- Institut de Pharmacologie et de Biologie Structurale (IPBS), Université de Toulouse, CNRS, Université Toulouse III - Paul Sabatier (UPS), Toulouse, France
Megakaryocytes (MKs) are large cells giving rise to platelets. It is well established that in adults, MKs develop from hematopoietic stem cells and reside in the bone marrow. MKs are also rare but normal constituents of the venous blood returning to the lungs, and MKs are found in the lung vasculature (MKcirc), suggesting that these cells are migrants from the bone marrow and get trapped in lung capillaries where the final steps of platelet production can occur. An unprecedented increase in the number of lung and circulating MKs was described in coronavirus disease 2019 (COVID-19) patients, suggesting that lung thrombopoiesis may be increased during lung infection and/or thromboinflammation. In addition to the population of platelet-producing intravascular MKs in the lung, a population of lung-resident megakaryocytes (MKL) has been identified and presents a specific immune signature compared to its bone marrow counterparts. Recent single-cell analysis and intravital imaging have helped us gain a better understanding of these populations in mouse and human. This review aims at summarizing the recent data on increased occurrence of lung MKs and discusses their origin, specificities, and potential role in homeostasis and inflammatory and infectious lung diseases. Here, we address remaining questions, controversies, and methodologic challenges for further studies of both MKcirc and MKL.
Introduction
Platelets are small anucleate blood cells with active roles in a wide range of physiological responses, including hemostasis, thrombosis, wound healing, and immunity. In humans, 150–450 billion platelets circulate per liter of blood, with a life span of 8–10 days, requiring a daily turnover of 100 billion platelets (70 million/min) to maintain a physiological platelet concentration. Platelets originate from megakaryocytes (MKs), large cells described for the first time in 1890 by Howell and Donahue (1) . With an approximate diameter of 100 μm, MKs are large but rare cells, accounting for 10 MK/ml in venous blood and representing only 0.01% of all nucleated cells in human bone marrow. This paucity, as well as the importance of their in vivo environment for their maintenance, differentiation, and platelet production, makes it difficult to isolate and study them. Thus, the understanding of MK biology still contains some gray areas compared to other hematopoietic cell types. Recently, the development of single-cell analysis and in vivo analysis have revealed new insights into the MK journey from progenitor cells to platelets, particularly regarding the mechanisms of differentiation, their heterogeneity, the final steps of platelet generation, and the different locations involved. The relative importance of lung thrombopoiesis and the location, occurrence, origin, and function of lung MKs in homeostasis and during infection and inflammation will be discussed in this review.
Sites of megakaryocyte maturation and platelet production
Thrombopoiesis, the process of platelet production, can be separated into two steps: the differentiation and maturation of MKs (megakaryopoiesis) (Figure 1), followed by the actual process of platelet release resulting from MK cytoplasmic extensions called proplatelets (Figure 2). These two steps can take place in different sites, in the bone marrow and outside, evolving and adapting to platelet needs during development, aging, or stress.
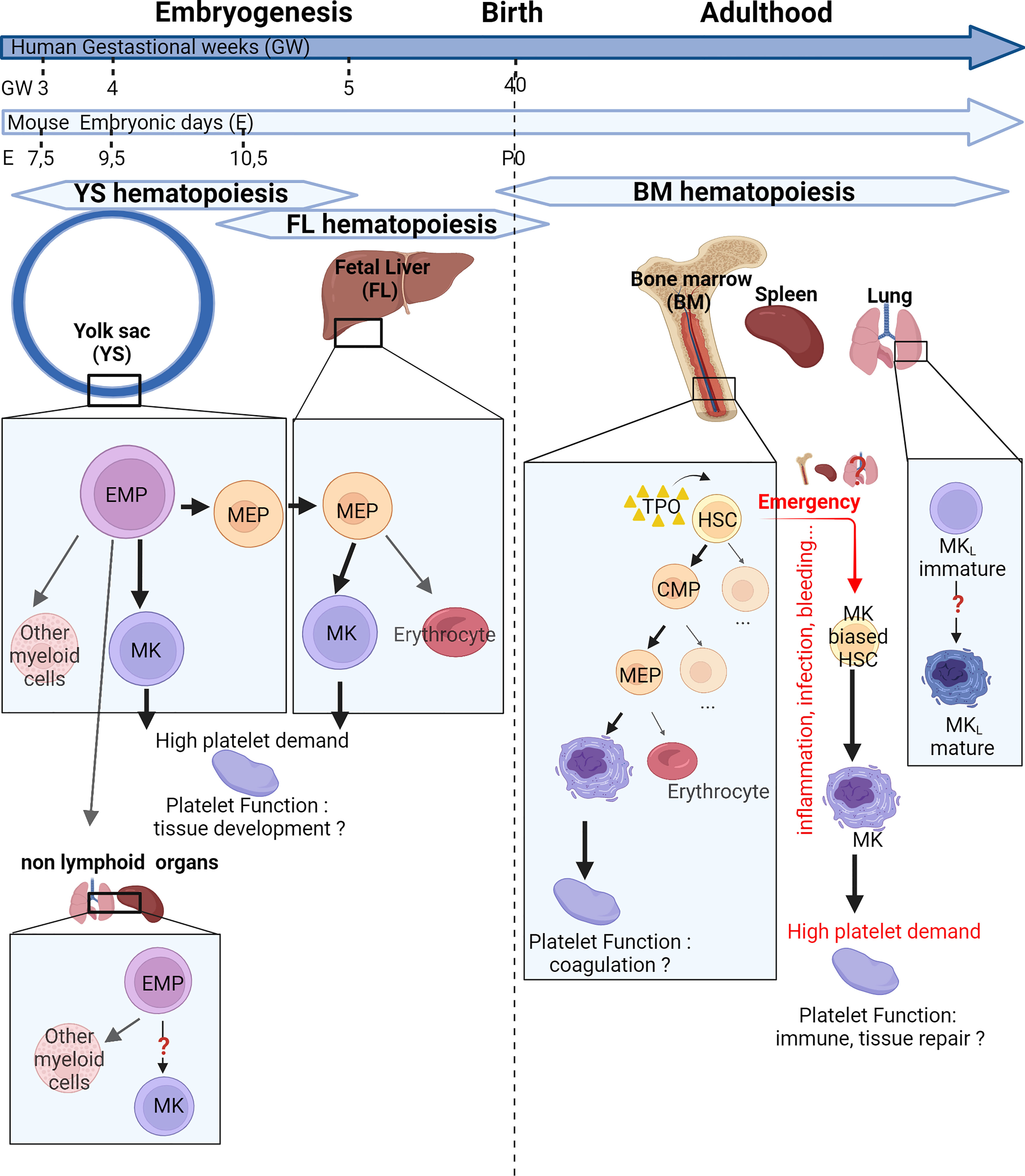
Figure 1 Place and mechanisms of megakaryocyte differentiation over time. The hematopoiesis begins in the yolk sac at 7.5 days post-conception (E7.5) in mice or 3 gestational weeks (GW) in human. At E9.5 or at GW4, the first megakaryocytes (MKs) originate from yolk sac-derived erythroid–myeloid progenitors (EMPs) bypassing the bipotential MK–erythrocyte progenitors (MEPs). Later, a new wave of MKs originate from bipotential MEPs in the fetal liver. EMPs can colonize nonlymphoid organs and are capable of differentiation in myeloid cells. After birth, the bone marrow gradually takes over from the liver. MKs can differentiate from multipotent progenitors (MPPs) or, in case of high platelet need, directly from MK-biased hematopoietic stem cells (HSCs). Extramedullary megakaryopoiesis also occurs in the spleen in case of sepsis. Finally, the lung presents MK progenitors and resident MKs presenting an immune phenotype. These different MK subsets could produce specialized platelet populations capable of immune functions and tissue development. CMP, common myeloid progenitor; MKL, Lung resident Megakaryocytes; TPO, Thrombopoietin.
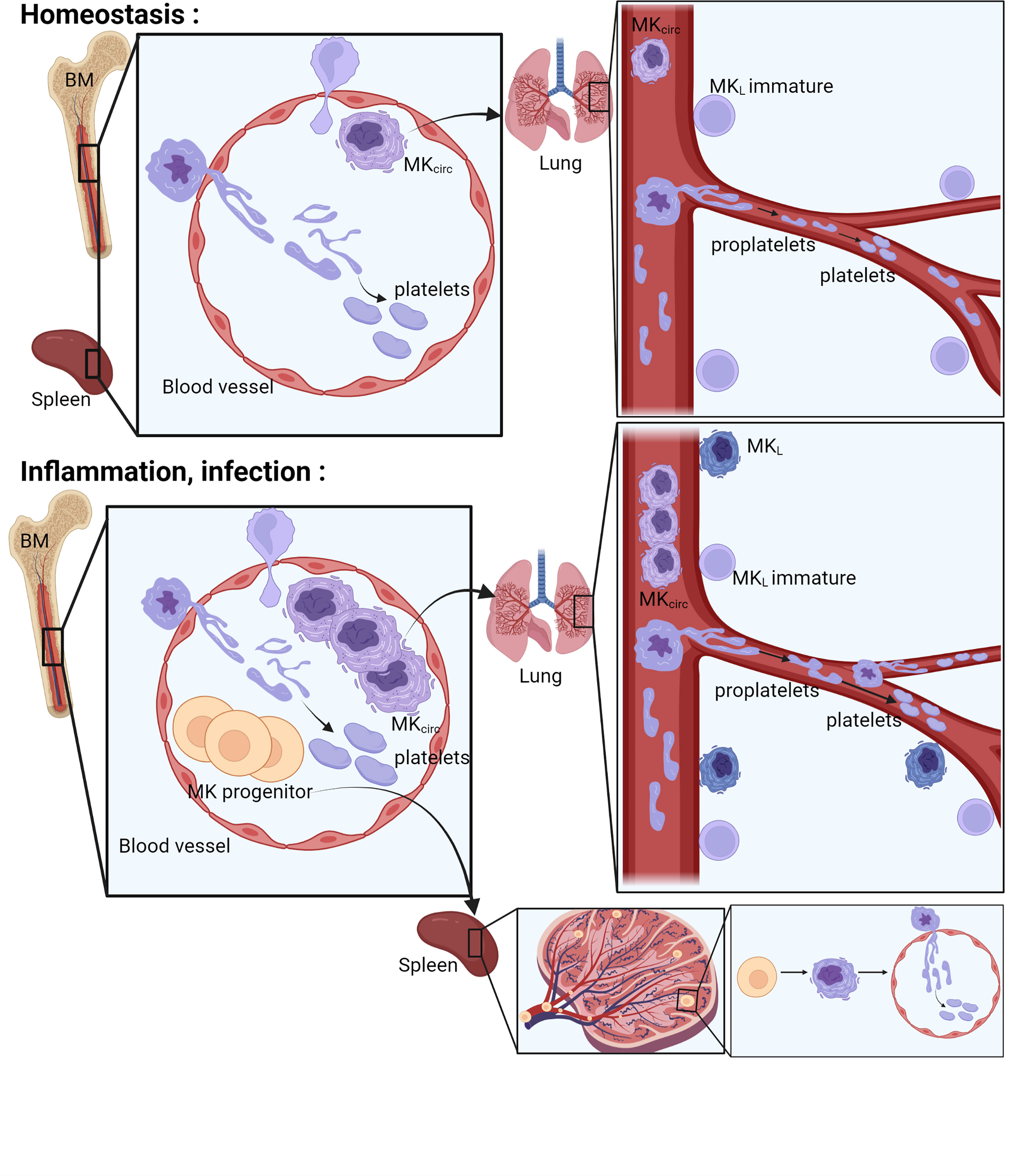
Figure 2 Place of platelet production. The bone marrow, the spleen, and the lung have been described as places where the final steps of platelet production occur. In the bone marrow and in the spleen, the MKs extend their cytoplasm to the lumen of the sinusoids and form proplatelets. Proplatelets and more rarely whole MKs are released in the bloodstream, get trapped in the lung vasculature where they will accomplish the final step of platelet production. During inflammation or infection, an increase of circulating MKs and MK progenitors is observed, followed by progenitor differentiation and platelet production in the spleen and increased platelet production in the lung vasculature. MK, megakaryocyte; MKL, Lung resident Megakaryocytes; MKcirc, Circulating Megakaryocytes; BM, Bone Marrow.
Pathways of megakaryocyte differentiation
Megakaryopoiesis has long been described as a hierarchical process, with hematopoietic stem cells (HSCs) giving rise to various blood lineages including the common lymphoid progenitor (CLP) and the common myeloid progenitor (CMP). The CMP further differentiates into the granulocyte/macrophage progenitor and the megakaryocyte–erythrocyte progenitor (MEP), which then further differentiate into MK progenitors and ultimately into mature MKs. Over the last few years, studies have challenged traditional paradigms about the origin of the MK lineage in both fetal and adult life, and the application of single-cell RNA sequencing (RNA-Seq) has led to a better characterization of embryonic, fetal, and adult MKs. The hierarchical model of MK differentiation was challenged by the observations of MK-biased hematopoietic progenitors and alternative routes by which MKs can directly differentiate from HSCs, bypassing traditional lineage checkpoints and progenitors (2–4). The production of platelets from MK-biased hematopoietic progenitors is observed preferentially after reduced platelet count (5), inflammation, myeloproliferative neoplasms, aging, and recently, respiratory viral infection (6), also called “emergency” megakaryopoiesis and reviewed by Noetzli et al. (7) (Figure 1). Mechanistically, MK-biased HSC is posttranscriptionally regulated by the repression of MK-specific transcript translation steady state (8) and before birth (9). The restriction, maintained by the RNA-binding protein LIN28B, is lifted during development or inflammatory stress by the downregulation of the protein, leading to the increase of MK-biased HSCs.
Places of megakaryocyte differentiation
During mouse embryogenesis, two pathways have recently been uncovered to produce definitive MKs (Figure 1). The initial source of platelets comes from the yolk sac and starts at Embryonic day (E) 9.5 directly from yolk sac-derived erythroid-myeloid progenitors (EMPs), the earliest precursors of erythrocytes, MKs, and macrophages, bypassing intermediate bipotent MEPs (10, 11). Later in development, yolk sac-EMPs give rise to bipotent MEPs migrating to the fetal liver starting from E10.5 and terminally differentiate into both erythrocytes and polyploid MKs in the fetal liver (10). In human, a shared megakaryocyte–erythroid–mast cell progenitor (MEMP) was identified in fetal liver, also present in non-lymphoid tissues (skin and kidney) from 7 to 12 weeks after conception. HSC/multipotent progenitors (MPPs) were absent in non-lymphoid tissues where MEMPs can locally differentiate (12). A few months after birth, the contribution of the fetal liver as a main site of platelet production declines progressively and the bone marrow takes over platelet production, lasting through adulthood. Other sites of hematopoiesis and megakaryopoiesis outside of the bone marrow have been described, particularly in response to hematopoietic stress, severe infection, and inflammation. MKs and progenitors are present in healthy spleen in mouse and human (13), where increased MK maturation has been observed after murine HSC transplantation (14, 15), sepsis (13), or myelofibrosis (16). Recent reports in the literature also indicate the presence of hematopoietic stem and progenitor cells (17, 18) and in particular MKs in fetal and adult lung, with a transcriptional signature suggesting a role in immunity [lung-resident megakaryocytes (MKL)] (19, 20). An idea of the relative presence of MKs in different locations Bone marrow (BM), spleen, and lung] is given by the quantification of MKs by flow cytometry in the adult mouse, indicating that 79% of MKs are found in the BM, 13% in the spleen, and 8% in the lungs (without exclusion of circulating MKs and cells aggregated with platelets) (21).
Places of final platelet production
In the final phases of differentiation, MKs, residing in close proximity to BM sinusoids (22), interact with endothelial cells and, using a transcellular route, extend protrusive podosomes to elongate their cytoplasm to form branched pseudopodial extensions called proplatelets (23) (Figure 2). These proplatelets extend into the lumen of bone marrow sinusoids and, under shear and turbulent flow, are released into the bloodstream. The final release of platelets then occurs within the blood where a particular role for the lung vasculature has been proposed. Indeed, human MKs transfused into mice end up trapped in the lung vasculature and release functional platelets (with the ability to incorporate into developing thrombi after laser-induced injuries) (24, 25). Proplatelets and entire MKs have been observed in the lung vasculature since 1930s (1, 26–28), and abundant cytoplasm-circulating MKs are found in higher number in the blood that enter the lung than in the blood exiting it. Thanks to the development of intravital microscopy technique to the lung, and as an ultimate proof of platelet release in the lung, direct and dynamic platelet production was observed in mouse lung vasculature (19). MKs are observed in the lung vasculature physiologically (MKcirc), but their frequency increases with pathological conditions, likely associated with an increased platelet production following platelet consumption observed during lung infection and/or thromboinflammation, as largely reported in coronavirus disease 2019 (COVID-19). This increased occurrence will be reviewed specifically in a following section.
Location of megakaryocytes in the lung of healthy individuals
Circulating megakaryocytes found in lung capillaries (MKcirc)
We can distinguish two types of lung MKs: those found in the vasculature (MKcirc) and those found residing in the tissue (MKL). In healthy human, most of the large MKs, recognized by the size of their cytoplasm and nucleus, are intravascular, localized within the capillary lumen of the alveolar wall. They are found in histological sections in 67% of healthy autopsy (individuals who died suddenly and without any injury of the thorax), with an average value of 4 MK/cm2 (maximum of 16 MK/cm2) of lung tissue (26, 29, 30). Most of the MKcirc (75%) are medium sized (20–49 µm; with a moderate amount of cytoplasm), 10% are large MKs (50–100 µm; with abundant cytoplasm), and 15% are small MKs (<20 µm; with a thin rim of cytoplasm, resembling naked or semi-naked nuclei) (28). MKcirc can also be quantified from the venous blood, before entering the lung. An average value of 10 MK/ml (maximum of 30 MK/ml) is detected, half of them with abundant cytoplasm and the numbers are markedly reduced in the arterial circulation (after lung filtration) with the majority of MKs devoid of cytoplasm (naked MKs). With the development of intravital microscopy, direct observation of dynamic events occurring in mouse lungs has been made possible (31). Using platelet/MK reporter mice, direct evidence was provided that platelet release occurs physiologically in the lung (19). Both nucleated MKs and large cytoplasmic fragments are observed in the pulmonary circulation, trapped at vascular bifurcations. MKs and proplatelets are clearly differentiated from platelet aggregates and thrombus that can occur in lung vessels following platelet activation (32). During a process ranging from 20 to 60 min, proplatelet extension results in the release of 150–2,500 single platelets (average of 700). In the subpleural region observed by lung intravital microscopy (2 mm2 × 40 µm), two MKs/proplatelets per hour were witnessed releasing platelets in the lung vasculature. Altogether, these reports indicate that platelet release occurs physiologically in the lung vasculature from circulating MKs. The contribution of the lung in platelet production has been a matter of debate, as a study indicates that in lung tissue, MKs were rare in whole cross sections from normal and perturbed mice, even during periods of strong stimulation of thrombopoiesis (33). Lung MKs are not uniformly distributed in the lung and are preferentially localized to the distal lung within the interstitial space or within the capillary lumen between adjacent alveoli (28) (34) but not deeper in the lung (33). The alveolar region is also the region accessible by intravital microscopy where platelet release in the lung was observed (19). Morphologically, MKcirc are squeezed within alveolar capillaries and will be harder to identify than resident MKs in the BM or spleen. Attempts were made and reviewed to estimate the contribution of the lung in the terminal step of platelet production (35–38). All of them propose a significant contribution of the lung, ranging from 7% to 98%, even though these calculations have weaknesses such as the use of approximated values for platelet life span, percentage of circulating MK reaching the lung, percentage of platelets sequestrated in the spleen, platelets produced per MK, or extrapolation of observed region to whole lung. Interestingly, thrombocytopenia is common in patients with congenital heart conditions associated with right to left shunting and following cardiopulmonary bypass, with an incidence over 30%. Two to three days following surgery, the average platelet count decreases by half. It is classically attributed to consumption of platelets activated during the extracorporeal circulation. However, an intriguing finding is that MKcirc increase 3-fold in these patients who have the lung circulation bypassed. Thrombocytopenia in these settings could be due to underproduction of platelets in the lung, in addition to consumption (39). One can wonder how these large cells (~50 µm) can pass through such small capillaries (5 µm). In vitro, in a circulatory flow system, it was shown that mature MKs can divide in the circulation. The MK deforms and elongates, and the nucleus tends to separate into two distinct lobes in parallel with proplatelet formation. Intact large MKs have been observed after lung filtration, suggesting that they can go through the capillary beds without fragmentation (40). The deformation occurring in vivo in the lung capillaries and its impact on the MK biology remain to be elucidated.
Lung-resident megakaryocytes in the interstitium (MKL)
In addition to the population of platelet-producing intravascular MKs in the mouse lung (MKcirc), a second population of MKs, residing in the pulmonary interstitium, has been identified in the mouse lung (MKL)—thanks to their expression of specific MK markers by three independent studies and were further analyzed by bulk or single-cell RNA-Seq analysis (19, 20, 34). MKL represent 0.1% to 2%–3% of lung cells. Different markers were used to identify the MKL population: Lin-, CD41+, PF4-expressing nucleated cells (19); Lin-CD41+ cells; or clustering based on Pf4, Itga2b, Ppbp, Itgb3, Gp9, and Cxcr4 (20) or isolation of top 1% of CD41+ events and MK-lineage clustering based on Fli1, Pf4, and Itga2b (34). Contrary to human observations of lung tissue section where most of lung MKs were intravascular, resident MKL actually represent the majority of lung MKs (60%–80%). This discrepancy may come from the fact that MKL are smaller and more immature than MKs present in the bone marrow or those found in the vasculature (MKcirc). MKL are mainly diploid and may not have been included in the human studies that identified MK by the large size of their cytoplasm and nucleus. Yeung et al. (34) did not find MKL to be immature, but it may be explained by their isolation strategy using only the top 1% of CD41+ events, thus focusing the analysis on more mature MKs. A common result from these three studies is the differential expression of many genes associated with innate immunity, inflammation, and pathogen recognition compared with bone marrow MKs, pointing to a potential immune role for lung extravascular MKs that will be further discussed.
Modulation of lung megakaryocyte occurrence with pathologies
Increased presence of megakaryocytes in the lung (MKL and MKcirc)
We have seen that MKs are found in 67% of healthy lungs (4 MK/cm2), but they are almost always found in 94% of hospitalized patients and in higher density with an average value of 37 MK/cm2 (maximum of 765 MK/cm2) (26, 29), suggesting an increased occurrence with various pathologies. Pathologies associated with an increase or decrease of lung MKs are listed in Table 1. In human, lung MKs were increased in association with vascular diseases often associated with excessive coagulation (thromboembolic disease, intravascular coagulation, myocardial infarction, and severe atheroma) (26, 28, 29), tissue damage and bleeding (shock, burns (41), hemorrhage), cancer (42, 43), inflammatory lung diseases [acute respiratory distress syndrome (ARDS) (44), idiopathic pulmonary arterial hypertension (28), fibrosis, asthma (45)], and infectious diseases. More recently, the COVID-19 pandemic has particularly revealed the presence of MKs in the lungs of patients. In fact, between 7 and 10 times more MKs have been counted in the lungs of patients with COVID-19 compared to healthy patients and between 2 and 3 times more compared to patients with other causes of ARDS (46–48). MKs found in COVID-19 non-survivor patients show signs of maturation (increased diameter and bigger nucleus) and are found 2.5 times more frequently in the alveoli/parenchyma than in the blood vessel. The majority of lung MKs (70%) are found outside of the vasculature in the lung. The increase in MKL is associated with an equal increase in the bronchoalveolar lavage (BAL), in the BM, and in the circulation of these patients (49). MKs entrapped in lung vasculature were also observed after influenza infection (50). In healthy lungs, MKs are not observed in alveolar or pleural fluid, but they were detected in human BAL of patients with severe COVID-19 (49) and suspected lung carcinoma (51) and in pleural fluids in patients with myeloproliferative disorders, trauma, and tumors. In those cases, the presence of MKL may be the result of increased vascular and epithelial permeability, inducing MKcirc entry in the tissue and fluid or the result of extramedullar hematopoiesis (52). The common pathogenic mechanism explaining the increased number of pulmonary MKs seems thus to be associated with platelet loss due to consumption (due to activation in case of excessive coagulation or inflammation) or bleeding and may be associated with accelerated thrombopoietic activity. In animal models, an increase in pulmonary MKs was also observed following Listeria monocytogenes infection (53), thrombin-induced microthrombi in dogs (54), systemic inflammation induced by Tumor necrosis factor alpha (TNF-α) in rats (55), bleomycin-induced lung damage and fibrosis (56), Thrombopoietin (TPO)-accelerated thrombopoiesis (19, 27), and bleeding (27) in mice.
Decreased presence of lung megakaryocytes
A reduced number of pulmonary MKs are less often described, but in human, low values were correlated with thrombocytopenia, leukemia (29), and Chronic obstructive pulmonary disease (COPD) (28). In mouse, a decrease was observed in the lung of hypoxic mice, associated with reduced platelet production (57). In vitro hypoxia enhances human lymphoid differentiation (58) and biased hematopoiesis toward lymphocyte generation or reduced thrombopoiesis may explain the decreased number of pulmonary MK.
Origin of circulating megakaryocytes
MK/proplatelet egress from extrapulmonary sources (bone marrow, spleen)
Platelet-producing MKs and proplatelets found in the lung circulation are thought to come from other organs such as the bone marrow and spleen. Indeed, by examining mouse lungs with non-fluorescent MK/platelet transplanted into MK/platelet fluorescent mice, fluorescent MKs were observed in the lung vasculature, while no MKcirc was observed in fluorescent MK/platelet lung transplanted into MK/platelet non-fluorescent mice. This means that the production of platelets in the lung circulation is dependent on MKs exiting the bone marrow or other extrapulmonary sources and traveling to the lung. Direct visualization of the bone marrow and spleen confirms the release of proplatelets exceeding platelet size into marrow sinusoids (23, 59–61). In most cases, proplatelets are released, but evidence of entire MKs leaving the bone marrow entering the circulation has been visualized, sometimes extruded from the BM attached to the released proplatelet extensions (19, 21, 22, 62, 63). The rare frequency of entire MKs in the lumen of the BM sinusoids (representing 1%–2% of all BM MKs) has been advanced to challenge the model whereby MKs emerge from the bone marrow and are trapped in the lung vasculature. MKcirc are indeed rare events (10 MK/ml), and in vivo, the majority of platelets released in the lung vasculature originate from large cytoplasmic fragments or proplatelets and ~15% are released from whole nucleated MKs (19). Considering that the lung vasculature carries the blood from the whole body, it is expected that the chance of observing entire MKs extruding from the BM will be lower than the observation of entire MKs releasing platelets in the lung vasculature. All bones are also not equal in their hematopoietic cell contents, and the sites of blood formation evolve with age, gradually shifting to the vertebrae, sternum, ribs, and pelvic bones. MK egress may occur preferentially in specific regions. The MKcirc could also come from other extrapulmonary sources such as the spleen, as maturation and large proplatelet extension have been observed in mouse and human (13, 19).
Mechanisms of MK/proplatelet egress
The production of platelets in the lung circulation is dependent on MKs and proplatelets exiting the bone marrow, but the mechanisms of egress need to be better understood. Thin proplatelets, thick proplatelets, or entire MKs can be released from the BM into the circulation, the last two being more likely to be trapped in the lung vasculature (60, 62, 64). If the thin proplatelets predominate in the physiological state, the large proplatelets, more immature, are more frequently observed in case of high platelet demand (acute thrombocytopenia, bleeding, inflammation). Other mechanisms besides proplatelet generation have been proposed such as MK rupture (60) and budding (21), even though these mechanisms were not observed by other groups (22), even in cases of acute platelet need. The mechanisms that have been recently proposed for regulating MK and proplatelet release are depicted in Figure 3. The first one involves the chemokine stromal cell-derived factor-1 (SDF-1/CXCL12), an important regulator of MK guidance to the sinusoidal vascular niche. In a mouse model of bleomycin-induced fibrosis, treatment with a specific inhibitor of CXCL12/CXCR4 axis was sufficient to prevent the migration of MKs to lung tissue ex vivo and in vivo (56). Confirming an important role of CXCL12/CXCR4 signaling in BM MK egress, another study showed that a subpopulation of MKs (CXCR4high MKs) leaves the BM after L. monocytogenes bacterial infection. Here, the infection decreased the expression of CXCL12 in bone marrow but increased its expression in the lung, liver, and spleen, suggesting that the different CXCL12 levels between tissues might drive the migration of CXCR4high MKs. In a sepsis model, however, where MKs are maturing in the spleen, inhibiting the CXCL12 pathway did not have a major role in MK egress from the BM. In that case, the release of MK progenitors from the BM was mediated by increased SCF (stem cell factor or kit-ligand) level in the blood and reduced SCF level in the BM. The decreased expression of SCF in LepR+ perivascular cells was regulated by the sympathetic nervous system (SNS) activity acting on β3-adrenergic receptors present on the perivascular cells (13). Another important pathway for transendothelial proplatelet migration and proplatelet shedding into the circulating blood includes the sphingosine-1 phosphate (S1P). S1P has been shown as an important regulator of proplatelet release. Higher concentrations of S1P are found in the blood compared with the bone marrow interstitium. S1P activates Sphingosine-1-phosphate receptor (S1PR) on MKs, guiding proplatelet elongation and shedding into the bloodstream (61). A gradient-independent mechanism has also been described where S1P release in the BM decreases thrombopoiesis and platelet release in the bloodstream (65).
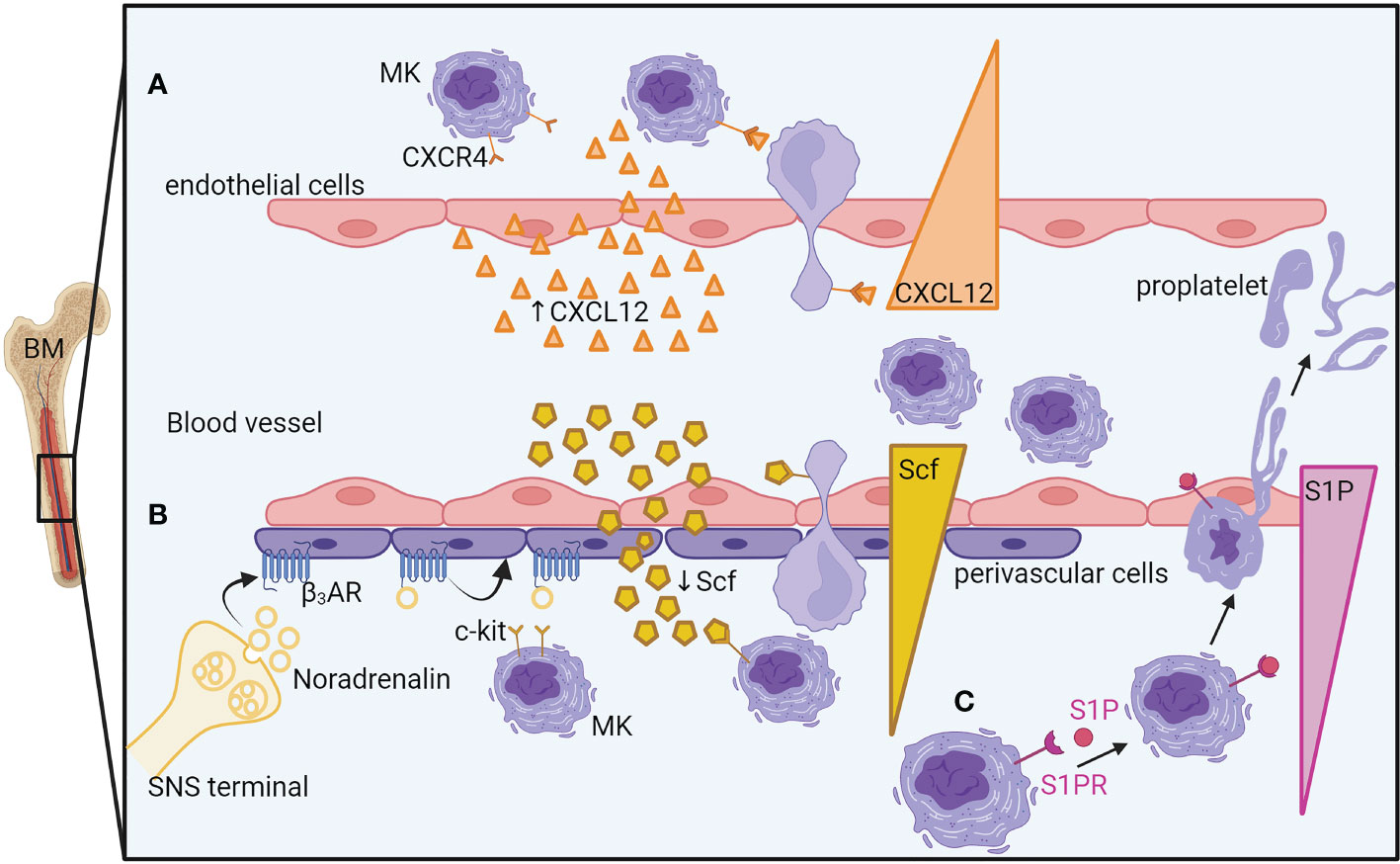
Figure 3 Mechanisms of megakaryocyte egress and proplatelet release from the bone marrow. (A) An increase of endothelial cell-derived CXCL12 in the blood guides the MK, thanks to its CXCL12 receptor, CXCR4, close to the sinusoidal vascular niche where it would deform itself to enter the vasculature. (B) During sepsis, the sympathetic nervous system releases noradrenaline, inducing the reduction of perivascular cell-produced SCF in the bone marrow. The SCF gradient between the bone marrow and the blood will guide the MKs to exit in the blood vessels. (C) A higher concentration of S1P in the blood than in the bone marrow guides MK elongation into the bloodstream by S1PR activation. BM, Bone marrow; MK, megakaryocyte; CXCR4, C-X-C chemokine receptor type 4; CXCL12/SDF1, C-X-C motif chemokine 12/ stromal cell-derived factor 1; SCF, Stem cell factor; c-kit/SCFR, Stem cell factor receptor; β3-AR, beta-3 adrenergic receptor; SNS, Sympathetic Nervous System; S1P, Sphingosine-1-phosphate; S1PR, S1P receptor.
MKcirc and emergency megakaryopoiesis
Single-cell analysis of circulating cells in COVID-19 has supported the hypothesis of a particular pathway of differentiation for MKcirc. The increased circulating MKs observed in COVID-19 are associated with a specific transcriptome and are enriched in genes associated with shortened MK differentiation process, inflammation, and viral sensing by Interferon (IFN)-stimulated genes (49, 66). Circulating and BM cells present altered hematopoiesis with MK-biased gene expression in the earliest CD34+CD38+ Hematopoietic stem and progenitor cells (HSPCs) and expanded MK progenitors in response to COVID-19, suggestive of inflammation-induced emergency megakaryopoiesis (67, 68). In Severe acute respiratory syndrome coronavirus 2 (SARS-CoV-2) infection, platelet consumption induced by the formation of microthrombi or by pulmonary inflammation occurs. Increased thrombopoiesis compensates for this platelet loss, resulting in a slight or no reduction in platelet count. High immature platelet fraction and increased mean platelet volume found in patients with COVID-19 support the accelerated rate of platelet turnover (69). These data from COVID-19 patients confirm the association between acute platelet need, increased thrombopoiesis, emergency megakaryopoiesis, and increased MKcirc. Increased MKcirc suggest a subsequent increase in lung platelet production even though dynamic visualization will be needed to observe if the MKcirc found in the lung vasculature in COVID-19 or during other inflammatory/infectious diseases are actually producing platelets or if the transit of MKcirc through the lung is perturbed, being trapped in the damaged/inflamed lung vasculature.
The lung vasculature—The ideal place for efficient platelet production?
Currently, in vitro platelet production systems failed to reproduce platelet biology and to achieve the yield obtained in the lung when MKs are injected into the circulation ex vivo (40) or in vivo (19, 24). The lung presents the largest capillary network, in which the hemodynamic forces (blood flow irregular fluctuation, shear stress), oxygenation, and interactions with the stroma could be ideal for platelet production (Figure 4).
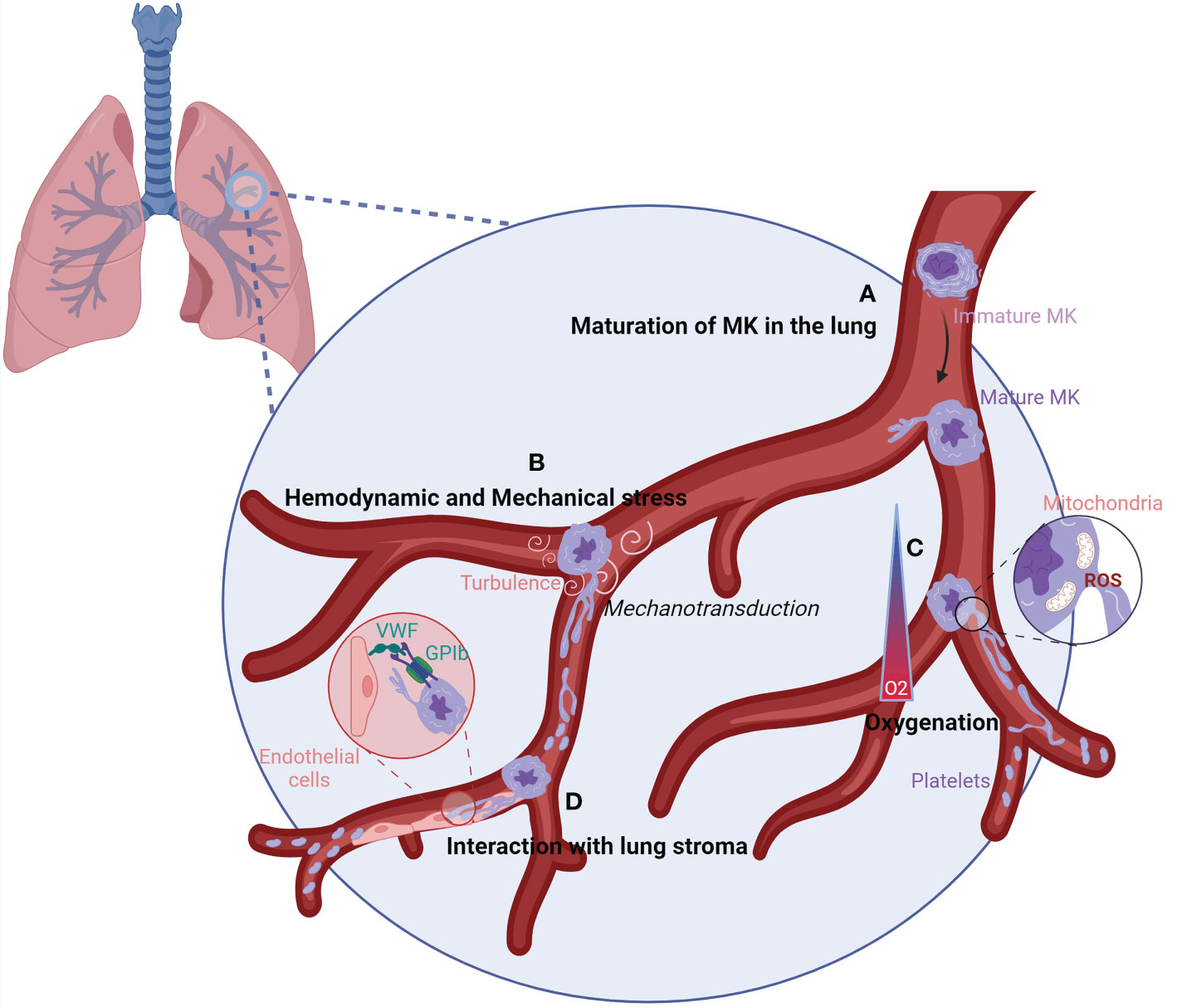
Figure 4 Characteristics of the lung vasculature that could favor efficient platelet production.(A) When MKs reach the lung vasculature, they can sense the lung microenvironment leading to their maturation into adaptated MKs. (B) The lung vasculature could harbor the perfect size and turbulence for MK elongation into platelets. Following sensing of mechanical stress and high turbulence by MKs, signals could be transmitted by mechanotransduction leading to proplatelet and platelet production. (C) The oxygenation of the lung vasculature is elevated, which could increase the mitochondrial fission and generation of mtROS at the MK membrane. ROS production induces the remodeling of the cytoskeleton, ending up in platelet production. (D) The interaction between vWF, enriched on lung endothelial cells, and GPIb on MKs could allow MKs to sense shear stress, resulting in the elongation of MKs and platelet formation. GP1b/CD42, Glycoprotein 1b/Cluster of Differenciation 42; MK, megakaryocyte; O2, Oxygen; ROS, Reactive Oxygen Species; vWF, von Willebrand factor.
Hemodynamic and mechanical stress
The large size of MKs naturally obstructs transit through the lung, which can have capillaries that are <5 µm in diameter. The size exclusion of MKs will induce mechanical stress. MKs are able to sense physical stress, and the activation of mechanotransduction pathways promotes activation and maturation of MKs, reorganization of the cytoskeleton, and generation of proplatelets (70–72). Increasing in vitro (73, 74) and in vivo evidence (75) suggests that blood flow-dependent shear stress and turbulence are crucial for platelet biogenesis. In vitro, final platelet release is best reproduced at high shear rates, superior to the shear rate at the sinusoid wall in the bone marrow. By coupling skull intravital imaging with particle image velocimetry, Ito et al. showed that MKs displaying proplatelet protrusion and platelet release were adjacent to areas of high vascular turbulence. In contrast, resting MKs that did not release platelets were exposed to continuous laminar flow with no turbulence. Pulmonary vessels, the first capillary bed encountered by MKs and proplatelets leaving the bone marrow, may provide an optimal level of turbulence and shear stress required for efficient platelet generation. Corroborating this hypothesis, murine and human MKs grown in vitro and injected intravenously are trapped in mouse lungs and shed platelets with normal size distribution, circulating half-life, and functionality (24, 25).
Oxygenation
The lung vasculature harbors a high level of oxygen compared to the bone marrow, which has recently been revealed as an important parameter for initiating proplatelet elongation in mature MKs. Ex vivo, MKs infused in the lung vasculature in the absence of ventilation or in the absence of oxygen have a reduced or abolished ability to produce platelets in the lung (40). In vitro, MKs exposed to increasing O2 levels strongly amplified thrombopoiesis by increasing the formation of proplatelets. High oxygen levels change intracellular reactive oxygen species (ROS) levels, resulting in enhanced mitochondrial fission, which in turn generates more mitochondrial ROS. ROS could contribute to the initiation of thrombopoiesis by the remodeling of the cytoskeleton for proplatelet and platelet release (76). Whether changes in oxygen concentration in the lung vasculature trigger a similar ROS-dependent mitochondrial fission and mitochondrial reactive oxygen species (mtROS) production remains to be studied in vivo.
Interaction with lung stroma
Finally, the pulmonary circulation may also provide additional signals promoting platelet generation through specific lung endothelial cell–megakaryocyte interactions. Among the possibilities, Von Willebrand factor (vWF) on endothelial cell interaction with Glycoprotein 1b (GP1b) on MKs could be a mechanism for MKs to sense shear (77) and is an important regulator of proplatelet formation (78). vWF expression in endothelial cells is heterogeneous throughout the vascular tree, and its high level in lung endothelial cells could be another reason for efficient platelet production in the lung (79, 80). Altogether, the combinations of these important elements present in the lung vasculature may further increase platelet production, as suggested by in vitro experiments using microfluidic devices, where hydrodynamic shear and vWF anchoring improve proplatelet elongation and platelet production (74, 81). Nonetheless, platelet count is normal in mice lacking vWF, and it is reasonable to think that other pathways are involved in the interaction of MKs with the lung stroma. More work is needed to define them and understand their importance in platelet production.
The lung environment modulates megakaryocytes and platelet functions
Role of platelets in immune response and lung development and repair
It is becoming increasingly clear that platelets are involved in inflammation, infection, host response, and tissue development and regeneration by direct and indirect mechanisms. They can capture and engulf pathogens, but they also express adhesive molecules and many pro-inflammatory molecules (chemokines, cytokines, immune receptors, growth factor), interacting with various cells of the innate and adaptive immune system and initiating and modulating immune responses and tissue development and regeneration, including the lung (82–84). These functions have been previously reviewed (85–87). One would thus expect that patients with inherited (primary) or acquired (secondary) thrombocytopenia will be more prone to infection or to impaired tissue healing. These defects are not largely reported, and thrombocytopenic mice deficient for TPO receptor do not have apparent lung deficiencies. It is possible that studies would require continuous evaluation of the occurrence of infection or tissue damage, occasional events that may be hidden by the major defects in hemostasis. Another reason may be that as we observed in thrombocytopenic mouse models, only platelet counts severely decreased and combined with other specific defects in platelet activation, such as the absence of C-type lectin receptor 2 (CLEC-2) signaling, are associated with lung developmental defects (83). Platelets involved in immunity or repair may come from nonclassical pathways of megakaryopoiesis and may not be affected by impaired classical pathways such as TPO signaling. Some studies do provide clinical relevance of the role of platelets in infection and lung development. Infections are for instance common in patients with primary immune thrombocytopenia (ITP). A systemic review analyzing 13 studies show that adult patients with primary ITP have an increased risk of infection, from two to six times higher compared to the general population, and pulmonary infection is consistently reported as the primary site of infection (88, 89). If immunosuppressive therapies can explain this increased risk of infection, retrospective studies suggest that it is not the only reason, as patients with primary ITP had an increased risk of infection even before their diagnosis (90). Moreover, in another study, low platelet count is independently correlated with an increased risk of infection, even when adjusted for leukocyte count (91). In other contexts, a low platelet count is an independent risk factor of postoperative pneumonia in patients with acute aortic dissection (92), and during sepsis, admission thrombocytopenia is associated with enhanced mortality and a more disturbed host response independently of disease severity (93). A low platelet count is also observed and a risk factor for the development of bronchopulmonary dysplasia (BPD) in premature infants, characterized by the arrest of lung development, a decrease in the alveolar number, an increase in alveolar volume, simplification of the alveolar structure, and abnormal pulmonary microvascular morphology (94, 95). In the Hermansky–Pudlack syndrome, a genetic disorder with platelet dysfunction and dense granule defect, a high frequency of related pulmonary fibrosis is observed.
MK heterogeneity—Immune MK populations
MKL have a transcriptional profile suggesting a role in immunity and inflammation. Recent single-cell analyses revealed the existence of different MK populations exhibiting transcriptional heterogeneity in both humans and mice, suggesting specialized functions (96–98). These data suggest that the various functions of MKs are not fulfilled by the entire MK population as a whole but rather by distinct MK subpopulations with dedicated roles. Particularly, a population of MKs with lower ploidy and putative immune functions, resembling MKL, has also been identified in the bone marrow, liver, and spleen (13, 53, 96). Interestingly, reports suggest that distinct MK subpopulations are generated along distinct developmental routes, immune MKs being associated with the “emergency” route (49, 68, 98) (Figure 1). Alignment of different sets of MK Single cell (sc)-RNA-Seq data showed that subpopulations of BM MKs shared transcriptional similarity with MKL gene profiles. After L. monocytogenes infection, one of the subpopulations achieved even greater similarity with MKL than otherwise (53).
MK plasticity and importance of the environment
Pariser et al. (20) have demonstrated that the immune phenotype of MKs is plastic and driven by the tissue immune environment, as evidenced by BM MKs having an MKL-like phenotype when adoptively transferred to the lung or under the influence of danger signals such as pathogen receptor challenge or Interleukin 33 (IL-33), a lung-associated danger signal, released upon cell damage. The important role of the lung microenvironment in MKL development and specificity is also demonstrated by Yeung et al. (34) who showed that fetal MKL still develop an immune phenotype, suggesting that the MKL microenvironment before interactions with foreign antigens is able to promote the immune phenotype. The microenvironment is also important for MK final size and ploidy, as neonatal cells, usually smaller and with lower ploidy, are capable of producing adult‐sized MKs when placed in an adult microenvironment (99). If the lung niche supports the specialization of pulmonary MKs, one question remains: What is the dedicated role of this distinct population of immune MKs found in the lung?
Role of the immune-MK population
The differential expressions of immune-regulatory genes in MKL show a potential role in pathogen recognition and response, chemotaxis, myeloid cell activation, and response to type I interferon. These specific immune functions can be fulfilled by the MK itself, acting locally and independently of platelet production or transferred to its progeny and disseminated in the whole body through circulating platelets. MK immune potential has been previously reviewed (100), and we will focus here on immune roles validated by functional studies with MKL, spleen immune MK, or immune MK from BM sharing transcriptional identity with MKL, depicted in Figure 5.
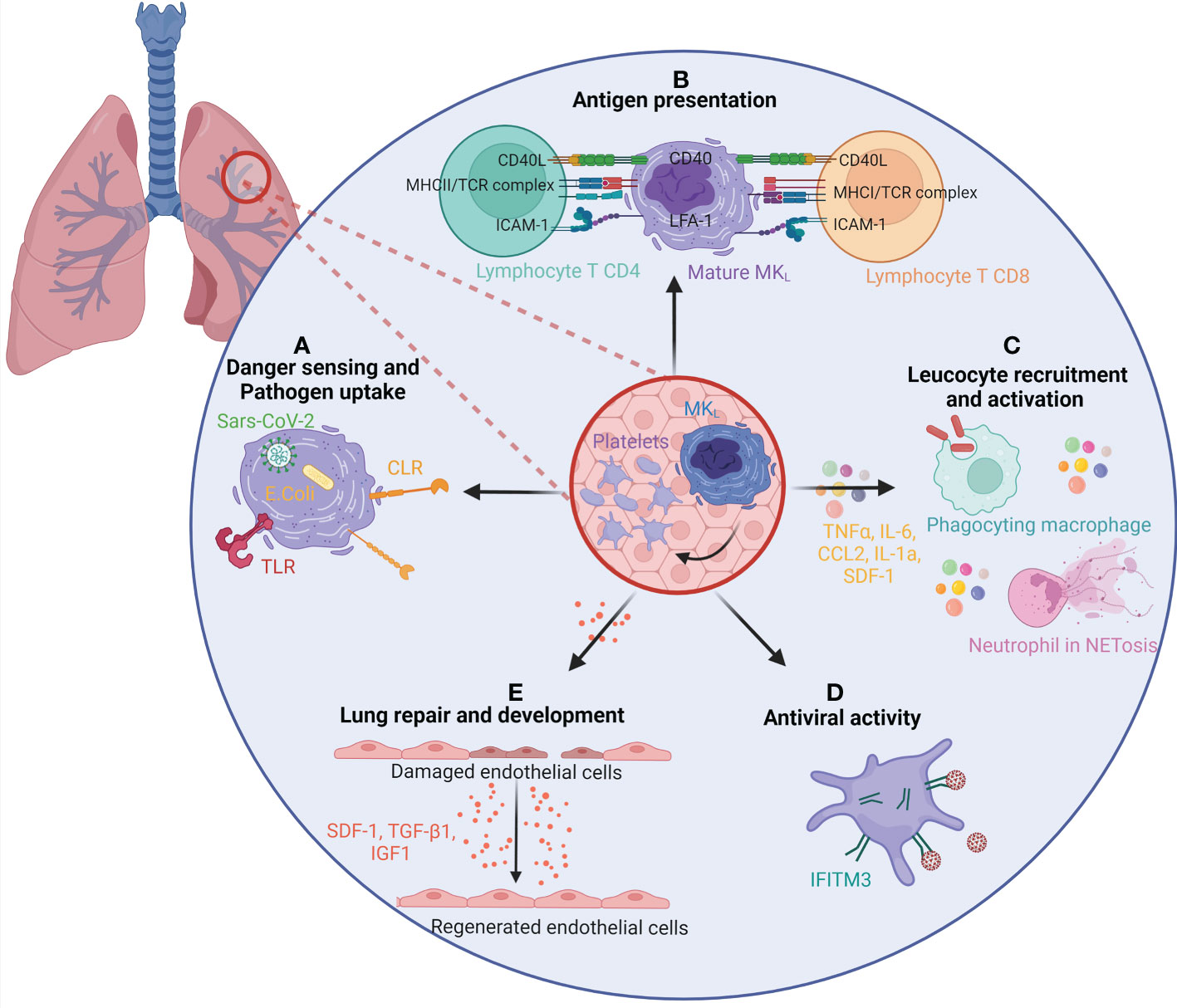
Figure 5 The role of lung megakaryocytes and platelets in immunity and repair. (A) MKL and/or platelets present in the lung are able to sense and uptake pathogen through many different pathways. Pathogen-associated molecular patterns, such as Toll-like receptors (TLRs) or C-type lectin receptors (CLRs), present on MKL surface can recognize the pathogens and uptake them. MKL can also recognize internalized pathogens in their cytoplasm, such as SARS-CoV-2 virus or E coli. (B) MKL and/or platelets can act as antigen-presenting cells for T CD8 and T CD4 lymphocytes. They uptake pathogens and antigens and present them on MHC class I and class II molecules. (C) The secretion of inflammatory molecules, such as TNFα, IL-6, CCL2, IL-1α, and SDF-1 confers an immune regulatory role to MKs and/or platelets. It enhances the migration and activation of leukocytes such as macrophages and neutrophils (bacterial uptake, NETosis). (D) The expression of interferon-stimulated genes, and particularly ifitm3, gives MKL and platelets an antiviral activity. (E) MKL and/or platelets participate in lung development and regeneration by the production of SDF-1, TGF-β1, and IGF1. CCL, chemokine (C-C motif) ligand; CD, Cluster of differentiation; IFITM3, Interferon-induced transmembrane protein 3; IGF1, Insulin-like growth factor 1; IL, Interleukin; MHC, Major Histocompatibility complex; MKL, Lung resident megakaryocytes; NET, Neutrophil Extracellular Traps; SDF-1/CXCL12, stromal cell-derived factor 1/ C-X-C motif chemokine 12; TCR, T cell receptor; TGF-β1, Transforming growth factor beta 1; TNF, Tumor Necrosis Factor.
Sensing danger/pathogen uptake
Compared to BM MK, MKL express higher levels of pattern recognition receptor (Toll-like receptors (TLRs), C-type lectin receptors (CLRs)). They possess the ability to phagocytose or uptake bacteria Escherichia coli in vitro (20) and virus SARS-CoV-2 in vivo (49). Although MKL and BM MKs both phagocytosed E. coli, MKL internalized and digested more bacteria into phagolysosome. Interestingly, the virus is found in platelets from severe COVID-19 patients in phagolysosome-like structures suggesting virophagy (101), but platelets cannot be directly infected by the virus. In the case of SARS-CoV-2 infection, the virus, uptaken by the MK, may be transferred to its daughter platelets, participating in its dissemination (49).
Antigen processing and presentation
MKs can act as potent antigen-presenting cells to modulate T-cell responses in vivo by processing and presenting endogenous and exogenous antigens on major histocompatibility complex (MHC) class I molecules. Compared to BM MKs, MKL express molecules like MHC class II (MHC-II) that are similar to tissue-resident leukocytes and Antigen Presenting Cell (APCs). Pariser et al. demonstrated in vitro and in vivo that MKL internalized and processed both antigenic proteins and bacterial pathogens. Furthermore, MKL induced CD4+ T-cell activation in an MHC II–dependent manner (20). In a similar manner, CXCR4high MKs, an immune subpopulation of BM MKs resembling MKL, are able to present the ovalbumin antigens on their surface via MHC-I, activating CD8+ T cells (53). In vitro MKs have the ability to transfer MHC-I molecules loaded with foreign antigen to proplatelets (102). A similar immune MK population, found in the circulation and in the spleen, was actually shown to be the main APC in lupus responsible for TH17 polarization via MHC-II lupus antigen presentation and costimulatory molecule CD86 (103).
Immune/inflammatory molecule secretion and immune cell migration and activation
Compared to their BM counterpart, MKL also express higher levels of inflammatory molecules such as chemokines, cytokines, or inflammatory lipids. At the protein level and in functional studies, lung MKs produced higher levels of inflammatory molecules [chemokine (C-X-C motif) ligand 1 (CXCL-1), Soluble Intercellular Adhesion Molecule 1 (sICAM-1), IL-1α, SDF-1, Macrophage Inflammatory Protein 3 (MIP-3), IL1RA, Tumor necrosis factor alpha (TNF-α), chemokine (C-C motif) ligand 2 (CCL-2)] after Lipopolysaccharide (LPS) stimulation compared with BM MKs (20). Wang et al. (53) showed that the immune MK subpopulation CXCR4high, but not CXCR4low MKs, efficiently enhanced neutrophil and macrophage migration and bacterial phagocytosis in part through higher levels of TNF-α and IL-6 secretion. Another example of specific immune molecule expression is the generation of CD40Lhi platelets by MKs differentiated in the spleen of a mouse model of sepsis that has been involved in promoting the release of Neutrophil Extracellular Traps (NETs) from neutrophils, enabling efficient bacterial containment (13).
Antiviral
Response to viruses is among the ontology biological processes and signaling pathways enriched in MKL. Platelets have been involved in viral infection as reviewed previously (104). Among the genes significantly enriched, ifitm3 is encountered in the three mouse MKL studies and in circulating MKs during SARS-CoV-2 infection, as well as in platelets from infected patients (105). IFITM3 is an antiviral protein inhibiting viral cell membrane fusion and viral entry. Platelets and MKs upregulate IFITM3 (transcript and/or protein) upon infection in human (dengue, influenza, and SARS-CoV-2) and in vitro; overexpression of IFITM3 in MKs was sufficient to prevent dengue infection (106).
Lung development and repair
Another biological process enriched in MKL is related to lung development and repair. MKL and more particularly fetal MKL are enriched in several growth factors and molecules critical in pulmonary alveolarization and development (Tgfb1, fgf, sdf1, igf1, clec1b). Fetal lung MKs may be a local source of growth factors that support the development of the embryonic lung (34). Platelet-derived CLEC2, encoded by clec1b, is required to separate blood/lymphatics in the developing lung interacting with lymphatic endothelial cells. Transforming growth factor beta (TGF‐β) production then drives the differentiation of myofibroblasts that are critical to primary septum formation and elastogenesis in alveolarization of the lung (83). Platelet-derived SDF-1 has been involved in lung regeneration after mouse lung pneumonectomy. SDF-1 stimulates the expression of SDF-1 receptors on pulmonary capillary endothelial cells, enhancing the proliferation of alveolar epithelial cells and lung regeneration (84). In adult lung, an excessive tissue repair response after injury can lead to fibrosis. In a mouse model of bleomycin-induced lung damage and fibrosis, pulmonary MKs have been involved in the proliferation of primary lung fibroblasts and transdifferentiation to myofibroblasts partially through the TGF-β1 pathway (56).
Origin of resident megakaryocytes
One important remaining question is the ontogeny of MKL. When do they seed the lung and where are they coming from? Lung HSCs and hematopoietic progenitors (17, 19) have been identified in the adult lung. These cells migrate to the bone marrow and are able to reconstitute normal platelet count in thrombocytopenic mice. It is currently unknown if these progenitor cells can locally differentiate into MKL. MKs are less abundant but present in fetal lung before birth at embryonic day 13 in mouse, which is when the majority of murine hematopoietic development is occurring in the liver (34). They were also detected by Pariser et al. (20) in neonatal lung at birth (P0). Immune phenotypes of MKL (MHC-II and ICAM1) were each expressed at much higher levels in adult MKL compared with P0 mouse MKL, suggesting that the MK immune phenotype may be regulated by the postnatal environment. MKL thus seed the lung before birth where they will acquire their specialization in contact to the lung environment. Fetal and neonatal megakaryopoiesis is characterized by rapid proliferation followed by full cytoplasmic maturation without polyploidization (107). Fetal MKs are smaller and with lower ploidy than adult MKs, but they are cytoplasmically mature, and they present characteristics of adult polyploid MKs, including the presence of granules, a well-developed demarcation membrane system, and proplatelet formation abilities (108). Thus, rather than immaturity, it has been suggested that fetal/neonatal megakaryopoiesis reflects a developmentally unique uncoupling of proliferation, polyploidization, and cytoplasmic maturation (107). This mechanism may allow fetuses and neonates to populate their rapidly expanding BM space and blood volume in this period of very rapid growth while maintaining normal platelet counts (109). Considering the shared properties between primitive MKs and MKL (mainly diploid, bypass of classical progenitors, specialized functions in lung development or immunity), it is tempting to speculate that, similarly to tissue-resident macrophages, MKL could differentiate from embryonic yolk sac-derived EMPs or MEMPs identified to colonize the organs during gestation (12, 110–112) (Figure 1). Clearly, questions remain to be fully addressed to determine the ontogeny, origin, and differentiation of MKL.
MK identity and methodology advices for further studies
Tool box for MK identification
The different subpopulations of MKs with specialized functions raise questions about MK identity and how to define an MK. The transcriptome profile of immune MKs has similarities with other myeloid cells. Are these cells really MKs with immune cell markers or could they be immune cells with MK markers or multipotential progenitors? Different techniques may be required to ensure the MK identity and are presented in Table 2. If the expression of specific markers such as CD41 is key in MK identification, it is important to make sure that platelets aggregated with leukocytes or other cell types are excluded. Platelets associate easily with other cells and will contaminate flow cytometry or RNA-Seq analysis of MKs identified or sorted as CD41+ cells. Results from analysis without attempt to exclude these aggregates should be interpreted with care. In mouse studies, the use of reporter mice expressing a nuclear-localized fluorescent protein can help in discriminating MKs from cells aggregated with platelets. The use of imaging flow cytometry is another way to differentiate MKs from leukocyte–platelet aggregates (114). It also adds morphological information using the shape, density, texture, and coincidence of fluorescence to discriminate different MK maturation steps (115). Morphology is an important parameter of MKs that can be obtained by immunohistology or immunofluorescence. Electron microscopy can be used to evaluate the identity and maturation status of MKs, the platelet-producing MKs usually presenting a large nucleus with a well-developed cytoplasm displaying demarcation membranes and numerous alpha-granules (113), as it has been shown for MKs retrieved from human pulmonary arteries (81). The ability of MKs to produce platelets is the final proof of MK identity and can be studied in vitro using flow chamber devices to mimic a more natural environment, but the dynamics of platelet formation can only be investigated since the development of two-photon microscopy (116). Reporter mice have been developed to visualize MKs in vivo, and their advantages and drawbacks are reported in Table 3 (118–121). Regarding the ability of extravascular MKL to produce platelets, they have been observed by intravital imaging, and these cells are sessile and have not been observed in vivo to release proplatelets/platelets during homeostasis (19). In vitro, Pariser et al. brought the evidence that isolated lung MKs were able to produce platelets after 3 days of culture. Wang et al. (53) also demonstrate the ability of an immune MK subpopulation identified in the BM (CXCR4high MKs) to produce platelets, although it has relatively lower polyploidy, smaller cell size, and generated platelets in lower efficiencies compared to CXCR4low MKs, suggesting that CXCR4high MKs might be specialized for immune functions. The ability of MKL to mature and to produce platelets in vivo during acute platelet need, inflammation, or infection remains however to be demonstrated.
Place and time of blood sampling for MKcirc quantification
The frequency of MKs in blood appears to depend upon the site in the body from which the blood is drawn. For example, blood from the inferior vena cava contains more MKs than the blood from the veins of the forearm (37). The cava drains an area rich in BM (pelvic girdle and spine), whereas the antecubital vein does not (bones of the forearm and hand). Moreover, with increasing age, bone marrow at several sites is replaced by adipose tissue. The primary sites of blood formation therefore gradually shift to the vertebrae, sternum, ribs, and pelvic bones. The number of circulating MKs in cubital venous blood declines during childhood: from 20 MK/ml in the first year of life to 5.5 MK/ml after the sixth year, reflecting the decrease in thrombopoiesis in the bone marrow of the fingers, hands, and forearms (117). Blood is almost always taken from antecubital veins and may be the reason why MKs are rarely observed in blood in normal conditions. The time of blood sampling may also be of importance. Traffic of hematopoietic cells and egress from the bone marrow are also impacted by biological rhythms. One study report that circulating MKs were found more frequently in the morning between 8:00 a.m. and 2:00 p.m (42). Circadian fluctuation was observed in platelet count and function (122), but more work is needed to understand if circulating MKs and platelet production in the lung are subjected to circadian oscillations.
Concluding remarks
Research in recent years suggests that MKs and platelet production are found in various locations within the body, challenging historical models. Among extramedullary organs, the lung vasculature, where circulating MKs are trapped, has been proposed as an ideal site for the final step of platelet release, a phenomenon further increased when platelets are rapidly consumed such as in infectious disease, lung inflammation, excessive coagulation, or tissue damage. However, many unknowns remain regarding the signals that trigger MK release from the marrow in steady state and during emergency thrombopoiesis. It is also unknown whether the lung endothelium or its microenvironment locally promotes or modulates platelet production. MK subpopulations, including MKL, have been described and exhibit specialized functions in immune responses, stem cell maintenance, and tissue development and regeneration. Whether MKs in the pulmonary microcirculation and MKL have other functions beyond their roles in the release of platelets is an emerging area of research. Challenging this cell identity crisis by ensuring quality control and multiple functional tests in vitro and in vivo is essential for future investigations. Finally, more studies are required to characterize the heterogeneity, plasticity, mobility, and roles of MKs according to their site of origin and development under basal conditions and disease.
Author contributions
LG and LF designed the figures and EL wrote the manuscript. All authors contributed to the article and approved the submitted version.
Funding
EL is supported by the Fonds de Recherche en Santé Respiratoire - Fondation du Souffle, the French National Research Agency (ANR JCJC), LG is supported by a PhD fellowship from the National Research Agency and LF is supported by a PhD fellowship from the Ministry of Research. Fondation du Souffle (Grant #198942) ANR JCJC (Grant #ANR-20-CE14-0045-01).
Acknowledgments
Figures were created with Biorender.com. We thank Jean-Philippe Girard for proofreading of the manuscript.
Conflict of interest
The authors declare that the research was conducted in the absence of any commercial or financial relationships that could be construed as a potential conflict of interest.
Publisher’s note
All claims expressed in this article are solely those of the authors and do not necessarily represent those of their affiliated organizations, or those of the publisher, the editors and the reviewers. Any product that may be evaluated in this article, or claim that may be made by its manufacturer, is not guaranteed or endorsed by the publisher.
References
1. Howell WH, Donahue DD. The production of blood platelets in the lungs. J Exp Med (1937) 65(2):177–203. doi: 10.1084/jem.65.2.177
2. Grover A, Sanjuan-Pla A, Thongjuea S, Carrelha J, Giustacchini A, Gambardella A, et al. Single-cell RNA sequencing reveals molecular and functional platelet bias of aged haematopoietic stem cells. Nat Commun (2016) 7:11075. doi: 10.1038/ncomms11075
3. Psaila B, Wang G, Rodriguez-Meira A, Li R, Heuston E F, Murphy L, et al. Single-cell analyses reveal megakaryocyte-biased hematopoiesis in myelofibrosis and identify mutant clone-specific targets. Mol Cell (2020) 78(3):477–492 e8. doi: 10.1016/j.molcel.2020.04.008
4. Sanjuan-Pla A, Macaulay I C, Jensen CT, Woll PS, Luis TC, Mead A, et al. Platelet-biased stem cells reside at the apex of the haematopoietic stem-cell hierarchy. Nature (2013) 502(7470):232–6. doi: 10.1038/nature12495
5. Giannini S, Lee-Sundlov MM, Rivadeneyra L, Di Buduo CA, Burns R, Lau JT, et al. beta4GALT1 controls beta1 integrin function to govern thrombopoiesis and hematopoietic stem cell homeostasis. Nat Commun (2020) 11(1):356. doi: 10.1038/s41467-019-14178-y
6. Rommel M, Walz L, Fotopoulou F, Kohlsheen S, Schenk S, Miskey C, et al. Influenza a virus infection instructs hematopoiesis to megakaryocyte-lineage output. Cell Rep (2022) 41(1):111447. doi: 10.1016/j.celrep.2022.111447
7. Noetzli LJ, French SL, Machlus KR. New insights into the differentiation of megakaryocytes from hematopoietic progenitors. Arteriosclerosis thrombosis Vasc Biol (2019) 39(7):1288–300. doi: 10.1161/ATVBAHA.119.312129
8. Haas S, Hansson J, Klimmeck D, Loeffler D, Velten L, Uckelmann H, et al. Inflammation-induced emergency megakaryopoiesis driven by hematopoietic stem cell-like megakaryocyte progenitors. Cell Stem Cell (2015) 17(4):422–34. doi: 10.1016/j.stem.2015.07.007
9. Kristiansen TA, Zhang Q, Vergani S, Boldrin E, Krausse N, Andre O, et al. Developmental cues license megakaryocyte priming in murine hematopoietic stem cells. Blood Adv (2022) 18:bloodadvances.2021006861. doi: 10.1182/bloodadvances.2021006861
10. Iturri L, Freyer Biton L. A, Dardenne P, Lallemand Y, Gomez Perdiguero E. Megakaryocyte production is sustained by direct differentiation from erythromyeloid progenitors in the yolk sac until midgestation. Immunity (2021) 54(7):1433–1446 e5. doi: 10.1016/j.immuni.2021.04.026
11. Tober J, McGrath KE, Palis J. Primitive erythropoiesis and megakaryopoiesis in the yolk sac are independent of c-myb. Blood (2008) 111(5):2636–9. doi: 10.1182/blood-2007-11-124685
12. Popescu DM, Botting R. A, Stephenson E, Green K, Webb S, Jardine L, et al. Decoding human fetal liver haematopoiesis. Nature (2019) 574(7778):365–71. doi: 10.1038/s41586-019-1652-y
13. Valet C, Magnen M, Qiu L, Cleary S. J, Wang K. M, Ranucci S, et al. Sepsis promotes splenic production of a protective platelet pool with high CD40 ligand expression. J Clin Invest (2022) 132(7):e153920. doi: 10.1172/JCI153920
14. Huang Z, Richmond TD, Muntean AG, Barber DL, Weiss MJ, Crispino JD, et al. STAT1 promotes megakaryopoiesis downstream of GATA-1 in mice. J Clin Invest (2007) 117(12):3890–9. doi: 10.1172/JCI33010
15. Slayton WB, Georgelas A, Pierce LJ, Elenitoba-Johnson KS, Perry SS, Marx M, et al. The spleen is a major site of megakaryopoiesis following transplantation of murine hematopoietic stem cells. Blood (2002) 100(12):3975–82. doi: 10.1182/blood-2002-02-0490
16. Zingariello M, Rosti V, Vannucchi AM, Guglielmelli P, Mazzarini M, Barosi G, et al. Shared and distinctive ultrastructural abnormalities expressed by megakaryocytes in bone marrow and spleen from patients with myelofibrosis. Front Oncol (2020) 10:584541. doi: 10.3389/fonc.2020.584541
17. Hillel-Karniel C, Rosen C, Milman-Krentsis I, Orgad R, Bachar-Lustig E, Shezen E, et al. Multi-lineage lung regeneration by stem cell transplantation across major genetic barriers. Cell Rep (2020) 30(3):807–819 e4. doi: 10.1016/j.celrep.2019.12.058
18. Summer R, Kotton DN, Liang S, Fitzsimmons K, Sun X, Fine A, et al. Embryonic lung side population cells are hematopoietic and vascular precursors. Am J Respir Cell Mol Biol (2005) 33(1):32–40. doi: 10.1165/rcmb.2005-0024OC
19. Lefrancais E, Ortiz-Munoz G, Caudrillier A, Mallavia B, Liu F, Sayah DM, et al. The lung is a site of platelet biogenesis and a reservoir for haematopoietic progenitors. Nature (2017) 544(7648):105–9. doi: 10.1038/nature21706
20. Pariser DN, Hilt ZT, Ture SK, Blick-Nitko SK, Looney MR, Cleary SJ, et al. Lung megakaryocytes are immune modulatory cells. J Clin Invest (2021) 131(1):e137377. doi: 10.1172/JCI137377
21. Potts KS, Farley A, Dawson CA, Rimes J, Biben C, de Graaf C, et al. Membrane budding is a major mechanism of in vivo platelet biogenesis. J Exp Med (2020) 217(9):e20191206. doi: 10.1084/jem.20191206
22. Stegner D, vanEeuwijk JMM, Angay O, Gorelashvili MG, Semeniak D, Pinnecker J, et al. Thrombopoiesis is spatially regulated by the bone marrow vasculature. Nat Commun (2017) 8(1):127. doi: 10.1038/s41467-017-00201-7
23. Eckly A, Scandola C, Oprescu A, Michel D, Rinckel JY, Proamer F, et al. Megakaryocytes use in vivo podosome-like structures working collectively to penetrate the endothelial barrier of bone marrow sinusoids. J Thromb haemostasis JTH (2020) 18(11):2987–3001. doi: 10.1111/jth.15024
24. Fuentes R, Wang Y, Hirsch J, Wang C, Rauova L, Worthen GS, et al. Infusion of mature megakaryocytes into mice yields functional platelets. J Clin Invest (2010) 120(11):3917–22. doi: 10.1172/JCI43326
25. Wang Y, Hayes V, Jarocha D, Sim X, Harper DC, Funtes R, et al. Comparative analysis of human ex vivo-generated platelets vs megakaryocyte-generated platelets in mice: a cautionary tale. Blood (2015) 125(23):3627–36. doi: 10.1182/blood-2014-08-593053
26. Sharma GK, Talbot IC. Pulmonary megakaryocytes: "missing link" between cardiovascular and respiratory disease? J Clin Pathol (1986) 39(9):969–76. doi: 10.1136/jcp.39.9.969
27. Zucker-Franklin D, Philipp CS. Platelet production in the pulmonary capillary bed: new ultrastructural evidence for an old concept. Am J Pathol (2000) 157(1):69–74. doi: 10.1016/S0002-9440(10)64518-X
28. Balko J, Havlin J, CasasMendez F, Zajacova A, Koblizek M, Svorcova M, et al. Mapping of the lung megakaryocytes: A role in pathogenesis of idiopathic pulmonary arterial hypertension? Pathology Res Pract (2022) 237:154060. doi: 10.1016/j.prp.2022.154060
29. Aabo K, Hansen KB. Megakaryocytes in pulmonary blood vessels. i. incidence at autopsy, clinicopathological relations especially to disseminated intravascular coagulation. Acta pathologica microbiologica Scandinavica. Section A Pathol (1978) 86(4):285–91. doi: 10.1111/j.1699-0463.1978.tb02045.x
30. Balko R, Edriss H, Nugent K, Test V, Zhu A. Pulmonary veno-occlusive disease: An important consideration in patients with pulmonary hypertension. Respir Med (2017) 132:203–9. doi: 10.1016/j.rmed.2017.10.015
31. Looney MR, et al. Stabilized imaging of immune surveillance in the mouse lung. Nat Methods (2011) 8(1):91–6. doi: 10.1038/nmeth.1543
32. Cleary SJ, Hobbs C, Amison RT, Arnold S, O'Shaughnessy BG, Lefrancais E, et al. LPS-induced lung platelet recruitment occurs independently from neutrophils, PSGL-1, and p-selectin. Am J Respir Cell Mol Biol (2019) 61(2):232–43. doi: 10.1165/rcmb.2018-0182OC
33. Davis RE, Stenberg PE, Levin J, Beckstead JH. Localization of megakaryocytes in normal mice and following administration of platelet antiserum, 5-fluorouracil, or radiostrontium: evidence for the site of platelet production. Exp Hematol (1997) 25(7):638–48.
34. Yeung AK, Villacorta-Martin C, Hon S, Rock JR, Murphy GJ. Lung megakaryocytes display distinct transcriptional and phenotypic properties. Blood Adv (2020) 4(24):6204–17. doi: 10.1182/bloodadvances.2020002843
35. Kaufman RM, Airo RM, Pollack R, Crosby S, WH. Circulating megakaryocytes and platelet release in the lung. Blood (1965) 26(6):720–31. doi: 10.1182/blood.V26.6.720.720
36. Levine RF, Eldor A, Shoff PK, Kirwin S, Tenza D, Cramer EM, et al. Circulating megakaryocytes: delivery of large numbers of intact, mature megakaryocytes to the lungs. Eur J haematology (1993) 51(4):233–46. doi: 10.1111/j.1600-0609.1993.tb00637.x
37. Pedersen NT. Occurrence of megakaryocytes in various vessels and their retention in the pulmonary capillaries in man. Scandinavian J haematology (1978) 21(5):369–75. doi: 10.1111/j.1600-0609.1978.tb00381.x
38. Lefrancais E, Looney MR. Platelet biogenesis in the lung circulation. Physiology (2019) 34(6):392–401. doi: 10.1152/physiol.00017.2019
39. Wilde NT, Burgess R, Keenan DJ, Lucas GS, et al. The effect of cardiopulmonary bypass on circulating megakaryocytes. Br J haematology (1997) 98(2):322–7. doi: 10.1046/j.1365-2141.1997.2373055.x
40. Zhao. Platelet generation from circulating megakaryocytes is triggered in the lung vasculature. BioRXiv (2021) bioRxiv 2021.11.01.466743. doi: 10.21203/rs.3.rs-690639/v1
41. Wells S, Sissons M, Hasleton PS. Quantitation of pulmonary megakaryocytes and fibrin thrombi in patients dying from burns. Histopathology (1984) 8(3):517–27. doi: 10.1111/j.1365-2559.1984.tb02361.x
42. Hume R, West JT, Malmgren RA, Chu EA. Quantitative observations of circulating megakaryocytes in the blood of patients with cancer. New Engl J Med (1964) 270:111–7. doi: 10.1056/NEJM196401162700301
43. Soares FA. Increased numbers of pulmonary megakaryocytes in patients with arterial pulmonary tumour embolism and with lung metastases seen at necropsy. J Clin Pathol (1992) 45(2):140–2. doi: 10.1136/jcp.45.2.140
44. Mandal RV, Mark EJ, Kradin RL. Megakaryocytes and platelet homeostasis in diffuse alveolar damage. Exp Mol Pathol (2007) 83(3):327–31. doi: 10.1016/j.yexmp.2007.08.005
45. Slater D, Martin J, Trowbridge A. The platelet in asthma. Lancet (1985) 1(8420):110. doi: 10.1016/S0140-6736(85)92002-1
46. Valdivia-Mazeyra MF, Salas C, Nieves-Alonso JM, Martin-Fragueiro L, Barcena C, Munoz-Hernandez P, et al. Increased number of pulmonary megakaryocytes in COVID-19 patients with diffuse alveolar damage: an autopsy study with clinical correlation and review of the literature. Virchows Archiv an Int J Pathol (2021) 478(3):487–96. doi: 10.1007/s00428-020-02926-1
47. Rapkiewicz AV, Mai X, Carsons SE, Pittaluga S, Kleiner DE, Berger JS, et al. Megakaryocytes and platelet-fibrin thrombi characterize multi-organ thrombosis at autopsy in COVID-19: A case series. EClinicalMedicine (2020) 24:100434. doi: 10.1016/j.eclinm.2020.100434
48. Roncati L, Ligabue G, Nasillo V, Lusenti B, Gennari W, Fabbiani L, et al. A proof of evidence supporting abnormal immunothrombosis in severe COVID-19: naked megakaryocyte nuclei increase in the bone marrow and lungs of critically ill patients. Platelets (2020) 31(8):1085–9. doi: 10.1080/09537104.2020.1810224
Zhu A, Real F, Capron C, Rosenberg AR, Silvin A, Dunsmore G, et al. Infection of lung megakaryocytes and platelets by SARS-CoV-2 anticipate fatal COVID-19. Cell Mol Life Sci CMLS (2022) 79(7):365. doi: 10.1007/s00018-022-04318-x
50. McMullen P, Smith H, Pytel P. Entrapped megakaryocytes in the microvasculature of brain tissues are not specific to COVID-19 but can be seen across a spectrum of acute lung injuries. J neuropathology Exp Neurol (2021) 80(11):1078–80. doi: 10.1093/jnen/nlab048
51. Martinez-Giron R, Martinez-Torre C, Martinez-Torre S. Megakaryocytes in bronchoalveolar fluid (BALF) samples. Acta bio-medica Atenei Parmensis (2022) 92(6):e2021300. doi: 10.23750/abm.v92i6.10077
52. Liu J, Luo X, Wang W, Zheng S, Lin H, Zeng J. Clinicopathological study of 9 cases of megakaryocytes in pleural and peritoneal fluids. Medicine (2018) 97(33):e11923. doi: 10.1097/MD.0000000000011923
53. Wang J, Xie J, Wang D, Han X, Chen M, Shi G, et al. CXCR4(high) megakaryocytes regulate host-defense immunity against bacterial pathogens. eLife (2022) 11:e78662. doi: 10.7554/eLife.78662
54. Warheit DB, Salley SO, Barnhart MI. Thrombin-stimulated effects on megakaryocytopoiesis and pulmonary-platelet interactions. Gen Physiol biophysics (1989) 8(6):611–31.
55. Sulkowski S, Terlikowski S, Sulkowska M. Occlusion of pulmonary vessels by megakaryocytes after treatment with tumour necrosis factor-alpha (TNF-alpha). J Comp Pathol (1999) 120(3):235–45. doi: 10.1053/jcpa.1998.0290
56. Zhou Y, Zhang B, Li C, Huang X, Cheng H, Bao X, et al. Megakaryocytes participate in the occurrence of bleomycin-induced pulmonary fibrosis. Cell Death Dis (2019) 10(9):648. doi: 10.1038/s41419-019-1903-8
57. Wu L, Guo Xu N, Wang Z, Xiong W, Hu Q, J, et al. Low arterial oxygen partial pressure induces pulmonary thrombocytopenia in patients and a mouse model. BMC pulmonary Med (2021) 21(1):3. doi: 10.1186/s12890-020-01381-7
58. Chabi S, Uzan B, Naguibneva I, Rucci J, Fahy L, Calvo J, et al. Hypoxia regulates lymphoid development of human hematopoietic progenitors. Cell Rep (2019) 29(8):2307–2320 e6. doi: 10.1126/science.1146304
59. Junt T, Schulze H, Chen Z, Massberg S, Goerge T, Krueger A, et al. Dynamic visualization of thrombopoiesis within bone marrow. Science (2007) 317(5845):1767–70. doi: 10.1126/science.1146304
60. Nishimura S, Nagasaki M, Kunishima S, Sawaguchi A, Sakata A, Sakaguchi H, et al. IL-1alpha induces thrombopoiesis through megakaryocyte rupture in response to acute platelet needs. J Cell Biol (2015) 209(3):453–66. doi: 10.1083/jcb.201410052
61. Zhang L, Orban M, Lorenz M, Barocke V, Braun D, Urtz N, et al. A novel role of sphingosine 1-phosphate receptor S1pr1 in mouse thrombopoiesis. J Exp Med (2012) 209(12):2165–81. doi: 10.1084/jem.20121090
62. Kowata S, Isogai S, Murai K, Ito S, Tohyama K, Ema M, et al. Platelet demand modulates the type of intravascular protrusion of megakaryocytes in bone marrow. Thromb haemostasis (2014) 112(4):743–56. doi: 10.1160/TH14-02-0123
63. Tavassoli M, Aoki M. Migration of entire megakaryocytes through the marrow–blood barrier. Br J haematology (1981) 48(1):25–9. doi: 10.1111/j.1365-2141.1981.00025.x
64. Brown E, Carlin LM, Nerlov C, Lo Celso C, Poole AW. Multiple membrane extrusion sites drive megakaryocyte migration into bone marrow blood vessels. Life Sci alliance (2018) 1(2):e201800061. doi: 10.26508/lsa.201800061
65. Niazi H, Zoghdani N, Couty L, Leuci A, Nitzsche A, Allende ML, et al. Murine platelet production is suppressed by S1P release in the hematopoietic niche, not facilitated by blood S1P sensing. Blood Adv (2019) 3(11):1702–13. doi: 10.1182/bloodadvances.2019031948
66. Bernardes JP, Mishra N, Tran F, Bahmer T, Best L, Blase JI, et al. Longitudinal multi-omics analyses identify responses of megakaryocytes, erythroid cells, and plasmablasts as hallmarks of severe COVID-19. Immunity (2020) 53(6):1296–1314 e9. doi: 10.1016/j.immuni.2020.11.017
67. Stephenson E, Reynolds G, Botting RA, Calero-Nieto FJ, Morgan MD, Tuong ZK, et al. Single-cell multi-omics analysis of the immune response in COVID-19. Nat Med (2021) 27(5):904–16. doi: 10.1038/s41591-021-01329-2
68. Wang X, Wen Y, Xie X, Liu Y, Tan X, Cai Q, et al. Dysregulated hematopoiesis in bone marrow marks severe COVID-19. Cell Discovery (2021) 7(1):60. doi: 10.1038/s41421-021-00296-9
69. Barrett TJ, Bilaloglu S, Cornwell M, Burgess HM, Virginio VW, Drenkova K., et al. Platelets contribute to disease severity in COVID-19. J Thromb haemostasis JTH (2021) 19(12):3139–53. doi: 10.1111/jth.15534
70. Aguilar A, Pertuy F, Eckly A, Strassel C, Collin D, Gachet C, et al. Importance of environmental stiffness for megakaryocyte differentiation and proplatelet formation. Blood (2016) 128(16):2022–32. doi: 10.1182/blood-2016-02-699959
71. Luff SA, Papoutsakis ET. Megakaryocytic maturation in response to shear flow is mediated by the activator protein 1 (AP-1) transcription factor via mitogen-activated protein kinase (MAPK) mechanotransduction. J Biol Chem (2016) 291(15):7831–43. doi: 10.1074/jbc.M115.707174
72. Ilkan Z, Wright JR, Goodall AH, Gibbins JM, Jones CI, Mahaut-Smith MP, et al. Evidence for shear-mediated Ca(2+) entry through mechanosensitive cation channels in human platelets and a megakaryocytic cell line. J Biol Chem (2017) 292(22):9204–17. doi: 10.1074/jbc.M116.766196
73. Dunois-Larde C, Capron C, Fichelson S, Bauer T, Cramer-Borde E, Baruch D, et al. Exposure of human megakaryocytes to high shear rates accelerates platelet production. Blood (2009) 114(9):1875–83. doi: 10.1182/blood-2009-03-209205
74. Blin A, Le Goff A, Magniez A, Poirault-Chassac S, Teste B, Sicot G., et al. Microfluidic model of the platelet-generating organ: beyond bone marrow biomimetics. Sci Rep (2016) 6:21700. doi: 10.1038/srep21700
75. Ito Y, Nakamura S, Sugimoto N, Shigemori T, Kato Y, Ohno M, et al. Turbulence activates platelet biogenesis to enable clinical scale ex vivo production. Cell (2018) 174(3):636–648 e18. doi: 10.1016/j.cell.2018.06.011
76. Poirault-Chassac S, Nivet-Antoine V, Houvert A, Kauskot A, Lauret E, Lai-Kuen R, et al. Mitochondrial dynamics and reactive oxygen species initiate thrombopoiesis from mature megakaryocytes. Blood Adv (2021) 5(6):1706–18. doi: 10.1182/bloodadvances.2020002847
77. Hansen CE, Qiu Y, McCarty OJT, Lam WA. Platelet mechanotransduction. Annu Rev Biomed Eng (2018) 20:253–75. doi: 10.1146/annurev-bioeng-062117-121215
78. Poirault-Chassac S, Nguyen KA, Pietrzyk A, Casari C, Veyradier A, Denis CV, et al. Terminal platelet production is regulated by von willebrand factor. PloS One (2013) 8(5):e63810. doi: 10.1371/journal.pone.0063810
79. Mojiri A, Alavi P, Lorenzana Carrillo MA, Nakhaei-Nejad M, Sergi CM, Thebaud B, et al. Endothelial cells of different organs exhibit heterogeneity in von willebrand factor expression in response to hypoxia. Atherosclerosis (2019) 282:1–10. doi: 10.1016/j.atherosclerosis.2019.01.002
80. Pan J, Dinh TT, Rajaraman A, Lee M, Scholz A, Czupalla CJ, et al. Patterns of expression of factor VIII and von willebrand factor by endothelial cell subsets in vivo. Blood (2016) 128(1):104–9. doi: 10.1182/blood-2015-12-684688
81. Ouzegdouh Y, Capron C, Bauer T, Puymirat E, Diehl JL, Martin JF, et al. The physical and cellular conditions of the human pulmonary circulation enable thrombopoiesis. Exp Hematol (2018) 63:22–27 e3. doi: 10.1016/j.exphem.2018.04.001
82. Mammoto T, Chen Z, Jiang A, Jiang E, Ingber DE, Mammoto A, et al. Acceleration of lung regeneration by platelet-rich plasma extract through the low-density lipoprotein receptor-related protein 5-Tie2 pathway. Am J Respir Cell Mol Biol (2016) 54(1):103–13. doi: 10.1165/rcmb.2015-0045OC
83. Tsukiji N, Inoue O, Morimoto M, Tatsumi N, Nagatomo H, Ueta K, et al. Platelets play an essential role in murine lung development through clec-2/podoplanin interaction. Blood (2018) 132(11):1167–79. doi: 10.1182/blood-2017-12-823369
84. Rafii S, Cao Z, Lis R, Siempos II, Chavez D, Shido K, et al. Platelet-derived SDF-1 primes the pulmonary capillary vascular niche to drive lung alveolar regeneration. Nat Cell Biol (2015) 17(2):123–36. doi: 10.1038/ncb3096
85. Semple JW, Italiano JE Jr., Freedman J. Platelets and the immune continuum. Nat Rev Immunol (2011) 11(4):264–74. doi: 10.1038/nri2956
86. Maouia A, Rebetz J, Kapur R, Semple JW. The immune nature of platelets revisited. Transfusion Med Rev (2020) 34(4):209–20. doi: 10.1016/j.tmrv.2020.09.005
87. Gawaz M, Vogel S. Platelets in tissue repair: control of apoptosis and interactions with regenerative cells. Blood (2013) 122(15):2550–4. doi: 10.1182/blood-2013-05-468694
88. Sandvad M, Pedersen EA, Frederiksen H, Mannering N. Risk of infection in adult patients with primary immune thrombocytopenia (ITP): a systematic review. Expert Rev Hematol (2021) 14(10):961–74. doi: 10.1080/17474086.2021.1976635
89. Norgaard M, Jensen AO, Engebjerg MC, Farkas DK, Thomsen RW, Cha S, et al. Long-term clinical outcomes of patients with primary chronic immune thrombocytopenia: a Danish population-based cohort study. Blood (2011) 117(13):3514–20. doi: 10.1182/blood-2010-10-312819
90. Ekstrand C, Linder M, Cherif H, Kieler H, Bahmanyar S. Increased susceptibility to infections before the diagnosis of immune thrombocytopenia. J Thromb haemostasis JTH (2016) 14(4):807–14. doi: 10.1111/jth.13267
91. Qu M, Liu Q, Zhao HG, Peng J, Ni H, Hou M, et al. Low platelet count as risk factor for infections in patients with primary immune thrombocytopenia: a retrospective evaluation. Ann Hematol (2018) 97(9):1701–6. doi: 10.1007/s00277-018-3367-9
92. Yao R, Liu X, He Y, Mei C, Shen Y, Zhan Q, et al. Low platelet count is a risk factor of postoperative pneumonia in patients with type a acute aortic dissection. J Thorac Dis (2020) 12(5):2333–42. doi: 10.21037/jtd.2020.03.84
93. Claushuis TA, van Vught LA, Scicluna BP, Wiewel MA, Klein Klouwenberg PM, Hoogendijk AJ, et al. Thrombocytopenia is associated with a dysregulated host response in critically ill sepsis patients. Blood (2016) 127(24):3062–72. doi: 10.1182/blood-2015-11-680744
94. Wang X, Ma Y, Wang S, Dong W, Lei X. Platelet is the early predictor of bronchopulmonary dysplasia in very premature infants: an observational cohort study. BMC pulmonary Med (2022) 22(1):109. doi: 10.1186/s12890-022-01895-2
95. Yan L, Ren Z, Wang J, Xia X, Yang L, Miao J, et al. The correlation between bronchopulmonary dysplasia and platelet metabolism in preterm infants. Front Pediatr (2021) 9:670469. doi: 10.3389/fped.2021.670469
96. Sun S, Jin C, Si J, Lei Y, Chen K, Cui Y, et al. Single-cell analysis of ploidy and the transcriptome reveals functional and spatial divergency in murine megakaryopoiesis. Blood (2021) 138(14):1211–24. doi: 10.1182/blood.2021010697
97. Liu C, Wu D, Xia M, Li M, Sun Z, Shen B, et al. Characterization of cellular heterogeneity and an immune subpopulation of human megakaryocytes. Advanced Sci (2021) 8(15):e2100921. doi: 10.1002/advs.202100921
98. Wang H, He J, Xu C, Chen X, Yang H, Shi S, et al. Decoding human megakaryocyte development. Cell Stem Cell (2021) 28(3):535–549 e8. doi: 10.1016/j.stem.2020.11.006
99. Slayton WB, Wainman DA, Li XM, Hu Z, Jotwani A, Cogle CR, et al. Developmental differences in megakaryocyte maturation are determined by the microenvironment. Stem Cells (2005) 23(9):1400–8. doi: 10.1634/stemcells.2004-0373
100. Cunin P, Nigrovic PA. Megakaryocytes as immune cells. J leukocyte Biol (2019) 105(6):1111–21. doi: 10.1002/JLB.MR0718-261RR
101. Garcia C, Au Duong J, Poette M, Ribes A, Payre B, Memier V, et al. Platelet activation and partial desensitization are associated with viral xenophagy in patients with severe COVID-19. Blood Adv (2022) 6(13):3884–98. doi: 10.1182/bloodadvances.2022007143
102. Zufferey A, Speck ER, Machlus KR, Aslam R, Guo L, McVey MJ, et al. Mature murine megakaryocytes present antigen-MHC class I molecules to T cells and transfer them to platelets. Blood Adv (2017) 1(20):1773–85. doi: 10.1182/bloodadvances.2017007021
103. Kang HK, Chiang MY, Ecklund D, Zhang L, Ramsey-Goldman R, Datta SK, et al. Megakaryocyte progenitors are the main APCs inducing Th17 response to lupus autoantigens and foreign antigens. J Immunol (2012) 188(12):5970–80. doi: 10.4049/jimmunol.1200452
104. Schrottmaier WC, Schmuckenschlager A, Pirabe A, Assinger A. Platelets in viral infections - brave soldiers or Trojan horses. Front Immunol (2022) 13:856713. doi: 10.3389/fimmu.2022.856713
105. Manne BK, Denorme F, Middleton EA, Portier I, Rowley JW, Stubben C, et al. Platelet gene expression and function in patients with COVID-19. Blood (2020) 136(11):1317–29. doi: 10.1182/blood.2020007214
106. Campbell RA, Schwertz H, Hottz ED, Rowley JW, Manne BK, Washington AV, et al. Human megakaryocytes possess intrinsic antiviral immunity through regulated induction of IFITM3. Blood (2019) 133(19):2013–26. doi: 10.1182/blood-2018-09-873984
107. Liu ZJ, Italiano J Jr, Ferrer-Marin F, Gutti R, Bailey M, Poterjoy B, et al. Developmental differences in megakaryocytopoiesis are associated with up-regulated TPO signaling through mTOR and elevated GATA-1 levels in neonatal megakaryocytes. Blood (2011) 117(15):4106–17. doi: 10.1182/blood-2010-07-293092
108. Mattia G, Vulcano F, Milazzo L, Barca A, Macioce G, Giampaolo A, et al. Different ploidy levels of megakaryocytes generated from peripheral or cord blood CD34+ cells are correlated with different levels of platelet release. Blood (2002) 99(3):888–97. doi: 10.1182/blood.V99.3.888
109. Davenport P, Liu ZJ, Sola-Visner M. Fetal vs adult megakaryopoiesis. Blood (2022) 139(22):3233–44. doi: 10.1182/blood.2020009301
110. Gomez Perdiguero E, Klapproth K, Schulz C, Busch K, Azzoni E, Crozet L, et al. Tissue-resident macrophages originate from yolk-sac-derived erythro-myeloid progenitors. Nature (2015) 518(7540):547–51. doi: 10.1038/nature13989
111. Hoeffel G, Chen J, Lavin Y, Low D, Almeida FF, See P, et al. C-myb(+) erythro-myeloid progenitor-derived fetal monocytes give rise to adult tissue-resident macrophages. Immunity (2015) 42(4):665–78. doi: 10.1016/j.immuni.2015.03.011
112. Li Z, Liu S, Xu J, Zhang X, Han D, Liu J, et al. Adult connective tissue-resident mast cells originate from late erythro-myeloid progenitors. Immunity (2018) 49(4):640–653 e5. doi: 10.1016/j.immuni.2018.09.023
113. Scandola C, Erhardt M, Rinckel JY, Proamer F, Gachet C, Eckly A, et al. Use of electron microscopy to study megakaryocytes. Platelets (2020) 31(5):589–98. doi: 10.1080/09537104.2019.1708885
114. McGrath KE. Utilization of imaging flow cytometry to define intermediates of megakaryopoiesis in vivo and in vitro. J Immunol Methods (2015) 423:45–51. doi: 10.1016/j.jim.2015.03.002
115. Bush LM, Healy CP, Marvin JE, Deans TL. High-throughput enrichment and isolation of megakaryocyte progenitor cells from the mouse bone marrow. Sci Rep (2021) 11(1):8268. doi: 10.1038/s41598-021-87681-2
116. Stegner D, Heinze KG. Intravital imaging of megakaryocytes. Platelets (2020) 31(5):599–609. doi: 10.1080/09537104.2020.1738366
117. Tinggaard Pedersen N, Cohn J. Intact megakaryocytes in the venous blood as a marker for thrombopoiesis. Scandinavian J haematology (1981) 27(1):57–63. doi: 10.1111/j.1600-0609.1981.tb00452.x
118. Pertuy F, Aguilar A, Strassel C, Eckly A, Freund JN, Duluc I, et al. Broader expression of the mouse platelet factor 4-cre transgene beyond the megakaryocyte lineage. J Thromb Haemost (2015) 13(1):115–25. doi: 10.1111/jth.12784
119. Gollomp K, Poncz M. Gp1ba-cre or Pf4-cre: pick your poison. Blood (2019) 133(4):287–8. doi: 10.1182/blood-2018-11-887513
120. Zhang J, Varas F, Stadtfeld M, Heck S, Faust N, Graf T, et al. CD41-YFP mice allow in vivo labeling of megakaryocytic cells and reveal a subset of platelets hyperreactive to thrombin stimulation. Exp Hematol (2007) 35(3):490–9. doi: 10.1016/j.exphem.2006.11.011
121. Nagy Z, Vogtle T, Geer MJ, Mori J, Heising S, Di Nunzio G, et al. The Gp1ba-cre transgenic mouse: a new model to delineate platelet and leukocyte functions. Blood (2019) 133(4):331–43. doi: 10.1182/blood-2018-09-877787
Keywords: lung megakaryocyte, platelet, emergency megakaryopoiesis, infection, inflammation
Citation: Gelon L, Fromont L and Lefrançais E (2022) Occurrence and role of lung megakaryocytes in infection and inflammation. Front. Immunol. 13:1029223. doi: 10.3389/fimmu.2022.1029223
Received: 26 August 2022; Accepted: 09 November 2022;
Published: 29 November 2022.
Edited by:
Fabrice Cognasse, U1059 SAnté INgéniérie BIOlogie (INSERM), FranceReviewed by:
Mortimer Poncz, Children’s Hospital of Philadelphia, United StatesKarin Hoffmeister, Versiti Blood Research Institute, United States
Copyright © 2022 Gelon, Fromont and Lefrançais. This is an open-access article distributed under the terms of the Creative Commons Attribution License (CC BY). The use, distribution or reproduction in other forums is permitted, provided the original author(s) and the copyright owner(s) are credited and that the original publication in this journal is cited, in accordance with accepted academic practice. No use, distribution or reproduction is permitted which does not comply with these terms.
*Correspondence: Emma Lefrançais, emma.lefrançais@ipbs.fr
†These authors have contributed equally to this work and share first authorship