- 1Department of Pediatric Pneumonology and Allergy, Medical University of Warsaw, Warsaw, Poland
- 2Doctoral School, Medical University of Warsaw, Warsaw, Poland
- 3Department of Laboratory Diagnostics and Clinical Immunology of Developmental Age, Medical University of Warsaw, Warsaw, Poland
- 4Chair and Department of Biochemistry, Medical University of Warsaw, Warsaw, Poland
Extracellular vesicles (EVs) have emerged as vital mediators in intracellular communication in the lung microenvironment. Environmental exposure to various triggers (e.g., viruses, allergens) stimulates the EV-mediated cascade of pro-inflammatory responses that play a key role in the asthma pathomechanism. This complex EV-mediated crosstalk in the asthmatic lung microenvironment occurs between different cell types, including airway epithelial cells and immune cells. The cargo composition of EVs mirrors hereby the type and activation status of the parent cell. Therefore, EVs collected in a noninvasive way (e.g., in nasal lavage, serum) could inform on the disease status as a “liquid biopsy”, which is particularly important in the pediatric population. As a heterogeneous disease, asthma with its distinct endotypes and phenotypes requires more investigation to develop novel diagnostics and personalized case management. Filling these knowledge gaps may be facilitated by further EV research. Here, we summarize the contribution of EVs in the lung microenvironment as potential novel players towards precision medicine in the development of asthma. Although rapidly evolving, the EV field is still in its infancy. However, it is expected that a better understanding of the role of EVs in the asthma pathomechanism will open up new horizons for precision medicine diagnostic and therapeutic solutions.
1 Introduction
Extracellular vesicles (EVs) are heterogenous membranous nanoparticles secreted from every cell type in our body, varying in size, morphology, and content (1). EVs carry a multitude of intracellular bioactive molecules between cells, including nucleic acids (e.g., DNA, messenger RNA (mRNA), and non-coding RNA), lipids, and proteins (2). Consequently, EVs play a vital role in intercellular communication (2, 3). It is well-established that, for instance, the composition of EV-associated RNA mirrors the type and activation status of their parent cell (4). Therefore, collecting EVs could inform on the disease status of the cells noninvasively, without removing the cells from the tissues, serving as a “liquid biopsy” (5).
EVs can be found in all human body fluids, taking part in regulation of various systemic processes such as immune function and local modulation of organ-specific reactions (6). Over the past decade, there has been a boost in EVs exploration across the scientific community, including the role of EVs in the complex lung microenvironment (7, 8).
Currently, no single optimal EVs’ isolation method is recommended, since it depends on the desired balance between recovery and specificity and EV end use (e.g., basic vs. clinical research), however the most popular are ultracentrifugation and size-exclusion chromatography. According to MISEV2018, EV separation/isolation procedures should be reported in detail and multi-step characterization is needed to attribute reported function or a biomarker to EVs (1).
Rapidly accumulating evidence from in vitro, in vivo, and human studies has demonstrated that EVs have a potential role in asthma diagnostic options (9, 10). Asthma is a major chronic inflammatory lung disease affecting the quality of life of people of all ages worldwide, characterized by reversible airway obstruction causing dyspnea and cough (11, 12). Due to the heterogeneity of the disease (with various endotypes/phenotypes), there are still gaps to be filled in terms of improving patient education, implementing new diagnostics, and personalized case management (13, 14). In the asthma inflammatory microenvironment, both resident cells (e.g., epithelial, endothelial cells, fibroblasts) and inflammatory cells (e.g., eosinophils, mast cells, T cells) interact and exchange soluble mediators (15). Thus, the emerging EV field opens up new horizons to understanding asthma pathomechanism and offers new targets for precision medicine therapies.
Here, the recent updates on the role of EVs in the asthma pathomechanism in a cell type-specific manner will be discussed.
2 Types of extracellular vesicles in asthma
2.1 Epithelial cell-derived extracellular vesicles
Airway epithelial cells (AECs) play an important role in asthma development, both as a barrier and as modulators of the immune response, including innate mucosal defense (16, 17). AECs released EVs apically and basally, with side-specific functions of the miRNA cargo (Figure 1) (18, 19). Epithelial remodeling processes such as mucin hypersecretion can be significantly altered by the changed miRNA profile of AEC-EVs (16). In a recent in vitro study, stimulation with T2 cytokines of human AECs upregulated the release of EV proteins involved in chronic airway inflammation and decreased the expression of the antimicrobial peptide S100A7, suggesting that EVs mediate endotype-specific mechanisms related to asthma (20–22). Under T17 immune response conditions, EV-associated proteins increased neutrophil recruitment and promote neutrophilic airway inflammation (20).
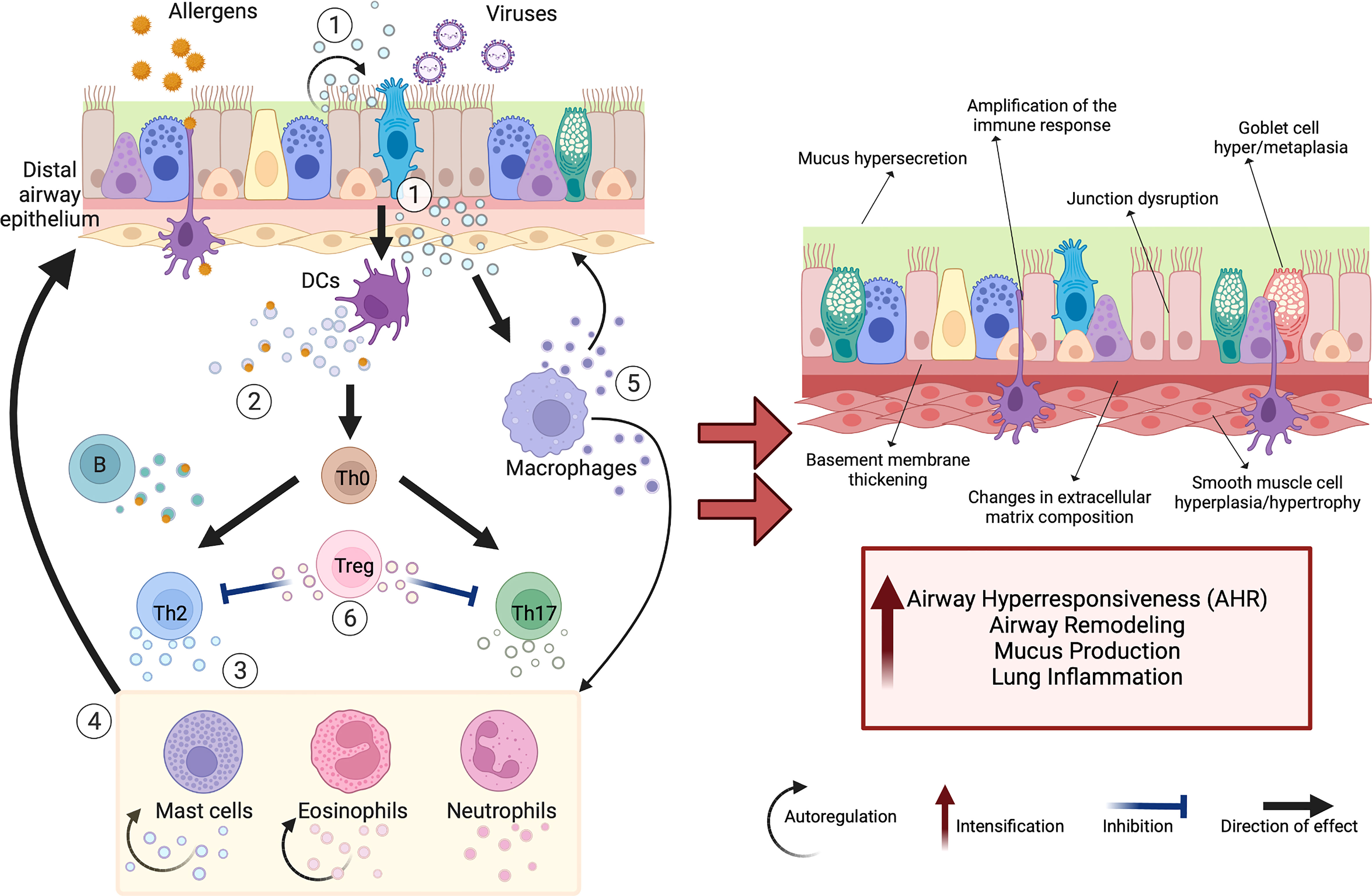
Figure 1 Schematic view of extracellular vesicles (EVs) as mediators of cellular interactions in the lung microenvironment. The complex EV-mediated crosstalk in the lung microenvironment occurs between many different cell types, including airway epithelial cells (AECs) and immune cells. Secreted EVs transfer specific cargo (e.g., proteins, miRNAs, mitochondria), which modulates the activity of target cells and can support tissue homeostasis or promote chronic respiratory changes. The exposure of airway epithelium to various environmental triggers (e.g., viruses, allergens) stimulates increased release of EVs with changed cargo composition that plays a critical role in asthma pathomechanism (including airway remodeling, airway hyperresponsiveness (AHR), mucus hypersecretion, increased lung inflammation). These EVs act in several ways (1): AEC-derived EVs target dendritic cells (DCs), macrophages, and themselves, promoting Th2- and Th17-polarized immune activation (2). DC-derived and B-cell-derived EVs can induce T-cell responses and serve as “antigen-presenting units” (3). T-cell-derived EVs cause Th2 skewing, eosinophil, neutrophil, and mast cell activation (4); that cells in turn produce EVs driving airway remodeling, and supply nitric oxide and reactive oxygen species, increasing the migration of other eosinophils and mast cells to the inflammation site (5). Macrophage-derived EVs also participate in airway remodeling, synthesizing leukotrienes and recruiting granulocytes to the inflammation site (6). In contrast, regulatory T cell (Treg)-derived EVs initiate anti-inflammatory activities. Created with BioRender.com.
Despite the current knowledge on various AECs phenotypes, we are still unable to easily detect or monitor airway epithelial cell viability/dysfunction in biosamples possessed in minimally invasive procedures, e.g., in nasal lavages, or exhaled breath condensate (EBC) (23, 24).
Some newly detected EV-related proteins were linked to asthma pathology: ezrin, contantin-1 (CNTN1) and Plexin B2 (PLXNB2). Firstly, ezrin as part of cytoskeletal elements of AEC, derives directly to EVs from AECs. EV-associated ezrin released by AECs contributed to IL-13-induced epithelial damage via the TNF-α-dependent pathway and was proposed as a biomarker of asthma control (25, 26). Secondly, CNTN1 promoted a Th2- and Th17-polarized immune activation through the Notch-2 pathway along with smooth muscle hyperresponsiveness (AHR) and mucus production in cell and mouse model (27). Thirdly, PLXNB2, a natural CD100 ligand, was released in AECs-EVs and augmented neutrophilic and monocytic airway inflammation in mice by activating macrophages via cleavage of CD100 by MMP14 (28). Furthermore, a lower expression of miR-34a, miR-92b, and miR-210 was found in EVs in nasal lavages from asthmatic children, and was associated with an obstruction of large (FEV1FVC%pred) and small airways (FEF25-75%pred) (18).
2.2 Immune cells-derived extracellular vesicles
2.2.1 EVs from mast cells
Mast cells (MCs) have been recognized as active participants in innate as well as specific immune responses (29). Their EVs were shown to play a role in positive immune regulation, including recruiting B and T cells to the lungs and facilitating the priming of naïve T cells in an in vitro and a mouse model (30–33). EVs from bone marrow-derived MCs, carrying high-affinity IgE receptors (FcϵRI), can bind to free IgE via FcϵRI, induce an anti-IgE effect, thus decreasing IgE levels and inhibiting the allergic cascade (34). In a mouse model of allergic asthma, these EVs modulated not only airway inflammation and AHR, but also partially the remodeling in chronic asthma (34). On the other hand, CC chemokine receptor (CCR1)-rich MCs-EVs could transfer CCR1 to other MCs in vivo, enhancing the co-activation of high-affinity IgE receptors (FcϵRI) with CCR1 (35, 36).
Furthermore, it was proven that MCs-EVs take part in the modulation of oxidative stress. In asthmatic mice, miR-21 released in MCs-EVs promotes oxidative stress and inflammatory responses via the DDAH1/Wnt/β-catenin signaling axis (37). Moreover, mouse MCs-EVs exposed to oxidative stress had a different mRNA profile, transferring resistance to further oxidative damage to recipient cells; however, this mechanism is far from being elucidated (38).
2.2.2 EVs from dendritic cells
The lung-resident dendritic cells (DCs) participate in asthma development, as they have a pivotal role in establishing an allergen-specific Th2 response in the airways after stimulation with epithelial alarmins (39, 40). It has been shown that activated DCs released EVs containing various protein ligand-like OX40L that induced the proliferation of CD4+ T cells, elevated the level of IL-4, and drove Th2 differentiation in vitro (41). Furthermore, DC-derived EVs provided enzymes for the biosynthesis of leukotrienes (LTs), key pro-inflammatory mediators important in the pathogenesis of asthma, to smooth muscle cells. Besides, these EVs contained chemotactic eicosanoids and promoted granulocyte migration in vitro (42).
Majority of the studies on DCs-EVs were conducted using either bone marrow-derived or monocyte-derived DCs. Recently, it has been recognized that pulmonary DCs present different DCs subsets, including conventional types 1 and 2 DCs and plasmacytoid DCs, each playing a varying role in asthma pathogenesis (43). This evidence emphasizes the need for researchers to further specify the origin and effect of DCs-EVs in a subtype manner.
2.2.3 EVs from macrophages
The growing appreciation of macrophage plasticity and polarization in asthma pathogenesis reflects the pro-inflammatory properties of M1 polarized macrophages and the anti-inflammatory properties of M2 polarized macrophages (44–46). M2-like alveolar macrophages were reported to secrete suppressor of cytokine signaling (SOCS)-1 and SOCS-3 proteins within EVs (47, 48). Epithelial cells exposed to these EVs presented alleviated cytokine signaling via the JAK-STAT pathway activation. Thus, impaired delivery of SOCS proteins through EVs could serve as a significant mechanism in the dysregulated cytokine responses in asthma.
Under the stress condition, rat alveolar macrophages produced EVs carrying high levels of several microRNAs, including miR-21-5p involved in the oxidative stress. The EVs transported miR-21-5p to tracheal epithelial cells and promoted airway remodeling through the TGF-β1/Smad signaling pathway by targeting Smad7 (49). Similarly to DCs, EVs released from macrophages took part in the biosynthesis of LTs and granulocyte migration in vitro (42).
2.2.4 EVs from eosinophils
For decades, eosinophilia has been recognized as one of the prominent features of allergic asthma, and eosinophils are linked with the so-called T2-high asthma endotype (50). Eosinophils from asthmatic patients produced higher EV levels than those from healthy subjects, and these EVs contained molecules relevant to human asthma, such as EPO (eosinophil peroxidase), MBP (major basic protein), and eosinophil cationic protein (EPC) (51). Furthermore, these EVs induced epithelial cell apoptosis and smooth muscle cell proliferation, which are fundamental aspects of asthma pathogenesis (52). Moreover, they demonstrated properties to autoregulated and promoted eosinophil function in asthmatic inflammation by producing nitric oxide and reactive oxygen species (53). Moreover, eosinophil-derived EVs acted in vivo as a chemotactic factor for eosinophils due to the expression of adhesion molecules, such as intercellular adhesion molecule (ICAM)-1 and integrin α2 (53). Altogether, eosinophilic EVs, acting in a feedback loop for their short-term lived parent cells, may prolong the inflammatory cellular infiltration and muscle and epithelial remodeling.
2.2.5 EVs from neutrophils
Neutrophilic airway infiltration is observed in non-allergic asthma, often in severe cases with poor response to corticosteroid treatment (54, 55). Proteomic composition of neutrophil-derived EVs, released spontaneously and upon lipopolysaccharides (LPS)-stimulation, significantly varied (56). The EVs from LPS-stimulated equine neutrophils contained higher levels of thrombospondin-1 and S100A9, and lower levels of neutrophil gelatinase–associated lipocalin and serpin peptidase inhibitor. This analysis provided evidence of neutrophils-derived EVs’ contribution to tissue inflammation, apoptosis modulation, and proliferation of smooth muscle cells (56). Therefore, it supported the involvement of these EVs in the progression of asthma and the promotion of airway remodeling in severe and corticosteroid-insensitive patients with asthma. In another study, EV transfer of activated neutrophil-derived long non-coding RNA CRNDE was suggested to promote proliferation and migration of airway smooth muscle cells in asthma (57). Thereby, in vivo silencing of CRNDE reduced the thickness of bronchial smooth muscle in asthmatic mice.
2.2.6 EVs from T- and B-cells
T cells are of great importance in the adaptive immune responses during the asthma pathomechanism by participating in IgE antibody class switching, Th2 skewing, eosinophil and mast cell activation. A recent study, using a proteomic approach, demonstrated that EVs from activated human T cells had enhanced expression of the RAS/MAPK signaling pathway proteins, which induced ERK kinase phosphorylation in recipient immune cells in vitro (58). By contrast, regulatory T cells (Tregs) are a subpopulation of T cells that aim to maintain immunological tolerance, prevent autoimmunity, and limit other immune responses (59). They achieve this through various mechanisms, including Treg-derived EVs. Upon LPS stimulation, Tregs-derived EVs were found to transfer miR-150-5p and miR-142-3p to DCs, modulating DCs cytokine secretion towards an anti-inflammatory profile of increased IL-10 and decreased IL-6 (59). In another in vitro study, following activation of Tregs, CD73-expressing Treg-derived EVs demonstrated their suppressive activity through the production of adenosine (60).
Th2-mediated inflammation is also promoted by B cell EVs that carry allergen peptides on MHC molecules. This antigen-presenting property of B cell-derived EVs was shown in vitro. Birch-allergen (Bet v 1)-loaded-B-cell EVs induced T cell proliferation and secretion of IL-5 and IL-13 cytokines, key signals in driving airway inflammation and remodeling in asthma (61).
2.3 Mesenchymal-stem-cell-derived extracellular vesicles
Mesenchymal stem cells (MSCs) refer to a group of cells from bone marrow, adipose tissue and umbilical cord, that has the capacity for adherent growth (62, 63). MSCs are widely used in cell-based therapy due to their remarkable ability for proliferation, differentiation, and immune regulation. However, translating MSCs into clinic remains more difficult than expected (64). Currently, there is accumulating evidence on a promising role of MSCs-derived EVs - rather than MSCs itself, in asthma therapy. The summary of available data associating various MSCs-derived EVs with possible therapeutic options for asthma is presented in Table 1 and is discussed in more detail in chapter 3.3.
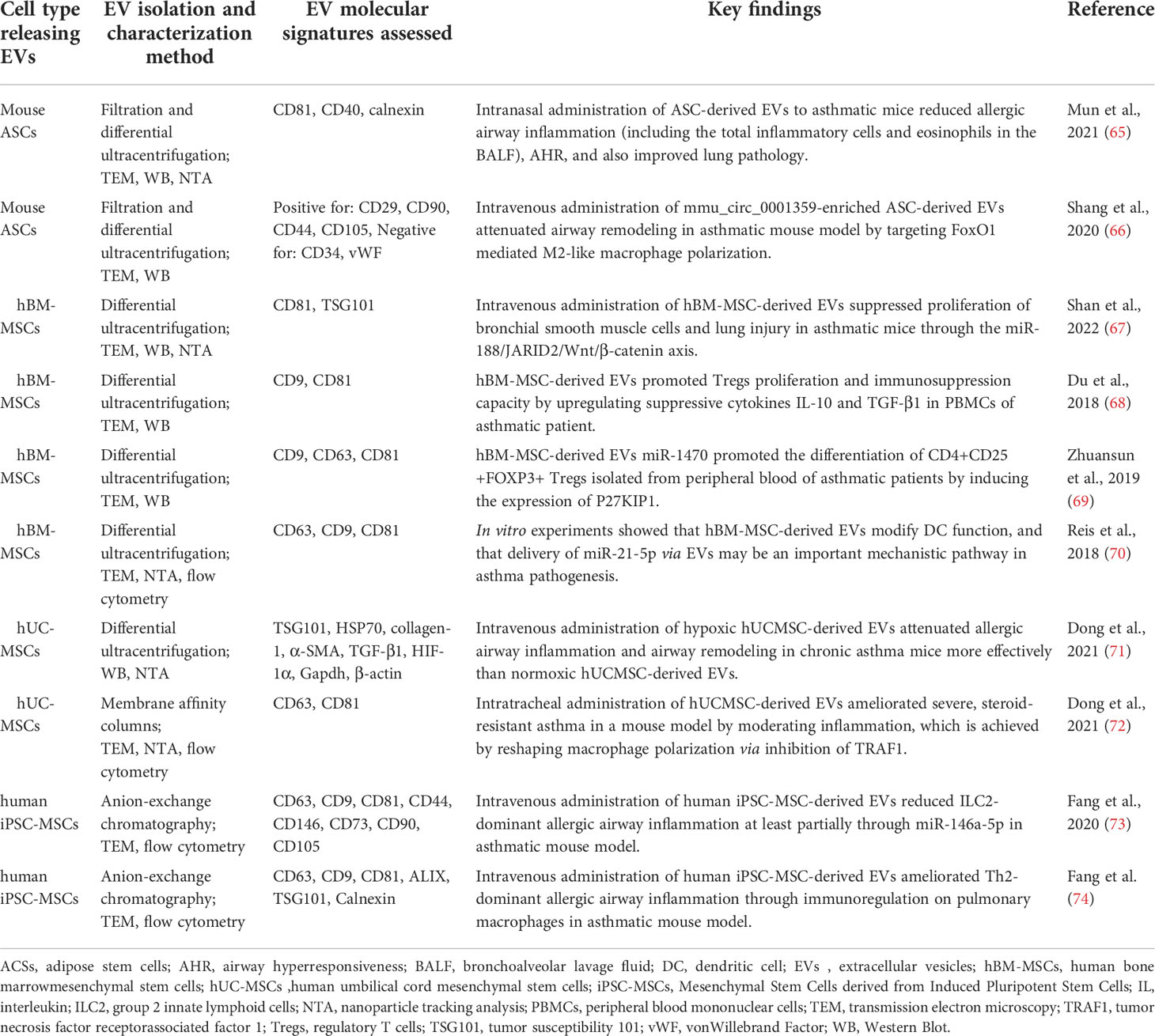
Table 1 Mesenchymal-Stem-Cell-Derived extracellular vesicles (MSC-EVs) and their reported role in attenuating asthma pathomechanisms.
The above discussed role of EVs in asthma pathobiology, derived from major cell types within the lung microenvironment, is summarized in Figure 1.
3 Discussion and future research directions
Since the discovery of EVs decades ago, tremendous progress has been made in the deciphering how they are involved in intracellular communication, impacting various physiological and pathological events. However, this novel field is still in its infancy. To date, in asthma research, the EVs have been successfully isolated and characterized from several human biofluids, obtained via non-invasive methods, including saliva (75), nasal lavage fluid (18), EBC and sputum (76). Despite our growing knowledge on asthma heterogeneity, there is an unmet need for development of molecular markers guiding further the precision medicine approach, both in asthma diagnostic and therapeutic management. Notably, specially pediatricians worldwide face a great number of obstacles in the management of their asthmatic patients (77). It includes a precarious invasive collection of biological samples, lack of precise diagnostic tests, as children are often unable to perform lung function tests, and the common failure to recognize the variability of the course of asthma. This frequently leads to young asthma patients being underdiagnosed, undertreated, and inadequately controlled (78).
3.1 Role of EVs in asthma pathology
Viral respiratory infections, particularly with the rhinovirus (RV) and the respiratory syncytial virus (RSV), are major causes of asthma development and exacerbations (79, 80). EVs offer new insight into the viral-induced chronic sequelae in the lung microenvironment. RSV-infected APCs were found to initiate EVs production that contain agents promoting inflammatory cytokine production in vitro in an alveolar epithelial cell line culture through IP-10, CCL2, and CXCL10 release (81). Interestingly, RSV infection of AECs was associated with significant changes in EVs RNA content (e.g., upregulated EVs-miRNAs: hsa-mir-6087, hsa-let-7e, hsa-miR-182-5p, hsa-miR-181b-5p; downregulated EVs-miRNAs: hsa-mir-223, hsa-mir-2964a, hsa-mir-205, hsa-mir-143) (81). Furthermore, EVs derived from virus- infected cells contain RSV components but do not transmit RSV infection (81). Moreover, these EVs induce pro-inflammatory mediator secretion in uninfected bystander cells, thereby impacting the additional way to modulate the immune responses during infection (81). Another comparative analysis of the airway secretory microRNAome in children under the age of three indicated that RV infection is associated with airway secretion of EVs enclosing miR-155, which in silico was predicted to regulate antiviral host immunity (82). Additionally, two distinct components of the inflammatory pathway regulating the immune response were revealed following RV infection and TLR3 (not TLR7) stimulation in asthmatic AECs. The first of these components highlighted a Tenascin-C protein release, including its upregulated expression in nasal lavage fluid (83). The second noted a secretion of EVs with a pro-inflammatory effect on AECs. By contrast, umbilical cord MSCs-derived EVs were shown to possess antiviral activities against other common human respiratory viruses (84).
Recent data revealed also the role of airway and even gut microbiota in asthma pathogenesis, and microbiota-derived EVs are emerging as the linking factor between microbiota and allergic reactions and asthma and are discussed in more detail elsewhere (85, 86).
3.2 EV-based asthma biomarkers
Recent evolution of high-throughput sequencing technology with advanced analytical methods enables precisely tracking changes in EVs’ cargo composition and delineating complex interactions within molecular networks of asthma endotypes. For instance, circulating and/or EVs- related non-coding RNAs have been introduced as the novel, valuable biomarkers for different pathological conditions, including asthma. In particular, a promising makrer – the long non-coding RNA (lncRNA) impacts a wide range of biological processes (e.g., transcriptional activation, transcriptional interference by competitively inhibiting the effect of miRNA on downstream mRNA) (87). At this moment, the very first steps were made to explore the lncRNA-miRNA-mRNA regulatory network in T2-high asthma (57, 88). For example, PCAT19 was suggested as a lncRNA that may serve as a promising immune-related biomarker to distinguish between T2-high and T2-low asthma; however, it was not studied as a part of the EVs content (88). A novel study associated the levels of four serum EV-miRNAs (miR-21-5p, miR-126-3p, miR146a-5p, miR-215-5p) with the severity of asthmatic in adults (89). miR-21-5p and miR-126-3p, involved in Th1/Th2 differentiation, were specifically augmented in T2-high asthma. By contrast, IL-6-high patients with MSA, which were older, more obese, with higher neutrophil and basophil counts and TNF levels, manifested a decrease of miR-21-5p, miR-126-3p and miR-146a-5p. Interestingly, the researchers observed a trend towards a decreased expression of all studied miRNAs in mild asthmatics compared to healthy controls, probably due to the effect of inhaled corticosteroids (89). More research is clearly needed to clarify the role of EV-miRNAs in asthma endotyping/phenotyping and for undergoing treatment, and the current data are too limited to speculate about their possible use in clinical practice.
3.3 EV-based therapeutical strategies for asthma
Compared with other commonly used drug delivery carriers, such as liposomes, EVs have the advantages of high internal targeting ability, low immunogenicity, high modification flexibility and high biological barrier permeability, which open up an exciting avenue for modern drug delivery (63, 90). For example, experimentally engineered nanoparticles – extracellular vesicle’s membrane from M2 macrophages combined with a lncRNA named methyltransferase 3A opposite strand (Dnmt3aos) smart silencer wrapped in a polylactic acid-glycolic acid (PLGA) copolymer – have been demonstrated to target M2 macrophages in vitro and in vivo and reduce airway inflammation, while not suppressing the overall immune function of the host (91). Therefore, these innovative nanoparticles can be an attractive candidate for the potential immunotherapy for asthma.
MSCs-EVs are the hot spot of current research as they have been identified to inherit the anti-inflammatory and immunomodulatory properties of stem cells, inducing the M2 polarization of macrophages, reducing inflammation, while avoiding the disadvantage of stem cells such as tumorigenicity (46). Importantly, MSCs-EVs present a specificity of the EV/macrophage axis (46). In allergic rhinitis, human MSCs-EVs could inhibit the differentiation of Th2 cells via the regulation of the miR-146a-5p/SERPINB2 pathway (92), which shall be extrapolated for allergic inflammation of lower airways.
Several potential therapeutic strategies for asthma were sparked by an expansion in multi-omic and EV research. Lee et al. suggested immunoregulatory effects of Lactococcus lactis-derived EVs by shifting the immune responses from Th2 to Th1, mediated by DCs activation in allergic asthma (93). Studies on EV-based therapeutics for asthma treatment are highly anticipated. Preliminary findings reported an opportunity for inhalable dry powder mRNA vaccines based on EVs, which may pave the way to decreasing the risk for severe early-life respiratory infections like bronchiolitis and, consequently, de-risk further asthma development (79, 94, 95). Also, microbial EVs have recently been considered promising diagnostic and therapeutic tools for various inflammatory diseases (86).
3.4 Challenges and perspectives
Despite the magnitude of reports on EVs’ advantages as diagnostic markers or therapeutic agents, there is still much to learn about their features, biological functions, and potential particle-particle interaction (96). Among the studies gathered in this review, considerable diversity in methodology could be noticed. Another key thing to remember is that most of the findings were reported by individual in vitro and animal studies, whereas studies on clinical samples from asthma patients are less numerous. With growing heterogeneity in EVs collection, isolation and characterization, clinical and basic researchers recognize the need for a more standardized scalable sample collection and processing methodology to obtain reproducible results. Work is underway to deliver an update in 2022 on detailed Minimal information for studies of extracellular vesicles 2018 (MISEV2018) recommendations by the International Society for Extracellular Vesicles (ISEV) (1). Moreover, from a technical point of view, long-term storage and a freeze–thaw cycle of biosamples may lead to disturbance of various structures. Evidence suggests storage of EVs isolated from bronchoalveolar lavage fluid (BALF), could destabilize the surface characteristics, morphological features, and protein content of these EVs (97). Due to the protein leakage from the EVs into the supernatant, around 50% of protein composition showed differences in abundance in BALF-EVs as a result of the storage at both +4°C and −80°C. Thus, it was proposed that airway EVs should be best analyzed immediately after isolation. This constitutes another critical challenge for the implementation of EVs into daily clinical practice.
4 Conclusions
Taken together, EVs represent a potential novel player towards precision medicine in the diagnosis and treatment of asthma. Although rapidly evolving, the EV field is still in its initial stage. In the near future, it is expected that research efforts in this area will enable further understanding of the role of EVs in the complex mechanisms underlying asthma pathogenesis; hence, providing a solid background for precision medicine diagnostic and therapeutic solutions.
Author contributions
DA prepared the first draft of the manuscript, designed the figure and the table, and made the editing following other authors’ comments. AS-E, MC-K and WF reviewed the first draft of the manuscript, gave directions around the table and the figure design and worked on the editing of manuscript to help reach the final version. All authors contributed to the article and approved the submitted version.
Funding
This research was funded by the National Science Centre, Poland under grant PRELUDIUM 20 2021/41/N/NZ5/02950 (DA). The funds for open access publication fees were received from Medical University of Warsaw.
Conflict of interest
The authors declare that the research was conducted in the absence of any commercial or financial relationships that could be construed as a potential conflict of interest.
Publisher’s note
All claims expressed in this article are solely those of the authors and do not necessarily represent those of their affiliated organizations, or those of the publisher, the editors and the reviewers. Any product that may be evaluated in this article, or claim that may be made by its manufacturer, is not guaranteed or endorsed by the publisher.
References
1. Théry C, Witwer KW, Aikawa E, Alcaraz MJ, Anderson JD, Andriantsitohaina R, et al. Minimal information for studies of extracellular vesicles 2018 (MISEV2018): A position statement of the international society for extracellular vesicles and update of the MISEV2014 guidelines. J Extracell vesicles (2018) 7:1535750. doi: 10.1080/20013078.2018.1535750
2. Wen C, Seeger RC, Fabbri M, Wang L, Wayne AS, Jong AY. Biological roles and potential applications of immune cell-derived extracellular vesicles. J Extracell vesicles (2017) 6:1400370. doi: 10.1080/20013078.2017.1400370
3. Colombo M, Raposo G, Théry C. Biogenesis, secretion, and intercellular interactions of exosomes and other extracellular vesicles. Annu Rev Cell Dev Biol (2014) 30:255–89. doi: 10.1146/annurev-cellbio-101512-122326
4. Mateescu B, Kowal EJK, van Balkom BWM, Bartel S, Bhattacharyya SN, Buzás EI, et al. Obstacles and opportunities in the functional analysis of extracellular vesicle RNA – an ISEV position paper. J Extracell Vesicles (2017) 6:1286095. doi: 10.1080/20013078.2017.1286095
5. Ignatiadis M, Sledge GW, Jeffrey SS. Liquid biopsy enters the clinic — implementation issues and future challenges. Nat Rev Clin Oncol (2021) 18:297–312. doi: 10.1038/s41571-020-00457-x
6. Yuana Y, Böing AN, Grootemaat AE, van der Pol E, Hau CM, Cizmar P, et al. Handling and storage of human body fluids for analysis of extracellular vesicles. J Extracell Vesicles (2015) 4:29260. doi: 10.3402/jev.v4.29260
7. Zareba L, Szymanski J, Homoncik Z, Czystowska-Kuzmicz M. EVs from BALF-mediators of inflammation and potential biomarkers in lung diseases. Int J Mol Sci (2021) 22(7):3651. doi: 10.3390/ijms22073651
8. Bartel S, Deshane J, Wilkinson T, Gabrielsson S. Extracellular vesicles as mediators of cellular cross talk in the lung microenvironment. Front Med (2020) 7:326. doi: 10.3389/fmed.2020.00326
9. Demkow U, Stelmaszczyk-Emmel A. Extracellular vesicles in allergic rhinitis and asthma and laboratory possibilities for their assessment. Int J Mol Sci (2021) 22:2273. doi: 10.3390/ijms22052273
10. Huang F, Jia H, Zou Y, Yao Y, Deng Z. Exosomes: An important messenger in the asthma inflammatory microenvironment. J Int Med Res (2020) 48:300060520903220. doi: 10.1177/0300060520903220
11. GBD 2019 Diseases and Injuries Collaborators. Global burden of 369 diseases and injuries in 204 countries and territories, 1990-2019: A systematic analysis for the Global Burden of Disease Study 2019. Lancet (2020) 396(10258):1204–22. doi: 10.1016/S0140-6736(20)30925-9. Erratum in: Lancet. 2020 Nov 14;396(10262):1562.
12. Zhang D, Zheng J. The burden of childhood asthma by age group, 1990-2019: A systematic analysis of global burden of disease 2019 data. Front Pediatr (2022) 10:823399. doi: 10.3389/fped.2022.823399
13. Scherzer R, Grayson MH. Heterogeneity and the origins of asthma. Ann Allergy Asthma Immunol (2018) 121:400–5. doi: 10.1016/J.ANAI.2018.06.009
14. Dharmage SC, Perret JL, Custovic A. Epidemiology of asthma in children and adults. Front Pediatr (2019) 7:246. doi: 10.3389/fped.2019.00246
15. Lambrecht BN, Hammad H. The immunology of asthma. Nat Immunol (2015) 16:45–56. doi: 10.1038/ni.3049
16. Gupta R, Radicioni G, Abdelwahab S, Dang H, Carpenter J, Chua M, et al. Intercellular communication between airway epithelial cells is mediated by exosome-like vesicles. Am J Respir Cell Mol Biol (2019) 60:209–20. doi: 10.1165/rcmb.2018-0156OC
17. Kesimer M, Scull M, Brighton B, DeMaria G, Burns K, O’Neal W, et al. Characterization of exosome-like vesicles released from human tracheobronchial ciliated epithelium: A possible role in innate defense. FASEB J (2009) 23:1858–68. doi: 10.1096/fj.08-119131
18. Bartel S, La Grutta S, Cilluffo G, Perconti G, Bongiovanni A, Giallongo A, et al. Human airway epithelial extracellular vesicle miRNA signature is altered upon asthma development. Allergy (2020) 75:346–56. doi: 10.1111/all.14008
19. Schindler VEM, Alhamdan F, Preußer C, Hintz L, Alashkar Alhamwe B, Nist A, et al. Side-directed release of differential extracellular vesicle-associated microRNA profiles from bronchial epithelial cells of healthy and asthmatic subjects. Biomedicines (2022) 10(3):622. doi: 10.3390/biomedicines10030622
20. Ax E, Jevnikar Z, Cvjetkovic A, Malmhäll C, Olsson H, Rådinger M, et al. T2 and T17 cytokines alter the cargo and function of airway epithelium-derived extracellular vesicles. Respir Res (2020) 21:155. doi: 10.1186/s12931-020-01402-3
21. Kvarnhammar AM, Rydberg C, Järnkrants M, Eriksson M, Uddman R, Benson M, et al. Diminished levels of nasal S100A7 (psoriasin) in seasonal allergic rhinitis: An effect mediated by Th2 cytokines. Respir Res (2012) 13:2. doi: 10.1186/1465-9921-13-2
22. Lässer C, O’Neil SE, Shelke GV, Sihlbom C, Hansson SF, Gho YS, et al. Exosomes in the nose induce immune cell trafficking and harbour an altered protein cargo in chronic airway inflammation. J Transl Med (2016) 14:181. doi: 10.1186/s12967-016-0927-4
23. Potaczek DP, Miethe S, Schindler V, Alhamdan F, Garn H. Role of airway epithelial cells in the development of different asthma phenotypes. Cell Signal (2020) 69:109523. doi: 10.1016/J.CELLSIG.2019.109523
24. Hewitt RJ, Lloyd CM. Regulation of immune responses by the airway epithelial cell landscape. Nat Rev Immunol (2021) 21:347–62. doi: 10.1038/s41577-020-00477-9
25. Jia M, Yan X, Jiang X, Wu Y, Xu J, Meng Y, et al. Ezrin, a membrane cytoskeleton cross-linker protein, as a marker of epithelial damage in asthma. Am J Respir Crit Care Med (2019) 199:496–507. doi: 10.1164/rccm.201802-0373OC
26. Tang S, Jiang J, Zhang N, Sun J, Sun G. Tumor necrosis factor-α requires ezrin to regulate the cytoskeleton and cause pulmonary microvascular endothelial barrier damage. Microvasc Res (2020) 133:104093. doi: 10.1016/j.mvr.2020.104093
27. Zhang M, Yu Q, Tang W, Wu Y, Lv J, Sun L, et al. Epithelial exosomal contactin-1 promotes monocyte-derived dendritic cells-dominant T cell responses in asthma. J Allergy Clin Immunol (2021) 148(6):1545–1558. doi: 10.1016/j.jaci.2021.04.025
28. Yu Y, Zhou Y, Di C, Zhao C, Chen J, Su W, et al. Increased airway epithelial cell–derived exosomes activate macrophage-mediated allergic inflammation via CD100 shedding. J Cell Mol Med (2021) 25:8850–62. doi: 10.1111/jcmm.16843
29. D’Incà F, Pucillo CE. Exosomes: Tiny clues for mast cell communication. Front Immunol (2015) 6:73. doi: 10.3389/fimmu.2015.00073
30. Skokos D, Le Panse S, Villa I, Rousselle JC, Peronet R, Namane A, et al. Nonspecific b and T cell-stimulatory activity mediated by mast cells is associated with exosomes. Int Arch Allergy Immunol (2001) 124:133–6. doi: 10.1159/000053691
31. Skokos D, Botros HG, Demeure C, Morin J, Peronet R, Birkenmeier G, et al. Mast cell-derived exosomes induce phenotypic and functional maturation of dendritic cells and elicit specific immune responses in vivo. J Immunol (2003) 170:3037–45. doi: 10.4049/jimmunol.170.6.3037
32. Molfetta R, Lecce M, Quatrini L, Caracciolo G, Digiacomo L, Masuelli L, et al. Immune complexes exposed on mast cell-derived nanovesicles amplify allergic inflammation. Allergy (2020) 75:1260–3. doi: 10.1111/all.14103
33. Mion F, D’Incà F, Danelli L, Toffoletto B, Guarnotta C, Frossi B, et al. Mast cells control the expansion and differentiation of IL-10-competent b cells. J Immunol (2014) 193:4568–79. doi: 10.4049/jimmunol.1302593
34. Xie G, Yang H, Peng X, Lin L, Wang J, Lin K, et al. Mast cell exosomes can suppress allergic reactions by binding to IgE. J Allergy Clin Immunol (2018) 141:788–91. doi: 10.1016/j.jaci.2017.07.040
35. Liang Y, Qiao L, Peng X, Cui Z, Yin Y, Liao H, et al. The chemokine receptor CCR1 is identified in mast cell-derived exosomes. Am J Transl Res (2018) 10:352–67.
36. Aye CC, Toda M, Morohoshi K, Ono SJ. Identification of genes and proteins specifically regulated by costimulation of mast cell fcϵ receptor I and chemokine receptor 1. Exp Mol Pathol (2012) 92:267–74. doi: 10.1016/j.yexmp.2012.02.002
37. Zou Y, Zhou Q, Zhang Y. MicroRNA-21 released from mast cells-derived extracellular vesicles drives asthma in mice by potentiating airway inflammation and oxidative stress. Am J Transl Res (2021) 13:7475–91.
38. Eldh M, Ekström K, Valadi H, Sjöstrand M, Olsson B, Jernås M, et al. Exosomes communicate protective messages during oxidative stress; possible role of exosomal shuttle RNA. PloS One (2010) 5:e15353. doi: 10.1371/journal.pone.0015353
39. Lambrecht BN, Hammad H. Biology of lung dendritic cells at the origin of asthma. Immunity (2009) 31:412–24. doi: 10.1016/j.immuni.2009.08.008
40. Patente TA, Pelgrom LR, Everts B. Dendritic cells are what they eat: how their metabolism shapes T helper cell polarization. Curr Opin Immunol (2019) 58:16–23. doi: 10.1016/j.coi.2019.02.003
41. Huang L, Zhang X, Wang M, Chen Z, Yan Y, Gu W, et al. Exosomes from thymic stromal lymphopoietin-activated dendritic cells promote Th2 differentiation through the OX40 ligand. Pathobiology (2019) 86:111–7. doi: 10.1159/000493013
42. Esser J, Gehrmann U, D’Alexandri FL, Hidalgo-Estévez AM, Wheelock CE, Scheynius A, et al. Exosomes from human macrophages and dendritic cells contain enzymes for leukotriene biosynthesis and promote granulocyte migration. J Allergy Clin Immunol (2010) 126:1032–40. doi: 10.1016/j.jaci.2010.06.039
43. Vroman H, Hendriks RW, Kool M. Dendritic cell subsets in asthma: Impaired tolerance or exaggerated inflammation? Front Immunol (2017) 8:941. doi: 10.3389/fimmu.2017.00941
44. Careau E, Turmel V, Lauzon-Joset J-F, Bissonnette EY. Alveolar macrophages reduce airway hyperresponsiveness and modulate cytokine levels. Exp Lung Res (2010) 36:255–61. doi: 10.3109/01902140903410757
45. Lee YG, Jeong JJ, Nyenhuis S, Berdyshev E, Chung S, Ranjan R, et al. Recruited alveolar macrophages, in response to airway epithelial-derived monocyte chemoattractant protein 1/CCl2, regulate airway inflammation and remodeling in allergic asthma. Am J Respir Cell Mol Biol (2015) 52:772–84. doi: 10.1165/rcmb.2014-0255OC
46. Tang D, Cao F, Yan C, Fang K, Ma J, Gao L, et al. Extracellular Vesicle/Macrophage axis: Potential targets for inflammatory disease intervention. Front Immunol (2022) 13:705472. doi: 10.3389/fimmu.2022.705472
47. Bourdonnay E, Zasłona Z, Penke LRK, Speth JM, Schneider DJ, Przybranowski S, et al. Transcellular delivery of vesicular SOCS proteins from macrophages to epithelial cells blunts inflammatory signaling. J Exp Med (2015) 212:729–42. doi: 10.1084/jem.20141675
48. Draijer C, Speth JM, Penke LRK, Zaslona Z, Bazzill JD, Lugogo N, et al. Resident alveolar macrophage-derived vesicular SOCS3 dampens allergic airway inflammation. FASEB J (2020) 34:4718–31. doi: 10.1096/fj.201903089R
49. Li X, Yang N, Cheng Q, Zhang H, Liu F, Shang Y. MiR-21-5p in macrophage-derived exosomes targets Smad7 to promote epithelial mesenchymal transition of airway epithelial cells. J Asthma Allergy (2021) 14:513–24. doi: 10.2147/JAA.S307165
50. McBrien CN, Menzies-Gow A. The biology of eosinophils and their role in asthma. Front Med (2017) 4:93. doi: 10.3389/fmed.2017.00093
51. Mazzeo C, Cañas JA, Zafra MP, Rojas Marco A, Fernández-Nieto M, Sanz V, et al. Exosome secretion by eosinophils: A possible role in asthma pathogenesis. J Allergy Clin Immunol (2015) 135:1603–13. doi: 10.1016/j.jaci.2014.11.026
52. Cañas JA, Sastre B, Rodrigo-Muñoz JM, Fernández-Nieto M, Barranco P, Quirce S, et al. Eosinophil-derived exosomes contribute to asthma remodelling by activating structural lung cells. Clin Exp Allergy (2018) 48:1173–85. doi: 10.1111/cea.13122
53. Cañas JA, Sastre B, Mazzeo C, Fernández-Nieto M, Rodrigo-Muñoz JM, González-Guerra A, et al. Exosomes from eosinophils autoregulate and promote eosinophil functions. J Leukoc Biol (2017) 101:1191–9. doi: 10.1189/jlb.3AB0516-233RR
54. Ray A, Kolls JK. Neutrophilic inflammation in asthma and association with disease severity. Trends Immunol (2017) 38:942–54. doi: 10.1016/j.it.2017.07.003
55. Chen G, Chen D, Feng Y, Wu W, Gao J, Chang C, et al. Identification of key signaling pathways and genes in eosinophilic asthma and neutrophilic asthma by weighted gene Co-expression network analysis. Front Mol Biosci (2022) 9:805570. doi: 10.3389/fmolb.2022.805570
56. Vargas A, Roux-Dalvai F, Droit A, Lavoie J-P. Neutrophil-derived exosomes: A new mechanism contributing to airway smooth muscle remodeling. Am J Respir Cell Mol Biol (2016) 55:450–61. doi: 10.1165/rcmb.2016-0033OC
57. Zhang X-Y, Chen Z-C, Li N, Wang Z-H, Guo Y-L, Tian C-J, et al. Exosomal transfer of activated neutrophil-derived lncRNA CRNDE promotes proliferation and migration of airway smooth muscle cells in asthma. Hum Mol Genet (2022) 31:638–50. doi: 10.1093/hmg/ddab283
58. Azoulay-Alfaguter I, Mor A. Proteomic analysis of human T cell-derived exosomes reveals differential RAS/MAPK signaling. Eur J Immunol (2018) 48:1915–7. doi: 10.1002/eji.201847655
59. Tung SL, Boardman DA, Sen M, Letizia M, Peng Q, Cianci N, et al. Regulatory T cell-derived extracellular vesicles modify dendritic cell function. Sci Rep (2018) 8:6065. doi: 10.1038/s41598-018-24531-8
60. Smyth LA, Ratnasothy K, Tsang JYS, Boardman D, Warley A, Lechler R, et al. CD73 expression on extracellular vesicles derived from CD4+ CD25+ Foxp3+ T cells contributes to their regulatory function. Eur J Immunol (2013) 43:2430–40. doi: 10.1002/eji.201242909
61. Admyre C, Bohle B, Johansson SM, Focke-Tejkl M, Valenta R, Scheynius A, et al. B cell-derived exosomes can present allergen peptides and activate allergen-specific T cells to proliferate and produce TH2-like cytokines. J Allergy Clin Immunol (2007) 120:1418–24. doi: 10.1016/j.jaci.2007.06.040
62. Weiss ARR, Dahlke MH. Immunomodulation by mesenchymal stem cells (MSCs): Mechanisms of action of living, apoptotic, and dead MSCs. Front Immunol (2019) 10:1191. doi: 10.3389/fimmu.2019.01191
63. Ma Y, Liu X, Long Y, Chen Y. Emerging therapeutic potential of mesenchymal stem cell-derived extracellular vesicles in chronic respiratory diseases: An overview of recent progress. Front Bioeng Biotechnol (2022) 10:845042. doi: 10.3389/fbioe.2022.845042
64. Planat-Benard V, Varin A, Casteilla L. MSCs and inflammatory cells crosstalk in regenerative medicine: Concerted actions for optimized resolution driven by energy metabolism. Front Immunol (2021) 12:626755. doi: 10.3389/fimmu.2021.626755
65. Mun SJ, Kang SA, Park H-K, Yu HS, Cho K-S, Roh H-J. Intranasally administered extracellular vesicles from adipose stem cells have immunomodulatory effects in a mouse model of asthma. Stem Cells Int (2021) 2021:6686625. doi: 10.1155/2021/6686625
66. Shang Y, Sun Y, Xu J, Ge X, Hu Z, Xiao J, et al. Exosomes from mmu_circ_0001359-modified ADSCs attenuate airway remodeling by enhancing FoxO1 signaling-mediated M2-like macrophage activation. Mol Ther Nucleic Acids (2020) 19:951–60. doi: 10.1016/j.omtn.2019.10.049
67. Shan L, Liu S, Zhang Q, Zhou Q, Shang Y. Human bone marrow-mesenchymal stem cell-derived exosomal microRNA-188 reduces bronchial smooth muscle cell proliferation in asthma through suppressing the JARID2/Wnt/β-catenin axis. Cell Cycle (2022) 21:352–67. doi: 10.1080/15384101.2021.2020432
68. Du Y, Zhuansun Y, Chen R, Lin L, Lin Y, Li J. Mesenchymal stem cell exosomes promote immunosuppression of regulatory T cells in asthma. Exp Cell Res (2018) 363:114–20. doi: 10.1016/J.YEXCR.2017.12.021
69. Zhuansun Y, Du Y, Huang F, Lin L, Chen R, Jiang S, et al. MSCs exosomal miR-1470 promotes the differentiation of CD4+CD25+FOXP3+ tregs in asthmatic patients by inducing the expression of P27KIP1. Int Immunopharmacol (2019) 77:105981. doi: 10.1016/J.INTIMP.2019.105981
70. Reis M, Mavin E, Nicholson L, Green K, Dickinson AM, Wang X. Mesenchymal stromal cell-derived extracellular vesicles attenuate dendritic cell maturation and function. Front Immunol (2018) 9:2538. doi: 10.3389/fimmu.2018.02538
71. Dong L, Wang Y, Zheng T, Pu Y, Ma Y, Qi X, et al. Hypoxic hUCMSC-derived extracellular vesicles attenuate allergic airway inflammation and airway remodeling in chronic asthma mice. Stem Cell Res Ther (2021) 12:4. doi: 10.1186/s13287-020-02072-0
72. Dong B, Wang C, Zhang J, Zhang J, Gu Y, Guo X, et al. Exosomes from human umbilical cord mesenchymal stem cells attenuate the inflammation of severe steroid-resistant asthma by reshaping macrophage polarization. Stem Cell Res Ther (2021) 12:204. doi: 10.1186/s13287-021-02244-6
73. Fang S-B, Zhang H-Y, Wang C, He B-X, Liu X-Q, Meng X-C, et al. Small extracellular vesicles derived from human mesenchymal stromal cells prevent group 2 innate lymphoid cell-dominant allergic airway inflammation through delivery of miR-146a-5p. J Extracell vesicles (2020) 9:1723260. doi: 10.1080/20013078.2020.1723260
74. Fang S-B, Zhang H-Y, Meng X-C, Wang C, He B-X, Peng Y-Q, et al. Small extracellular vesicles derived from human MSCs prevent allergic airway inflammation via immunomodulation on pulmonary macrophages. Cell Death Dis (2020) 11:409. doi: 10.1038/s41419-020-2606-x
75. Comfort N, Bloomquist TR, Shephard AP, Petty CR, Cunningham A, Hauptman M, et al. Isolation and characterization of extracellular vesicles in saliva of children with asthma. Extracell vesicles Circ Nucleic Acids (2021) 2:29–48. doi: 10.20517/evcna.2020.09
76. Lucchetti D, Santini G, Perelli L, Ricciardi-Tenore C, Colella F, Mores N, et al. Detection and characterization of extracellular vesicles in exhaled breath condensate and sputum of COPD and severe asthma patients. Eur Respir J (2021) 58(2):2003024. doi: 10.1183/13993003.03024-2020
77. Szefler SJ, Chipps B. Challenges in the treatment of asthma in children and adolescents. Ann Allergy Asthma Immunol (2018) 120(4):382–388. doi: 10.1016/j.anai.2018.01.003 Erratum in: Ann Allergy Asthma Immunol. 2018 Sep;121(3):382.
78. Elenius V, Chawes B, Malmberg PL, Adamiec A, Ruszczyński M, Feleszko W, et al. Lung function testing and inflammation markers for wheezing preschool children: A systematic review for the EAACI clinical practice recommendations on diagnostics of preschool wheeze. Pediatr Allergy Immunol (2021) 32:501–13. doi: 10.1111/pai.13418
79. Makrinioti H, Hasegawa K, Lakoumentas J, Xepapadaki P, Tsolia M, Castro-Rodriguez JA, et al. The role of respiratory syncytial virus- and rhinovirus-induced bronchiolitis in recurrent wheeze and asthma–a systematic review and meta-analysis. Pediatr Allergy Immunol (2022) 33(3):e13741. doi: 10.1111/pai.13741
80. Nakagome K, Nagata M. Innate immune responses by respiratory viruses, including rhinovirus, during asthma exacerbation. Front Immunol (2022) 13:865973. doi: 10.3389/fimmu.2022.865973
81. Chahar HS, Corsello T, Kudlicki AS, Komaravelli N, Casola A. Respiratory syncytial virus infection changes cargo composition of exosome released from airway epithelial cells. Sci Rep (2018) 8:387. doi: 10.1038/s41598-017-18672-5
82. Gutierrez MJ, Gomez JL, Perez GF, Pancham K, Val S, Pillai DK, et al. Airway secretory microRNAome changes during rhinovirus infection in early childhood. PLoS One (2016) 11:e0162244. doi: 10.1371/journal.pone.0162244
83. Mills JT, Schwenzer A, Marsh EK, Edwards MR, Sabroe I, Midwood KS, et al. Airway epithelial cells generate pro-inflammatory tenascin-c and small extracellular vesicles in response to TLR3 stimuli and rhinovirus infection. Front Immunol (2019) 10:1987. doi: 10.3389/fimmu.2019.01987
84. Oh S-J, Lee E-N, Park J-H, Lee JK, Cho GJ, Park I-H, et al. Anti-viral activities of umbilical cord mesenchymal stem cell-derived small extracellular vesicles against human respiratory viruses. Front Cell Infect Microbiol (2022) 12:850744. doi: 10.3389/fcimb.2022.850744
85. Choi Y, Park H, Park HS, Kim YK. Extracellular vesicles, a key mediator to link environmental microbiota to airway immunity. Allergy Asthma Immunol Res (2017) 9:101–6. doi: 10.4168/aair.2017.9.2.101
86. Yang J, Shin T-S, Kim JS, Jee Y-K, Kim Y-K. A new horizon of precision medicine: Combination of the microbiome and extracellular vesicles. Exp Mol Med (2022) 54:466–82. doi: 10.1038/s12276-022-00748-6
87. Kopp F, Mendell JT. Functional classification and experimental dissection of long noncoding RNAs. Cell (2018) 172:393–407. doi: 10.1016/j.cell.2018.01.011
88. Wang Z, Zhang J, Feng T, Zhang D, Pan Y, Liu X, et al. Construction of lncRNA-mediated competing endogenous RNA networks correlated with T2 asthma. Front Genet (2022) 13:872499. doi: 10.3389/fgene.2022.872499
89. Vázquez-Mera S, Martelo-Vidal L, Miguéns-Suárez P, Saavedra-Nieves P, Arias P, González-Fernández C, et al. Serum exosome inflamma-miRs are surrogate biomarkers for asthma phenotype and severity. Allergy (2022). doi: 10.1111/all.15480
90. Herrmann IK, Wood MJA, Fuhrmann G. Extracellular vesicles as a next-generation drug delivery platform. Nat Nanotechnol (2021) 16:748–59. doi: 10.1038/s41565-021-00931-2
91. Pei W, Li X, Bi R, Zhang X, Zhong M, Yang H, et al. Exosome membrane-modified M2 macrophages targeted nanomedicine: Treatment for allergic asthma. J Control Release (2021) 338:253–67. doi: 10.1016/J.JCONREL.2021.08.024
92. Zhou J, Lu Y, Wu W, Feng Y. HMSC-derived exosome inhibited Th2 cell differentiation via regulating miR-146a-5p/SERPINB2 pathway. J Immunol Res (2021) 2021:6696525. doi: 10.1155/2021/6696525
93. Lee D-H, Park H-K, Lee H-R, Sohn H, Sim S, Park HJ, et al. Immunoregulatory effects of lactococcus lactis-derived extracellular vesicles in allergic asthma. Clin Transl Allergy (2022) 12:e12138. doi: 10.1002/clt2.12138
94. Popowski KD, Moatti A, Scull G, Silkstone D, Lutz H, López de Juan Abad B, et al. Inhalable dry powder mRNA vaccines based on extracellular vesicles. Matter (2022) 5(9):2960–2974. doi: 10.1016/j.matt.2022.06.012
95. Fujiogi M, Dumas O, Hasegawa K, Jartti T, Camargo CA. Identifying and predicting severe bronchiolitis profiles at high risk for developing asthma: Analysis of three prospective cohorts. eClinicalMedicine (2022) 43:101257. doi: 10.1016/j.eclinm.2021.101257
96. Beit-Yannai E, Tabak S, Stamer WD. Physical exosome: Exosome interactions. J Cell Mol Med (2018) 22:2001–6. doi: 10.1111/jcmm.13479
Keywords: asthma, extracellular vesicle, exosome, precision medicine, intercellular communication, microRNA, allergy, inflammation
Citation: Ambrożej D, Stelmaszczyk-Emmel A, Czystowska-Kuźmicz M and Feleszko W (2022) “Liquid biopsy” - extracellular vesicles as potential novel players towards precision medicine in asthma. Front. Immunol. 13:1025348. doi: 10.3389/fimmu.2022.1025348
Received: 22 August 2022; Accepted: 31 October 2022;
Published: 17 November 2022.
Edited by:
Hideaki Morita, National Center for Child Health and Development (NCCHD), JapanReviewed by:
Hae-Sim Park, Ajou University Hospital, South KoreaCopyright © 2022 Ambrożej, Stelmaszczyk-Emmel, Czystowska-Kuźmicz and Feleszko. This is an open-access article distributed under the terms of the Creative Commons Attribution License (CC BY). The use, distribution or reproduction in other forums is permitted, provided the original author(s) and the copyright owner(s) are credited and that the original publication in this journal is cited, in accordance with accepted academic practice. No use, distribution or reproduction is permitted which does not comply with these terms.
*Correspondence: Wojciech Feleszko, d29qY2llY2guZmVsZXN6a29Ad3VtLmVkdS5wbA==