which subsequently activates fibroblasts, initiates and promotes the process of fibrosis (74, 75). Another potential concern is that long-term maintenance of the M2 phenotype may harm immune defenses and cause serious health consequences, such as tumor development (17). However, the dominance of M1 macrophages/microglia and the reduction in the number of M2 macrophages/microglia following SCI can expand tissue damage (76). Given the evidence in favor of a role for M2 macrophages/microglia in SCI anti-inflammatory and regeneration, M1-to-M2 phenotypic switches may create a favorable microenvironment for SCI repair.
Macrophages/microglia polarization.
Macrophages/microglia polarization involves the interaction of various cytokines, chemokines, and transcription factors (Figure 3). Signaling molecules from the microenvironment bind specifically to receptors on the surface of the macrophages/microglia membrane and activate the corresponding signaling pathways and transcription factors, which initiate the macrophages/microglia polarization pathway (77, 78).
Macrophages/microglia polarization to the M1 phenotype.
Classically activated macrophages/microglia are activated by Th1 cytokines (IFN-γ, GM-CSF, and TNF-α) and lipopolysaccharide (LPS) (79, 80). Studies have shown that the TLR4/NF-κB pathway plays an essential role in M1 macrophages/microglia polarization (78, 81). For example, The LPS/TLR4 pathway activates NF-κB and interferon regulatory factor 3 (IRF3) to induce M1 polarization and facilitate the production of inflammatory factors (82). Members of the STAT family, including STAT1 and STAT3, are involved in regulating the M1 macrophages/microglia phenotype. For instance, IFN-γ binds to its receptor and activates Janus kinase, which phosphorylates STAT1, thereby inducing macrophages/microglia polarization to M1 (83). The roles of STAT3 are various as they are not only related to IL-6 induced M1 polarization but are also involved in IL-10 stimulated M2 polarization (84, 85). Notably, IL-17 enhances IFN-γM1-induced polarization by enhancing STAT1 phosphorylation while inhibiting IL-4-mediated M2 transformation by inhibiting STAT6 activation (86). To date, transcription factors, including NF-κB, STAT1, STAT5, IRF3, IRF5, and IRF8, have been shown to regulate transcription programs that control M1 macrophages/microglia polarizations (Figure 3) (85, 87–89).
Macrophages/microglia polarization to the M2 phenotype.
The M2 phenotype can be primed by Th 2 cytokines, IL-13 and IL-4 (77, 90), as well as other factors, including IL-10, IL-33, M-CSF and TGF-β (77, 87, 91). Interestingly, IL-13 and IL-4 directly induce M2 macrophages/microglia activation, while other cytokines, such as IL-25 and IL-33, indirectly induce the M2 phenotype through the production of Th2 cytokines (92). STAT6 was the main transcription factor that induced M2 polarization (93, 94). IL-4 and IL-13 regulate the balance between M1/M2 macrophages/microglia in favor of the M2 phenotype via the IL-4Rα-STAT6 pathway (82, 95). We also found that IL-10 can polarize macrophages/microglia towards the anti-inflammatory M2 phenotype by activating the IL-10/STAT3 pathway (96). The importance of other transcription factors in phenotypic regulation has also been highlighted in several recent discoveries. For instance, IRF4 is an important transcription factor that controls M2 polarization (97, 98), and PPAR-γ and Kruppel-like factor 4(KLF4) control M2 polarization (99, 100).
M2 produce complex cytokines and have multiple functions, so they can be further subdivided into M2a, M2b, M2c, and M2d subtypes (Figure 3) (101). M2a, also known as wound healing macrophages/microglia, is induced by IL-4 and IL-13, express CD206 and C-C motif chemokine ligand 17 (CCL17), and secrete IL-10, TGF-β, and insulin-like growth factor (IGF) to promote tissue repair (102, 103). In addition, M2a macrophages/microglia are involved in tissue fibrosis, Th2 immune responses, and allergic reactions (78). Polarization of M2a macrophages/microglia promotes arginase 1 expression, restores axonal regeneration, promotes axonal regeneration and improves functional recovery post-SCI (104). M2b and M2c have similar immunomodulatory functions but different inducers. M2b macrophages/microglia are induced by immune complexes, Toll-like receptor (TLR) agonists or IL-1 receptor ligands, which produce both pro-inflammatory factors (IL-1β, IL-6 and TNF-α) and anti-inflammatory factor IL-10 (103), and play a role in Th2 activation and immune regulation (105, 106). In addition, M2b releases anti-inflammatory cytokines without causing neurotoxicity, facilitating recovery from SCI (107). M2c shows a regulatory phenotype induced by glucocorticoids, IL-10, and TGF-β exerts anti-inflammatory effects by secreting IL-10 and TGF-β (106, 108) and is associated with immunosuppressive behavior, matrix remodeling, and tissue repair (109, 110). Enhances M2b and M2c phenotypes. May coordinate the proliferative phase of wound healing and promote SCI repair (111). M2d macrophages/microglia, also called tumor-associated macrophages/microglia (TAM), are triggered by co-stimulation with TLR ligands and A2 adenosine receptor (A2R) agonists or IL-6 (103); these cells secrete IL-10, vascular endothelial growth factor (VEGF) and TGF-β (109), and contribute to angiogenesis and tumor metastasis (106).
Much knowledge of the phenotypic and functional characteristics of macrophages/microglia mentioned above is obtained from rodents. Notably, there are apparent differences between rodent macrophages/microglia and their human counterparts (112). For example, iNOS and Arg1 are mouse M1 and M2 markers, but are not expressed at significant levels in human macrophages/microglia at all (113, 114). Furthermore, macrophages/microglia responses to stimuli are variable between species, with GM-CSF and M-CSF being inducers of M1 and M2 polarization in mice but not in human macrophages/microglia (115). In several mouse tumor models, circulating monocytes are the major precursors of TAM (116, 117); in humans, in the context of bone marrow transplantation, lymphoma-associated macrophages/microglia were found to originate from bone marrow precursors (116), which suggests that human and mouse macrophages/microglia may differ in origin. More importantly, the M1/M2 dichotomy following macrophage/microglia polarization in animal models is not as evident in humans, and there is a continuum of activation states between M1 macrophages/microglia and M2 macrophages/microglia, the boundaries of which remain unclear (118). Some studies have found that human monocytes polarize to an M1 phenotype and M2 repair macrophages/microglia when microenvironmental conditions change, and vice versa (119, 120). This indicates that the phenotype and polarization mechanisms of human macrophages/microglia are much more complex than those of rodents. Consequently, a better understanding of the phenotypic and functional characteristics of human macrophages/microglia will provide a theoretical and research basis for the therapy of SCI.
Advances in neuroprotective therapies that regulate macrophages/microglia polarization in SCI.
In healing wounds, the M1 to M2 macrophage/microglia phenotype transition supports the regression of inflammation and tissue repair (121). However, a similar M1 to M2 transition was not observed in spinal cord tissue repair process. Therefore, regulating macrophages/microglia to an anti-inflammatory phenotype is potentially a prospective therapeutic strategy for treating SCI.
Delivery of molecules to alter the macrophages/microglia phenotype.
Several studies have demonstrated that inhibition of the M1 phenotype can be applied to the treatment of SCI, including blocking the activity of inflammatory cytokines and transcription factors involved in the induction of the phenotype. For example, in a mouse model of SCI, the blockade of IL-7 receptors further inhibits M1 polarization and promotes M2 polarization via down-regulating the expression of Th1 cytokines (IFN-γ, TNF-α) and up-regulating Th2 cytokines (IL-4, IL-13), which improved function following SCI (122). Similarly, blockade of IL-6 signaling promotes functional regeneration by inhibiting M1 and stimulating M2 macrophages/microglia activation post-SCI (123). In addition, Infliximab antagonism of TNF-α inhibited NF-κB activity, which is essential for M1 polarization (124), resulted in suppression of M1 polarization and improved motor function after acute SCI in rats (125). It was found that TNF-α levels increased shortly after SCI and that TNF-α activity had to be blocked immediately after injury to reduce the deleterious effects induced by TNF-α (126). Because delayed blockade of TNF-α activity do not effectively suppress M1 activation, which has no effect on repair following SCI (127). Finally, Nanoparticle delivery of siRNA silences the transcription factor IRF5, which downregulates M1 macrophage/microglia-related gene expression, resulting in a dramatic reduction in M1 macrophages/microglia numbers and a significant increase in the number of M2 macrophages/microglia in the wound, reducing neuroinflammation, inhibiting demyelination and promoting wound healing (128).
Numerous studies have shown that in addition to supplying M1-inhibiting molecules, releasing M2-promoting molecules can also promote tissue repair. For instance, systemic or intraspinal administration of IL-4 after SCI increased levels of the anti-inflammatory cytokine IL-10 and promoted M2 replacement activation of macrophages/microglia (129, 130). Regardless of the route of administration, IL-4 treatment significantly reduced the expression of the inflammatory marker nitric oxide synthase (iNOS) (129, 130). Similarly, sustained delivery of IL-10 improved neurological function post-SCI, which induces conversion of macrophages/microglia to an anti-inflammatory M2 phenotype and reduces the inflammatory response following SCI with depression of TNF-α and IL-1β production (68). In addition, IL-10 upregulates anti-apoptotic factors such as B-cell lymphoma 2 (Bcl-2), which exhibits direct trophic effects on neurons and improves the neurotoxic microenvironment (131).
Peroxisome proliferator-activated receptor gamma (PPAR-γ) is a ligand-dependent nuclear receptor that regulates the immune-inflammatory response (132). As we mentioned earlier, PPAR-γ plays an important role in macrophages/microglia polarization. Several studies have revealed that PPAR-γ activation-induced anti-inflammatory effects are associated with macrophages/microglia polarization (133, 134). activation of PPAR-γ induces macrophages/microglia polarization towards the M2 subtype, thus exerting its beneficial anti-inflammatory effects (135). Importantly, PPAR-γ is a broadly distributed nuclear receptor whose activation has caused to a reduction in the pro-inflammatory cascade in various CNS diseases (136). Because activation of PPAR-γ in CNS diseases suppresses the expression of proinflammatory cytokines TNF-α, IL-1β and iNOS, increases neuronal survival (137, 138). For example, the PPAR-γ agonist thiazolidinediones (TZDs) inhibited the inflammatory cascade and increased tissue retention, thereby improving motility in a rodent model of SCI (139, 140), and TZDs effectively reduced the release of pro-inflammatory factors such as IL-1β, IL-6 and TNF-α from macrophages/microglia (136). It is clear that PPAR-γ activation plays an important anti-inflammatory role in SCI. However, to date, there is very little direct in vivo evidence demonstrate that PPAR-γ activation improves anatomical and motor recovery after SCI through promoting the polarization of M2 macrophages/microglia.
Mesenchymal stem cell and exosome therapy.
The therapeutic effect of MSCs on SCI may be partly attributed to the transplantation-induced polarization of macrophages/microglia towards an anti-inflammatory phenotype (141). Because MSCs have immunomodulatory properties and secrete immunomodulatory factors such as IL-10, TGF-β, IDO, TNF-inducible gene-6 (TSG-6) and prostaglandin-E2 (PGE2), these cytokines induce polarization of macrophages/microglia towards the M2 phenotype (96, 142–145), thereby inhibiting inflammation leading to remodeling and disease healing effects. Our previous study found that PBMSCs effectively induced macrophages/microglia polarization towards the M2 phenotype, upregulated the expression of M2 surface markers (Arg-1, CD206) and cytokines (IL-10, CCL22, TGF-β1), while significantly inhibiting the production of IL-1β, IL-6 and TNF-α, and promoted functional recovery after spinal cord injury (146, 147). In the acute phase of SCI, MSC transplantation regulated macrophages/microglia polarization from M1 to M2 cells, promoted the expression of anti-inflammatory mediators IL-4 and IL-13, inhibited the production of pro-inflammatory mediators TNF-α and IL-6, and suppressed scar formation and neuronal demyelination, providing a permissive environment for axonal extension and functional recovery (148, 149).
Although MSCs have great potential to treat SCI, the poor microenvironment after injury is not conducive to MSCs survival, which hinders their further clinical application. Therefore, any approach to improve the biological activity of transplanted MSCs in vitro and in vivo is of great value. Notably, pretreated MSCs show better immunomodulatory capacity, enhanced cell survival and homing ability, resulting in more effective repair of damaged spinal cord (150, 151). A number of pretreatment methods have been explored to enhance MSC therapeutic capacity, including hypoxia, inflammatory cytokines, genetic modifications and 3D culture (150, 152–154). For example, MSCs cultured under hypoxic conditions secrete more cytokines and growth factors, resulting in enhanced therapeutic function (155, 156). In vivo, hypoxic preconditioning (HP) improves the survival and migration of MSCs and enhances their therapeutic potential against SCI (157). It was found that stimulation of MSCs with IL-1β and IFN-γ enhanced their ability to induce macrophages/microglia polarization to the M2 phenotype and immunomodulatory functions (158, 159). TNF-α was also used as a pretreatment for MSCs, and TNF-α pretreatment exhibited anti-inflammatory effects by promoting the secretion of immunomodulatory factors (such as IDO, PGE2 and HGF) by MSCs (160). Genetic modifications have been reported to improve the efficacy of MSCs. Basic fibroblast growth factor overexpressing MSCs significantly improved treatment outcomes, for example, reducing glial scar formation, improving nerve regeneration and endogenous neural stem cell (NSC) proliferation, and increasing recovery of motor function in the hind limb (161). Similarly, MSCs transplanted with IL-10 overexpressing cells were more capable of inducing M2 type macrophages/microglia, significantly improving functional recovery and reducing lesion size and demyelination area after SCI (162). 3D culture could be another unique method to further improve the immunomodulatory capacity of MSCs. Compared to 2D culture, MSCs in 3D culture were able to secrete high yields of highly productive and active exosomes (163). Han et al (164). found that bone marrow-derived mesenchymal stem cells cultured in 3D collagen scaffolds exhibited unique characteristics, including significantly reduced in vitro LPS-induced macrophage/microglia activation and enhanced neurotrophic factor secretagogues. These results suggest that pretreatment enhances the biological function of MSCs in vivo and in vitro and could help them adapt to the new transplant microenvironment.
Recent studies have found that the therapeutic effects of MSCs are largely attributable to exosomes (165). Exosomes are important paracrine factors that can be used as direct therapeutic agents (166). They not only modulate the immune response and suppress inflammation, promote axonal regeneration and angiogenesis, but also play an important role in the repair of SCI by inhibiting apoptosis as well as maintaining the integrity of the blood-spinal cord barrier (166–169). Several studies have confirmed the therapeutic effect of MSC-exosomes in relation to the induction of macrophages/microglia polarization (76). For example, BMSCs-derived exosome miR-124-3p attenuated spinal cord ischemia-reperfusion injury (SCIRI)-induced tissue damage and nerve injury by silencing endoplasmic reticulum-to-nucleus signaling 1 leading to polarization of macrophages/microglia towards M2 and inhibiting apoptosis (170). Sun et al (165). found that human umbilical cord mesenchymal stem cells exocytosis triggered the polarization of macrophages/microglia from M1 to M2 phenotype and improved functional recovery after SCI by downregulating inflammatory cytokines such as IL-6, TNF-α, and IFN-γ. Subsequently, MSC-derived exosomes exhibited neuroprotective effects by altering the M1/M2 macrophages/microglia ratio and shifting the balance towards the M2 phenotype (171, 172). Compared to MSCs, MSC-derived exosome therapy avoids the problems of low MSC survival and the potential differentiation of MSCs into other cell types (172). In addition, exosomes are more stable, safer and less immunogenic (168). Therefore, MSC-derived exosomes may be a promising therapeutic strategy for the treatment of SCI.
MicroRNAs regulate macrophages/microglia polarization.
A large number of microRNAs (miRNAs) have been identified in the mammalian central nervous system and play an important role in neural regeneration, genesis and development (3, 173). miRNAs are a class of small non-coding RNAs that act as transcriptional regulators, which are involved in the pathophysiological processes of SCI and are considered to be effective therapeutics for SCI (174, 175). Some investigators have found that miR-155 deletion not only reduces macrophages/microglia-mediated neurotoxicity and increases neuroprotection, but also improves functional recovery and reduces neuropathic pain (176, 177). miR-182 has inhibitory effects on the inflammatory response to various types of injury, and in a mouse model of SCI, miR-182 ameliorates the secondary injury after SCI by blocking the IKKβ/NF-κB pathway to inhibit apoptosis and inflammatory responses (178). Similarly, miR-494 is important in regulating the onset, progression and repair of SCI; Huang et al. Showed that miR-494-modified exosomes delivered to the diseased spinal cord improved the local immune environment, inhibited neuronal apoptosis and the release of pro-inflammatory factors, thereby promoting neurofilament regeneration and recovery of behavioral function (3). This evidence from the literature suggests that miRNAs are modulators of secondary injury and repair after SCI and a novel intervention pathway to improve recovery after SCI.
Recent studies have shown that miRNAs control macrophage/microglia differentiation and activity by regulating the signaling of key transcription factors (179, 180), which subsequently influence the progression of inflammation. The main transcription factors involved in regulating macrophages/microglia polarization include signal transducers and activators of transcription (STATs), KLF, interferon regulatory factors (IRFs), PPAR, C-MYC, and C/EBPs (Figure 4) (179, 181). miR-146b, for example, targets the transcription factor IRF5 to significantly inhibit M1 polarization and improve the development of colitis (182). miR-155 reduced myocardial inflammation and improved cardiac function by increasing STAT6 phosphorylation that promoted M2 but prevented M1 polarization (183). Subsequently, miR-125a negatively regulated IRF5, promoting M2 polarization and improving inflammation after SCI (184). Another study revealed that miR-22-3p reduced ischemia/reperfusion (I/R) injury in the spinal cord by inhibiting IRF5 to promote macrophages/microglia shift to the M2 phenotype and suppress tissue inflammation (185). Interestingly, multiple miRNAs can target the same transcription factor, each with a different target. For example, miR-27a, miR-130a and miR-130b promote M1 polarization by inhibiting PPAR-γ expression (186–188). In contrast, miR-124 can target different transcription factors, such as STAT3 and C/EBP-α, respectively, to promote M2 polarization (189, 190). To date, accumulating evidence confirms that miR-27a (186), miR-27b (191), miR-130a (187), miR-155 (183, 192), miR-21 (193), miRNA-125b (194) and miRNA-26a (195, 196) have been shown to promote M1 polarization, whereas miR-146b (197), miR-223 (198), miR-93 (199), miR-124 (189), and let-7c (200) induce M2 macrophages/microglia polarization via targeting various transcription factors and adapter proteins (Figure 4). These miRNAs regulate the transcriptional expression of target genes by targeting transcription factors, which determine the functional polarization of macrophages/microglia.
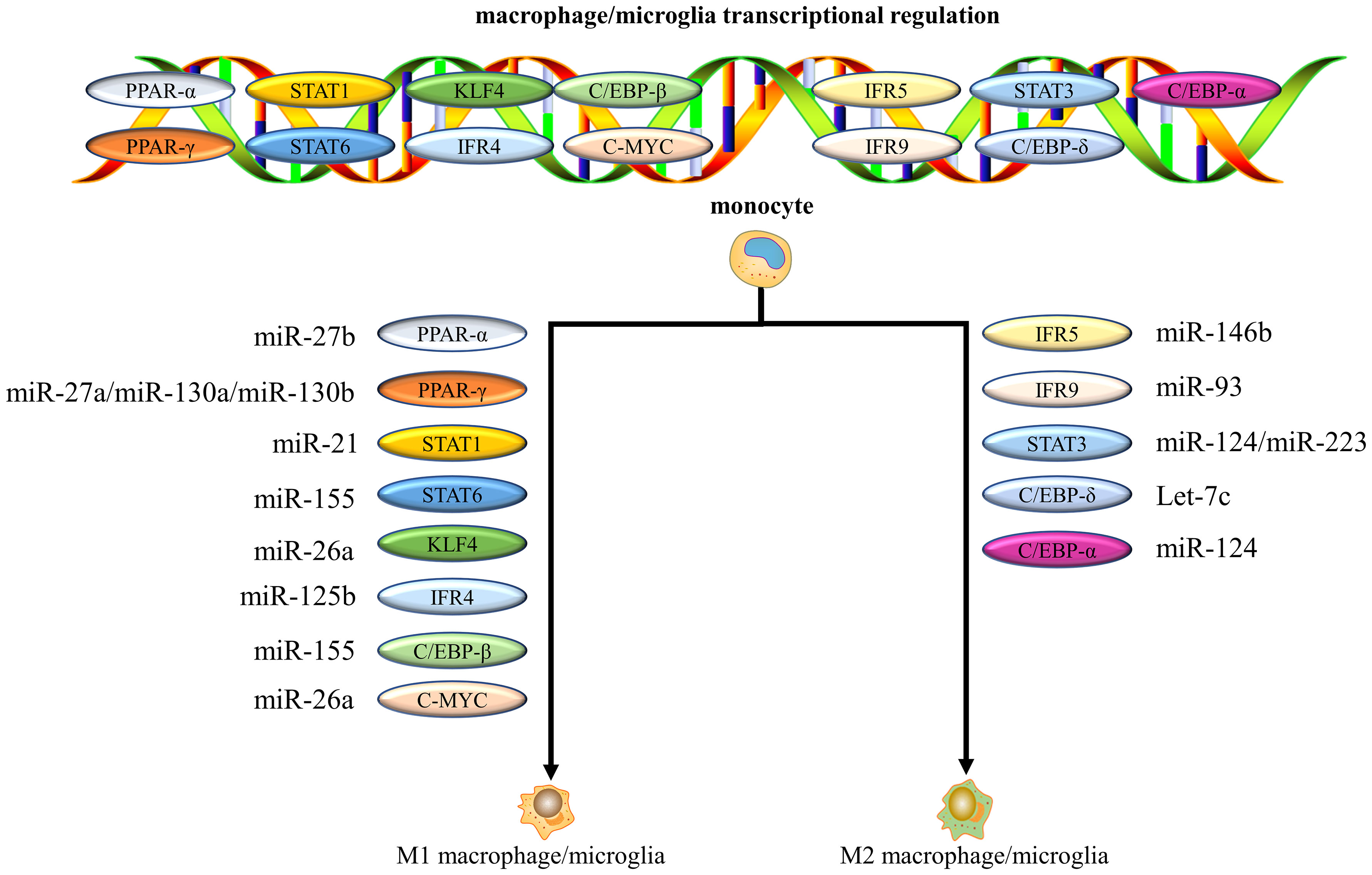
Figure 4 miRNAs control macrophages/microglia polarization by regulating the signaling of key transcription factors. Multiple miRNAs can target the same transcription factor, and each miRNA can have a different target.
The mechanisms by which miRNAs regulate macrophage/microglia polarization via transcription factors appear complex. For example, it has been demonstrated that in adipocytes miR-34a promotes M1 and inhibits M2 polarization by directly targeting KLF4 (100, 201). However, it has also been shown that miR-34a can inhibit pro-inflammatory macrophages/microglia polarization and enhance anti-inflammatory macrophages/microglia phenotypes (181, 202). The reasons behind the conflicting effects of miR-34a on macrophage/microglia polarization are unclear. These may be attributed to the specificity of miRNAs for different tissues and cell types. And each miRNA can have different targets and multiple miRNAs can have the same target gene (203, 204). This may result in miR-34a having different effects on macrophage/microglia polarization. Understanding the molecular mechanisms by which miRNAs regulate macrophages/microglia can help provide the basis for macrophages/microglia-centric therapeutic strategies.
M2 macrophages/microglia adoptive immunotherapy.
M1 macrophages/microglia and anti-inflammatory M2 macrophages/microglia were detected early in the local microenvironment after SCI injury, but 1 week after injury, M1 was predominant and M2 was minimal (205). It has been found that using bone marrow-derived M2 adoptive transfer 7 days after SCI increases the proportion of M2 macrophages/microglia in the injured spinal cord and has a neuroprotective effect (206, 207). The increase in the number of M2, through the production of anti-inflammatory cytokines such as IL-10 and TGF-β, shifts the immune response from a Th1-dominant to a Th2-dominant one, induces more local macrophages/microglia polarization to the M2 phenotype, and creates a local microenvironment conducive to the protection of neuronal function and inhibition of demyelination (206). Chen et al. found that M2 macrophages/microglia adoptive immunity can reduce the expression of genes associated with inflammatory signaling pathways such as antigen processing and presentation, phagosomes, cell adhesion molecules, cell-mediated cytotoxic natural killing, endocytosis, and proteasomal and Toll-like receptor signaling pathways (207). These could explain the mechanism by which M2 adoptive immunotherapy provides neuroprotection against SCI. However, the specific mechanisms of adoptive immunotherapy remain to be investigated in depth.
Conclusion.
SCI causes complex pathophysiological changes that are fundamental to the pathogenesis of secondary injury. Among all changes, inflammation is the main obstacle to neurological recovery and directly influences disease progression. Macrophages/microglia are one of the primary cells involved in neuroinflammation in SCI. In response to damaged spinal cord microenvironment signals, macrophages/microglia can polarize into pro-inflammatory (M1) and anti-inflammatory (M2) macrophages/microglia. In recent years, many studies have demonstrated that the transition of inflammatory macrophages/microglia to the M2 phenotype leads to a reduced secondary injury and recovery of motor capacity following SCI. Based on insights into the effects of macrophages/microglia and their polarization on SCI and repair, several neuroprotective therapies targeting macrophages/microglia polarization in SCI have been investigated. These approaches have contributed to neural tissue regeneration and functional recovery post-SCI. However, the therapeutic approach to inflammation in SCI should switch from broad promoting macrophages/microglia polarization towards M2 to subtle regulation of the balance among their phenotypes. As mentioned above, excessive accumulation of any one phenotype of macrophages/microglia is detrimental. The accumulation of large numbers of M1 macrophages/microglia results in chronic inflammation and tissue destruction; at the same time, excessive or prolonged M2 polarization can promote scar tissue proliferation that will impede axonal regeneration. As a result, we must carefully enhance the correct phenotype at the right time to effect neuroinflammation regression, reduce secondary damage and promote functional recovery.
Data availability statement
The original contributions presented in the study are included in the article/supplementary material Further inquiries can be directed to the corresponding author.
Author contributions
S-PF wrote the manuscript. S-YC, Q-MP, MZ, X-CW, XW, W-HW, JA, and TZ edited the paper. All authors contributed to the article and approved the submitted version.
Funding
This work was supported by grants from the National Natural Science Foundation of China (No.81960299), the Guizhou Provincical Science and Technology Foundation (No.[2020]1Y324).
Conflict of interest
The authors declare that the research was conducted in the absence of any commercial or financial relationships that could be construed as a potential conflict of interest.
Publisher’s note
All claims expressed in this article are solely those of the authors and do not necessarily represent those of their affiliated organizations, or those of the publisher, the editors and the reviewers. Any product that may be evaluated in this article, or claim that may be made by its manufacturer, is not guaranteed or endorsed by the publisher.
References
1. Feng Z, Min L, Chen H, Deng W, Tan M, Liu H, et al. Iron overload in the motor cortex induces neuronal ferroptosis following spinal cord injury. Redox Biol (2021) 43:101984. doi: 10.1016/j.redox.2021.101984
2. Singh A, Tetreault L, Kalsi-Ryan S, Nouri A, Fehlings MG. Global prevalence and incidence of traumatic spinal cord injury. Clin Epidemiol (2014) 6:309–31. doi: 10.2147/CLEP.S68889
3. Huang W, Lin M, Yang C, Wang F, Zhang M, Gao J, et al. Rat bone mesenchymal stem cell-derived exosomes loaded with miR-494 promoting neurofilament regeneration and behavioral function recovery after spinal cord injury. Oxid Med Cell Longev (2021) 2021:1634917. doi: 10.1155/2021/1634917
4. Hellenbrand DJ, Quinn CM, Piper ZJ, Morehouse CN, Fixel JA, Hanna AS. Inflammation after spinal cord injury: a review of the critical timeline of signaling cues and cellular infiltration. J Neuroinflamm (2021) 18:284. doi: 10.1186/s12974-021-02337-2
5. Wang J, Chen J, Jin H, Lin D, Chen Y, Chen X, et al. BRD4 inhibition attenuates inflammatory response in microglia and facilitates recovery after spinal cord injury in rats. J Cell Mol Med (2019) 23:3214–23. doi: 10.1111/jcmm.14196
6. Liu X, Zhang Y, Wang Y, Qian T. Inflammatory response to spinal cord injury and its treatment. World Neurosurg (2021) 155:19–31. doi: 10.1016/j.wneu.2021.07.148
7. Ahuja CS, Nori S, Tetreault L, Wilson J, Kwon B, Harrop J, et al. Traumatic spinal cord injury-repair and regeneration. Neurosurgery (2017) 80:S9–S22. doi: 10.1093/neuros/nyw080
8. Ahuja CS, Wilson JR, Nori S, Kotter MRN, Druschel C, Curt A, et al. Traumatic spinal cord injury. Nat Rev Dis Primers (2017) 3:17018. doi: 10.1038/nrdp.2017.18
9. Kong X, Gao J. Macrophage polarization: a key event in the secondary phase of acute spinal cord injury. J Cell Mol Med (2017) 21:941–54. doi: 10.1111/jcmm.13034
10. Popovich PG, Hickey WF. Bone marrow chimeric rats reveal the unique distribution of resident and recruited macrophages in the contused rat spinal cord. J Neuropathol Exp Neurol (2001) 60:676–85. doi: 10.1093/jnen/60.7.676
11. Gaojian T, Dingfei Q, Linwei L, Xiaowei W, Zheng Z, Wei L, et al. Parthenolide promotes the repair of spinal cord injury by modulating M1/M2 polarization via the NF-kappaB and STAT 1/3 signaling pathway. Cell Death Discovery (2020) 6:97. doi: 10.1038/s41420-020-00333-8
12. Kobayakawa K, Ohkawa Y, Yoshizaki S, Tamaru T, Saito T, Kijima K, et al. Macrophage centripetal migration drives spontaneous healing process after spinal cord injury. Sci Adv (2019) 5:eaav5086. doi: 10.1126/sciadv.aav5086
13. Van Broeckhoven J, Sommer D, Dooley D, Hendrix S, Franssen A. Macrophage phagocytosis after spinal cord injury: when friends become foes. Brain (2021) 144:2933–45. doi: 10.1093/brain/awab250
14. Hu X, Leak RK, Shi Y, Suenaga J, Gao Y, Zheng P, et al. Microglial and macrophage polarization-new prospects for brain repair. Nat Rev Neurol (2015) 11:56–64. doi: 10.1038/nrneurol.2014.207
15. Sarode P, Zheng X, Giotopoulou GA, Weigert A, Kuenne C, Gunther S, et al. Reprogramming of tumor-associated macrophages by targeting beta-catenin/FOSL2/ARID5A signaling: A potential treatment of lung cancer. Sci Adv (2020) 6:eaaz6105. doi: 10.1126/sciadv.aaz6105
16. Zhou HF, Yan H, Hu Y, Springer LE, Yang X, Wickline SA, et al. Fumagillin prodrug nanotherapy suppresses macrophage inflammatory response via endothelial nitric oxide. ACS Nano (2014) 8:7305–17. doi: 10.1021/nn502372n
17. Weng YS, Tseng HY, Chen YA, Shen PC, Al Haq AT, Chen LM, et al. MCT-1/miR-34a/IL-6/IL-6R signaling axis promotes EMT progression, cancer stemness and M2 macrophage polarization in triple-negative breast cancer. Mol Cancer (2019) 18:42. doi: 10.1186/s12943-019-0988-0
18. Ren Y, Young W. Managing inflammation after spinal cord injury through manipulation of macrophage function. Neural Plast (2013) 2013:945034. doi: 10.1155/2013/945034
19. Golabchi A, Wu B, Li X, Carlisle DL, Kozai TDY, Friedlander RM, et al. Melatonin improves quality and longevity of chronic neural recording. Biomaterials (2018) 180:225–39. doi: 10.1016/j.biomaterials.2018.07.026
20. Sun G, Yang S, Cao G, Wang Q, Hao J, Wen Q, et al. Gammadelta T cells provide the early source of IFN-gamma to aggravate lesions in spinal cord injury. J Exp Med (2018) 215:521–35. doi: 10.1084/jem.20170686
21. Njock MS, Cheng HS, Dang LT, Nazari-Jahantigh M, Lau AC, Boudreau E, et al. Endothelial cells suppress monocyte activation through secretion of extracellular vesicles containing antiinflammatory microRNAs. Blood (2015) 125:3202–12. doi: 10.1182/blood-2014-11-611046
22. Wang L, Wu JP, He XJ. Butylphthalide has an anti-inflammatory role in spinal cord injury by promoting Macrophage/Microglia M2 polarization via p38 phosphorylation. Spine (Phila Pa 1976) (2020) 45:E1066–76. doi: 10.1097/BRS.0000000000003503
23. Zhang B, Bailey WM, Kopper TJ, Orr MB, Feola DJ, Gensel JC. Azithromycin drives alternative macrophage activation and improves recovery and tissue sparing in contusion spinal cord injury. J Neuroinflamm (2015) 12:218. doi: 10.1186/s12974-015-0440-3
24. Tran AP, Warren PM, Silver J. The biology of regeneration failure and success after spinal cord injury. Physiol Rev (2018) 98:881–917. doi: 10.1152/physrev.00017.2017.
25. Jiang Y, Fu P, Liu Y, Wang C, Zhao P, Chu X, et al. Near-infrared light-triggered NO release for spinal cord injury repair. Sci Adv (2020) 6:eabc3513. doi: 10.1126/sciadv.abc3513
26. Ge X, Tang P, Rong Y, Jiang D, Lu X, Ji C, et al. Exosomal miR-155 from M1-polarized macrophages promotes EndoMT and impairs mitochondrial function via activating NF-kappaB signaling pathway in vascular endothelial cells after traumatic spinal cord injury. Redox Biol (2021) 41:101932. doi: 10.1016/j.redox.2021.101932
27. Yao C, Cao X, Yu B. Revascularization after traumatic spinal cord injury. Front Physiol (2021) 12:631500. doi: 10.3389/fphys.2021.631500
28. Matsushita T, Lankford KL, Arroyo EJ, Sasaki M, Neyazi M, Radtke C, et al. Diffuse and persistent blood-spinal cord barrier disruption after contusive spinal cord injury rapidly recovers following intravenous infusion of bone marrow mesenchymal stem cells. Exp Neurol (2015) 267:152–64. doi: 10.1016/j.expneurol.2015.03.001
29. Xin W, Qiang S, Jianing D, Jiaming L, Fangqi L, Bin C, et al. Human bone marrow mesenchymal stem cell-derived exosomes attenuate blood-spinal cord barrier disruption via the TIMP2/MMP pathway after acute spinal cord injury. Mol Neurobiol (2021) 58:6490–504. doi: 10.1007/s12035-021-02565-w
30. Ying X, Xie Q, Li S, Yu X, Zhou K, Yue J, et al. Water treadmill training attenuates blood-spinal cord barrier disruption in rats by promoting angiogenesis and inhibiting matrix metalloproteinase-2/9 expression following spinal cord injury. Fluids Barriers CNS (2020) 17:70. doi: 10.1186/s12987-020-00232-1
31. Mietto BS, Mostacada K, Martinez AM. Neurotrauma and inflammation: CNS and PNS responses. Mediators Inflammation (2015) 2015:251204. doi: 10.1155/2015/251204
32. Liu H, Zhang J, Xu X, Lu S, Yang D, Xie C, et al. SARM1 promotes neuroinflammation and inhibits neural regeneration after spinal cord injury through NF-kappaB signaling. Theranostics (2021) 11:4187–206. doi: 10.7150/thno.49054
33. Lukacova N, Kisucka A, Kiss Bimbova K, Bacova M, Ileninova M, Kuruc T, et al. Glial-neuronal interactions in pathogenesis and treatment of spinal cord injury. Int J Mol Sci (2021) 22:13577. doi: 10.3390/ijms222413577
34. Maldonado-Lasuncion I, Verhaagen J, Oudega M. Mesenchymal stem cell-macrophage choreography supporting spinal cord repair. Neurotherapeutics (2018) 15:578–87. doi: 10.1007/s13311-018-0629-0
35. Bellver-Landete V, Bretheau F, Mailhot B, Vallieres N, Lessard M, Janelle ME, et al. Microglia are an essential component of the neuroprotective scar that forms after spinal cord injury. Nat Commun (2019) 10:518. doi: 10.1038/s41467-019-08446-0
36. Fan H, Tang HB, Chen Z, Wang HQ, Zhang L, Jiang Y, et al. Inhibiting HMGB1-RAGE axis prevents pro-inflammatory macrophages/microglia polarization and affords neuroprotection after spinal cord injury. J Neuroinflamm (2020) 17:295. doi: 10.1186/s12974-020-01973-4
37. Ji H, Zhang Y, Chen C, Li H, He B, Yang T, et al. D-dopachrome tautomerase activates COX2/PGE2 pathway of astrocytes to mediate inflammation following spinal cord injury. J Neuroinflamm (2021) 18:130. doi: 10.1186/s12974-021-02186-z
38. Sawyer AJ, Tian W, Saucier-Sawyer JK, Rizk PJ, Saltzman WM, Bellamkonda RV, et al. The effect of inflammatory cell-derived MCP-1 loss on neuronal survival during chronic neuroinflammation. Biomaterials (2014) 35:6698–706. doi: 10.1016/j.biomaterials.2014.05.008
39. Yang T, Zhu Z, Yin E, Wang Y, Zhang C, Yuan H, et al. Alleviation of symptoms of alzheimer's disease by diminishing abeta neurotoxicity and neuroinflammation. Chem Sci (2019) 10:10149–58. doi: 10.1039/c9sc03042e
40. Jiang X, Yu M, Ou Y, Cao Y, Yao Y, Cai P, et al. Downregulation of USP4 promotes activation of microglia and subsequent neuronal inflammation in rat spinal cord after injury. Neurochem Res (2017) 42:3245–53. doi: 10.1007/s11064-017-2361-2
41. Chen S, Ye J, Chen X, Shi J, Wu W, Lin W, et al. Valproic acid attenuates traumatic spinal cord injury-induced inflammation via STAT1 and NF-kappaB pathway dependent of HDAC3. J Neuroinflamm (2018) 15:150. doi: 10.1186/s12974-018-1193-6
42. Fu H, Zhao Y, Hu D, Wang S, Yu T, Zhang L. Depletion of microglia exacerbates injury and impairs function recovery after spinal cord injury in mice. Cell Death Dis (2020) 11:528. doi: 10.1038/s41419-020-2733-4
43. Farina C, Aloisi F, Meinl E. Astrocytes are active players in cerebral innate immunity. Trends Immunol (2007) 28:138–45. doi: 10.1016/j.it.2007.01.005
44. Chapouly C, Tadesse Argaw A, Horng S, Castro K, Zhang J, Asp L, et al. And VEGFA drive blood-brain barrier opening in inflammatory central nervous system lesions. Brain (2015) 138:1548–67. doi: 10.1093/brain/awv077
45. Zhang Y, Zhou Y, Chen S, Hu Y, Zhu Z, Wang Y, et al. Macrophage migration inhibitory factor facilitates prostaglandin E2 production of astrocytes to tune inflammatory milieu following spinal cord injury. J Neuroinflamm (2019) 16:85. doi: 10.1186/s12974-019-1468-6
46. Li X, Li M, Tian L, Chen J, Liu R, Ning B. Reactive astrogliosis: Implications in spinal cord injury progression and therapy. Oxid Med Cell Longev (2020) 2020:9494352. doi: 10.1155/2020/9494352
47. Zuidema JM, Hyzinski-Garcia MC, Van Vlasselaer K, Zaccor NW, Plopper GE, Mongin AA, et al. Enhanced GLT-1 mediated glutamate uptake and migration of primary astrocytes directed by fibronectin-coated electrospun poly-l-lactic acid fibers. Biomaterials (2014) 35:1439–49. doi: 10.1016/j.biomaterials.2013.10.079
48. Yates AG, Jogia T, Gillespie ER, Couch Y, Ruitenberg MJ, Anthony DC. Acute IL-1RA treatment suppresses the peripheral and central inflammatory response to spinal cord injury. J Neuroinflamm (2021) 18:15. doi: 10.1186/s12974-020-02050-6
49. Neirinckx V, Coste C, Franzen R, Gothot A, Rogister B, Wislet S. Neutrophil contribution to spinal cord injury and repair. J Neuroinflamm (2014) 11:150. doi: 10.1186/s12974-014-0150-2
50. Pang QM, Chen SY, Xu QJ, Fu SP, Yang YC, Zou WH, et al. Neuroinflammation and scarring after spinal cord injury: Therapeutic roles of MSCs on inflammation and glial scar. Front Immunol (2021) 12:751021. doi: 10.3389/fimmu.2021.751021
51. Feng Z, Min L, Liang L, Chen B, Chen H, Zhou Y, et al. Neutrophil extracellular traps exacerbate secondary injury via promoting neuroinflammation and blood-spinal cord barrier disruption in spinal cord injury. Front Immunol (2021) 12:698249. doi: 10.3389/fimmu.2021.698249
52. Zeng MY, Pham D, Bagaitkar J, Liu J, Otero K, Shan M, et al. An efferocytosis-induced, IL-4-dependent macrophage-iNKT cell circuit suppresses sterile inflammation and is defective in murine CGD. Blood (2013) 121:3473–83. doi: 10.1182/blood-2012-10-461913
53. Kwak HJ, Liu P, Bajrami B, Xu Y, Park SY, Nombela-Arrieta C, et al. Myeloid cell-derived reactive oxygen species externally regulate the proliferation of myeloid progenitors in emergency granulopoiesis. Immunity (2015) 42:159–71. doi: 10.1016/j.immuni.2014.12.017
54. Popovich PG, Guan Z, Wei P, Huitinga I, van Rooijen N, Stokes BT. Depletion of hematogenous macrophages promotes partial hindlimb recovery and neuroanatomical repair after experimental spinal cord injury. Exp Neurol (1999) 158:351–65. doi: 10.1006/exnr.1999.7118
55. Jakovcevski I, Forster E, Reiss G, Schachner M. Impact of depletion of Microglia/Macrophages on regeneration after spinal cord injury. Neuroscience (2021) 459:129–41. doi: 10.1016/j.neuroscience.2021.02.010
56. Donnelly DJ, Longbrake EE, Shawler TM, Kigerl KA, Lai W, Tovar CA, et al. Deficient CX3CR1 signaling promotes recovery after mouse spinal cord injury by limiting the recruitment and activation of Ly6Clo/iNOS+ macrophages. J Neurosci (2011) 31:9910–22. doi: 10.1523/JNEUROSCI.2114-11.2011
57. Luo Y, Yao F, Hu X, Li Y, Chen Y, Li Z, et al. M1 macrophages impair tight junctions between endothelial cells after spinal cord injury. Brain Res Bull (2022) 180:59–72. doi: 10.1016/j.brainresbull.2021.12.019
58. Garcia E, Aguilar-Cevallos J, Silva-Garcia R, Ibarra A. Cytokine and growth factor activation In vivo and In vitro after spinal cord injury. Mediators Inflammation (2016) 2016:9476020. doi: 10.1155/2016/9476020
59. Beck KD, Nguyen HX, Galvan MD, Salazar DL, Woodruff TM, Anderson AJ. Quantitative analysis of cellular inflammation after traumatic spinal cord injury: evidence for a multiphasic inflammatory response in the acute to chronic environment. Brain (2010) 133:433–47. doi: 10.1093/brain/awp322
60. Ishii H, Jin X, Ueno M, Tanabe S, Kubo T, Serada S, et al. Adoptive transfer of Th1-conditioned lymphocytes promotes axonal remodeling and functional recovery after spinal cord injury. Cell Death Dis (2012) 3:e363. doi: 10.1038/cddis.2012.106
61. Ruytinx P, Proost P, Van Damme J, Struyf S. Chemokine-induced macrophage polarization in inflammatory conditions. Front Immunol (2018) 9:1930. doi: 10.3389/fimmu.2018.01930
62. Bermudez S, Khayrullina G, Zhao Y, Byrnes KR. NADPH oxidase isoform expression is temporally regulated and may contribute to microglial/macrophage polarization after spinal cord injury. Mol Cell Neurosci (2016) 77:53–64. doi: 10.1016/j.mcn.2016.10.001
63. Herd HL, Bartlett KT, Gustafson JA, McGill LD, Ghandehari H. Macrophage silica nanoparticle response is phenotypically dependent. Biomaterials (2015) 53:574–82. doi: 10.1016/j.biomaterials.2015.02.070
64. Fan H, Tang HB, Shan LQ, Liu SC, Huang DG, Chen X, et al. Quercetin prevents necroptosis of oligodendrocytes by inhibiting macrophages/microglia polarization to M1 phenotype after spinal cord injury in rats. J Neuroinflamm (2019) 16:206. doi: 10.1186/s12974-019-1613-2
65. Fan H, Zhang K, Shan L, Kuang F, Chen K, Zhu K, et al. Reactive astrocytes undergo M1 microglia/macrohpages-induced necroptosis in spinal cord injury. Mol Neurodegener (2016) 11:14. doi: 10.1186/s13024-016-0081-8
66. Alexander KA, Flynn R, Lineburg KE, Kuns RD, Teal BE, Olver SD, et al. CSF-1-dependant donor-derived macrophages mediate chronic graft-versus-host disease. J Clin Invest (2014) 124:4266–80. doi: 10.1172/JCI75935
67. Guo M, Hartlova A, Gierlinski M, Prescott A, Castellvi J, Losa JH, et al. Triggering MSR1 promotes JNK-mediated inflammation in IL-4-activated macrophages. EMBO J (2019) 38:e100299. doi: 10.15252/embj.2018100299
68. Hellenbrand DJ, Reichl KA, Travis BJ, Filipp ME, Khalil AS, Pulito DJ, et al. Sustained interleukin-10 delivery reduces inflammation and improves motor function after spinal cord injury. J Neuroinflamm (2019) 16:93. doi: 10.1186/s12974-019-1479-3
69. Tang TT, Wang B, Wu M, Li ZL, Feng Y, Cao JY, et al. Extracellular vesicle-encapsulated IL-10 as novel nanotherapeutics against ischemic AKI. Sci Adv (2020) 6:eaaz0748. doi: 10.1126/sciadv.aaz0748
70. Zajac E, Schweighofer B, Kupriyanova TA, Juncker-Jensen A, Minder P, Quigley JP, et al. Angiogenic capacity of M1- and M2-polarized macrophages is determined by the levels of TIMP-1 complexed with their secreted proMMP-9. Blood (2013) 122:4054–67. doi: 10.1182/blood-2013-05-501494
71. Han GH, Kim SJ, Ko WK, Lee D, Han IB, Sheen SH, et al. Transplantation of tauroursodeoxycholic acid-inducing M2-phenotype macrophages promotes an anti-neuroinflammatory effect and functional recovery after spinal cord injury in rats. Cell Prolif (2021) 54:e13050. doi: 10.1111/cpr.13050
72. Miron VE, Boyd A, Zhao JW, Yuen TJ, Ruckh JM, Shadrach JL, et al. M2 microglia and macrophages drive oligodendrocyte differentiation during CNS remyelination. Nat Neurosci (2013) 16:1211–8. doi: 10.1038/nn.3469
73. David S, Kroner A. Repertoire of microglial and macrophage responses after spinal cord injury. Nat Rev Neurosci (2011) 12:388–99. doi: 10.1038/nrn3053
74. Song G, Yang R, Zhang Q, Chen L, Huang D, Zeng J, et al. TGF-beta secretion by M2 macrophages induces glial scar formation by activating astrocytes in vitro. J Mol Neurosci (2019) 69:324–32. doi: 10.1007/s12031-019-01361-5
75. Wang Y, Zhang L, Wu GR, Zhou Q, Yue H, Rao LZ, et al. MBD2 serves as a viable target against pulmonary fibrosis by inhibiting macrophage M2 program. Sci Adv (2021) 7:eabb6075. doi: 10.1126/sciadv.abb6075
76. Liu WZ, Ma ZJ, Li JR, Kang XW. Mesenchymal stem cell-derived exosomes: therapeutic opportunities and challenges for spinal cord injury. Stem Cell Res Ther (2021) 12:102. doi: 10.1186/s13287-021-02153-8
77. Munoz J, Akhavan NS, Mullins AP, Arjmandi BH. Macrophage polarization and osteoporosis: A review. Nutrients (2020) 12:2999. doi: 10.3390/nu12102999
78. Wang C, Ma C, Gong L, Guo Y, Fu K, Zhang Y, et al. Macrophage polarization and its role in liver disease. Front Immunol (2021) 12:803037. doi: 10.3389/fimmu.2021.803037
79. Abernathy LM, Fountain MD, Rothstein SE, David JM, Yunker CK, Rakowski J, et al. Soy isoflavones promote radioprotection of normal lung tissue by inhibition of radiation-induced activation of macrophages and neutrophils. J Thorac Oncol (2015) 10:1703–12. doi: 10.1097/JTO.0000000000000677
80. Zhang Y, Choksi S, Chen K, Pobezinskaya Y, Linnoila I, Liu ZG. ROS play a critical role in the differentiation of alternatively activated macrophages and the occurrence of tumor-associated macrophages. Cell Res (2013) 23:898–914. doi: 10.1038/cr.2013.75
81. Sica A, Erreni M, Allavena P, Porta C. Macrophage polarization in pathology. Cell Mol Life Sci (2015) 72:4111–26. doi: 10.1007/s00018-015-1995-y
82. Saradna A, Do DC, Kumar S, Fu QL, Gao P. Macrophage polarization and allergic asthma. Transl Res (2018) 191:1–14. doi: 10.1016/j.trsl.2017.09.002
83. Wang F, Zhang S, Jeon R, Vuckovic I, Jiang X, Lerman A, et al. Interferon gamma induces reversible metabolic reprogramming of M1 macrophages to sustain cell viability and pro-inflammatory activity. EBioMedicine (2018) 30:303–16. doi: 10.1016/j.ebiom.2018.02.009
84. Quero L, Tiaden AN, Hanser E, Roux J, Laski A, Hall J, et al. miR-221-3p drives the shift of M2-macrophages to a pro-inflammatory function by suppressing JAK3/STAT3 activation. Front Immunol (2019) 10:3087. doi: 10.3389/fimmu.2019.03087
85. Panagi I, Jennings E, Zeng J, Gunster RA, Stones CD, Mak H, et al. Salmonella effector SteE converts the mammalian Serine/Threonine kinase GSK3 into a tyrosine kinase to direct macrophage polarization. Cell Host Microbe (2020) 27:41–53.e6. doi: 10.1016/j.chom.2019.11.002
86. Zhang Q, Atsuta I, Liu S, Chen C, Shi S, Shi S, et al. IL-17-mediated M1/M2 macrophage alteration contributes to pathogenesis of bisphosphonate-related osteonecrosis of the jaws. Clin Cancer Res (2013) 19:3176–88. doi: 10.1158/1078-0432.CCR-13-0042
87. Yao Y, Xu XH, Jin L. Macrophage polarization in physiological and pathological pregnancy. Front Immunol (2019) 10:792. doi: 10.3389/fimmu.2019.00792
88. Vayrynen JP, Haruki K, Lau MC, Vayrynen SA, Zhong R, Dias Costa A, et al. The prognostic role of macrophage polarization in the colorectal cancer microenvironment. Cancer Immunol Res (2021) 9:8–19. doi: 10.1158/2326-6066.CIR-20-0527
89. Liu M, Tong Z, Ding C, Luo F, Wu S, Wu C, et al. Transcription factor c-maf is a checkpoint that programs macrophages in lung cancer. J Clin Invest (2020) 130:2081–96. doi: 10.1172/JCI131335
90. Lacerda Mariano L, Rousseau M, Varet H, Legendre R, Gentek R, Saenz Coronilla J, et al. Functionally distinct resident macrophage subsets differentially shape responses to infection in the bladder. Sci Adv (2020) 6:eabc5739. doi: 10.1126/sciadv.abc5739
91. Petty AJ, Li A, Wang X, Dai R, Heyman B, Hsu D, et al. Hedgehog signaling promotes tumor-associated macrophage polarization to suppress intratumoral CD8+ T cell recruitment. J Clin Invest (2019) 129:5151–62. doi: 10.1172/JCI128644
92. O'Shea JJ, Paul WE. Mechanisms underlying lineage commitment and plasticity of helper CD4+ T cells. Science (2010) 327:1098–102. doi: 10.1126/science.1178334
93. Murray PJ. Macrophage polarization. Annu Rev Physiol (2017) 79:541–66. doi: 10.1146/annurev-physiol-022516-034339
94. Su S, Zhao Q, He C, Huang D, Liu J, Chen F, et al. miR-142-5p and miR-130a-3p are regulated by IL-4 and IL-13 and control profibrogenic macrophage program. Nat Commun (2015) 6:8523. doi: 10.1038/ncomms9523
95. Wei Q, Sha Y, Bhattacharya A, Abdel Fattah E, Bonilla D, Jyothula SS, et al. Regulation of IL-4 receptor signaling by STUB1 in lung inflammation. Am J Respir Crit Care Med (2014) 189:16–29. doi: 10.1164/rccm.201305-0874OC
96. Pang QM, Yang R, Zhang M, Zou WH, Qian NN, Xu QJ, et al. Peripheral blood-derived mesenchymal stem cells modulate macrophage plasticity through the IL-10/STAT3 pathway. Stem Cells Int (2022) 2022:5181241. doi: 10.1155/2022/5181241
97. Xu H, Zhu J, Smith S, Foldi J, Zhao B, Chung AY, et al. Notch-RBP-J signaling regulates the transcription factor IRF8 to promote inflammatory macrophage polarization. Nat Immunol (2012) 13:642–50. doi: 10.1038/ni.2304
98. Nakamura A, Ebina-Shibuya R, Itoh-Nakadai A, Muto A, Shima H, Saigusa D, et al. Transcription repressor Bach2 is required for pulmonary surfactant homeostasis and alveolar macrophage function. J Exp Med (2013) 210:2191–204. doi: 10.1084/jem.20130028
99. Yu T, Gao M, Yang P, Liu D, Wang D, Song F, et al. Insulin promotes macrophage phenotype transition through PI3K/Akt and PPAR-gamma signaling during diabetic wound healing. J Cell Physiol (2019) 234:4217–31. doi: 10.1002/jcp.27185
100. Pan Y, Hui X, Hoo RLC, Ye D, Chan CYC, Feng T, et al. Adipocyte-secreted exosomal microRNA-34a inhibits M2 macrophage polarization to promote obesity-induced adipose inflammation. J Clin Invest (2019) 129:834–49. doi: 10.1172/JCI123069
101. Li P, Ma C, Li J, You S, Dang L, Wu J, et al. Proteomic characterization of four subtypes of M2 macrophages derived from human THP-1 cells. J Zhejiang Univ Sci B (2022) 23:407–22. doi: 10.1631/jzus.B2100930
102. Shan K, Feng N, Cui J, Wang S, Qu H, Fu G, et al. Resolvin D1 and D2 inhibit tumour growth and inflammation via modulating macrophage polarization. J Cell Mol Med (2020) 24:8045–56. doi: 10.1111/jcmm.15436
103. Wang LX, Zhang SX, Wu HJ, Rong XL, Guo J. M2b macrophage polarization and its roles in diseases. J Leukoc Biol (2019) 106:345–58. doi: 10.1002/JLB.3RU1018-378RR
104. Zhang J, Li Y, Duan Z, Kang J, Chen K, Li G, et al. The effects of the M2a macrophage-induced axonal regeneration of neurons by arginase 1. Biosci Rep (2020) 40::BSR20193031. doi: 10.1042/BSR20193031
105. Shapouri-Moghaddam A, Mohammadian S, Vazini H, Taghadosi M, Esmaeili SA, Mardani F, et al. Macrophage plasticity, polarization, and function in health and disease. J Cell Physiol (2018) 233:6425–40. doi: 10.1002/jcp.26429
106. Yang R, Liao Y, Wang L, He P, Hu Y, Yuan D, et al. Exosomes derived from M2b macrophages attenuate DSS-induced colitis. Front Immunol (2019) 10:2346. doi: 10.3389/fimmu.2019.02346
107. Zhang B, Bailey WM, Braun KJ, Gensel JC. Age decreases macrophage IL-10 expression: Implications for functional recovery and tissue repair in spinal cord injury. Exp Neurol (2015) 273:83–91. doi: 10.1016/j.expneurol.2015.08.001
108. Junior YS, Nguyen HT, Salmanida FP, Chang KT. MERTK(+/hi) M2c macrophages induced by baicalin alleviate non-alcoholic fatty liver disease. Int J Mol Sci (2021) 22:10604. doi: 10.3390/ijms221910604
109. Avila-Ponce de Leon U, Vazquez-Jimenez A, Matadamas-Guzman M, Pelayo R, Resendis-Antonio O. Transcriptional and microenvironmental landscape of macrophage transition in cancer: A boolean analysis. Front Immunol (2021) 12:642842. doi: 10.3389/fimmu.2021.642842
110. Martinez FO, Gordon S. The M1 and M2 paradigm of macrophage activation: time for reassessment. F1000Prime Rep (2014) 6:13. doi: 10.12703/P6-13
111. Gensel JC, Zhang B. Macrophage activation and its role in repair and pathology after spinal cord injury. Brain Res (2015) 1619:1–11. doi: 10.1016/j.brainres.2014.12.045
112. Smith AM, Dragunow M. The human side of microglia. Trends Neurosci (2014) 37:125–35. doi: 10.1016/j.tins.2013.12.001
113. Raes G, Van den Bergh R, De Baetselier P, Ghassabeh GH, Scotton C, Locati M, et al. Arginase-1 and Ym1 are markers for murine, but not human, alternatively activated myeloid cells. J Immunol (2005) 174:6561. doi: 10.4049/jimmunol.174.11.6561
114. Murray PJ, Wynn TA. Obstacles and opportunities for understanding macrophage polarization. J Leukoc Biol (2011) 89:557–63. doi: 10.1189/jlb.0710409
115. Tarique AA, Logan J, Thomas E, Holt PG, Sly PD, Fantino E. Phenotypic, functional, and plasticity features of classical and alternatively activated human macrophages. Am J Respir Cell Mol Biol (2015) 53:676–88. doi: 10.1165/rcmb.2015-0012OC
116. Mantovani A, Marchesi F, Malesci A, Laghi L, Allavena P. Tumour-associated macrophages as treatment targets in oncology. Nat Rev Clin Oncol (2017) 14:399–416. doi: 10.1038/nrclinonc.2016.217
117. Qian BZ, Pollard JW. Macrophage diversity enhances tumor progression and metastasis. Cell (2010) 141:39–51. doi: 10.1016/j.cell.2010.03.014
118. Atri C, Guerfali FZ, Laouini D. Role of human macrophage polarization in inflammation during infectious diseases. Int J Mol Sci (2018) 19:1801. doi: 10.3390/ijms19061801
119. Italiani P, Mazza EM, Lucchesi D, Cifola I, Gemelli C, Grande A, et al. Transcriptomic profiling of the development of the inflammatory response in human monocytes in vitro. PloS One (2014) 9:e87680. doi: 10.1371/journal.pone.0087680
120. Rackov G, Hernandez-Jimenez E, Shokri R, Carmona-Rodriguez L, Manes S, Alvarez-Mon M, et al. p21 mediates macrophage reprogramming through regulation of p50-p50 NF-kappaB and IFN-beta. J Clin Invest (2016) 126:3089–103. doi: 10.1172/JCI83404
121. Silva AM, Moura SR, Teixeira JH, Barbosa MA, Santos SG, Almeida MI. Long noncoding RNAs: a missing link in osteoporosis. Bone Res (2019) 7:10. doi: 10.1038/s41413-019-0048-9
122. Bao C, Wang B, Yang F, Chen L. Blockade of interleukin-7 receptor shapes macrophage alternative activation and promotes functional recovery after spinal cord injury. Neuroscience (2018) 371:518–27. doi: 10.1016/j.neuroscience.2017.10.022
123. Guerrero AR, Uchida K, Nakajima H, Watanabe S, Nakamura M, Johnson WE, et al. Blockade of interleukin-6 signaling inhibits the classic pathway and promotes an alternative pathway of macrophage activation after spinal cord injury in mice. J Neuroinflamm (2012) 9:40. doi: 10.1186/1742-2094-9-40
124. Ma J, Liu R, Wang X, Liu Q, Chen Y, Valle RP, et al. Crucial role of lateral size for graphene oxide in activating macrophages and stimulating pro-inflammatory responses in cells and animals. ACS Nano (2015) 9:10498–515. doi: 10.1021/acsnano.5b04751
125. Chengke L, Weiwei L, Xiyang W, Ping W, Xiaoyang P, Zhengquan X, et al. Effect of infliximab combined with methylprednisolone on expressions of NF-kappaB, TRADD, and FADD in rat acute spinal cord injury. Spine (Phila Pa 1976) (2013) 38:E861–9. doi: 10.1097/BRS.0b013e318294892c
126. Wang L, Wei FX, Cen JS, Ping SN, Li ZQ, Chen NN, et al. Early administration of tumor necrosis factor-alpha antagonist promotes survival of transplanted neural stem cells and axon myelination after spinal cord injury in rats. Brain Res (2014) 1575:87–100. doi: 10.1016/j.brainres.2014.05.038
127. Vidal PM, Lemmens E, Geboes L, Vangansewinkel T, Nelissen S, Hendrix S. Late blocking of peripheral TNF-alpha is ineffective after spinal cord injury in mice. Immunobiology (2013) 218:281–4. doi: 10.1016/j.imbio.2012.05.007
128. Li J, Liu Y, Xu H, Fu Q. Nanoparticle-delivered IRF5 siRNA facilitates M1 to M2 transition, reduces demyelination and neurofilament loss, and promotes functional recovery after spinal cord injury in mice. Inflammation (2016) 39:1704–17. doi: 10.1007/s10753-016-0405-4
129. Francos-Quijorna I, Amo-Aparicio J, Martinez-Muriana A, Lopez-Vales R. IL-4 drives microglia and macrophages toward a phenotype conducive for tissue repair and functional recovery after spinal cord injury. Glia (2016) 64:2079–92. doi: 10.1002/glia.23041
130. Lima R, Monteiro S, Lopes JP, Barradas P, Vasconcelos NL, Gomes ED, et al. Systemic interleukin-4 administration after spinal cord injury modulates inflammation and promotes neuroprotection. Pharm (Basel) (2017) 10423–4. doi: 10.3390/ph10040083
131. Thompson CD, Zurko JC, Hanna BF, Hellenbrand DJ, Hanna A. The therapeutic role of interleukin-10 after spinal cord injury. J Neurotrauma (2013) 30:1311–24. doi: 10.1089/neu.2012.2651
132. Luo W, Xu Q, Wang Q, Wu H, Hua J. Effect of modulation of PPAR-gamma activity on kupffer cells M1/M2 polarization in the development of non-alcoholic fatty liver disease. Sci Rep (2017) 7:44612. doi: 10.1038/srep44612
133. Zhang Y, Shi X, Han J, Peng W, Fang Z, Zhou Y, et al. Convallatoxin promotes M2 macrophage polarization to attenuate atherosclerosis through PPARgamma-integrin alphavbeta5 signaling pathway. Drug Des Devel Ther (2021) 15:803–12. doi: 10.2147/DDDT.S288728
134. Cheng WL, Zhang Q, Li B, Cao JL, Jiao L, Chao SP, et al. PAK1 silencing attenuated proinflammatory macrophage activation and foam cell formation by increasing PPARgamma expression. Oxid Med Cell Longev (2021) 2021:6957900. doi: 10.1155/2021/6957900
135. Abdalla HB, Napimoga MH, Lopes AH, de Macedo Maganin AG, Cunha TM, Van Dyke TE, et al. Activation of PPAR-gamma induces macrophage polarization and reduces neutrophil migration mediated by heme oxygenase 1. Int Immunophar (2020) 84:106565. doi: 10.1016/j.intimp.2020.106565
136. McTigue DM, Tripathi R, Wei P, Lash AT. The PPAR gamma agonist pioglitazone improves anatomical and locomotor recovery after rodent spinal cord injury. Exp Neurol (2007) 205:396–406. doi: 10.1016/j.expneurol.2007.02.009
137. Schutz B, Reimann J, Dumitrescu-Ozimek L, Kappes-Horn K, Landreth GE, Schurmann B, et al. The oral antidiabetic pioglitazone protects from neurodegeneration and amyotrophic lateral sclerosis-like symptoms in superoxide dismutase-G93A transgenic mice. J Neurosci (2005) 25:7805–12. doi: 10.1523/JNEUROSCI.2038-05.2005
138. Zhao X, Zhang Y, Strong R, Grotta JC, Aronowski J. 15d-prostaglandin J2 activates peroxisome proliferator-activated receptor-gamma, promotes expression of catalase, and reduces inflammation, behavioral dysfunction, and neuronal loss after intracerebral hemorrhage in rats. J Cereb Blood Flow Metab (2006) 26:811–20. doi: 10.1038/sj.jcbfm.9600233
139. Li H, Zhang Q, Yang X, Wang L. PPAR-gamma agonist rosiglitazone reduces autophagy and promotes functional recovery in experimental traumaticspinal cord injury. Neurosci Lett (2017) 650:89–96. doi: 10.1016/j.neulet.2017.02.075
140. Meng QQ, Feng ZC, Zhang XL, Hu LQ, Wang M, Zhang HF, et al. PPAR-gamma activation exerts an anti-inflammatory effect by suppressing the NLRP3 inflammasome in spinal cord-derived neurons. Mediators Inflammation (2019) 2019:6386729. doi: 10.1155/2019/6386729
141. Orr MB, Gensel JC. Spinal cord injury scarring and inflammation: Therapies targeting glial and inflammatory responses. Neurotherapeutics (2018) 15:541–53. doi: 10.1007/s13311-018-0631-6
142. Liu F, Qiu H, Xue M, Zhang S, Zhang X, Xu J, et al. MSC-secreted TGF-beta regulates lipopolysaccharide-stimulated macrophage M2-like polarization via the Akt/FoxO1 pathway. Stem Cell Res Ther (2019) 10:345. doi: 10.1186/s13287-019-1447-y
143. Francois M, Romieu-Mourez R, Li M, Galipeau J. Human MSC suppression correlates with cytokine induction of indoleamine 2,3-dioxygenase and bystander M2 macrophage differentiation. Mol Ther (2012) 20:187–95. doi: 10.1038/mt.2011.189
144. Zhao Y, Zhu XY, Song T, Zhang L, Eirin A, Conley S, et al. Mesenchymal stem cells protect renal tubular cells via TSG-6 regulating macrophage function and phenotype switching. Am J Physiol Renal Physiol (2021) 320:F454–63. doi: 10.1152/ajprenal.00426.2020
145. Wang J, Liu Y, Ding H, Shi X, Ren H. Mesenchymal stem cell-secreted prostaglandin E2 ameliorates acute liver failure via attenuation of cell death and regulation of macrophage polarization. Stem Cell Res Ther (2021) 12:15. doi: 10.1186/s13287-020-02070-2
146. Yang R, Gao H, Chen L, Fang N, Chen H, Song G, et al. Effect of peripheral blood-derived mesenchymal stem cells on macrophage polarization and Th17/Treg balance in vitro. Regener Ther (2020) 14:275–83. doi: 10.1016/j.reth.2020.03.008
147. Pang QM, Qian NN, Zou WH, Yang YC, Chen H, Zhang M, et al. PBMSCs transplantation facilitates functional recovery after spinal cord injury by regulating microglia/macrophages plasticity. Transpl Immunol (2022) 72:101592. doi: 10.1016/j.trim.2022.101592
148. Nakajima H, Uchida K, Guerrero AR, Watanabe S, Sugita D, Takeura N, et al. Transplantation of mesenchymal stem cells promotes an alternative pathway of macrophage activation and functional recovery after spinal cord injury. J Neurotrauma (2012) 29:1614–25. doi: 10.1089/neu.2011.2109
149. Zheng G, Ge M, Qiu G, Shu Q, Xu J. Mesenchymal stromal cells affect disease outcomes via macrophage polarization. Stem Cells Int (2015) 2015:989473. doi: 10.1155/2015/989473
150. Pang QM, Chen SY, Fu SP, Zhou H, Zhang Q, Ao J, et al. Regulatory role of mesenchymal stem cells on secondary inflammation in spinal cord injury. J Inflammation Res (2022) 15:573–93. doi: 10.2147/JIR.S349572
151. Miceli V, Bulati M, Iannolo G, Zito G, Gallo A, Conaldi PG. Therapeutic properties of mesenchymal Stromal/Stem cells: The need of cell priming for cell-free therapies in regenerative medicine. Int J Mol Sci (2021) 22:763. doi: 10.3390/ijms22020763
152. Hu C, Li L. Preconditioning influences mesenchymal stem cell properties in vitro and in vivo. J Cell Mol Med (2018) 22:1428–42. doi: 10.1111/jcmm.13492
153. Ferreira JR, Teixeira GQ, Santos SG, Barbosa MA, Almeida-Porada G, Goncalves RM. Mesenchymal stromal cell secretome: Influencing therapeutic potential by cellular pre-conditioning. Front Immunol (2018) 9:2837. doi: 10.3389/fimmu.2018.02837
154. Lee BC, Kang KS. Functional enhancement strategies for immunomodulation of mesenchymal stem cells and their therapeutic application. Stem Cell Res Ther (2020) 11:397. doi: 10.1186/s13287-020-01920-3
155. Boyette LB, Creasey OA, Guzik L, Lozito T, Tuan RS. Human bone marrow-derived mesenchymal stem cells display enhanced clonogenicity but impaired differentiation with hypoxic preconditioning. Stem Cells Transl Med (2014) 3:241–54. doi: 10.5966/sctm.2013-0079
156. Wang X, Liu C, Li S, Xu Y, Chen P, Liu Y, et al. Hypoxia precondition promotes adipose-derived mesenchymal stem cells based repair of diabetic erectile dysfunction via augmenting angiogenesis and neuroprotection. PloS One (2015) 10:e0118951. doi: 10.1371/journal.pone.0118951
157. Wang W, Huang X, Lin W, Qiu Y, He Y, Yu J, et al. Hypoxic preconditioned bone mesenchymal stem cells ameliorate spinal cord injury in rats via improved survival and migration. Int J Mol Med (2018) 42:2538–50. doi: 10.3892/ijmm.2018.3810
158. Philipp D, Suhr L, Wahlers T, Choi YH, Paunel-Gorgulu A. Preconditioning of bone marrow-derived mesenchymal stem cells highly strengthens their potential to promote IL-6-dependent M2b polarization. Stem Cell Res Ther (2018) 9:286. doi: 10.1186/s13287-018-1039-2
159. Gray A, Schloss RS, Yarmush M. Donor variability among anti-inflammatory pre-activated mesenchymal stromal cells. Technol (Singap World Sci) (2016) 4:201–15. doi: 10.1142/S2339547816500084
160. Prasanna SJ, Gopalakrishnan D, Shankar SR, Vasandan AB. Pro-inflammatory cytokines, IFNgamma and TNFalpha, influence immune properties of human bone marrow and Wharton jelly mesenchymal stem cells differentially. PloS One (2010) 5:e9016. doi: 10.1371/journal.pone.0009016
161. Huang F, Gao T, Wang W, Wang L, Xie Y, Tai C, et al. Engineered basic fibroblast growth factor-overexpressing human umbilical cord-derived mesenchymal stem cells improve the proliferation and neuronal differentiation of endogenous neural stem cells and functional recovery of spinal cord injury by activating the PI3K-Akt-GSK-3beta signaling pathway. Stem Cell Res Ther (2021) 12:468. doi: 10.1186/s13287-021-02537-w
162. Gao T, Huang F, Wang W, Xie Y, Wang B. Interleukin-10 genetically modified clinical-grade mesenchymal stromal cells markedly reinforced functional recovery after spinal cord injury via directing alternative activation of macrophages. Cell Mol Biol Lett (2022) 27:27. doi: 10.1186/s11658-022-00325-9
163. Haraszti RA, Miller R, Stoppato M, Sere YY, Coles A, Didiot MC, et al. Exosomes produced from 3D cultures of MSCs by tangential flow filtration show higher yield and improved activity. Mol Ther (2018) 26:2838–47. doi: 10.1016/j.ymthe.2018.09.015
164. Han S, Wang B, Li X, Xiao Z, Han J, Zhao Y, et al. Bone marrow-derived mesenchymal stem cells in three-dimensional culture promote neuronal regeneration by neurotrophic protection and immunomodulation. J BioMed Mater Res A (2016) 104:1759–69. doi: 10.1002/jbm.a.35708
165. Sun G, Li G, Li D, Huang W, Zhang R, Zhang H, et al. hucMSC derived exosomes promote functional recovery in spinal cord injury mice via attenuating inflammation. Mater Sci Eng C Mater Biol Appl (2018) 89:194–204. doi: 10.1016/j.msec.2018.04.006
166. Liu W, Wang Y, Gong F, Rong Y, Luo Y, Tang P, et al. Exosomes derived from bone mesenchymal stem cells repair traumatic spinal cord injury by suppressing the activation of A1 neurotoxic reactive astrocytes. J Neurotrauma (2019) 36:469–84. doi: 10.1089/neu.2018.5835
167. Ren Z, Qi Y, Sun S, Tao Y, Shi R. Mesenchymal stem cell-derived exosomes: Hope for spinal cord injury repair. Stem Cells Dev (2020) 29:1467–78. doi: 10.1089/scd.2020.0133
168. Zhou W, Silva M, Feng C, Zhao S, Liu L, Li S, et al. Exosomes derived from human placental mesenchymal stem cells enhanced the recovery of spinal cord injury by activating endogenous neurogenesis. Stem Cell Res Ther (2021) 12:174. doi: 10.1186/s13287-021-02248-2
169. Huang JH, Yin XM, Xu Y, Xu CC, Lin X, Ye FB, et al. Systemic administration of exosomes released from mesenchymal stromal cells attenuates apoptosis, inflammation, and promotes angiogenesis after spinal cord injury in rats. J Neurotrauma (2017) 34:3388–96. doi: 10.1089/neu.2017.5063
170. Li R, Zhao K, Ruan Q, Meng C, Yin F. Bone marrow mesenchymal stem cell-derived exosomal microRNA-124-3p attenuates neurological damage in spinal cord ischemia-reperfusion injury by downregulating Ern1 and promoting M2 macrophage polarization. Arthritis Res Ther (2020) 22:75. doi: 10.1186/s13075-020-2146-x
171. Lo Sicco C, Reverberi D, Balbi C, Ulivi V, Principi E, Pascucci L, et al. Mesenchymal stem cell-derived extracellular vesicles as mediators of anti-inflammatory effects: Endorsement of macrophage polarization. Stem Cells Transl Med (2017) 6:1018–28. doi: 10.1002/sctm.16-0363
172. Lee JR, Kyung JW, Kumar H, Kwon SP, Song SY, Han IB, et al. Targeted delivery of mesenchymal stem cell-derived nanovesicles for spinal cord injury treatment. Int J Mol Sci (2020) 21:4185. doi: 10.3390/ijms21114185
173. Zolboot N, Du JX, Zampa F, Lippi G. MicroRNAs instruct and maintain cell type diversity in the nervous system. Front Mol Neurosci (2021) 14:646072. doi: 10.3389/fnmol.2021.646072
174. Li F, Zhou MW. MicroRNAs in contusion spinal cord injury: pathophysiology and clinical utility. Acta Neurol Belg (2019) 119:21–7. doi: 10.1007/s13760-019-01076-9
175. Sun F, Zhang H, Huang T, Shi J, Wei T, Wang Y. miRNA-221 regulates spinal cord injury-induced inflammatory response through targeting TNF-alpha expression. BioMed Res Int (2021) 2021:6687963. doi: 10.1155/2021/6687963
176. Gaudet AD, Mandrekar-Colucci S, Hall JC, Sweet DR, Schmitt PJ, Xu X, et al. miR-155 deletion in mice overcomes neuron-intrinsic and neuron-extrinsic barriers to spinal cord repair. J Neurosci (2016) 36:8516–32. doi: 10.1523/JNEUROSCI.0735-16.2016
177. Gaudet AD, Fonken LK, Ayala MT, Maier SF, Watkins LR. Aging and miR-155 in mice influence survival and neuropathic pain after spinal cord injury. Brain Behav Immun (2021) 97:365–70. doi: 10.1016/j.bbi.2021.07.003
178. Fei M, Li Z, Cao Y, Jiang C, Lin H, Chen Z. MicroRNA-182 improves spinal cord injury in mice by modulating apoptosis and the inflammatory response via IKKbeta/NF-kappaB. Lab Invest (2021) 101:1238–53. doi: 10.1038/s41374-021-00606-5
179. Li H, Jiang T, Li MQ, Zheng XL, Zhao GJ. Transcriptional regulation of macrophages polarization by MicroRNAs. Front Immunol (2018) 9:1175. doi: 10.3389/fimmu.2018.01175
180. Nazari-Jahantigh M, Wei Y, Noels H, Akhtar S, Zhou Z, Koenen RR, et al. MicroRNA-155 promotes atherosclerosis by repressing Bcl6 in macrophages. J Clin Invest (2012) 122:4190–202. doi: 10.1172/JCI61716
181. Essandoh K, Li Y, Huo J, Fan GC. MiRNA-mediated macrophage polarization and its potential role in the regulation of inflammatory response. Shock (2016) 46:122–31. doi: 10.1097/SHK.0000000000000604
182. Peng L, Zhang H, Hao Y, Xu F, Yang J, Zhang R, et al. Reprogramming macrophage orientation by microRNA 146b targeting transcription factor IRF5. EBioMedicine (2016) 14:83–96. doi: 10.1016/j.ebiom.2016.10.041
183. Zhang Y, Zhang M, Li X, Tang Z, Wang X, Zhong M, et al. Silencing MicroRNA-155 attenuates cardiac injury and dysfunction in viral myocarditis via promotion of M2 phenotype polarization of macrophages. Sci Rep (2016) 6:22613. doi: 10.1038/srep22613
184. Chang Q, Hao Y, Wang Y, Zhou Y, Zhuo H, Zhao G. Bone marrow mesenchymal stem cell-derived exosomal microRNA-125a promotes M2 macrophage polarization in spinal cord injury by downregulating IRF5. Brain Res Bull (2021) 170:199–210. doi: 10.1016/j.brainresbull.2021.02.015
185. Fang H, Yang M, Pan Q, Jin HL, Li HF, Wang RR, et al. MicroRNA-22-3p alleviates spinal cord ischemia/reperfusion injury by modulating M2 macrophage polarization via IRF5. J Neurochem (2021) 156:106–20. doi: 10.1111/jnc.15042
186. Yao F, Yu Y, Feng L, Li J, Zhang M, Lan X, et al. Adipogenic miR-27a in adipose tissue upregulates macrophage activation via inhibiting PPARgamma of insulin resistance induced by high-fat diet-associated obesity. Exp Cell Res (2017) 355:105–12. doi: 10.1016/j.yexcr.2017.03.060
187. Lin L, Lin H, Wang L, Wang B, Hao X, Shi Y. miR-130a regulates macrophage polarization and is associated with non-small cell lung cancer. Oncol Rep (2015) 34:3088–96. doi: 10.3892/or.2015.4301
188. Zhang M, Zhou Z, Wang J, Li S. MiR-130b promotes obesity associated adipose tissue inflammation and insulin resistance in diabetes mice through alleviating M2 macrophage polarization via repression of PPAR-gamma. Immunol Lett (2016) 180:1–8. doi: 10.1016/j.imlet.2016.10.004
189. Sun Y, Li Q, Gui H, Xu DP, Yang YL, Su DF, et al. MicroRNA-124 mediates the cholinergic anti-inflammatory action through inhibiting the production of pro-inflammatory cytokines. Cell Res (2013) 23:1270–83. doi: 10.1038/cr.2013.116
190. Yu A, Zhang T, Duan H, Pan Y, Zhang X, Yang G, et al. MiR-124 contributes to M2 polarization of microglia and confers brain inflammatory protection via the C/EBP-alpha pathway in intracerebral hemorrhage. Immunol Lett (2017) 182:1–11. doi: 10.1016/j.imlet.2016.12.003
191. Jennewein C, von Knethen A, Schmid T, Brune B. MicroRNA-27b contributes to lipopolysaccharide-mediated peroxisome proliferator-activated receptor gamma (PPARgamma) mRNA destabilization. J Biol Chem (2010) 285:11846–53. doi: 10.1074/jbc.M109.066399
192. Arranz A, Doxaki C, Vergadi E, Martinez de la Torre Y, Vaporidi K, Lagoudaki ED, et al. Akt1 and Akt2 protein kinases differentially contribute to macrophage polarization. Proc Natl Acad Sci U.S.A. (2012) 109:9517–22. doi: 10.1073/pnas.1119038109
193. Wang Z, Brandt S, Medeiros A, Wang S, Wu H, Dent A, et al. MicroRNA 21 is a homeostatic regulator of macrophage polarization and prevents prostaglandin E2-mediated M2 generation. PloS One (2015) 10:e0115855. doi: 10.1371/journal.pone.0115855
194. Chaudhuri AA, So AY, Sinha N, Gibson WS, Taganov KD, O'Connell RM, et al. MicroRNA-125b potentiates macrophage activation. J Immunol (2011) 187:5062–8. doi: 10.4049/jimmunol.1102001
195. Sahu SK, Kumar M, Chakraborty S, Banerjee SK, Kumar R, Gupta P, et al. MicroRNA 26a (miR-26a)/KLF4 and CREB-C/EBPbeta regulate innate immune signaling, the polarization of macrophages and the trafficking of mycobacterium tuberculosis to lysosomes during infection. PloS Pathog (2017) 13:e1006410. doi: 10.1371/journal.ppat.1006410
196. Li J, Liang Y, Lv H, Meng H, Xiong G, Guan X, et al. miR-26a and miR-26b inhibit esophageal squamous cancer cell proliferation through suppression of c-MYC pathway. Gene (2017) 625:1–9. doi: 10.1016/j.gene.2017.05.001
197. Chen L, Dai YM, Ji CB, Yang L, Shi CM, Xu GF, et al. MiR-146b is a regulator of human visceral preadipocyte proliferation and differentiation and its expression is altered in human obesity. Mol Cell Endocrinol (2014) 393:65–74. doi: 10.1016/j.mce.2014.05.022
198. Chen Q, Wang H, Liu Y, Song Y, Lai L, Han Q, et al. Inducible microRNA-223 down-regulation promotes TLR-triggered IL-6 and IL-1beta production in macrophages by targeting STAT3. PloS One (2012) 7:e42971. doi: 10.1371/journal.pone.0042971
199. Ganta VC, Choi MH, Kutateladze A, Fox TE, Farber CR, Annex BH. A MicroRNA93-interferon regulatory factor-9-Immunoresponsive gene-1-Itaconic acid pathway modulates M2-like macrophage polarization to revascularize ischemic muscle. Circulation (2017) 135:2403–25. doi: 10.1161/CIRCULATIONAHA.116.025490
200. Banerjee S, Xie N, Cui H, Tan Z, Yang S, Icyuz M, et al. MicroRNA let-7c regulates macrophage polarization. J Immunol (2013) 190:6542–9. doi: 10.4049/jimmunol.1202496
201. Khan MJ, Singh P, Dohare R, Jha R, Rahmani AH, Almatroodi SA, et al. Inhibition of miRNA-34a promotes M2 macrophage polarization and improves LPS-induced lung injury by targeting Klf4. Genes (Basel) (2020) 11:966. doi: 10.3390/genes11090966
202. Jiang P, Liu R, Zheng Y, Liu X, Chang L, Xiong S, et al. MiR-34a inhibits lipopolysaccharide-induced inflammatory response through targeting Notch1 in murine macrophages. Exp Cell Res (2012) 318:1175–84. doi: 10.1016/j.yexcr.2012.03.018
203. Chen XY, Yang Y, Ran LP, Dong ZD, Zhang EJ, Yu XR, et al. Novel insights into miRNA regulation of storage protein biosynthesis during wheat caryopsis development under drought stress. Front Plant Sci (2017) 8:1707. doi: 10.3389/fpls.2017.01707
204. Goettsch C, Hutcheson JD, Aikawa E. MicroRNA in cardiovascular calcification: focus on targets and extracellular vesicle delivery mechanisms. Circ Res (2013) 112:1073–84. doi: 10.1161/CIRCRESAHA.113.300937
205. Chen YJ, Zhu H, Zhang N, Shen L, Wang R, Zhou JS, et al. Temporal kinetics of macrophage polarization in the injured rat spinal cord. J Neurosci Res (2015) 93:1526–33. doi: 10.1002/jnr.23612
206. Ma SF, Chen YJ, Zhang JX, Shen L, Wang R, Zhou JS, et al. Adoptive transfer of M2 macrophages promotes locomotor recovery in adult rats after spinal cord injury. Brain Behav Immun (2015) 45:157–70. doi: 10.1016/j.bbi.2014.11.007
Keywords: macrophages, spinal cord injury, polarization, inflammatory response, miRNA, mesenchymal stem cells, microglia
Citation: Fu S-P, Chen S-Y, Pang Q-M, Zhang M, Wu X-C, Wan X, Wan W-H, Ao J and Zhang T (2022) Advances in the research of the role of macrophage/microglia polarization-mediated inflammatory response in spinal cord injury. Front. Immunol. 13:1014013. doi: 10.3389/fimmu.2022.1014013
Received: 09 August 2022; Accepted: 18 November 2022;
Published: 01 December 2022.
Edited by:
Juan Pablo de Rivero Vaccari, University of Miami, United StatesCopyright © 2022 Fu, Chen, Pang, Zhang, Wu, Wan, Wan, Ao and Zhang. This is an open-access article distributed under the terms of the Creative Commons Attribution License (CC BY). The use, distribution or reproduction in other forums is permitted, provided the original author(s) and the copyright owner(s) are credited and that the original publication in this journal is cited, in accordance with accepted academic practice. No use, distribution or reproduction is permitted which does not comply with these terms.
*Correspondence: Tao Zhang, b2NlYW56dEAxNjMuY29t